- 1Institute for Sustainable Agriculture, Spanish National Research Council (Consejo Superior de Investigaciones Científicas), Córdoba, Spain
- 2Institute for Mediterranean and Subtropical Horticulture “La Mayora” (IHSM-UMA-Consejo Superior de Investigaciones Científicas), Department of Botany and Plant Physiology, University of Malaga, Málaga, Spain
- 3Agronomy Department, University of Córdoba, Córdoba, Spain
Host resistance is the most practical, long-term, and economically efficient disease control measure for Verticillium wilt in olive caused by the xylem-invading fungus Verticillium dahliae (Vd), and it is at the core of the integrated disease management. Plant’s microbiome at the site of infection may have an influence on the host reaction to pathogens; however, the role of xylem microbial communities in the olive resistance to Vd has been overlooked and remains unexplored to date. This research was focused on elucidating whether in vitro olive propagation may alter the diversity and composition of the xylem-inhabiting microbiome and if those changes may modify the resistance response that a wild olive clone shows to the highly virulent defoliating (D) pathotype of Vd. Results indicated that although there were differences in microbial communities among the different propagation methodologies, most substantial changes occurred when plants were inoculated with Vd, regardless of whether the infection process took place, with a significant increase in the diversity of bacterial communities when the pathogen was present in the soil. Furthermore, it was noticeable that olive plants multiplied under in vitro conditions developed a susceptible reaction to D Vd, characterized by severe wilting symptoms and 100% vascular infection. Moreover, those in vitro propagated plants showed an altered xylem microbiome with a decrease in total OTU numbers as compared to that of plants multiplied under non-aseptic conditions. Overall, 10 keystone bacterial genera were detected in olive xylem regardless of infection by Vd and the propagation procedure of plants (in vitro vs nursery), with Cutibacterium (36.85%), Pseudomonas (20.93%), Anoxybacillus (6.28%), Staphylococcus (4.95%), Methylobacterium-Methylorubrum (3.91%), and Bradyrhizobium (3.54%) being the most abundant. Pseudomonas spp. appeared as the most predominant bacterial group in micropropagated plants and Anoxybacillus appeared as a keystone bacterium in Vd-inoculated plants irrespective of their propagation process. Our results are the first to show a breakdown of resistance to Vd in a wild olive that potentially may be related to a modification of its xylem microbiome and will help to expand our knowledge of the role of indigenous xylem microbiome on host resistance, which can be of use to fight against main vascular diseases of olive.
Introduction
Verticillium wilt, caused by the vascular-colonizing, soil-borne fungus, Verticillium dahliae (Vd), is one of the main diseases threatening the health and growth of olive (Olea europaea subsp. europaea var. europaea) production worldwide. This disease, first reported in Italy in 1946, has steadily increased in prevalence and incidence to become an actual threat to olive cultivation in the Mediterranean Basin due to the high rates of tree mortality and important reductions in yields (Jiménez-Díaz et al., 2011; Landa et al., 2019). Infection of olive plants by Vd takes place through the root system; then, the pathogen colonizes the xylem vessels and impairs the sap flow by means of mycelial proliferation, the formation of occlusions and tyloses that ultimately cause the tree death (Báidez et al., 2007; Jiménez-Díaz et al., 2011). Two pathotypes have been identified among Vd isolates in olive, namely, defoliating (D) and non-defoliating (ND), which differ much in virulence and determine the severity of Verticillium wilt. The ND pathotype induces moderately severe branch die back and leaf necrosis, whereas the highly virulent D pathotype induces severe falling of green leaves, necrosis of entire plant canopy sectors, and eventually tree death (Navas-Cortés et al., 2008; Jiménez-Díaz et al., 2011).
The most practical and economically efficient method for the management of Verticillium wilt is the use of resistant cultivars. However, most of the olive cultivars widely grown in Spain are moderately to highly susceptible to D Vd (López-Escudero et al., 2010; Jiménez-Díaz et al., 2011; Ostos et al., 2020). Recently, a few wild olive genotypes were identified as highly resistant to D Vd that may have a valuable potential as rootstocks for the management of Verticillium wilt (Jiménez-Fernández et al., 2016). Nevertheless, use of a single control measure may not be fully effective for the management of Verticillium wilt in olives, as shown for other wilt diseases (Jiménez-Díaz et al., 2011). Thus, an integrated management strategy is advisable, combining the use of resistant olive cultivars, or of tolerant ones grafted onto resistant rootstocks, with adequate irrigation management and agricultural practices that prevent the spread of inoculum of the pathogen (Jiménez-Díaz et al., 2011; Bubici and Cirulli, 2012; Jiménez-Fernández et al., 2016).
Although plants have evolved their own adaptations to alleviate most biotic and abiotic stresses in nature, they also rely on their microbial partners to survive and defend themselves against microbial invaders and pathogens (Hassani et al., 2018). In nature, healthy plants live in association and interact with a myriad of microorganisms, collectively called the plant microbiome, which is now known to bear important roles in plant health. Endophytes are bacteria and fungi that live within plants where they establish non-pathogenic relationships with their hosts (Azevedo et al., 2000) and promote plant growth directly by phytostimulation and biofertilization, and/or indirectly by inducing stress tolerance and disease suppression (Compant et al., 2016; Hassani et al., 2018). Therefore, a thorough knowledge of the microbial communities residing within the xylem vessels of olive trees may be crucial for understanding their potential influence on the healthy growth of this plant as well as on the resistance shown by specific olive genotypes against D Vd or other vascular plant pathogens (Hong and Park, 2016). Different methodological approaches, including culture-dependent procedures complemented with next-generation sequencing (NGS) technologies, have recently made it possible to characterize microbial communities associated with olive xylem tissue (Hardoim et al., 2015; Fausto et al., 2018; Anguita-Maeso et al., 2020; Zicca et al., 2020). However, although it can be considered that most of the olive xylem microbiota should be assembled by microorganisms with neutral or positive effects, their mechanistic role in defense against vascular pathogens has not yet been addressed.
The modification or attenuation of the diversity and composition of xylem microbial communities might result in different responses from the plant host to cope with vascular pathogens. The transmission of microbiota to the progeny in plants vegetatively propagated represents a way to ensure the presence of beneficial symbionts within the plant (Vannier et al., 2018; Liu et al., 2019). However, it is unknown how xylem endophytic microbiota in olive may be transmitted from shoot tips to explants, as well as to mature plants, and how stable would that be during this process. Micropropagation has become an important tool to reproduce selected olive genotypes and guarantees true type and pathogen-free plants (Fabbri et al., 2009). Olive micropropagation, through tissue culture, which is conducted in vitro under aseptic conditions, for at least a certain period of time, can potentially induce changes and alter the composition of the xylem microbiome. Thus, by producing olive plants by tissue culture, some beneficial and non-pathogenic endophytes may be excluded, since tissue cultures are initiated from shoots after extensive surface sterilization and then plants are maintained under aseptic conditions. Consequently, this propagation procedure represents an ideal experimental approach to test whether the xylem microbiome has a role on the resistance shown by specific olive genotypes to vascular pathogens.
This present research was conceived to elucidate how plants protect themselves by shaping their xylem microbiome in a resistant wild olive genotype as a first step to assess the complex plant–microbe interactions in the xylem that can contribute to maintain olive health and its resistance response against vascular pathogens. The specific objectives of this work were to determine whether (i) in vitro propagation methodology can modify the diversity and composition of the xylem microbiome in olive; (ii) those changes may alter host resistance response to the vascular pathogen Vd; and (iii) inoculation and vascular infection of olive by Vd may induce changes in the olive xylem microbiome. Determining those effects may be essential to identify microbial communities that are triggered after pathogen infection and could act as antagonists against Vd. Furthermore, understanding the tight relationships between xylem-inhabiting microorganisms and vascular pathogens will help to reveal determinant microbial players that may contribute to produce olive plants more resilient to infection by vascular pathogens.
Materials and Methods
Olive Plant Material
A wild olive (O. europaea var. sylvestris) clone “Ac-18” highly resistant to the D pathotype of Vd (Jiménez-Fernández et al., 2016) was used in this study. The high resistance of “Ac-18” plants to D Vd is characterized by symptomless infection, together with plugging of xylem vessels, no re-isolation of the fungus from stem vascular tissue, and the plant’s ability to quantitatively reduce the extent of stem colonization by the pathogen (Jiménez-Fernández et al., 2016). Also, olive cv. “Picual” highly susceptible to D and susceptible to ND Vd pathotypes (Calderon et al., 2014) and a wild olive clone “Ac-15” highly susceptible to D Vd (Narváez et al., 2019) were used in the pathogenicity experiments as controls of disease reaction.
Three types of “Ac-18” plant materials were used in the study, which were derived from shoots of the same mother adult plant, namely: (i) in vitro-standard plants: plants micropropagated using the standard olive methodology of axillary shoot elongation; (ii) in vitro-adapted plants: plants micropropagated and subsequently adapted to greenhouse conditions; and (iii) nursery propagated: plants propagated following standard semi-woody stacking procedure at a commercial olive nursery.
For in vitro-standard propagation of “Ac-18” and “Ac-15” plants, 1.5- to 2.0-cm-long shoots bearing two nodes were multiplied as in Narváez et al. (2020) using RP medium [DKW macro- and micronutrients and vitamins as modified by Roussos and Pontikis (2002)] supplemented with 2 mg/L zeatin riboside (Vidoy-Mercado et al., 2012). For rooting, 2-cm-long shoots were cultured for 3 days in basal RP liquid medium supplemented with 10 mg/L IBA and subsequently transferred to basal solid RP medium supplemented with 1 g/L activated charcoal. Plants were incubated under a 16-h photoperiod at 40 μmol m–2 s–1 at 25 ± 2°C for 8 weeks until ensuring at least three to four roots. Adaptation to ex vitro conditions was carried out initially using 35 × 24 × 18 cm germination boxes under high levels of sterility. Propagated “Ac-18” rootlets were transplanted to 300-mL pots filled with a sterilized (121°C for 20 min) perlite and vermiculite (1:1) mixture. Plants were sprayed with sterile water, and the cover was sealed with transparent film, and incubated at 25 ± 2°C in darkness in a growth chamber. After 2 days, a 2-h cycle of indirect fluorescent light of 360 μmol m–2 s–1 was provided, the duration of which was increased daily until reaching a 12-h light cycle within 1 week. The starting high relative humidity provided by the closed environment made it unnecessary to water the plants for 3 weeks. Afterward, plants were watered (3 mL per pot) weekly with sterile water using a 10-mL syringe and fertilized once per month with Hoagland’s nutrient solution (Hoagland and Arnon, 1950). After 8 weeks, plants were transplanted to 500-mL pots filled with a sterile peat:perlite (3:1) mixture. Plants grew for additional 6 months in a growth chamber adjusted to 22 ± 2°C, 60–80% relative humidity, and a 14-h photoperiod of fluorescent light of 360 μmol m–2 s–1. Plants were watered as needed and fertilized weekly with 100 ml of Hoagland’s nutrient solution.
In vitro-adapted “Ac-18” plants were produced using the same methodology than for in vitro-standard plants, with the exception that plants were grown for additional 12 months in a glass greenhouse at a fluctuating minimum/maximum temperature of 15 ± 5 and 25 ± 5°C across the entire growing period and daylight conditions. Plants were watered using tap water as needed and fertilized weekly as described before.
Finally, nursery propagated “Ac-18” and “Picual” plants followed standard semi-woody stacking procedure at a commercial olive nursery (Plantas Continental S.A, Córdoba, Spain). Briefly, semi-hard stem cuttings with two active leaves on the top were dipped in an indole butyric acid solution to stimulate rooting and planted on peat:coconut fiber (1:5) pellets under mist conditions in plastic tunnels (Caballero and Del Río, 2010). Once callus was formed and roots appeared (three to four roots), plants were transplanted to 500-mL pots containing a perlite:coconut fiber:peat (1.5:5:3.5) mixture amended with 4 g L–1 of slow release fertilizer (Osmocote® Exact standard 15-9-12 + 2MgO; ICL Specialty Fertilizers, Netherlands). Plants were incubated under natural environmental conditions for 6 months in a plastic greenhouse. During this time, plants received water as needed but no additional fertilizers.
At the time of inoculation with the pathogen in vitro-standard, nursery propagated and in vitro-adapted “Ac-18” plants were 10, 10, and 18 months old, respectively. Apparently, plants from all types of propagation procedures had a similar degree of bark lignification of the main stem and root development (data not shown).
Pathogenicity Experiment
A monoconidial Vd isolate (V138I) from defoliated “Coker 310” cotton plants at Córdoba (Spain) and a representative of the D pathotype was used in the experiment. This isolate proved highly virulent on olive in a previous work (Jiménez-Fernández et al., 2016). Inoculum consisted of an infested cornmeal–sand mixture (CMS; sand:cornmeal:deionized water, 9:1:2, w/w) produced as described by Jiménez-Fernández et al. (2016). The infested CMS was homogenized, allowed to desiccate in an incubator adjusted to 33°C for 3 days, and thoroughly mixed with a pasteurized soil mixture (clay loam:peat, 2:1, v/v) at a rate of approximately 1:20 (w/w) to reach an inoculum density of 5 × 107 CFU g–1 soil of Vd as determined by dilution-plating on chlortetracycline-amended water agar (CWA; 1 L distilled water, 20 g agar, 30 mg chlortetracycline) (Jiménez-Fernández et al., 2016).
Plants were then transplanted to pots filled with the pasteurized soil mixture. For transplanting inoculation, plants uprooted from the potting substrate were shaken to retain the rhizosphere soil and placed in pots filled with the infested soil mixture. Control plants were treated similarly with sterile distilled water (root-dip inoculation), or grown in non-infested CMS mixed with the pasteurized soil mixture at the same rate as infested CMS (transplanting inoculation). Inoculated and control plants were incubated in the growth chamber adjusted to the above conditions for 3 months.
Plants of “Ac-18” clone, representative of each propagation procedure, and susceptible “Picual” and “Ac-15” plants, were then transplanted to 1500-mL pots filled with the D Vd-infested soil mixture. Before transplanting, plants were uprooted from the potting substrate, gently shaken to retain only the rhizosphere soil, and placed in pots filled with the infested soil mixture. Non-inoculated plants were treated similarly and transplanted to the pasteurized soil mixture mixed with non-infested CMS at the same rate as infested CMS. Inoculated and control plants were incubated in a growth chamber adjusted to 22 ± 2°C, 60–80% relative humidity, and a 14-h photoperiod of fluorescent light of 360 μmol m–2 s–1 for 3 months. During this time, plants were watered as needed with tap water and fertilized weekly as previously described. There were six replicated pots (one plant per pot) for inoculated and non-inoculated plants of each plant genotype, respectively, in a completely randomized design.
Disease reaction was assessed by the incidence (percentage of plants showing disease symptoms) and severity of foliar symptoms. Symptoms were assessed on individual plants on a 0–4 rating scale according to the percentage of affected leaves and twigs at 2- to 3-day intervals throughout the duration of the experiment (Jiménez-Fernández et al., 2016). Upon termination of the experiment, the extent of colonization by Vd was determined by isolations of the fungus in CWA (Jiménez-Fernández et al., 2016) from 6-cm-long stem pieces sampled from the main stem at the same time as similar samples were processed for extraction of xylem microbiome (see below). Data of pathogen isolation from the stem were used to calculate the intensity of stem vascular colonization for each individual plant, according to a stem colonization index (SCI) as described before (Jiménez-Fernández et al., 2016).
Additionally, the amount of Vd present in “Ac-18” stem samples was determined by using the TaqMan qPCR assay developed by Bilodeau et al. (2012) as described in Gramaje et al. (2013). The same DNA samples used for the xylem microbiome characterization were used for pathogen quantification, with each sample being analyzed in duplicate. All qPCR assays were performed in a LightCycler480 (Roche Diagnostics) apparatus. The cycle threshold (Ct) values for each qPCR reaction were calculated using the default estimation criteria in the manufacturer’s software. The quantification limit of the TaqMan qPCR assay was fixed at a Ct of 36 (0.1 pg of Vd DNA μL–1) (Gramaje et al., 2013).
DNA Xylem Microbiome Extraction and Sequencing
The xylem microbiome was extracted following the procedure described by Anguita-Maeso et al. (2020). Briefly, a number of three 6-cm-long pieces from the bottom, middle, and upper stem of each “Ac-18” plant were debarked and xylem chips were obtained by scraping the most external layer of the debarked woody pieces with a sterile scalpel. Xylem chips from an “Ac-18” plant were mixed together, and a 0.5-g sample was placed in a Bioreba bag containing 5 mL of sterile phosphate-buffered saline (PBS); the bags were closed with a thermal sealer and the content was macerated with a hand homogenizer (BIOREBA, Reinach, Switzerland). Extracts were stored at −80°C until DNA extraction. All the processes described above took place under sterile conditions within a flow hood chamber (Anguita-Maeso et al., 2020). Aliquots (0.5 mL) of macerated xylem chips in PowerBead tubes (DNeasy PowerSoil Kit, QIAGEN) were homogenized 7 min at 50 pulses s–1 with the Tissuelyser LT (QIAGEN) prior to incubation in lysis buffer for 1 h at 60°C for increasing cell lysis, and then processed following the DNeasy PowerSoil Kit manufacturer’s protocol.
Extracted DNA was used directly to amplify the V5–V6 rRNA region with the primers 799F (5’-AAC MGGATTAGATACCCKG-3’) and 1115R (5’-AGGGTTGCG CTCGTTG-3’). PCR products were purified using Agencourt AMPure XP (Beckman Coulter) following manufacturer instructions. Purified PCR products were quantified using Quant-iTTM PicoGreenTM dsDNA Assay Kit (Thermo Fisher Scientific) and a Tecan Safire microplate reader (Tecan Group, Männedorf, Switzerland). Equimolecular amounts from each individual sample in 10 mM of Tris were combined, and the pooled library was sequenced by the Genomics Unit at “Fundación Parque Científico de Madrid,” Madrid, Spain using the Illumina MiSeq platform (Nano-V2; PE 2 × 250 bp). The ZymoBIOMICS microbial standard (Zymo Research Corp., Irvine, CA, United States) and water (no template DNA) were used as internal positive and negative controls, respectively, for library construction and sequencing. Raw sequence data have been deposited in the Sequence Read Archive (SRA) database at the NCBI under BioProject accession number PRJNA679263.
Statistical and Bioinformatics Analyses
Quality control and adapter trimming of demultiplexed raw fastq 16S rRNA sequences obtained from MiSeq output was performed with FastQC and TrimGalore tools. Truncation length in 225 bp of the forward and reverse reads was needed to increase the Phred quality (Q > 30) score visualized in MultiQC tool. No trimming length was needed. Quality reads were then analyzed using the Quantitative Insights into Microbial Ecology bioinformatics pipeline, QIIME2 (version 2020.21) (Caporaso et al., 2010; Bolyen et al., 2019) with default parameters unless otherwise noted. DADA2 pipeline was used for denoising fastq paired-end sequences along with filtering chimeras. Operational taxonomic units (OTUs) were obtained at 99% similarity and were taxonomically classified using VSEARCH consensus taxonomy classifier (Rognes et al., 2016) against Silva SSU v.138 database. Singletons were discarded for taxonomy and statistical analysis.
Differences in bacterial communities were calculated in QIIME2 using rarefaction curves of alpha-diversity indexes (Richness, Shannon, Evenness, and Simpson) at OTU level. Alpha and beta diversity as well as alpha rarefaction curves were conducted rarefying all samples to the minimum number of reads found. The non-parametric Scheirer–Ray–Hare test (P < 0.05) was used to assess the effects of the inoculation treatment, propagation method, and their interaction in alpha diversity indexes, using the rcompanion package (Mangiafico, 2020) in R. Dunn’s Kruskal–Wallis multiple comparisons were performed for post hoc analysis. The P-value was adjusted with the Benjamini–Hochberg method (Benjamini and Hochberg, 1995). Venn diagrams were generated from non-rarefied data using Venn package (Dusa, 2018) in R, and were used to identify shared (core microbiome) or unique taxa according to the inoculation treatment and propagation methods. Linear discriminant analysis effect size (LEfSe) method to identify differentially abundant bacterial taxa associated to inoculation treatments and propagation methods was carried out (Segata et al., 2011).
Additionally, weighted UniFrac distances were estimated at OTU level taking into account the phylogenetic distance among bacterial communities (Lozupone and Knight, 2005). Principal coordinate analysis (PCoA) of weighted UniFrac distance matrix was used to evaluate similarities among the bacterial communities according to the inoculation treatment or propagation procedure. Additionally, the adonis function within the vegan package in R (999 permutations) was performed to test the effects (P < 0.05) of the inoculation treatment, the plant propagation method, and their interaction.
Results
Pathogenicity Experiment
Typical Verticillium wilt symptoms consisting of early dropping of green leaves, and necrosis and death of some branches, characteristics of infection by D Vd, started to develop in “Ac-15” and “Picual” plants, as expected, by 21 and 27 days after pathogen inoculation, respectively (Supplementary Figure S1). The mean incubation period was of 21.5 ± 1.7 and 29.5 ± 1.7 days for “Ac-15” and “Picual,” respectively. All “Picual” and “Ac-15” plants were dead by 2 months after inoculation. On the contrary, no symptoms developed on nursery and in vitro-adapted propagated “Ac-18” plants as expected (Jiménez-Fernández et al., 2016). Surprisingly, plants of “Ac-18” that underwent in vitro propagation under aseptic conditions (i.e., in vitro-standard) started to develop disease symptoms 29 days after inoculation (mean incubation period of 33.8 ± 1.8 days), reaching a disease incidence of 100% and a final disease severity of 1.95 ± 0.6 on a 0–4 rating scale at the end of the experiment (Figure 1 and Supplementary Figure S1). Vd was not re-isolated from any of the stem zones sampled from “Ac-18” nursery and in vitro-adapted propagated plants, but was isolated from all “Ac-18” in vitro-standard propagated plants, with a mean SCI value of 80.95 ± 6.55%. No symptoms were developed on non-inoculated control plants (Supplementary Figure S1).
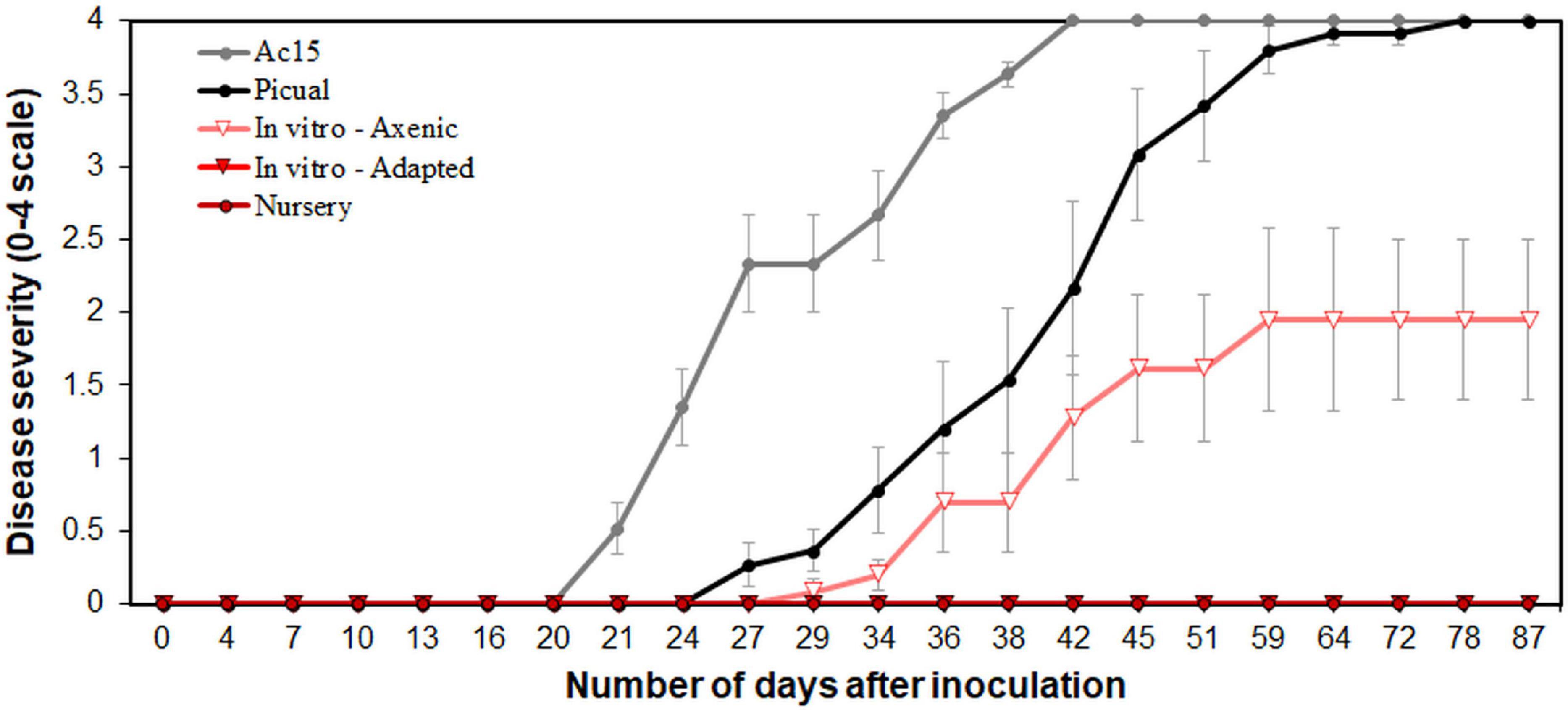
Figure 1. Verticillium wilt disease progression in “Ac-18” in vitro (standard and adapted) and nursery propagated olive plants inoculated with the defoliating pathotype of Verticillium dahliae. “Picual” and “Ac-15” olive genotypes were used as positive controls to determine the inoculation success and development of the disease. Each point represents the mean disease severity (0–4 scale: 0 = healthy, 4 = dead plant) of data and error bars show the standard error from six plants per treatment.
In parallel with the Vd isolations, the pathogen was detected in DNA samples from all in vitro-standard propagated plants, with mean Ct values of 28.84 ± 0.18. In contrast, Vd was not detected in DNA samples of any of the nursery propagated plants, and Vd was detected only in three samples from the in vitro-adapted propagated plants, which showed Ct values slightly above the detection limit (i.e., 37.0 ± 0.30).
Alpha and Beta Diversity Analysis
Sequencing analysis resulted in a total of 125,685 raw reads. After removal of chimeras, unassigned, or mitochondrial reads, we finished with 19,936 good quality reads assigned to bacteria with a mean length of 333 bp. No chloroplast reads were detected in our samples. A total of 118 OTUs were identified for all treatments, with 18 OTUs being retained for alpha and beta diversity analysis after rarefying all data to the minimum number of reads obtained and singleton removal. High values of Good’s coverage (data not shown) and rarefaction curves of observed OTUs reaching a plateau (Supplementary Figure S2) were obtained for all samples indicating enough sequencing depth.
Rarefaction curves of observed OTUs clearly showed a higher number of OTUs on Vd-inoculated plants when compared to that on non-inoculated plants, with lower differences among propagation methods within them (Supplementary Figure S2). Similarly, the Scheirer–Ray–Hare test indicated significant differences (P < 0.05) for two alpha-diversity indexes (Richness and Shannon) according to the inoculation treatment (H > 9.94, P < 0.002), with no significant differences (P ≥ 0.05) among propagation methods (H < 5.14, P > 0.076) or its interaction with the inoculation treatment (H < 1.08, P > 0.581) (Figure 2 and Supplementary Table S1). Interestingly, inoculation with Vd significantly increased Richness and Shannon alpha diversity indexes in all types of propagation; i.e., inoculated plants presented a higher number of OTUs either analyzed as counts or considering their relative abundances (Figure 2 and Supplementary Figure S2). On the other hand, for the Evenness index, we found a significant effect (H = 10.79, P = 0.004) for the propagation method, but not for the inoculation treatment (H = 2.51, P = 0.113) or their interaction (H = 0.87, P = 0.648), due to a less even distribution of OTUs for the nursery propagated plants (Figure 2 and Supplementary Table S1).
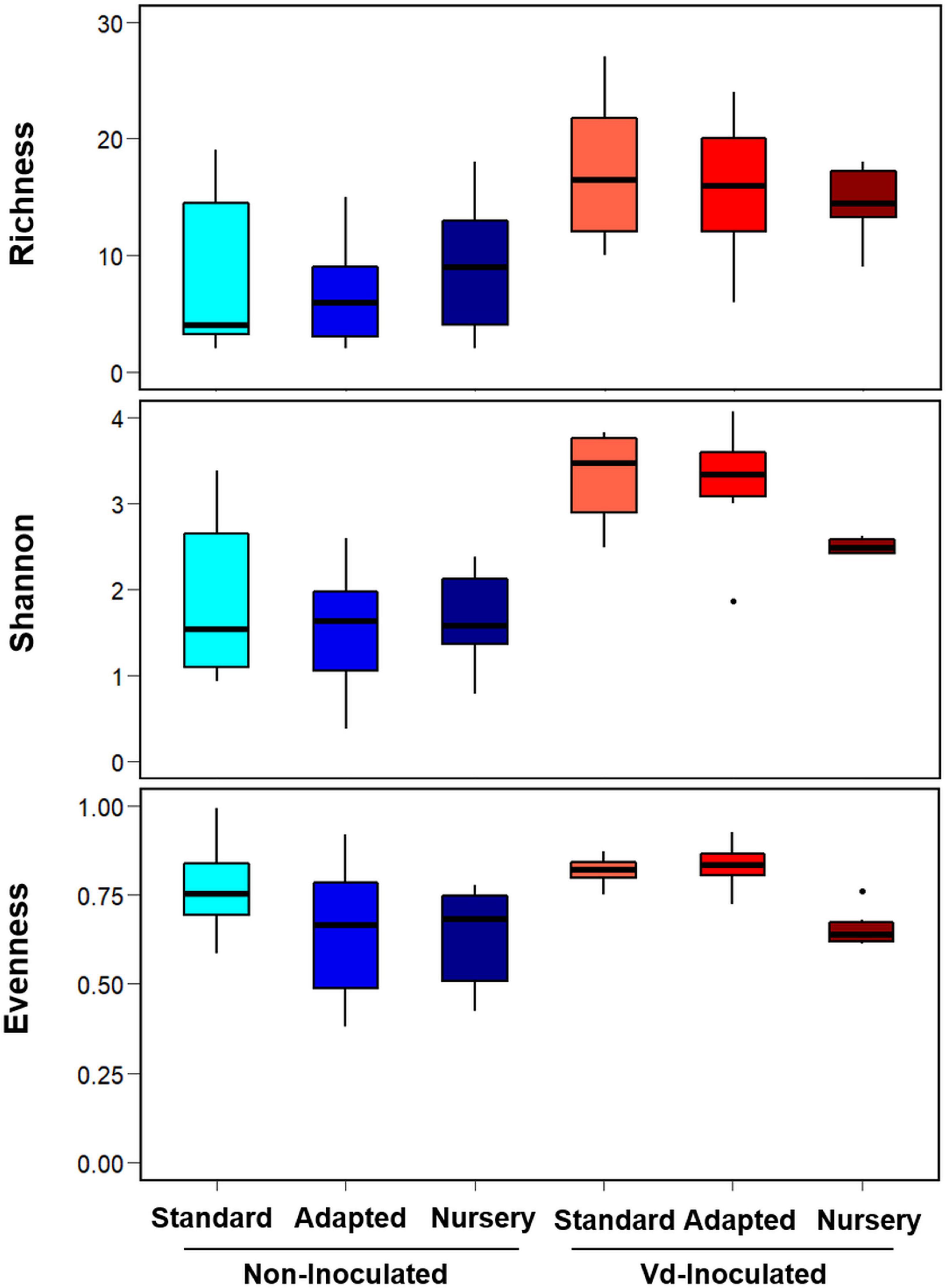
Figure 2. Boxplots of Richness, Shannon, and Evenness alpha diversity indices at OTU taxonomic level in olive xylem from Verticillium dahliae (Vd)-inoculated and non-inoculated “Ac-18” plants following in vitro (standard and adapted) and nursery propagation methods. Boxes represent the interquartile range, while the horizontal line inside the box defines the median and whiskers represent the lowest and highest values of six values for each treatment combination. For all three indexes and propagation methods, values on Vd-inoculated plants were significantly higher compared to that on non-inoculated treatments according to the Scheirer–Ray–Hare test at P < 0.05.
Principal coordinate analysis of weighted UniFrac distances differentiated xylem bacterial communities mainly according to the inoculation treatment. Thus, with a few exceptions, there was a clear trend to group the bacterial communities along Axis 1, which explained 51.3% of the variation, mainly by the presence of Vd in the soil mixture, and then along Axis 2, which explained 17.2% of the variation, also according to the inoculation procedure and in a lower extent according to the propagation method, with in vitro-adapted and nursery propagated plants being more similar between them (Figure 3). Thus, ADONIS analysis supported these results and indicated a main significant effect of the propagation method (R2 = 0.268, P < 0.001) followed by the inoculation treatment (R2 = 0.112, P = 0.004), with no interaction effect (R2 = 0.062, P = 0.175) (Supplementary Table S2).
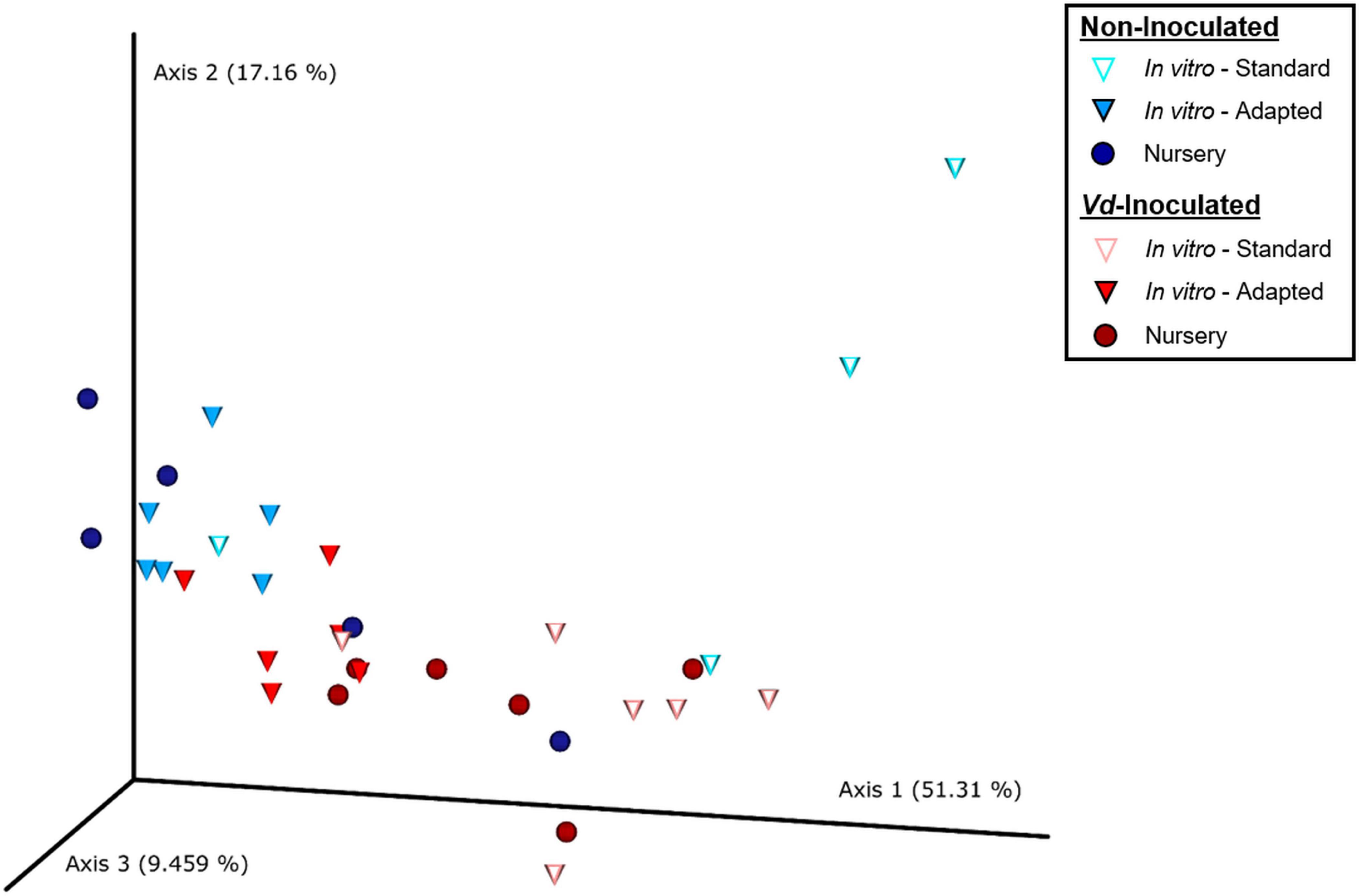
Figure 3. Principal coordinates plot of weighted UniFrac distances of bacterial communities at OTU taxonomic level in olive xylem from Verticillium dahliae (Vd)-inoculated and non-inoculated “Ac-18” plants following in vitro (standard and adapted) and nursery propagation methods. Points are colored by plant inoculation treatment and shaped by propagation methods.
Composition and Abundance of Olive Xylem Bacterial Communities
A total of 10 phyla, 15 classes, 45 orders, 67 families, 103 genera, and 118 OTUs were identified considering all treatments, with four phyla, five classes, 11 orders, 11 families, 10 genera, and five OTUs being shared among all of them (Supplementary Figure S3). When analyzing the samples according to the inoculation procedure, a higher number of OTUs were found in “Ac-18” plants inoculated with Vd (94), while 61 were found on non-inoculated plants. This trend of detecting higher number of taxa on Vd-inoculated plants was observed at all the taxonomic levels. The lowest number of OTUs from all taxonomy ranks was found in non-inoculated plants propagated under in vitro conditions. Additionally, in vitro-adapted plants shared a higher number of OTUs with nursery instead of with in vitro-standard propagated plants (Supplementary Figure S3).
At the genus level, a total of 18 genera formed the core microbiome of Vd-inoculated plants when considering all propagation methods jointly, whereas 10 genera were shared within non-inoculated plants. Those same 10 genera were shared between Vd-inoculated and non-inoculated treatments (Acidibacter, Anoxybacillus, Bradyrhizobium, Corynebacterium, Cutibacterium, Methylobacterium-Methylorubrum, Paenibacillus, Pseudomonas, Sphingomonas, and Staphylococcus), while eight genera (Acinetobacter, Caulobacter, Comamonadaceae, Dermacoccus, Flavisolibacter, Massilia, Paracoccus, and Sericytochromatia) were detected exclusively in Vd-inoculated plants. In addition, a higher number of genera were found in Vd-inoculated plants (82) compared to those in non-inoculated plants (53) (Figure 4A).
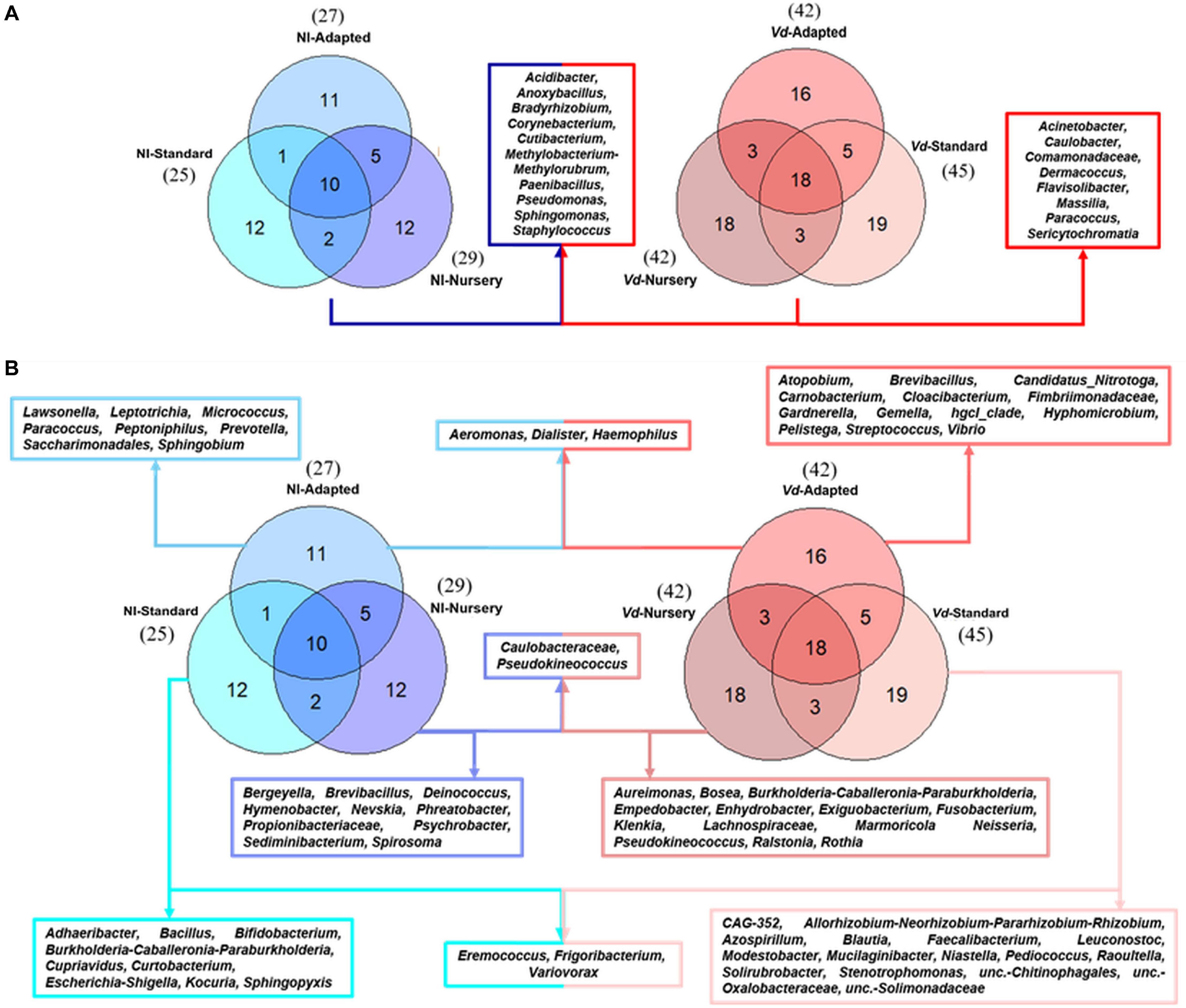
Figure 4. Prevalence Venn diagram showing the unique and shared bacterial genera in olive xylem from the core microbiome (A) or by each propagation approach (B) obtained from Verticillium dahliae (Vd)-inoculated and non-inoculated (NI) “Ac-18” plants following in vitro (standard and adapted) and nursery propagation methods.
When analyzing only non-inoculated plants, 25 and 27 genera were identified in in vitro-standard and in vitro-adapted propagated plants, respectively, while 29 genera were identified in nursery propagated plants. Additionally, a high number of unique genera were found in each propagation method. A total of 12 unique genera were identified for in vitro-standard propagated plants (Adhaeribacter, Bacillus, Bifidobacterium, Burkholderia-Caballeronia-Paraburkholderia, Cupriavidus, Curtobacterium, Eremococcus, Escherichia-Shigella, Frigoribacterium, Kocuria, Sphingopyxis, and Variovorax), 12 for nursery propagated plants (Bergeyella, Brevibacillus, Caulobacteraceae, Deinococcus, Hymenobacter, Nevskia, Phreatobacter, Propionibacteriaceae, Pseudokineococcus, Psychrobacter, Sediminibacterium, and Spirosoma), and 11 for in vitro-adapted plants (Aeromonas, Dialister, Haemophilus, Lawsonella, Leptotrichia, Micrococcus, Paracoccus, Peptoniphilus, Prevotella, Saccharimonadales, and Sphingobium) (Figure 4B). On the other hand, when analyzing each propagation methodology for Vd-inoculated plants, 45, 42, and 42 genera were identified in in vitro-standard, in vitro-adapted, and nursery propagated plants, respectively. Unique genera differed according to the propagation approach. Thus, a total of 19 unique genera were found in in vitro-standard propagated plants (CAG-352, Allorhizobium-Neorhizobium-Pararhizobium-Rhizobium, Azospirillum, Blautia, Eremococcus, Faecalibacterium, Frigoribacterium, Leuconostoc, Modestobacter, Mucilaginibacter, Niastella, Pediococcus, Raoultella, Solirubrobacter, Stenotrophomonas, unc.-Chitinophagales, unc.-Oxalobacteraceae, unc.-Solimonadaceae, and Variovorax), 16 genera were exclusive from in vitro-adapted propagated plants (Aeromonas, Atopobium, Brevibacillus, Candidatus_Nitrotoga, Carnobacterium, Cloacibacterium, Dialister, Fimbriimonadaceae, Gardnerella, Gemella, Haemophilus, hgcI_clade, Hyphomicrobium, Pelistega, Streptococcus, and Vibrio), and 18 from nursery propagated plants (Actinomyces, Aeromicrobium, Agromyces, Aureimonas, Bosea, Burkholderia-Caballeronia-Paraburkholderia, Caulobacteraceae, Empedobacter, Enhydrobacter, Exiguobacterium, Fusobacterium, Klenkia, Lachnospiraceae, Marmoricola Neisseria, Pseudokineococcus, Ralstonia, and Rothia) (Figure 4B).
At the phylum level, Actinobacteriota presented the highest relative abundance considering all experimental treatments together (43.62%), followed by Proteobacteria (38.72%), Firmicutes (15.24%), and Bacteroidota (1.63%). However, these relative abundances varied within each treatment tested. Actinobacteria was more abundant in non-inoculated plants, with a proportion of 46.41%, decreasing to 40.82% for Vd-inoculated plants. For non-inoculated plants, this phylum was the most abundant for in vitro-adapted propagated plants (70.98%), followed by nursery (52.21%) and in vitro-standard (16.04%) propagation methods, and it showed the same trend in Vd-inoculated plants, reaching 58.56, 39.16, and 24.73%, for these same propagation methods, respectively. Proteobacteria were present at a similar percentage in non-inoculated (40.77%) and Vd-inoculated (36.66%) plants, but varied within propagation methods. Thus, it was the most abundant phylum for the in vitro-standard plants for both non-inoculated (75.86%) and Vd-inoculated plants (49.94%), finding its lowest relative abundance for in vitro-adapted propagated plants with 18.79 and 22.12%, for non- and Vd-inoculated plants, respectively. Finally, Firmicutes showed a different response to both inoculation treatment and propagation methods compared to the two previous phyla. First, it showed the highest global relative abundance in Vd-inoculated plants with 18.92%, which decreased to 11.56% in non-inoculated plants. Second, while in non-inoculated plants, the highest relative abundance was estimated in nursery propagated plants (17.53%), it represented ca. 8.57% in both in vitro propagation methods, but similar abundance values were reached in Vd-inoculated plants (17.61–20.34%), irrespective of the plant propagation method (Figure 5A).
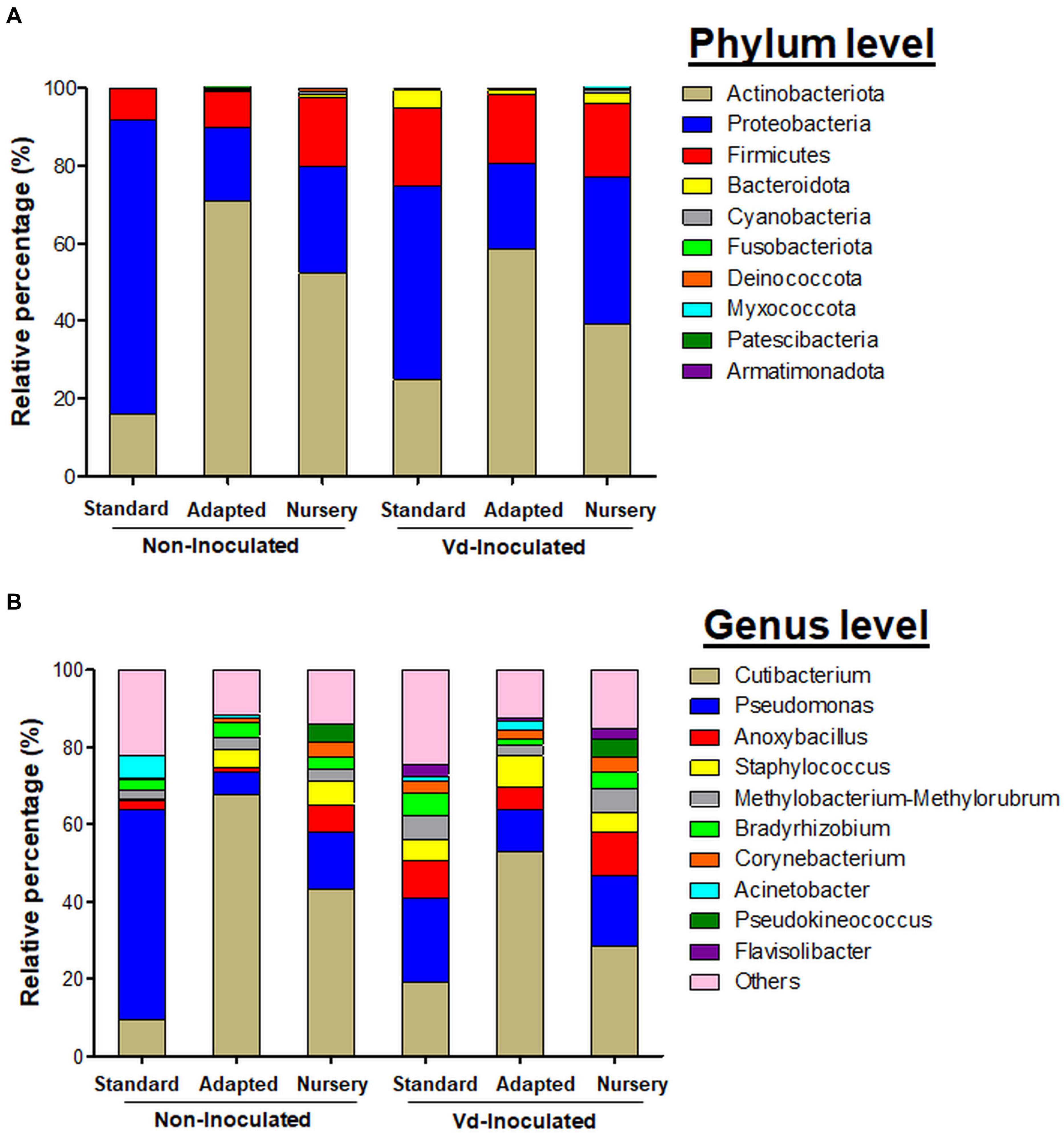
Figure 5. Bar plots showing the relative bacterial abundance taxa at phylum (A) and genera (B) level present in olive xylem from Verticillium dahliae (Vd)-inoculated and non-inoculated “Ac-18” plants following in vitro (standard and adapted) and nursery propagation methods.
In line with these results, LEfSe was used to identify the key phylotypes that could be differentially associated to the different experimental treatments. When comparing the three propagation methods within each inoculation treatment, Proteobacteria-Gammaproteobacteria was the most significant Phylum-Class for non-inoculated in vitro-standard propagated plants. For plants growing in Vd-infested soils, more diversity was observed among propagation methods, with Proteobacteria-Gammaproteobacteria and Bacteroidota-Bacteroidia, and Actinobacteriota-Actinobacteria being the most prevalent Phylum-Class in in vitro-standard and in in vitro-adapted propagated plants, respectively, while the Phylum-Class Proteobacteria-Alphaproteobacteria was the most prominent in nursery propagated plants. On the other hand, when comparing the effect of the inoculation with the pathogen within each propagation method, Firmicutes-Bacilli was a significant Phylum-Class for Vd-inoculated plants, in both in vitro-standard and in vitro-adapted propagated plants, whereas Proteobacteria-Gammaproteobacteria and Bacteroidota-Bacteroidia were also the prevalent Phyla-Class for in vitro-standard and nursery propagated plants, respectively. For non-inoculated plants, only a Phylum-Class (Actinobacteriota-Actinobacteria) appeared as the most prominent and only for in vitro-adapted plants (Figure 6).
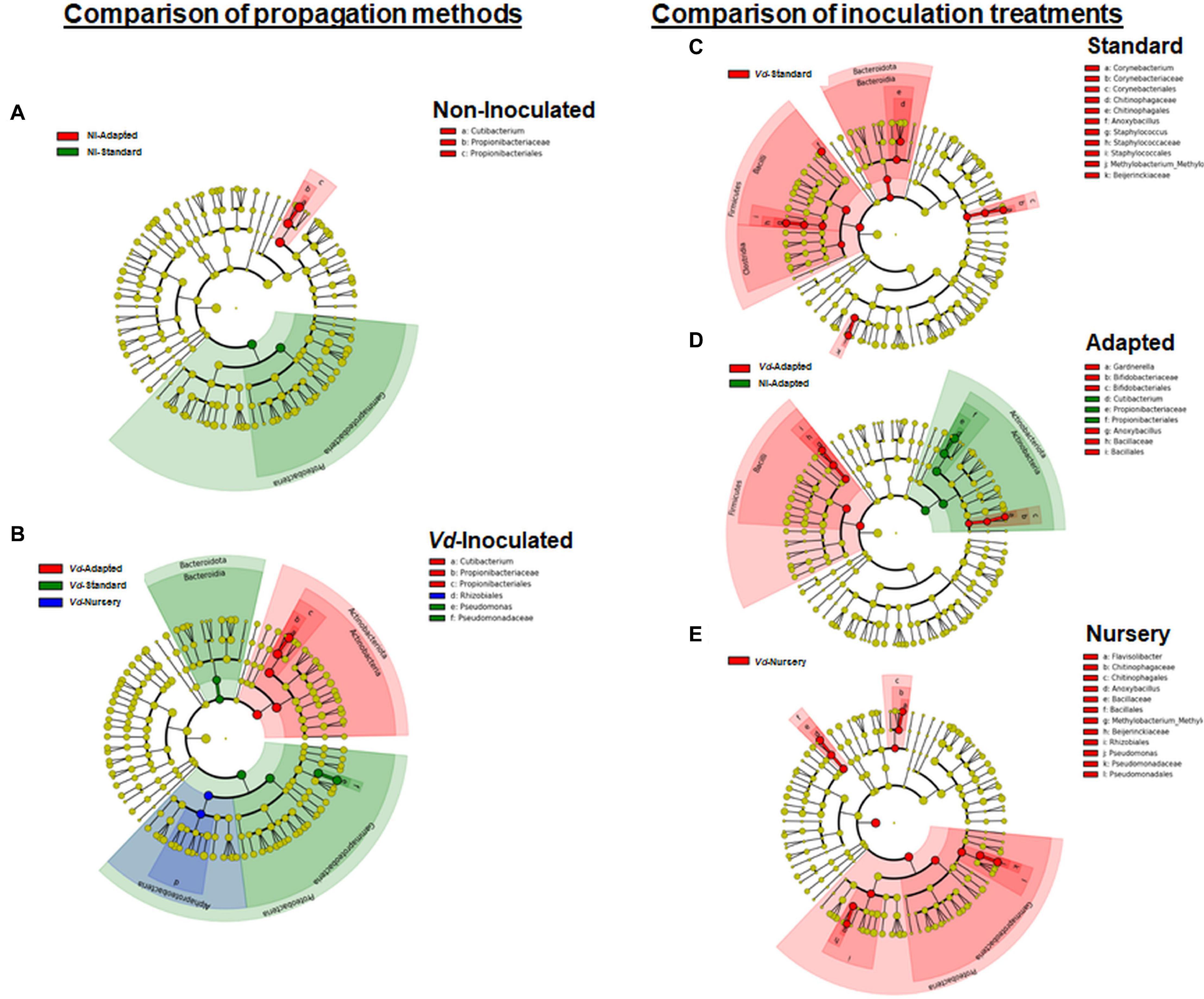
Figure 6. Cladogram representation from LEfSe analysis showing the taxonomic ranks from the innermost phylum ring to the outermost genera ring. Each point is a member within each taxonomic rank. Significant taxa (P < 0.05) appearing as dominant for each treatment when comparing each propagation method by inoculation treatment (A,B), or each inoculation treatment by propagation method (C–E) are shown in different colors (red, green, or blue) associated to the legend of each individual cladogram.
The most abundant genera identified among all treatments were Cutibacterium (36.85%), Pseudomonas (20.93%), Anoxybacillus (6.28%), Staphylococcus (4.95%), Methylobacterium-Methylorubrum (3.91%), Bradyrhizobium (3.54%), Corynebacterium (2.53%), Acinetobacter (1.77%), Pseudokineococcus (1.59%), and Flavisolibacter (1.07%) (Figure 5B). Cutibacterium was the genus with the highest relative abundance, reaching maximum frequencies in in vitro-adapted plants [both in non-inoculated (67.81%) and Vd-inoculated plants (53.04%)]. Other predominant genera varied in proportion depending on the treatment combination. Pseudomonas (54.25%) was the most representative genera in non-inoculated plants, propagated by the in vitro-standard method, whereas similar abundances were found for the remaining treatments (14.66%), with the exception of in vitro-adapted non-inoculated plants (5.77%). A noticeable lower relative abundance of Anoxybacillus was found in non-inoculated plants from both in vitro propagation methods (standard and adapted with 2.42 and 1.06%, respectively) compared with the nursery propagation (7.32%). Also, the small proportion of Staphylococcus found in in vitro-standard propagated and non-inoculated plants (0.52%) compared with the rest of the treatments (5.84%) was remarkable. Also, Acinetobacter showed a high proportion (3.34%) in in vitro propagated plants, but was much less relatively abundant in nursery propagated plants (0.04%) (Figure 5B).
In line with these results, when comparing the propagation methods within each inoculation treatment, LEfSe displayed Cutibacterium as the only genus with significant changes in relative abundance for in vitro-adapted propagated plants and for both inoculation treatments (Figures 6A,B), whereas for Vd-inoculated plants, Pseudomonas appeared as the most dominant genera for in vitro-standard propagated plants (Figure 6B). On the other hand, when comparing the effect of pathogen inoculation within each propagation method, Anoxybacillus appeared as a genus with significant higher relative abundances in Vd-inoculated plants for all propagation methods (Figures 6C–E). Furthermore, other distinct genera appeared as the most dominant within each propagation method when Vd was infesting the soil including Flavisolibacter, Methylobacterium-Methylorubrum, and Pseudomonas in nursery propagated plants (Figure 6E); Corynebacterium, Staphylococcus, and Methylobacterium-Methylorubrum in in vitro-standard propagated plants (Figure 6C); and Gardnerella in in vitro-adapted plants (Figure 6D).
Discussion
The use of host resistance is the most practical, long-term, and economically efficient disease control measure for vascular diseases in olive, and it is at the core of integrated disease strategies that must be practiced for the efficient management of these diseases (Jiménez-Díaz et al., 2011; Schneider et al., 2020). For Verticillium wilt, the use of wild olive rootstocks highly resistant to D Vd can provide an improved mean for its management, especially for grafting susceptible olive cultivars that are agronomically adapted, commercially desirable, or used in protected designation of origin extra virgin olive oils (Jiménez-Díaz et al., 2011; Trapero et al., 2012, 2013; Jiménez-Fernández et al., 2016; Ostos et al., 2020). In addition to that, use of endophytic plant-associated microorganisms with a specific beneficial interaction with the host plant could help to improve olive health and productivity providing a potential perspective for sustainable plant protection (Ryan et al., 2008; Berg, 2009; Berg et al., 2014; Müller et al., 2015).
In olive, several defense mechanisms, including both biochemical responses and plant structural characteristics, have been proposed as factors contributing to the resistance shown by different genotypes against its vascular pathogens Vd and/or Xylella fastidiosa. Those mechanisms should operate within the xylem tissues contributing to reduce systemic colonization by the pathogen and may include buildup of vessel occlusions by gums, gels or tyloses, phenolics and lignin content or their accumulation, induction of pathogenesis-related proteins, antioxidant-related enzymes, and ionome content (Báidez et al., 2007; Markakis et al., 2010; Jiménez-Fernández et al., 2016; de la O Leyva-Pérez et al., 2017; Gharbi et al., 2017a, b; Luvisi et al., 2017; Sabella et al., 2017, 2019; D’Attoma et al., 2019).
Although some cultivated and wild olive clones, including the “Ac-18” used in this study, have been described as resistant to D Vd based on asymptomatic pathogen colonization; for most of them, the pathogen could be detected by molecular methods or re-isolated from roots and stem tissues (Colella et al., 2008; Bubici and Cirulli, 2012; Gramaje et al., 2013; Jiménez-Fernández et al., 2016), indicating the plant’s ability to reduce the extent of stem colonization or other pathogenesis mechanisms that result in the absence of visible disease symptoms. However, the role that xylem microbial communities may play in that resistant response to Vd has been overlooked and remains unexplored to date. In this study, we tested the hypothesis that xylem microbiome may have a functional role on plant resistance. With that purpose, we explored whether or not in vitro propagation of “Ac-18” plants can alter the diversity and composition of xylem-inhabiting bacteria, and to which extent this could result in a modification of the high resistance response of that wild olive clone to the highly virulent D pathotype of Vd. Surprisingly, plants that underwent in vitro propagation under aseptic conditions lost the high resistance phenotype characteristic of the “Ac-18” clone. Actually, those plants developed wilting symptoms similar to those reported for other olive cultivars with a moderate-susceptible reaction to D Vd such as “Frantoio,” “Oblonga,” “Koroneiki,” “Empeltre,” or “Leccino” in similar inoculation experiments using olive plants of age similar to that in our study (López-Escudero et al., 2004; Martos-Moreno et al., 2006; Trapero et al., 2013).
Some authors found that disease severity in olive cultivars susceptible to Vd decreases with host age (López-Escudero et al., 2010; Trapero et al., 2013). In this present study, the loss of resistance shown by “Ac-18” in vitro-standard propagated plants cannot be associated to a more juvenile stage since those plants were of the same age as that of nursery-propagated plants and showed a similar growth (i.e., similar bark lignification and root development). However, “Ac-18” plants showed distinct xylem microbiome profiles according to the propagation procedure. The most significant change associated to in vitro-standard propagation was a decrease in the total number of OTUs detected, and a significantly higher number of Gammaproteobacteria (mainly Pseudomonas) and a lower number of Actinobacteria (mainly Cutibacterium). In parallel, beta-diversity indexes of xylem microbiome differed among propagation procedures, with plants that were initially propagated under in vitro-standard conditions and then challenged to less restricted aseptic environmental conditions (i.e., in vitro-adapted plants) showing a xylem microbiome more similar with the commercial nursery propagated plants. The olive explants from tissue culture may contain a genotype-specific core xylem microbiome that is transmitted from shoot tips of last generation (Liu et al., 2019). In our study, the explants grew under aseptic conditions and roots that differentiated from in vitro-standard plants did not get into contact with outside microbes, at least until the challenge with the pathogen. Thus, most of the differences found with in vitro-adapted or nursery propagated plants may be attributed to bacteria that were present at very low level, below the detection limit, at the beginning of micropropagation procedure and could not be detected by NGS. Alternatively, those bacteria might have been acquired by roots after recruitment when plants grew on a non-sterile substrate under less aseptic environmental conditions as proposed for other woody crops, including olive (Antoniou et al., 2017; Fausto et al., 2018; Deyett and Rolshausen, 2019).
Plant core microorganisms are considered to be consistently established in plants not being influenced by differences in space, time, or plant organs (Vorholt et al., 2017). In our study, 10 keystone bacterial genera could be considered the core microbiome being transmitted from generation to generation in olive, since they were detected in all samples regardless of plant propagation procedure (in vitro vs nursery) or inoculation with the pathogen, with Cutibacterium, Pseudomonas, Anoxybacillus, Staphylococcus, Methylobacterium-Methylorubrum, and Bradyrhizobium being the most abundant, in that order. These bacterial taxa have also been identified in olive xylem in other works in which olive trees of different ages, belonging to different cultivars or growing under different environments, were evaluated (Müller et al., 2015; Fausto et al., 2018; Sofo et al., 2019; Anguita-Maeso et al., 2020; Giampetruzzi et al., 2020), which strengthens the hypothesis that those genera may represent keystone olive xylem bacteria. More interestingly, some of these genera have already been reported or used as plant growth-promoting bacteria (Otieno et al., 2015; Subramanian et al., 2015) or proposed as biological control agents against V. dahliae (Berg et al., 2006; Aranda et al., 2011; Gómez-Lama Cabanás et al., 2018; Tao et al., 2020). Interestingly, the ratio Cutibacterium/Pseudomonas seemed to be an important factor associated to the plant propagation procedure. Thus, Pseudomonas spp. and Cutibacterium were present at high and low relative abundance, respectively, for in vitro-standard propagated plants, that lost resistance to D Vd, whereas for in vitro-adapted and nursery propagated plants, the opposite trend occurred. However, whereas little is known about the role of the genus Cutibacterium as a component of plant microbiomes, the beneficial functions of Pseudomonas spp. in plants have been widely reported for several crops, including olive (Mercado-Blanco et al., 2004; Weller, 2007; Loper et al., 2012).
The role of microorganisms in the biocontrol of Verticillium wilt diseases has been reported mostly on non-woody plant species such as cotton, potato, strawberry, or tomato (Azad et al., 1985; Nallanchakravarthula et al., 2014; Cao et al., 2016; Wei et al., 2019; Snelders et al., 2020) with few studies focused on woody hosts including olive (Mercado-Blanco et al., 2004; Aranda et al., 2011; Gómez-Lama Cabanás et al., 2018). However, the characterization of microbial communities inhabiting xylem vessels colonized by Vd has not been studied to date, despite some work done on other tree species or other vascular pathogens (Martín et al., 2015; Pérez-Martínez et al., 2018; Giampetruzzi et al., 2020; Vergine et al., 2020). To the best of our knowledge, the present study is the first to address this knowledge gap, by determining changes in xylem bacterial communities of a resistant olive clone after challenge inoculation with D Vd. Our results indicated that a significantly higher diversity and number of OTUs occur in Vd-inoculated plants regardless of the plant propagation method and success of stem vascular infection by the pathogen. Additionally, several genera appeared as unique in Vd-inoculated plants and Anoxybacillus represented keystone bacterial taxa that significantly increased its frequency after challenge inoculation with Vd in all treatments.
The significantly higher xylem microbial diversity in Vd-inoculated plants is in line with results from other studies involving vascular pathogens on woody crops. For instance, Deyett and Rolshausen (2019) found higher diversity in X. fastidiosa-infected vines as compared to healthier ones. Several of the unique or keystone bacterial genera (e.g., Acinetobacter, Comamonas, Caulobacter, Massilia, and Methylobacterium) detected in our study in the xylem of Vd-inoculated plants were also found in the xylem of other plant species such as banana, citrus, grapevine, and olive, so that those bacteria may be biomarkers of plant infection by vascular-plant pathogens (Araújo et al., 2002; Deyett and Rolshausen, 2019; Liu et al., 2019). The role of these bacterial genera in Vd-infected olive plants remains unknown, although several studies suggested that antifungal activity, induction of plant resistance, production of diverse cellulose-, hemicellulose-, lignin-, and starch-degrading enzymes might be involved in pathogen suppression and reduction of disease symptoms (Liu et al., 2007; Rajendran et al., 2009; Ardanov et al., 2012; Goh et al., 2013; Azevedo et al., 2016; AlMatar et al., 2017).
The general increase in alpha diversity and abundance of specific bacterial taxa observed in our study after challenging with D Vd may be explained by several hypotheses, including (i) a passive entry or direct recruitment of new bacterial species from the plant rhizosphere or soil, taking advantage of injuries caused during root infection and colonization by the pathogen (Ardanov et al., 2012; Liu et al., 2019); (ii) the secretion of specific molecules by the pathogen (such as effector proteins) with antimicrobial activity that modify host microbiome to facilitate host colonization (Snelders et al., 2020); and/or (iii) the pathogen provokes a series of host physiological responses that trigger multiplication and increase in abundance of a specific microbiome to cope with the pathogen infection in order to mitigate its effect (Ardanov et al., 2012; Hassani et al., 2018; Carrión et al., 2019). These hypotheses emphasize the need for better understanding of the changes occurring in xylem microbial communities in response to vascular infection by pathogens, in order to determine specifically activated disease-suppressive and/or plant-protecting microbiome-mediated activities in olive.
Our study provides new insights for the characterization of changes occurring in the xylem microbial communities of a wild olive genotype following inoculation with the vascular plant pathogen Vd. Also, it provides a quantitative and qualitative assessment of the effect of specific propagation methods where the attenuation and reduction of olive xylem microbiome is a unique approach described to date. We are aware of limitations in our study, in part because only some propagation methods and a single host genotype were evaluated. However, this present research is relevant for future studies on the olive xylem microbiome that may lead to identification of xylem-inhabiting bacteria potentially involved in host resistance and plant defense by acting as biocontrol agents against xylem-inhabiting pathogens. Deciphering the core olive xylem microbiome and their correlation with the host plant and its pathogens is a first critical step for exploiting the microbiome in order to enhance olive growth and health.
Data Availability Statement
Raw sequence data have been deposited in the Sequence Read Archive (SRA) database at the NCBI under BioProject accession number PRJNA679263.
Author Contributions
MA-M and BL conceived the research, performed statistical and bioinformatics analyses, interpreted the results, and wrote the manuscript. MA-M, JT-C, CO-G, DR-R, and EP-R prepared materials and equipment and performed the experiments. JN-C and RJ-D contributed to reviewing the manuscript and interpreted the results. All authors viewed the draft of the manuscript.
Funding
This study was funded by project AGL2016-75606-R (Programa Estatal de I+D Orientado a los Retos de la Sociedad from the Spanish Government, the Spanish State Research Agency, and FEDER-EU). MA-M is a recipient of a research fellowship BES-2017-082361 from the Spanish Ministry of Economy and Competitiveness.
Conflict of Interest
The authors declare that the research was conducted in the absence of any commercial or financial relationships that could be construed as a potential conflict of interest.
Supplementary Material
The Supplementary Material for this article can be found online at: https://www.frontiersin.org/articles/10.3389/fpls.2021.632689/full#supplementary-material
Supplementary Figure 1 | Effect of inoculation with the defoliating pathotype of Verticillium dahliae in “Picual,” “Ac-15,” and “Ac-18,” plants obtained using in vitro-standard, in vitro-adapted, and nursery propagation methods. “Picual” and “Ac-15” were used as positive control to determine the inoculation success and the development of the disease. Note the defoliation of green leaves observed for in vitro-standard propagated “Ac-18” plants. Picture was taken 44 days after inoculation with the pathogen.
Supplementary Figure 2 | Richness rarefaction curves at OTU taxonomic level in olive xylem from Verticillium dahliae (Vd)-inoculated and non-inoculated (NI) “Ac-18” plants following in vitro (standard and adapted) and nursery propagation methods. Error bars represent standard error of six values.
Supplementary Figure 3 | General prevalence Venn diagram showing the unique and shared bacteria at different taxonomy ranks in olive xylem from Verticillium dahliae (Vd)-inoculated and non-inoculated (NI) “Ac-18” plants following in vitro (standard and adapted) and nursery propagation methods.
Footnotes
References
AlMatar, M., Eldeeb, M., Makky, E. A., Köksal, F., Var, I., and Kayar, B. (2017). Are there any other compounds isolated from Dermacoccus spp at all? Curr. Microbiol. 74, 132–144. doi: 10.1007/s00284-016-1152-3
Anguita-Maeso, M., Olivares-García, C., Haro, C., Imperial, J., Navas-Cortés, J. A., and Landa, B. B. (2020). Culture-dependent and culture-independent characterization of the olive xylem microbiota: effect of sap extraction methods. Front. Plant Sci. 10:1708. doi: 10.3389/fpls.2019.01708
Antoniou, A., Tsolakidou, M.-D., Stringlis, I. A., and Pantelides, I. S. (2017). Rhizosphere microbiome recruited from a suppressive compost improves plant fitness and increases protection against vascular wilt pathogens of tomato. Front. Plant Sci. 8:2022. doi: 10.3389/fpls.2017.02022
Aranda, S., Montes-Borrego, M., Jiménez-Díaz, R. M., and Landa, B. B. (2011). Microbial communities associated with the root system of wild olives (Olea europaea L. subsp. europaea var. sylvestris) are good reservoirs of bacteria with antagonistic potential against Verticillium dahliae. Plant Soil 343, 329–345. doi: 10.1007/s11104-011-0721-2
Araújo, W. L., Marcon, J., Maccheroni, W. J., Van Elsas, J. D., Van Vuurde, J. W. L., and Azevedo, J. L. (2002). Diversity of endophytic bacterial populations and their interaction with Xylella fastidiosa in citrus plants. Appl. Environ. Microbiol. 68, 4906–4914. doi: 10.1128/aem.68.10.4906-4914.2002
Ardanov, P., Sessitsch, A., Häggman, H., Kozyrovska, N., and Pirttilä, A. M. (2012). Methylobacterium-induced endophyte community changes correspond with protection of plants against pathogen attack. PLoS One 7:e46802. doi: 10.1371/journal.pone.0046802
Azad, H. R., Davis, J. R., Schnathorst, W. C., and Kado, C. I. (1985). Relationships between rhizoplane and rhizosphere bacteria and verticillium wilt resistance in potato. Arch. Microbiol. 140, 347–351. doi: 10.1007/BF00446976
Azevedo, J., Araújo, W. L., and Lacava, P. T. (2016). The diversity of citrus endophytic bacteria and their interactions with Xylella fastidiosa and host plants. Genet. Mol. Biol. 39, 476–491. doi: 10.1590/1678-4685-GMB-2016-0056
Azevedo, J. L., Maccheroni Junior, W., Pereira, J. O., and Araújo, W. L. (2000). Endophytic microorganisms: a review on insect control and recent advances on tropical plants. Electron. J. Biotechnol. 3, 40–65. doi: 10.2225/vol3-issue1-fulltext-4
Báidez, A. G., Gómez, P., Del Río, J. A., and Ortuño, A. (2007). Dysfunctionality of the xylem in Olea europaea L. Plants associated with the infection process by Verticillium dahliae Kleb. Role of phenolic compounds in plant defense mechanism. J. Agric. Food Chem. 55, 3373–3377. doi: 10.1021/jf063166d
Benjamini, Y., and Hochberg, Y. (1995). Controlling the false discovery rate: a practical and powerful approach to multiple testing. J. R. Stat. Soc. Ser. B 57, 289–300. doi: 10.1111/j.2517-6161.1995.tb02031.x
Berg, G. (2009). Plant–microbe interactions promoting plant growth and health: perspectives for controlled use of microorganisms in agriculture. Appl. Microbiol. Biotechnol. 84, 11–18. doi: 10.1007/s00253-009-2092-7
Berg, G., Grube, M., Schloter, M., and Smalla, K. (2014). Unraveling the plant microbiome: looking back and future perspectives. Front. Microbiol. 5:148. doi: 10.3389/fmicb.2014.00148
Berg, G., Opelt, K., Zachow, C., Lottmann, J., Götz, M., Costa, R., et al. (2006). The rhizosphere effect on bacteria antagonistic towards the pathogenic fungus Verticillium differs depending on plant species and site. FEMS Microbiol. Ecol. 56, 250–261. doi: 10.1111/j.1574-6941.2005.00025.x
Bilodeau, G. J., Koike, S. T., Uribe, P., and Martin, F. N. (2012). Development of an assay for rapid detection and quantification of Verticillium dahliae in soil. Phytopathology 102, 331–343. doi: 10.1094/PHYTO-05-11-0130
Bolyen, E., Rideout, J. R., Dillon, M. R., Bokulich, N. A., Abnet, C., Al-Ghalith, G. A., et al. (2019). Reproducible, interactive, scalable and extensible microbiome data science using QIIME 2. Nat. Biotechnol. 37, 852–857. doi: 10.1038/s41587-019-0209-9
Bubici, G., and Cirulli, M. (2012). Control of Verticillium wilt of olive by resistant rootstocks. Plant Soil 352, 363–376. doi: 10.1007/s11104-011-1002-9
Caballero, J., and Del Río, C. (2010). “Propagation methods. Olive grow,” in Pendle Hill, eds D. Barranco, R. Fernández-Escobar, and L. Rallo (Australia: RIRDC), 83–112. doi: 10.1353/syl.2003.0004
Calderon, R., Lucena, C., Trapero-Casas, J. L., Zarco-Tejada, P. J., and Navas-Cortes, J. A. (2014). Soil temperature determines the reaction of olive cultivars to Verticillium dahliae pathotypes. PLoS One 9:e110664. doi: 10.1371/journal.pone.0110664
Cao, P., Liu, C., Sun, P., Fu, X., Wang, S., Wu, F., et al. (2016). An endophytic Streptomyces sp. strain DHV3-2 from diseased root as a potential biocontrol agent against Verticillium dahliae and growth elicitor in tomato (Solanum lycopersicum). Antonie Van Leeuwenhoek 109, 1573–1582. doi: 10.1007/s10482-016-0758-6
Caporaso, J. G., Kuczynski, J., Stombaugh, J., Bittinger, K., Bushman, F. D., Costello, E. K., et al. (2010). QIIME allows analysis of high-throughput community sequencing data. Nat. Methods 7:335. doi: 10.1038/nmeth.f.303
Carrión, V. J., Perez-Jaramillo, J., Cordovez, V., Tracanna, V., de Hollander, M., Ruiz-Buck, D., et al. (2019). Pathogen-induced activation of disease-suppressive functions in the endophytic root microbiome. Science 366, 606–612. doi: 10.1126/science.aaw9285
Colella, C., Miacola, C., Amenduni, M., D’Amico, M., Bubici, G., and Cirulli, M. (2008). Sources of verticillium wilt resistance in wild olive germplasm from the Mediterranean region. Plant Pathol. 57, 533–539. doi: 10.1111/j.1365-3059.2007.01785.x
Compant, S., Saikkonen, K., Mitter, B., Campisano, A., and Mercado-Blanco, J. (2016). Editorial special issue: soil, plants and endophytes. Plant Soil 405, 1–11. doi: 10.1007/s11104-016-2927-9
D’Attoma, G., Morelli, M., Saldarelli, P., Saponari, M., Giampetruzzi, A., Boscia, D., et al. (2019). Ionomic differences between susceptible and resistant olive cultivars infected by Xylella fastidiosa in the outbreak area of Salento. Italy. Pathogens 8:272. doi: 10.3390/pathogens8040272
de la O Leyva-Pérez, M., Jiménez-Ruiz, J., Gómez-Lama Cabanás, C., Valverde-Corredor, A., Barroso, J. B., Luque, F., et al. (2017). Tolerance of olive (Olea europaea) cv Frantoio to Verticillium dahliae relies on both basal and pathogen-induced differential transcriptomic responses. New Phytol. 217, 671–686. doi: 10.1111/nph.14833
Deyett, E., and Rolshausen, P. E. (2019). Temporal dynamics of the sap microbiome of grapevine under high Pierce’s disease pressure. Front. Plant Sci. 10:1246. doi: 10.3389/fpls.2019.01246
Fabbri, A., Lambardi, M., and Ozden-Tokatli, Y. (2009). Olive Breeding BT - Breeding Plantation Tree Crops: Tropical Species. New York, NY: Springer, 423–465. doi: 10.1007/978-0-387-71201-7_12
Fausto, C., Mininni, A. N., Sofo, A., Crecchio, C., Scagliola, M., Dichio, B., et al. (2018). Olive orchard microbiome: characterisation of bacterial communities in soil-plant compartments and their comparison between sustainable and conventional soil management systems. Plant Ecol. Divers. 11, 597–610. doi: 10.1080/17550874.2019.1596172
Gharbi, Y., Barkallah, M., Bouazizi, E., Gdoura, R., and Triki, M. A. (2017a). Differential biochemical and physiological responses of two olive cultivars differing by their susceptibility to the hemibiotrophic pathogen Verticillium dahliae. Physiol. Mol. Plant Pathol. 97, 30–39. doi: 10.1016/j.pmpp.2016.12.001
Gharbi, Y., Barkallah, M., Bouazizi, E., Hibar, K., Gdoura, R., and Triki, M. A. (2017b). Lignification, phenols accumulation, induction of PR proteins and antioxidant-related enzymes are key factors in the resistance of Olea europaea to Verticillium wilt of olive. Acta Physiol. Plant. 39:43. doi: 10.1007/s11738-016-2343-z
Giampetruzzi, A., Baptista, P., Morelli, M., Cameirão, C., Lino Neto, T., Costa, D., et al. (2020). Differences in the endophytic microbiome of olive cultivars infected by Xylella fastidiosa across seasons. Pathogens 9:732. doi: 10.3390/pathogens9090723
Goh, K. M., Kahar, U. M., Chai, Y. Y., Chong, C. S., Chai, K. P., Ranjani, V., et al. (2013). Recent discoveries and applications of Anoxybacillus. Appl. Microbiol. Biotechnol. 97, 1475–1488. doi: 10.1007/s00253-012-4663-2
Gómez-Lama Cabanás, C., Legarda, G., Ruano-Rosa, D., Pizarro-Tobías, P., Valverde-Corredor, A., Niqui, J. L., et al. (2018). Indigenous Pseudomonas spp. strains from the olive (Olea europaea L.) rhizosphere as effective biocontrol agents against Verticillium dahliae: from the host roots to the bacterial genomes. Front. Microbiol. 9:277. doi: 10.3389/fmicb.2018.00277
Gramaje, D., Pérez-Serrano, V., Montes-Borrego, M., Navas-Cortés, J. A., Jiménez-Díaz, R. M., and Landa, B. B. (2013). A comparison of Real-Time PCR protocols for the quantitative monitoring of asymptomatic olive infections by Verticillium dahliae pathotypes. Phytopathology 103, 1058–1068. doi: 10.1094/PHYTO-11-12-0312-R
Hardoim, P. R., van Overbeek, L. S., Berg, G., Pirttilä, A. M., Compant, S., Campisano, A., et al. (2015). The hidden world within plants: ecological and evolutionary considerations for defining functioning of microbial endophytes. Microbiol. Mol. Biol. Rev. 79, 293–320. doi: 10.1128/MMBR.00050-14
Hassani, M. A., Durán, P., and Hacquard, S. (2018). Microbial interactions within the plant holobiont. Microbiome 6:58. doi: 10.1186/s40168-018-0445-0
Hoagland, D. R., and Arnon, D. I. (1950). The water-culture method for growing plants without soil. Circ. Calif. Agric. Exp. Stn. 347:32.
Hong, C. E., and Park, J. M. (2016). Endophytic bacteria as biocontrol agents against plant pathogens: current state-of-the-art. Plant Biotechnol. Rep. 10, 353–357. doi: 10.1007/s11816-016-0423-6
Jiménez-Díaz, R. M., Cirulli, M., Bubici, G., del Mar Jiménez-Gasco, M., Antoniou, P. P., and Tjamos, E. C. (2011). Verticillium Wilt, a major threat to olive production: current status and future prospects for its management. Plant Dis. 96, 304–329. doi: 10.1094/PDIS-06-11-0496
Jiménez-Fernández, D., Trapero-Casas, J. L., Landa, B. B., Navas-Cortés, J. A., Bubici, G., Cirulli, M., et al. (2016). Characterization of resistance against the olive-defoliating Verticillium dahliae pathotype in selected clones of wild olive. Plant Pathol. 65, 1279–1291. doi: 10.1111/ppa.12516
Landa, B. B., Pérez, A. G., Luaces, P., Montes-Borrego, M., Navas-Cortés, J. A., and Sanz, C. (2019). Insights into the effect of Verticillium dahliae defoliating-pathotype infection on the content of phenolic and volatile compounds related to the sensory properties of virgin olive oil. Front. Plant Sci. 10:232. doi: 10.3389/fpls.2019.00232
Liu, C. H., Chen, X., Liu, T. T., Lian, B., Gu, Y., Caer, V., et al. (2007). Study of the antifungal activity of Acinetobacter baumannii LCH001 in vitro and identification of its antifungal components. Appl. Microbiol. Biotechnol. 76, 459–466. doi: 10.1007/s00253-007-1010-0
Liu, Y., Zhu, A., Tan, H., Cao, L., and Zhang, R. (2019). Engineering banana endosphere microbiome to improve Fusarium wilt resistance in banana. Microbiome 7:74. doi: 10.1186/s40168-019-0690-x
Loper, J. E., Hassan, K. A., Mavrodi, D. V., Davis, E. W. II, Lim, C. K., Shaffer, B. T., et al. (2012). Comparative genomics of plant-associated Pseudomonas spp.: insights into diversity and inheritance of traits involved in multitrophic interactions. PLoS Genet. 8:e1002784. doi: 10.1371/journal.pgen.1002784
López-Escudero, F. J., del Río, C., Caballero, J. M., and Blanco-López, M. A. (2004). Evaluation of olive cultivars for resistance to Verticillium dahliae. Eur. J. Plant Pathol. 110, 79–85. doi: 10.1023/B:EJPP.0000010150.08098.2d
López-Escudero, F. J., Mercado-Blanco, J., Roca, J. M., Valverde-Corredor, A., and Blanco-López, M. (2010). Verticillium wilt of olive in the Guadalquivir Valley (southern Spain): relations with some agronomical factors and spread of Verticillium dahliae. Phytopathol. Mediterr. 49, 370–380. doi: 10.14601/Phytopathol_Mediterr-3154
Lozupone, C., and Knight, R. (2005). UniFrac: a new phylogenetic method for comparing microbial communities. Appl. Environ. Microbiol. 71, 8228–8235. doi: 10.1128/AEM.71.12.8228-8235.2005
Luvisi, A., Aprile, A., Sabella, E., Vergine, M., Nicolì, F., Nutricati, E., et al. (2017). Xylella fastidiosa subsp. pauca (CoDiRO strain) infection in four olive (Olea europaea L.) cultivars: profile of phenolic compounds in leaves and progression of leaf scorch symptoms. Phytopathol. Mediterr. 56, 259–273. doi: 10.14601/PHYTOPATHOL_MEDITERR-20578
Mangiafico, S. (2020). rcompanion: Functions to Support Extension Education Program Evaluation. R Package Version 2.3.25.
Markakis, E. A., Tjamos, S. E., Antoniou, P. P., Roussos, P. A., Paplomatas, E. J., and Tjamos, E. C. (2010). Phenolic responses of resistant and susceptible olive cultivars induced by defoliating and nondefoliating Verticillium dahliae pathotypes. Plant Dis. 94, 1156–1162. doi: 10.1094/PDIS-94-9-1156
Martín, J. A., Macaya-Sanz, D., Witzell, J., Blumenstein, K., and Gil, L. (2015). Strong in vitro antagonism by elm xylem endophytes is not accompanied by temporally stable in planta protection against a vascular pathogen under field conditions. Eur. J. Plant Pathol. 142, 185–196. doi: 10.1007/s10658-015-0602-2
Martos-Moreno, C., Lòpez-Escudero, F. J., and Blanco-Lòpez, M. A. (2006). Resistance of olive cultivars to the defoliating pathotype of Verticillium dahliae. HortScience 41, 1313–1316. doi: 10.21273/HORTSCI.41.5.1313
Mercado-Blanco, J., Rodrìguez-Jurado, D., Hervás, A., and Jiménez-Dìaz, R. M. (2004). Suppression of Verticillium wilt in olive planting stocks by root-associated fluorescent Pseudomonas spp. Biol. Control 30, 474–486. doi: 10.1016/j.biocontrol.2004.02.002
Müller, H., Berg, C., Landa, B. B., Auerbach, A., Moissl-Eichinger, C., and Berg, G. (2015). Plant genotype-specific archaeal and bacterial endophytes but similar Bacillus antagonists colonize Mediterranean olive trees. Front. Microbiol. 6:138. doi: 10.3389/fmicb.2015.00138
Nallanchakravarthula, S., Mahmood, S., Alström, S., and Finlay, R. D. (2014). Influence of soil type, cultivar and Verticillium dahliae on the structure of the root and rhizosphere soil fungal microbiome of strawberry. PLoS One 9:e111455. doi: 10.1371/journal.pone.0111455
Narváez, I., Martín, C., Jiménez-Díaz, R. M., Mercado, J. A., and Pliego-Alfaro, F. (2019). Plant regeneration via somatic embryogenesis in mature wild olive genotypes resistant to the defoliating pathotype of Verticillium dahliae. Front. Plant Sci. 10:1471. doi: 10.3389/fpls.2019.01471
Narváez, I., Pliego Prieto, C., Palomo-Ríos, E., Fresta, L., Jiménez-Díaz, R. M., Trapero-Casas, J. L., et al. (2020). Heterologous expression of the AtNPR1 gene in olive and its effects on fungal tolerance. Front. Plant Sci. 11:308. doi: 10.3389/fpls.2020.00308
Navas-Cortés, J. A., Landa, B. B., Mercado-Blanco, J., Trapero-Casas, J. L., Rodríguez-Jurado, D., and Jiménez-Díaz, R. M. (2008). Spatiotemporal analysis of spread of infections by Verticillium dahliae pathotypes within a high tree density olive orchard in southern Spain. Phytopathology 98, 167–180. doi: 10.1094/PHYTO-98-2-0167
Ostos, E., Garcia-Lopez, M. T., Porras, R., Lopez-Escudero, F. J., Trapero-Casas, A., Michailides, T. J., et al. (2020). Effect of cultivar resistance and soil management on spatial–temporal development of Verticillium wilt of olive: a long-term study. Front. Plant Sci. 11:1595. doi: 10.3389/fpls.2020.584496
Otieno, N., Lally, R., Kiwanuka, S., Lloyd, A., Ryan, D., Germaine, K., et al. (2015). Plant growth promotion induced by phosphate solubilizing endophytic Pseudomonas isolates. Front. Microbiol. 6:745. doi: 10.3389/fmicb.2015.00745
Pérez-Martínez, J., Ploetz, R. C., and Konkol, J. L. (2018). Significant in vitro antagonism of the laurel wilt pathogen by endophytic fungi from the xylem of avocado does not predict their ability to control the disease. Plant Pathol. 67, 1768–1776. doi: 10.1111/ppa.12878
Rajendran, P., Sundaram, S. P., and Kumutha, K. (2009). In vitro biocontrol activity of Methylobacterium Extorquens against fungal pathogens. Int. J. Plant Prot. 2, 59–62. doi: 10.13140/2.1.3086.0163
Rognes, T., Flouri, T., Nichols, B., Quince, C., and Mahe, F. (2016). VSEARCH: a versatile open source tool for metagenomics. PeerJ 4:e2584. doi: 10.7717/peerj.2584
Roussos, P. A., and Pontikis, C. A. (2002). In vitro propagation of olive (Olea europaea L.) cv. Koroneiki. Plant Growth Regul. 37, 295–304. doi: 10.1023/A:1020824330589
Ryan, R. P., Germaine, K., Franks, A., Ryan, D. J., and Dowling, D. N. (2008). Bacterial endophytes: recent developments and applications. FEMS Microbiol. Lett. 278, 1–9. doi: 10.1111/j.1574-6968.2007.00918.x
Sabella, E., Aprile, A., Genga, A., Siciliano, T., Nutricati, E., Nicolì, F., et al. (2019). Xylem cavitation susceptibility and refilling mechanisms in olive trees infected by Xylella fastidiosa. Sci. Rep. 9:9602. doi: 10.1038/s41598-019-46092-0
Sabella, E., Luvisi, A., Aprile, A., Negro, C., Vergine, M., Nicolì, F., et al. (2017). Xylella fastidiosa induces differential expression of lignification related-genes and lignin accumulation in tolerant olive trees cv. Leccino. J. Plant Physiol. 220, 60–68. doi: 10.1016/j.jplph.2017.10.007
Schneider, K., van der Werf, W., Cendoya, M., Mourits, M., Navas-Cortés, J. A., Vicent, A., et al. (2020). Impact of Xylella fastidiosa subspecies Pauca in European olives. Proc. Natl. Acad. Sci. U.S.A. 117, 9250–9259. doi: 10.1073/pnas.1912206117
Segata, N., Izard, J., Waldron, L., Gevers, D., Miropolsky, L., Garrett, W. S., et al. (2011). Metagenomic biomarker discovery and explanation. Genome Biol. 12, R60–R60. doi: 10.1186/gb-2011-12-6-r60
Snelders, N. C., Rovenich, H., Petti, G. C., Rocafort, M., van den Berg, G. C. M., Vorholt, J. A., et al. (2020). Microbiome manipulation by a soil-borne fungal plant pathogen using effector proteins. Nat. Plants 6, 1365–1374. doi: 10.1038/s41477-020-00799-5
Sofo, A., Mininni, A. N., Fausto, C., Scagliola, M., Crecchio, C., Xiloyannis, C., et al. (2019). Evaluation of the possible persistence of potential human pathogenic bacteria in olive orchards irrigated with treated urban wastewater. Sci. Total Environ. 658, 763–767. doi: 10.1016/j.scitotenv.2018.12.264
Subramanian, P., Kim, K., Krishnamoorthy, R., Sundaram, S., and Sa, T. (2015). Endophytic bacteria improve nodule function and plant nitrogen in soybean on co-inoculation with Bradyrhizobium japonicum MN110. Plant Growth Regul. 76, 327–332. doi: 10.1007/s10725-014-9993-x
Tao, X., Zhang, H., Gao, M., Li, M., Zhao, T., and Guan, X. (2020). Pseudomonas species isolated via high-throughput screening significantly protect cotton plants against Verticillium wilt. AMB Express 10:193. doi: 10.1186/s13568-020-01132-1
Trapero, C., Díez, C. M., Rallo, L., Barranco, D., and López-Escudero, F. J. (2013). Effective inoculation methods to screen for resistance to Verticillium wilt in olive. Sci. Hortic. 162, 252–259. doi: 10.1016/j.scienta.2013.08.036
Trapero, C., Serrano, N., Arquero, O., Del Río, C., Trapero, A., and López-Escudero, F. J. (2012). Field resistance to Verticillium wilt in selected olive cultivars grown in two naturally infested soils. Plant Dis. 97, 668–674. doi: 10.1094/PDIS-07-12-0654-RE
Vannier, N., Mony, C., Bittebiere, A.-K., Michon-Coudouel, S., Biget, M., and Vandenkoornhuyse, P. (2018). A microorganisms’ journey between plant generations. Microbiome 6:79. doi: 10.1186/s40168-018-0459-7
Vergine, M., Meyer, J. B., Cardinale, M., Sabella, E., Hartmann, M., Cherubini, P., et al. (2020). The Xylella fastidiosa-resistant olive cultivar “Leccino” has stable endophytic microbiota during the olive quick decline syndrome (OQDS). Pathogens 9:35. doi: 10.3390/pathogens9010035
Vidoy-Mercado, I., Imbroda-Solano, I., Barceló-Muñoz, A., and Pliego-Alfaro, F. (2012). Differential in vitro behaviour of the Spanish olive (Olea europaea L.) cultivars ‘Arbequina’ and ‘Picual.’. Acta Hortic. 949, 27–30. doi: 10.17660/ActaHortic.2012.949.1
Vorholt, J. A., Vogel, C., Carlström, C. I., and Müller, D. B. (2017). Establishing causality: opportunities of synthetic communities for plant microbiome research. Cell Host Microbe 22, 142–155. doi: 10.1016/j.chom.2017.07.004
Wei, F., Zhao, L., Xu, X., Feng, H., Shi, Y., Deakin, G., et al. (2019). Cultivar-dependent variation of the cotton rhizosphere and endosphere microbiome under field conditions. Front. Plant Sci. 10:1659. doi: 10.3389/fpls.2019.01659
Weller, D. M. (2007). Pseudomonas biocontrol agents of soilborne pathogens: looking back over 30 years. Phytopathology 97, 250–256. doi: 10.1094/PHYTO-97-2-0250
Keywords: microbiome, xylem, olive, Verticillium dahliae, micropropagation, host resistance
Citation: Anguita-Maeso M, Trapero-Casas JL, Olivares-García C, Ruano-Rosa D, Palomo-Ríos E, Jiménez-Díaz RM, Navas-Cortés JA and Landa BB (2021) Verticillium dahliae Inoculation and in vitro Propagation Modify the Xylem Microbiome and Disease Reaction to Verticillium Wilt in a Wild Olive Genotype. Front. Plant Sci. 12:632689. doi: 10.3389/fpls.2021.632689
Received: 23 November 2020; Accepted: 01 February 2021;
Published: 03 March 2021.
Edited by:
Roeland Lucas Berendsen, Utrecht University, NetherlandsReviewed by:
Sotiris Tjamos, Agricultural University of Athens, GreeceAntonio José Fernández-González, Consejo Superior de Investigaciones Científicas (CSIC), Spain
Wu Xiong, Utrecht University, Netherlands
Hanna Rövenich, University of Cologne, Germany
Copyright © 2021 Anguita-Maeso, Trapero-Casas, Olivares-García, Ruano-Rosa, Palomo-Ríos, Jiménez-Díaz, Navas-Cortés and Landa. This is an open-access article distributed under the terms of the Creative Commons Attribution License (CC BY). The use, distribution or reproduction in other forums is permitted, provided the original author(s) and the copyright owner(s) are credited and that the original publication in this journal is cited, in accordance with accepted academic practice. No use, distribution or reproduction is permitted which does not comply with these terms.
*Correspondence: Blanca B. Landa, YmxhbmNhLmxhbmRhQGNzaWMuZXM=