Corrigendum: Partial Substitution of K by Na Alleviates Drought Stress and Increases Water Use Efficiency in Eucalyptus Species Seedlings
- 1Stable Isotope Laboratory, Center for Nuclear Energy in Agriculture, University of São Paulo, Piracicaba, Brazil
- 2Applied Ecology Laboratory, Department of Forest Sciences, Luiz de Queiroz College of Agriculture, University of São Paulo, Piracicaba, Brazil
- 3Federal Institute of Mato Grosso Do Sul, Nova Andradina, Brazil
- 4Institute of Forest Research and Studies, Piracicaba, Brazil
Eucalyptus, the most widely planted tree genus worldwide, is frequently cultivated in soils with low water and nutrient availability. Sodium (Na) can substitute some physiological functions of potassium (K), directly influencing plants’ water status. However, the extent to which K can be replaced by Na in drought conditions remains poorly understood. A greenhouse experiment was conducted with three Eucalyptus genotypes under two water conditions (well-watered and water-stressed) and five combination rates of K and Na, representing substitutions of 0/100, 25/75, 50/50, 75/25, and 100/0 (percentage of Na/percentage of K), to investigate growth and photosynthesis-related parameters. This study focused on the positive effects of Na supply since, depending on the levels applied, the Na supply may induce plants to salinity stress (>100 mM of NaCl). Plants supplied with low to intermediate K replacement by Na reduced the critical level of K without showing symptoms of K deficiency and provided higher total dry matter (TDM) than those Eucalyptus seedlings supplied only with K in both water conditions. Those plants supplied with low to intermediate K replacement by Na had improved CO2 assimilation (A), stomatal density (Std), K use efficiency (UEK), and water use efficiency (WUE), in addition to reduced leaf water potential (Ψw) and maintenance of leaf turgidity, with the stomata partially closed, indicated by the higher values of leaf carbon isotope composition (δ13C‰). Meanwhile, combination rates higher than 50% of K replacement by Na led to K-deficient plants, characterized by the lower values of TDM, δ13C‰, WUE, and leaf K concentration and higher leaf Na concentration. There was positive evidence of partial replacement of K by Na in Eucalyptus seedlings; meanwhile, the ideal percentage of substitution increased according to the drought tolerance of the species (Eucalyptus saligna < Eucalyptus urophylla < Eucalyptus camaldulensis).
Introduction
The genus Eucalyptus plays an important role in meeting the growing global wood demand (Paquette and Messier, 2010). However, it is largely dependent on fertilization (Smethurst, 2010) and vulnerable to drought, the main limiting factors for plant growth (Gonçalves et al., 2017). Against the background of a changing climate, the intensity, and frequency of drought will increase in the near future (IPCC, 2019). Adequate management strategies to improve tolerance to water deficit, such as enhancing plant water use efficiency (WUE), are necessary to mitigate the adverse impacts of drought (Asensio et al., 2020). Stomatal closure by osmotic adjustment (Oddo et al., 2011) is a key factor to mitigating the negative impacts of drought, avoiding excessive water loss at the expense of photosynthetic rate restrictions (Anjum et al., 2011) and turgor loss, decreasing cell growth (Steudle, 2000). Among the macronutrients, potassium (K) is one of the most required nutrients for Eucalyptus, enhancing yields by 50% compared to plants under K deficiency (Battie-Laclau et al., 2013). Changes in cell turgor involve the controlled uptake of K and other ions, mediated by voltage-gated K+ transporters at the cellular plasma membrane, inducing solute accumulation (Shabala and Lew, 2002), water uptake from the apoplast, and, finally, stomata aperture (Ahmad and Maathuis, 2014). Thus, the ion flux in and out of the guard cells mediates stomatal aperture and closure (Kim et al., 2010).
Sodium (Na), a beneficial element, is absorbed and taken up as Na+ and might replace K partially as an osmotically active solute, stimulating cell elongation and improving stomatal control, which, in turn, contributes to maintain cell turgor (Jeschke, 1977), directly affecting plant WUE (Mateus et al., 2019). Additionally, some ATPases require both K and Na for maximal activity (Marschner, 2012). This occurs due to the similarity between the hydrated ionic radii of Na (0.358 nm) and K (0.331 nm) (Marschner, 2012). Despite the well-known importance of K, the effects of Na application on water balance are not well studied (Gattward et al., 2012). A major benefit of replacing K fertilization by Na is the relatively lower cost of NaCl compared to KCl, bringing greater profitability to the forest sector; besides, nutrient interaction may be a strategy to increase K use efficiency and decrease the critical K leaf concentration (Laclau et al., 2003). K deficiency reduces plant tolerance to water deficit due to its influence on plant osmoregulation, playing a critical role in stress avoidance and adaptation (Tränkner et al., 2018). It also reduces the photosynthetic efficiency (Jin et al., 2011), consequently affecting carbon partitioning to wood production, influencing the plant’s anatomical composition (Epron et al., 2012). Moreover, maximum growth can be reached with concomitant application of Na and K, in addition to improving stomatal conductance (gs) and mitigating the anatomical and biochemical deficiencies of plants caused by low K availability (Battie-Laclau et al., 2013; Mateus et al., 2019).
A comprehensive literature indicates the benefits of Na supply to plants (Hampe and Marschner, 1982; Subbarao et al., 1999; Martínez et al., 2005; Idowu and Aduayi, 2006; Ivahupa et al., 2006; Ma et al., 2011; Wakeel et al., 2011; Kronzucker et al., 2013; Erel et al., 2014; Krishnasamy et al., 2014; Pi et al., 2014), as also in drought adaptations of halophyte plants (Lv et al., 2012; Yue et al., 2012; Xi et al., 2018). Non-halophytic plants, such as Eucalyptus, although salt-sensitive (Pardo and Quintero, 2002), are also able to utilize Na to some extent (Subbarao et al., 2003; Mateus et al., 2019). Depending on the species and the levels applied, the Na supply may be toxic for plants (Kronzucker et al., 2013), which in turn demands more attention with regard to using Na in fertilizing non-halophytes in order to fulfill plants’ nutritional requirements under K deficiency (Mateus et al., 2019). Plants can behave differently under nutritional stress conditions and vary in nutritional efficiency (Pita-Barbosa et al., 2016), which allows some species to grow more at the highest levels of Na (Subbarao et al., 2003). However, despite the great variety of studies involving nutrient application, plant growth, and water deficit (Müller et al., 2017), studies involving K and Na use efficiency of different species and water regimes are still scarce. Thus, this study aimed to evaluate the partial replacement of K by Na and its impacts on water use and K use efficiency in three useful Eucalyptus species under different water conditions, investigating to what extent Na can substitute K and attenuate the effects of drought.
Materials and Methods
Experimental Design and Growth Conditions
The experiment was carried out in a greenhouse at the Center for Nuclear Energy in Agriculture (CENA-USP) in Piracicaba, São Paulo State, Brazil, from July to November 2018. Plants were grown at temperatures between 18 and 32°C (mean of 25°C) with an average relative humidity of 65%. Three Eucalyptus species with different levels of drought tolerance (Eucalyptus saligna Sm., drought sensitive; Eucalyptus urophylla S.T. Blake, moderate tolerance; and Eucalyptus camaldulensis Dehn., drought tolerant) (Gonçalves et al., 2017) of approximately 90 days old and 30 cm in height, germinated from seeds obtained from the Institute of Forest Research and Studies (IPEF, Brazil), were transplanted into individual plastic pots (5 kg) containing Oxisol soil (16% clay, 5% silt, and 79% sand), collected from the top layer at the Itatinga Experimental Station, Itatinga, São Paulo State, Brazil. The physiochemical characteristics were: pH = 4.2; organic matter = 25 g dm–3; P = 4 mg dm–3; K = 0.3 mmolc dm–3; Ca = 1 mmolc dm–3; Mg = 1 mmolc dm–3; H + Al = 25 mmolc dm–3; Al = 3 mmolc dm–3; B = 0.14 mg dm–3; Cu = 0.6 mg dm–3; Fe = 33 mg dm–3; Mn = 0.8 mg dm–3; and Zn = 0.8 mg dm–3.
Based on the soil K critical level (<1.20 mmolc dm–3 of K) for Eucalyptus to respond to potassium fertilization, K was replaced by Na (as NaCl), representing substitutions of 0/100, 25/75, 50/50, 75/25, and 100/0 (percentage of Na/percentage of K) for 120 days. Thus, the treatments consisted of five combinations of Na and K application rates (0/0.90, 0.22/0.67, 0.44/0.44, 0.67/0.22, and 0.90/0 mmolc dm–3 of Na/millimoles of charge per cubic decimeter of K), which, when added to the soil K content, reached the soil K critical level (1.20 mmolc dm–3 of K). The rates 0 and 0.90 mmolc dm–3 of Na represented the control (solely K supplied plants) and the K deficiency treatments, respectively. We would like to emphasize that the Na rates employed in studies regarding salinity-induced stress in plants are higher than those used hereby. For instance, Zhang et al. (2016) investigated salinity-induced stress on wheat seedlings’ growth in nutrient solution by adding a NaCl rate of 150 mM, while Quais et al. (2020) characterized the mechanisms of the underlying interactions between rice plants and brown planthopper under salinity stress (100 mM salinity level). According to Madsen and Mulligan (2006), the emergence of Eucalyptus citriodora, E. camaldulensis, Eucalyptus populnea, and Acacia salicina was substantially reduced by adding 100 mM of NaCl, while the survival of established plants was reduced only at 300 and 400 mM of NaCl.
In addition to the treatments with K and Na, all samples received the following complementary fertilization: 135 mg dm–3 of N plus 20 mg dm–3 of N in coverage at 2 months after the onset of the treatments, 300 mg kg–1 of P, 92 mg kg–1 of Ca, 7.2 mg kg–1 of Mg (reaching 7 mmolc dm–3 in a Ca+2/Mg+2 ratio of 4:1), 45 mg kg–1 of S, 0.82 mg kg–1 of B, 4.0 mg kg–1 of Zn, 3.66 mg kg–1 of Mn, 1.55 mg kg–1 of Fe, 1.39 mg kg–1 Cu, and 0.20 mg kg–1 of Mo, facilitating adequate plant development (Novais et al., 1991). The sources of the elements used were as follows: (NH4)H2PO4, CaCO3, MgCO3, elementary S, CuSO4.5H2O, ZnSO4.7H2O, FeSO4.7H2O, H3BO3, MnSO4.H2O, and MoO3.H2O.
The plants were exposed to two water conditions starting 60 days after the onset of the treatments: well-watered (WW) and water stress (WS) conditions, simulating adequate water availability and drought conditions, respectively. The soil relative water content (SRWC) of both water conditions was controlled daily by the gravimetric method using irrigation with deionized water up to 80 and 35% of the field capacity under WW and WS, respectively. Weighing and watering were conducted on a daily basis at dusk until the pots reached their corresponding target-adapted SRWC (Equation 1) (Xu et al., 2009); every 15 days, two plants were harvested and weighed to maintain the desired SRWC in the pots.
where Wtotal is the current soil total weight (pot + soil + plant + water), Wpot is the weight of the empty pot, DWsoil is the dry soil weight, SFW is the fresh weight of two plants harvested every 15 days, and WFC is the soil weight at field capacity (soil + pot + water).
The experiment was performed in randomized blocks, with four replications in a 5 × 2 factorial design (five rates of K replacement by Na and two water conditions) for each Eucalyptus species, totaling 120 experimental units with one plant each.
Leaf Gas Exchange and Leaf Water Potential
The youngest fully expanded leaf of each plant was used to evaluate gas exchange in the morning (from 9 a.m. to 11 a.m.) using an infrared gas analyzer (IRGA, Li-6400XT, LICOR Inc., Lincoln, NE, United States) at environmental humidity and temperature. The external CO2 concentration (Ca) was fixed at 400 μmol and the photosynthetically active radiation (PAR) flux density at 1,200 μmol m–2 s–1. Photosynthesis (A), stomatal conductance (gs), and transpiration (E) were measured (Mateus et al., 2019). Mean leaf temperature during the measurement was 30°C. WUE (in grams dry matter per kilogram H2O–1) was calculated by dividing the total dry matter (TDM) value (belowground plus aboveground) by water consumption (WC) throughout the experiment (Martin and Thorstenson, 1988), which was obtained by calculating the daily weight reduction due to transpiration (Equation 2).
where Sevap is the soil evaporation from the mean weight loss of four plantless pots.
In the same leaves, the predawn (3 a.m.) and noon (12 p.m.) leaf water potentials (ΨwPD and ΨwN, respectively) were also measured using a Scholander pressure chamber (Turner, 1981). All evaluations were realized prior to harvesting.
Stomatal Density and Leaf Area
Stomatal density (Std, stomates per square millimeter) was calculated using the two youngest fully expanded leaves per plant (Mateus et al., 2019) on abaxial and adaxial surfaces, applying the software package ImageJ1. Complementary micrograph material of Std was obtained by scanning electron microscopy (JEOL JSM-IT300 LV, Tokyo-Japan) at 20 kV, and digital images were recorded (Lavres et al., 2019).
Plants were harvested 120 days after the onset of the treatments, and their leaves, stems, branches, and roots were separated. Leaf area (LA) was obtained by passing all leaves through a leaf area integrator (LI-3100).
Dry Matter Production and Mineral Element Analysis
After drying in a forced air ventilation oven at 60°C for 72 h, each plant part was weighed to determine dry matter. Subsequently, the plant material was ground in a Wiley-type mill and forwarded to nitric–perchloric digestion (Malavolta et al., 1997) to quantify K and Na by inductively coupled plasma optical emission spectrometry (ICP-OES; iCAP 7000 Series, Thermo Fisher Scientific, Waltham, United States). Based on the leaf K and Na concentrations, we calculated the K/Na ratio, which was correlated with the estimated rate of maximum dry matter production (critical level of 90% maximum yield) of each genotype, obtained by equaling the equation to zero. The accumulations of K and Na were obtained by multiplying the concentration of each element in the tissue by the dry matter production of the respective tissue (root, stems, and leaves) and used to determine the use efficiency (UE, in grams per milligram) (Siddiqi and Glass, 1981) according to Equation 3.
where nutrient refers to K or Na accumulation.
Leaf Carbon Isotope Composition (δ13C‰)
The same samples used for leaf dry matter determination were also used to assess the carbon isotope composition, determined using a mass spectrometer (ANCA-GSL Hydra 20-20 model, SERCON Co., Crewe, GBR) coupled to a C automatic analyzer (Barrie and Prosser, 1996), and the isotope values (in per mill) were calculated via Equation 4 (Farquhar and Sharkey, 1982).
where R is the ratio of 13C/12C. The reference material is the Vienna Pee Dee Belemnite (PDB).
Statistical Analyses
Data were analyzed by the F test (p < 0.05), and significant differences among means were determined via Tukey’s post-hoc test (p < 0.05) to compare the WW and WS conditions. The significant effects of Na application were described by linear, quadratic, and square root regression models, in which the significant model (p < 0.05) with the highest determination coefficient (R2) was selected. The original data were standardized to be analyzed by principal component analysis (PCA) and cluster analysis, correlating the measured variables in each genotype and water condition. In cluster analysis, the treatments were grouped into functional units by their similarity; for the PCA, we used the treatments with Na supply for the first two main components (PC1 and PC2).
Statistical analyses were performed using the software packages SAS version 9.1 (SAS Institute Inc, 2004) and R version 3.5.1 (R Development Core Team, 2018). Data variability was indicated with standard error and shown graphically using SigmaPlot 11.0 (Systat Software Inc., San Jose, CA, United States).
Results
Adaxial and Abaxial Stomatal Density
The leaves of E. saligna and E. urophylla were hypostomatic, occurring mainly on the abaxial surface, with lower than 25 stomates per square millimeter. In E. camaldulensis, however, the leaves were amphistomatous, with stomates occurring on both surfaces. Adaxial stomatal density (StdAD) was influenced by Na, WS, and Na∗WS in E. saligna, E. urophylla, and E. camaldulensis (Figures 1A–C). Abaxial stomatal density (StdAB) was influenced by Na and WS in E. saligna and E. urophylla, whereas in E. camaldulensis, it was affected by Na application (Figures 1D–F). E. saligna and E. camaldulensis seedlings grown under WW and WS conditions showed higher StdAD and StdAB levels with partial K replacement by Na. Additionally, the highest Na rate (0.9 mmolc dm–3 of Na) led to decreased StdAB levels by 50, 30, and 20% in E. saligna, E. urophylla, and E. camaldulensis, respectively. Water stress also decreased the mean StdAB by 15 and 10% in E. saligna and E. urophylla, respectively, irrespective of the Na rate.
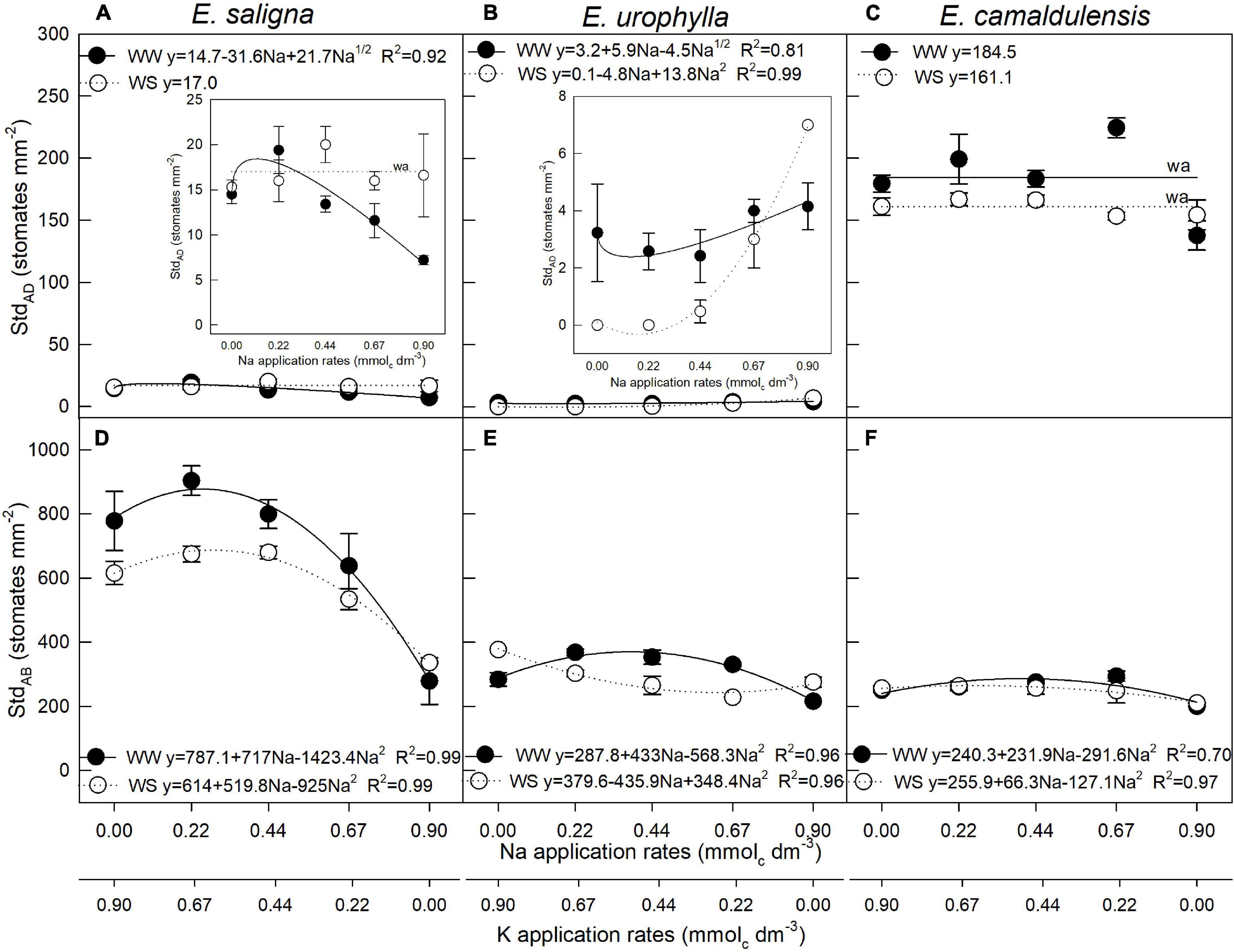
Figure 1. Stomatal density of the adaxial (StdAD) (A–C) and abaxial (StdAB) (D–F) leaf surfaces of Eucalyptus saligna (A,D), Eucalyptus urophylla (B,E), and Eucalyptus camaldulensis (C,F) seedlings under K partial replacement by Na in well-watered (WW) and water-stressed (WS) conditions. Vertical bars indicate standard errors among blocks (n = 4). The adjustment model is indicated by not significant (ns) and without suitable adjustment (wa).
Leaf Gas Exchange
Parameters A (assimilation rate; Figures 2A–C), E (transpiration rate; Figures 2D–F), and gs (Figures 2G–I) were influenced by Na and WS in all species. Partial K replacement by Na (up to 0.44 mmolc dm–3) increased A up to 55, 50, and 20% in E. saligna, E. urophylla, and E. camaldulensis, respectively, when compared to the control (0 mmolc dm–3 of Na). Meanwhile, the K-deficient plants of all genotypes had lower A. Compared to the control, the intermediary rates of Na also increased E up to 200 and 50% in E. saligna and E. urophylla, respectively, under both water conditions, and 40% in E. camaldulensis under WW. Against the other genotypes, E decreased until the intermediary Na rates for E. camaldulensis under WS conditions. The K-deficient plants had higher E, except for E. urophylla under WW. The gs increased with partial K replacement by Na up to 250% in E. saligna and to 50% in E. urophylla and E. camaldulensis under both water conditions compared to the control. K-deficient plants had significantly lower gs in E. urophylla under WW and E. camaldulensis under WS. Considering the mean of all rates, drought increased A by 20% in E. saligna and decreased its values by 15% in E. urophylla and E. camaldulensis; it also reduced the E values by 45, 35, and 75% and the gs values by 50, 30, and 55%, in E. saligna, E. urophylla, and E. camaldulensis, respectively, compared to those under WW.
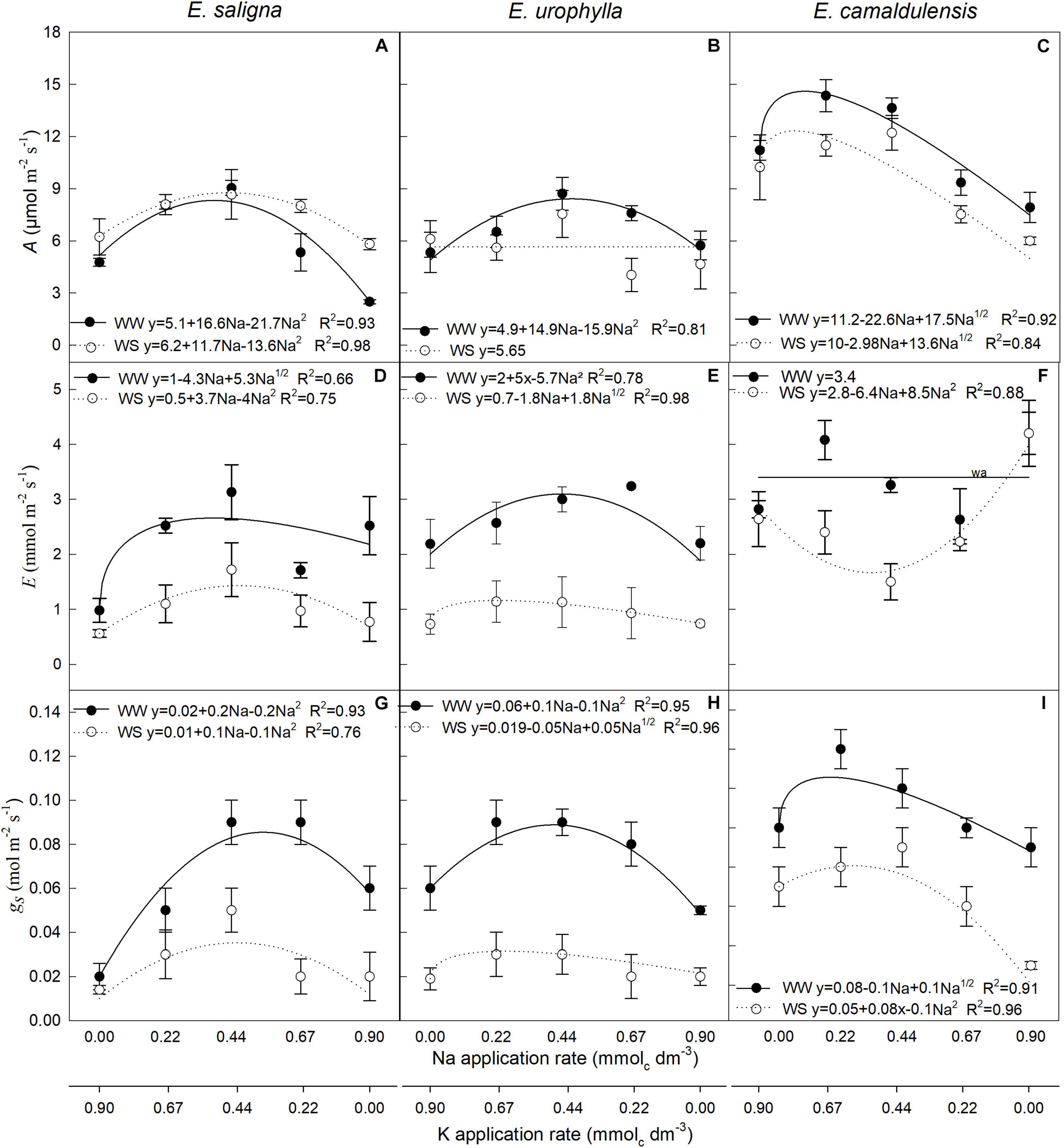
Figure 2. Photosynthetic rate (A) (A–C), leaf transpiration rate (E) (D–F), and stomatal conductance (gs) (G–I) in the leaves of Eucalyptus saligna (A,D,G), Eucalyptus urophylla (B,E,H), and Eucalyptus camaldulensis (C,F,I) seedlings under K partial replacement by Na in well-watered (WW) and water-stressed (WS) conditions. Vertical bars indicate standard errors between blocks (n = 4). The without suitable adjustment of a model is indicated by wa.
Leaf Carbon Isotope Composition (δ13C‰)
Factors Na, WS, and Na∗WS significantly influenced the leaf carbon isotopic compositions (δ13C‰) of E. saligna and E. urophylla, whereas for E. camaldulensis, it was affected by Na and WS (Figures 3A–C). Under WW, for E. saligna and E. camaldulensis, the δ13C‰ increased with lower Na application rates (0.22 mmolc dm–3 of Na) and decreased with higher Na rates. In contrast, the values of δ13C‰ for E. urophylla were reduced at lower rates (0.22 mmolc dm–3 of Na). The lowest δ13C‰ values were observed in K-deficient plants (0.9 mmolc dm–3 of Na) of E. urophylla and E. camaldulensis under both water conditions and in the intermediate Na rates (0.44 and 0.67 mmolc dm–3 of Na) of E. saligna under WS. Drought stress increased the δ13C‰ values of all species when compared to WW.
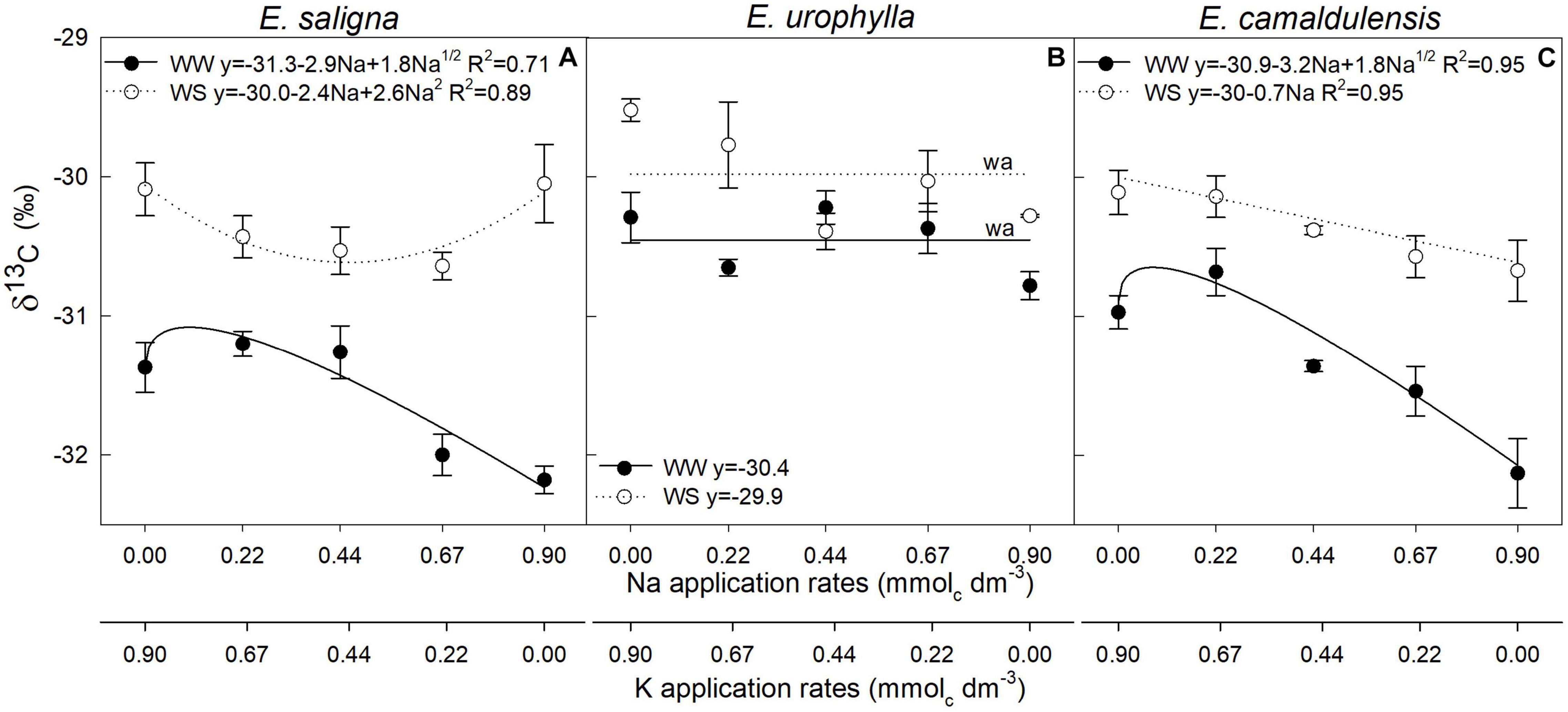
Figure 3. Leaf Carbon Isotope Composition (δ13C‰) of Eucalyptus saligna (A), Eucalyptus urophylla (B), and Eucalyptus camaldulensis (C) seedlings under K partial replacement by Na in well-watered (WW) and water-stressed (WS) conditions. Vertical bars indicate standard errors among blocks (n = 4). The adjustment model is indicated by not significant (ns) and without suitable adjustment (wa).
Predawn and Noon Leaf Water Potentials
In E. saligna, both predawn (ΨwPD) and noon (ΨwN) leaf water potentials were affected by Na, WS, and Na∗WS (Figures 4A,D), while in E. urophylla (Figures 4B,E) and E. camaldulensis (Figures 4C,F), these were affected by Na and WS. The lowest ΨwPD and ΨwN values were found at low to intermediate Na rates (0.22 and 0.44 mmolc dm–3) in E. saligna as well as for E. urophylla under both water conditions, except the ΨwN of E. saligna under WW, which increased linearly with Na application. Otherwise, the ΨwPD of E. camaldulensis increased up to 0.67 mmolc dm–3 in both conditions, while the ΨwN decreased with increasing Na application rates. The ΨwPD values of all genotypes were lower under WS than under WW, while the opposite was found in ΨwN values since the WS conditions decreased up to 25, 10, and 55% for E. saligna, E. urophylla, and E. camaldulensis, respectively, considering the mean of all Na application rates.
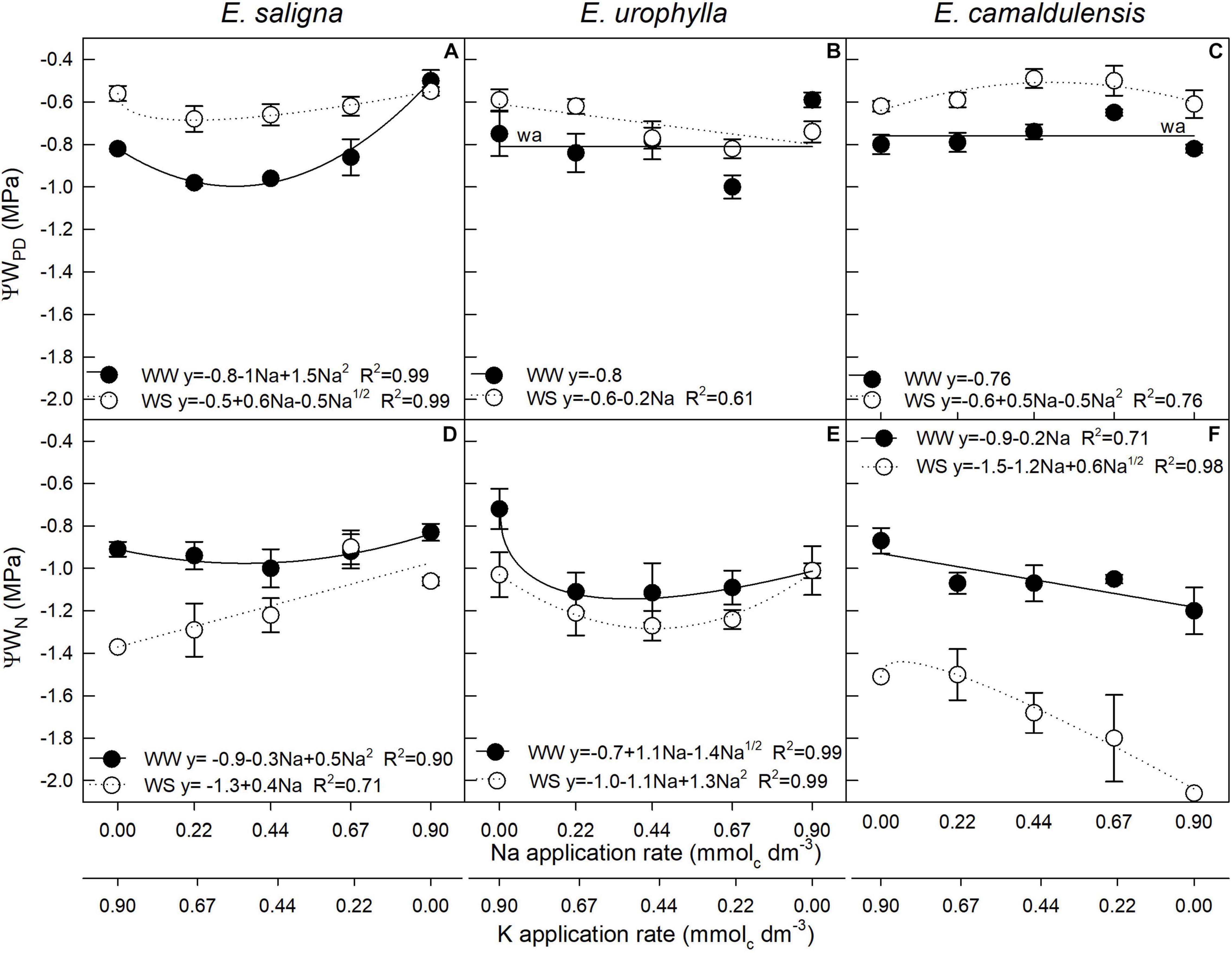
Figure 4. Predawn (ΨwPD) (A–C) and noon (ΨwN) (D–F) leaf water potentials of Eucalyptus saligna (A,D), Eucalyptus urophylla (B,E), and Eucalyptus camaldulensis (C,F) seedlings under K partial replacement by Na in well-watered (WW) and water-stressed (WS) conditions. Vertical bars indicate standard errors between blocks (n = 4).
Total Dry Matter Yield
The TDM of all genotypes was affected by Na, WS, and Na∗WS (Table 1). Partial K replacement by Na increased the TDM of all genotypes under both conditions, except in E. saligna under WS, which had a higher TDM than the control (0 mmolc dm–3 of Na), decreasing linearly with higher Na rates. The K-deficient plants (0.9 mmolc dm–3 of Na) had lower TDM levels by 35% under both water conditions compared to the control. Under WW, the maximum TDM (critical level) was reached with 0.048 mmolc dm–3 Na and 0.852 mmolc dm–3 K, corresponding to 5.3% substitution and 48.9 g per plant.
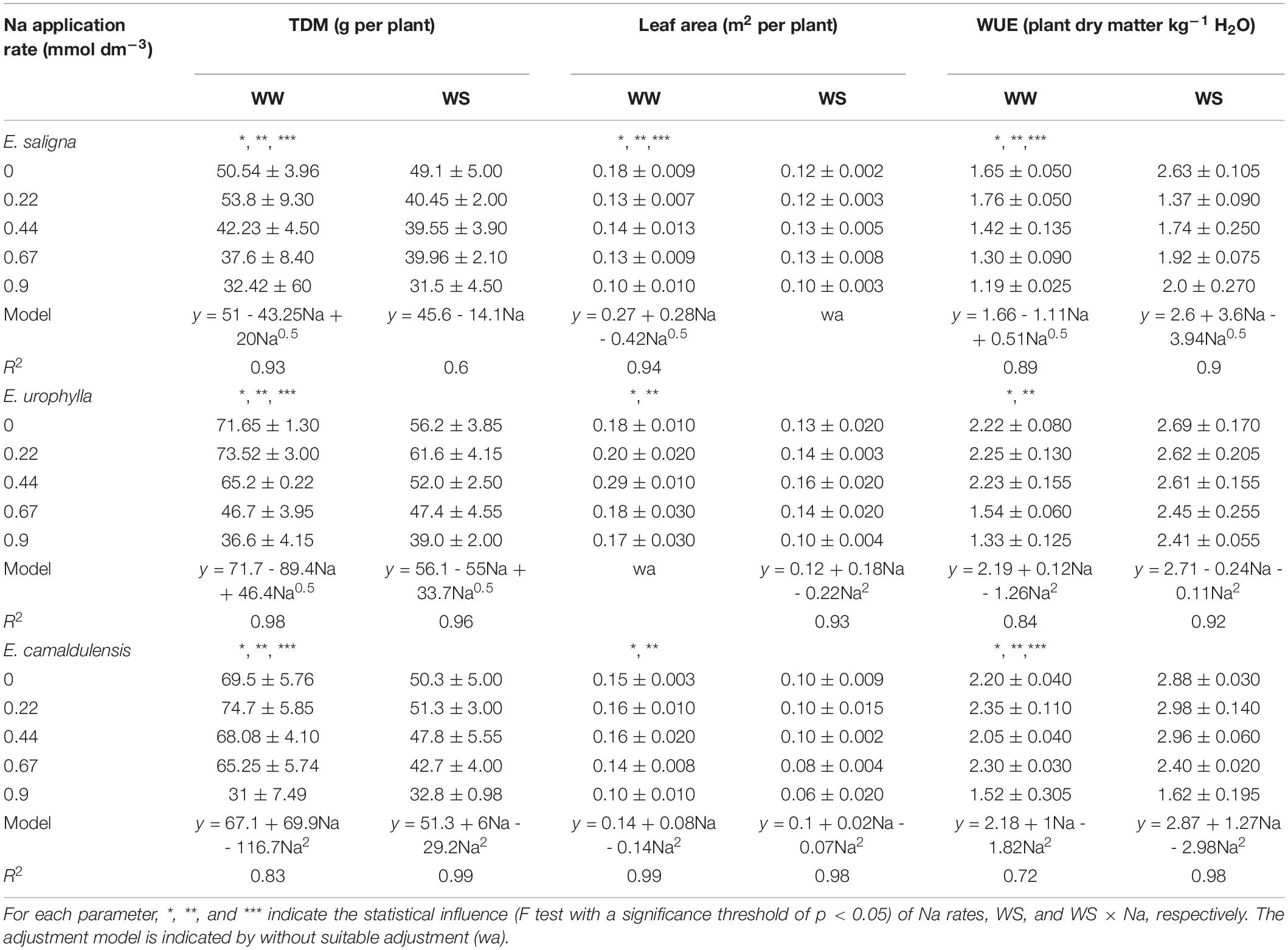
Table 1. Mean values (±standard errors, n = 4) of total dry matter (TDM) production, leaf area, and long-term water use efficiencies (WUEL) in the leaves of Eucalyptus saligna, Eucalyptus urophylla, and Eucalyptus camaldulensis seedlings under K partial replacement by Na in well-watered (WW) and water-stressed (WS) conditions.
In E. urophylla, a higher TDM level was found in plants with low Na rates (0.22 mmolc dm–3) at both water conditions, whereas K-deficient plants had decreased TDM by 50 and 30% than the control treatment in WW and WS, respectively. The estimated Na rate to give the maximum TDM was 0.06 mmolc dm–3 of Na and 0.84 mmolc dm–3 of K, which corresponded to 6.7% of substitution reaching 69.9 g per plant. Under WS, the estimated Na rate was 0.085 mmolc dm–3 and 0.815 mmolc dm–3 of K, corresponding to 9.3% substitution and 55.13 g per plant.
In contrast to the other genotypes, the higher TDM values of E. camaldulensis were observed up to an Na rate of 0.44 mmolc dm–3 under both water conditions, which means a substitution of K by Na around 50%. The K-deficient plants had lower TDM by 55 and 35% than the control treatment under the WW and WS conditions, respectively. The rates estimated to obtain the maximum TDM under WW was 0.27 mmol dm–3 of Na and 0.63 mmolc dm–3 of K, corresponding to a substitution level of 30% and 69.81 g per plant. Under WS, the estimated rates were 0.09 mmolc dm–3 of Na and 0.81 mmolc dm–3 of K, corresponding to 10% K replacement by Na and 46.4 g per plant.
Leaf Area
Leaf area (LA) was influenced by Na, WS, and Na∗WS in E. saligna (Table 1). Under WW, LA decreased up to 30% with higher Na application, while under WS, an increase around 10% occurred with intermediary rates of Na (0.44 and 0.67 mmolc dm–3) when compared to the control (0 mmolc dm–3 of Na). The LA of E. urophylla and E. camaldulensis was affected by Na and WS. Low to intermediary Na rates (0.22 and 0.44 mmolc dm–3) increased the LA of E. urophylla by up to 60 and 25% compared to the control under WW and WS, respectively. In E. camaldulensis, these Na rates increased the LA by 6% compared to the control under WW. Otherwise, K-deficient plants (0.9 mmolc dm–3 of Na) significantly decreased the LA up to 40% in all genotypes compared to the control. Water stress decreased the LA by 15, 35, and 36% in E. saligna, E. urophylla, and E. camaldulensis, respectively, according to the mean of all Na rates.
Water Use Efficiency
Water use efficiency (WUE) was influenced by Na, WS, and Na∗WS in E. saligna and E. camaldulensis, whereas in E. urophylla, it was only affected by Na and WS (Table 1). In E. saligna and E. urophylla under WW and in E. camaldulensis under both conditions, low K replacement by Na increased the WUE and decreased it in higher Na rates. Otherwise, plants of E. saligna and E. urophylla under WS decreased the WUE due to Na supply. Drought stress increased the WUE by 33, 35, and 17% in E. saligna, E. urophylla, and E. camaldulensis, respectively, irrespective of the Na rate. In addition, the mean WUE was higher in E. camaldulensis (drought tolerant), followed by E. urophylla (moderate tolerance) and E. saligna (drought sensitive) in both water conditions.
K and Na Leaf Concentrations and Efficiency of Use
The Na leaf concentration [Na] was influenced by Na and WS in E. saligna (Figure 5A), E. urophylla (Figure 5B), and E. camaldulensis (Figure 5C), While the K leaf concentration [K] was affected by Na, WS, and Na∗WS (Figure 5D) in E. saligna, E. urophylla (Figure 5E), and E. camaldulensis (Figure 5F). This affected only the Na rates and WS. Overall, K decreased and Na increased with increasing Na rates. In addition, the concentration levels were higher in plants under WW than in WS. In E. saligna under WW, the replacement of 5.3%, corresponding to 90% of TDM and reaching rates of 0.041 and 0.85 mmolc dm–3 of Na and K, respectively, decreased the K by 0.06 g kg–1, while Na increased by 0.12 g kg–1 compared to the application of only K, increasing plant growth. Furthermore, K of 2.9 g kg–1 was still above the critical level of K, without symptoms of deficiency. E. urophylla under WW with 6.7% of K replacement by Na reached the rates 0.06 and 0.83 mmolc dm–3 of Na and K, respectively; K decreased by 0.13 g kg–1, while Na increased by 0.018 g kg–1 compared to the application of only K. Under WS, 9.3% of K replacement by Na reached the rates 0.085 and 0.815 mmolc dm–3 of Na and K, respectively; K decreased by 0.07 g kg–1, while Na increased by 0.052 g kg–1 compared to the application of only K. Conversely, E. camaldulensis under WW, with 30% of K replacement by Na, reached the rates 0.27 and 0.63 mmolc dm–3 Na and K, respectively; K decreased by 0.46 g kg–1, while Na increased by 0.44 g kg–1 compared to the application of only K.
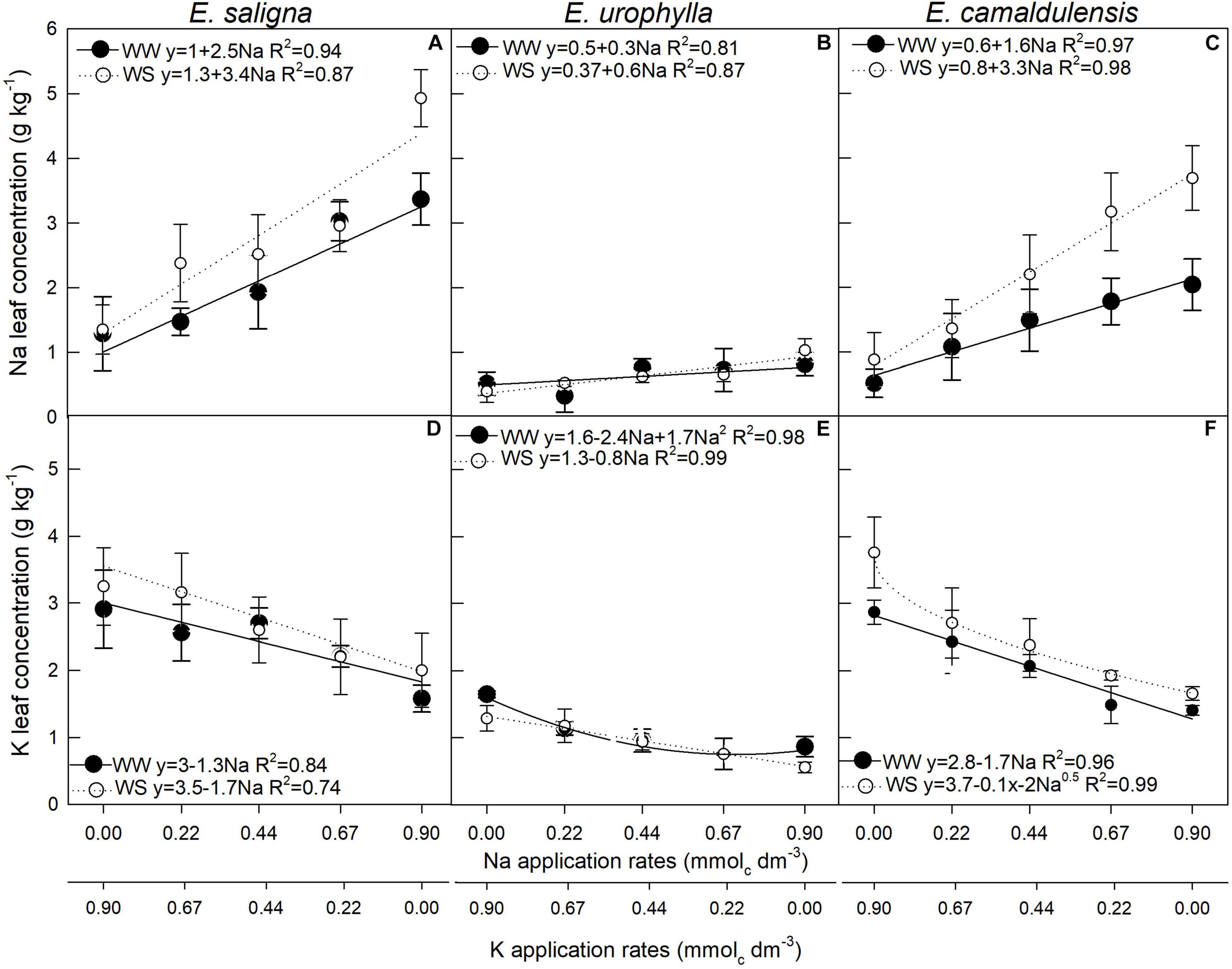
Figure 5. Leaf Na (A–C) and leaf K (D–F) concentrations of Eucalyptus saligna (A,D), Eucalyptus urophylla (B,E), and Eucalyptus camaldulensis (C,F) seedlings under K partial replacement by Na in well-watered (WW) and water-stressed (WS) conditions. Vertical bars indicate standard errors between blocks (n = 4).
Additionally, the leaf K/Na ratios decreased with increasing Na levels. According to the estimated rate of Na to achieve the maximum TDM of E. saligna, E. urophylla, and E. camaldulensis, the optimal leaf K/Na ratios were 1.7, 2.9, and 2.2, respectively, under WW and 2.6, 2.2, and 3.2, respectively, under WS (Table 2). UEK was affected by Na and WS, increasing up to 30% mainly with low K replacement by Na, while UENa was affected by Na, WS, and Na∗WS (Table 2), decreasing up to 70% with higher K replacement by Na. Water stress decreased the UE of both K and Na compared to WW.
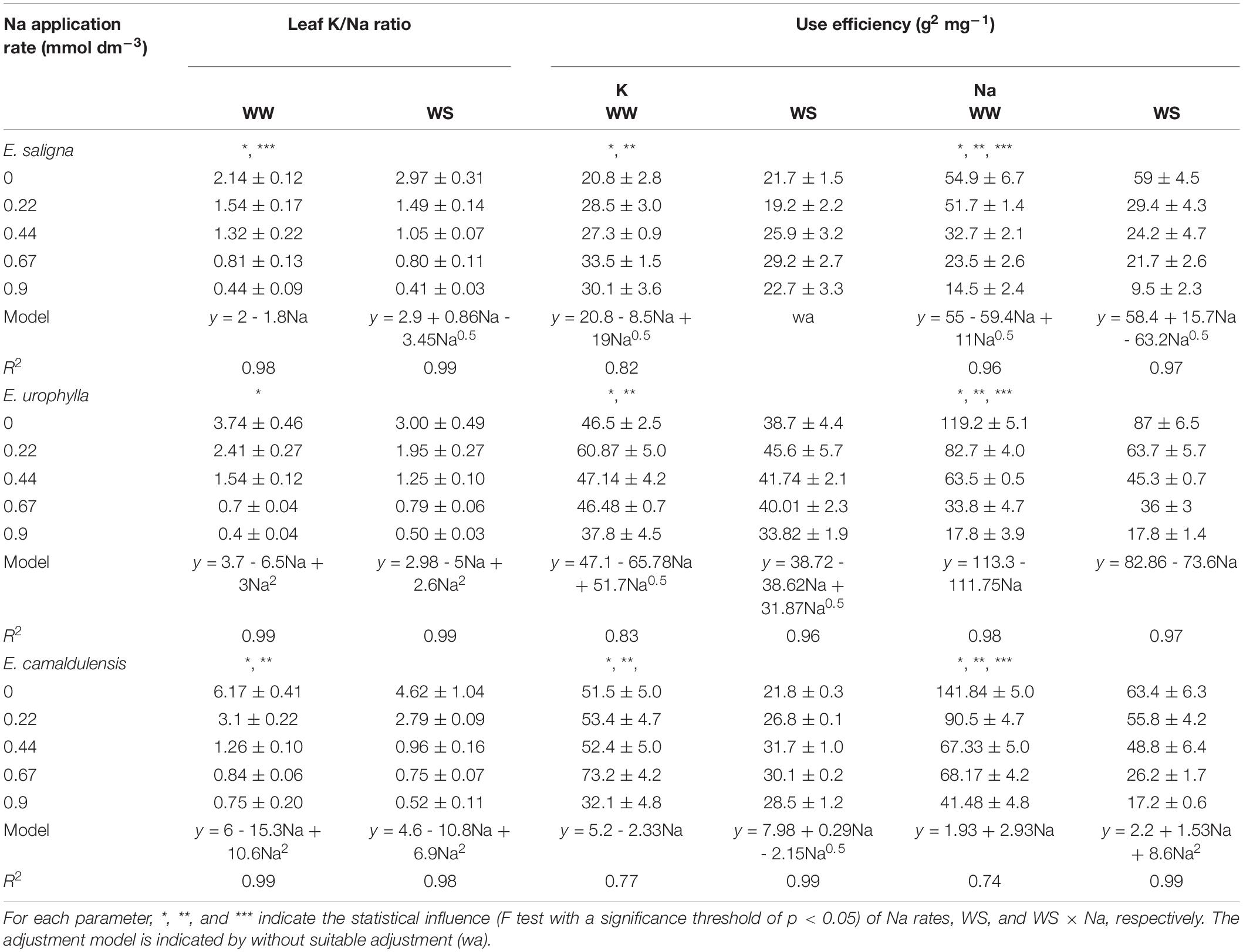
Table 2. Mean values (±standard errors, n = 4) of leaf K/Na ratio and K and Na use efficiency of Eucalyptus saligna, Eucalyptus urophylla, and Eucalyptus camaldulensis seedlings under K partial replacement by Na in well-watered (WW) and water-stressed (WS) conditions.
Characterization Among Genotypes
In E. saligna (Figure 6A), the total variance was explained by 64% (PC1 + PC2), with PC1 being explained by ΨwN, UEK, [K], and δ13C‰, while PC2 was explained by TDM, StdAB, and [Na]. The parameters A, WUE, LA, E, and gs contributed with average weights to explain the data variance in PC1 and PC2. Under WW, low to intermediate Na rates (up to 0.44 mmolc dm–3) were characterized by higher values of TDM and StdAB and lower values of Na and δ13C‰. Plants under lower Na rates and WS showed higher WUE and δ13C‰ values. The Na rate of 0.9 mmolc dm–3 resulted in higher Na levels and lower K, TDM, and StdAB values.
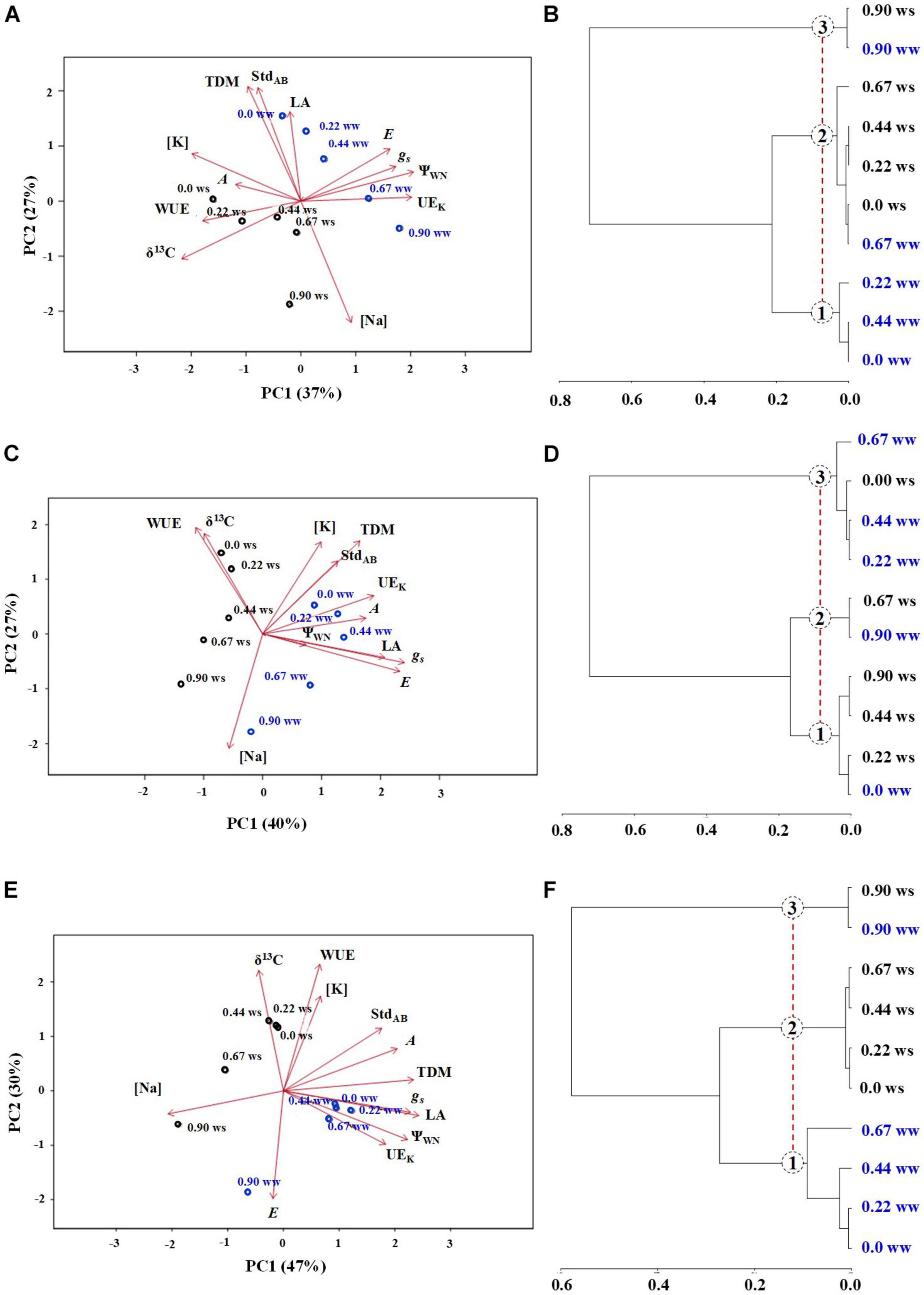
Figure 6. Principal component analysis (PCA) (A,C,E) and hierarchical cluster analyses (B,D,F) of Eucalyptus saligna (A,B), Eucalyptus urophylla (C,D), and Eucalyptus camaldulensis (E,F) seedlings under K partial replacement by Na (0, 0.22, 0.44, 0.67, and 0.90 mmolc dm– 3 of Na) in well-watered (WW) and water-stressed (WS) conditions.
In E. urophylla (Figure 6C), the total variance was explained by 66% (PC1 + PC2), with PC1 being explained by gs and E and PC2 by WUE, δ13C‰, [K], TDM, and Na. The parameters StdAB, UEK, A, ΨwN, and LA contributed with average weights to explain the data variance in PC1 and PC2. Under WW, low to intermediate Na rates were characterized by higher values of TDM, StdAB, UEK, A, LA, gs, and E. Higher Na rates were identified by higher Na and lower TDM, [K], WUE, and δ13C‰ levels. Under WS, Na rates of 0 and 0.22 mmolc dm–3 resulted in higher WUE and δ13C‰ and lower LA, E, and gs levels.
In E. camaldulensis (Figure 6E), the total variance was explained by 77% (PC1 + PC2), with PC1 being explained by TDM, gs, LA, ΨwN, and [Na] and PC2 by WUE, δ13C‰, and E. The parameters [K], StdAB, A, and UEK contributed with average weights to explain the data variance in PC1 and PC2. Under WW, low to intermediate Na rates were characterized by higher values of TDM, gs, LA, ΨwN, and EUK and by lower values of [Na]. Moreover, the Na rate of 0.9 mmolc dm–3 resulted in higher E and [Na] and lower δ13C‰, WUE, and [K] values. Plants under WS with low to intermediate Na supply were characterized by higher values of [K], WUE, and δ13C‰ and by lower E values.
Cluster analysis showed the formation of three main groups among the treatments in all genotypes. In E. saligna (Figure 6B), group 1 was represented by Na rates of 0, 0.22, and 0.44 mmolc dm–3 under WW, which represented the control and low to intermediate rates; group 2 by the rate of 0.67 mmolc dm–3 under WW and the rates of 0, 0.22, 0.44, and 0.67 mmolc dm–3 under WS; and group 3 contained K-deficient plants (0.9 mmolc dm–3 of Na) under both conditions. In E. urophylla (Figure 6D), group 1 comprised plants receiving no Na under WW and 0.22, 0.44, and 0.9 mmolc dm–3 of Na under WS, while group 2 contained plants receiving 0.67 and 0.9 mmolc dm–3 of Na under WS. In group 3, the plants received 0.22, 0.44, and 0.67 mmolc dm–3 of Na under WW and no Na under WS. In E. camaldulensis (Figure 6F), group 1 was composed of plants receiving 0, 0.22, 0.44, and 0.67 mmolc dm–3 of Na under WW, while in group 2, plants received 0, 0.22, 0.44, and 0.67 mmolc dm–3 of Na under WS. In group 3, plants received only 0.9 mmolc dm–3 of Na under both water regimes.
Discussion
To withstand drought periods, plants have evolved numerous mechanisms that vary among species (Merchant et al., 2007), which include morphological adaptations such as growth inhibition and stomatal closure (Warren et al., 2007), lowering its LA to avoid overheating (Ahrens et al., 2020) and water loss by leaf transpiration rates (Drake et al., 2019). For this, the plant reduces its tissue water content as a coordination of physiological and structural adaptations (Merchant et al., 2006), as well as cell contraction, turgor loss (Cosgrove, 1997), and slower leaf expansion (Pita-Barbosa et al., 2016). The ΨwN inducing stomatal closure plays a critical role in drought avoidance by protecting the integrity of xylem water transport, given that early stomatal closure and leaf shedding precede the beginning of embolism during prolonged drought stress (Li et al., 2020). The drought-induced reductions in plant growth were accompanied by a decrease in LA and leaf gas exchanges, differing in degree among species. Our findings clearly suggest that different genotypes provided adaptations to face drought stress, as indicated by the gs, ΨwN, and LA reductions, mainly observed in E. camaldulensis, showing a higher tolerance to drought stress (Table 1) and confirming Ψw as an effective indicator for measuring the drought tolerance of plants.
Furthermore, plants grown under WW showed the lowest decreases from ΨwPD to ΨwN, demonstrating a great reduction in osmotic pressure to maintain cell turgor in plants grown under WS, which unexpectedly showed higher ΨwPD than did plants under WW. Although different hydraulic systems have been found among species and genotypes of the same species (Costa e Silva et al., 2004), a lower ΨwPD was expected in WS relative to the WW condition (Drake et al., 2019). The replacement of K by Na in the vacuoles promoted a faster decrease in cell osmotic potential in plants under drought, as it also increased cell expansion in plants under adequate water availability (Hampe and Marschner, 1982). Albeit the three species demonstrated adaptive capacity to the experimental conditions, the values measured differently respond to treatments and water conditions due to the contrasting genotypic patterns that control drought tolerance (Ahrens et al., 2020). The variance in the Ψw values under the WW and WS conditions indicated that the differences in drought tolerance between the Eucalyptus species are associated with osmotic adjustments and drought avoidance mechanisms, turning essential the integration of several adaptive strategies simultaneously (Shvaleva et al., 2006). According to the authors, differences in the metabolic responses may also reflect distinct degrees of stress experienced throughout the experimental period. In general, osmolyte accumulation as a consequence of drought reduces the cell osmotic potential and improves the water absorption and cell turgor, sustaining future physiological processes, such as stomatal opening, photosynthesis, cell growth, and enhanced dehydration tolerance under drought conditions. As observed, the drought-avoidance mechanisms of E. camaldulensis did not reach the same degree of tolerance to drought stress by E. saligna and E. urophylla. Stomata distribution on the leaf surface was also related to the amount of energy used in transpiration (latent heat transfer; Jarvis and McNaughton, 1986). In E. saligna and E. urophylla, stomata occurred on the underside (hypostomatous leaves), which is common in plants of mesophytic habitats (Figure 1 and Supplementary Figure S1). However, the stomata of E. camaldulensis, the drought-tolerant genotype (Gonçalves et al., 2017), occurred on both sides (amphistomatous leaves), which is common in arid environments (Parkhurst, 1978) and has been correlated with a reduced internal diffusion resistance by the lower pathway length of CO2 molecules to the carboxylation sites (Mott and Michaelson, 1991). According to the authors, the occurrence of stomata on both sides would be advantageous in plants growing under high light intensity, where the internal CO2 concentration limits the photosynthetic rates. As observed in our study, adaptations to drought stress were stimulated in all Eucalyptus species with partial K replacement by Na.
An adequate K nutritional status of plants promotes tolerance to abiotic stress (Cakmak, 2005) and enhances the WUE of trees (Battie-Laclau et al., 2016) since water uptake by the roots and stomatal opening are facilitated by K accumulation in the root xylem vessels and guard cells, increasing the tissue’s water status and improving tolerance to water stress (Mengel et al., 2001). According to Ahrens et al. (2020), the WUE is correlated with δ13C‰, which in turn relates to leaf gas exchange properties. These statements are in agreement with our findings. Due to drought, the reduction in E (biophysical process) as a consequence of the significant decline in gs was not accompanied in the same degree by A (biophysical/biochemical process), increasing WUE, δ13C‰, and plant drought resistance (Egilla et al., 2005; Sarabi et al., 2019). In contrast to E. urophylla and E. camaldulensis, the drought increased A in E. saligna, which was unexpected. We hypothesize that evaluations in leaf scale as A, E, and gs produce accurate data of a specific time and may not always be reliable in predicting whole plant responses throughout their cycle (Jákli et al., 2016), which in turn can be reflected by δ13C‰ and WUE (Condon et al., 2002), integrative indicators of changes in the environmental conditions that occur during the entire experimental period (Seibt et al., 2008). Furthermore, drought stress reduced EUK and EUNa, being an adaptive strategy favoring nutrient accumulation in an unfavorable soil–climate situation to subsequently increase nutrient translocation and use under favorable growth conditions (Müller et al., 2017).
Our studies indicate that, to a certain degree, the replacement of K by Na promoted Eucalyptus growth (Subbarao et al., 1999; Krishnasamy et al., 2014), with a small amount of Na being equivalent to K in their function (Ivahupa et al., 2006). Plants with low K replacement by Na showed higher TDM compared to those receiving only K (0 mmolc dm–3 of Na) or of K-deficient plants (0.9 mmolc dm–3 of Na) even in those under WS, except for E. saligna, the drought-sensitive genotype (Table 1). As Na can partially substitute K in the vacuole, making more K available to the cytosol (Rodríguez-Navarro and Rubio, 2006), low K replacement by Na contributed to enhancing cell turgor and expansion (Wakeel et al., 2011), promoting plant growth (Martínez et al., 2005; Ma et al., 2011; Schulze et al., 2012; Battie-Laclau et al., 2013), as observed by the higher TDM concomitant to the lower ΨwN in plants. Furthermore, the estimated ideal percentage of substitution increased according to the drought tolerance of the genotypes, reaching 30% in E. camaldulensis under WW, confirming Na as a beneficial element in plant dry matter (Subbarao et al., 2003; Idowu and Aduayi, 2006; Wakeel et al., 2011; Kronzucker et al., 2013) even under drought (Yue et al., 2012; Xi et al., 2018). The leaf K/Na ratio is commonly used as a predictor of plant performance (Munns and Tester, 2008), varying among Eucalyptus genotypes (Marcar and Termaat, 1990). An appropriate leaf K/Na ratio was found for low K replacement by Na, as observed by the estimated maximum yield, varying from 1.5 to 3.1 among the Eucalyptus genotypes (Marcar and Termaat, 1990) and water regimes (Table 1). A similar leaf K/Na ratio of 3.4 was found by Mateus et al. (2019) in hybrid Eucalyptus subjected to K replacement by Na in the nutrient solution. This indicates that Na can reduce the critical levels of leaf K under an adequate K/Na ratio, providing changes in plant performance and demand, albeit without any symptoms of K deficiency (Besford, 1978; Krishnasamy et al., 2014). There is no evidence of key cytosolic components being hampered by a low Na supply, unlike under salinity conditions (Gattward et al., 2012), although a greater efficiency in the osmotic function of plants supplied with both K and Na was observed in our study, corroborating the results of previous studies (Jeschke, 1977). These authors suggested that the replacement of K by Na in the process of osmoregulation in vacuoles improved turgor and cell expansion (Pi et al., 2014). In our study, the low K replacement by Na (up to 50%) increased Std and, consequently, A, E, and gs at the expense of higher WUE values, evidencing the benefits of nutrient interaction to a certain degree.
Low K replacement by Na confirmed that nutrient interaction is a strategy to increase the UEK under low soil K availability, proposed by Laclau et al. (2003), not only allowing the maintenance of productivity despite the lower K supply but also favoring plant development (Ma et al., 2011) and allowing the increase in plant TDM. Notably, large proportions of substitution decreased the K/Na ratio and led to lower photosynthetic performance and biomass, as observed by the negative relationship between TDM and Na (Figure 6), evidencing the interaction among mineral nutrition, nutrient use, and soil water availability in Eucalyptus (Tariq et al., 2019). Potassium decreased concomitantly to the higher Na rates in Eucalyptus (Figure 5), suggesting that the Na ions were directed toward the vacuole as an alternative inorganic osmoticum (Flowers and Lauchli, 1983), including guard cells (Terry and Ulrich, 1973), and releasing K to the cytoplasm and metabolic pathways, such as in the chloroplast (Speer and Kaiser, 1991), stimulating photosynthesis (Krishnasamy et al., 2014) and water retention (Xi et al., 2018). It is widely hypothesized that despite the drop in K, the K cytoplasm concentration is maintained near 100 mmol L–1 K, which is required for adequate enzyme activities (Britto and Kronzucker, 2008), as also suggested by Gattward et al. (2012).
However, the stomata of plants under high K replacement by Na cannot function properly, favoring stomatal opening and promoting E. The absence of K stimulates ethylene synthesis (Benlloch-Gonzalez et al., 2010), impairing the action of abscisic acid on the stomata, decreasing gs and delaying stomatal closure (Tanaka et al., 2006), thus reducing WUE and TDM (White et al., 2009; Christina et al., 2018). The LA development was also directly affected by the plant mineral nutritional status (Marschner, 2012), especially K nutrition (Egilla et al., 2005; Battie-Laclau et al., 2013; Tavakol et al., 2018). In our study, yellowing in the leaf margins and even delayed stomatal closure were noticed in K-deficient plants (0.9 mmolc dm–3 of Na) (Wang et al., 2013), dramatically reducing LA (Bednarz et al., 1998). Plant yield also decreased due to K deficiency, an essential element that cannot be completely replaced (Arnon and Stout, 1939) due to its specific functions, such as enzyme activation, initiation (Spyrides, 1964), elongation (Lubin and Ennis, 1964), termination of translation (Näslund and Hultin, 1971), and conformation of ribosomes (Klein, 2004). Our results indicated that since K-deficient plants occurred at Na rates higher than 0.67 mmolc dm–3, a K/Na ratio of 1:0 is critical for Eucalyptus growth since lower ratios significantly decreased plant TDM. Thus, understanding the K/Na ratio mechanisms may be useful for the development of strategies to reduce K fertilization by replacing it with more cost- and energy-efficient alternatives (Benito et al., 2014).
Drought results in gs and gm impairments (Chaves et al., 2009), decreasing Ci and resulting in the fixation of available CO2 molecules. Thus, under drought, stomatal closure leads to the enrichment in 13C and, consequently, in a higher δ13C‰ (Robinson et al., 2000). In contrast, the decrease in δ13C‰ indicated higher stomatal aperture (Farquhar et al., 1989), as shown by the lower WUE, confirming that the stomata of K-deficient plants cannot function properly, favoring stomatal opening and promoting E. Therefore, K-deficient plants under both conditions were characterized by lower δ13C‰, WUE, TDM, and K levels and higher Na levels, which explains their grouping in the same cluster (0.9 mmolc dm–3 of Na under WW and WS) (Figure 6). Low K replacement, markedly under drought, also resulted in lower numbers of open stomata, indicated by the higher δ13C‰ (more positive) and WUE values, which was associated with a better response to drought, confirming the statement that the richer plants are in δ13C‰, the greater the WUE, as proposed by Farquhar et al. (1989). Our results also showed greater relative whole plant transpiration than the control plants, suggesting adequate stomatal closure by osmotic adjustment to avoid water loss at the expense of photosynthetic restriction and mitigating drought impacts. Moreover, plants with low replacement levels of K by Na were grouped into the same cluster, with similar responses characterized by higher Std, UEK, δ13C‰, WUE, TDM, and K levels and lower Na levels.
Conclusion
Regardless of the water condition, the substitution of K by Na at a level of 25–50% reduced the critical level of K without symptoms of K deficiency and allowed optimum Eucalyptus dry matter production. It also improved CO2 assimilation, Std, UEK, and WUE and maintained leaf turgidity by reducing ΨWN, with the stomata partially closed, indicated by the higher δ13C‰, mitigating the negative impacts of drought. Furthermore, the estimated ideal percentage of substitution increased according to the drought tolerance of the genotypes (E. saligna < E. urophylla < E. camaldulensis). When only Na was supplied, inferring K-deficient plants, in addition to the lower growth, plants were characterized by lower δ13C‰, WUEL, and K levels and higher Na levels. The ideal leaf K/Na ratio to provide the maximum yield varied from 1.7 to 3.2 among genotypes and water regimes; values below 1:0 were critical for Eucalyptus growth since lower ratios significantly decreased plant development.
Data Availability Statement
All datasets generated for this study are included in the article/Supplementary Material, further inquiries can be directed to the corresponding author/s.
Author Contributions
All authors contributed to the literature search, discussion, and writing of the manuscript. NM, AVF, JG, and JL conceived and designed the study. NM performed most experiments. ALF and ES assisted with the management of pot culture and plant material, analysis, and interpretation of data. All authors checked and approved the submitted version.
Funding
This work was supported financially in part by the National Council for Scientific and Technological Development (CNPq, Project No. 137864/2017-5) and the agreement of the Coordination of Superior Level Staff Improvement (CAPES) and the São Paulo Research Foundation (FAPESP, Project No. 2021/00463-7 to JL, and Project No. 2017/24410-4).
Conflict of Interest
The authors declare that the research was conducted in the absence of any commercial or financial relationships that could be construed as a potential conflict of interest.
Acknowledgments
We gratefully acknowledge the two reviewers for constructive suggestions on an earlier version of this manuscript. JL is grateful for the scholarship support to NM. JL thanks the “National Council for Scientific and Technological Development” from Brazil (Conselho Nacional de Desenvolvimento Científico e Tecnológico – CNPq) for the research fellowship (Grant No. #310572/2017-7).
Supplementary Material
The Supplementary Material for this article can be found online at: https://www.frontiersin.org/articles/10.3389/fpls.2021.632342/full#supplementary-material
Abbreviations
StdAD, adaxial stomatal density of leaf surface; StdAB, abaxial stomatal density of leaf surface; A, CO2 assimilation rate; gs, stomatal conductance; E, transpiration rate; δ13C ‰, leaf carbon isotopic composition; Ψw, leaf water potential; K, potassium; LA, leaf area; WUE, water use efficiency; UE, use efficiency; WW, well-watered; WS, water stressed.
Footnotes
References
Ahmad, I., and Maathuis, F. J. M. (2014). Cellular and tissue distribution of potassium: physiological relevance, mechanisms and regulation. J. Plant Physiol. 171, 708–714. doi: 10.1016/j.jplph.2013.10.016
Ahrens, C. W., Andrew, M. E., Mazanec, R. A., Ruthrof, K. X., Challis, A., Hardy, G., et al. (2020). Plant functional traits differ in adaptability and are predicted to be differentially affected by climate change. Ecol. Evol. 10, 232–248. doi: 10.1002/ece3.5890
Anjum, S. A., Xie, X.-Y., Wang, L.-C., Saleem, M. F., Man, C., and Lei, W. (2011). Morphological, physiological and biochemical responses of plants to drought stress. African J. Agric. Res. 6, 2026–2032. doi: 10.5897/AJAR10.027
Arnon, D., and Stout, P. (1939). The essentiality of certain elements in minute quantify for plants with special reference to copper. Plant Physiol. 14, 371–375. doi: 10.1104/pp.14.2.371
Asensio, V., Domec, J.-C., Nouvellon, Y., Laclau, J.-P., Bouillet, J.-P., Jordan-Meille, L., et al. (2020). Potassium fertilization increases hydraulic redistribution and water use efficiency for stemwood production in Eucalyptus grandis plantations. Environ. Exp. Bot. 176:104085. doi: 10.1016/j.envexpbot.2020.104085
Barrie, A., and Prosser, S. (1996). “Automated analysis of light-element stable isotopes by isotope ratio mass spectrometry,” in Mass Spectrometry of Soils, ed. Y. S. Boutton (Crewe,Ches: Europa Scientific Ltd), 1–46.
Battie-Laclau, P., Delgado-Rojas, J. S., Christina, M., Nouvellon, Y., Bouillet, J. P., de Cassia Piccolo, M., et al. (2016). Potassium fertilization increases water-use efficiency for stem biomass production without affecting intrinsic water-use efficiency in Eucalyptus grandis plantations. For. Ecol. Manage. 364, 77–89. doi: 10.1016/j.foreco.2016.01.004
Battie-Laclau, P., Laclau, J. P., Piccolo, M., de, C., Arenque, B. C., Beri, C., et al. (2013). Influence of potassium and sodium nutrition on leaf area components in Eucalyptus grandis trees. Plant Soil. 371, 19–35. doi: 10.1007/s11104-013-1663-7
Bednarz, C., Oosterhuis, D., and Evans, R. (1998). Leaf photosynthesis and carbon isotope discrimination of cotton in response to potassium deficiency. Environ. Exp. Bot. 39, 131–139. doi: 10.1016/S0098-8472(97)00039-7
Benito, B., Haro, R., Amtmann, A., and Cuin, T. A. (2014). The twins K+ and Na+ in plants. J. Plant Physiol. 171, 723–731. doi: 10.1016/J.JPLPH.2013.10.014
Benlloch-Gonzalez, M., Romera, J., Cristescu, S., Harren, F., Fournier, J. M., and Benlloch, M. (2010). K+ starvation inhibits water-stress-induced stomatal closure via ethylene synthesis in sunflower plants. J. Exp. Bot. 61, 1139–1145. doi: 10.1093/jxb/erp379
Besford, R. T. (1978). Effect of replacing nutrient potassium by sodium on uptake and distribution of sodium in tomato plants. Plant Soil. 50, 399–409. doi: 10.1007/BF02107188
Britto, D. T., and Kronzucker, H. J. (2008). Cellular mechanisms of potassium transport in plants. Physiol. Plant. 133, 637–650. doi: 10.1111/j.1399-3054.2008.01067.x
Cakmak, I. (2005). The role of potassium in alleviating detrimental effects of abiotic stresses in plants. J. Plant Nutr. Soil Sci. 168, 521–530. doi: 10.1002/jpln.200420485
Chaves, M. M., Flexas, J., and Pinheiro, C. (2009). Photosynthesis under drought and salt stress: regulation mechanisms from whole plant to cell. Ann. Bot. 103, 551–560. doi: 10.1093/AOB/MCN125
Christina, M., le Maire, G., Nouvellon, Y., Vezy, R., Bordon, B., Battie-Laclau, P., et al. (2018). Simulating the effects of different potassium and water supply regimes on soil water content and water table depth over a rotation of a tropical Eucalyptus grandis plantation. For. Ecol. Manage. 418, 4–14. doi: 10.1016/j.foreco.2017.12.048
Condon, A. G., Richards, R. A., Rebetzke, G. J., and Farquhar, G. D. (2002). Improving intrinsic water-use efficiency and crop yield. Crop Sci. 42, 122–131. doi: 10.2135/cropsci2002.1220
Cosgrove, D. J. (1997). Relaxation in a high-stress environment: the molecular bases of extensible cell walls and cell enlargement. Plant Cell 9, 1031–1041. doi: 10.1105/tpc.9.7.1031
Costa e Silva, F., Shvaleva, A., Maroco, J. P., Almeida, M. H., Chaves, M. M., and Pereira, J. S. (2004). Responses to water stress in two Eucalyptus globulus clones differing in drought tolerance. Tree Physiol. 24, 1165–1172. doi: 10.1093/treephys/24.10.1165
Drake, J. E., Tjoelker, M. G., Aspinwall, M. J., Reich, P. B., Pfautsch, S., and Barton, C. V. M. (2019). The partitioning of gross primary production for young Eucalyptus tereticornis trees under experimental warming and altered water availability. New Phytol. 222, 1298–1312. doi: 10.1111/nph.15629
Egilla, J. N., Davies, F. T., and Boutton, T. W. (2005). Drought stress influences leaf water content, photosynthesis, and water-use efficiency of Hibiscus rosa-sinensis at three potassium concentrations. Photosynthetica 43, 135–140. doi: 10.1007/s11099-005-5140-2
Epron, D., Laclau, J. P., Almeida, J. C. R., Gonalves, J. L. M., Ponton, S., Sette, C. R., et al. (2012). Do changes in carbon allocation account for the growth response to potassium and sodium applications in tropical Eucalyptus plantations? Tree Physiol. 32, 667–679. doi: 10.1093/treephys/tpr107
Erel, R., Ben-Gal, A., Dag, A., Schwartz, A., and Yermiyahu, U. (2014). Sodium replacement of potassium in physiological processes of olive trees (var. Barnea) as affected by drought. Tree Physiol. 34, 1102–1117. doi: 10.1093/treephys/tpu081
Farquhar, G. D., Ehleringer, J. R., and Hubick, K. T. (1989). Carbon isotope discrimination and photosynthesis. Annu. Rev. Plant Physiol. Plant Mol. Biol. 40, 503–537. doi: 10.1146/annurev.pp.40.060189.002443
Farquhar, G. D., and Sharkey, T. D. (1982). Stomatal conductance and photosynthesis. Annu. Rev. Plant Physiol. 33, 317–345. doi: 10.1146/annurev.pp.33.060182.001533
Flowers, T. J., and Lauchli, A. (1983). Sodium versus potassium: substitution and compartmentation. Encycl. Plant Physiol. 15, 651–681.
Gattward, J. N., Almeida, A. A. F., Souza, J. O., Gomes, F. P., and Kronzucker, H. J. (2012). Sodium-potassium synergism in Theobroma cacao: stimulation of photosynthesis, water-use efficiency and mineral nutrition. Physiol. Plant 146, 350–362. doi: 10.1111/j.1399-3054.2012.01621.x
Gonçalves, J. L. M., Alvares, C. A., Rocha, J. H. T., Brandani, C. B., and Hakamada, R. (2017). Eucalypt plantation management in regions with water stress. South. For. 79, 169–183. doi: 10.2989/20702620.2016.1255415
Hampe, T., and Marschner, H. (1982). Effect of sodium on morphology, water relations and net photosynthesis of sugar beet leaves. Z. Pflanzenphysiol. 108, 151–162. doi: 10.1016/s0044-328x(82)80066-4
Idowu, M. K., and Aduayi, E. A. (2006). Effects of sodium and potassium application on water content and yield of tomato in Southwestern Nigeria. J. Plant Nutr. 29, 2131–2145. doi: 10.1080/01904160600972761
IPCC (2019). Climate Change and Land: An IPCC Special Report on Climate Change, Desertification, Land Degradation, Sustainable Land Management, Food Security, and Greenhouse Gas Fluxes in Terrestrial Ecosystems. Geneva: IPCC.
Ivahupa, S. R., Asher, C. J., Blamey, F. P. C., and O’Sullivan, J. N. (2006). Effects of sodium on potassium nutrition in three tropical root crop species. J. Plant Nutr. 29, 1095–1108. doi: 10.1080/01904160600689241
Jákli, B., Tränkner, M., Senbayram, M., and Dittert, K. (2016). Adequate supply of potassium improves plant water-use efficiency but not leaf water-use efficiency of spring wheat. J. Plant Nutr. Soil Sci. 179, 733–745. doi: 10.1002/jpln.201600340
Jarvis, P. G., and McNaughton, K. G. (1986). Stomatal control of transpiration: scaling up from leaf to region. Adv. Ecol. Res. 15, 1–49. doi: 10.1016/S0065-2504(08)60119-1
Jeschke, W. D. (1977). K+-Na+ exchange and selectivity in barley root cells effects of K+, Rb+, Cs+, and Li+ on the Na+ fluxes. Z. Pflanzenphysiol. 84, 247–264. doi: 10.1016/S0044-328X(77)80027-5
Jin, S. H., Huang, J. Q., Li, X. Q., Zheng, B. S., Wu, J. S., Wang, Z. J., et al. (2011). Effects of potassium supply on limitations of photosynthesis by mesophyll diffusion conductance in Carya cathayensis. Tree Physiol. 31, 1142–1151. doi: 10.1093/treephys/tpr095
Kim, T.-H., Böhmer, M., Hu, H., Nishimura, N., and Schroeder, J. I. (2010). Guard cell signal transduction network: advances in understanding abscisic acid, CO2, and Ca2+ signaling. Annu. Rev. Plant Biol. 61, 561–591. doi: 10.1146/annurev-arplant-042809-112226
Klein, D. J. (2004). The contribution of metal ions to the structural stability of the large ribosomal subunit. RNA 10, 1366–1379. doi: 10.1261/rna.7390804
Krishnasamy, K., Bell, R., and Ma, Q. (2014). Wheat responses to sodium vary with potassium use efficiency of cultivars. Front. Plant Sci. 5:631. doi: 10.3389/fpls.2014.00631
Kronzucker, H. J., Coskun, D., Schulze, L. M., Wong, J. R., and Britto, D. T. (2013). Sodium as nutrient and toxicant. Plant Soil 369, 1–23. doi: 10.1007/s11104-013-1801-2
Laclau, J.-P., Ranger, J., Bouillet, J.-P., de Dieu Nzila, J., and Deleporte, P. (2003). Nutrient cycling in a clonal stand of Eucalyptus and an adjacent savanna ecosystem in Congo: 1. Chemical composition of rainfall, throughfall and stemflow solutions. For. Ecol. Manage. 176, 105–119. doi: 10.1016/S0378-1127(02)00280-3
Lavres, J., Silveira Rabêlo, F. H., Capaldi, F. R., dos Reis, A. R., Rosssi, M. L., Franco, M. R., et al. (2019). Investigation into the relationship among Cd bioaccumulation, nutrient composition, ultrastructural changes and antioxidative metabolism in lettuce genotypes under Cd stress. Ecotoxicol. Environ. Saf. 170, 578–589. doi: 10.1016/J.ECOENV.2018.12.033
Li, X., Smith, R., Choat, B., and Tissue, D. T. (2020). Drought resistance of cotton (Gossypium hirsutum) is promoted by early stomatal closure and leaf shedding. Funct. Plant Biol. 47:91. doi: 10.1071/FP19093
Lubin, M., and Ennis, H. L. (1964). On the role of intracellular potassium in protein synthesis. Biochim. Biophys. Acta - Spec. Sect. Nucleic Acids Relat. Subj. 80, 614–631. doi: 10.1016/0926-6550(64)90306-8
Lv, S., Nie, L., Fan, P., Wang, X., Jiang, D., Chen, X., et al. (2012). Sodium plays a more important role than potassium and chloride in growth of Salicornia europaea. Acta Physiol. Plant. 34, 503–513. doi: 10.1007/s11738-011-0847-0
Ma, Q., Bell, R., and Brennan, R. (2011). Moderate sodium has positive effects on shoots but not roots of salt-tolerant barley grown in a potassium-deficient sandy soil. Crop Pasture Sci. 62:972. doi: 10.1071/CP11162
Madsen, P. A., and Mulligan, D. R. (2006). Effect of NaCl on emergence and growth of a range of provenances of Eucalyptus citriodora, Eucalyptus populnea, Eucalyptus camaldulensis and Acacia salicina. For. Ecol. Manage. 228, 152–159. doi: 10.1016/j.foreco.2006.02.044
Malavolta, E., Vitti, G. C., and de Oliveira, S. A. (1997). Avaliação do Estado Nutricional Das Plantas: Princípios E Aplicações. Piracicaba: POTAFOS.
Marcar, N. E., and Termaat, A. (1990). Effects of root-zone solutes on Eucalyptus camaldulensis and Eucalyptus bicostata seedlings: responses to Na+, Mg2+ and Cl. Plant Soil 125, 245–254. doi: 10.1007/BF00010663
Martin, B., and Thorstenson, Y. R. (1988). Stable carbon isotope composition (deltaC), water use efficiency, and biomass productivity of Lycopersicon esculentum, Lycopersicon pennellii, and the F(1) hybrid. Plant Physiol. 88, 213–217. doi: 10.1104/pp.88.1.213
Martínez, J.-P., Kinet, J.-M., Bajji, M., and Lutts, S. (2005). NaCl alleviates polyethylene glycol-induced water stress in the halophyte species Atriplex halimus L. J. Exp. Bot. 56, 2421–2431. doi: 10.1093/jxb/eri235
Mateus, N., Ferreira, E., Arthur, J. Jr., Domec, J., Jordan-Meille, J., Goncalves, J., et al. (2019). The ideal percentage of K substitution by Na in Eucalyptus seedlings: evidences from leaf carbon isotopic composition, leaf gas exchanges and plant growth. Plant Physiol. Biochem. 137, 102–112. doi: 10.1016/j.plaphy.2019.02.006
Mengel, K., Kirkby, E. A., Kosegarten, H., and Appel, T. (2001). “Potassium,” in Principles of Plant Nutrition, 5th Edn, eds K. Mengel, E. A. Kirkby, H. Kosegarten, and T. Appel (Dordrecht: Springer Netherlands), 481–511. doi: 10.1007/978-94-010-1009-2_10
Merchant, A., Callister, A., Arndt, S., Tausz, M., and Adams, M. (2007). Contrasting physiological responses of six Eucalyptus species to water deficit. Ann. Bot. 100, 1507–1515. doi: 10.1093/aob/mcm234
Merchant, A., Tausz, M., Arndt, S. K., and Adams, M. A. (2006). Cyclitols and carbohydrates in leaves and roots of 13 Eucalyptus species suggest contrasting physiological responses to water deficit. Plant Cell Environ. 29, 2017–2029. doi: 10.1111/j.1365-3040.2006.01577.x
Mott, K. A., and Michaelson, O. (1991). Amphistomy as an adaptation to high light intensity in Ambrosia cordifolia (Compositae). Am. J. Bot. 78, 76–79. doi: 10.2307/2445230
Müller, C., Hodecker, B. E. R., Merchant, A., and de Barros, N. F. (2017). Nutritional efficiency of eucalyptus clones under water stress. Rev. Bras. Cienc. Solo 41:e0160528. doi: 10.1590/18069657rbcs20160528
Munns, R., and Tester, M. (2008). Mechanisms of salinity tolerance. Annu. Rev. Plant Biol. 59, 651–681. doi: 10.1146/annurev.arplant.59.032607.092911
Näslund, P. H., and Hultin, T. (1971). Structural and functional defects in mammalian ribosomes after potassium deficiency. Biochim. Biophys. Acta Nucleic Acids Protein Synth. 254, 104–116. doi: 10.1016/0005-2787(71)90117-1
Novais, R., Neves, J., and Barros, N. (1991). “Ensaio em ambiente controlado,” in Métodos de Pesquisa Em Fertilidade do Solo, ed. A. J. Oliveira (Brasilília: EMBRAPA-SEA), 189–253.
Oddo, E., Inzerillo, S., La Bella, F., Grisafi, F., Salleo, S., and Nardini, A. (2011). Short-term effects of potassium fertilization on the hydraulic conductance of Laurus nobilis L. Tree Physiol. 31, 131–138. doi: 10.1093/treephys/tpq115
Paquette, A., and Messier, C. (2010). The role of plantations in managing the world’s forests in the Anthropocene. Front. Ecol. Environ. 8:27–34. doi: 10.1890/080116
Pardo, J. M., and Quintero, F. J. (2002). Plants and sodium ions: keeping company with the enemy. Genome Biol. 3:reviews1017.1. doi: 10.1186/gb-2002-3-6-reviews1017
Parkhurst, D. F. (1978). The adaptive significance of stomatal occurrence on one or both surfaces of leaves. J. Ecol. 66, 367–383. doi: 10.2307/2259142
Pi, Z., Stevanato, P., Yv, L. H., Geng, G., Guo, X. L., Yang, Y., et al. (2014). Effects of potassium deficiency and replacement of potassium by sodium on sugar beet plants. Russ. J. Plant Physiol. 61, 224–230. doi: 10.1134/S1021443714020101
Pita-Barbosa, A., Hodecker, B. E. R., and de Barros, N. F. (2016). Boron as mitigator of drought damage in Eucalyptus: a genotype-dependent mechanism? Sci. For. 44, 851–861. doi: 10.18671/scifor.v44n112.07
Quais, M. K., Munawar, A., Ansari, N. A., Zhou, W. W., and Zhu, Z. R. (2020). Interactions between brown planthopper (Nilaparvata lugens) and salinity stressed rice (Oryza sativa) plant are cultivar-specific. Sci. Rep. 10:8051. doi: 10.1038/s41598-020-64925-1
R Development Core Team (2018). R: A Language and Environment for Statistical Computing. Vienna: R Foundation for Statistical Computing.
Robinson, D., Handley, L. L., Scrimgeour, C. M., Gordon, D. C., Forster, B. P., and Ellis, R. P. (2000). Using stable isotope natural abundances (delta 15N and delta 13C) to integrate the stress responses of wild barley (Hordeum spontaneum C. Koch.) genotypes. J. Exp. Bot. 51, 41–50.
Rodríguez-Navarro, A., and Rubio, F. (2006). High-affinity potassium and sodium transport systems in plants. J. Exp. Bot. 57, 1149–1160. doi: 10.1093/jxb/erj068
Sarabi, B., Fresneau, C., Ghaderi, N., Bolandnazar, S., Streb, P., Badeck, F.-W., et al. (2019). Stomatal and non-stomatal limitations are responsible in down-regulation of photosynthesis in melon plants grown under the saline condition: application of carbon isotope discrimination as a reliable proxy. Plant Physiol. Biochem. 141, 1–19. doi: 10.1016/J.PLAPHY.2019.05.010
SAS Institute Inc (2004). SAS/INSIGHT® 9.1 User’s Guide, Volumes 1 and 2. Cary, NC: SAS Institute Inc.
Schulze, L. M., Britto, D. T., Li, M., and Kronzucker, H. J. (2012). A pharmacological analysis of high-affinity sodium transport in barley (Hordeum vulgare L.): A 24Na+/42K+ study. J. Exp. Bot. 63, 2479–2489. doi: 10.1093/jxb/err419
Seibt, U., Rajabi, A., Griffiths, H., and Berry, J. A. (2008). Carbon isotopes and water use efficiency: sense and sensitivity. Oecologia 155, 441–454. doi: 10.1007/s00442-007-0932-7
Shabala, S. N., and Lew, R. R. (2002). Turgor regulation in osmotically stressed Arabidopsis epidermal root cells. Direct support for the role of inorganic ion uptake as revealed by concurrent flux and cell turgor measurements. Plant Physiol. 129, 290–299. doi: 10.1104/pp.020005
Shvaleva, A. L., Costa, F., Breia, E., Jouve, L., Hausman, J. F., Almeida, M. H., et al. (2006). Metabolic responses to water deficit in two Eucalyptus globulus clones with contrasting drought sensitivity. Tree Physiol. 26, 239–248. doi: 10.1093/treephys/26.2.239
Siddiqi, M. Y., and Glass, A. D. M. (1981). Utilization index: a modified approach to the estimation and comparison of nutrient utilization efficiency in plants. J. Plant Nutr. 4, 289–302. doi: 10.1080/01904168109362919
Smethurst, P. J. (2010). Forest fertilization: trends in knowledge and practice compared to agriculture. Plant Soil 335, 83–100. doi: 10.1007/s11104-010-0316-3
Speer, M., and Kaiser, W. M. (1991). Ion relations of symplastic and apoplastic space in leaves from Spinacia oleracea L. and Pisum sativum L. under salinity. Plant Physiol. 97, 990–997. doi: 10.1104/pp.97.3.990
Spyrides, G. J. (1964). The effect of univalent cations on the binding of sRNA to the template-ribosome complex. Proc. Natl. Acad. Sci.U.S.A. 51, 1220–1226. doi: 10.1073/pnas.51.6.1220
Steudle, E. (2000). Water uptake by roots: effects of water deficit. J. Exp. Bot. 51, 1531–1542. doi: 10.1093/jexbot/51.350.1531
Subbarao, G. V., Ito, O., Berry, W. L., and Wheeler, R. M. (2003). Sodium - A functional plant nutrient. CRC. Crit. Rev. Plant Sci. 22, 391–416. doi: 10.1080/07352680390243495
Subbarao, G. V., Wheeler, R. M., Stutte, G. W., and Levine, L. H. (1999). How-far can sodium substitute for potassium in red beet? J. Plant Nutr. 22, 1745–1761. doi: 10.1080/01904169909365751
Tanaka, Y., Sano, T., Tamaoki, M., Nakajima, N., Kondo, N., and Hasezawa, S. (2006). Cytokinin and auxin inhibit abscisic acid-induced stomatal closure by enhancing ethylene production in Arabidopsis. J. Exp. Bot. 57, 2259–2266. doi: 10.1093/jxb/erj193
Tariq, A., Pan, K., Olatunji, O. A., Graciano, C., Li, Z., Li, N., et al. (2019). Impact of phosphorus application on drought resistant responses of Eucalyptus grandis seedlings. Physiol. Plant 166, 894–908. doi: 10.1111/ppl.12868
Tavakol, E., Jákli, B., Cakmak, I., Dittert, K., Karlovsky, P., Pfohl, K., et al. (2018). Optimized potassium nutrition improves plant-water-relations of barley under PEG-induced osmotic stress. Plant Soil 430, 23–35. doi: 10.1007/s11104-018-3704-8
Terry, N., and Ulrich, A. (1973). Effects of potassium deficiency on the photosynthesis and respiration of leaves of sugar beet. Plant Physiol. 51, 783–786. doi: 10.1104/pp.51.4.783
Tränkner, M., Tavakol, E., and Jákli, B. (2018). Functioning of potassium and magnesium in photosynthesis, photosynthate translocation and photoprotection. Physiol. Plant 163, 414–431. doi: 10.1111/ppl.12747
Turner, N. C. (1981). Techniques and experimental approaches for the measurement of plant water status. Plant Soil 58, 339–366.
Wakeel, A., Farooq, M., Qadir, M., and Schubert, S. (2011). Potassium substitution by sodium in plants. CRC. Crit. Rev. Plant Sci. 30, 401–413. doi: 10.1080/07352689.2011.587728
Wang, M., Zheng, Q., Shen, Q., and Guo, S. (2013). The critical role of potassium in plant stress response. Int. J. Mol. Sci. 14, 7370–7390. doi: 10.3390/ijms14047370
Warren, C. R., Bleby, T., and Adams, M. A. (2007). Changes in gas exchange versus leaf solutes as a means to cope with summer drought in Eucalyptus marginata. Oecologia 154, 1–10. doi: 10.1007/s00442-007-0803-2
White, D. A., Crombie, D. S., Kinal, J., Battaglia, M., McGrath, J. F., Mendham, D. S., et al. (2009). Managing productivity and drought risk in Eucalyptus globulus plantations in south-western Australia. For. Ecol. Manage. 259, 33–44. doi: 10.1016/J.FORECO.2009.09.039
Xi, J. J., Chen, H. Y., Bai, W. P., Yang, R. C., Yang, P. Z., Chen, R. J., et al. (2018). Sodium-related adaptations to drought: new insights from the xerophyte plant zygophyllum xanthoxylum. Front. Plant Sci. 871:1678. doi: 10.3389/fpls.2018.01678
Xu, Z., Zhou, G., and Shimizu, H. (2009). Are plant growth and photosynthesis limited by pre-drought following rewatering in grass? J. Exp. Bot. 60, 3737–3749. doi: 10.1093/jxb/erp216
Yue, L. J., Li, S. X., Ma, Q., Zhou, X. R., Wu, G. Q., Bao, A. K., et al. (2012). NaCl stimulates growth and alleviates water stress in the xerophyte Zygophyllum xanthoxylum. J. Arid Environ. 87, 153–160. doi: 10.1016/j.jaridenv.2012.06.002
Keywords: sodium application, drought, stable carbon isotope, water use efficiency, water consumption, photosynthesis
Citation: Mateus NS, Florentino AL, Santos EF, Ferraz AV, Goncalves JLM and Lavres J (2021) Partial Substitution of K by Na Alleviates Drought Stress and Increases Water Use Efficiency in Eucalyptus Species Seedlings. Front. Plant Sci. 12:632342. doi: 10.3389/fpls.2021.632342
Received: 23 November 2020; Accepted: 28 January 2021;
Published: 15 March 2021.
Edited by:
Jorge Rodriguez-Celma, John Innes Centre, United KingdomReviewed by:
Haijun Gong, Northwest A and F University, ChinaRoslyn Gleadow, Monash University, Australia
Copyright © 2021 Mateus, Florentino, Santos, Ferraz, Goncalves and Lavres. This is an open-access article distributed under the terms of the Creative Commons Attribution License (CC BY). The use, distribution or reproduction in other forums is permitted, provided the original author(s) and the copyright owner(s) are credited and that the original publication in this journal is cited, in accordance with accepted academic practice. No use, distribution or reproduction is permitted which does not comply with these terms.
*Correspondence: Nikolas de Souza Mateus, nikolas.mateus@usp.br