- 1Institut Sophia Agrobiotech, INRAE, CNRS, Université Côte d’Azur, Sophia Antipolis, France
- 2Graduate School of Science and Technology, Kumamoto University, Kumamoto, Japan
- 3Department of Plant Pathology and Key Laboratory of Pest Monitoring and Green Management of the Ministry of Agriculture, China Agricultural University, Beijing, China
Root-knot nematodes are obligate endoparasites that maintain a biotrophic relationship with their hosts over a period of several weeks. They induce the differentiation of root cells into specialized multinucleate hypertrophied feeding cells known as giant cells. Nematode effectors synthesized in the esophageal glands and injected into the plant tissue through the syringe-like stylet play a key role in giant cell ontogenesis. The Meloidogyne incognita MiEFF1 is one of the rare effectors of phytopathogenic nematodes to have been located in vivo in feeding cells. This effector specifically targets the giant cell nuclei. We investigated the Arabidopsis functions modulated by this effector, by using a yeast two-hybrid approach to identify its host targets. We characterized a universal stress protein (USP) and cytosolic glyceraldehyde-3-phosphate dehydrogenases (GAPCs) as the targets of MiEFF1. We validated the interaction of MiEFF1 with these host targets in the plant cell nucleus, by bimolecular fluorescence complementation (BiFC). A functional analysis with Arabidopsis GUS reporter lines and knockout mutant lines showed that GAPCs were induced in giant cells and that their non-metabolic functions were required for root-knot nematode infection. These susceptibility factors are potentially interesting targets for the development of new root-knot nematode control strategies.
Introduction
Root-knot nematodes (RKNs), Meloidogyne spp., are extremely polyphagous biotrophic plant parasites with a worldwide distribution. These microscopic worms cause dramatic root deformations, known as galls or root knots, which decrease crop yields, resulting in considerable economic losses (Abad et al., 2008; Jones et al., 2013). RKN are obligate parasites with a life cycle of 3–8 weeks, depending on the nematode species and environmental conditions. They spend most of their active life within plant roots. Four juvenile stages in addition to egg-laying adult female line the success of their life cycle. The hatching second-stage juvenile (J2) in the soil are attracted by the plant root, burrow into the host root close to the growing tip, and migrate intercellular to reach the vascular cylinder. They establish and maintain permanent feeding cells in the host root to supply them with nutrients. Feeding cell formation requires a reprograming of the selected root vascular cells into specialized hypertrophied and hypermetabolic feeding cells. Successive nuclear divisions without cell division and isotropic growth lead to multinucleate feeding “giant cells” containing up to 100 endoreduplicated and hypertrophied nuclei (Favery et al., 2016). The induction of these giant cells, which are found only in RKN parasitism, is mediated by effector proteins secreted into the host tissues by the nematode (Truong et al., 2015; Nguyen et al., 2018; Vieira and Gleason, 2019). These effectors are produced principally in the nematode esophageal glands (one dorsal and two subventral glands) and are delivered to the plant via a syringe-like stylet. Some effectors may also be produced by other secretory organs such as the chemosensory amphids or the hypodermis (Zhao et al., 2019). Effectors may be secreted into the apoplast (i.e., the intercellular space) to facilitate root penetration, intracellular migration, and the suppression of host defenses or into the cytoplasm of the 5–7 vascular cells destined to become the giant cells. The effectors involved in giant cells neo-organogenesis and functioning may target different subcellular compartments and cellular host functions. Indeed, transcriptomic studies of the plant response to RKN infection have revealed that RKN can hijack several key host cellular processes, such as the cell cycle, phytohormone signaling, intercellular transport, and metabolism to favor parasitism (Caillaud et al., 2008; Gheysen and Mitchum, 2019; Favery et al., 2020).
Meloidogyne incognita secretes hundreds of effectors into host plants (Bellafiore et al., 2008; Wang et al., 2012). A role in parasitism has been demonstrated for very few of these effectors, and the target in the plant has been identified for only 10 of these proteins (Mejias et al., 2019, 2020; Zhao et al., 2020). RKN effectors often target multifunctional proteins regulating key biological processes and conserved in all eukaryotes. The known target proteins include annexins, which are involved in development and responses to the biotic and abiotic environment in plants (Baucher et al., 2012) and have been shown to interact with the M. incognita MiMIF-2 effector (Zhao et al., 2019). The M. chitwoodi Mc1194 effector targets a papain-like cysteine protease (PLCP; Davies et al., 2015). In plants, PCLPs are involved in physiological processes as diverse as seed germination, leaf senescence, abiotic stress responses, and immunity (Liu et al., 2018). Another example is provided by the PASSE-MURAILLE effector (MiPM), which has been shown to interact with the Arabidopsis thaliana CSN5 protein (Bournaud et al., 2018), a subunit of the COP9 signalosome (CSN), a multifunctional eukaryotic protein complex (Cope and Deshaies, 2003). Finally, the nuclear MiEFF18 targets the Arabidopsis core spliceosomal protein SmD1 (Mejias et al., 2020), which regulates pre-mRNA splicing and alternative splicing, thereby increasing the diversity of the giant cell proteome (Elvira-Matelot et al., 2016; Mejias et al., 2020).
We describe here the identification and functional analysis of the direct targets of M. incognita EFFECTOR 1 (MiEFF1), the first RKN effector shown to be secreted in planta and to target the nuclei of the feeding cells within the host giant cells (Jaouannet et al., 2012). MiEFF1 (Minc17998) is specifically expressed in the dorsal esophageal gland of parasitic juveniles. However, MiEFF1 displays no similarity to any of the sequences present in database and has no domain of known function other than a predicted nuclear localization signal (NLS). Using the yeast two-hybrid (Y2H) approach and in planta bimolecular fluorescence complementation (BiFC), we demonstrated that, in A. thaliana, MiEFF1 interacts with multifunctional cytoplasmic proteins, a universal stress protein (AtUSP) and cytosolic glyceraldehyde-3-phosphate dehydrogenases (AtGAPCs) displaying moonlighting or having alternative nuclear functions required for plant responses to abiotic and/or biotic stress (Zaffagnini et al., 2013; Chi et al., 2019). We also showed that the AtUSP and AtGAPC genes are induced upon RKN parasitism. Finally, we demonstrated that AtGAPCs are required for Arabidopsis susceptibility to M. incognita.
Materials and Methods
Plant Material and Growth Conditions
The Arabidopsis lines used for the experiments were from the Wassilewskija (WS-4) and Columbia (Col-0) genetic backgrounds. The usp mutants, the SALK_146059 mutant line, referred to here as usp.1, previously described by Jung et al. (2015) and SALK_071209, referred to here as usp.2, were purchased from the Arabidopsis Biological Resource Center (United States). The gapc1 (SALK_010839) and gapc2 (SALK_016539) mutant lines and the AtGAPC1 transcriptional reporter GUS line have been described elsewhere (Vescovi et al., 2013). The abp39 and abx27 mutants, harboring T-DNA insertions in AtGAPC1 and AtGAPC2, respectively, were obtained from the INRAE Versailles T-DNA insertion collection (Bechtold et al., 1993). Homozygous mutants were identified by PCR-based genotyping, and new lines were further analyzed by RT-PCR and RT-qPCR, with the primers listed in Supplementary Table 1. Seeds of wild-type A. thaliana, mutants, and transgenic lines were surface-sterilized and sown on Murashige and Skoog (Duchefa) agar plates (0.5 × MS salts, 1% sucrose, 0.8% agar, and pH 6.4) or on a mixture of soil and sand. Seeds were incubated at 4°C for 2 days, and the plates were then transferred to a growth chamber with an 8 h photoperiod at 21°C. For propagation and transformation, seedlings were transferred to a growth chamber with a 16 h photoperiod at 21°C. Nicotiana benthamiana plants were grown in soil, under a 16 h photoperiod, at 24°C.
Sequence Analysis and Alignment
The sequences of EFF1 orthologs were obtained from NCBI and Wormbase parasite. Protein sequences were aligned with the MAFFT tool on the EBI server.1
Plasmid Constructs
The M. incognita MiEFF1 (Minc17998; GenBank MW345915) and MiEFF18 (Minc18636; GenBank KX907770) coding sequences (CDS) lacking the signal peptide, the A. thaliana AtUSP (At3g53990), AtGAPC1 (At3g04120), AtGAPC2 (At1g13440), and AtSmD1b (At4g02840), the AtUSP and AtGAPC2 promoters, the SV40 T antigen and the murin P53 sequences were amplified by PCR with specific primers (Supplementary Table 1) and inserted into the pDON207 donor vector. The entry clones were recombined with pK7WGF2 (p35S:eGFP-GW), pK7WGR2 (p35S:RFP-GW), pKGWFS7 (GWpromoter:eGFP-GUS), BiFC vectors (pAM-35SS:GW-YFPc, pAM-35SS:GW-YFPn, pAM-35SS:YFPc-GW, and pAM-35SS:YFPn-GW) or Y2H vectors, pGBK-GW, pB27-GW, or pP6-GW (Karimi et al., 2002; Caillaud et al., 2009; Zhao et al., 2020), with Gateway technology (Invitrogen). All constructs were sequenced (GATC Biotech) and used to transform Agrobacterium tumefaciens strain GV3101 or Saccharomyces cerevisiae strains AH109, L40ΔGal4, or Y187.
Y2H Assay
The yeast-two hybrid (Y2H) screen was performed according to the instructions supplied with the BD Matchmaker Library Construction and Screening Kit (Clontech). The coding sequence of MiEFF1 was inserted into the bait vector pGBKT7, which was used to transform S. cerevisiae strain AH109. For screening, cells carrying the bait construct were cotransformed with the commercial “normalized A. thaliana universal P02403 cDNA library” constructed by Dualsystems Biotech (Switzerland), using RNAs extracted from different Arabidopsis tissues, mixed in equal quantities and covering >95% of Arabidopsis genes. Yeasts displaying an interaction were recovered on selective SD medium without leucine, tryptophan, histidine, and adenine (SD/−Leu-Trp-His-Ade). To further validate the interactions, the MiEFF1 CDS lacking the peptide signal was cloned into the bait pB27 vector and used to transform yeast strain L40ΔGal4. The whole sequences encoding the AtUSP, AtGAPC1, and AtGAPC2 were cloned into the pP6 prey vectors, which were used to transform yeast strain Y187. The pairwise mating experiments with Y187 (matα) and L40ΔGal4 (mata) yeast strains were performed as previously described (Zhao et al., 2020).
In planta Subcellular Localization and BiFC
Leaves from 3–4-week-old N. benthamiana plants were subjected to agroinfiltration with recombinant strains of A. tumefaciens containing GFP, RFP, or BiFC vectors, as described by Caillaud et al. (2009). Images were captured, 48 h after agroinfiltration, with an inverted confocal microscope (Zeiss LSM880) equipped with an argon and HeNe laser as the excitation source. Samples were excited at 488 nm for GFP/YFP and 543 nm for RFP. GFP or YFP emission was detected selectively with a 505–530 nm band-pass emission filter. We detected RFP fluorescence with a 560–615 nm band-pass emission filter.
RNA Methods
Total RNA was extracted from A. thaliana seedlings or hand-dissected root segments and galls 7 and 14 days post-infection (dpi) with TRIzol reagent (Invitrogen), according to the manufacturer’s instructions. We reverse-transcribed 1 μg DNAse-treated (Ambion) RNA with the Superscript IV reverse transcriptase (Invitrogen). Semi quantitative RT-PCR was performed to analyze transcript abundancy in wild-type Arabidopsis and in the various newly generated mutant lines, with the primers described in Supplementary Table 1. Transcripts of the constitutively expressed OXA1 gene (At5g62050) were amplified to check that the amounts of intact cDNA used were similar in RT-PCR experiments. RT-qPCR analyses were performed and analyzed as described by Mejias et al. (2020), with the primers described in Supplementary Table 1. OXA1 and UBP22 (AT5G10790) were used for the normalization of RT-qPCR data. Data were analyzed using the 2-∆∆Ct method and were presented as Log2 fold change or as Normalized Relative Quantity generated using qBase software (Hellemans et al., 2007). Three technical replicates for 2–3 independent biological experiments were performed.
Nematode Infection Assays
Meloidogyne incognita strain “Morelos” was multiplied in tomato (Solanum lycopersicum cv. “Saint Pierre”) grown in a growth chamber (25°C and 16 h photoperiod). Freshly hatched J2s were collected as previously described (Caillaud and Favery, 2016). Three-week-old Arabidopsis plants grown individually in small pots of soil/sand were inoculated with 150 M. incognita J2 larvae per plant. Roots were collected and weighted 6 weeks after infection, and the females laying egg masses stained with 4.5% eosin under a binocular microscope were counted. For each experiment, we used n = 17–25 plants per line. Four independent infection experiments were performed for each gapc mutant line, and one infection experiment was performed for each usp mutant line.
Transgenic GUS Reporter Lines and Histochemical Analysis
The AtGAPC1promoter::GUS construct was previously described (Vescovi et al., 2013), and the Arabidopsis line was a kind gift from Prof. Alex Costa. The AtGAPC2promoter::GUS and AtUSPpromoter::GUS constructs were generated and introduced in A. tumefaciens as described above. Arabidopsis thaliana plants were transformed with those constructs by the floral dip method (Clough and Bent, 1998). Homozygous T3 transformants were used for further analysis. Plants were inoculated with M. incognita as described above, and GUS activity was analyzed histochemically 7 and 21 days after inoculation with M. incognita, as previously described (Caillaud et al., 2009). Reporter GUS expression in galls was revealed following 2–16 h staining. Two to four independent biological experiments were performed.
Results
MiEFF1 Interacts With a Universal Stress Protein and Cytosolic GAPDHs in Arabidopsis
MiEFF1 is a pioneer protein of unknown function. Orthologs can be identified in the eight available RKNs genome sequences: M. incognita, M. javanica, M. arenaria (Blanc-Mathieu et al., 2017), M. hapla (Opperman et al., 2008), M. enterolobii (Koutsovoulos et al., 2020), M. floridensis (Lunt et al., 2014), M. luci (Susič et al., 2020), and M. graminicola (Phan et al., 2020; Supplementary Figure 1). EFF1 is, however, absent from other plant parasitic nematodes and other organisms. We searched for possible host targets of the MiEFF1 effector, by screening a normalized Arabidopsis prey Y2H library. Approximately 1.2 × 105 yeast cells transformed with the prey and bait vectors were obtained, and 48 clones carrying a potential target were selected on SD/−Leu-Trp-His-Ade. Bait vector sequencing identified 30 sequences in frame with the transcriptional activation domain of GAL4 (Figure 1A). These sequences include that for the universal stress protein AtUSP (AT3G53990), which was captured eight times. All the clones isolated carried the complete sequence of AtUSP encoding a 160-amino acid (aa) protein containing the USPA domain (PF00582). In addition, five sequences encoding a cytosolic glyceraldehyde 3-phosphate dehydrogenase (AtGAPC2, AT1G13440) were identified. AtGAPC2 is 338 aa protein containing a NADP binding domain (aa 6–156, PF00044) and a catalytic domain (aa 161–318, PF02800). Two proteins were captured twice: a Glycine Rich Protein (AtGRP19, AT5G07550) and the Fragile Histidine Triad (AT5G58240) that are not nuclear proteins, and thus are not expected to interact with the MiEFF1 in vivo (Figure 1A). Finally, 13 proteins were captured once, that were not retained for further analysis (Figure 1A). Thus, only AtUSP and AtGAPC2 proteins were considered as potential targets of MiEFF1 in A. thaliana. We were also interested in analyzing whether AtGAPC1 (encoded by At3g04120), which is 97.9% identical to AtGAPC2 (Vescovi et al., 2013), could also interact with MiEFF1. For the confirmation of interactions, full-length sequences of AtGAPC1, AtGAPC2, and AtUSP were inserted into prey vectors to test the interactions with MiEFF1 in a LexA-based Y2H assay. The growth of diploids on selective SD/−Leu-Trp-His medium confirmed that the interactions between MiEFF1 and the full-length AtUSP, AtGAPC2, and AtGAPC1 proteins occurred in yeast (Figure 1B). These results demonstrate that MiEFF1 interacts with AtUSP, AtGAPC1, and AtGAPC2 in yeast.
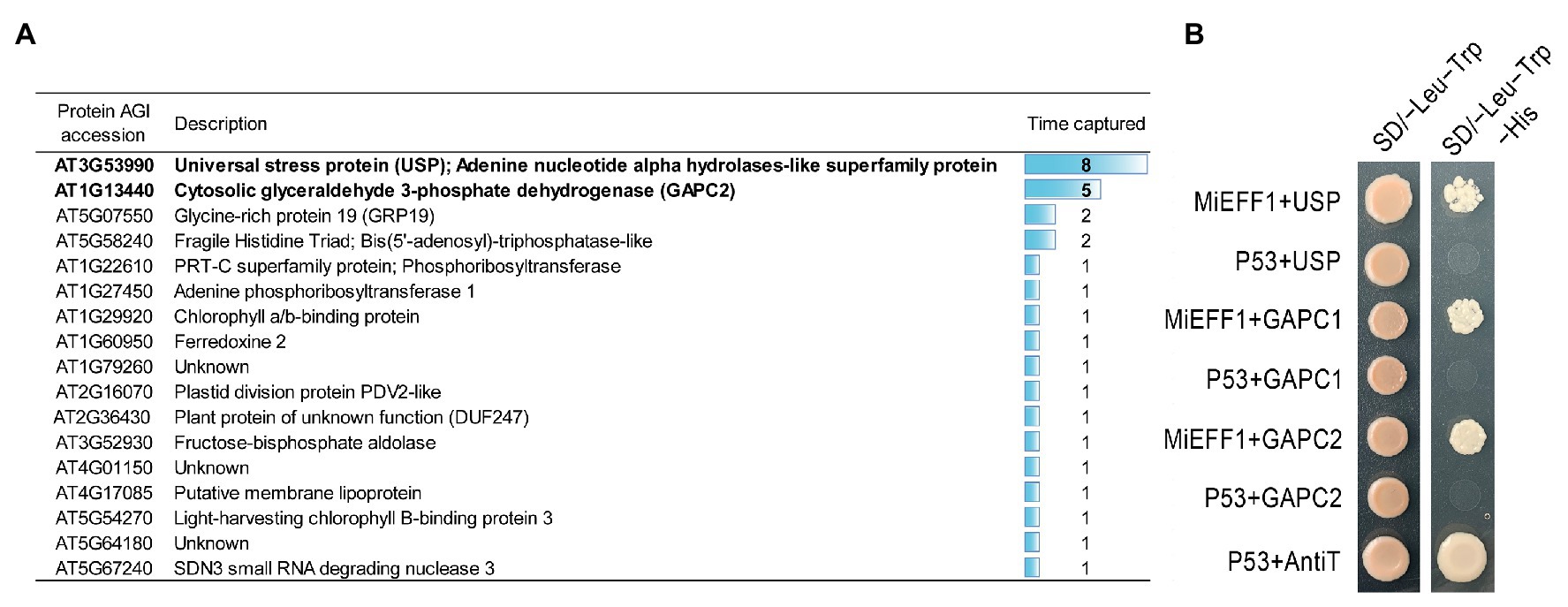
Figure 1. MiEFF1 interacts with AtUSP, AtGAPC1, and AtGAPC2 in yeast. (A) List of putative MiEFF1-interacting proteins from Arabidopsis obtained in our Y2H screen. (B) Yeasts containing the bait and prey plasmids carrying MiEFF1 and AtUSP, AtGAPC1, or AtGAPC2 and controls were spotted onto plates. SD/−Leu-Trp (Leu, leucine; Trp, tryptophane) is the non-selective medium without leucine and tryptophan. Only yeasts carrying a protein-protein interaction can survive on SD/−Leu-Trp-His (His, histidine) selective medium. Murine p53 and SV40 T antigen (anti T) were used as a positive control. Murine P53 and AtUSP, AtGAPC1, or AtGAPC2 were used as a negative control.
MiEFF1 Interacts With AtUSP, AtGAPC1, and AtGAPC2 in the Plant Cell Nucleus
We investigated whether MiEFF1 would be able to interact physically with AtUSP and AtGAPCs in plant cells, by determining the subcellular distributions of these proteins in planta. We expressed the AtUSP, AtGAPC1, and AtGAPC2 proteins, fused to GFP or RFP, in N. benthamiana epidermal leaf cells, by agroinfiltration. AtGAPC1, AtGAPC2, and AtUSP were localized to the cytoplasm and the nucleus of agroinfiltrated N. benthamiana epidermal leaf cells (Figure 2A), consistent with the results obtained in Arabidopsis stable transgenic lines or protoplasts (Vescovi et al., 2013; Schneider et al., 2018), and in Nicotiana tabacum agro-infiltrated leaves (Melencion et al., 2017). These results suggest that the nuclear MiEFF1 (Figure 2A), and its identified targets would easily be able to interact in the nucleus of plant cells. We then performed BiFC to check the interactions between Mi-EFF1 and the AtGAPCs and AtUSP in planta. The co-expression of MiEFF1-YFPc and YFPn-GAPCs or YFPn-USP fusion proteins reconstituted YFP fluorescence signals in the nucleus of agro-infiltrated N. benthamiana epidermal cells (Figure 2B). Analogous combinations with the nuclear effector MiEFF18 (Mejias et al., 2020) as negative controls resulted in no interaction with any of the identified targets of MiEFF1 (Figure 2B). Similarly, no interaction was observed between MiEFF1-YFPc and the previously described nuclear protein AtSmD1b (Mejias et al., 2020), fused to an N-terminal YFPn (Figure 2B). Various other constructs, resulting in fusions with YFPc or YFPn at the C- or N-terminus of the encoded protein, were used to check the interactions between MiEFF1 and the three interacting proteins identified; all gave results similar to those presented in Figure 2B (Supplementary Figure 2). These results confirm that a specific interaction occurs between MiEFF1 and AtUSP, AtGAPC1, and AtGAPC2 in plant cells.
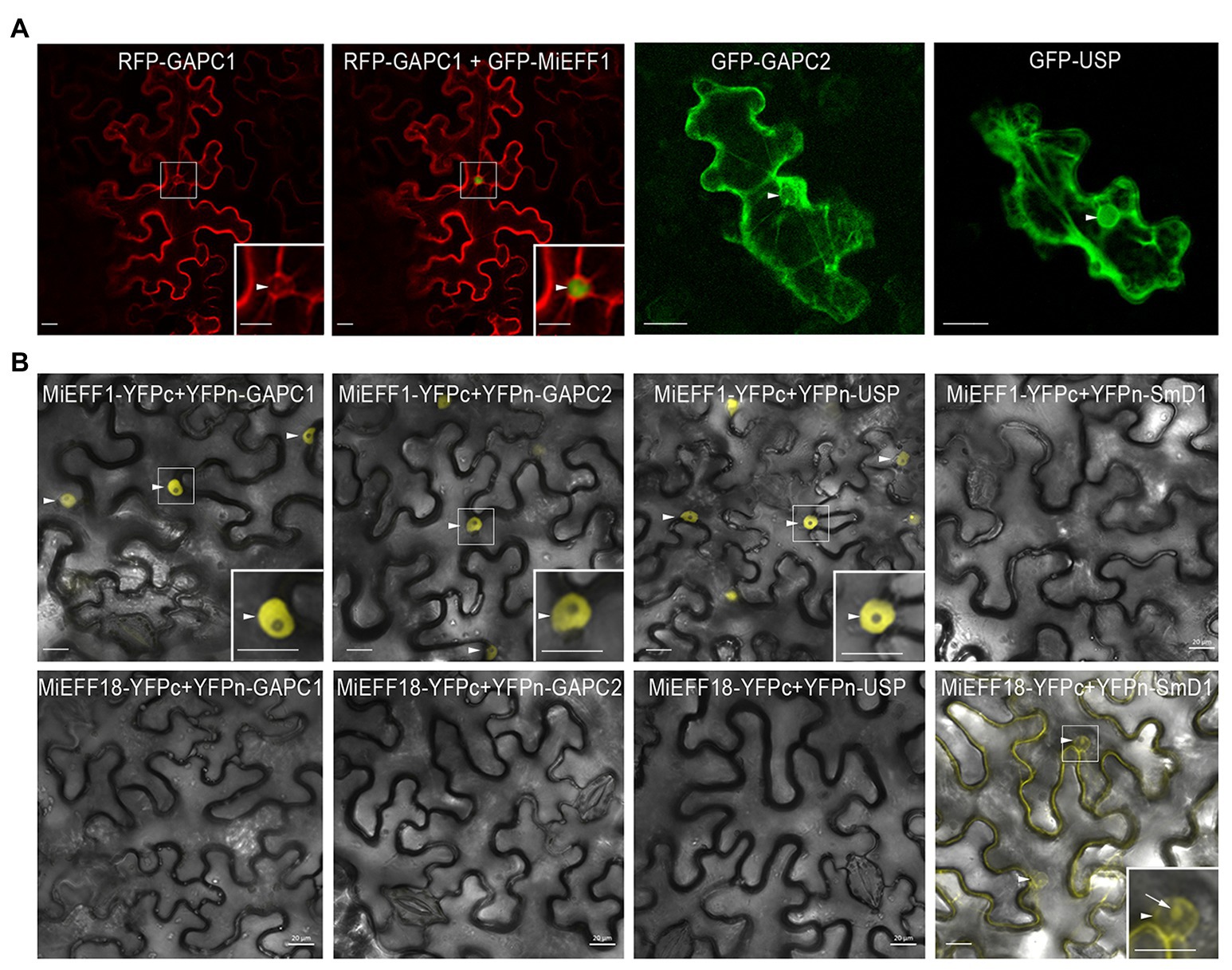
Figure 2. MiEFF1 interacts with AtUSP, AtGAPC1, and AtGAPC2 in planta. (A) Localization of GFP-AtUSP, -AtGAPC1, and -AtGAPC2 in Nicotiana benthamiana epidermal leaf cells. Enlargements of the area framed are shown. Arrowheads indicate nuclei. (B) MiEFF1 interacts with AtUSP, AtGAPC1, and AtGAPC2 in the nucleus in Nicotiana benthamiana cells. Confocal images of YFP fluorescence in bimolecular fluorescence complementation (BiFC) experiments with MiEFF1-YFPc and YFPn-GAPCs/USP fusion proteins expressed in Nicotiana benthamiana epidermal cells. MiEFF18 and AtSmD1b were used as controls. Three independent experiments were performed with similar results. Enlargements of the area framed are shown. Arrowheads indicate nuclei, arrow indicates nucleolus. Bars = 20 μm.
AtGAPC and AtUSP Accumulate in RKN-Induced Feeding Sites
We then investigated the expression of AtUSP, AtGAPC2, and AtGAPC1 in giant cells and galls, using published transcriptomic data (Yamaguchi et al., 2017). These RNAseq data showed induction of AtGAPC1 gene in galls at 3, 5, and 7 dpi (Figure 3A), suggesting a possible role for AtGAPC1 in the plant response to nematode infection. No accumulation of AtGAPC2 and AtUSP was observed in galls according to those RNAseq data (Figure 3A). The expression pattern of AtGAPC1, AtGAPC2 and AtUSP in galls, at 7 and 14 dpi, was further investigated using RT-qPCR. As shown in Figure 3A, AtGAPC1 and AtGAPC2 transcripts accumulated significantly in galls at 7 dpi. AtUSP expression increased slightly only at 14 dpi.
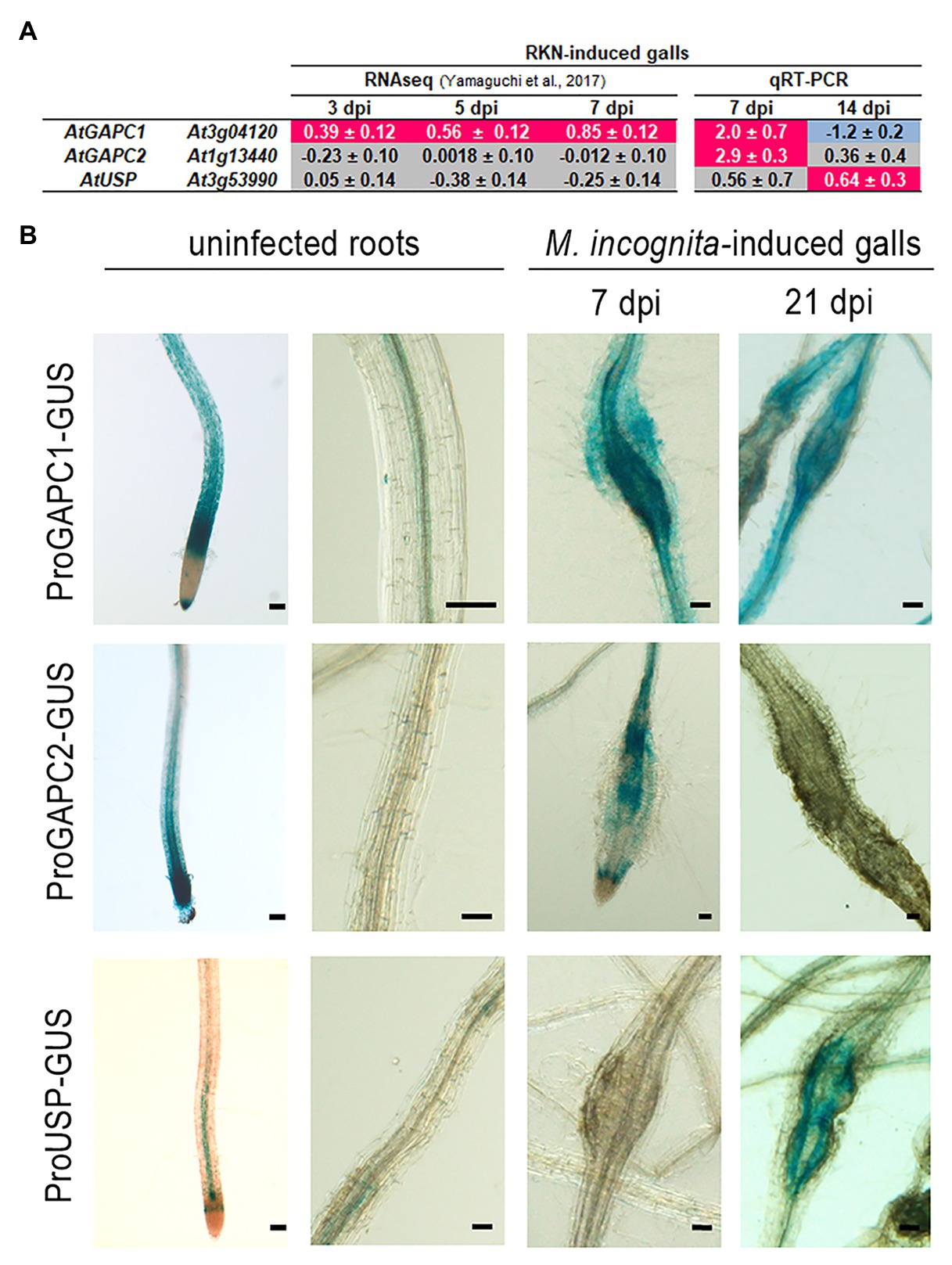
Figure 3. MiEFF1-interacting proteins accumulate in RKN-induced feeding sites. (A) Expression ratios of the AtUSP, AtGAPC1, and AtGAPC2 genes in galls vs. uninfected roots (log2) were obtained from RNAseq (Yamaguchi et al., 2017) data. Data presented are log2 fold changes ± standard deviation (SD) from three biological replicates. The mRNA expression level of these genes was further measured by quantitative real-time PCR (RT-qPCR) in galls at 7 and 14 days post M. incognita infection (dpi) and uninfected roots. Data were normalized against OXA1 and UBP22 as constitutive genes. Data presented are log2 fold changes ± SD from two biological replicates. dpi, days post-infection. Gray coloring indicates an absence of differential expression, a magenta coloring indicates an upregulation and a blue coloring a downregulation. (B) GUS expression in galls at 7 and 21 dpi, and uninfected roots, of plants transformed with AtGAPC1, AtGAPC2, and AtUSP promoter –GUS fusions. Reporter GUS expression in galls was revealed following 2 h (AtGAPC1promoter::GUS) or 16 h (AtGAPC2promoter::GUS and AtUSPpromoter::GUS) staining. Two to three independent experiments were performed with similar results. NI, not infected. Bars = 50 μm.
Arabidopsis transgenic lines carrying promoter::GUS constructs were used to analyze AtGAPC1, AtGAPC2, and AtUSP spatio-temporal expression upon M. incognita infection. As previously described (Vescovi et al., 2013), AtGAPC1 is expressed in roots of non-infected plants (Figure 3B; Supplementary Figures 3, 4). Consistent with our RT-qPCR data, the transgenic plants carrying the AtGAPC1promoter::GUS construct (Vescovi et al., 2013) displayed strong promoter activity in galls at 7 dpi and to a lesser extent at 21 dpi (Figure 3B). The AtGAPC2promoter::GUS construct was generated by cloning a 1,200 bp sequence immediately upstream from the translation initiation codon. Transgenic plants carrying the AtGAPC2promoter::GUS construct displayed promoter activity in non-inoculated root tips (Figure 3B; Supplementary Figures 3, 4). Induction of AtGAPC2 was observed in galls only at 7 dpi (Figure 3B), validating the transcriptomic data. No activity of the AtGAPC2 promoter was detected at 21 dpi. Moreover, an AtUSPpromoter::GUS construct was generated, harboring the 1,070 bp sequence immediately upstream from the translation initiation codon. The AtUSP promoter displayed activity in vascular tissues of non-inoculated root tips (Supplementary Figure 5). No GUS signal was detected in galls at 7 dpi, but a signal was observed in galls at 21 dpi (Figure 3B; Supplementary Figures 3, 4). Thus, these results showed that AtGAPC1 and AtGAPC2 transcripts accumulated significantly in galls at 7 dpi, while AtUSP is expressed at a later stage of the interaction. Altogether, these data support the hypothesis that MiEFF1-interacting proteins may be involved in plant responses to RKN parasitism.
AtGAPC1 and AtGAPC2 Are Involved in Plant Susceptibility to Root-Knot Nematodes
We further investigated the possible involvement of the proteins encoded by the AtUSP, AtGAPC1, and AtGAPC2 genes in the development and/or physiology of the giant cells induced by RKN, by performing infection tests with M. incognita in Arabidopsis loss-of-function mutant lines. A previously characterized usp.1 knockout (KO) line (SALK_146059; Jung et al., 2015) and a new usp.2 KO mutant line (SALK_071209; Supplementary Figure 5) carrying a T-DNA insertion in the third exon were challenged with M. incognita. We scored the number of females producing egg masses 6 weeks after infection. The test of these two alleles indicated no significant difference in the number of females producing egg masses between the usp mutants and Col-0 wild-type plants (Supplementary Figure 5). The two previously described gapc1 and gapc2 KO lines in the Col-0 genetic background (Vescovi et al., 2013; Figure 4A) were used here to investigate the role of AtGAPC genes in the plant-RKN interaction. We also selected two new mutant alleles in the WS genetic background, KO abp39 and knockdown abx27, carrying T-DNA insertions in the sixth exon of AtGAPC1, and in the AtGAPC2 promoter, respectively (Figures 4A,B; Supplementary Figure 6). These lines were inoculated with M. incognita juveniles. For all the lines tested, the number of females producing egg masses was smaller in the mutants than in wild-type plants: 19.4–94.0% fewer egg masses were found in atgapc1 mutants and 25.7–88.3% fewer in atgapc2 mutants (Figure 4C). A slight root developmental phenotype was observed in the gapc2 mutant, but not in the abx27 (Supplementary Figure 6), and both these gapc2 mutant lines exhibited a rather similar decreased susceptibility to RKN. For the two atgapc1 mutants, a significant and reproducible reductions in the egg mass number were observed over four independent experiments (Figure 4C), and this phenotype is not associated to a defect in root development (Supplementary Figure 6). Overall, these results demonstrate that AtGAPC genes play a role in Arabidopsis susceptibility to RKN.
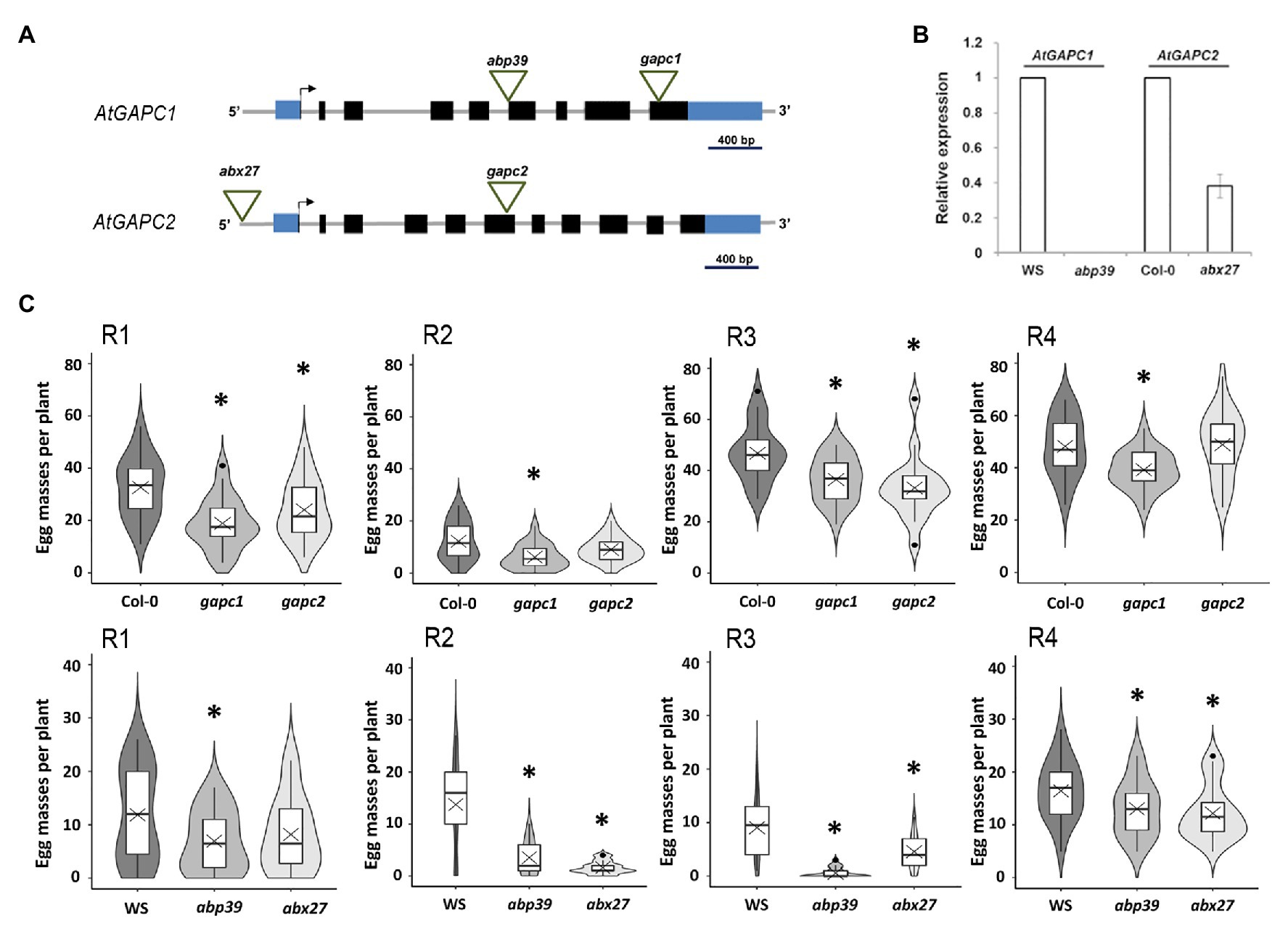
Figure 4. AtGAPC1 and AtGAPC2 are involved in Arabidopsis susceptibility to M. incognita. (A) Schematic illustration of the genomic organization of AtGAPC1 and AtGAPC2 and T-DNA insertion sites. Black boxes represent exons, gray lines correspond to introns, blue boxes represent untranslated sequences and the arrows represent START codons. (B) RT-qPCR analysis of AtGAPC1 and AtGAPC2 expression in abp39 and abx27 mutants. Data were normalized against OXA1 and UBP22 constitutive genes. (C) Results of four independent nematode infection assays, performed on Arabidopsis mutants AtGAPC1 (gapc1 and abp39) and AtGAPC2 (gapc2 and abx27), relative to wild-type plants (Col-0 and WS). Number of egg masses (y-axis), at 6 weeks post infection, in independent nematode infection assays, is shown as violin-plot diagrams. Box indicates interquartile range (25–75th percentile). The horizontal bar in the box indicates the median of the reported values. The crosses show the mean value. Whiskers mark the lowest and highest values within 1.5 times the interquartile range, and black dots indicate outliers. The box plot is included in a kernel density plot (shade of grays) showing the entire distribution of the data. R1–R4 indicate independent replicates. n = 17–25 plants per line. Asterisks indicate a significant difference between the wild-type and the mutant lines, as shown by Student’s t test (p < 0.05).
AtGAPC1 and AtGAPC2 Regulate Expression of Defense-Associated Genes
To investigate whether AtGAPC1 and AtGAPC2 manipulation by MiEFF1 could affect Arabidopsis immunity, we measured, using RT-qPCR, the expression of genes involved in antioxidative functions (AtCDS2) and abiotic (AtADH1 and AtSAP12) or biotic (AtPR1a, AtPDF1.2a, and AtPR4) stress responses (Zhao et al., 2020) in abp39 and abx27 mutants. In both mutants, AtPR1a and AtPDF1.2a, regulators of salicylic acid (SA)- and ethylene- and jasmonate (JA)-mediated defense responses, respectively, showed a strong constitutive expression, while AtPR4, encoding an ethylene-responsive PR protein, was repressed in both lines (Figure 5). The AtCDS2 gene encoding a Cu/Zn superoxide dismutase was also significantly induced in both mutants while the AtADH1, AtSAP12 encoding an alcohol dehydrogenase and a stress-associated protein, respectively, were not differentially expressed (Figure 5). These results indicate that targets of MiEFF1 are involved in regulating the expression of SA and JA defense-related genes in Arabidopsis.
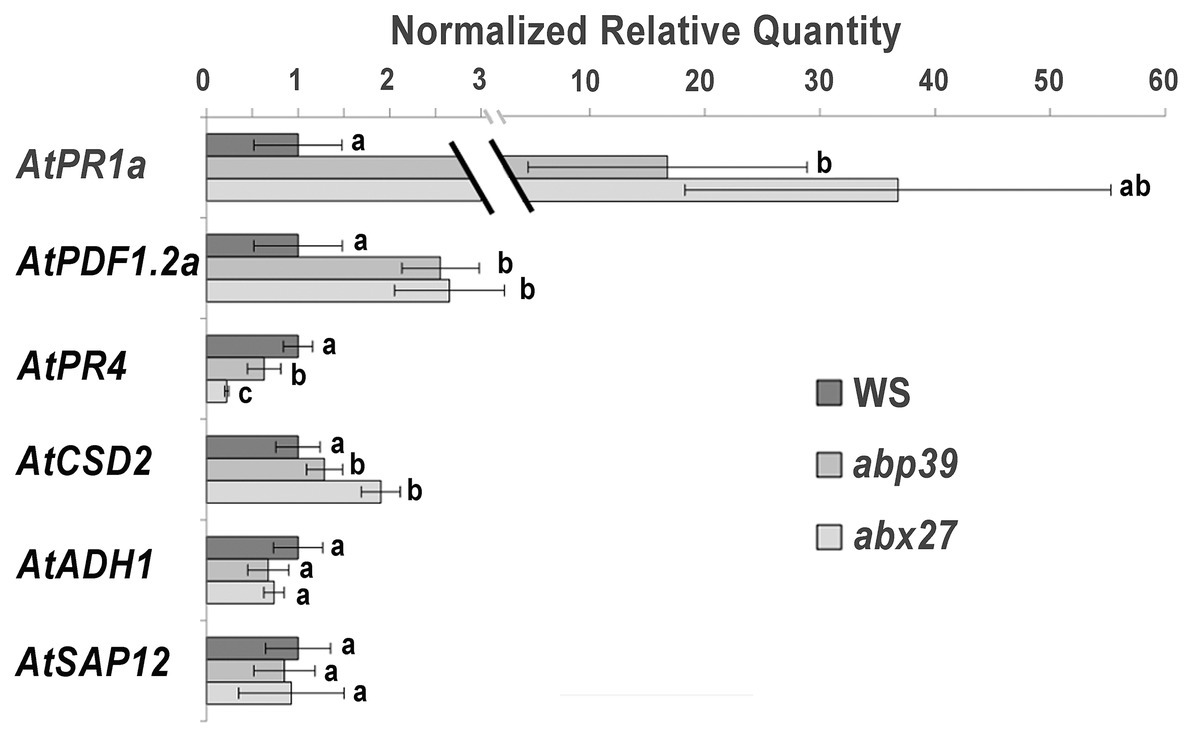
Figure 5. AtGAPC1 and AtGAPC2 regulate expression of defense-associated genes in Arabidopsis. RT-qPCR was used to investigate expression of defense- and stress-associates genes in abp39 and abx27 mutant seedlings. The genes considered were AtPR1a (At2g14610; salicylic acid (SA)-mediated defense response marker gene), AtPDF1.2a (At5g44420; encoding ethylene- and jasmonate-responsive plant defenses), AtPR4 (At3g04720; ethylene-responsive pathogenesis-related protein), AtCSD2 (At2g28190; chloroplastic Cu/Zn superoxide dismutase), AtADH1 (At1g77120; catalyzing the reduction of acetaldehyde with NADH as reductant), and AtSAP12 (At3g28210; stress-associated protein). AtOXA1 (AT5G62050) and AtUBP22 (AT5G10790) were used as internal controls. Data are presented as means ± standard deviation (SD) from three biological replicates. Different letters indicate statistically significant differences, *p < 0.05, Wilcoxon sign-rank test.
Discussion
Plant parasitic nematodes of the genus Meloidogyne have developed original and complex mechanisms of parasitism. By injecting proteins called as “effectors” into the host plant, they induce cellular reprograming and the transformation of root cells into hypertrophied polynucleate feeding cells known as “giant cells.” The development and maintenance of giant cells induced by RKN requires the manipulation of several host cellular processes (Favery et al., 2016). The nucleus is a key cellular compartment that must be targeted by the parasite (Quentin et al., 2013), and effectors, such as Mi16D10 and MiEFF18, have already been shown to target host transcription factors and the splicing machinery, respectively (Huang et al., 2006; Mejias et al., 2020). MiEFF1, a protein carrying a NLS, is produced in the parasitic RKN dorsal gland, secreted in planta and has been shown to accumulate in host giant cell nuclei during parasitism (Jaouannet et al., 2012). However, the function of MiEFF1 remained unknown. The identification of pathogen effector targets is now widely used as an approach to elucidating the molecular functions of these effectors (Khan et al., 2018; Mejias et al., 2019). Our yeast two-hybrid experiments identified three potential targets of the MiEFF1 nuclear effector in Arabidopsis: AtUSP, and two cytosolic GAPDHs, AtGAPC1 and AtGAPC2.
USPs were originally discovered in Escherichia coli and have been implicated in responses to various stress conditions in bacteria, archaea, plants, and some invertebrate animals (Vollmer and Bark, 2018). Plant USPs are multifunctional proteins involved in the development in addition to their role in responses to biotic and abiotic stresses (Chi et al., 2019). USPs proteins containing the E. coli universal stress protein A (USPA) domain and are encoded by members of a multigene family in Arabidopsis (Kerk et al., 2003; Chi et al., 2019). AtUSP (AT3G53990) has been implicated in plant responses to drought (Isokpehi et al., 2011), oxidative stress (Jung et al., 2015), and heat and cold stress (Jung et al., 2015; Melencion et al., 2017). A role in plant defense against pathogens was recently documented, with AtUSP accumulating in plants exposed to Pseudomonas syringae and displaying antimicrobial activity against various fungi (Park et al., 2017). We confirmed the presence of AtUSP in both the cytoplasm and the nucleus (Melencion et al., 2017), consistent with a possible dual function for this protein. In the cytoplasm, AtUSP acts as a protein chaperone in response to heat shock (Jung et al., 2015; Chi et al., 2019), whereas, in the nucleus, it may bind and protect nucleic acids, particularly RNA, enabling the plant to tolerate cold stress (Melencion et al., 2017; Chi et al., 2019). The induction of AtUSP upon RKN attack, at the end of giant cell formation, and its interaction with MiEFF1 within the plant cell nucleus suggest that it is the nuclear function of AtUSP in nucleic acid protection that is targeted by MiEFF1. Further investigation will be required, using multiple KO lines because of the redundancy of USPs in A. thaliana (Kerk et al., 2003), to conclude on AtUSP function in plant-RKN interaction.
Interestingly, we also found that nuclear MiEFF1 interacted with two cytosolic GAPDHs. Not only these proteins act as key enzymes in glycolysis, but are also well-known “moonlighting” (multifunctional) proteins with functions in several processes unrelated to metabolism in animal and plant cells, such as apoptosis, autophagy, gene expression regulation, and responses to abiotic or biotic stress (Sirover, 2011; Zaffagnini et al., 2013). This functional versatility is regulated, in part, by redox-based post-translational modifications that alter GAPDH catalytic activity and influence the subcellular distribution of the enzyme. Animals have a single isoform of GAPDH, with well-established moonlighting properties, whereas plants have multiple isoforms, the non-metabolic roles of which have yet to be discovered (Zaffagnini et al., 2013). Arabidopsis has four gene families encoding seven phosphorylating GAPDH isoenzymes and one non-phosphorylating GAPDH isoenzyme. Only AtGAPC1 and AtGAPC2 and the non-phosphorylating GAPDH (NP-GAPDH) are cytosolic. The other GAPDHs are located in other cellular compartments such as chloroplasts (Zaffagnini et al., 2013). Interestingly, the two cytoplasmic GAPCs have been shown to relocalize to the plant cell nucleus upon exposure to oxidative stresses, such as H2O2 (Schneider et al., 2018) or nitric oxide (Testard et al., 2016; Schneider et al., 2018), and following exposure to cadmium (Vescovi et al., 2013), salt stress (Wawer et al., 2010), or heat stress (Kim et al., 2020). Similarly, a strong accumulation of AtGAPC1 has been shown in the nucleus following the perception of flagellin during infection with P. syringae, and a mutation of AtGAPC1 renders Arabidopsis less susceptible to these pathogenic bacteria (Henry et al., 2015). The function of GAPCs in the nucleus remains unclear. They have been shown to interact with plant nucleic acids, suggesting a potential role of GAPCs in protecting nucleic acids during stress responses (Testard et al., 2016). GAPCs were recently shown to interact with the NF-YC10 transcription factor to promote the expression of heat-inducible genes and heat tolerance in Arabidopsis (Kim et al., 2020). We show here that AtGAPC1 and AtGAPC2 were induced during giant cell formation and that the corresponding proteins were targeted by the nuclear effector MiEFF1, suggesting that one of the moonlighting nuclear functions of these proteins is targeted by RKN. A stronger and faster response of AtGAPC1 was observed in response to M. incognita, in line with the response to biotic and abiotic stress previously described (Vescovi et al., 2013; Henry et al., 2015). We also found that GAPCs were important for parasitic success in RKNs, as shown for several bacterium-host and virus-host interactions (Han et al., 2015; Henry et al., 2015; Zeng et al., 2018). Finally, N. benthamiana GAPCs have been shown to be targeted by the citrus tristeza virus (CTV), via interaction with the viral p23, to facilitate the infectious cycle of the virus (Ruiz-Ruiz et al., 2018). Thus, GAPCs appear to be common targets of evolutionarily diverse plant-pathogens. Considering AtGAPC1 induction in galls, and susceptibility of gapc1 mutants to M. incognita, we postulate that AtGAPC1 assume most of GAPCs function required for disease establishment. GAPC2 would reinforce the action of GAPC1 at a key moment of giant cell formation. Previous studies showed GAPCs have multiple functions in the regulation of autophagy, hypersensitive response, and plant innate immunity, and described a role of GAPCs as negative regulators of plant defense in Arabidopsis (Henry et al., 2015), N. benthamiana (Han et al., 2015), or Manihot esculenta (Zeng et al., 2018). Microarray analysis on Arabidopsis gapc mutants showed that several genes encoding for enzymes regulating reactive oxygen species (ROS) homeostasis, such as peroxidases, catalases, or superoxide dismutases, had altered expression (Rius et al., 2008), and here, using a RT-qPCR approach, we demonstrated a differential expression of defense- and stress-associated genes in abp39 and abx27 mutants, both mutant lines showing strong constitutive expression of AtPR1a and AtPDF1.2a genes.
While several RKN effectors were shown to be responsible for host defense suppression (Quentin et al., 2013; Vieira and Gleason, 2019), very few have had their host target identified, i.e., MiMIF2 (Zhao et al., 2019), MiPDI1 (Zhao et al., 2020), Mg16820 (Naalden et al., 2018), MgMO237 (Chen et al., 2018), and MjTTL5 (Lin et al., 2016). This work adds MiEFF1 to the list of RKN effectors modulating host immunity and confirms that defense- and stress-related proteins are key targets of effectors, particularly those of nematodes, during disease establishment. These genes, particularly those encoding GAPCs, constitute attractive conserved candidates for targeting to reduce susceptibility in novel breeding strategies aiming to develop durable and broad-spectrum resistance (van Schie and Takken, 2014; Zaidi et al., 2018).
Data Availability Statement
The original contributions presented in the study are included in the article/Supplementary Material, further inquiries can be directed to the corresponding authors.
Author Contributions
NT designed and performed the experiments and interpreted the results. YC contributed to BiFC and Y2H experiments. JM contributed to subcellular localizations. SSo and KM performed the qRT-PCR analysis. MJ contributed to material and data analysis. NT, PA, BF, and MQ wrote the article. SJ-P, SSa, PA, BF, and MQ obtained the funding, designed the work, supervised the experiments, and data analyses. All authors contributed to the article and approved the submitted version.
Funding
NT was supported by a USTH fellowship, 911-USTH program, from the Ministry of Education and Training of The Socialist Republic of Vietnam, and received a Short Term Scientific Mission grant (STSM) from the COST action FA1208 (Pathogen-informed strategies for sustainable broad-spectrum crop resistance). YC got scholarships from China Scholarship Council (No. 201806350108) for studying at INRAE, France. JM benefited from a doctoral fellowship of the Ministère de lʼEnseignement Supérieur, de la Recherche et de lʼInnovation (MENRT grant). SSo was supported by a doctoral fellowship from INRAE SPE department and IDEX UCAJedi ANR-15-IDEX-01. This work was supported by the INRAE, the French Government (National Research Agency, ANR) through the programs LABEX SIGNALIFE ANR-11-LABX-0028 and IDEX UCAJedi ANR-15-IDEX-01, the French-Japanese bilateral collaboration programs (PHC SAKURA 2016 #35891VD and 2019 #43006VJ), and the French-Chinese bilateral collaboration program PHC XU GUANGQI 2020 #45478PF.
Conflict of Interest
The authors declare that the research was conducted in the absence of any commercial or financial relationships that could be construed as a potential conflict of interest.
The handling editor declared a past co-authorship with one of the authors PA.
Acknowledgments
We thank Prof. Alex Costa (Università di Milano, Italy) for the generous gift of the gapc1 and gapc2 mutants and the AtGAPC1promoter::GUS reporter line, Dr. Olivier Pierre for technical support, and Nathalie Marteu for producing nematodes. We also thank Hybrigenics Services (France) for providing the pB27 and pP6 vectors and the L40∆Gal4 and Y187 yeast strains. Microscopy work was performed at the PlantBIOs imaging facility of the Institut Sophia Agrobiotech.
Supplementary Material
The Supplementary Material for this article can be found online at: https://www.frontiersin.org/articles/10.3389/fpls.2021.641480/full#supplementary-material
Footnotes
References
Abad, P., Gouzy, J., Aury, J.-M., Castagnone-Sereno, P., Danchin, E. G. J., Deleury, E., et al. (2008). Genome sequence of the metazoan plant-parasitic nematode Meloidogyne incognita. Nat. Biotechnol. 26, 909–915. doi: 10.1038/nbt.1482
Baucher, M., Pérez-Morga, D., and El Jaziri, M. (2012). Insight into plant annexin function. From shoot to root signaling. Plant Signal. Behav. 7, 524–528. doi: 10.4161/psb.19647
Bechtold, N., Elis, J., and Pelletier, G. (1993). In planta agrobacterium mediated gene transfer by infiltration of adult Arabidopsis thaliana. C.R. Acad. Sci. 316, 1194–1199.
Bellafiore, S., Shen, Z., Rosso, M.-N., Abad, P., Shih, P., and Briggs, S. P. (2008). Direct identification of the Meloidogyne incognita secretome reveals proteins with host cell reprogramming potential. PLoS Pathog. 4:e1000192. doi: 10.1371/journal.ppat.1000192
Blanc-Mathieu, R., Perfus-Barbeoch, L., Aury, J.-M. M., Da Rocha, M., Gouzy, J., Sallet, E., et al. (2017). Hybridization and polyploidy enable genomic plasticity without sex in the most devastating plant-parasitic nematodes. PLoS Genet. 13:e1006777. doi: 10.1371/journal.pgen.1006777
Bournaud, C., Gillet, F.-X., Murad, A. M., Bresso, E., Albuquerque, E. V. S., and Grossi-de-Sá, M. F. (2018). Meloidogyne incognita PASSE-MURAILLE (MiPM) gene encodes a cell-penetrating protein that interacts with the CSN5 subunit of the COP9 signalosome. Front. Plant Sci. 9:904. doi: 10.3389/fpls.2018.00904
Caillaud, M.-C., Dubreuil, G., Quentin, M., and Favery, B. (2008). Root-knot nematodes manipulate plant cell functions during a compatible interaction. J. Plant Physiol. 165, 104–113. doi: 10.1016/j.jplph.2007.05.007
Caillaud, M. C., and Favery, B. (2016). In vivo imaging of microtubule organization in dividing giant cell. Methods Mol. Biol. 1370, 137–144. doi: 10.1007/978-1-4939-3142-2_11
Caillaud, M.-C., Paganelli, L., Lecomte, P., Deslandes, L., Quentin, M., Pecrix, Y., et al. (2009). Spindle assembly checkpoint protein dynamics reveal conserved and unsuspected roles in plant cell division. PLoS One 4:e6757. doi: 10.1371/journal.pone.0006757
Chen, J., Hu, L., Sun, L., Lin, B., Huang, K., Zhuo, K., et al. (2018). A novel Meloidogyne graminicola effector, MgMO237, interacts with multiple host defence-related proteins to manipulate plant basal immunity and promote parasitism. Mol. Plant Pathol. 19, 1942–1955. doi: 10.1111/mpp.12671
Chi, Y. H., Koo, S. S., Oh, H. T., Lee, E. S., Park, J. H., Phan, K. A. T., et al. (2019). The physiological functions of universal stress proteins and their molecular mechanism to protect plants from environmental stresses. Front. Plant Sci. 10:750. doi: 10.3389/fpls.2019.00750
Clough, S. J., and Bent, A. F. (1998). Floral dip: a simplified method for agrobacterium-mediated transformation of Arabidopsis thaliana. Plant J. 16, 735–743. doi: 10.1046/j.1365-313x.1998.00343.x
Cope, G. A., and Deshaies, R. J. (2003). COP9 signalosome: a multifunctional regulator of SCF and other cullin-based ubiquitin ligases. Cell 14, 663–671. doi: 10.1016/s0092-8674(03)00722-0
Davies, L. J., Zhang, L., and Elling, A. A. (2015). The Arabidopsis thaliana papain like cysteine protease RD21 interacts with a root-knot nematode effector protein. Nematology 17, 655–666. doi: 10.1163/15685411-00002897
Elvira-Matelot, E., Bardou, F., Ariel, F., Jauvion, V., Bouteiller, N., Le Masson, I., et al. (2016). The nuclear ribonucleoprotein SmD1 interplays with splicing, RNA quality control, and posttranscriptional gene silencing in Arabidopsis. Plant Cell 28, 426–438. doi: 10.1105/tpc.15.01045
Favery, B., Dubreuil, G., Chen, M.-S., Giron, D., and Abad, P. (2020). Gall-inducing parasites: convergent and conserved strategies of plant manipulation by insects and nematodes. Annu. Rev. Phytopathol. 58, 1–22. doi: 10.1146/annurev-phyto-010820-012722
Favery, B., Quentin, M., Jaubert-Possamai, S., and Abad, P. (2016). Gall-forming root-knot nematodes hijack key plant cellular functions to induce multinucleate and hypertrophied feeding cells. J. Insect Physiol. 84, 60–69. doi: 10.1016/j.jinsphys.2015.07.013
Gheysen, G., and Mitchum, M. G. (2019). Phytoparasitic nematode control of plant hormone pathways. Plant Physiol. 179, 1212–1226. doi: 10.1104/pp.18.01067
Han, S., Wang, Y., Zheng, X., Jia, Q., Zhao, J., Bai, F., et al. (2015). Cytoplastic glyceraldehyde-3-phosphate dehydrogenases interact with ATG3 to negatively regulate autophagy and immunity in Nicotiana benthamiana. Plant Cell 27, 1316–1331. doi: 10.1105/tpc.114.134692
Hellemans, J., Mortier, G., De Paepe, A., Speleman, F., and Vandesompele, J. (2007). qBase relative quantification framework and software for management and automated analysis of real-time quantitative PCR data. Genome Biol. 8, 1–14. doi: 10.1186/gb-2007-8-2-r19
Henry, E., Fung, N., Liu, J., Drakakaki, G., and Coaker, G. (2015). Beyond glycolysis: GAPDHs are multi-functional enzymes involved in regulation of ROS, autophagy, and plant immune responses. PLoS Genet. 11:e1005199. doi: 10.1371/journal.pgen.1005199
Huang, G., Dong, R., Allen, R., Davis, E. L., Baum, T. J., and Hussey, R. S. (2006). A root-knot nematode secretory peptide functions as a ligand for a plant transcription factor. Mol. Plant-Microbe Interact. 19, 463–470. doi: 10.1094/MPMI-19-0463
Isokpehi, R. D., Simmons, S. S., Cohly, H. H. P., Ekunwe, S. I. N., Begonia, G. B., and Ayensu, W. K. (2011). Identification of drought-responsive universal stress proteins in viridiplantae. Bioinform. Biol. Insights 5, 41–58. doi: 10.4137/BBI.S6061
Jaouannet, M., Perfus-Barbeoch, L., Deleury, E., Magliano, M., Engler, G., Vieira, P., et al. (2012). A root-knot nematode-secreted protein is injected into giant cells and targeted to the nuclei. New Phytol. 194, 924–931. doi: 10.1111/j.1469-8137.2012.04164.x
Jones, J. T., Haegeman, A., Danchin, E. G. J., Gaur, H. S., Helder, J., Jones, M. G. K., et al. (2013). Top 10 plant-parasitic nematodes in molecular plant pathology. Mol. Plant Pathol. 14, 946–961. doi: 10.1111/mpp.12057
Jung, Y. J., Melencion, S. M. B., Lee, E. S., Park, J. H., Alinapon, C. V., Oh, H. T., et al. (2015). Universal stress protein exhibits a redox-dependent chaperone function in arabidopsis and enhances plant tolerance to heat shock and oxidative stress. Front. Plant Sci. 6:1141. doi: 10.3389/fpls.2015.01141
Karimi, M., Inzé, D., and Depicker, A. (2002). GATEWAY® vectors for agrobacterium-mediated plant transformation. Trends Plant Sci. 7, 193–195. doi: 10.1016/S1360-1385(02)02251-3
Kerk, D., Bulgrien, J., Smith, D. W., and Gribskov, M. (2003). Arabidopsis proteins containing similarity to the universal stress protein domain of bacteria. Plant Physiol. 131, 1209–1219. doi: 10.1104/pp.102.016006
Khan, M., Seto, D., Subramaniam, R., and Desveaux, D. (2018). Oh, the places they’ll go ! A survey of phytopathogen effectors and their host targets. Plant J. 93, 651–663. doi: 10.1111/tpj.13780
Kim, S.-C., Guo, L., and Wang, X. (2020). Nuclear moonlighting of cytosolic glyceraldehyde-3-phosphate dehydrogenase regulates Arabidopsis response to heat stress. Nat. Commun. 11:3439. doi: 10.1038/s41467-020-17311-4
Koutsovoulos, G. D., Poullet, M., Elashry, A., Kozlowski, D. K. L., Sallet, E., Da Rocha, M., et al. (2020). Genome assembly and annotation of Meloidogyne enterolobii, an emerging parthenogenetic root-knot nematode. Sci. Data. 7:324. doi: 10.1038/s41597-020-00666-0
Lin, B., Zhuo, K., Chen, S., Hu, L., Sun, L., Wang, X., et al. (2016). A novel nematode effector suppresses plant immunity by activating host reactive oxygen species-scavenging system. New Phytol. 209, 1159–1173. doi: 10.1111/nph.13701
Liu, H., Hu, M., Wang, Q., Lin Cheng, L., and Zhang, Z. (2018). Role of papain-like cysteine proteases in plant development. Front. Plant Sci. 9:1717. doi: 10.3389/fpls.2018.01717
Lunt, D. H., Kumar, S., Koutsovoulos, G., and Blaxter, M. L. (2014). The complex hybrid origins of the root knot nematodes revealed through comparative genomics. PeerJ 2:e356. doi: 10.7717/peerj.356
Mejias, J., Bazin, J., Truong, N.-M., Chen, Y., Marteu, N., Bouteiller, N., et al. (2020). The root-knot nematode effector MiEFF18 interacts with the plant core spliceosomal protein SmD1 required for giant cell formation. New Phytol. 229, 3408–3423. doi: 10.1111/nph.17089
Mejias, J., Truong, N. M., Abad, P., Favery, B., and Quentin, M. (2019). Plant proteins and processes targeted by parasitic nematode effectors. Front. Plant Sci. 10:970. doi: 10.3389/fpls.2019.00970
Melencion, S. M. B., Chi, Y. H., Pham, T. T., Paeng, S. K., Wi, S. D., Lee, C., et al. (2017). RNA chaperone function of a universal stress protein in arabidopsis confers enhanced cold stress tolerance in plants. Int. J. Mol. Sci. 18:2546. doi: 10.3390/ijms18122546
Naalden, D., Haegeman, A., de Almeida-Engler, J., Birhane Eshetu, F., Bauters, L., and Gheysen, G. (2018). The Meloidogyne graminicola effector Mg16820 is secreted in the apoplast and cytoplasm to suppress plant host defense responses. Mol. Plant Pathol. 19, 2416–2430. doi: 10.1111/mpp.12719
Nguyen, C.-N., Perfus-Barbeoch, L., Quentin, M., Zhao, J., Magliano, M., Marteu, N., et al. (2018). A root-knot nematode small glycine and cysteine-rich secreted effector, MiSGCR1, is involved in plant parasitism. New Phytol. 217, 687–699. doi: 10.1111/nph.14837
Opperman, C. H., Bird, D. M., Williamson, V. M., Rokhsar, D. S., Burke, M., Cohn, J., et al. (2008). Sequence and genetic map of Meloidogyne hapla: a compact nematode genome for plant parasitism. Proc. Natl. Acad. Sci. U. S. A. 105, 14802–14807. doi: 10.1073/pnas.0805946105
Park, S. C., Jung, Y. J., Lee, Y., Kim, I. R., Seol, M. A., Kim, E. J., et al. (2017). Functional characterization of the Arabidopsis universal stress protein AtUSP with an antifungal activity. Biochem. Biophys. Res. Commun. 486, 923–929. doi: 10.1016/j.bbrc.2017.03.126
Phan, N. T., Orjuela, J., Danchin, E. G. J., Klopp, C., Perfus-Barbeoch, L., Kozlowski, D. K., et al. (2020). Genome structure and content of the rice root-knot nematode (Meloidogyne graminicola). Ecol. Evol. 10, 11006–11021. doi: 10.1002/ece3.6680
Quentin, M., Abad, P., and Favery, B. (2013). Plant parasitic nematode effectors target host defense and nuclear functionsto establish feeding cells. Front. Plant Sci. 4:53. doi: 10.3389/fpls.2013.00053
Rius, S. P., Casati, P., Iglesias, A. A., and Gomez-Casati, D. F. (2008). Characterization of Arabidopsis lines deficient in GAPC-1, a cytosolic NAD-dependent glyceraldehyde-3-phosphate dehydrogenase. Plant Physiol. 148, 1655–1667. doi: 10.1104/pp.108.128769
Ruiz-Ruiz, S., Spanò, R., Navarro, L., Moreno, P., Peña, L., and Flores, R. (2018). Citrus tristeza virus co-opts glyceraldehyde 3-phosphate dehydrogenase for its infectious cycle by interacting with the viral-encoded protein p23. Plant Mol. Biol. 98, 363–373. doi: 10.1007/s11103-018-0783-0
Schneider, M., Knuesting, J., Birkholz, O., Heinisch, J. J., and Scheibe, R. (2018). Cytosolic GAPDH as a redox-dependent regulator of energy metabolism. BMC Plant Biol. 18:184. doi: 10.1186/s12870-018-1390-6
Sirover, M. A. (2011). On the functional diversity of glyceraldehyde-3-phosphate dehydrogenase: biochemical mechanisms and regulatory control. Biochim. Biophys. Acta 1810, 741–751. doi: 10.1016/j.bbagen.2011.05.010
Susič, N., Koutsovoulos, G. D., Riccio, C., Danchin, E. G. J., Blaxter, M. L., Lunt, D. H., et al. (2020). Genome sequence of the root-knot nematode Meloidogyne luci. J. Nematol. 52, 1–5. doi: 10.21307/jofnem-2020-025
Testard, A., da Silva, D., Ormancey, M., Pichereaux, C., Pouzet, C., Jauneau, A., et al. (2016). Calcium and nitric oxide-dependent nuclear accumulation of cytosolic glyceraldehyde-3-phosphate dehydrogenase in response to long chain bases in tobacco BY-2 cells. Plant Cell Physiol. 57, 2221–2231. doi: 10.1093/pcp/pcw137
Truong, N. M., Nguyen, C.-N., Abad, P., Quentin, M., and Favery, B. (2015). Function of root-knot nematode effectors and their targets in plant parasitism. Adv. Bot. Res. 75, 293–324. doi: 10.1016/bs.abr.2014.12.010
van Schie, C. C. N., and Takken, F. L. W. (2014). Susceptibility genes 101: how to be a good host. Annu. Rev. Phytopathol. 52, 551–581. doi: 10.1146/annurev-phyto-102313-045854
Vescovi, M., Zaffagnini, M., Festa, M., Trost, P., Lo Schiavo, F., and Costa, A. (2013). Nuclear accumulation of cytosolic glyceraldehyde-3-phosphate dehydrogenase in cadmium-stressed arabidopsis roots. Plant Physiol. 162, 333–346. doi: 10.1104/pp.113.215194
Vieira, P., and Gleason, C. (2019). Plant-parasitic nematode effectors—insights into their diversity and new tools for their identification. Curr. Opin. Plant Biol. 50, 37–43. doi: 10.1016/j.pbi.2019.02.007
Vollmer, A. C., and Bark, S. J. (2018). Twenty-five years of investigating the universal stress protein: function, structure, and applications. Adv. Appl. Microbiol. 102, 1–36. doi: 10.1016/bs.aambs.2017.10.001
Wang, X. R., Moreno, Y. A., Wu, H. R., Ma, C., Li, Y. F., Zhang, J. A., et al. (2012). Proteomic profiles of soluble proteins from the esophageal gland in female Meloidogyne incognita. Int. J. Parasitol. 42, 1177–1183. doi: 10.1016/j.ijpara.2012.10.008
Wawer, I., Bucholc, M., Astier, J., Anielsaa-Mazur, A., Dahan, J., Kulik, A., et al. (2010). Regulation of Nicotiana tabacum osmotic stress-activated protein kinase and its cellular partner GAPDH by nitric oxide in response to salinity. Biochem. J. 429, 73–83. doi: 10.1042/BJ20100492
Yamaguchi, Y. L., Suzuki, R., Cabrera, J., Nakagami, S., Sagara, T., Ejima, C., et al. (2017). Root-knot and cyst nematodes activate procambium-associated genes in Arabidopsis roots. Front. Plant Sci. 8:1195. doi: 10.3389/fpls.2017.01195
Zaffagnini, M., Fermani, S., Costa, A., Lemaire, S. D., and Trost, P. (2013). Plant cytoplasmic GAPDH: redox post-translational modifications and moonlighting properties. Front. Plant Sci. 4:450. doi: 10.3389/fpls.2013.00450
Zaidi, S. S., Mukhtar, M. S., and Mansoor, S. (2018). Genome editing: targeting susceptibility genes for plant disease resistance. Trends Biotechnol. 36, 898–906. doi: 10.1016/j.tibtech.2018.04.005
Zeng, H., Xie, Y., Liu, G., Lin, D., He, C., and Shi, H. (2018). Molecular identification of GAPDHs in cassava highlights the antagonism of MeGAPCs and MeATG8s in plant disease resistance against cassava bacterial blight. Plant Mol. Biol. 97, 201–214. doi: 10.1007/s11103-018-0733-x
Zhao, J., Li, L., Liu, Q., Liu, P., Li, S., Yang, D., et al. (2019). A MIF-like effector suppresses plant immunity and facilitates nematode parasitism by interacting with plant annexins. J. Exp. Bot. 70, 5943–5958. doi: 10.1093/jxb/erz348
Keywords: root-knot nematode (Meloidogyne incognita), effector, giant cell, cytosolic glyceraldehyde-3-phosphate dehydrogenases, susceptibility gene
Citation: Truong NM, Chen Y, Mejias J, Soulé S, Mulet K, Jaouannet M, Jaubert-Possamai S, Sawa S, Abad P, Favery B and Quentin M (2021) The Meloidogyne incognita Nuclear Effector MiEFF1 Interacts With Arabidopsis Cytosolic Glyceraldehyde-3-Phosphate Dehydrogenases to Promote Parasitism. Front. Plant Sci. 12:641480. doi: 10.3389/fpls.2021.641480
Edited by:
Shahid Siddique, University of California, Davis, United StatesReviewed by:
Paulo Vieira, Virginia Tech, United StatesHenok Yimer, University of California, Davis, United States
Copyright © 2021 Truong, Chen, Mejias, Soulé, Mulet, Jaouannet, Jaubert-Possamai, Sawa, Abad, Favery and Quentin. This is an open-access article distributed under the terms of the Creative Commons Attribution License (CC BY). The use, distribution or reproduction in other forums is permitted, provided the original author(s) and the copyright owner(s) are credited and that the original publication in this journal is cited, in accordance with accepted academic practice. No use, distribution or reproduction is permitted which does not comply with these terms.
*Correspondence: Michaël Quentin, bWljaGFlbC5xdWVudGluQGlucmFlLmZy; Bruno Favery, YnJ1bm8uZmF2ZXJ5QGlucmFlLmZy