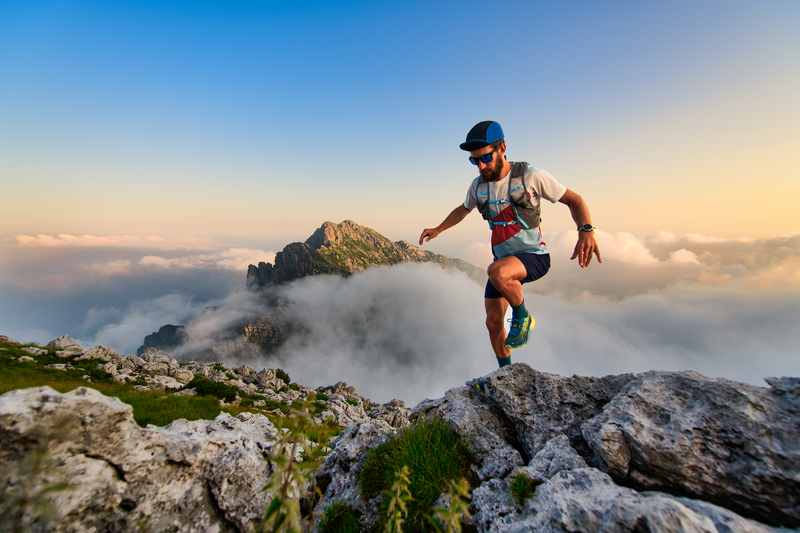
95% of researchers rate our articles as excellent or good
Learn more about the work of our research integrity team to safeguard the quality of each article we publish.
Find out more
ORIGINAL RESEARCH article
Front. Plant Sci. , 10 February 2021
Sec. Plant Symbiotic Interactions
Volume 12 - 2021 | https://doi.org/10.3389/fpls.2021.642576
This article is part of the Research Topic Early signaling in the Rhizobium-legume symbiosis View all 11 articles
One of the greatest inputs of available nitrogen into the biosphere occurs through the biological N2-fixation to ammonium as result of the symbiosis between rhizobia and leguminous plants. These interactions allow increased crop yields on nitrogen-poor soils. Exopolysaccharides (EPS) are key components for the establishment of an effective symbiosis between alfalfa and Ensifer meliloti, as bacteria that lack EPS are unable to infect the host plants. Rhizobium favelukesii LPU83 is an acid-tolerant rhizobia strain capable of nodulating alfalfa but inefficient to fix nitrogen. Aiming to identify the molecular determinants that allow R. favelukesii to infect plants, we studied its EPS biosynthesis. LPU83 produces an EPS I identical to the one present in E. meliloti, but the organization of the genes involved in its synthesis is different. The main gene cluster needed for the synthesis of EPS I in E. meliloti, is split into three different sections in R. favelukesii, which probably arose by a recent event of horizontal gene transfer. A R. favelukesii strain devoided of all the genes needed for the synthesis of EPS I is still able to infect and nodulate alfalfa, suggesting that attention should be directed to other molecules involved in the development of the symbiosis.
Alfalfa (Medicago sativa), is a widely cultivated legume used worldwide for feeding cattle mainly due to its high nutritional value, but also because its cultivation does not cause a high soil erosion (Li et al., 2011; Li and Brummer, 2012). This legume establishes a highly specific symbiosis with rhizobia, in which the model symbiont is Ensifer (Sinorhizobium) meliloti. This symbiosis allows the biological reduction of atmospheric N2 to plant-usable forms of nitrogen (Roy et al., 2020). The interaction between rhizobia and leguminous plants is a complex process, and needs the production of specific compounds from both organisms. A successful symbiosis requires a sophisticated exchange of signals between bacteria and plant (Oldroyd, 2013). This communication involves molecules of the plant, as flavonoids present in exudates or proteins such as lectins, and molecules produced by rhizobia: Nod factors or surface polysaccharides (Niehaus and Becker, 1998). The plant secretes a set of different flavonoids for which rhizobia have a specific receptor, the NodD protein (Peck et al., 2006). The recognition of flavonoids induces expression of Nod factors (NFs) in bacteria (Peters et al., 1986; Peters and Long, 1988), which are responsible for root hair curling and induction of cell division in the root cortex (Oldroyd et al., 2011). Bacteria proliferate within the infection thread and achieve invasion and colonization of the root interior (Jones et al., 2007).
Rhizobia can produce different types of surface polysaccharides relevant for the symbiosis establishment, such as exopolysaccharides (EPS), lipopolysaccharides (LPS), capsular polysaccharides (KPS), and cyclic β-glucans (CG) (Niehaus and Becker, 1998; Fraysse et al., 2003). The surface polysaccharides are required for a successful invasion (Fraysse et al., 2003; Kawaharada et al., 2015). Rhizobial mutants defective in the production of distinct polysaccharides show deficiencies in symbiosis establishment (Diebold and Noel, 1989; Hotter and Scott, 1991; Cheng and Walker, 1998; Fraysse et al., 2003; Hozbor et al., 2004). As examples, in E. meliloti 2011, a mutation of exoB (which encodes an UDP-glucose epimerase) leads to non-infected nodules on alfalfa (Buendia et al., 1991) and, also in E. meliloti 2011, mutants of exoY (encoding an undecaprenyl-phosphate galactose phosphotransferase) are not able to elongate infection threads (Reuber and Walker, 1993; Jones, 2012). Nevertheless, each symbiotic relationship has to be carefully evaluated, as it has been reported that a mutant of Sinorhizobium fredii HH103 that lacks EPS, shows an increase in the competitive capacity and forms N2-fixing nodules on Vigna unguiculata (Rodriguez-Navarro et al., 2014).
The relevance of each polysaccharide is specific for each rhizobia-leguminous plant interaction (Niehaus and Becker, 1998). Different strains of E. meliloti can produce more than one symbiotically active polysaccharide (Table 1). E. meliloti 1021 (henceforth, Eme1021) produces an exopolysaccharide called EPS I or succinoglycan, which is necessary for infection initiation and extension of the infection thread (Cheng and Walker, 1998). E. meliloti Rm41 (EmeRm41) produces succinoglycan and KPS active forms, also required for infection initiation (Pellock et al., 2000). An exoB mutant of EmeRm41 (EmeAK631) is defective in EPS I production but still produces KPS and shows a similar symbiotic phenotype as its parental strain, EmeRm41 (Pellock et al., 2000). A derivative of Eme1021 with a mutation in expR101, acquires the capacity to produce EPS II, which differs in composition to that of EPS I. This strain showed a less effective invasion compared to the strain producing succinoglycan, but still showed induction of effective nodules (Pellock et al., 2000). Although the different surface polysaccharides can partially restore the nodulation capacity of mutants defective in EPS production, efficiency in the different steps of the nodulation process vary depending on the structure of the polysaccharide produced. Succinoglycan consists of octasaccharide repeating units of one galactose and seven glucose residues, but the repeating units can be associated with additional acetyl, pyruvyl, and succinyl groups. Cheng and Walker (1998) described that the acetyl modification is not critical for the symbiotic function of succinoglycan in E. meliloti, but the lack of this modification reduces the efficiency of infection thread formation. At the same time, they visualized that a deficiency in succinylation is associated with the formation of aborted infection threads. Microarrays assays of Medicago truncatula showed that succinylation of EPS changes the transcriptomic response during infection, especially the lack of succinoglycan enhances the transcription of plant defense genes (Jones and Walker, 2008; Jones et al., 2008). EPS can be produced in a high-molecular-weight (HMW) and a low-molecular-weight (LMW), and succinylation of EPS is necessary for the cleavage that generates the LMW (York and Walker, 1998). A mutant in exoH of Eme1021, which produces EPS I of HMW without the succinyl modification, is not able to extend infection threads and cannot perform a fruitful symbiosis (Cheng and Walker, 1998). It was recently confirmed that the succinyl modification is the essential feature, rather than the production of EPS I of LMW (Mendis et al., 2016).
Table 1. Relevant characteristics of different E. meliloti strains with different polysaccharides [Data obtained from Pellock et al. (2000)].
Alfalfa can also be nodulated by other rhizobia, such as the acid tolerant Rhizobium favelukesii (Torres Tejerizo et al., 2016). This bacterium has the ability to nodulate different Medicago species, Phaseolus vulgaris and Leucaena leucocephala, but this interaction leads to inefficient nitrogen fixation nodules (Eardly et al., 1985; Wegener et al., 2001). The nodules developed in alfalfa by R. favelukesii are white, small and contain fewer bacteroids than those developed by E. meliloti (Wegener et al., 2001). Nevertheless, R. favelukesii is very competitive for the nodulation of alfalfa in acid soils (Segundo et al., 1999). During the symbiosis between M. sativa and R. favelukesii LPU83, it was shown that this bacterium does not require sulfated forms of the NFs (Torres Tejerizo et al., 2011). This is a remarkable difference in comparison with the symbiosis of alfalfa and E. meliloti, which absolutely needs sulfated NFs to nodulate (Schultze et al., 1995). Moreover, in alfalfa nodules developed by E. meliloti, only one bacteroid is found within the peribacteroidal membrane (Vasse et al., 1990), while in R. favelukesii it was shown that up to six bacteroids were found within a single peribacteroidal membrane, separated by matrix material (Wegener et al., 2001). Up to now, nodulation of alfalfa by R. favelukesii has shown differences during nodule development, nitrogen fixation and requirement of decorations on the NFs. In this work, we aimed to analyze another of the molecules necessary for infection: the EPS, which are involved in the development of the symbiosis between alfalfa and E. meliloti.
The strains and plasmids used in this work are listed in Supplementary Table 5. Escherichia coli was grown on Luria–Bertani (LB) (Miller, 1972) medium at 37°C. Rhizobium and Ensifer strains were grown on Tryptone–Yeast extract (TY) (Beringer, 1974),Yeast extract–Mannitol (YEM) (Vincent, 1970), or Vincent Minimal Medium (VMM) (Vincent, 1970) at 28°C. For solid media 15 g of agar per liter of medium were added. When needed, Congo Red was added at 0.25% (w/v) and Calcofluor was added at 0.02% (w/v). The final concentration of antibiotics used was (in μg ml–1): gentamicin (Gm) 10, kanamycin (Km) 25, and tetracycline (Tc) 10 for E. coli. For Rhizobium and Ensifer: streptomycin (Sm) 400, nalidixic acid (Nal) 20, neomycin (Nm) 60, rifampicin (Rif) 100, spectinomycin (Sp) 100, Gm 30, and Tc 5.
Ensifer meliloti and R. favelukesii were cultivated in YEM medium. Cultures were centrifuged 45 min at 10,000 × g. The EPS in the supernatant was precipitated with three volumes of ethanol overnight at −20°C and stored until processed. Then, the samples were centrifuged for 45 min at 10,000 × g and the pellets were resuspended in water. The quantification was performed by the anthrone method (Loewus, 1952). Amounts of EPS were normalized per mg of total proteins, which was determined by Bradford assay with Coomassie brilliant blue (Bradford, 1976).
Bacteria were grown in shaker flasks containing 200 ml of VMM at 30°C for 7 days. The precipitation and purification of EPS were done according to Müller et al. (1988). For monosaccharide analysis, the EPS was hydrolysed in 2 M trifluoroacetic acid for 2 h at 120°C in sealed glass vials. The hydrolysate was subsequently dried using a rotary evaporator. Remaining amounts of trifluoracetic acid were removed by the addition of isopropanol and dried again using the rotary evaporator. This treatment was carried out twice. Samples were dissolved in water and the sugars were analyzed by high-performance anion-exchange chromatography with pulsed amperometric detection on a Carbo Pac PA1 column (250 by 4 mm; Dionex, Sunnyvale, Calif.) and isocratic elution (1 ml/min) with 16 mM NaOH.
The EPS was analyzed by proton nuclear magnetic resonance (1H-NMR) spectroscopy. For 1H-NMR analysis, purified EPS was dissolved in deuterium oxide (99.7%), lyophilized, redissolved, and again lyophilized. Finally, 10 mg/ml of EPS was dissolved in deuterium oxide (99.99%), and sonicated for 5 min at room temperature. Spectra were recorded at 600 MHz and 80°C (Bruker Avance III–600).
Bacterial matings were performed as described by Simon et al. (1983). The visualization of plasmids in the transconjugants (plasmid profiles) was evaluated on Eckhardt-gels (Eckhardt, 1978) as modified by Hynes and McGregor (1990).
Total DNA and plasmid preparations, restriction-enzyme analysis, cloning procedures, and E. coli transformation were performed according to previously established techniques (Sambrook et al., 1989). PCR amplification was carried out with recombinant Taq DNA polymerase or Pfu DNA polymerase as specified by the manufacturers. Primers are listed in Supplementary Table 6.
The deletion of the exo cluster located in the plasmid, yielding strain LPU83 Δplasmid, was generated as follows. Firstly, a fragment of 414 bp of the exoV gene was amplified with Taq polymerase and primers exoV-BamHI-del-LEFT/exoV-XbaI-del-RIGHT and cloned into the commercial vector pCR 2.1-TOPO (Invitrogen), obtaining pTOPO-exoV (4365 bp). Plasmid pHP45 Sp allows the release of the Sp resistance gene as blunt SmaI fragment of 2066 bp. This SmaI fragment of pHP45 Sp was introduced into the EcoRV site of pTOPO-exoV, selecting the construction with Kmr Spr Ampr (pTOPO-exoV-Sp, 6431 bp). In parallel, a fragment of 382 bp of the exoP gene was amplified with taq polymerase and primers exoP-BamHI-del-LEFT/exoP-XbaI-del-RIGHT and cloned into the commercial vector pCR 2.1-TOPO, obtaining pTOPO-exoPp (4313 bp). Digestion of pTOPO-exoPp with EcoRI allows the release of the exoPp fragment (400 bp). The EcoRI fragment of pTOPO-exoPp was cloned into the EcoRI site of pK18mobSacB. The vector with the desired orientation was called pK18mobSacB-exoPp (6121 bp). This construction was digested with NheI and SalI, and ligated with the SpeI/XhoI product of TOPO-exoV-Sp (2576 bp), which contains both the exoV fragment and the Sp resistance gene, generating pK18mobSacB exoV-Sp-exoPp (8505 bp). To introduce the pK18mobSacB-exoV-Sp-exoPp into R. favelukesii LPU83, firstly pK18mobSacB-exoV-Sp-exoPp was transformed in E. coli S17-1 and then matings were carried out. Double recombinants were selected as Nms, and Spr. To corroborate the insertion, PCRs were carried out with primer Sm-Sp and L_exoV-out-LEFT (682 bp) and Sm-Sp and L_exoP-out-RIGHT (626 bp).
For the construction of strain LPU83 Δchromo, fragments of exoZ and exoP genes were amplified (185 and 210 bp, respectively), using Phusion polymerase (Thermo Scientific) and primers exoZ_Fw_cro83_Eco/exoZ_Rv_cro83_Sma and exoP_Fw_cro83_Bam/exoP_Rv_cro83_Hin, respectively. The fragments were cloned into the SmaI site of pK18mob. pK18mob-exoPc (4027 bp) was digested with BamHI and HindIII, and the released fragment (216 bp) was cloned into pK18mobSacB, obtaining pK18mobSacB-exoPc (5907 bp). Then, an EcoRI/SmaI fragment from pK18mob-exoZ (193 bp) containing the exoZ fragment was cloned into the EcoRI/SmaI sites of pK18mobSacB-exoPc, obtaining pK18mobSacB-exoZ-exoPc (6082 bp). To add the Tc resistance gene, the SmaI fragment of pHP45 Tc (2160 bp) containing the Tc resistance gene was cloned into the SmaI site of pK18mobSacB-exoZ-exoPc, selecting the Kmr Tcr vector, called pK18mobSacB-exoZ-Tc-exoPc. This vector was introduced by conjugation into R. favelukesii LPU83. Double recombinants were selected as Kms, and Tcr. To corroborate the insertion, PCRs were carried out with primer side_exoZ_out and Tc-out-Nter (774 bp) and side_exoP_out and Tc-out-Cter (983 bp). The result is a LPU83 derivative with a deletion on the chromosome exo cluster.
The LPU83 mutant in the exoB gene was carried out by a simple crossing over. For this, a fragment of 295 bp of the exoB gene was amplified using Phusion polymerase and primers exoB-Fw-int/exoB-Rv-int. The product was cloned into the SmaI site of pK18mob, obtaining pK18mob-exoB (4088 bp). This vector was introduced by conjugation into R. favelukesii LPU83. Simple recombinants mutants were selected as Nmr. To corroborate the insertion, PCRs were carried out with primer M13-Fw-40 and exoB-Rv-comp (1072 bp) and M13-Rv-40 and exoB-Fw-comp (726 bp). The result is a LPU83 derivative with an insertion on the exoB gene.
The vector for the exoB complementation assay was constructed as follows. A fragment containing the full exoB gene was amplified (1372 bp) with Phusion polymerase and primers exoB-Fw-comp/exoB-Rv-comp. The fragment was cloned into the SmaI site of the broad host range vector pBBR1MCS-5. The orientation of the gene was evaluated to make sure that the Lac promoter that is present in the vector would express the exoB gene. The resulting vector was called pBBR1MCS5-exoB (6140 bp).
For comparative genomics studies, the genes were searched for by means of BLASTp on the NCBI webpage. To examine genetic neighborhood, https://img.jgi.doe.gov/ was also used.
For the construction of the phylogenetic trees, the proteins were aligned with the module of Clustal implemented in MEGAX (Kumar et al., 2018). Prottest2.4 was used to determine the models of protein evolution (Abascal et al., 2005). The best model was LG+I+G for ExoB, LG+G+F for ExoH, LG+G for ExoV and LG+G for ExoY. Maximum likelihood (ML) trees were inferred under the selected model using PhyML v3.1 (Guindon and Gascuel, 2003). The robustness of the ML topologies was evaluated using a Shimodaira-Hasegawa-like test for branches implemented in PhyML v3.1 (values display in the nodes are multiplied by 100). We employed the best of NNIs and SPRs algorithms to search the tree topology and 100 random trees as initial trees. The accession numbers for the proteins selected for the phylogeny are display in each tree.
Medicago sativa (Alfalfa Sardi ten) seeds where surface-sterilized for 5 min in a 70% (v/v) ethanol solution, then washed with sterile distilled water. Subsequently, seeds were immersed in a solution of sodium hypochlorite (11 g l–1) for 15 min and washed six times with sterile distilled water. Seeds where placed on water agar (0.8% w/v) overnight. Afterward, seeds were placed on ethylene-oxide-sterilized plastic growth pouches containing 10 ml of Rolfe medium (Rolfe et al., 1980), with modifications (Barsch et al., 2006). Germination occurred in the pouches, and the roots developed through the hole in the paper. Seeds that did not germinate or did not grown through the hole were discarded. Three days post-germination, plants were inoculated with 106 colony-forming units of each strain used in this work (at least 25 plants per strain). Plants were cultured in a growth chamber at 22°C with a 16-h photoperiod, watered with modified Rolfe medium and water. The CFU that each inocula contained were estimated by plate counts. Plant assays were repeated at least twice.
Plants were harvested 4 weeks after inoculation. Nodules were counted, weighted and conserved at 4°C. The shoot dry matter weight was measured. For nodule occupancy, nodules were sterilized and treated as previously described (Torres Tejerizo et al., 2010; Montiel et al., 2016). Briefly, the nodules that previously were weighted and counted, were surface-sterilized with H2O2 (30 volume) for 10 min, washed with sterile distilled water and then crushed in 200 ul of sterile isotonic solution followed by plating serial dilutions on TY with the corresponding antibiotics. 2–3 days after, CFU were counted.
For microscopy, nodules were embedded in 6% (w/v) agarose. Nodule sections of 60 μm were obtained using a Leica VT1000 S Vibratome. Fresh sections were then stained with Live/Dead BacLight bacterial Viability Kit (Thermo Fisher). The staining solutions were then removed and nodule sections were resuspended in PBS buffer. Images were acquired with a Leica TCS SP5 confocal microscope.
During the symbiotic interaction between E. meliloti and alfalfa, different polysaccharides play relevant roles. Among them, EPS I (succinoglycan) is critical (Niehaus and Becker, 1998), but in some strains, the lack of EPS I may be overcome by EPS II (galactoglucan) or KPS (capsular polysaccharide, also referred as K antigen) (Pellock et al., 2000). As R. favelukesii LPU83 is able to infect alfalfa with some particular features (as mentioned in the introduction), the orthologs to the genes known to be involved in polysaccharides synthesis were searched for using BLAST. In addition to establishing a minimum of 35% identity as a cut-off to define orthologs (Rost, 1999), query coverage (>70%) and synteny were also considered in the analyses.
As mentioned in the Introduction, EmeAK631 only produces KPS (Putnoky et al., 1988; Putnoky et al., 1990; Reuhs et al., 1995) and, despite the lack of EPS I and EPS II, it is able to infect alfalfa (Putnoky et al., 1990; Pellock et al., 2000). Three clusters involved in KPS synthesis were described in EmeAK631 (Supplementary Figure 1): rkp-1, formed by rkpABCDEFGHIJ (Petrovics et al., 1993; Kiss et al., 1997), rkp-2 which harbors lpsL and rkpK (Kereszt et al., 1998) and rkp-3, constituted by rkpLMNOPQRSTZ (Kiss et al., 2001). Later on, rkpU was found to be located upstream of rkpA in EmeAK631 and in S. fredii HH103 (Parada et al., 2006; Hidalgo et al., 2010). Recently, the parental strain of EmeAK631, EmeRm41, was completely sequenced (Weidner et al., 2013). Surprisingly, in the annotation of the genome of EmeRm41 a very large rkpA gene was detected, which comprises the rkpABCDEF genes from EmeAK631 (Supplementary Figure 1). A similar gene-fusion was observed in Eme1021 and S. fredii HH103 (Parada et al., 2006). By means of BLASTp, orthologs for the genes involved in KPS synthesis were searched for in LPU83. rkpA was not found as a large ORF in LPU83. We compared the genes of both, EmeAK631 and Eme2011. Some orthologs were found, but with low identity at the amino acid sequence (ca. 25–45%) and low coverage (Supplementary Tables 1, 2). In addition, none of the genes found were distributed in clusters.
For the rkp-2 cluster, proteins with high similarity were found (for lpsL, LPU83_3231 and for rkpK, LPU83_3230). These genes showed ca. 70% identity and almost a full coverage in comparison to Eme2011 (Supplementary Figure 1). It is notable that in LPU83 lpsL is located downstream of rkpK, while in the E. meliloti strains the order of the genes is inverted, with rkpK situated downstream of lpsL (Supplementary Figure 1B).
For the genes that formed rkp-3 cluster, again, differences were observed between EmeAK631 and EmeRm41 (Supplementary Figure 1). Furthermore, it was observed that in Eme2011 the rkp-3 cluster was not complete (Supplementary Figure 1), lacking rkpLMNOPQ. A similar lack of genes is observed in Eme1021 (not shown). In LPU83, only some orthologs were found, and they are not organized in clusters (Supplementary Table 3). The structure of KPS has been described in EmeAK631 (and also in other rhizobia) as repetitive units of hexose linked with 3-deoxy-D-manno-2-octulosonic acid (Kdo) or related 1-carboxy-2-keto-3-deoxy sugars (Reuhs et al., 1993; Reuhs et al., 1998; Fraysse et al., 2003). The KPS of Eme1021 is composed solely by Kdo and a phospholipid anchor (Pellock et al., 2000). Eme1021 has been shown to produce KPS, but it is biologically inefficient (Fraysse et al., 2005) and cannot support the symbiosis with alfalfa (Pellock et al., 2000).
The lack of orthologs in Eme2011 (and in Eme1021) to some of the genes involved in the biosynthesis of KPS (Supplementary Figure 1) could be related to the particular inefficient KPS described by Fraysse et al. (2005). Likewise, as LPU83 lacks almost all the orthologs for KPS synthesis, the probabilities of producing an efficient KPS should be very low.
Ensifer meliloti is able to synthetize, under specific environmental conditions or certain mutations, a galactoglucan called EPS II, which was described as a non-Calcofluor-binding exopolysaccharide that reacts with anthrone (Glazebrook and Walker, 1989). EPS II is composed of glucose and galactose residues in a 1:1 ratio. The proteins needed for its production are encoded in the exp gene cluster located in the pSymB (organized in five transcriptional units, see Supplementary Figure 2) (Becker et al., 2002). It has been demonstrated that strains producing EPS II, but neither EPS I nor KPS, are able to infect alfalfa, reinforcing a relevant role in the symbiosis (Pellock et al., 2000). Despite this infection being less efficient than that of strains expressing EPS I alone, EPS II is able to restore the nodule development and nitrogen fixation that is lacking in EPS I mutants (Glazebrook and Walker, 1989). For the production of EPS II, the transcriptional regulator ExpR must be expressed to activate the transcription of the genes in the exp cluster. ExpR is a homolog of the LuxR family regulators (Pellock et al., 2002). ExpR homologs were searched for in LPU83. Three hits were found, LPU83_4100 (65% identity and 97% coverage), LPU83_pLPU83b_0215 (41% identity and 47% coverage), and LPU83_pLPU83d_1676 (46% identity and 14% coverage), suggesting that some of them could play a similar role as that of ExpR in E. meliloti. Thus, the orthologs to the 21 genes that form the exp cluster of Eme2011 were searched in LPU83 (Supplementary Table 4). The results showed no hits for six genes (expE8, expE5, expC, expA4, expA5, and expA6) but for the remaining genes, some hits were found mainly dispersed among the chromosome and plasmids of LPU83. In most of the cases with low identity scores (ca. 30–40% of identity) and without synteny, with exception of the genes LPU83_pLPU83d_1427, LPU83_pLPU83d_1428, and LPU83_pLPU83d_1429 that are similar to expD1, expD2, and expE1, respectively. Altogether, and as will be shown below, the results suggest that no galactoglucan should be produced by LPU83.
Together with the already mentioned ExpR master regulator, E. meliloti possesses another master regulator of EPS biosynthesis, namely MucR representing a homolog of the RosR protein from Agrobacterium tumefaciens (Keller et al., 1995). Mutation of mucR increases EPS II production and reduces EPS I synthesis (Becker et al., 2002). It was also shown that MucR increases nod factor production (Mueller and Gonzalez, 2011). We found a homolog to MucR from Eme1021 (P55323.1) in LPU83, namely LPU83_1234 (81% identity and 99% coverage), which is actually annotated as rosR and is located on the chromosome. This suggests that EPS biosynthesis and nod factor production might be regulated by LPU83_1234, the MucR/RosR homolog.
Succinoglycan, also namely EPS I, is an acidic Calcofluor-binding exopolysaccharide that has been extensively studied and demonstrated as a key molecule for the proper symbiosis of Eme2011 with alfalfa (Leigh et al., 1985; Leigh et al., 1987; Niehaus and Becker, 1998; Jones et al., 2007; Marczak et al., 2017). EPS I is composed of repeating octasaccharidic subunits with glucose and galactose in a 7:1 ratio and different substituents (succinyl, acetyl, and pyruvyl) (Becker and Pühler, 1998; Fraysse et al., 2003). The synthesis of the EPS I is carried out by several proteins encoded in the gene cluster (exoBZQFYXUVWTIHKLAMONP) located in the pSymB (Figure 1). Mutants in exoZ, exoX, exoI, exoK, exoO, and exoN have been described as able to infect alfalfa [reviewed by Niehaus and Becker (1998)]. The genes involved in EPS I synthesis were searched for in the LPU83 genome using E. meliloti as query (Figure 1). Orthologs to E. meliloti genes were found distributed among a cluster in the chromosome (exoZQFYXUWHKAMOP), the smallest of the plasmids present in LPU83, pLPU83a (exoVWTHKLAMO1O3P) and elsewhere, but not clustered, in the chromosome (exoB, exoI–LPU83_RS49150 Id 33% Qc 92% and exoN–LPU83_RS55945 Id 65% Qc 92%) (Figure 1). In this case, the scores were higher in comparison to similar genes involved in EPS II or KPS biosynthesis. Remarkably, some genes are duplicated between the plasmid and chromosome clusters. exoV and exoT are only present in pLPU83a, while exoZQFYXU are only present in the chromosome of LPU83. exoV encodes for a glycosyltransferase that is necessary for the addition of the pyruvyl substituent (Glucksmann et al., 1993; Reuber and Walker, 1993). exoT encodes for a transmembrane protein needed for the polymerization and/or export of the EPS I (Becker et al., 1993; Glucksmann et al., 1993; Reuber and Walker, 1993). Both genes were early recognized as indispensable for the production of EPS I and for the infection of alfalfa by E. meliloti (Becker et al., 1993). Thus, the exo gene cluster present in pLPU83a could be essential for a proper EPS I production.
Figure 1. Genetic organization of the EPS I and APS genes. The E. meliloti pSymB figure (middle) shows the organization of the cluster of genes involved in APS (left) and EPS I (right) synthesis. The upper box shows the distribution of orthologs to EPS I production genes, distributed among R. favelukesii LPU83 chromosome. The bottom box shows the distribution of orthologs to APS (left) and EPS I (right) production genes, distributed among plasmids pLPU83d and pLPU83a, respectively. Numbers at the beginning and at the end of each cluster indicate the position of each gene. Orthologs are marked and connected with similar colors. Percentages in bold stand for amino acidic identity of the proteins. Percentages below bold-numbers stand for query coverage. All the comparisons were made using E. meliloti 2011 exo genes as query. The striped lines within gray boxes indicate the deletions made in the exo cluster of the chromosome and the plasmid.
It was recently shown that Eme2011 is also able to produce a Congo Red-binding extracellular polysaccharide (Schäper et al., 2017). This polysaccharide is an arabinose-containing polysaccharide (APS), whose production is enhanced when cyclic di-GMP levels are elevated. Through an over-expression of pleD, encoding a diguanylate cyclase, and cuxR encoding a c-di-GMP responsive transcriptional activator of the APS operon, Schäper et al. (2019) were able to study the regulation of genes involved in the synthesis of the APS operon: the operon uxs1-uxe-apsS-apsH1-apsE-apsH2 (Figure 1). Strains that produce APS generate wrinkled red-colored macro-colonies on Congo Red containing medium (Schäper et al., 2019), but at this time there is no evidence of its role in symbiosis. A gene cluster of APS genes was found in pLPU83d, the largest plasmid of LPU83. Most of the APS-like genes showed identity values higher than 50%, with exception of apsH1 and apsH2 (Figure 1). Nevertheless, as it will be shown below, wrinkled macro-colonies were not observed in LPU83 nor in the mutants made in this work.
Cyclic ß-glucans in E. meliloti consist of 17–25 glucose residues linked by ß-1,2 bonds [CGs bonds may be different in other rhizobia (Breedveld and Miller, 1994)]. CGs synthesis in E. meliloti is directed by the translated products of the genes ndvA and ndvB, and mutants in any of these genes are impaired in the symbiosis with alfalfa (Dylan et al., 1986; Dickstein et al., 1988; Stanfield et al., 1988; Jones et al., 2007). Homologs to Eme1021 NdvA (CAC47862.1) and NdvB (P20471.2) are present in strain LPU83, namely LPU83_4101 (CDM59736.1, 76% identity and 94% coverage when compared with NdvA) and LPU83_4179 (CDM59813.1 and 69% identity and 99% coverage when compared with NdvB). Despite such sequence similarity, ndvA and ndvB are not syntenic in E. meliloti and R. favelukesii. While in Eme1021 the ndv genes are separated by 3 Kbp, in R. favelukesii LPU83 the same genes are separated by more than 70 Kbp. Currently available data demonstrate that ndvA and ndvB are strongly transcribed at low extracellular pH in R. favelukesii LPU83 (Nilsson et al., 2020), a result that suggests a possible role of the CGs during the response of the bacteria to an increased concentration of extracellular hydrogen ions. Nonetheless, further biochemical analyses are necessary to investigate whether there is or not CGs production in R. favelukesii LPU83.
Under artificial increments of cyclic di-GMP levels it has been shown that E. meliloti 8530 is able to produce a different polysaccharide named mixed-linkage ß-glucans (MLGs). MLGs are linear (1→3) (1→4)-ß-D-glucans that are relevant for adhesion and colonization of alfalfa roots but not for nodule development (Pérez-Mendoza et al., 2015). Rhizobial colonies that produce MLGs bind both Calcofluor and Congo Red. The synthesis of MLGs in E. meliloti 8530 is mediated by BgsA (GT-2 protein, CAC48777.1) and BgsB (a membrane fusion protein, CAC48776.1). Homologs to E. meliloti BgsA and BgsB were found in R. favelukesii LPU83 (i.e., LPU83_pLPU83c_0651, CDM61213.1, 80% identity and 99% coverage; and LPU83_pLPU83c_0650, CDM61212.1, 68% identity and 100% coverage; respectively). Despite the synteny between the bgs genes in E. meliloti and R. favelukesii, we do not have yet evidence of MLGs production in strain LPU83.
The data presented suggests that LPU83 has the potential to produce EPS I and, under specific conditions, as increments of cyclic di-GMP levels, APS as stated by Schäper et al. (2017, 2019) or MLG as suggested by Pérez-Mendoza et al. (2015). Morphological observations of LPU83 show mucoid macro-colonies which predict the production of polysaccharides (Torres Tejerizo et al., 2016). The particular genetic organization that we found for the EPS I gene cluster raises some questions: which is the evolutionary relationship of the genes present in the plasmids? Are both genetic clusters essential for EPS synthesis? Is the EPS produced by LPU83 similar to the one produced by E. meliloti? Finally, how relevant is the EPS of LPU83 for the infection of alfalfa?
The presence of two main clusters of exo genes in LPU83, one in the chromosome and another in plasmid pLPU83a point out that an event of horizontal gene transfer (HGT) could have occurred during the evolution of LPU83, where the strain acquired the exo genes from other bacteria. It is worth mentioning that previous work from our group has shown that pLPU83a is able to perform conjugative transfer (Torres Tejerizo et al., 2010), and the regulation of this phenomena depends on their genomic background and environmental conditions (Torres Tejerizo et al., 2014). Moreover, the plasmid gene cluster could be complementing the chromosomal genes to get a fully functional EPS I production.
To understand if such event of HGT occurred, phylogenetic analyses were made employing four genes. One only present in the chromosomal gene cluster (exoY), one present only in the plasmid gene cluster (exoV), one present in both clusters (exoH) and the bonafide exoB, that was present in the chromosome but not in the exo gene cluster. For the phylogenetic inference, the translated products of the genes were used. The ExoB phylogenetic tree showed that it was related to Rhizobium tibeticum CCBAU85039 and Rhizobium grahamii CCGE 502 (Figure 2), strains with which it shares a close chromosomal relationship (Torres Tejerizo et al., 2016). In a similar way, ExoY (also harbored in the chromosome) clusters with the same strains. Meanwhile, ExoV, which is present in pLPU83a, groups with E. meliloti and Ensifer medicae strains. No orthologs to ExoV were found in R. tibeticum CCBAU85039 and R. grahamii CCGE 502. Finally, the phylogenetic tree of ExoH, locates the chromosomal-encoded copy near to R. tibeticum CCBAU85039 and R. grahamii CCGE 502 while the plasmid-encoded copy is closer to E. meliloti and E. medicae. These results support the hypothesis that the plasmid exo cluster arose from an HGT event, probably from an Ensifer-related strain. It was previously shown that the exo cluster present in the plasmid is flanked by two inverted repeats, which encode a Tn3-like transposase and recombinase (Castellani et al., 2019). These structures could be a reminiscence of an insertion/excision of foreign DNA.
Figure 2. Phylogenetic analyses of EPS I genes of LPU83. Phylogenetic tree based on (A) ExoB (only in the chromosome of LPU83), (B) ExoH (in the chromosome of LPU83 and pLPU83a), (C) ExoY (only in the chromosome of LPU83), and (D) ExoV (only in the plasmid pLPU83a). Analyses were conducted by means of the Maximum Likelihood method. Support values (SH like × 100) greater than 50 are indicated at the nodes. Bars indicate substitution/site.
Evidence of HGT events toward new-symbiotic strains has been shown previously, although the efficiency of the resulting symbioses may be low. Sullivan et al. (1995) and Sullivan and Ronson (1998) showed the lateral transfer of a symbiotic island from Mesorhizobium loti to non-symbiotic mesorhizobia allowing the evolution of the latter into symbiotic rhizobia. A similar event was observed in soils of the Brazilian Cerrados (Batista et al., 2007), where evidence of HGT from inoculated Bradyrhizobium strains to soil bacteria was detected. Recently, it was shown that the symbiotic plasmid of Rhizobium etli CFN42, or at least fragments of it, can be transferred to bean-endophytic bacteria, generating new strains with the ability to nodulate and fix nitrogen (Bañuelos-Vazquez et al., 2020). In our group, we showed that the gene cluster involved in the synthesis of the nodulation factors (NFs) of LPU83 has a structure similar to that of E. meliloti, with whom it also shares a close phylogenetic relationship (Del Papa et al., 2007; Torres Tejerizo et al., 2011). Thus, not only genes involved in NFs synthesis could have been recruited by HGT, but also a gene cluster involved in EPS biosynthesis, during the evolution of LPU83.
Several determinants, including EPS, play essential roles during the complex molecular crosstalk before and during the interaction between rhizobia and plants (Jones et al., 2007). Rhizobial EPS varies among strains and species, harboring different substitutions (Skorupska et al., 2006). Due to the relevant role of EPS during the symbiosis between rhizobia and Medicago spp. and the ability of LPU83 to nodulate Medicago spp. and other legumes (Wegener et al., 2001), the structure of the EPS produced by LPU83 was analyzed. EPS was precipitated from culture supernatants. Monosaccharide analysis of the hydrolyzed EPS of LPU83 indicated that the strain produces an EPS that consists of glucose and galactose in a ratio of 7 to 1, similar to EPS I of Eme2011 (Figure 3). The EPS of LPU83 was further analyzed by 1H-NMR and gas chromatography/mass spectrometry (GC/MS) studies. A 1H-NMR spectrum of EPS I isolated from Eme2011 was recorded as a control (Figure 3B). This spectrum was in accordance with data already published for this EPS I (Leigh et al., 1987; Keller et al., 1995). The proton resonances between 3.8 and 5.4 ppm were assigned to glycosyl components arising from ring protons, and the singlet resonances at 2.1 and 2.8 ppm were assigned to methyl protons of the 1-carboxyethylidene (pyruvate) and acetyl groups, respectively. The characteristic triplets at 3.1 and 3.25 ppm represent the methylene protons of the succinyl groups. The EPS isolated from LPU83 gave rise to virtually the same proton NMR spectrum, indicating that the main polysaccharide secreted by this strain is identical to succinoglycan from Eme2011.
Figure 3. Composition and structural analyses of EPS. (A) Separation of monosaccharides obtained by acid hydrolysis of cetylpyridinium chloride (CPC) and ethanol precipitated culture supernatant of E. meliloti 2011 and R. favelukesii LPU83. The monosaccharides were separated by high-performance anion-exchange chromatography with pulsed amperometric detection on a Carbo Pac PA1 column. Only the relevant parts of the chromatograms are shown. (B) 1H-NMR spectra of isolated acidic exopolysaccharides (EPS) from R. favelukesii LPU83 and E. meliloti 2011. The singlets at 2.1 and 2.7 ppm represent the methyl protons from pyruvate and acetyl groups, respectively. The triplets at 3.1 and 3.25 ppm arise from the methylene protons of the succinyl group, while the complex region between 3.9 and 5.5 ppm represents signals from the ring protons of the carbohydrate constituents. (C) Structure of E. meliloti EPS I (succinoglycan). Diagram showing the octasaccharide repeating unit of succinoglycan (OAc, acyl group; Pyr, pyruvyl group; OSuc, succinyl group).
At first glance, it may have been proposed that the different substitution/modifications of the EPS could be related to the failure in the nodule development and differentiation observed in R. favelukesii (Eardly et al., 1985; Del Papa et al., 1999; Wegener et al., 2001). Our results demonstrate that despite the different organization of EPS I genes, whose localization is not restricted to one cluster and that the evolution of both clusters seems to integrate genes from different rhizobial linages, the EPS 1 produced is identical to that of E. meliloti. The question that remains is whether these clusters are essential for this EPS production and plant infection or not.
We showed that LPU83 produces a succinoglycan identical to the one produced by E. meliloti and that the genes involved in the synthesis are distributed between the chromosome of LPU83 and plasmid pLPU83a. This particular organization leads to the question whether both gene clusters are relevant for the production of EPS I. To determine this, a genetic approach was used. The chromosome cluster was deleted by double crossing-over and inserting a resistance to Tc, generating strain LPU83 Δchromo (the region is indicated in Figure 1), as indicated in section “Materials and Methods”. The cluster located in pLPU83a was deleted in a similar way, but a Sp resistance was used instead, generating strain LPU83 Δplasmid (also indicated in Figure 1). ExoB has been recognized as a UDP-glucose 4-epimerase which provides the UDP-galactose in E. meliloti (Buendia et al., 1991). Mutants in exoB of E. meliloti fail to produce proper EPS I and EPS II due to the lack of galactose (Putnoky et al., 1990; Buendia et al., 1991; Reuhs et al., 1995). Due to the relevance of exoB, a mutant was also made. In this case, by single recombination through the integration of pK18mob-exoB plasmid (LPU83-exoB–). Afterward, the mutations were combined to generate a double deletion mutant (LPU83 Δchromo Δplasmid) and a triple mutant (LPU83-exoB– Δchromo Δplasmid). Finally, LPU83-exoB– was complemented in trans with a full copy of exoB (pBBR1MCS5-exoB). Wild type Eme2011 and Eme2011 Δexo [which harbors a markerless deletion of the exo gene cluster from exoP to exoZ (Schäper et al., 2019)] were used as controls. EPS production was quantified with the anthrone method (Figure 4A) and EPS I was visualized due to its fluorescence in medium containing calcofluor (Figure 4B). The calcofluor assays demonstrated that only wild type strains (Eme2011 and LPU83) or the LPU83-exoB– complemented in trans were able to produce EPS I. In agreement with this result, anthrone assays also showed EPS production by the same strains. The levels of EPS of the exoB mutant complemented were slightly lower than the wild type (Figure 4A), but the calcofluor fluorescence phenotype was clearly observed (Figure 4B).
Figure 4. Characterization of EPS production of LPU83 mutants. (A) EPS quantification by the anthrone method. The EPS amount normalized per mg of total proteins is shown for each evaluated strain of E. meliloti and R. favelukesii. (B) Phenotypic analyses of the LPU83 mutants. EPS production was evaluated in YEM with Congo Red (first line) and in YEM with calcofluor under visible light and under UV light (lines below). The presence of EPS I is evidenced in the last condition due to its fluorescence. The statistical analysis was done by a t-test using three independent biological replicates. Representative pictures of at least three different experiments are shown.
Schäper et al. (2016, 2017) have shown that in Eme2011 EPS production is enhanced when cyclic di-GMP levels are elevated. Also, a new polysaccharide was described: an arabinose-containing polysaccharide (APS) (Schäper et al., 2019). The ectopical overexpression of pleD (diguanylate cyclase) and cuxR (c-di-GMP responsive transcriptional activator) leads to high di-GMP levels and, consequently, to an APS overproduction, generating wrinkled red-colored macro-colonies on medium containing Congo red (CR). Moreover, Pérez-Mendoza et al. (2015) showed that when cyclic di-GMP levels increase, MLGs can be produced. MLGs also bind Calcofluor and CR. The morphology of LPU83 and its mutants was evaluated in medium with CR, to determine binding of this dye. Neither LPU83 nor the mutants showed wrinkled macro-colonies nor red staining. Mutants of LPU83 did not show Calcofluor fluorescence (Figure 4). These results indicate that under our experimental conditions, APS and MLGs are not synthetized.
Exopolysaccharides production depends on many genes (Niehaus and Becker, 1998). In LPU83, the genes needed for the production of EPS I are distributed among different replicons. As shown above, both clusters and the exoB gene are needed for EPS production. In addition to the lack of orthologs of the genes needed for the synthesis of EPS II, the lack of glucose in the mutants suggest that galactoglucan is not produced in LPU83. The lack of CR staining suggests that also APS is not produced under our laboratory conditions. Altogether, LPU83 seems to be producing only EPS I, requiring both exo clusters and exoB.
As mentioned earlier, KPS and EPS II may replace, at least partially, the lack of EPS I in the effective nodulation of alfalfa by E. meliloti (Pellock et al., 2000). But when only EPS I is produced, mutants in its biosynthesis fail to nodulate (Cheng and Walker, 1998; Niehaus and Becker, 1998; Mendis et al., 2016). Also in Eme2011, mutants in the genes involved in the sulfation of NFs are not able to nodulate (Roche et al., 1991; Schultze et al., 1995). LPU83 has the particular capacity to nodulate alfalfa even in absence of sulfated NFs (Torres Tejerizo et al., 2011). This peculiarity encouraged us to evaluate the nodulation of alfalfa using all the constructed strains. As control, we included Eme2011 and Eme2011 Δexo strains. Eme2011 Δexo is a deletion mutant that lacks the exo gene cluster from exoP to exoZ, but retains the exoB gene. Eme2011 Δexo lacks the same genes as LPU83 Δchromo Δplasmid. Nodules induced by Eme2011 exhibited the typical cylindrical shape of alfalfa nodules with pink coloration. In contrast, Eme2011 Δexo showed small white round nodules (Figure 5). Some of the nodules induced by wild-type LPU83 showed the typical cylindrical shape, however they were mostly white instead of pink. Similar to the nodules observed for the Eme2011 exoB mutant (Schäper et al., 2019), the nodules induced by LPU83-exoB– and by LPU83-exoB– (pBBR1MCS5 empty vector) were tiny and round, while the complemented mutant, LPU83-exoB– (pBBR1MCS5-exoB), showed bigger nodules than LPU83-exoB–. The nodules induced by LPU83 Δplasmid were mainly round, but some of them were cylindrical. In contrast, the ones induced by LPU83 Δchromo were mainly cylindrical, but some were round. Unexpectedly, most of the nodules induced by the double and triple mutant were cylindrical. Nodules from all the derivatives of LPU83 were mostly white (Figure 5).
Figure 5. Analysis of the nodulation of LPU83 EPS I mutant strains. M. sativa plants infected by the indicated strains were harvested at 4 weeks post-inoculation. In the top panel, a representative plant was photographed. In second panel, zoom on the nodules of each plant was made. The number of nodules per plant were counted and weighted. Nodules were surfaced-sterilized, then crushed in sterile isotonic solution followed by plating on TY with the corresponding antibiotics and after 2 days CFU were counted. The shoot dry weight per plant was measured. Non-inoculated roots did not show nodules and the dry weight per plant was 3.98 ± 0.78b. Results were statistically analyzed by the ANOVA and least significant difference tests (Box et al., 1978). Values followed by different letters differed significantly with p < 0.05.
In the double and triple mutants, many nodules appeared with brown necrotic areas, while in the wild-type LPU83, these brown necrotic areas were observed in only a few nodules. These brown necrotic areas have been previously reported and related to plant defense symptoms, in nodules induced by EPS-deficient rhizobial mutants (Niehaus et al., 1993; Parniske et al., 1994; Fraysse et al., 2003; Berrabah et al., 2015). This led to the assumption that rhizobial EPS could act as suppressor of the plant defense response (Niehaus and Becker, 1998; Jones and Walker, 2008; Jones et al., 2008). This could cause the presence of the brown necrotic areas in nodules developed by LPU83 mutants, but it cannot explain their presence in the wild-type LPU83. One possibility is that the wild-type LPU83 triggers, at low levels, a plant defense mechanism. Studies with transcriptional fusions demonstrated that exo genes of Eme1021 were highly expressed in free-living cells but their expression decreased in later stages of symbiosis (Reuber et al., 1991). These observations were confirmed with recent high-throughput transcriptome studies (Capela et al., 2006; Roux et al., 2014). The expression of these genes might be fine-tuned by nodule signals, to allow a proper modulation of the plant defense. It cannot be disregarded that, despite the identical structure of EPS I of Eme2011 and LPU83, the spatial and temporal expression pattern of the exo genes could differ, causing a different response of the plant inside the nodules induced by LPU83.
Fresh nodules were counted, weighted and the number of CFU per nodule was evaluated (Figure 5). As expected, the comparison between Eme2011 and Eme2011 Δexo showed statistical significant differences in fresh nodule numbers and weight. Among the LPU83 derivatives, wild-type LPU83, double and triple mutants and LPU83 Δplasmid showed similar fresh weight, while the LPU83-exoB– showed lower fresh weight. LPU83-exoB– and LPU83-exoB– (pBBR1MCS5 empty vector) showed the highest numbers of nodules per plant, but harbored less bacteria inside. The complemented mutant, LPU83-exoB– (pBBR1MCS5-exoB), generates similar numbers of nodules as LPU83, but less than the LPU83-exoB– mutant. No CFU were detected in Eme2011 Δexo nodules, indicating the lack of a proper infection. The absence of bacteria in mutants in EPS I production of Eme2011 was previously shown (Cheng and Walker, 1998; Niehaus and Becker, 1998). Nodules occupied by LPU83 showed the highest number of CFU per nodule. Unexpectedly, bacteria could be recovered from the nodules induced by all LPU83 mutants. LPU83 Δplasmid and the double and triple mutants showed CFU per nodule values similar to those of Eme2011. The estimation of nitrogen-fixing ability was evaluated by comparisons of the shoot-dry weights. As expected, Eme2011 showed nitrogen fixation, while Eme2011 Δexo did not. LPU83 and its mutants showed a poor nitrogen fixation and the constructed mutations did not change the behavior of the wild-type strain (Figure 5).
As the recovery of bacteria from the nodules induced by all LPU83 mutants was unexpected, nodule histology was evaluated by confocal microscopy. Nodules were cut and stained with Live-Dead BacLight. As a control, Eme2011 showed the typical tissue organization, where zones delimitation and living bacteria stained by SYTO9 were clearly visible (green fluorescence) and distributed in the nodule after the meristem (zone I) (Figures 6A,G). Nodules infected with LPU83 showed a similar pattern, but red-stained bacteria (indicative of dead bacteria) were observed. Moreover, less plant cells seem to be occupied by LPU83 than by Eme2011 (Figures 6B,H). The triple mutant, LPU83 Δplasmid and LPU83 Δchromo showed a similar pattern to the one observed in LPU83, but more cells seem to be alive. Also, more plant cells appear to be occupied by the mentioned mutants (Figures 6D–F,J–L). The LPU83-exoB– strain showed smaller nodules, with an unclear definition of the zones and a large portion of the nodule was empty. Nevertheless, bacteria were observed inside these nodules but many of them were red-stained bacteria (Figures 6C,I). The size and shape of the nodules, the CFU per nodule obtained and the observed histology in nodules from LPU83-exoB–, show that the alteration in exoB severely impairs the nodulation of LPU83 but, contrary to E. meliloti exoB mutant (Schäper et al., 2019), LPU83-exoB– is still able to infect. Remarkably, LPU83-exoB– Δchromo Δplasmid, which lacks all exo genes, presents number of nodules, size, occupation and histology more similar to LPU83 than to the mutant LPU83-exoB–.
Figure 6. Morphology and occupancy of M. sativa nodules generated by EPS-I mutants of LPU83. Plants infected by E. meliloti 2011 and the indicated EPS I mutant strains of R. favelukesii LPU83 were harvested at 4 weeks post-inoculation. Nodule sections of 60 μm were obtained by means of a vibratome, and then stained with Syto 9 (green fluorescence that indicates living cells) and Propidium Iodide (red fluorescence that indicates cells with a damage in the membrane, i.e., dead). Light (A–F) and fluorescence (G–L) micrographs of full nodule slices were acquired with a confocal microscope. The photographs are representative of nodules of different plants experiments.
Overall, our results indicate that R. favelukesii LPU83 shows a lack of differentiation inside the nodules, which could explain the low nitrogen fixing rate. During the last years, nodule-specific cysteine-rich (NCRs) peptides have been intensively studied due to their role in the differentiation of the rhizobia inside the nodules (Van de Velde et al., 2010). Despite that NCRs show toxicity for the rhizobia in vitro, they are needed for the proper differentiation of the bacteria into bacteroids (Kondorosi et al., 2013; Pan and Wang, 2017). Furthermore, the membrane, the EPS and the LPS have been suggested as key components in the resistance to the NCRs (Montiel et al., 2017; Lima et al., 2020). It could be hypothesized that some other difference in the LPU83 cell envelope does not allow a correct perception of NCRs or that the expression of NCRs differs between the infection of E. meliloti and LPU83, resulting in non-proper symbiotic nodules in the case of infection by LPU83.
Legumes are of growing importance to reduce the global carbon dioxide footprint, since they do not need nitrogen fertilizers. The symbiosis between bacteria and leguminous plants has been therefore widely studied due its agronomical importance and also for providing the opportunity to understand the biochemical process involved in the communication between both partners (Jones et al., 2007). This communication includes several determinants from both organisms. One of them, the EPS, has been demonstrated as a key feature in different bacteria, with a role in recognition and signaling (Fraysse et al., 2003; Kawaharada et al., 2015). E. meliloti (especially the isogenic strains Eme1021 and Eme 2011) is considered as the model bacterium to study the symbiotic interaction between rhizobia and alfalfa. Nevertheless, R. favelukesii is a species that is also able to infect alfalfa, but its symbiosis is deficient. This failure seems to be related with the differentiation of the bacteria inside the nodules. Many determinants are involved in the differentiation, not only expressed in the late stages, but also during an early recognition. The synthesis of different EPS involves many genes, and their production could change according to different environmental conditions. The results presented here show that R. favelukesii produces an identical EPS I to the one produced by E. meliloti, whose synthesis is directed by genes located within a chromosomal cluster and a plasmid cluster. Both clusters have a different evolutionary origin and both of them are essential for the EPS I synthesis.
Remarkably, R. favelukesii does not need EPS I production to infect alfalfa. We observed that the mutants defective in EPS synthesis are able to generate nodules, with high titers of bacteria recovered from them. Noteworthy, a mutation affecting only exoB seems to be more severe than the lack of the whole exo genes. This could suggest that a punctual change in EPS I composition, as the lack of galactose, may be more harmful than the complete lack of it. In terms of specific determinants for the infection of alfalfa, R. favelukesii is a special species that differs from E. meliloti, since it is able to infect alfalfa, but with NFs that are somewhat different and with no EPS. Indeed, discerning the mechanism and determinants employed by R. favelukesii will lead us to a better understanding of the molecular pathway for a proper symbiosis and probably to alternative pathways or molecules for invasion that have yet to be described.
The original contributions presented in the study are included in the article/Supplementary Material, further inquiries can be directed to the corresponding author/s.
LC, ALu, JN, JPG, CW, MP, KN, and GTT did the experiments. AS, AP, ALa, SB, MP, KN, and GTT performed the data analysis and interpretation. LC, ALu, JN, and GTT wrote the manuscript. JPG, AS, AP, ALa, SB, MP, KN, and GTT manage the project and the fundings. All authors contributed to the discussion, provided comments on the manuscript, revised the manuscript, and have given approval to the final version of the manuscript.
This work was partially supported by grants PICT2016-0210 to GTT, PICT2017-2833 to MP, and PICT2016-0604 to JPG. LC, ALu, and JN are fellows of CONICET. JPG, ALa, MP, and GTT are members of the Research Career of CONICET. GTT has received a fellowship from the Alexander von Humboldt Foundation.
The authors declare that the research was conducted in the absence of any commercial or financial relationships that could be construed as a potential conflict of interest.
The authors are grateful to Claudio Mazo, Paula Giménez, and Silvana Tongiani (members of CPA CONICET at IBBM). We acknowledge the financial support of the German Research Foundation (DFG) and the Open Access Publication Fund of Bielefeld University for the article processing charge.
The Supplementary Material for this article can be found online at: https://www.frontiersin.org/articles/10.3389/fpls.2021.642576/full#supplementary-material
Abascal, F., Zardoya, R., and Posada, D. (2005). ProtTest: selection of best-fit models of protein evolution. Bioinformatics 21, 2104–2105. doi: 10.1093/bioinformatics/bti263
Bañuelos-Vazquez, L. A., Cazares, D., Rodríguez, S., Cervantes-De, La Luz, L., Sánchez-López, R., et al. (2020). Transfer of the symbiotic plasmid of Rhizobium etli CFN42 to endophytic bacteria inside nodules. Front. Microbiol. 11:1752. doi: 10.3389/fmicb.2020.01752
Barsch, A., Tellstrom, V., Patschkowski, T., Kuster, H., and Niehaus, K. (2006). Metabolite profiles of nodulated alfalfa plants indicate that distinct stages of nodule organogenesis are accompanied by global physiological adaptations. Mol. Plant Microbe Interact. 19, 998–1013. doi: 10.1094/MPMI-19-0998
Batista, J. S., Hungria, M., Barcellos, F. G., Ferreira, M. C., and Mendes, I. C. (2007). Variability in Bradyrhizobium japonicum and B. elkanii seven years after introduction of both the exotic microsymbiont and the soybean host in a cerrados soil. Microb. Ecol. 53, 270–284. doi: 10.1007/s00248-006-9149-2
Becker, A., Kleickmann, A., Kuster, H., Keller, M., Arnold, W., and Puhler, A. (1993). Analysis of the Rhizobium meliloti genes exoU, exoV, exoW, exoT, and exoI involved in exopolysaccharide biosynthesis and nodule invasion: exoU and exoW probably encode glucosyltransferases. Mol. Plant Microbe Interact. 6, 735–744. doi: 10.1094/mpmi-6-735
Becker, A., and Pühler, A. (1998). “Production of exopolysaccharides,” in The Rhizobiaceae: Molecular Biology of Model Plant-Associated Bacteria, eds H. P. Spaink, A. Kondorosi, and P. J. J. Hooykaas (Dordrecht: Springer), 97–118.
Becker, A., Ruberg, S., Baumgarth, B., Bertram-Drogatz, P. A., Quester, I., and Puhler, A. (2002). Regulation of succinoglycan and galactoglucan biosynthesis in Sinorhizobium meliloti. J. Mol. Microbiol. Biotechnol. 4, 187–190.
Beringer, J. E. (1974). R factor transfer in Rhizobium leguminosarum. J. Gen. Microbiol. 84, 188–198. doi: 10.1099/00221287-84-1-188
Berrabah, F., Ratet, P., and Gourion, B. (2015). Multiple steps control immunity during the intracellular accommodation of rhizobia. J. Exp. Bot. 66, 1977–1985. doi: 10.1093/jxb/eru545
Bradford, M. M. (1976). A rapid and sensitive method for the quantitation of microgram quantities of protein utilizing the principle of protein-dye binding. Anal. Biochem. 72, 248–254. doi: 10.1006/abio.1976.9999
Breedveld, M. W., and Miller, K. J. (1994). Cyclic beta-glucans of members of the family Rhizobiaceae. Microbiol. Rev. 58, 145–161.
Buendia, A. M., Enenkel, B., Koplin, R., Niehaus, K., Arnold, W., and Puhler, A. (1991). The Rhizobium meliloti exoZl exoB fragment of megaplasmid 2: ExoB functions as a UDP-glucose 4-epimerase and ExoZ shows homology to NodX of Rhizobium leguminosarum biovar viciae strain TOM. Mol. Microbiol. 5, 1519–1530. doi: 10.1111/j.1365-2958.1991.tb00799.x
Capela, D., Filipe, C., Bobik, C., Batut, J., and Bruand, C. (2006). Sinorhizobium meliloti differentiation during symbiosis with alfalfa: a transcriptomic dissection. Mol. Plant Microbe Interact. 19, 363–372. doi: 10.1094/MPMI-19-0363
Castellani, L. G., Nilsson, J. F., Wibberg, D., Schluter, A., Puhler, A., Brom, S., et al. (2019). Insight into the structure, function and conjugative transfer of pLPU83a, an accessory plasmid of Rhizobium favelukesii LPU83. Plasmid 103, 9–16. doi: 10.1016/j.plasmid.2019.03.004
Cheng, H. P., and Walker, G. C. (1998). Succinoglycan is required for initiation and elongation of infection threads during nodulation of alfalfa by Rhizobium meliloti. J. Bacteriol. 180, 5183–5191. doi: 10.1128/JB.180.19.5183-5191.1998
Del Papa, M. F., Balagué, L. J., Sowinski, S. C., Wegener, C., Segundo, E., Abarca, F. M., et al. (1999). Isolation and characterization of alfalfa-nodulating rhizobia present in acidic soils of central argentina and uruguay. Appl. Environ. Microbiol. 65, 1420–1427. doi: 10.1128/AEM.65.4.1420-1427.1999
Del Papa, M. F., Pistorio, M., Draghi, W. O., Lozano, M. J., Giusti, M. A., Medina, C., et al. (2007). Identification and characterization of a nodH ortholog from the alfalfa-nodulating Or191-like rhizobia. Mol. Plant Microbe Interact. 20, 138–145. doi: 10.1094/MPMI-20-2-0138
Dickstein, R., Bisseling, T., Reinhold, V. N., and Ausubel, F. M. (1988). Expression of nodule-specific genes in alfalfa root nodules blocked at an early stage of development. Genes Dev. 2, 677–687. doi: 10.1101/gad.2.6.677
Diebold, R., and Noel, K. D. (1989). Rhizobium leguminosarum exopolysaccharide mutants: biochemical and genetic analyses and symbiotic behavior on three hosts. J. Bacteriol. 171, 4821–4830. doi: 10.1128/jb.171.9.4821-4830.1989
Dylan, T., Ielpi, L., Stanfield, S., Kashyap, L., Douglas, C., Yanofsky, M., et al. (1986). Rhizobium meliloti genes required for nodule development are related to chromosomal virulence genes in Agrobacterium tumefaciens. Proc. Natl. Acad. Sci. U.S.A. 83, 4403–4407. doi: 10.1073/pnas.83.12.4403
Eardly, B. D., Hannaway, D. B., and Bottomley, P. J. (1985). Characterization of rhizobia from ineffective alfalfa nodules: ability to nodulate bean plants [Phaseolus vulgaris (L.) Savi.]. Appl. Environ. Microbiol. 50, 1422–1427. doi: 10.1128/AEM.50.6.1422-1427.1985
Eckhardt, T. (1978). A rapid method for the identification of plasmid desoxyribonucleic acid in bacteria. Plasmid 1, 584–588. doi: 10.1016/0147-619x(78)90016-1
Fraysse, N., Couderc, F., and Poinsot, V. (2003). Surface polysaccharide involvement in establishing the rhizobium-legume symbiosis. Eur. J. Biochem. 270, 1365–1380. doi: 10.1046/j.1432-1033.2003.03492.x
Fraysse, N., Lindner, B., Kaczynski, Z., Sharypova, L., Holst, O., Niehaus, K., et al. (2005). Sinorhizobium meliloti strain 1021 produces a low-molecular-mass capsular polysaccharide that is a homopolymer of 3-deoxy-D-manno-oct-2-ulosonic acid harboring a phospholipid anchor. Glycobiology 15, 101–108. doi: 10.1093/glycob/cwh142
Glazebrook, J., and Walker, G. C. (1989). A novel exopolysaccharide can function in place of the calcofluor-binding exopolysaccharide in nodulation of alfalfa by Rhizobium meliloti. Cell 56, 661–672.
Glucksmann, M. A., Reuber, T. L., and Walker, G. C. (1993). Genes needed for the modification, polymerization, export, and processing of succinoglycan by Rhizobium meliloti: a model for succinoglycan biosynthesis. J. Bacteriol. 175, 7045–7055. doi: 10.1128/jb.175.21.7045-7055.1993
Guindon, S., and Gascuel, O. (2003). A simple, fast, and accurate algorithm to estimate large phylogenies by maximum likelihood. Syst. Biol. 52, 696–704. doi: 10.1080/10635150390235520
Hidalgo, A., Margaret, I., Crespo-Rivas, J. C., Parada, M., Murdoch Pdel, S., Lopez, A., et al. (2010). The rkpU gene of Sinorhizobium fredii HH103 is required for bacterial K-antigen polysaccharide production and for efficient nodulation with soybean but not with cowpea. Microbiology 156, 3398–3411. doi: 10.1099/mic.0.042499-0
Hotter, G. S., and Scott, D. B. (1991). Exopolysaccharide mutants of Rhizobium loti are fully effective on a determinate nodulating host but are ineffective on an indeterminate nodulating host. J. Bacteriol. 173, 851–859. doi: 10.1128/jb.173.2.851-859.1991
Hozbor, D. F., Pich Otero, A. J., Lodeiro, A. R., Del Papa, M. F., Pistorio, M., and Lagares, A. (2004). The symbiotic defect in a Sinorhizobium meliloti lipopolysaccharide mutant can be overcome by expression of other surface polysaccharides. Res. Microbiol. 155, 855–860. doi: 10.1016/j.resmic.2004.06.012
Hynes, M. F., and McGregor, N. F. (1990). Two plasmids other than the nodulation plasmid are necessary for formation of nitrogen-fixing nodules by Rhizobium leguminosarum. Mol. Microbiol. 4, 567–574. doi: 10.1111/j.1365-2958.1990.tb00625.x
Jones, K. M. (2012). Increased production of the exopolysaccharide succinoglycan enhances Sinorhizobium meliloti 1021 symbiosis with the host plant Medicago truncatula. J. Bacteriol. 194, 4322–4331. doi: 10.1128/JB.00751-12
Jones, K. M., Kobayashi, H., Davies, B. W., Taga, M. E., and Walker, G. C. (2007). How rhizobial symbionts invade plants: the Sinorhizobium-Medicago model. Nat. Rev. Microbiol. 5, 619–633. doi: 10.1038/nrmicro1705
Jones, K. M., Sharopova, N., Lohar, D. P., Zhang, J. Q., Vandenbosch, K. A., and Walker, G. C. (2008). Differential response of the plant Medicago truncatula to its symbiont Sinorhizobium meliloti or an exopolysaccharide-deficient mutant. Proc. Natl. Acad. Sci. U.S.A. 105, 704–709. doi: 10.1073/pnas.0709338105
Jones, K. M., and Walker, G. C. (2008). Responses of the model legume Medicago truncatula to the rhizobial exopolysaccharide succinoglycan. Plant Signal Behav. 3, 888–890. doi: 10.4161/psb.3.10.6512
Kawaharada, Y., Kelly, S., Nielsen, M. W., Hjuler, C. T., Gysel, K., Muszynski, A., et al. (2015). Receptor-mediated exopolysaccharide perception controls bacterial infection. Nature 523, 308–312. doi: 10.1038/nature14611
Keller, M., Roxlau, A., Weng, W. M., Schmidt, M., Quandt, J., Niehaus, K., et al. (1995). Molecular analysis of the Rhizobium meliloti mucR gene regulating the biosynthesis of the exopolysaccharides succinoglycan and galactoglucan. Mol. Plant Microbe Interact. 8, 267–277. doi: 10.1094/mpmi-8-0267
Kereszt, A., Kiss, E., Reuhs, B. L., Carlson, R. W., Kondorosi, A., and Putnoky, P. (1998). Novel rkp gene clusters of Sinorhizobium meliloti involved in capsular polysaccharide production and invasion of the symbiotic nodule: the rkpK gene encodes a UDP-glucose dehydrogenase. J. Bacterio.l 180, 5426–5431. doi: 10.1128/JB.180.20.5426-5431.1998
Kiss, E., Kereszt, A., Barta, F., Stephens, S., Reuhs, B. L., Kondorosi, A., et al. (2001). The rkp-3 gene region of Sinorhizobium meliloti Rm41 contains strain-specific genes that determine K antigen structure. Mol. Plant Microbe Interact. 14, 1395–1403. doi: 10.1094/MPMI.2001.14.12.1395
Kiss, E., Reuhs, B. L., Kim, J. S., Kereszt, A., Petrovics, G., Putnoky, P., et al. (1997). The rkpGHI and -J genes are involved in capsular polysaccharide production by Rhizobium meliloti. J. Bacteriol. 179, 2132–2140. doi: 10.1128/jb.179.7.2132-2140.1997
Kondorosi, E., Mergaert, P., and Kereszt, A. (2013). A paradigm for endosymbiotic life: cell differentiation of Rhizobium bacteria provoked by host plant factors. Annu. Rev. Microbiol. 67, 611–628. doi: 10.1146/annurev-micro-092412-155630
Kumar, S., Stecher, G., Li, M., Knyaz, C., and Tamura, K. (2018). MEGA X: molecular evolutionary genetics analysis across computing platforms. Mol. Biol. Evol. 35, 1547–1549. doi: 10.1093/molbev/msy096
Leigh, J. A., Reed, J. W., Hanks, J. F., Hirsch, A. M., and Walker, G. C. (1987). Rhizobium meliloti mutants that fail to succinylate their calcofluor-binding exopolysaccharide are defective in nodule invasion. Cell 51, 579–587. doi: 10.1016/0092-8674(87)90127-9
Leigh, J. A., Signer, E. R., and Walker, G. C. (1985). Exopolysaccharide-deficient mutants of Rhizobium meliloti that form ineffective nodules. Proc. Natl. Acad. Sci. U.S.A. 82, 6231–6235. doi: 10.1073/pnas.82.18.6231
Li, X., and Brummer, E. C. (2012). Applied genetics and genomics in alfalfa breeding. Agronomy 2, 40–61. doi: 10.3390/agronomy2010040
Li, X., Wei, Y., Moore, K. J., Michaud, R., Viands, D. R., Hansen, J. L., et al. (2011). Association mapping of biomass yield and stem composition in a tetraploid alfalfa breeding population. Plant Genome 4, 24–35. doi: 10.3835/plantgenome2010.09.0022
Lima, R. M., Kylarova, S., Mergaert, P., and Kondorosi, E. (2020). Unexplored arsenals of legume peptides with potential for their applications in medicine and agriculture. Front. Microbiol. 11:1307. doi: 10.3389/fmicb.2020.01307
Loewus, F. A. (1952). Improvement in anthrone method for determination of carbohydrates. Anal. Chem. 24, 219–219. doi: 10.1021/ac60061a050
Marczak, M., Mazur, A., Koper, P., Zebracki, K., and Skorupska, A. (2017). Synthesis of rhizobial exopolysaccharides and their importance for symbiosis with legume plants. Genes 8, 360. doi: 10.3390/genes8120360
Mendis, H. C., Madzima, T. F., Queiroux, C., and Jones, K. M. (2016). Function of succinoglycan polysaccharide in Sinorhizobium meliloti host plant invasion depends on succinylation, not molecular weight. mBio 7:e00606-16. doi: 10.1128/mBio.00606-16
Miller, J. H. (1972). Experiments in Molecular Genetics. Cold Spring Harbor, NY: Cold Spring Harbor Laboratory.
Montiel, J., Downie, J. A., Farkas, A., Bihari, P., Herczeg, R., Bálint, B., et al. (2017). Morphotype of bacteroids in different legumes correlates with the number and type of symbiotic NCR peptides. Proc. Natl. Acad. Sci. U.S.A. 114, 5041–5046. doi: 10.1073/pnas.1704217114
Montiel, J., Szucs, A., Boboescu, I. Z., Gherman, V. D., Kondorosi, E., and Kereszt, A. (2016). Terminal bacteroid differentiation is associated with variable morphological changes in legume species belonging to the inverted repeat-lacking clade. Mol. Plant Microbe Interact. 29, 210–219. doi: 10.1094/MPMI-09-15-0213-R
Mueller, K., and Gonzalez, J. E. (2011). Complex regulation of symbiotic functions is coordinated by MucR and quorum sensing in Sinorhizobium meliloti. J. Bacteriol. 193, 485–496. doi: 10.1128/JB.01129-10
Müller, P., Hynes, M., Kapp, D., Niehaus, K., and Pühler, A. (1988). Two classes of Rhizobium meliloti infection mutants differ in exopolysaccharide production and in coinoculation properties with nodulation mutants. Mol. Gen. Genet. 211, 17–26. doi: 10.1007/bf00338388
Niehaus, K., and Becker, A. (1998). The role of microbial surface polysaccharides in the Rhizobium-legume interaction. Subcell Biochem. 29, 73–116. doi: 10.1007/978-1-4899-1707-2_3
Niehaus, K., Kapp, D., and Pühler, A. (1993). Plant defence and delayed infection of alfalfa pseudonodules induced by an exopolysaccharide (EPS I)-deficient Rhizobium meliloti mutant. Planta 190, 415–425. doi: 10.1007/BF00196971
Nilsson, J. F., Castellani, L. G., Draghi, W. O., Mogro, E. G., Wibberg, D., Winkler, A., et al. (2020). Global transcriptome analysis of Rhizobium favelukesii LPU83 in response to acid stress. FEMS Microbiol. Ecol. 97:fiaa235. doi: 10.1093/femsec/fiaa235
Oldroyd, G. E. (2013). Speak, friend, and enter: signalling systems that promote beneficial symbiotic associations in plants. Nat. Rev. Microbiol. 11, 252–263. doi: 10.1038/nrmicro2990
Oldroyd, G. E., Murray, J. D., Poole, P. S., and Downie, J. A. (2011). The rules of engagement in the legume-rhizobial symbiosis. Annu. Rev. Genet. 45, 119–144. doi: 10.1146/annurev-genet-110410-132549
Pan, H., and Wang, D. (2017). Nodule cysteine-rich peptides maintain a working balance during nitrogen-fixing symbiosis. Nat. Plants 3:17048. doi: 10.1038/nplants.2017.48
Parada, M., Vinardell, J. M., Ollero, F. J., Hidalgo, A., Gutierrez, R., Buendia-Claveria, A. M., et al. (2006). Sinorhizobium fredii HH103 mutants affected in capsular polysaccharide (KPS) are impaired for nodulation with soybean and Cajanus cajan. Mol. Plant Microbe Interact. 19, 43–52. doi: 10.1094/MPMI-19-0043
Parniske, M., Schmidt, P. E., Kosch, K., and Muller, P. (1994). Plant defense responses of host plants with determinate nodules induced by EPS-defective exoB mutants of Bradyrhizobium japonicum. Mol. Plant Microbe Interact. 7, 631–638. doi: 10.1094/MPMI-7-0631
Peck, M. C., Fisher, R. F., and Long, S. R. (2006). Diverse flavonoids stimulate NodD1 binding to nod gene promoters in Sinorhizobium meliloti. J. Bacteriol. 188, 5417–5427. doi: 10.1128/JB.00376-06
Pellock, B. J., Cheng, H. P., and Walker, G. C. (2000). Alfalfa root nodule invasion efficiency is dependent on Sinorhizobium meliloti polysaccharides. J. Bacteriol. 182, 4310–4318. doi: 10.1128/jb.182.15.4310-4318.2000
Pellock, B. J., Teplitski, M., Boinay, R. P., Bauer, W. D., and Walker, G. C. (2002). A LuxR homolog controls production of symbiotically active extracellular polysaccharide II by Sinorhizobium meliloti. J. Bacteriol. 184, 5067–5076. doi: 10.1128/jb.184.18.5067-5076.2002
Pérez-Mendoza, D., Rodriguez-Carvajal, M. A., Romero-Jimenez, L., Farias Gde, A., Lloret, J., Gallegos, M. T., et al. (2015). Novel mixed-linkage beta-glucan activated by c-di-GMP in Sinorhizobium meliloti. Proc. Natl. Acad. Sci. U.S.A. 112, E757–E765. doi: 10.1073/pnas.1421748112
Peters, N. K., Frost, J. W., and Long, S. R. (1986). A plant flavone, luteolin, induces expression of Rhizobium meliloti nodulation genes. Science 233, 977–980. doi: 10.1126/science.3738520
Peters, N. K., and Long, S. R. (1988). Alfalfa root exudates and compounds which promote or inhibit induction of Rhizobium meliloti nodulation genes. Plant Physiol. 88, 396–400. doi: 10.1104/pp.88.2.396
Petrovics, G., Putnoky, P., Reuhs, B., Kim, J., Thorp, T. A., Noel, K. D., et al. (1993). The presence of a novel type of surface polysaccharide in Rhizobium meliloti requires a new fatty acid synthase-like gene cluster involved in symbiotic nodule development. Mol. Microbiol. 8, 1083–1094. doi: 10.1111/j.1365-2958.1993.tb01653.x
Putnoky, P., Grosskopf, E., Ha, D. T., Kiss, G. B., and Kondorosi, A. (1988). Rhizobium fix genes mediate at least two communication steps in symbiotic nodule development. J. Cell Biol. 106, 597–607. doi: 10.1083/jcb.106.3.597
Putnoky, P., Petrovics, G., Kereszt, A., Grosskopf, E., Ha, D. T., Banfalvi, Z., et al. (1990). Rhizobium meliloti lipopolysaccharide and exopolysaccharide can have the same function in the plant-bacterium interaction. J. Bacteriol. 172, 5450–5458. doi: 10.1128/jb.172.9.5450-5458.1990
Reuber, T. L., Long, S., and Walker, G. C. (1991). Regulation of Rhizobium meliloti exo genes in free-living cells and in planta examined by using TnphoA fusions. J. Bacteriol. 173, 426–434. doi: 10.1128/jb.173.2.426-434.1991
Reuber, T. L., and Walker, G. C. (1993). Biosynthesis of succinoglycan, a symbiotically important exopolysaccharide of Rhizobium meliloti. Cell 74, 269–280. doi: 10.1016/0092-8674(93)90418-p
Reuhs, B. L., Carlson, R. W., and Kim, J. S. (1993). Rhizobium fredii and Rhizobium meliloti produce 3-deoxy-D-manno-2-octulosonic acid-containing polysaccharides that are structurally analogous to group II K antigens (capsular polysaccharides) found in Escherichia coli. J. Bacteriol. 175, 3570–3580. doi: 10.1128/jb.175.11.3570-3580.1993
Reuhs, B. L., Geller, D. P., Kim, J. S., Fox, J. E., Kolli, V. S., and Pueppke, S. G. (1998). Sinorhizobium fredii and Sinorhizobium meliloti produce structurally conserved lipopolysaccharides and strain-specific K antigens. Appl. Environ. Microbiol. 64, 4930–4938. doi: 10.1128/AEM.64.12.4930-4938.1998
Reuhs, B. L., Williams, M. N., Kim, J. S., Carlson, R. W., and Cote, F. (1995). Suppression of the Fix- phenotype of Rhizobium meliloti exoB mutants by lpsZ is correlated to a modified expression of the K polysaccharide. J. Bacteriol. 177, 4289–4296. doi: 10.1128/jb.177.15.4289-4296.1995
Roche, P., Debellé, F., Maillet, F., Lerouge, P., Faucher, C., Truchet, G., et al. (1991). Molecular basis of symbiotic host specificity in rhizobium meliloti: nodH and nodPQ genes encode the sulfation of lipo-oligosaccharide signals. Cell 67, 1131–1143. doi: 10.1016/0092-8674(91)90290-f
Rodriguez-Navarro, D. N., Rodriguez-Carvajal, M. A., Acosta-Jurado, S., Soto, M. J., Margaret, I., Crespo-Rivas, J. C., et al. (2014). Structure and biological roles of Sinorhizobium fredii HH103 exopolysaccharide. PLoS One 9:e115391. doi: 10.1371/journal.pone.0115391
Rolfe, B. G., Gresshoff, P. M., and Shine, J. (1980). Rapid screening for symbiotic mutants of Rhizobium and white clover. Plant Sci. Lett. 19, 277–284. doi: 10.1016/0304-4211(80)90082-6
Rost, B. (1999). Twilight zone of protein sequence alignments. Protein Eng. 12, 85–94. doi: 10.1093/protein/12.2.85
Roux, B., Rodde, N., Jardinaud, M. F., Timmers, T., Sauviac, L., Cottret, L., et al. (2014). An integrated analysis of plant and bacterial gene expression in symbiotic root nodules using laser-capture microdissection coupled to RNA sequencing. Plant J. 77, 817–837. doi: 10.1111/tpj.12442
Roy, S., Liu, W., Nandety, R. S., Crook, A., Mysore, K. S., Pislariu, C. I., et al. (2020). Celebrating 20 years of genetic discoveries in legume nodulation and symbiotic nitrogen fixation. Plant Cell 32, 15–41. doi: 10.1105/tpc.19.00279
Sambrook, J., Fritsch, E. F., and Maniatis, T. (1989). Molecular Cloning: A Laboratory Manual, 2nd Edn. Cold Spring Harbor, NY: Cold Spring Harbor Laboratory Press.
Schäper, S., Krol, E., Skotnicka, D., Kaever, V., Hilker, R., Søgaard-Andersen, L., et al. (2016). Cyclic Di-GMP regulates multiple cellular functions in the symbiotic alphaproteobacterium Sinorhizobium meliloti. J. Bacteriol. 198, 521–535. doi: 10.1128/jb.00795-15
Schäper, S., Steinchen, W., Krol, E., Altegoer, F., Skotnicka, D., Søgaard-Andersen, L., et al. (2017). AraC-like transcriptional activator CuxR binds c-di-GMP by a PilZ-like mechanism to regulate extracellular polysaccharide production. Proc. Natl. Acad. Sci. U.S.A. 114, E4822–E4831. doi: 10.1073/pnas.1702435114
Schäper, S., Wendt, H., Bamberger, J., Sieber, V., Schmid, J., and Becker, A. (2019). A bifunctional UDP-Sugar 4-Epimerase supports biosynthesis of multiple cell surface polysaccharides in Sinorhizobium meliloti. J. Bacteriol. 201::e00801-18. doi: 10.1128/JB.00801-18
Schultze, M., Staehelin, C., Röhrig, H., John, M., Schmidt, J., Kondorosi, E., et al. (1995). In vitro sulfotransferase activity of Rhizobium meliloti NodH protein: lipochitooligosaccharide nodulation signals are sulfated after synthesis of the core structure. Proc. Natl. Acad. Sci. U.S.A. 92, 2706–2709. doi: 10.1073/pnas.92.7.2706
Segundo, E., Martinez-Abarca, F., Dillewijn, P., Fernández-López, M., Lagares, A., Martinez-Drets, G., et al. (1999). Characterisation of symbiotically efficient alfalfa-nodulating rhizobia isolated from acid soils of Argentina and Uruguay. FEMS Microbiol. Ecol. 28, 169–176. doi: 10.1111/j.1574-6941.1999.tb00572.x
Simon, R., Priefer, U., and Pühler, A. (1983). A broad host range mobilization system for in vivo genetic engineering: transposon mutagenesis in gram negative bacteria. Bio/Technology 1, 784–791. doi: 10.1128/jb.177.1.52-58.1995
Skorupska, A., Janczarek, M., Marczak, M., Mazur, A., and Krol, J. (2006). Rhizobial exopolysaccharides: genetic control and symbiotic functions. Microb. Cell Fact. 5:7. doi: 10.1186/1475-2859-5-7
Stanfield, S. W., Ielpi, L., O’brochta, D., Helinski, D. R., and Ditta, G. S. (1988). The ndvA gene product of Rhizobium meliloti is required for beta-(1—-2)glucan production and has homology to the ATP-binding export protein HlyB. J. Bacteriol. 170, 3523–3530. doi: 10.1128/jb.170.8.3523-3530.1988
Sullivan, J. T., Patrick, H. N., Lowther, W. L., Scott, D. B., and Ronson, C. W. (1995). Nodulating strains of Rhizobium loti arise through chromosomal symbiotic gene transfer in the environment. Proc. Natl. Acad. Sci. U.S.A. 92, 8985–8989. doi: 10.1073/pnas.92.19.8985
Sullivan, J. T., and Ronson, C. W. (1998). Evolution of rhizobia by acquisition of a 500-kb symbiosis island that integrates into a phe-tRNA gene. Proc. Natl. Acad. Sci. U.S.A. 95, 5145–5149. doi: 10.1073/pnas.95.9.5145
Torres Tejerizo, G., Del Papa, M. F., Giusti, M. A., Draghi, W., Lozano, M., Lagares, A., et al. (2010). Characterization of extrachromosomal replicons present in the extended host range Rhizobium sp. LPU83. Plasmid 64, 177–185. doi: 10.1016/j.plasmid.2010.07.004
Torres Tejerizo, G., Del Papa, M. F., Soria-Diaz, M. E., Draghi, W., Lozano, M., Giusti, M. A., et al. (2011). The nodulation of alfalfa by the acid tolerant Rhizobium sp. strain LPU83 does not require sulfated forms of lipo-chitooligosaccharide nodulation signals. J. Bacteriol. 193, 30–39. doi: 10.1128/JB.01009-10
Torres Tejerizo, G., Pistorio, M., Althabegoiti, M. J., Cervantes, L., Wibberg, D., Schlüter, A., et al. (2014). Rhizobial plasmid pLPU83a is able to switch between different transfer machineries depending on its genomic background. FEMS Microbiol. Ecol. 88, 565–578. doi: 10.1111/1574-6941.12325
Torres Tejerizo, G., Rogel, M. A., Ormeño-Orrillo, E., Althabegoiti, M. J., Nilsson, J. F., Niehaus, K., et al. (2016). Rhizobium favelukesii sp. nov., isolated from the root nodules of alfalfa (Medicago sativa L). Int. J. Syst. Evol. Microbiol. 66, 4451–4457. doi: 10.1099/ijsem.0.001373
Van de Velde, W., Zehirov, G., Szatmari, A., Debreczeny, M., Ishihara, H., Kevei, Z., et al. (2010). Plant peptides govern terminal differentiation of bacteria in symbiosis. Science 327, 1122–1126. doi: 10.1126/science.1184057
Vasse, J., De Billy, F., Camut, S., and Truchet, G. (1990). Correlation between ultrastructural differentiation of bacteroids and nitrogen fixation in alfalfa nodules. J. Bacteriol. 172, 4295–4306. doi: 10.1128/jb.172.8.4295-4306.1990
Vincent, J. M. (1970). A Manual for the Practical Study of the Root-Nodule Bacteria.: IBP Handbook No. 15. Oxford: Blackwell Scientific.
Wegener, C., Schröder, S., Kapp, D., Pühler, A., Lopez, E. S., Martinez-Abarca, F., et al. (2001). Genetic uniformity and symbiotic properties of acid-tolerant alfalfa-nodulating rhizobia isolated from dispersed locations throughout Argentina. Symbiosis 30, 141–162.
Weidner, S., Baumgarth, B., Gottfert, M., Jaenicke, S., Puhler, A., Schneiker-Bekel, S., et al. (2013). Genome Sequence of Sinorhizobium meliloti Rm41. Genome Announc. 1:e00013-12. doi: 10.1128/genomeA.00013-12
Keywords: rhizobia, exopolysaccharide, alfalfa, symbiosis, nitrogen fixation
Citation: Castellani LG, Luchetti A, Nilsson JF, Pérez-Giménez J, Wegener C, Schlüter A, Pühler A, Lagares A, Brom S, Pistorio M, Niehaus K and Torres Tejerizo GA (2021) Exopolysaccharide Characterization of Rhizobium favelukesii LPU83 and Its Role in the Symbiosis With Alfalfa. Front. Plant Sci. 12:642576. doi: 10.3389/fpls.2021.642576
Received: 16 December 2020; Accepted: 20 January 2021;
Published: 10 February 2021.
Edited by:
Jose María Vinardell, University of Seville, SpainReviewed by:
Monika Janczarek, Maria Curie-Skłodowska University, PolandCopyright © 2021 Castellani, Luchetti, Nilsson, Pérez-Giménez, Wegener, Schlüter, Pühler, Lagares, Brom, Pistorio, Niehaus and Torres Tejerizo. This is an open-access article distributed under the terms of the Creative Commons Attribution License (CC BY). The use, distribution or reproduction in other forums is permitted, provided the original author(s) and the copyright owner(s) are credited and that the original publication in this journal is cited, in accordance with accepted academic practice. No use, distribution or reproduction is permitted which does not comply with these terms.
*Correspondence: Gonzalo A. Torres Tejerizo, Z2F0dEBiaW9sLnVubHAuZWR1LmFy
†These authors have contributed equally to this work and share first authorship
Disclaimer: All claims expressed in this article are solely those of the authors and do not necessarily represent those of their affiliated organizations, or those of the publisher, the editors and the reviewers. Any product that may be evaluated in this article or claim that may be made by its manufacturer is not guaranteed or endorsed by the publisher.
Research integrity at Frontiers
Learn more about the work of our research integrity team to safeguard the quality of each article we publish.