- 1Laboratory of Applied Mycology and Phenomics, Department of Plants and Crops, Faculty of Bioscience Engineering, Ghent University, Ghent, Belgium
- 2Centre of Excellence in Mycotoxicology and Public Health, Department of Bioanalysis, Faculty of Pharmaceutical Sciences, Ghent University, Ghent, Belgium
- 3Business Unit Biointeractions and Plant Health, Wageningen University and Research, Wageningen, Netherlands
- 4Laboratory of Microbiology, Department of Biochemistry and Microbiology, Faculty of Sciences, Ghent University, Ghent, Belgium
Fusarium head blight (FHB) in wheat (Triticum aestivum L.) is caused by a consortium of mutually interacting Fusarium species. In the field, the weakly pathogenic F. poae often thrives on the infection sites of the virulent F. graminearum. In this ecological context, we investigated the efficacy of chemical and biocontrol agents against F. graminearum in wheat ears. For this purpose, one fungicide comprising prothioconazole + spiroxamine and two bacterial biocontrol strains, Streptomyces rimosus LMG 19352 and Rhodococcus sp. R-43120 were tested for their efficacy to reduce FHB symptoms and mycotoxin (deoxynivalenol, DON) production by F. graminearum in presence or absence of F. poae. Results showed that the fungicide and both actinobacterial strains reduced FHB symptoms and concomitant DON levels in wheat ears inoculated with F. graminearum. Where Streptomyces rimosus appeared to have direct antagonistic effects, Rhodococcus and the fungicide mediated suppression of F. graminearum was linked to the archetypal salicylic acid and jasmonic acid defense pathways that involve the activation of LOX1, LOX2 and ICS. Remarkably, this chemical- and biocontrol efficacy was significantly reduced when F. poae was co-inoculated with F. graminearum. This reduced efficacy was linked to a suppression of the plant’s intrinsic defense system and increased levels of DON. In conclusion, our study shows that control strategies against the virulent F. graminearum in the disease complex causing FHB are hampered by the presence of the weakly pathogenic F. poae. This study provides generic insights in the complexity of control strategies against plant diseases caused by multiple pathogens.
Introduction
The need for resilient crops to cope with pests and diseases gains importance in a changing climate (Bebber et al., 2013). Driven by globalization and trade, pathogen pressure has increased due to emergent plant pathogens which threaten our agro-ecosystems (Corredor-Moreno and Saunders, 2020). In the context of global warming, the disease Fusarium head blight (FHB) is of particular interest as it is considered an increasing problem for cereal crops (Juroszek and von Tiedemann, 2013). The disease is highly dynamic and caused by a complex of different Fusarium species that can each respond differently to the changing climate (Yli-Mattila, 2010; Vaughan et al., 2016).
Within this species complex causing FHB, the hemi-biotrophic ascomycete fungus Fusarium graminearum is the predominant species worldwide. F. graminearum deploys a hemi-biotrophic strategy to infect plants and produces amongst others the trichothecene mycotoxin deoxynivalenol (DON) as a virulence factor during infection (Audenaert et al., 2014; Ameye et al., 2015). This molecule is the main virulence factor allowing F. graminearum to colonize the rachilla and rachis of the spikelets and ear, respectively by interacting with the primary N- and C-metabolism of the plant and with archetypal plant defense pathways, such as the oxidative burst and the salicylic acid (SA) and jasmonic acid (JA) directed signaling networks (Gardiner et al., 2009; Seifi et al., 2013; Audenaert et al., 2014; Ameye et al., 2015).
However, F. graminearum is only one member of the species complex causing FHB and the composition of the complex is geo-dependent. In addition, pathogens of secondary importance such as F. asiaticum and F. boothii emerge occasionally or gain in importance due to environmental or agronomic factors (Parry et al., 1995). In general, F. graminearum, F. culmorum, F. avenaceum, and F. poae are considered as the predominant species associated with FHB worldwide (Ioos et al., 2005; Xu et al., 2005). Despite the presence of these species in diseased kernels, only F. graminearum and F. culmorum are considered highly pathogenic, while the other species are less aggressive on wheat (Triticum aestivum L.). For a long time it was thought that they may infect, but do not proliferate, grow superficially and thrive primarily at the sites of inoculation (Stack and McMullen, 1985; Brennan et al., 2003). However, novel insights have shown that the multispecies interaction goes beyond a merely ‘one plus one is two’ theorem.
Recently, we showed that symptom development upon infection by two members of the FHB disease, F. graminearum and F. poae, is determined by timing of infection. Pre-inoculation of wheat ears with the weakly pathogenic F. poae prior to F. graminearum resulted in a disappearance of FHB symptoms and a reduction of mycotoxin levels compared to a single F. graminearum infection (Tan et al., 2020). In contrast, in these interactions, F. poae exhibited increased growth demonstrating that this weak pathogen takes advantage from its co-occurrence with F. graminearum. Moreover, F. poae was shown to asymptomatically induce SA and JA related defenses which hamper a later infection by F. graminearum (Ameye et al., 2015; Tan et al., 2020).
The multi-species nature of this disease also influences its control. From a crop protection point of view, the multispecies composition implies that different species of the FHB complex might react differently upon exposure to fungicides or biocontrol agents. In this regard, it has been demonstrated that especially weak pathogens such as F. langsethiae, F. avenaceum, and F. poae are more resistant to the ergosterol biosynthesis inhibiting azole fungicides than F. graminearum (Tini et al., 2020). Upon treatment in the field with azole fungicides, this results in an increased share of F. poae in the FHB population at the expense of F. graminearum (Audenaert et al., 2011). In view of these issues, authorities aspire to reduce the dependency, risk and use of fungicides and opt for alternative control measures.
Anticipating this paradigm shift, research has turned to the exploration of agricultural inoculants composed of bioactive bacterial isolates that exhibit significant biocontrol activities and new innovative strategies to apply them in the field. Actinobacteria have been described to exhibit a wide variety of potential biocontrol activities such as mycoparasitism, production of antibiotics and production of cell wall degrading enzymes (Palaniyandi et al., 2013). In addition, they combine these traits with a huge metabolic versatility, filamentous growth, spore formation capability and an omnipresence in the agro-ecological relevant niche of the soil (Newitt et al., 2019). Besides these direct interactions with the pathogen, evidence is accumulating that several Actinobacteria can also interact with the plant defense system. For example, it is known that several Actinobacteria can tailor their secondary metabolite production upon exposure to plant defense hormones (Van der Meij et al., 2018). Finally, they can prime the SA- and/or JA/ethylene dependent defense pathways in plants (Conn et al., 2008; Kurth et al., 2014; Vergnes et al., 2020). However, these defense-related pathways form an intricate signaling network with synergistic as well as antagonistic interactions, which adds another layer of complexity on the efficacy of biocontrol agents in plant disease management (Robert-Seilaniantz et al., 2011).
In the present paper, we investigated the impact of two promising Actinobacteria strains for their effectiveness to reduce FHB and concomitant mycotoxins in wheat. We unraveled their mode of action and showed that their capacity to reduce FHB symptoms caused by F. graminearum depends on the presence or absence of F. poae in the ear. In addition, this change in biocontrol capacity is linked to the archetypal SA- and JA- dependent plant defense pathways and the capacity to glucosylate DON in the ear.
Materials and Methods
Fusarium Strains: Growth and Production of Conidia
The Fusarium strains used in present study were the GFP transformants of F. graminearum PH-1 (Trail and Common, 2000) and F. poae 2516 (Vanheule et al., 2016) that have been previously introduced in the study by Tan et al. (2020). The GFP transformants of F. graminearum strain PH-1 or F. poae 2516 were grown on potato dextrose agar (PDA, Sigma-Aldrich) for 7 days at 21°C under a regime of 12 h of dark and 12 h of combined UVA and UVC light (2 × TUV 8W T5 and 1 × TL 8W BLB; Philips, Eindhoven, Netherlands). Conidia were harvested by adding a solution of sterile 0.01% (v/v) Tween 80 to the PDA plates and rubbing the mycelium with a Drigalski spatula. Four layers of sterile miracloth were used to filter the conidia and remove the mycelium. The suspension was adjusted to a final concentration of 1 × 106 conidia mL–1. This suspension was used for inoculations on both wheat leaves and ears.
Actinobacterial Strains
The actinobacterial strains comprising both Streptomyces and Rhodococcus species are listed in Table 1. The bacterial strains were grown from the –80°C glycerol stock for 3 days at 25°C in 5 mL Tryptic Soy Broth (TSB, Sigma-Aldrich) at 200 rpm. Subsequently, the bacteria were washed twice with PBS (Phosphate buffered saline) to be used in the biocontrol assays.
In vitro Antagonism
In an in vitro plate assay, the antagonism of S. rimosus LMG 19352 and Rhodococcus sp. R-43120 toward F. graminearum and F. poae was assessed. Bacterial suspensions and fungal conidia were obtained as previously described. Five μL of a conidial suspension at 1 × 106 mL–1 of either strains was inoculated in the center of a petri dish. Subsequently, four spots of 5 μL of the actinobacterial suspensions were applied in a square at 3 cm from the fungal inoculation spot. Control plates were inoculated with either of both fungi (F. graminearum or F. poae). Fungal diameter was assessed daily till 5 days after inoculation.
Plant Materials, Detached Leaf Assay and Whole Plant Ear Assay
The biocontrol capabilities of the actinobacterial strains were tested in a high throughput detached leaf assay. For this assay, spring wheat cultivar (cv) Tybalt was grown in pots (4.5-cm diameter × 6.5-cm height) in a growth chamber (21°C, 16 h: 8 h, light: dark) for 10 days. Leaf segments of 4 cm were cut from the tip of the oldest leaves of 10 days old seedlings. These leaves were placed on their abaxial surface in Petri dishes containing 0.5% (w/v) water agar amended with 40 mg L–1 benzimidazole. GFP tagged F. graminearum PH-1 was applied as a conidial suspension of 2.5 μL which was deposited in the center of the leaf segment which had been wounded by using a sterile inoculation needle prior to the inoculation (Ameye et al., 2015). Subsequently, a droplet of 2.5 μL of a bacterial suspension containing 1 × 108 CFUs per mL was applied on the same spot as the conidial suspension. The disease progress was assessed using a multispectral imaging approach (see below).
Based on the detached leaf assay, the two best performing biocontrol strains, i.e., Streptomyces rimosus LMG 19352 and Rhodococcus sp. R-43120 were used to assess their impact on two members of the FHB disease complex. These experiments were done using a wheat ear bioassay on intact plants previously optimized by Tan et al. (2020). For the wheat ear bioassay, about 10 spring wheat cv Tybalt plants were grown per pot (15-cm diameter × 30-cm height) under glasshouse conditions till Zadok’s Growth Scale (GS) 65 (Zadoks et al., 1974). In total 6 replicates were included per treatment and the experiment was repeated once in time. For each treatment, the 6 replicates always originated from different pots.
To assess the impact of Streptomyces rimosus strain LMG 19352, Rhodococcus sp. strain R-43120 and the fungicide cocktail prothioconazole + spiroxamine on the infection of F. graminearum and/or F. poae, wheat ears were infected with F. graminearum (g + w), F. poae (p + w) or co-inoculated with both pathogens (pg + w). The biocontrol treatments for F. poae comprised F. poae + LMG 19352 (p + b1), R-43120 (p + b2) and fungicide (p + f). Similar treatments with F. graminearum resulted in treatment labels (g + b1), (g + b2), and (g + f). Simultaneously infected F. poae and F. graminearum ears comprised (pg + w), (pg + b1), (pg + b2), and (pg + f). Control treatments to assess the phytotoxicity of the applied biocontrol strains or the fungicide consisted of (w + b1), (w + b2), and (w + f).
Disease Progress: Visual Assessment and Multispectral Phenomics
Visual scoring assessment of ears inoculation was performed at 7 days after inoculation (dai) by using a five class disease index, where Level 1 = no symptoms visible, Level 2 = one spikelet with symptoms, Level 3 = two spikelets with symptoms, and Level 4 = all of the spikelets from the inoculation site upwards have symptoms (Supplementary Figure S4).
To assess disease progression in detached leaves and in wheat ears, we employed a custom-build multispectral phenotyping platform. This platform allows the visualization of diverse physiological traits in real time, based on specific absorption, reflection and emission spectra at a high temporal and spatial resolution at 6 μm. A monochrome camera system including a filter wheel allowed pixel to pixel capturing of Red Green Blue (RGB) values, chlorophyll fluorescence (Chl) and GFP fluorescence (CropReporter, Phenovation, Wageningen, Netherlands). This camera system was mounted on a Cartesian coordinate robot that was housed in a temperature, light and humidity controlled environment. The chamber is equipped with sun LED modules (SLMs) (Phenovation, Wageningen, Netherlands).
Fungal proliferation was assessed based on symptom development using the RGB image. In addition, the effect of disease on the efficiency of photosystem II (FV/FM) was assessed (Baker, 2008). Finally, the GFP signal of F. graminearum or F. poae was used as a hallmark for fungal presence. GFP signals were corrected for auto-fluorescence of senescing leaves and ears resulting in corrected GFP values (cGFP).
Using this approach, images were taken at 3 dai in the screening assay on detached wheat leaves. As the disease progress and symptom development was slower in the ears, images of ears were taken at 4 and 7 dai.
RNA Extraction and Quantitative Reverse Transcription-PCR
Total RNA from ears was extracted using Trizol reagent (Sigma-Aldrich) according to the manufacturer’s instructions and subsequently quantified with a spectrophotometer (Quantus fluorometer, Promega). First strand cDNA was synthesized using the GoScriptTM Reverse Transcription System (Promega). By using GoTaq® qPCR Master Mix (Promega), quantitative reverse transcription (RT-qPCR) analysis was performed using a CFX96 system (Bio-Rad) with the following thermal settings: 95°C for 2 min; 40 cycles of 95°C for 15 s and 60°C for 1 min; melting curve analysis was performed using a temperature profile of heating to 95°C at the rate of 0.5°C per 1 s. Primers used for all genes are listed in Supplementary Table S1. Normalization of defense genes was performed by using the cell division control protein gene (Ta54227) in wheat as reference. Fungal biomass was quantified using a pre-mRNA slicing factor of F. graminearum (FGSG_01244) and F. poae (FPOA_01282) as described before (Ameye et al., 2015). Gene expression analysis was performed using qBase + software (Biogazelle) based on the fold change which was calculated by dividing the CNRQ values (calibrated normalized relative quantities) of the treated samples by control samples.
Quantification of Type A and Type B Trichothecenes in Wheat Ears
To investigate whether co-inoculation of F. poae and F. graminearum on ears impacts the mycotoxin production, ten different mycotoxins produced by either F. poae or F. graminearum were screened using LC-MS/MS based on (De Boevre et al., 2012).
Briefly, individual mycotoxin solid standards (1 mg) of DON, nivalenol (NIV), 3-acetyl deoxynivalenol (3-ADON), 15-acetyl deoxynivalenol (15-ADON), neosolaniol (NEO), fusarenon-X (FUS-X), T-2 toxin (T-2), HT-2 toxin (HT-2), diacetoxyscirpenol (DAS) and deepoxydeoxynivalenol (DOM) were supplied by Coring System Diagnostics (Gernsheim, Germany) as certified solutions. All mycotoxin solid standards were dissolved in acetonitrile (1 mg mL–1), and were storable for a minimum of 1 year at –18°C (Spanjer et al., 2008). Working solutions of 10 ng μL–1 for DON, NIV, 3-ADON, 15-ADON, NEO, FUS-X, T-2, HT-2, DAS and DOM were prepared in methanol and stored at –18°C. From the individual working solutions, a mixture was prepared in methanol, stored at –18°C and renewed monthly with the following concentrations: the mycotoxin mix (mycotoxins, 10 ng μL–1) and the internal standard (DOM, 10 ng μL–1). For the plant tissue, 10 mg of the leaf or 1 g of the ear were crushed using liquid nitrogen. Subsequently, 0.8 mL and 8 mL of the extraction solvent [ethyl acetate/formic acid (99/1, v/v)] were added to each leaf and ear sample, respectively. The mixture was vortexed for 60 s and shaken for 1 h. The samples were centrifuged at 3000 rpm for 15 min, an aliquot of 200 μL from the supernatant was transferred into a glass tube and evaporated under a gentle stream of nitrogen at 40°C till complete dryness. To re-dissolve the residue, 180 μL from the injection solvent (mixture of mobile phase A and B, 60/40, v/v) were added. The tubes were vortexed for 60 s, sonicated for 10 min and the contents were filtered using ultracentrifugation tubes at 10,000 rpm for 5 min. The re-dissolved sample was transferred together with 20 μL of internal standard (DOM, 10 ng μL–1) into injection vials, and subjected to LC-MS/MS analysis.
LC-MS/MS analysis was performed using a Waters Acquity UPLC-Quattro Premier XE MS (Waters, Milford, MA, United States) in positive electrospray ionization mode (ESI+) mode. Chromatographic separation was achieved using a Symmetry C18 (150 mm × 2.1 mm, i.d. 5 μm) column with a guard column (10 mm × 2.1 mm i.d., Waters, Zellik, Belgium). The mobile phases consisted of water/methanol/acetic acid (94/5/1, v/v/v) and 5 mM ammonium acetate (mobile phase A), and methanol/water/acetic acid (97/2/1, v/v/v) and 5 mM ammonium acetate (mobile phase B), and were used at a flow rate of 0.3 mL min–1 with a gradient elution program. This gradient program was as follow; 0–7 min, 95–35% A; 7–11 min, 35–25% A; 11–13 min, 25–0% A; 13–14 min, 0% A; 14–16 min, 0–40% A; 16–26 min, 40–60% A; 26–28 min, 60–95% A. The column was reconditioned for 5 min before the next injection, with a total analytical run time of 28 min. Data acquisition and processing was facilitated using MassLynx and QuanLynx version® 4.1 software (Micromass, Manchester, United Kingdom). To achieve the optimal selectivity of the MS conditions, data acquisition was performed by applying selected reaction monitoring (SRM). For each target analyte, one precursor and two product ion transitions were selected. The capillary voltage was 3.2 kV and nitrogen was used as the desolvation gas. Source and desolvation temperatures were set at 150 and 350°C, respectively.
Statistical Analysis
For statistical evaluation and plot generation, the R software version 3.6.0 (RCoreTeam, 2019) and the packages ggplot2 (Wickham, 2016) and agricolae (de Mendiburu, 2014) were used. For multiple comparisons, normality and homoscedasticity assumptions were verified using diagnostic plots. Treatments were statistically compared using ANOVA analyses (with a WELCH correction when homoscedasticity was not met) followed by a post hoc Tukey test. All analyses were run at a significance level of P = 0.05.
Results
In planta Screening for Efficient Actinobacterial Strains Against Fusarium
Using an in house developed detached leaf assay (Ameye et al., 2015), the in planta biocontrol capacity of several actinobacterial strains comprising both Rhodococcus sp. and Streptomyces sp. was assessed against the main causal FHB pathogen F. graminearum. The strains were first identified using MALDI T-MS (data not shown). Results of the detached leaf assay are shown in Figure 1. A wide variety of interactions was observed ranging from a complete blocking of infection to enhanced disease development. Symptom development (Fv/Fm, chlorophyll fluorescence) was completely blocked for Rhodococcus sp. R-43120 and Streptomyces rimosus LMG 19352 (Figures 1A,C). In addition, the GFP signal was absent and resembled the mock inoculated control treatment (Figures 1B,D). With some bacterial strains, a more severe infection was observed than in the uninfected control treatment. This was the case for R. fascians D188, R. erythropolis BD2, R. globerolus DSM 43954, R. rodnii LMG 5362, S. humiferus B5, S. cacaoi subsp. asoensis K234, S. turgudicabies 90, and S. rimosus K189. Some of the actinobacterial strains resulted in an increased cGFP signal of F. graminearum (R. fascians SR13; S. cacaoi subsp. asoensis K234; S. rimosus K189). This points to a proliferated growth of F. graminearum due to the presence of these actinobacterial strains.
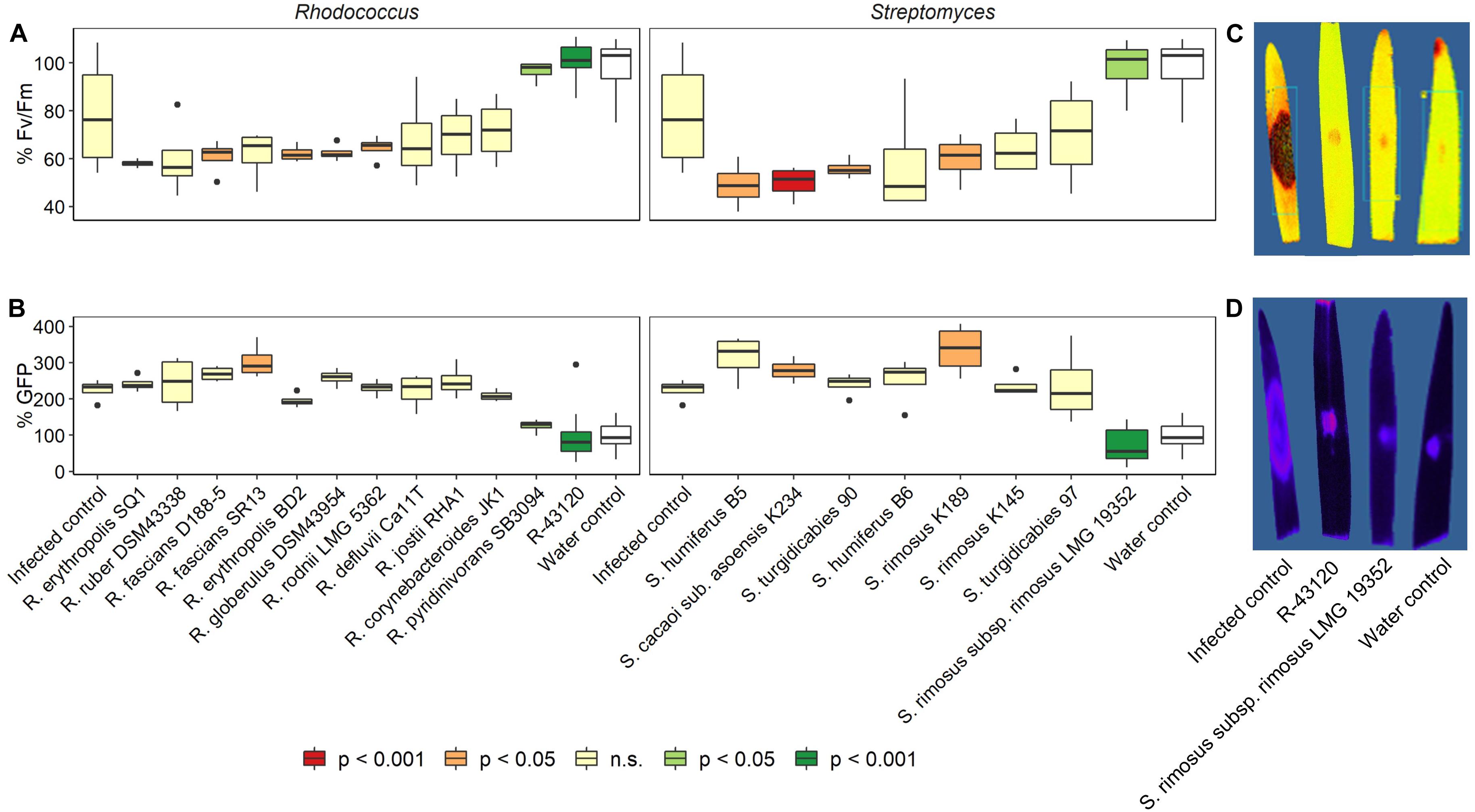
Figure 1. Biocontrol capacity of Streptomyces and Rhodococcus strains against F. graminearum as the main causal agent of FHB. Strains were co-applied with GFP tagged F. graminearum PH-1 in a detached leaf assay. (A) % Fv/Fm (percentage increase/decrease in efficiency of photosystem II) compared to mock-inoculated control plants; values represent symptom development based on the efficiency of photosystem II. Strains with significant positive biocontrol capacities are indicated in green. (B) Assessment of the relative active fungal biomass based on the GFP signal of the mock infected control plants. (C) Picture of R-43120 and LMG 19352 treatment based on the efficiency of photosystem II. (D) Picture of R-43120 and LMG 19352 treatment based on the GFP signal. Significant differences (positive or negative, based on the P-values) are indicated by the colors of the boxes. Boxplots indicate the median (horizontal lines), 25th and 75th percentile range (boxes) and up to 1.5 × IQR (Interquartile Range) (whiskers).
Based on these results, two actinobacterial strains Streptomyces rimosus LMG 19352 and Rhodococcus sp. R-43120 were further used in this paper to investigate their modes of action, their interaction with the two main members of the FHB complex F. graminearum and F. poae in vitro and in planta.
In vitro Antagonism of Streptomyces rimosus LMG 19352 (b1) and Rhodococcus sp. R-43120 (b2)
The direct antagonistic effect of Streptomyces rimosus strain LMG 19352 (b1) and Rhodococcus sp. strain R-43120 (b2) was assessed against F. poae 2516 and F. graminearum PH-1 in co-cultivation assays on TSA medium. These analyses revealed that biocontrol strain b1 displayed a very strong antagonistic activity against F. graminearum PH-1 (g + b1) as well as against F. poae 2516 (p + b1) (Figure 2) and against a combined inoculum of F. poae and F. graminearum (pg + b1) (Supplementary Figure S1). The antagonistic effects were visible from day 2 onward and were identical against both F. poae and F. graminearum. For biocontrol strain b2, there was no effect on the growth of F. poae (p + b2) or F. graminearum (g + b2) (Figure 2). Similar results were obtained in the co-inoculations of F. poae and F. graminearum (Supplementary Figure S1). The in vitro inhibition of F. poae and F. graminearum by b1 was statistically evaluated by comparing the slope of the growth curves in the linear region (day 2–4 after inoculation). In the presence of the b1 antagonist, fungal growth of Fusarium poae, Fusarium graminearum and combined F. poae and F. graminearum were significantly reduced, resulting in significant lower slopes of the linear regression curves (ANOVA, all p-values < 0.001). Strain b2 showed no significant effect compared to the water control (p = 0.980, p = 0.218, and p = 0.184 resp.).
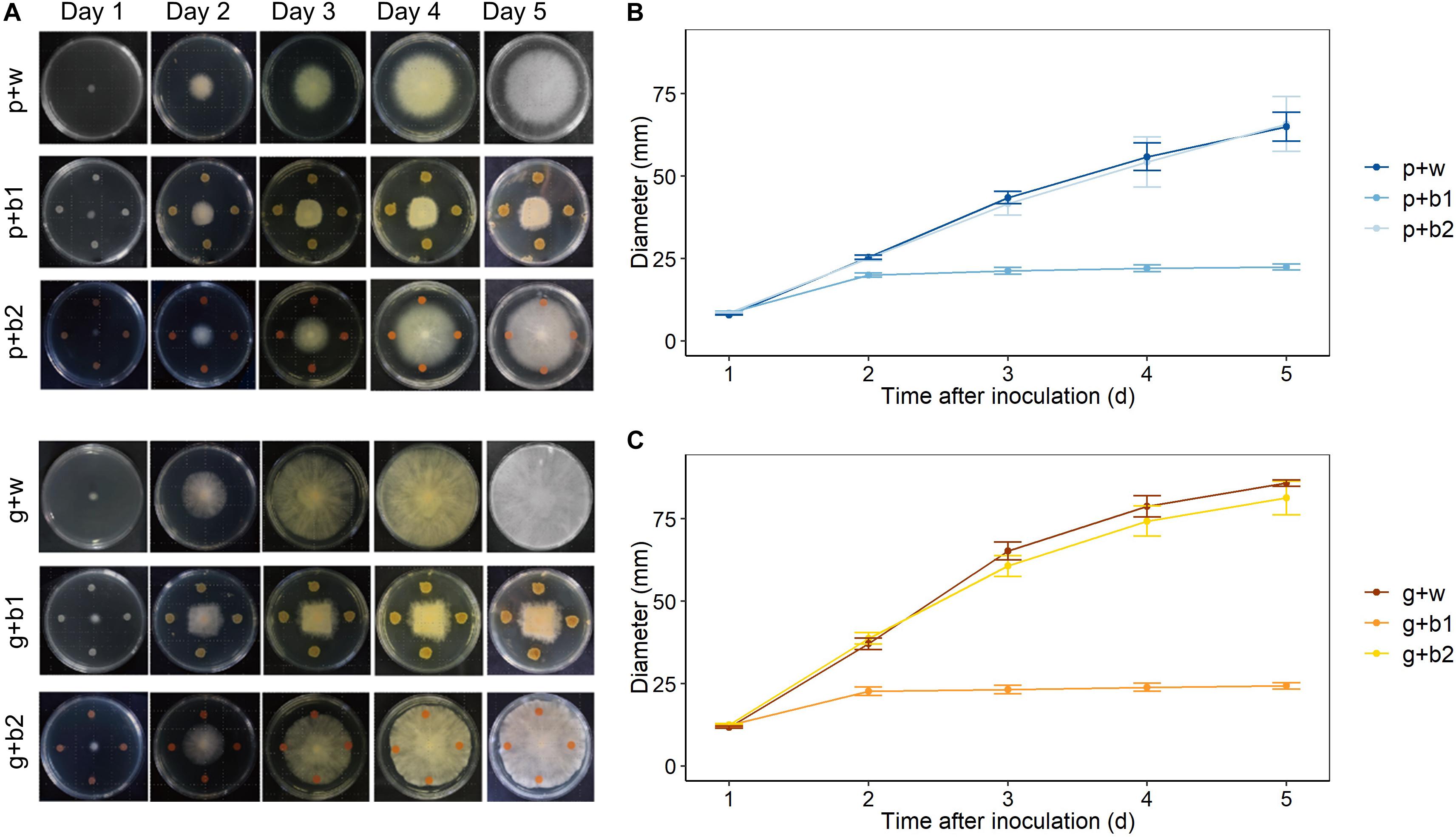
Figure 2. Effect of Streptomyces rimosus strain LMG 19352 (b1) and Rhodococcus sp. R-43120 (b2) on the growth of F. graminearum PH-1 and F. poae 2516 on TSA plates. Treatments: p + w (Inoculation with F. poae and water); p + b1 (Inoculation with F. poae and LMG 19352); p + b2 (Inoculation with F. poae and R-43120); g + w (Inoculation with F. graminearum and water); g + b1 (Inoculation with F. graminearum and LMG 19352); g + b2 (Inoculation with F. graminearum and R-43120). (A) Images taken from day 1 till day 5. (B) F. poae growth from day 1 till day 5, n = 5 (five biological reps per time point). (C) F. graminearum growth from day 1 till day 5, n = 5 (five biological reps per time point).
Impact of Streptomyces rimosus LMG 19352 (b1) and Rhodococcus sp. R-43120 (b2) on FHB on Wheat Ears Infected With F. graminearum, F. poae or a Combined Infection of F. graminearum and F. poae
We assessed the impact of biocontrol strains b1 and b2 when applied with F. graminearum (g + b1; g + b2), F. poae (p + b1; p + b2), or a combination of both FHB members (pg + b1; pg + b2). The fungicide prothioconazole + spiroxamine (g + f; p + f; pg + f) was included as a control treatment. The disease was assessed by multispectral analysis of the dark-adapted Fv/Fm chlorophyll fluorescence or by the GFP signal. Results are shown in Figure 3.
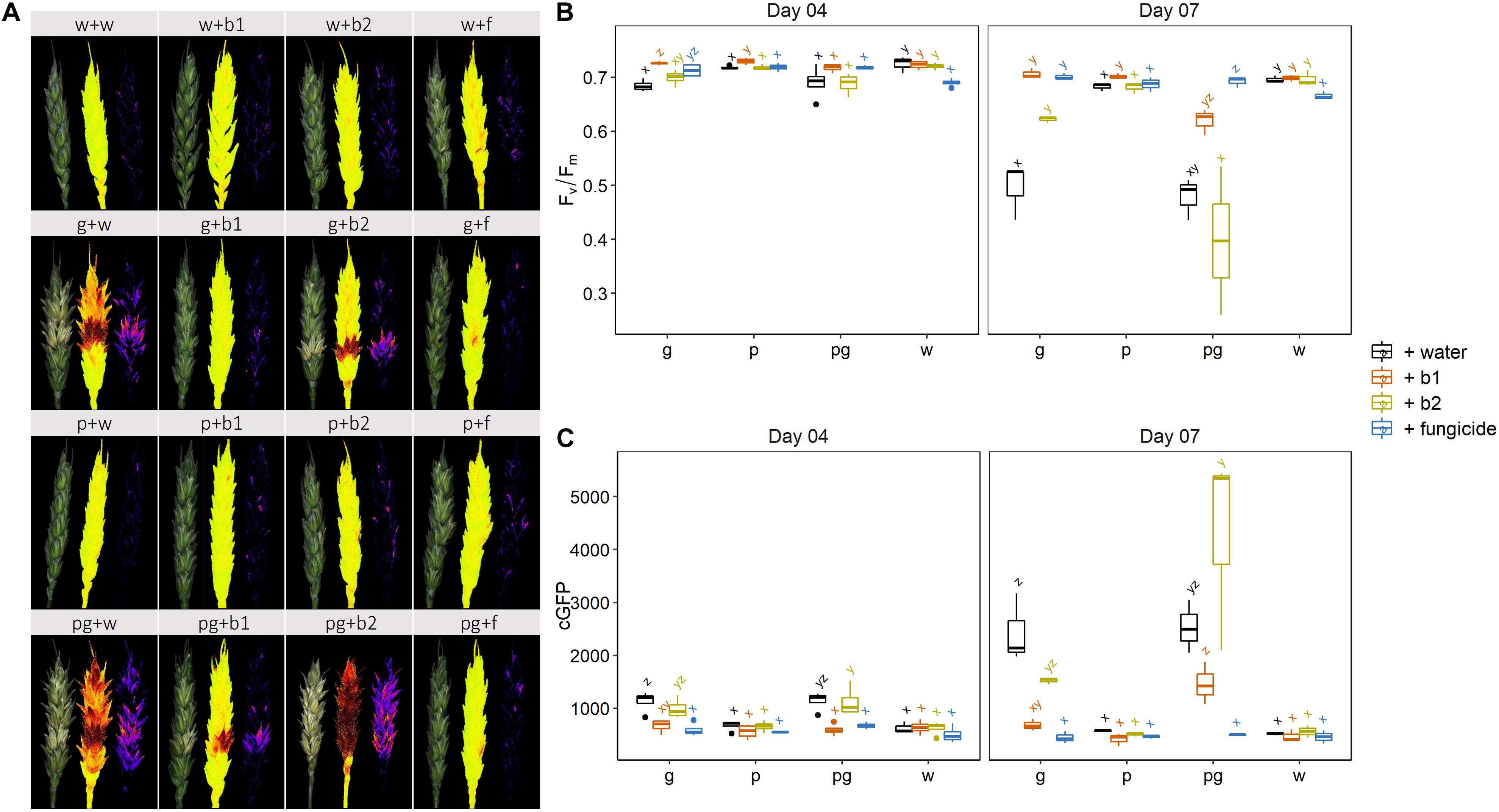
Figure 3. (A) Visual symptoms on wheat ears after infection by F. graminearum PH-1 (g), F. poae 2516 (p) or a combination of both pathogens (pg) and treated with water (+w), with S. rimosus LMG 19352 (+b1), with Rhodococcus sp. R-43120 (+b2) or with the fungicides prothioconazole + spiroxamine (f) at 7 dai. (B) Results of quantitation of the Fv/Fm (efficiency of photosystem II) signal at 4 and 7 dai, n = 6 (six biological reps per treatment and per pathogen combination). (C) Results of quantitation of the cGFP (corrected GFP value) signal (background signal) at 4 and 7 dai, n = 6 (six biological reps per treatment and per pathogen combination). Boxplots indicate the median (horizontal lines), 25th and 75th percentile range (boxes) and up to 1.5 × IQR (Interquartile Range) (whiskers). Different letters indicate significant differences (P < 0.05) between treatments for each time point within the same pathogen combination. The experiment was repeated twice in time.
At 4 dai, a slight but significant phytotoxic effect was observed in the mock inoculated ears treated with the fungicide which was hallmarked by a reduction in Fv/Fm. This slight phytotoxic effects were not observed in the combined treatments with both pathogens. In the F. graminearum infected ears, a small but significant biocontrol effect was observed for biocontrol strain b1 and the fungicide prothioconazole + spiroxamine, whereas b2 showed Fv/Fm values comparable to the control (Figure 3B). Finally, no biocontrol effects by either of both strains were observed in the ears co-inoculated with F. graminearum and F. poae. In parallel with symptoms (Fv/Fm) the fungal presence was also assessed through its GFP signal. Results of this analysis are shown in Figure 3C. The results of the F. graminearum biomass assessment through its GFP signal were similar to the Fv/Fm results.
At 7 dai, the mock-inoculated controls confirmed the very mild but significant negative effects of the fungicide treatment on the Fv/Fm values. In addition, it was clear that a single infection of wheat ears with F. graminearum, was efficiently controlled by both b1, b2 and the fungicide treatment which resulted in Fv/Fm values comparable to the mock inoculated control ears. Interestingly, the GFP signal indicated that b2 showed intermediate effects on the F. graminearum biomass, with values between the mock inoculated and inoculated control treatments. In contrast, the F. graminearum biomass in combination with b1 was strongly reduced and comparable to the fungicide treatment. In all the singular F. poae inoculations, no symptoms developed and Fv/Fm values of ears were not significantly different from the mock inoculated ears (Figures 3A,B).
Remarkably, despite the fact that the infection levels between the singular F. graminearum inoculation and the combined infection of F. graminearum and F. poae were not statistically different at Fv/Fm level or GFP level (p-values were respectively p = 0.123 and p = 0.405 after an independent sample t-test), none of the two biocontrol agents b1 or b2 displayed the same biocontrol capacity as observed in the singular F. graminearum infection. Lower Fv/Fm values were observed in the b1 and b2 treatments after dual infection with F. poae and F. graminearum compared to a singular infection with F. graminearum with p-values of 0.031 and 0.024 respectively (independent sample t-test). In addition, the Fv/Fm values of ears co-inoculated with F. poae and F. graminearum and treated with b1 and b2 were not significantly different from the infected controls. When assessing the GFP signal, an even more interesting phenomenon was observed for b1 and b2. In the combined infections of F. poae and F. graminearum, this biocontrol agent resulted in a consistent, significant increase in GFP signal compared to the singular F. graminearum infected control ears (with one-sided p-values of 0.015 and 0.040 respectively, t-test). This result shows that the efficacy of the biocontrol strains in regard to symptom development and fungal presence assessed through the GFP tag, is reduced when the FHB disease complex comprises both F. poae and F. graminearum (Figure 3).
Subsequently, we assessed the actively growing F. poae and F. graminearum through RT-qPCR of household genes (Supplementary Figure S2). These data show that biocontrol strain b1 efficiently reduces actively growing F. poae and F. graminearum on wheat ears. In addition, b2 does have an intermediate effect on F. poae when comparing with the F. poae infected water control, while no effect on F. graminearum PH-1 was observed. Again, similar as for Fv/Fm and GFP, the biocontrol strain b2 was less effective in reducing the biomass in the combined infection of F. poae and F. graminearum compared to a singular F. graminearum infection (p-value = 0.034, one-sided independent sample t-test).
In conclusion, the impact of the fungicide treatment seems independent of the FHB disease complex constitution, while both biocontrol treatments seemed less effective on F. graminearum when the latter was co-applied with the weak pathogen F. poae.
Impact of Streptomyces rimosus LMG 19352 (b1) and Rhodococcus sp. R-43120 (b2) on Type A and Type B Trichothecene Production When Co-applied With Fusarium sp. on Wheat Ears
We studied the impact of the biocontrol strains and the fungicide treatment on mycotoxin production by both F. poae and F. graminearum. None of the type A or type B trichothecenes known to be produced by F. poae 2516 were detected in any of the treatments. So in none of the interactions nivalenol (NIV); diacetoxyscirpenol (DAS) or neosolaniol (NEO) were observed above the detection limit. For the F. graminearum mycotoxins, we assessed the presence of the type B trichothecenes DON, 3-ADON and 15-ADON (Figure 4).
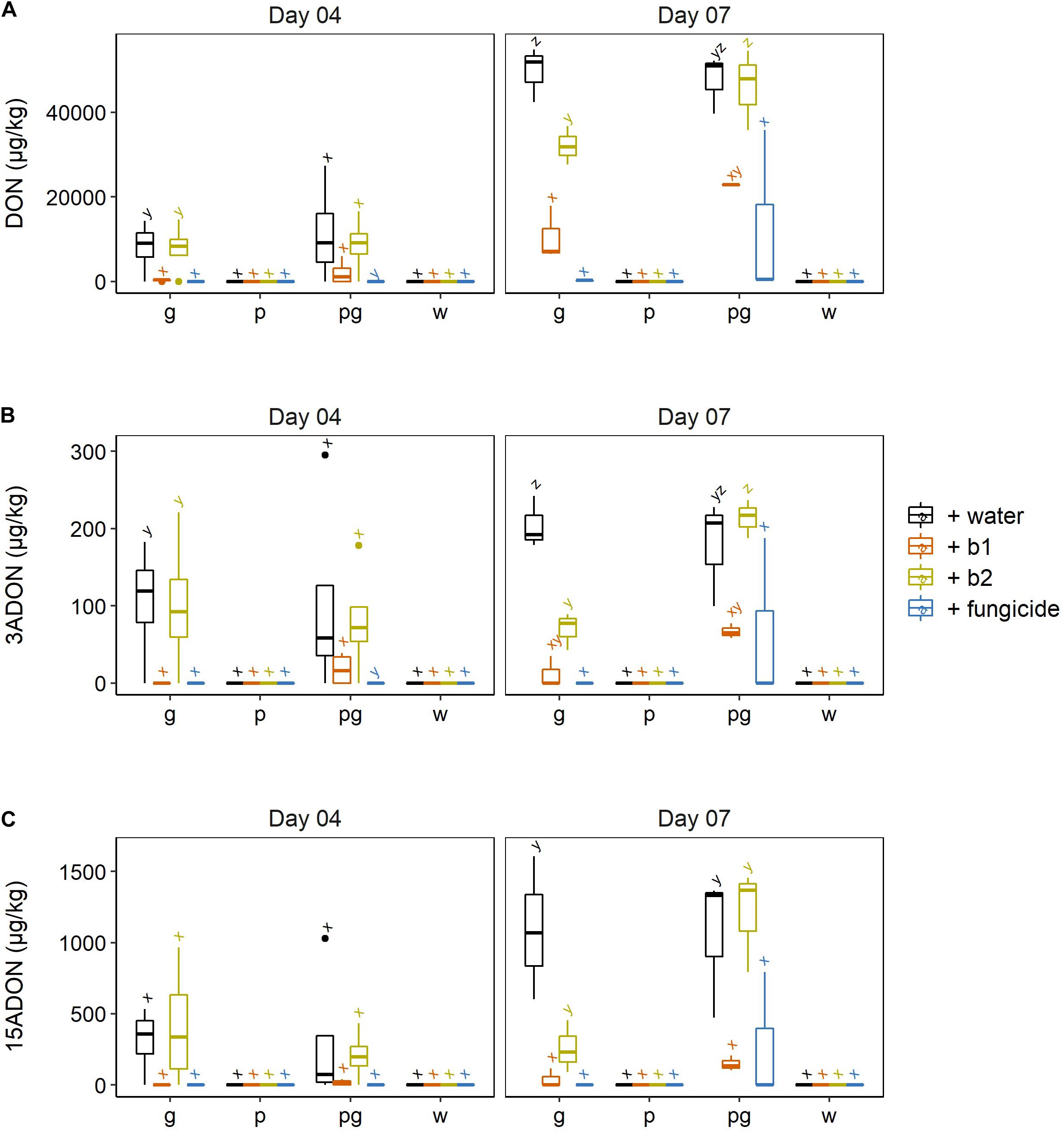
Figure 4. (A) DON, (B) 3ADON, and (C) 15ADON concentrations in wheat ears after infection by F. graminearum PH-1 (g), F. poae 2516 (p) or a combination of both pathogens (pg) and treated with water (+w), with S. rimosus LMG 19352 (+b1), with Rhodococcus sp. R-43120 (+b2) or with the fungicides prothioconazole + spiroxamine (f). Boxplots indicate the median (horizontal lines), 25th and 75th percentile range (boxes) and up to 1.5 × IQR (Interquartile Range) (whiskers). Different letters indicate significant differences (P < 0.05) between treatments for each time point within the same pathogen combination. The experiment was repeated twice in time.
At 4 dai, the ears that were infected by a singular F. graminearum infection and treated with the fungicide or the biocontrol agent b1 showed a significant decrease in DON. In contrast application of biocontrol strain b2 resulted in DON levels not significantly different from the inoculated singular F. graminearum control ears. Similar observations were done in regard to 3-ADON and 15-ADON although the differences of the latter ones were not significant.
In the dual inoculation of F. graminearum and F. poae, no significant reduction of DON nor its derivatives were observed due to the presence of biocontrol agents b1 and b2. Nevertheless, a non-significant reduction was observed for DON, 3-ADON, and 15-ADON for b1.
This trend was also apparent at 7 dai. In the singular F. graminearum infections, the application of b1 resulted in a significant reduction of DON, which was comparable to the reduction by the fungicide treatment. The biocontrol strain b2 resulted in a significant reduction of DON, but this reduction was significantly less pronounced than the reduction realized by b1. Similar conclusions could be drawn for 3-ADON (Figure 4B) and 15-ADON (Figure 4C).
At 7 dai, the impact of both biocontrol strains b1 and b2 on the dual inoculations of F. graminearum and F. poae was different compared to their effect in the singular F. graminearum interaction. Firstly, the capacity of b2 to reduce DON, 3-ADON, and 15-ADON was completely lost and the levels of these mycotoxins were not significantly different from levels in the infected control ears. In addition, also b1’s capacity to reduce F. graminearum, DON and its acetylated derivatives in the co-inoculations of F. graminearum and F. poae were significantly reduced compared to the singular F. graminearum inoculation (two-sided p-values = 0.029 and 0.013 for DON and 3-ADON, a one-sided p-value of 0.045 for 15-ADON).
Also the impact of the prothioconazole + spiroxamine fungicide treatment on DON, 3-ADON and 15-ADON levels appeared different between the singular F. graminearum versus dual infection with F. graminearum and F. poae. In the singular F. graminearum infection hardly any toxin was detected while in the dual infection with F. graminearum and F. poae sometimes high amounts were observed for all three toxins although this difference was not significant due to the high variability in the toxin data in the latter interaction (Figure 4). These data on the chemical control of FHB demonstrate that the efficacy of the chemical control shows an increased variability and as such is less consistent when both F. poae and F. graminearum are present in the FHB disease complex.
Impact of Streptomyces rimosus LMG 19352 (b1) and Rhodococcus sp. R-43120 (b2) on Plant Derived DON-3G When Co-applied With Fusarium spp. on Wheat Ears
As DON is a phytotoxin, plants have developed detoxification strategies for DON (Berthiller et al., 2013). One of the best described strategies for plants to cope with DON is the glucosylation of DON, after which DON-3-glucoside (DON3G) is directed to the vacuole (Audenaert et al., 2014). In this respect, we also checked whether the presence of biocontrol strains affected the plant’s capacity to glucosylate DON (Figure 5A). Here, the results were less clear: although b1 resulted in a lower ratio of glucosylated DON, there were no significant differences between treatments (Figure 5B).
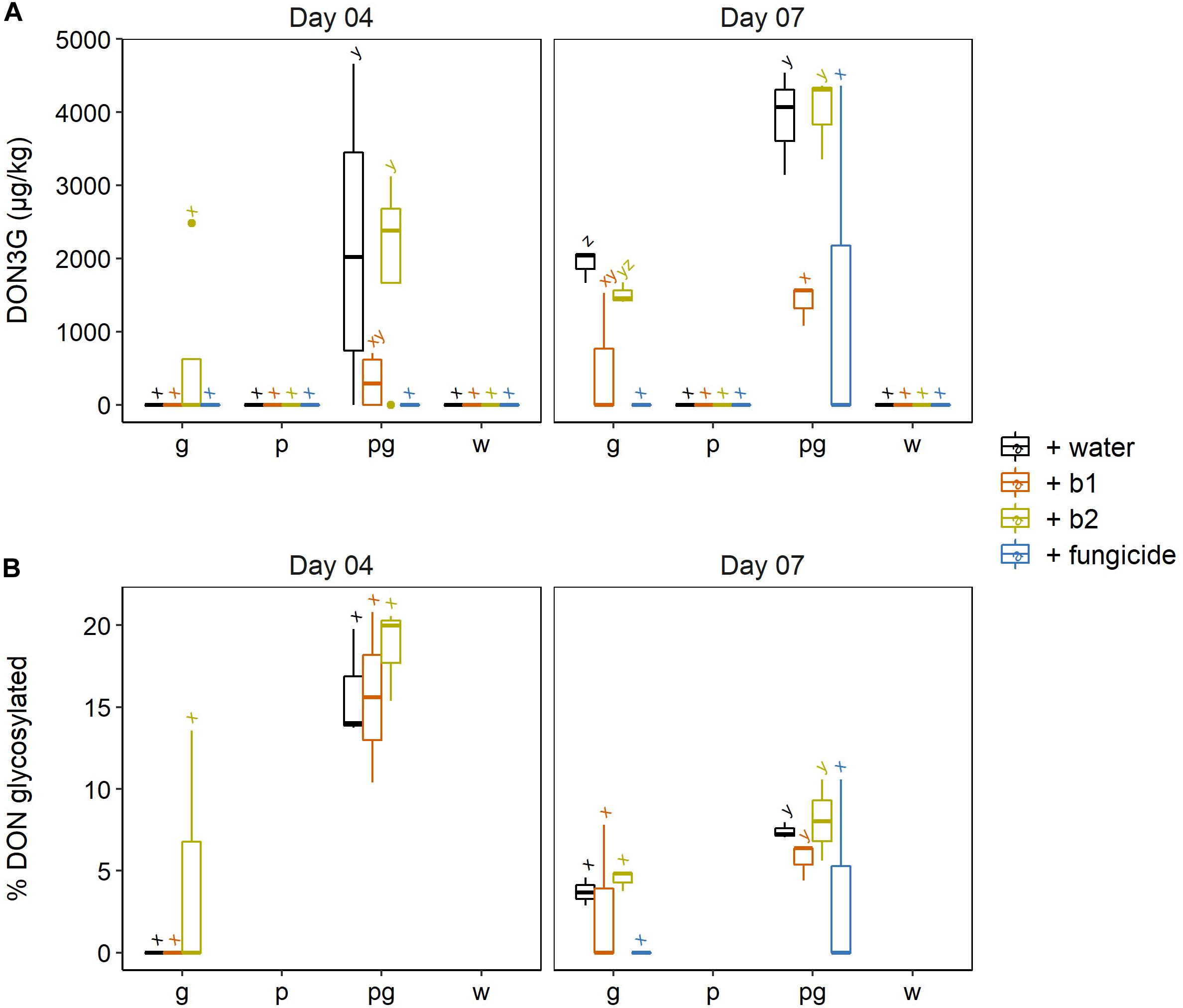
Figure 5. (A) DON-3G and (B) percentage of DON being glycosylated in wheat ears after infection by F. graminearum PH-1 (g), F. poae 2516 (p) or a combination of both pathogens (pg) and treated with water (+ w), with S. rimosus LMG 19352 (+b1), with Rhodococcus sp. R-43120 (+b2) or with the fungicides prothioconazole + spiroxamine (f). Boxplots indicate the median (horizontal lines), 25th and 75th percentile range (boxes) and up to 1.5 × IQR (Interquartile Range) (whiskers). Different letters indicate significant differences (P < 0.05) between treatments for each time point within the same pathogen combination. The experiment was repeated twice in time.
At 4 dai, the singular F. graminearum inoculations did not result in any accumulation of DON-3G, with the exception of 1 positive sample in the ears treated with b2. On the contrary, in the dual inoculations with F. graminearum and F. poae, all treatments resulted in the accumulation of DON-3G and the highest DON-3G levels were observed in the water- and b2 treated wheat ears (Figure 5A). This difference in glucosylation was not due the fact that less DON, 3-ADON or 15-ADON had been formed in the singular F. graminearum interactions as the content of these trichothecenes was the same in the singular F. graminearum inoculations versus the dual F. graminearum and F. poae inoculations. This points to a higher DON glucosylation rate in the dual inoculations independently of the water, b1, b2 or fungicide treatment.
Also at 7 dai, both DON-3G levels and DON glucosylation ratios per treatment (water, b1, b2 or fungicide) were always higher in the dual inoculation of F. poae and F. graminearum compared to the singular F. graminearum infection (Figures 5A,B). These differences in DON-3G levels were not attributed to the fact that less DON, 3-ADON or 15-ADON had been formed in the singular F. graminearum interactions, as the content of these trichothecenes was the same in the singular F. graminearum inoculations versus the dual F. graminearum and F. poae inoculations (cf. Figure 4). This points to a higher DON glucosylation rate in the dual inoculations, independently of the water, b1, b2 or fungicide treatment. At 7 dai, the increased ratio of DON-3G in ears co-inoculated with F. graminearum and F. poae, compared to ears inoculated with F. graminearum alone, was observed for all treatments (water, b1, b2, fungicide) although it was not significant for the fungicide treatment due to the high variability of the data.
Wheat ears treated with biocontrol strain b1 resulted in almost no symptom development. Counterintuitively, this strain showed the lowest DON-3G ratios in wheat ears which shows that DON-glucosylation is probably not involved in symptom reduction by S. rimosus LMG19352.
Impact of Streptomyces rimosus LMG 19352 (b1) and Rhodococcus sp. R-43120 (b2) on Key Genes in the Biosynthesis of Jasmonic Acid and Salicylic Acid
Timely upregulation of ICS- and LOX genes can result in a good control of FHB symptoms caused by F. graminearum (Ameye et al., 2015) and F. poae interferes with these processes and thereby influences symptom development by F. graminearum (Tan et al., 2020). Hence we wanted to assess the involvement of these genes in the biocontrol of b1 and b2 and in the chemical control by the fungicide application. Therefore, the expression levels were determined of genes encoding hallmark enzymes phenylalanine ammonia-lyase (PAL) and isochorismate synthase (ICS) (for the SA biosynthesis) and lipoxygenases LOX1 and LOX2 (for the biosynthetic pathway leading to JA).
At 4 dai, we observed a significant induction of ICS by the fungicide (prothioconazole + spiroxamine) compared to the mock inoculated water treatment (Figure 6). In addition, a small but significant upregulation of ICS was observed in the singular F. graminearum infected wheat ears treated with biocontrol strain b1. Finally, when considering the impact of the pathogen alone on the expression of ICS, a small but significant upregulation of ICS compared to mock inoculated wheat ears was observed after singular inoculation of wheat ears with F. poae but not of F. graminearum in the water treatment (p = 0.014 and p = 0.993, respectively independent sample t-test).
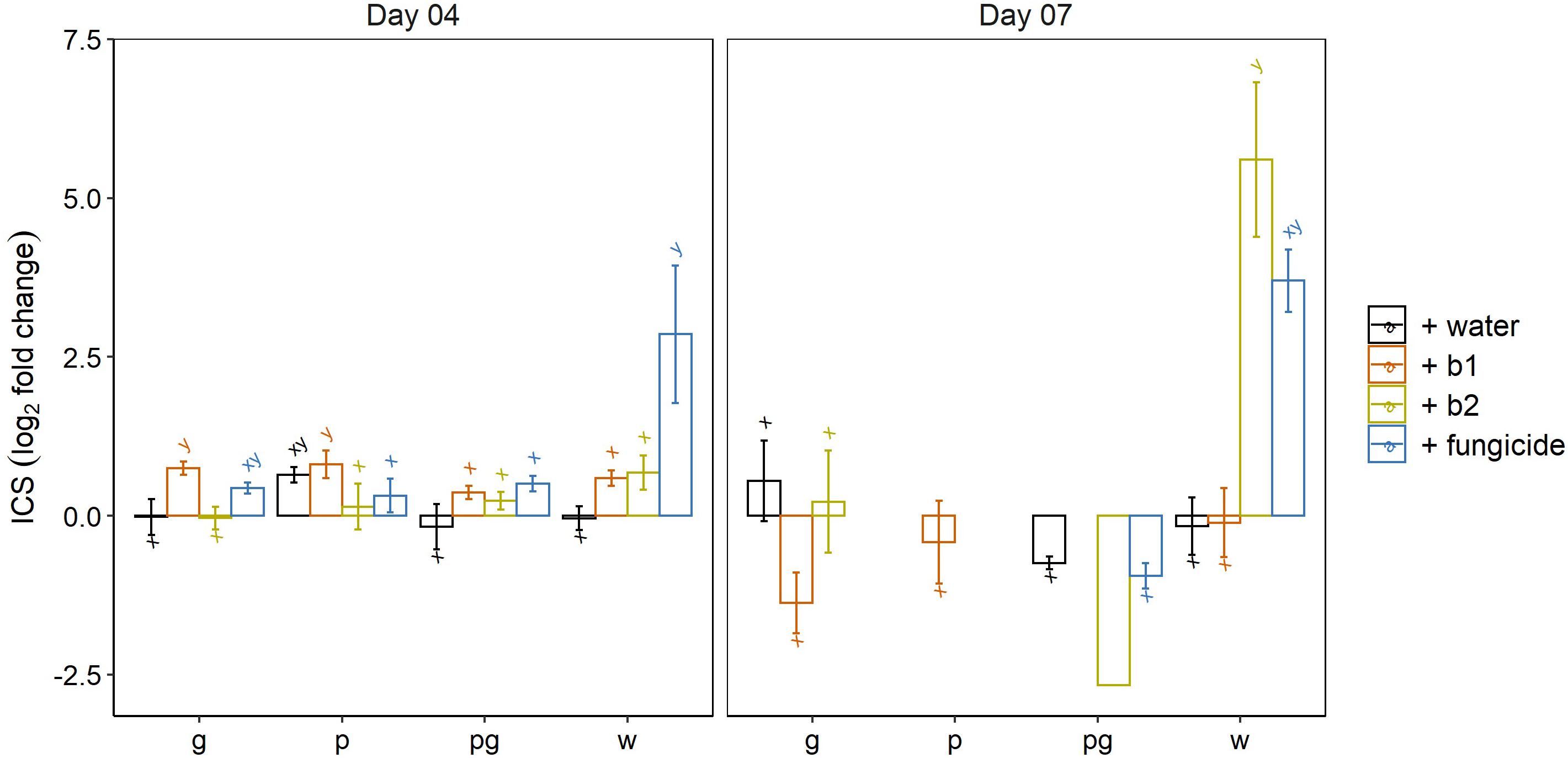
Figure 6. Expression profile of isochorismate synthase (ICS) at 4 and 7 dai by F. graminearum PH-1 (g), F. poae 2516 (p) or a combination of both pathogens (pg) and treated with water (+w), with S. rimosus LMG 19352 (+b1), with Rhodococcus sp. R-43120 (+b2) or with the fungicides prothioconazole + spiroxamine (f). Bars represent log2-transformed means of four biological replicates; error bars indicate ± 1 standard error. Different letters indicate significant differences (P < 0.05) between treatments for each time point within the same pathogen combination. Fold change was calculated by dividing the CNRQ values (calibrated normalized relative quantities) of the treated ears by values of the control ears. Normalization of defense genes was performed by using the cell division control protein gene (Ta54227) in wheat as reference. Missing bars for the F. graminearum (g), the F. poae (p) and the combined infection (pg) point to an ICS expression level (and Ct value) below the limit of detection. The experiment was repeated twice in time.
At 7 dai we observed a significant upregulation of ICS in the mock inoculated wheat ears treated with biocontrol strain b2. This induction of ICS was comparable to the induction of ICS by the fungicide prothioconazole + spiroxamine. However, the latter one was not significantly different from the control due to variability in the data. This result shows that biocontrol strain b2 triggers the SA biosynthesis pathway. Remarkably, the induction of ICS disappeared in all interactions of b2 in which a pathogen was involved (F. graminearum and/or F. poae). Similar expression analyses were done for phenylalanine ammonia lyase (PAL). At 4 dai, an induction of PAL was observed in all treatments comprising a singular F. graminearum inoculation. At 7 dai, only the combined application of b2 and F. graminearum remained significant when comparing with the mock-inoculated control treatment (Supplementary Figure S3).
Jasmonic acid mediated plant defense was monitored by following the expression profiles of lipoxygenases 1 and 2. At 4 dai, a clear induction of LOX1 was observed in the mock-inoculated wheat ears treated with the fungicide prothioconazole + spiroxamine and wheat ears treated with the biocontrol strain b2. Both inductions were highly variable and therefore not significantly different from the mock-inoculated water control (Figure 7A). We attribute this high variability to the fact that 4 dai is a critical time point in which in some ears LOX1 gene expression has been triggered while in others, the level of LOX1 expression is still at basal levels. A clear and significant induction of LOX1 was observed in all treatments of the singular F. graminearum inoculated wheat ears and in the water and b2 treated ears inoculated with F. poae which confirmed our previous results on the induction of LOX1 by F. poae and F. graminearum (Tan et al., 2020). In addition, per treatment (water, b1, b2 and fungicide) this induction of LOX1 by singular inoculations by F. graminearum was suppressed when F. graminearum was co-inoculated with F. poae (Figure 7A).
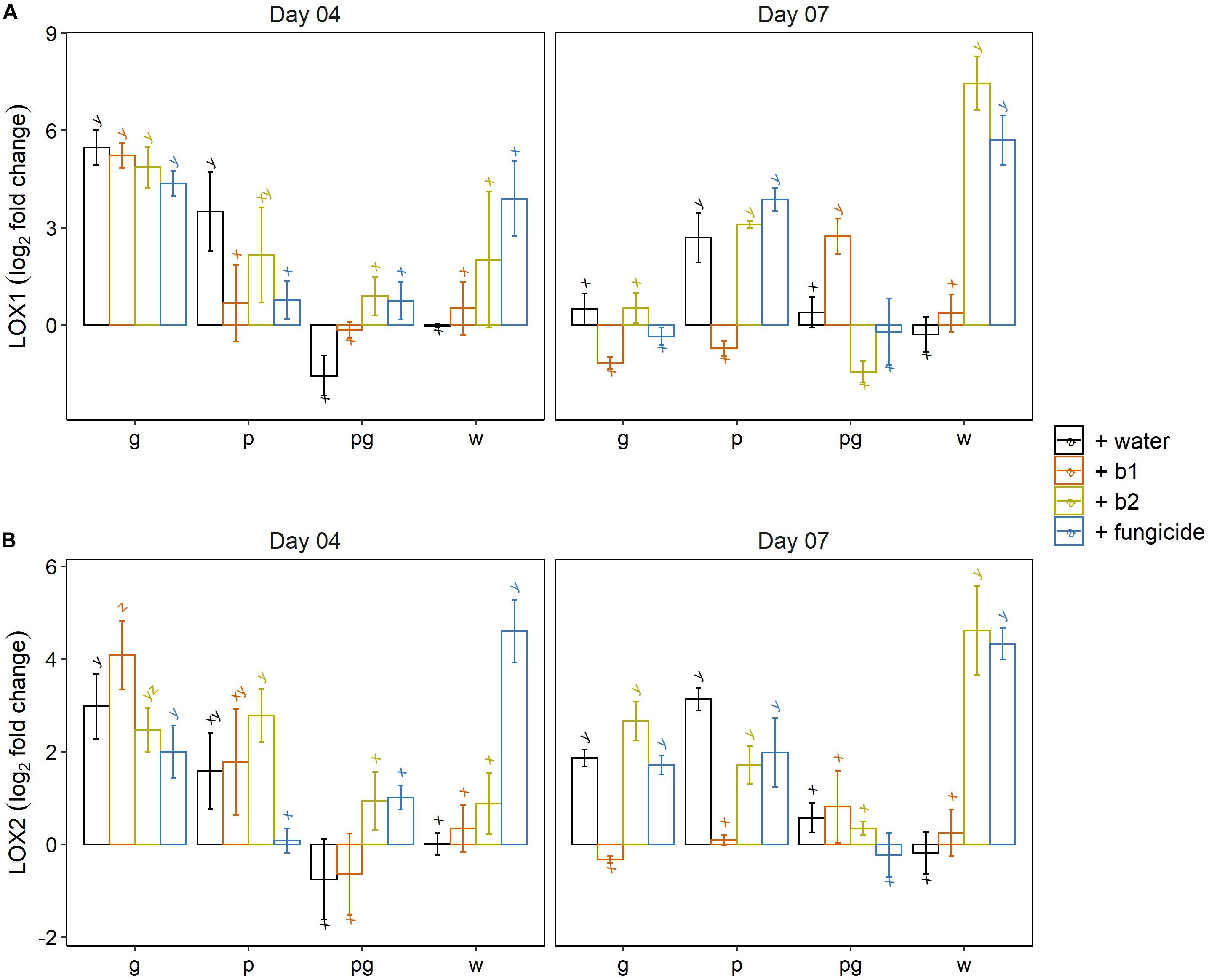
Figure 7. Expression profile of Lipoxygenase 1 (A) and Lipoxygenase 2 (B) (LOX1 and LOX2) at 4 and 7 dai by F. graminearum PH-1 (g), F. poae 2516 (p) or a combination of both pathogens (pg) and treated with water (+w), with S. rimosus LMG 19352 (+b1), with Rhodococcus sp. R-43120 (+b2) or with the fungicides prothioconazole + spiroxamine (f). Bars represent log2-transformed means of four biological replicates; error bars indicate ± 1 standard error. Different letters indicate significant differences (P < 0.05) between treatments for each time point within the same pathogen combination. Fold change was calculated by dividing the CNRQ values (calibrated normalized relative quantities) of the treated ears to the control ears. Normalization of defense genes was performed by using the cell division control protein gene (Ta54227) in wheat as reference. The experiment was repeated twice in time.
At 7 dai, a clear and consistent induction of LOX1 was observed in the mock-inoculated controls treated with b2 and the fungicide prothioconazole + spiroxamine. The wheat ears that were inoculated by F. poae all showed an induction of LOX1 with the exception of the b1 treatment. The biocontrol agent b1 resulted in a slight repression of LOX1 gene expression compared to the mock-inoculated water control.
For LOX2 at 4 dai, the fungicide prothioconazole + spiroxamine resulted in a significant upregulation of LOX2 compared to the mock inoculated water control. In addition, the induction of LOX2 by a singular F. graminearum and F. poae infection was observed. Within the singular F. poae interactions, the induction of LOX2 in all treatments except for the fungicide treatment was significant. Again, the induction of LOX2 that was observed in the singular inoculations, was suppressed in all treatments in which F. graminearum was co-inoculated with F. poae.
At 7 dai, a significant induction of LOX2 was observed in the mock-inoculated control ears treated with the biocontrol strain b2 or the fungicide. In the singular F. poae and F. graminearum inoculations, the induction of LOX2 was observed in the water, b2 and fungicide treatments while the application of biocontrol strain b1 resulted in a slight suppression of LOX2 gene expression. In all treatments (water, b2 and fungicide) the induction of LOX2 that was observed in the singular inoculations, was suppressed when F. graminearum was co-inoculated with F. poae.
In conclusion, the ICS, LOX1 and LOX2 expression data show that biocontrol strain b2 and the fungicide prothioconazole + spiroxamine induce ICS, LOX1 and LOX2 even in absence of a pathogen. Secondly, a singular F. graminearum inoculation resulted in an induction of LOX1 and LOX2 gene expression independently of the treatment (water, b1, b2 and fungicide). Similar results were obtained for the singular F. poae inoculations although the b1 treatment for LOX1 and the fungicide treatment for LOX2 did not show this induction. Finally, co-inoculation of F. graminearum and F. poae resulted in LOX1 and LOX2 expression levels similar to the expression levels in mock-inoculated control ears which points to a complete suppression of JA-dependent defense in wheat ears inoculated with F. poae and F. graminearum.
Discussion
When a plant is infected by a pathogen, a complex interaction between plant, pathogen and the residing microbial community starts (e.g., del Barrio-Duque et al., 2020). The outcome of this interaction is then typically determined by the mutual interplay of plant, microbial and plant pathogen-derived signals and pathways. The knowledge that plant pathogens can be controlled through the action of other microbes has spurred researchers to explore the use of these antagonistic bacteria and fungi as biocontrol agents against fungal, bacterial, and oomycete pathogens. Their modes of action comprise direct antagonism, antibiosis, mycoparasitism, competition for niche, and induction of the plant’s intrinsic plant defense amongst others (De Silva et al., 2019; Khan et al., 2020; Raymaekers et al., 2020).
Yet, to date, no in-depth information is available on the impact of chemical control agents or biocontrol agents on the outcome of plant diseases caused by complexes of microbes from the same or other genus (Lamichhane and Venturi, 2015). Nevertheless, such disease complexes comprise devastating diseases such as Black sigatoka disease in banana caused by several Mycosphaerella sp. (Arzanlou et al., 2007), potato leaf spot which is caused by a complex of Alternaria sp. (Vandecasteele et al., 2018), rice sheath rot disease caused by Fusarium sp. and Sarocladium oryzae (Bigirimana et al., 2015) and the FHB disease in wheat which is considered in the present paper. Studies on control strategies to control these fungal disease complexes are still at their infancy and to our knowledge when available, studies on biocontrol strategies only target one member of a disease complex rather than looking at the impact of biocontrol strains on the multiple members of such a disease complex. For FHB, focus is commonly on F. graminearum and biocontrol agents against this pathogen have been described in detail. Streptomyces sp. (Palazzini et al., 2017), Pseudomonas sp. (Wang et al., 2015; Chen et al., 2018), Bacillus sp. (Palazzini et al., 2007), and fungal strains Trichoderma sp. (Mahmoud, 2016; Sarrocco et al., 2020), Cryptococcus sp. (Zhang et al., 2007), and Clonostachys sp. (Xue et al., 2014; Demissie et al., 2020) are examples of a non-exhaustive list of biocontrol agents that have been shown to reduce FHB symptoms by F. graminearum. However, the impact of other members residing in the FHB disease complex on the biocontrol efficacy of biocontrol strains was not studied so far.
In present work, we used Actinobacteria belonging to the genera of Streptomyces and Rhodococcus to investigate their impact on FHB caused by a complex of F. poae and F. graminearum. A fungicide with prothioconazole + spiroxamine as active ingredients was included as a control. In wheat ears, both strains were good biocontrol agents against a singular F. graminearum infection although b1 showed a better control capacity based on the reduction of the fungal biomass than b2. However, when applied in wheat ears co-inoculated with F. poae and F. graminearum, both biocontrol strains had significantly reduced biocontrol capacity, which shows that the presence of F. poae hampers the biocontrol of F. graminearum by both strains. This finding was striking as both biocontrol strains had different modes of action and interacted differently with the wheat plant. Moreover, Streptomyces rimosus LMG 19352 (b1) showed a clear antagonism with F. graminearum (Figure 2) and Rhodococcus sp. R-43120 (b2) showed a significantly induction of plant defense (Figures 6, 7).
The reduced biocontrol efficacy was also observed at the level of the mycotoxin production by F. graminearum in the combined inoculations with F. poae. In search for an explanation, we found that this reduced control of F. graminearum was not mediated by F. poae producing type A and type B trichothecenes (NIV, DAS, NEO) during its colonization as these trichothecenes were not present at detectable levels in wheat ears. For the chemical control using prothioconazole + spiroxamine, a decreased efficacy was observed for accumulation of DON, 15-ADON and 3-ADON in ears colonized by a combination of F. graminearum and F. poae compared to the singular F. graminearum infections. This result shows that efficacy of chemical control in FHB depends on the complexity of the disease complex and that some Fusarium species might hamper a good control.
When assessing the plant defense response, we observed that Rhodococcus sp. R-43120 (b2) was shown to induce ICS, LOX1, and LOX2 genes in mock inoculated wheat ears which shows that this strain can trigger the innate plant’s defense system especially at later timepoints after application. The induction of LOX genes by Actinobacteria is not new. Previously, Abbasi et al. (2019) demonstrated an activation of plant defense including the induction of LOX genes by Actinobacteria. In order to link this observation with the biocontrol capacity of the strain, we investigated the expression of the LOX genes in the presence of both Fusarium pathogens. When looking at the expression levels of LOX1 and LOX2 at 4 dai, both genes were induced by F. poae and F. graminearum independently of the presence of biocontrol strain Rhodococcus sp. R-43120 (b2) while the combined F. graminearum and F. poae inoculations in absence or presence of b2 resulted in the induction of LOX1 or LOX2. LOX1 lacks a chloroplast targeting peptide while LOX2 is targeted to the chloroplast (Feng et al., 2010). At 7 dai, the expression got more differential and we observed that b2 induced both LOX1 and LOX2 in the mock inoculated controls and in the singular F. poae infections. In addition, the F. poae inoculation itself results in the induction of both LOX genes. However, in the interaction with F. graminearum only LOX2 was induced, while LOX1 was not significantly different from the mock inoculated controls. Finally, in the interaction with the dual infection with both F. poae and F. graminearum neither of the LOX genes was significantly induced. As in the latter interaction, no biocontrol effect of b2 was observed while the strain showed good biocontrol effects in the singular F. graminearum interaction, we hypothesize that especially the induction of LOX2 could be crucial for the biocontrol effect of biocontrol strain b2, while LOX1 is probably not involved in the biocontrol trait. The role of 9-LOXs in susceptibility of wheat and in DON production by F. graminearum has previously been established (Nobili et al., 2014; Nalam et al., 2015). In the past, 13-LOXs have been associated with high basal resistance of the well-known wheat cultivar Sumai 3 (Zhuang et al., 2013). From work in other crops, it is known that 9-LOXs are targets for microbial effectors which can lead to transcriptional reprogramming and subsequent increased resistance or susceptibility (Battilani et al., 2018). In addition, in maize it has been shown that 9-LOXs and 13-LOXs play reciprocal roles in the susceptibility or resistance against respectively, F. verticillioides and A. flavus (Gao et al., 2009). We want to pinpoint that it is known that LOXs are found to yield mixtures of 9- or 13 hydroperoxide variants but that the presence of a chloroplast targeting peptide in LOX2 versus LOX1 is discriminative (Feng et al., 2010).
The biocontrol of F. graminearum by application of b2 through induction of LOX genes and ICS confirms the importance of SA-and JA-dependent defense responses against this hemi-biotrophic pathogen. It has been shown previously that a meticulously time-dependent up-and down regulation of SA- and JA-dependent defenses determines the outcome of F. graminearum in wheat (Ameye et al., 2015).
In addition, we have previously demonstrated that F. poae, when present in the FHB disease complex together with F. graminearum, influences the outcome of the F. graminearum infection by modulating the SA- and JA derived plant defenses (Tan et al., 2020). Small distortions in these interconnected pathways might have serious impacts on the outcome of the interaction of wheat with F. graminearum. These small aberrancies can be different environments, control strategies, different timing of infection of FHB members or different composition of the FHB disease complex. Several of these components have been nicely reviewed by Xu and Nicholson (2009).
In addition, these aberrations can comprise cross kingdom interactions. This was also reported in a different study, where we investigated an inter-kingdom interaction between wheat, F. graminearum and Sitobion avenae (English grain aphid) (De Zutter et al., 2017). In that work, pre-infestation of wheat ears with aphids resulted in a down regulation of PAL at early time points, which resulted in a suppression of PAL during a subsequent F. graminearum infection and a more proliferated outgrowth of the fungal pathogen compared to a single F. graminearum infection (De Zutter et al., 2017).
For the chemical control, we could demonstrate that in mock-inoculated wheat ears that were treated with prothioconazole + spiroxamine a clear induction of LOX1, LOX2, and ICS was observed. Knowledge on the impact of fungicides at the level of the intrinsic plant defense system is still scarce and unexplored. Our results suggest that the chemical control of F. graminearum might be the result of a combination of fungicidal effects and plant-mediated antifungal responses.
Because especially biocontrol strain b2 interfered with the plant defense mechanism at the level of LOX- and ICS gene expression, we further explored the presence of DON-3G, a metabolite resulting from the glucosylation of DON by the plant enzyme uridine diphosphate-glucosyltransferase. From literature, it is known that glucosylation in wheat is associated with the quantitative trait locus Fhb1. Moreover, wheat cultivars carrying this locus have higher DON-3G/DON ratios (Berthiller et al., 2013; Audenaert et al., 2014; Kluger et al., 2015). Finally, it is known that several biocontrol agents such as Clonostachys rosea can increase the plant’s capacity to glucosylate DON (Abdallah et al., 2018). Despite the fact that DON glucosylation is frequently reported in literature as an efficient strategy of plants or biocontrol agents to detoxify DON, no clear induction of DON glucosylation was observed in wheat ears upon application of biocontrol strains b1, b2 or the fungicide prothioconazole + spiroxamine in our experiments. However, co-inoculation of wheat ears by F. poae and F. graminearum resulted in an increased DON-3G/DON ratio compared to singular F. graminearum inoculations. This observation was found for all treatments (water, b1, b2 and fungicide indicating that the presence of F. poae in the disease complex results in an increased DON-glucosylation activity.
In conclusion, we have shown that Streptomyces rimosus LMG 19352 and Rhodococcus sp. R-43120 efficiently reduced F. graminearum symptoms in wheat ears. In addition, we demonstrated that the biocontrol capacity of these actinobacterial strains and to a lesser extent the fungicide prothioconazole + spiroxamine was critically hampered by the presence of the weakly pathogenic F. poae. As a more generic conclusion, our study shows that integrated control strategies against FHB and other plant diseases that are caused by a multiple fungi might be far more complex and difficult than initially thought due to the presence of multiple species in these so-called pathobiomes, calling for an articulation of Koch’s postulates. As we start to unravel the complex aspects of pathobiomes, it may become clear how these species complexes operate in a multiplayer mode to cause symptoms in plants.
Data Availability Statement
The original contributions presented in the study are included in the article/Supplementary Materials, further inquiries can be directed to the corresponding author/s.
Author Contributions
JT, MA, and KA designed the experiments. JT performed all the experiments. ND performed the image and data analysis. PV and AW coordinated the identification of the actinobacterial isolates. SDS, TT, and MD interpreted the mycotoxin data. JT, MA, KA, CW, TVDL, and MD wrote the manuscript. ND, CW, TVDL, and AW read and revised the manuscript. All the authors contributed to the article and approved the submitted version.
Funding
This work was supported by a BOF doctoral fellowship of Ghent University that was given to JT and an FWO-SB scholarship (grant number 1S01620N) that was given to ND. We acknowledge the Hercules initiative for the multispectral imaging platform that was granted (grant number: AUGE/15/17).
Conflict of Interest
The authors declare that the research was conducted in the absence of any commercial or financial relationships that could be construed as a potential conflict of interest.
Supplementary Material
The Supplementary Material for this article can be found online at: https://www.frontiersin.org/articles/10.3389/fpls.2021.641890/full#supplementary-material
Supplementary Figure S1 | Effect of Streptomyces rimosus strains LMG 19352 (b1) and Rhodococcus sp. R-43120 (b2) on the growth of F. poae 2516 and F. graminearum PH-1 combined inoculation on TSA plates. Treatments: pg + w (Inoculation with F. poae 2516, F. graminearum PH-1 and water); pg + b1 (Inoculation with F. poae 2516, F. graminearum PH-1 and LMG 19352); p + b2 (Inoculation with F. poae 2516, F. graminearum PH-1 and R-43120). (A) Images taken from day 1 till day 5. (B) Results of F. poae and F. graminearum growth from day 1 till day 5, n = 5 (five biological reps per time point). Boxplots indicate the median (horizontal lines), 25th and 75th percentile range (boxes) and up to 1.5 × IQR (whiskers).
Supplementary Figure S2 | Effect of Streptomyces rimosus strains LMG 19352 (b1) and Rhodococcus sp. R-43120 (b2) on the biomass of F. graminearum PH-1 and F. poae 2516 on wheat ears, respectively. Normalized quantitative relative values (NRQ) of F. graminearum PH-1 (A) and F. poae 2516 (B) biomass in wheat ears, n = 6 (number of ears per treatment, per pathogen combination and per time point). Boxplots indicate the median (horizontal lines), 25th and 75th percentile range (boxes) and up to 1.5 × IQR (Interquartile Range) (whiskers). Different letters indicate significant differences between treatments (P < 0.05). NRQ values were assessed at 4 dai in wheat ears.
Supplementary Figure S3 | Expression profile of phenylalanine ammonia-lyase (PAL) at 4 and 7 dai by F. graminearum PH-1 (g), F. poae 2516 (p) or a combination of both pathogens (pg) and treated with water (+w), with S. rimosus LMG 19352 (+b1), with Rhodococcus sp. R-43120 (+b2) or with the fungicides prothioconazole + spiroxamine (f). Bars represent log2-transformed means of four biological replicates; error bars indicate ± 1 standard error. Different letters indicate significant differences (P < 0.05) between treatments for each time point within the same pathogen combination. Fold change was calculated by dividing the CNRQ values (calibrated normalized relative quantities) of the treated ears by values of the control ears. Normalization of defense genes was performed by using the cell division control protein gene (Ta54227) in wheat as reference. The experiment was repeated twice in time.
Supplementary Figure S4 | Visual scoring assessment of ears inoculation.
Supplementary Table S1 | Primers used for RT-qPCR.
References
Abbasi, S., Safaie, N., Sadeghi, A., and Shamsbakhsh, M. (2019). Streptomyces strains induce resistance to Fusarium oxysporum f. sp. lycopersici race 3 in tomato through different molecular mechanisms. Front. Microbiol. 10:1505. doi: 10.3389/fmicb.2019.01505
Abdallah, F. M., De Boevre, M., Landschoot, S., De Saeger, S., Haesaert, G., and Audenaert, K. (2018). Fungal endophytes control Fusarium graminearum and reduce trichothecenes and zearalenone in maize. Toxins 10:493. doi: 10.3390/toxins10120493
Ameye, M., Audenaert, K., De Zutter, N., Steppe, K., Van Meulebroek, L., Vanhaecke, L., et al. (2015). Priming of wheat with the green leaf volatile Z-3-hexenyl acetate enhances defense against Fusarium graminearum but boosts deoxynivalenol production. Plant Physiol. 167, 1671–1684. doi: 10.1104/pp.15.00107
Arzanlou, M., Abeln, E. C., Kema, G. H., Waalwijk, C., Carlier, J., and Vries, I. d, et al. (2007). Molecular diagnostics for the sigatoka disease complex of banana. Phytopathology 97, 1112–1118. doi: 10.1094/phyto-97-9-1112
Audenaert, K., Landschoot, S., Vanheule, A., Waegeman, W., De Baets, B., and Haesaert, G. (2011). “Impact of fungicide timing on the composition of the Fusarium head blight disease complex and the presence of deoxynivalenol (DON) in wheat,” in Fungicides-Beneficial and Harmfull Aspects, ed. N. Thajuddin (Rijeka: InTech), 79–98. doi: 10.3390/microorganisms9010079
Audenaert, K., Vanheule, A., Höfte, M., and Haesaert, G. (2014). Deoxynivalenol: a major player in the multifaceted response of Fusarium to its environment. Toxins 6, 1–19. doi: 10.3390/toxins6010001
Baker, N. R. (2008). Chlorophyll fluorescence: a probe of photosynthesis in vivo. Ann. Rev. Plant Biol. 59, 89–113. doi: 10.1146/annurev.arplant.59.032607.092759
Battilani, P., Lanubile, A., Scala, V., Reverberi, M., Gregori, R., Falavigna, C., et al. (2018). Oxylipins from both pathogen and host antagonize jasmonic acid-mediated defense via the 9-lipoxygenase pathway in Fusarium verticillioides infection of maize. Mol. Plant Pathol. 19, 2162–2176. doi: 10.1111/mpp.12690
Bebber, D. P., Ramotowski, M. A., and Gurr, S. J. (2013). Crop pests and pathogens move polewards in a warming world. Nat. Clim. Chang. 3, 985–988. doi: 10.1038/nclimate1990
Berthiller, F., Crews, C., Dall’Asta, C., Saeger, S. D., Haesaert, G., Karlovsky, P., et al. (2013). Masked mycotoxins: a review. Mol. Nutr. Food Res. 57, 165–186.
Bigirimana, V. d. P, Hua, G. K., Nyamangyoku, O. I, and Höfte, M. (2015). Rice sheath rot: an emerging ubiquitous destructive disease complex. Front. Plant Sci. 6:1066. doi: 10.3389/fpls.2015.01066 eCollection 2015
Brennan, J., Fagan, B., Van Maanen, A., Cooke, B., and Doohan, F. (2003). Studies on in vitro growth and pathogenicity of European Fusarium fungi. Eur. J. Plant Pathol. 109, 577–587.
Chen, Y., Wang, J., Yang, N., Wen, Z., Sun, X., Chai, Y., et al. (2018). Wheat microbiome bacteria can reduce virulence of a plant pathogenic fungus by altering histone acetylation. Nat. Commun. 9, 1–14.
Conn, V., Walker, A., and Franco, C. (2008). Endophytic actinobacteria induce defense pathways in Arabidopsis thaliana. Mol. Plant Microbe Interact. 21, 208–218. doi: 10.1094/mpmi-21-2-0208
Corredor-Moreno, P., and Saunders, D. G. (2020). Expecting the unexpected: factors influencing the emergence of fungal and oomycete plant pathogens. New Phytol. 225, 118–125. doi: 10.1111/nph.16007
De Boevre, M., Di Mavungu, J. D., Maene, P., Audenaert, K., Deforce, D., Haesaert, G., et al. (2012). Development and validation of an LC-MS/MS method for the simultaneous determination of deoxynivalenol, zearalenone, T-2-toxin and some masked metabolites in different cereals and cereal-derived food. Food Addit. Contam. Part A 29, 819–835. doi: 10.1080/19440049.2012.656707
de Mendiburu, F. (2014). Agricolae: Statistical Procedures for Agricultural Research. R Package Version, 1.
De Silva, N. I., Brooks, S., Lumyong, S., and Hyde, K. D. (2019). Use of endophytes as biocontrol agents. Fungal Biol. Rev. 33, 133–148. doi: 10.1016/j.fbr.2018.10.001
De Zutter, N., Audenaert, K., Ameye, M., De Boevre, M., De Saeger, S., Haesaert, G., et al. (2017). The plant response induced in wheat ears by a combined attack of sitobion avenae aphids and Fusarium graminearum boosts fungal infection and deoxynivalenol production. Mol. Plant Pathol. 18, 98–109. doi: 10.1111/mpp.12386
del Barrio-Duque, A., Samad, A., Nybroe, O., Antonielli, L., Sessitsch, A., and Compant, S. (2020). Interaction between endophytic Proteobacteria strains and Serendipita indica enhances biocontrol activity against fungal pathogens. Plant Soil 451, 277–305. doi: 10.1007/s11104-020-04512-5
Demissie, Z. A., Witte, T., Robinson, K. A., Sproule, A., Foote, S. J., Johnston, A., et al. (2020). Transcriptomic and exometabolomic profiling reveals antagonistic and defensive modes of Clonostachys rosea action against Fusarium graminearum. Mol. Plant Microbe Interact. 33, 842–858. doi: 10.1094/mpmi-11-19-0310-r
Desomer, J., Dhaese, P., and Van Montagu, M. (1988). Conjugative transfer of cadmium resistance plasmids in Rhodococcus fascians strains. J. Bacteriol. 170, 2401–2405. doi: 10.1128/jb.170.5.2401-2405.1988
Dueholm, M. S., Albertsen, M., D’Imperio, S., Tale, V. P., Lewis, D., Nielsen, P. H., et al. (2014). Complete genome of Rhodococcus pyridinivorans SB3094, a methyl-ethyl-ketone-degrading bacterium used for bioaugmentation. Genome Announc. 2:e00525-14.
Feng, B., Dong, Z., Xu, Z., An, X., Qin, H., Wu, N., et al. (2010). Molecular analysis of lipoxygenase (LOX) genes in common wheat and phylogenetic investigation of LOX proteins from model and crop plants. J. Cereal. Sci. 52, 387–394. doi: 10.1016/j.jcs.2010.06.019
Francis, I. M., Jourdan, S., Fanara, S., Loria, R., and Rigali, S. (2015). The cellobiose sensor CebR is the gatekeeper of Streptomyces scabies pathogenicity. MBio 6:e02018-14.
Gao, X., Brodhagen, M., Isakeit, T., Brown, S. H., Göbel, C., Betran, J., et al. (2009). Inactivation of the lipoxygenase ZmLOX3 increases susceptibility of maize to Aspergillus spp. Mol. Plant Microbe Interact. 22, 222–231. doi: 10.1094/mpmi-22-2-0222
Gardiner, D. M., Kazan, K., and Manners, J. M. (2009). Nutrient profiling reveals potent inducers of trichothecene biosynthesis in Fusarium graminearum. Fungal Genet. Biol. 46, 604–613. doi: 10.1016/j.fgb.2009.04.004
Harkai, P., Szabó, I., Cserháti, M., Krifaton, C., Risa, A., Radó, J., et al. (2016). Biodegradation of aflatoxin-B1 and zearalenone by Streptomyces sp. collection. Int. Biodeter. Biodegradation 108, 48–56. doi: 10.1016/j.ibiod.2015.12.007
Ioos, R., Belhadj, A., Menez, M., and Faure, A. (2005). The effects of fungicides on Fusarium spp. and Microdochium nivale and their associated trichothecene mycotoxins in French naturally-infected cereal grains. Crop Prot. 24, 894–902. doi: 10.1016/j.cropro.2005.01.014
Juroszek, P., and von Tiedemann, A. (2013). Climate change and potential future risks through wheat diseases: a review. Eur. J. Plant Pathol. 136, 21–33. doi: 10.1007/s10658-012-0144-9
Kämpfer, P., Dott, W., Martin, K., and Glaeser, S. (2014). Rhodococcus defluvii sp. nov., isolated from wastewater of a bioreactor and formal proposal to reclassify [Corynebacteriumhoagii] and Rhodococcus equi as Rhodococcus hoagii comb. nov. Int. J. Syst. Evol. Microbiol. 64, 755–761. doi: 10.1099/ijs.0.053322-0
Khan, H., McDonald, M. C., Williams, S. J., and Solomon, P. S. (2020). Assessing the efficacy of CRISPR/Cas9 genome editing in the wheat pathogen Parastagonspora nodorum. Fungal Biol. Biotechnol. 7, 1–8.
Kluger, B., Bueschl, C., Lemmens, M., Michlmayr, H., Malachova, A., Koutnik, A., et al. (2015). Biotransformation of the mycotoxin deoxynivalenol in Fusarium resistant and susceptible near isogenic wheat lines. PLoS One 10:e0119656. doi: 10.1371/journal.pone.0119656
Kurth, F., Mailänder, S., Bönn, M., Feldhahn, L., Herrmann, S., Große, I., et al. (2014). Streptomyces-induced resistance against oak powdery mildew involves host plant responses in defense, photosynthesis, and secondary metabolism pathways. Mol. Plant Microbe Int. 27, 891–900. doi: 10.1094/mpmi-10-13-0296-r
Lamichhane, J. R., and Venturi, V. (2015). Synergisms between microbial pathogens in plant disease complexes: a growing trend. Front. Plant Sci. 6:385. doi: 10.3389/fpls.2015.00385
Mahmoud, A. F. (2016). Genetic variation and biological control of Fusarium graminearum isolated from wheat in Assiut-Egypt. Plant Pathol. J. 32, 145. doi: 10.5423/ppj.oa.09.2015.0201
McLeod, M. P., Warren, R. L., Hsiao, W. W., Araki, N., Myhre, M., Fernandes, C., et al. (2006). The complete genome of Rhodococcus sp. RHA1 provides insights into a catabolic powerhouse. Proc. Natl. Acad. Sci. U.S.A. 103, 15582–15587. doi: 10.1073/pnas.0607048103
Nalam, V. J., Alam, S., Keereetaweep, J., Venables, B., Burdan, D., Lee, H., et al. (2015). Facilitation of Fusarium graminearum infection by 9-lipoxygenases in Arabidopsis and wheat. Mol. Plant Microbe Interact. 28, 1142–1152. doi: 10.1094/mpmi-04-15-0096-r
Newitt, J. T., Prudence, S. M., Hutchings, M. I., and Worsley, S. F. (2019). Biocontrol of cereal crop diseases using streptomycetes. Pathogens 8:78. doi: 10.3390/pathogens8020078
Nobili, C., D’Angeli, S., Altamura, M. M., Scala, V., Fabbri, A. A., Reverberi, M., et al. (2014). ROS and 9-oxylipins are correlated with deoxynivalenol accumulation in the germinating caryopses of Triticum aestivum after Fusarium graminearum infection. Eur. J. Plant Pathol. 139, 429–444. doi: 10.1007/s10658-014-0401-1
Pachebat, J. A., van Keulen, G., Whitten, M. M., Girdwood, S., Del Sol, R., Dyson, P. J., et al. (2013). Draft genome sequence of Rhodococcus rhodnii strain LMG5362, a symbiont of Rhodnius prolixus (hemiptera, reduviidae, triatominae), the principle vector of Trypanosoma cruzi. Genome Announc. 1:e00329-13. doi: 10.1128/genomeA.00329-13
Palaniyandi, S. A., Yang, S. H., Zhang, L., and Suh, J.-W. (2013). Effects of actinobacteria on plant disease suppression and growth promotion. Appl. Microbiol. Biotechnol. 97, 9621–9636. doi: 10.1007/s00253-013-5206-1
Palazzini, J. M., Ramirez, M. L., Torres, A. M., and Chulze, S. N. (2007). Potential biocontrol agents for Fusarium head blight and deoxynivalenol production in wheat. Crop Prot. 26, 1702–1710. doi: 10.1016/j.cropro.2007.03.004
Palazzini, J. M., Yerkovich, N., Alberione, E., Chiotta, M., and Chulze, S. N. (2017). Reprint of “an integrated dual strategy to control Fusarium graminearum sensu stricto by the biocontrol agent Streptomyces sp. RC 87B under field conditions”. Plant Gene 11, 2–7. doi: 10.1016/j.plgene.2017.07.002
Parry, D., Jenkinson, P., and McLeod, L. (1995). Fusarium ear blight (scab) in small grain cereals—a review. Plant Pathol. 44, 207–238. doi: 10.1111/j.1365-3059.1995.tb02773.x
Quan, S., and Dabbs, E. R. (1993). Nocardioform arsenic resistance plasmid characterization and improved Rhodococcus cloning vectors. Plasmid 29, 74–79. doi: 10.1006/plas.1993.1010
Raymaekers, K., Ponet, L., Holtappels, D., Berckmans, B., and Cammue, B. P. (2020). Screening for novel biocontrol agents applicable in plant disease management–a review. Biol. Control 144:104240. doi: 10.1016/j.biocontrol.2020.104240
RCoreTeam (2019). R: A Language and Environment for Statistical Computing. Vienna: R Foundation for Statistical Computing.
Robert-Seilaniantz, A., Grant, M., and Jones, J. D. (2011). Hormone crosstalk in plant disease and defense: more than just jasmonate-salicylate antagonism. Ann. Rev. Phytopathol. 49, 317–343. doi: 10.1146/annurev-phyto-073009-114447
Sarrocco, S., Esteban, P., Vicente, I., Bernardi, R., Plainchamp, T., Domenichini, S., et al. (2020). Straw competition and wheat root enophytism of Trichoderma gamsii T6085 as useful traits in the biocontrol of Fusarium head blight. Phytopathology. doi: 10.1094/PHYTO-09-20-0441-R
Seifi, H. S., Van Bockhaven, J., Angenon, G., and Höfte, M. (2013). Glutamate metabolism in plant disease and defense: friend or foe? Mol. Plant Microbe Interact. 26, 475–485. doi: 10.1094/mpmi-07-12-0176-cr
Spanjer, M. C., Rensen, P. M., and Scholten, J. M. (2008). LC–MS/MS multi-method for mycotoxins after single extraction, with validation data for peanut, pistachio, wheat, maize, cornflakes, raisins and figs. Food Addit. and Contam. 25, 472–489. doi: 10.1080/02652030701552964
Stack, R. W., and McMullen, M. P. (1985). Head blighting potential of Fusarium species associated with spring wheat heads. C. J. Plant Pathol. 7, 79–82. doi: 10.1080/07060668509501519
Stamler, R. A., Kilcrease, J., Kallsen, C., Fichtner, E. J., Cooke, P., Heerema, R. J., et al. (2015). First report of Rhodococcus isolates causing pistachio bushy top syndrome on ‘UCB-1’rootstock in California and Arizona. Plant Dis. 99, 1468–1476. doi: 10.1094/pdis-12-14-1340-re
Stecker, C., Johann, A., Herzberg, C., Averhoff, B., and Gottschalk, G. (2003). Complete nucleotide sequence and genetic organization of the 210-kilobase linear plasmid of Rhodococcus erythropolis BD2. J. Bacteriol. 185, 5269–5274. doi: 10.1128/jb.185.17.5269-5274.2003
Tan, J., Ameye, M., Landschoot, S., De Zutter, N., De Saeger, S., De Boevre, M., et al. (2020). At the scene of the crime: new insights into the role of weakly pathogenic members of the Fusarium head blight disease complex. Mol. Plant Pathol. 21, 1559–1572. doi: 10.1111/mpp.12996
Tini, F., Beccari, G., Onofri, A., Ciavatta, E., Gardiner, D. M., and Covarelli, L. (2020). Fungicides may have differential efficacies towards the main causal agents of Fusarium head blight of wheat. Pest Manag. Sci. 76, 3738–3748. doi: 10.1002/ps.5923
Trail, F., and Common, R. (2000). Perithecial development by Gibberella zeae: a light microscopy study. Mycologia 92, 130–138. doi: 10.2307/3761457
Van der Meij, A., Willemse, J., Schneijderberg, M. A., Geurts, R., Raaijmakers, J. M., and van Wezel, G. P. (2018). Inter-and intracellular colonization of Arabidopsis roots by endophytic actinobacteria and the impact of plant hormones on their antimicrobial activity. Antonie Van Leeuwenhoek 111, 679–690. doi: 10.1007/s10482-018-1014-z
Vandecasteele, M., Landschoot, S., Carrette, J., Verwaeren, J., Höfte, M., Audenaert, K., et al. (2018). Species prevalence and disease progression studies demonstrate a seasonal shift in the Alternaria population composition on potato. Plant Pathol. 67, 327–336. doi: 10.1111/ppa.12734
Vanheule, A., Audenaert, K., Warris, S., van de Geest, H., Schijlen, E., Höfte, M., et al. (2016). Living apart together: crosstalk between the core and supernumerary genomes in a fungal plant pathogen. BMC Genomics 17:670. doi: 10.1186/s12864-016-2941-6
Vaughan, M., Backhouse, D., and Del Ponte, E. M. (2016). Climate change impacts on the ecology of Fusarium graminearum species complex and susceptibility of wheat to Fusarium head blight: a review. World Mycotoxin J. 9, 685–700. doi: 10.3920/wmj2016.2053
Vergnes, S., Gayrard, D., Veyssière, M., Toulotte, J., Martinez, Y., Dumont, V., et al. (2020). Phyllosphere colonization by a soil Streptomyces sp. promotes plant defense responses against fungal infection. Mol. Plant Microbe Interact. 33, 223–234. doi: 10.1094/mpmi-05-19-0142-r
Wang, L. Y., Xie, Y. S., Cui, Y. Y., Xu, J., He, W., Chen, H. G., et al. (2015). Conjunctively screening of biocontrol agents (BCAs) against fusarium root rot and fusarium head blight caused by Fusarium graminearum. Microbiol. Res. 177, 34–42. doi: 10.1016/j.micres.2015.05.005
Xu, X., and Nicholson, P. (2009). Community ecology of fungal pathogens cusing wheat head blight. Ann. Rev. Phytopathol. 47, 83–103. doi: 10.1146/annurev-phyto-080508-081737
Xu, X.-M., Parry, D., Nicholson, P., Thomsett, M., Simpson, D., Edwards, S. G., et al. (2005). Predominance and association of pathogenic fungi causing Fusarium ear blight in wheat in four European countries. Eur. J. Plant Pathol. 112, 143–154. doi: 10.1007/s10658-005-2446-7
Xue, A. G., Chen, Y., Voldeng, H. D., Fedak, G., Savard, M. E., Längle, T., et al. (2014). Concentration and cultivar effects on efficacy of CLO-1 biofungicide in controlling Fusarium head blight of wheat. Biol. Control 73, 2–7. doi: 10.1016/j.biocontrol.2014.02.010
Yli-Mattila, T. (2010). Ecology and evolution of toxigenic Fusarium species in cereals in northern Europe and Asia. J. Plant Pathol. 92, 7–18.
Zadoks, J. C., Chang, T. T., and Konzak, C. F. (1974). A decimal code for the growth stages of cereals. Weed Res. 14, 415–421. doi: 10.1111/j.1365-3180.1974.tb01084.x
Zhang, S., Schisler, D. A., Boehm, M. J., and Slininger, P. J. (2007). Utilization of chemical inducers of resistance and Cryptococcus flavescens OH 182.9 to reduce Fusarium head blight under greenhouse conditions. Biol. Control 42, 308–315. doi: 10.1016/j.biocontrol.2007.05.020
Keywords: Fusarium, actinobacteria, pathobiome, biocontrol, multispectral imaging
Citation: Tan J, De Zutter N, De Saeger S, De Boevre M, Tran TM, van der Lee T, Waalwijk C, Willems A, Vandamme P, Ameye M and Audenaert K (2021) Presence of the Weakly Pathogenic Fusarium poae in the Fusarium Head Blight Disease Complex Hampers Biocontrol and Chemical Control of the Virulent Fusarium graminearum Pathogen. Front. Plant Sci. 12:641890. doi: 10.3389/fpls.2021.641890
Received: 15 December 2020; Accepted: 28 January 2021;
Published: 17 February 2021.
Edited by:
Christina Cowger, Plant Science Research Unit, Agricultural Research Service, United States Department of Agriculture, United StatesReviewed by:
Martha Marie Vaughan, United States Department of Agriculture (USDA), United StatesSabrina Sarrocco, University of Pisa, Italy
Copyright © 2021 Tan, De Zutter, De Saeger, De Boevre, Tran, van der Lee, Waalwijk, Willems, Vandamme, Ameye and Audenaert. This is an open-access article distributed under the terms of the Creative Commons Attribution License (CC BY). The use, distribution or reproduction in other forums is permitted, provided the original author(s) and the copyright owner(s) are credited and that the original publication in this journal is cited, in accordance with accepted academic practice. No use, distribution or reproduction is permitted which does not comply with these terms.
*Correspondence: Kris Audenaert, Kris.Audenaert@ugent.be; Maarten Ameye, maarten.ameye@ugent.be
†These authors have contributed equally to this work and share senior authorship