- 1Department of Biological Sciences, Korea Advanced Institute of Science and Technology (KAIST), Daejeon, South Korea
- 2Graduate School of Biotechnology and Crop Biotech Institute, Kyung Hee University, Yongin-si, South Korea
- 3Research Center for Materials Analysis, Korea Basic Science Institute, Daejeon, South Korea
Starch granules in the endodermis of plant hypocotyls act as statoliths that promote hypocotyl negative gravitropism—the directional growth of hypocotyls against gravity—in the dark. To identify the molecular components that regulate hypocotyl negative gravitropism, we performed a mutagenesis screen and isolated reduced gravitropic 1 (rgv1) mutants that lack starch granules in their hypocotyl endodermis and show reduced hypocotyl negative gravitropism in the dark. Using whole genome sequencing, we identified three different rgv1 mutants that are allelic to the previously reported early starvation 1 mutant, which is rapidly depleted of starch just before the dawn. ESV1 orthologs are present in starch-producing green organisms, suggesting ESV1 is a functionally conserved protein necessary for the formation of starch granules. Consistent with this, we found that liverwort and rice ESV1 can complement the Arabidopsis ESV1 mutant phenotype for both starch granules and hypocotyl negative gravitropism. To further investigate the function of ESV1 in other plants, we isolated rice ESV1 mutants and found that they show reduced levels of starch in their leaves and loosely packed starch granules in their grains. Both Arabidopsis and rice ESV1 mutants also lack starch granules in root columella and show reduced root gravitropism. Together, these results indicate ESV1 is a functionally conserved protein that promotes gravitropic responses in plants via its role in starch granule formation.
Introduction
As sessile organisms, plants need to adjust their growth and development in response to various environmental factors. Gravity is one of these environmental factors that determines the growth orientation of plants via a process called gravitropism. Roots show positive gravitropism when they grow in the direction of gravity, while shoots including hypocotyls show negative gravitropism by growing in the direction opposite to gravity (Knight, 1806; Kiss, 2000). Plant gravitropic responses can be conceptually divided into four steps: gravity sensing, cellular signal formation, intercellular signal transmission, and asymmetric organ growth (Morita and Tasaka, 2004; Morita, 2010; Hashiguchi et al., 2013; Nakamura et al., 2019). Plants sense gravity via the directional sedimentation of amyloplasts, which are plastids filled with starch granules (Sack, 1991; Toyota et al., 2013). These amyloplasts move with gravity inside statocytes, gravity-sensing cells that include the columella cells in roots and the endodermal cells in hypocotyls and stems (Hashiguchi et al., 2013; Nakamura et al., 2019). The directional sedimentation of these amyloplasts induces an asymmetric localization of auxin efflux carriers including PIN-FORMED 3 (PIN3) in the direction of gravity in the presence of LAZY family proteins (Friml et al., 2002; Yoshihara and Iino, 2007; Harrison and Masson, 2008; Kleine-Vehn et al., 2010; Rakusova et al., 2011; Yoshihara et al., 2013; Taniguchi et al., 2017; Yoshihara and Spalding, 2017). Asymmetric localization of PIN3, in turn, produces an increasing auxin concentration gradient in the direction of gravity. In response to this asymmetric distribution of auxin, plant organs elongate asymmetrically and bend either toward or against gravity. Amyloplast sedimentation does not occur via a free-falling process. In stems, endodermal cells contain a large central vacuole that limits the movement of amyloplasts to trans-vacuolar strands and to the narrow spaces between the vacuolar and plasma membranes (Saito et al., 2005). Such constriction makes proper vacuolar membrane dynamics essential for shoot gravitropism. Indeed, several shoot gravitropism mutants that are defective in vacuolar membrane dynamics show abnormal localization and sedimentation of amyloplasts (Kato et al., 2002; Morita et al., 2002; Yano et al., 2003; Hashiguchi et al., 2014).
Starch synthesis occurs in plastids with the supply of ADP-Glucose (ADP-Glc) (Zeeman et al., 2010; Streb and Zeeman, 2012). In photosynthetic cells like Arabidopsis leaf mesophyll cells, fructose 6-phosphate (Fru-6-P) produced in the Calvin-Benson cycle is converted to glucose 6-phosphate (Glc-6-P) by plastidic phosphoglucoisomerase (PGI) (Yu et al., 2000). This, in turn, is converted to glucose 1-phosphate (Glc-1-P) by phosphoglucomutase (PGM) in plastids (Caspar et al., 1985). In non-photosynthetic cells like Arabidopsis hypocotyl endodermal cells and root columella cells, sucrose is metabolized to produce Glc-6-P in the cytosol (Slewinski and Braun, 2010). Cytosolic Glc-6-P is then transported into plastids by Glc-6-P/Phosphate translocator (GPT) and converted to Glc-1-P by PGM (Kofler et al., 2000; Fischer and Weber, 2002; Niewiadomski et al., 2005; Kunz et al., 2010). In the plastids of both photosynthetic and non-photosynthetic cells, Glc-1-P is converted to ADP-Glc by ADP-glucose pyrophosphorylase (AGPase). In cereal endosperm, however, Glc-1-P is converted to ADP-Glc by cytosolic AGPase and transported into the plastids (Jeon et al., 2010; Tuncel and Okita, 2013). ADP-Glc in plastids is then used for starch synthesis. Glucan chains are branched by branching enzymes (BE) and branching patterns are further reorganized by debranching enzyme (DBE). Linear (amylose) and highly branched (amylopectin) glucan chains are then packed in multiple layers of semi-crystalline and amorphous structures to form large starch granules (Zeeman et al., 2010). Starch granules in plastids are degraded by several enzymes (Smith et al., 2005; Streb and Zeeman, 2012). Starch degradation is initiated by the transient phosphorylation of glucosyl residues on the surface of amylopectin at the C6 and C3 positions by glucan, water dikinase (GWD) and phosphoglucan, water dikinase (PWD), respectively (Lorberth et al., 1998; Ritte et al., 2002, 2006; Baunsgaard et al., 2005; Kotting et al., 2005). This phosphorylation loosens the semi-crystalline structure of amylopectins on the surface of starch granules, making it accessible to other enzymes (Hejazi et al., 2008, 2009).
Phytochromes (phyA to phyE) are red and far-red light receptors promoting light responses (Legris et al., 2019). The phytochromes accomplish this in part via their inhibition of Phytochrome Interacting Factors (PIFs), a group of bHLH transcription factors (Leivar and Quail, 2011; Lee and Choi, 2017; Pham et al., 2018). Phytochromes suppress hypocotyl negative gravitropism by inhibiting PIFs in the endodermis (Shin et al., 2009; Kim et al., 2011). In the dark, Arabidopsis seedlings possess endodermal amyloplasts with large starch granules that act as statoliths. These allow them to grow against the direction of gravity, thus showing hypocotyl negative gravitropism. In monochromatic red or far-red light, which activate phyB and phyA, respectively, these endodermal plastids show much smaller starch granules. This causes the seedlings to grow in random directions, displaying hypocotyl agravitropism. Unlike wild type seedlings, pifQ mutant seedlings (lacking PIF1, PIF3, PIF4, and PIF5) have endodermal plastids with small starch granules and show reduced hypocotyl negative gravitropism in the dark (Shin et al., 2009; Kim et al., 2011). These pifQ mutant phenotypes are fully rescued by endodermis-specific expression of PIF1. This indicates PIFs promote hypocotyl negative gravitropism in the endodermis in part by maintaining large starch granules in endodermal amyloplasts in the dark (Kim et al., 2011). It is not yet clear how PIFs promote starch granule formation and hypocotyl negative gravitropism in the endodermis.
In a mutagenesis screen with SCRp:PIF1/pifQ, a transgenic line expressing PIF1 under the endodermis-specific SCARECROW (SCR) promoter in the pifQ mutant background, we isolated several reduced gravitropic (rgv) mutants. We then further characterized rgv1, allelic to ESV1, mutants, which lack starch granules both in the endodermis and the root columella in the dark. By analyzing Arabidopsis and rice ESV1 mutants as well as transgenic plants expressing ESV1 from liverwort, Arabidopsis, and rice, we have identified ESV1 as a functionally conserved protein that promotes gravitropism in land plants via its role in the formation of starch granules.
Results
Three reduced gravitropic Mutants Lack Starch Granules in the Endodermis
We previously showed that the endodermis-specific expression of PIF1 is sufficient to rescue the endodermal starch granule and hypocotyl negative gravitropism phenotypes of pifQ mutants in the dark (Kim et al., 2011). To identify the molecular components that regulate hypocotyl negative gravitropism downstream of endodermal PIF1, we performed an ethyl methansulfonate (EMS) mutagenesis screen using a transgenic parental line expressing PIF1 under the endodermis-specific SCR promoter in pifQ mutant background (SCRp:PIF1/pifQ). From this screen, we isolated 30 reduced gravitropic (rgv) mutants that show reduced hypocotyl negative gravitropism in the dark. We classified these 30 rgv mutants into three groups based on the status of their endodermal starch granules when stained by Lugol's iodine: a starchless group lacking starch granules, a pifQ-like group with reduced starch granules, and a WT-like group with large starch granules (Figure 1A). First, we characterized the starchless group of mutants and found that it includes seven mutants that belong to three complementation groups (rgv1-1 to rgv1-3, rgv2-1 to rgv2-2, and rgv3-1). Both rgv1 and rgv2 mutants lack starch granules not only in the endodermis but also in the root columella, whereas the rgv3 mutant lacks starch granules in the endodermis but has weakly stained granules in the root columella (Figure 1B).
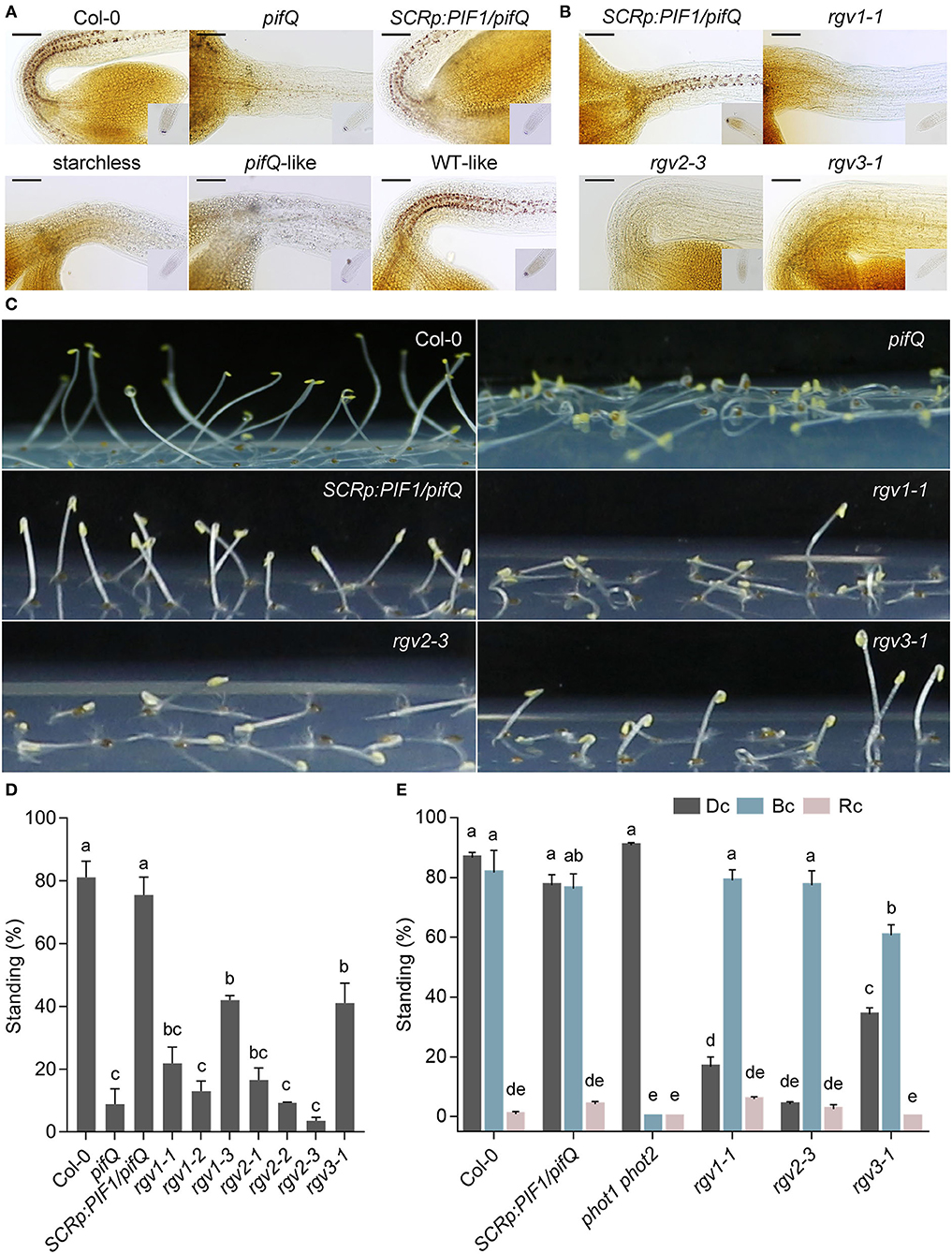
Figure 1. Three reduced gravitropic (rgv) mutants lack endodermal starch granules and show reduced hypocotyl negative gravitropism. (A) Classification of rgv mutants into three groups based on the presence of endodermal starch granules. Endodermal starch granules were visualized by staining 3-day-old dark-grown seedlings with Lugol's iodine. Col-0: wild type, pifQ: pif1 pif3 pif4 pif5 mutant, SCRp:PIF1/pifQ: the parental line expressing PIF1 under the SCR promoter in the pifQ mutant background, starchless: rgv mutants with no endodermal starch granules, pifQ-like: rgv mutants with reduced starch granules, WT-like: rgv mutants with clear endodermal starch granules. Scale bar = 100 μm. (B) Three complementation groups of starchless rgv mutants. rgv1-1, rgv2-3, and rgv3-1 represent each of the three starchless complementation groups. Scale bar = 100 μm. (C) Reduced hypocotyl negative gravitropism of three starchless rgv mutants. For the hypocotyl negative gravitropism assay, seedlings were grown for 3 days in the dark on horizontal plates. Images of three starchless rgv mutants are shown. (D) Quantification of reduced hypocotyl negative gravitropism for three starchless rgv mutants. Seedlings were grown as in (C). The degree of negative gravitropism is presented as the percentage of standing seedlings in the dark. Seedlings with cotyledons not touching the agar surface were counted as standing. Letters indicate statistical significance determined by an ANOVA with Tukey's HSD post-hoc test for multiple comparisons (p < 0.01). Error bars=SEM (n = 3 biological replicates, 40 seedlings each). (E) Normal blue light phototropism of three starchless rgv mutants. Standing seedlings were counted after being grown for 4 days in different overhead light conditions on horizontal plates. Percentages of standing seedlings in the dark and under red light represent the degrees of hypocotyl negative gravitropism in the dark and under red light, respectively. The percentage of standing seedlings under blue light represent the degree of phototropism. Dc: continuous dark, Rc: continuous red light (20 μmol/m2s), Bc: continuous blue light (1 μmol/m2s). Letters indicate statistical significance determined by an ANOVA with Tukey's HSD post-hoc test for multiple comparisons (p < 0.01). Error bars=SEM (n = 3 biological replicates, 40 seedlings each).
All three groups of rgv mutants show reduced hypocotyl negative gravitropism. When grown on horizontal agar plates in the dark, the three types of rgv mutants, like the pifQ mutant, grow along on the agar surface instead of upward like wild type and parental seedlings (Figures 1C,D). The three rgv mutants also show reduced bending toward the new direction of gravity when dark-grown seedlings are rotated by 135 degrees (Supplementary Figure 1A). Reduced gravitropic responses of these rgv mutants are not due to either a growth defect or an inherent defect in asymmetric growth of their hypocotyls; We found hypocotyl lengths of all three rgv mutants are not much different from that of parental line (Supplementary Figure 2). We also found all three rgv mutants either grow upward in the presence of overhead blue light illumination (Figure 1E) or bend toward blue light illuminated from the side (Supplementary Figure 1B). In contrast, the PHOTOTROPIN double mutant, phot1 phot2, does not bend toward blue light. Thus, the three rgv mutants show proper phototropism, which requires asymmetric growth. In addition, adult rgv mutants produce axillary branches with wider branch angles (Supplementary Figure 3). Together, these results indicate that these three rgv mutants lack endodermal starch granules and display reduced hypocotyl negative gravitropism.
Identification of RGV1, RGV2, and RGV3 Genes
We first determined if any of the rgv mutants are allelic to known starch biosynthetic mutants. Of these, the pgm and ADP-glucose pyrophosphorylase small subunit 1 (aps1) mutants, like rgv1 and rgv2, lack starch granules in both the hypocotyl endodermis and the root columella. The isoamylase 1 (isa1) and isoamylase 2 (isa2) mutants, like rgv3, lack starch granules in the hypocotyl endodermis but do show stainable granules in the root columella (Supplementary Figure 4A). We tested allelism by crossing the rgv mutants to these known starch biosynthetic mutants and staining the F1 progeny with Lugol's iodine. The F1 progeny of the cross between rgv2 and pgm did not show any starch granules in either the endodermis or the root columella, indicating rgv2 is allelic to pgm (Supplementary Figure 4B). Similarly, rgv3 is allelic to isa1. The rgv1 mutant, however, is neither allelic to pgm, nor to aps1. We further sequenced the genomic locations of PGM, APS1, ISA1, and ISA2 in the rgv mutants. Consistent with their apparent allelism, we found the rgv2 mutants have mutations in the PGM gene and the rgv3 mutant has a mutation in the ISA1 gene (Supplementary Figure 4C). The rgv1 mutants, however, did not show any mutation in PGM, APS1, ISA1, or ISA2. These results indicate the rgv1 mutants are novel mutants that lack starch granules in both the endodermis and the root columella.
To identify the gene responsible for the rgv1 phenotype, we sequenced the rgv1 mutant genomes and found that all three rgv1 mutants (rgv1-1, rgv1-2, rgv1-3) carry non-sense mutations in AT1G42430, which is previously reported as EARLY STARVATION 1 (ESV1) (Figure 2A) (Feike et al., 2016). Thus, we will refer the rgv1 mutant as the ESV1 mutant hereinafter. To prove that mutations in this gene reduce negative gravitropism, we isolated a T-DNA insertion mutant (ESV1-2, GABI_031C11) in this gene. We found that this T-DNA insertion mutant lacks starch granules in both the endodermis and root columella (Figure 2B) and shows reduced hypocotyl negative gravitropism in the dark (Figure 2C). Furthermore, we found that the ectopic expression of ESV1 under the 35S promoter in the ESV1 mutant background (ESV1-OX/ESV1-2) rescues both endodermal starch granules and hypocotyl negative gravitropism (Figures 2B,C). These results indicate the rgv1 mutant is caused by mutations in the ESV1 gene (AT1G42430). It is noticeable, however, that the ESV1 mutant still produces high levels of starch granules in hypocotyl cortex and cotyledons at the end of long day (ZT16), which is different from the pgm mutant that does not produce any starch granule and the phosphoglucoisomerase (pgi) mutant that produces high levels of starch granules in the hypocotyl endodermis but strongly reduced levels in cotyledons at ZT16 (Supplementary Figure 5). At 4 h after the start of night (ZT20), starch granules are depleted in the ESV1 mutant but not in wild type and the pgi mutant. Such starch granule accumulation patterns in different tissues indicate that ESV1 is essential to form starch granules in the endodermis where starch is formed from transported glucose-6-phosphates and to maintain starch granules from the hasty depletion during the night. Arabidopsis also possesses an ESV1 homolog (AT3G55760, LESV) that is 44% identical at the amino acid level to ESV1. Unlike ESV1 mutants, however, mutations in LESV do not disrupt endodermal starch granules (Figure 2B), neither do they affect hypocotyl negative gravitropism in the dark (Figure 2C). The ectopic expression of LESV under the 35S promoter in the ESV1 mutant background (LESV-OX/ESV1-2) does not rescue the ESV1 mutant endodermal starch granule or hypocotyl negative gravitropism phenotypes (Figures 2B,C), indicating ESV1 and LESV do not have the same function.
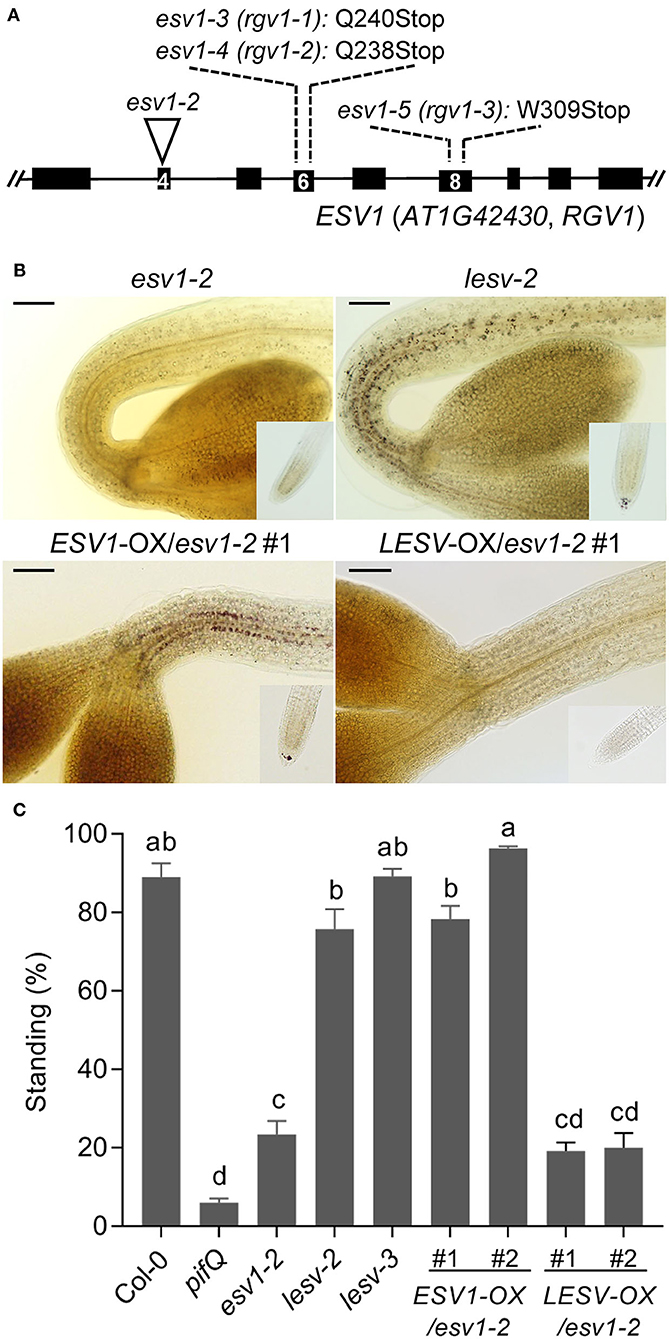
Figure 2. Three reduced gravitropic 1 mutant alleles have non-sense mutations in AT1G42430. (A) A diagram showing these three rgv1 mutant alleles and a T-DNA insertion mutant allele. The mutated loci in these three rgv1 alleles (rgv1-1, rgv1-2, rgv1-3) were determined by whole-genome sequencing. All three rgv1 alleles have non-sense mutations in AT1G42430, indicated by the wild type amino acid with its position number and Stop. A T-DNA insertion line (ESV1-2, GABI_031C11) was obtained from the stock center. Black bars: exons, Lines: introns, Numbers in black bars: exon number, Inverted triangle: T-DNA insertion site. (B) Restoration of endodermal starch granules in the ESV1 mutant via ectopic expression of ESV1 but not LESV. Endodermal starch granules were visualized by staining 3-day-old dark-grown seedlings with Lugol's iodine. ESV1-2: a T-DNA insertion mutant in AT1G42430 (GABI-031C11), lesv-2: a T-DNA insertion mutant in AT3G55760 (Salk_006705), ESV1-OX/ESV1-2: a transgenic line expressing ESV1 under the 35S promoter in the ESV1-2 mutant background, LESV-OX/ESV1-2: a transgenic line expressing LESV under the 35S promoter in the ESV1-2 mutant background. Scale bar = 100 μm. (C) Restoration of hypocotyl gravitropism in ESV1 mutants via the ectopic expression of ESV1 but not LESV. Seedlings were grown for 3 days in the dark on horizontal plates and the degree of negative gravitropism was measured by quantifying the percentage of standing seedlings. Seedlings were counted as standing if their cotyledons did not touch the agar surface. Col-0: wild type, pifQ: pif1 pif3 pif4 pif5 mutant, ESV1-2: a T-DNA insertion mutant of AT1G42430 (GABI-031C11), lesv-2: a T-DNA insertion mutant of AT3G55760 (Salk_006705), lesv-3: a T-DNA insertion mutant of AT3G55760 (Salk_006697), ESV1-OX/ESV1-2: transgenic plants expressing ESV1 under the 35S promoter in the ESV1-2 mutant background, LESV-OX/ESV1-2: transgenic plants expressing LESV under the 35S promoter in the ESV1-2 mutant background. Letters indicate statistical significance determined by an ANOVA with Tukey's HSD post-hoc test for multiple comparisons (p < 0.01). Error bars=SEM (n = 3 biological replicates, 40 seedlings each).
ESV1 is reported to be localized in plastids and prevents the hasty depletion of starch reserves during the night (Feike et al., 2016). Consistent with previous reports, we observed punctate ESV1-GFP signal in the chloroplasts of light-grown mesophyll cells (Supplementary Figure 6A) and in the plastids of dark-grown upper hypocotyl endodermal cells possessing large starch granules. In dark-grown hypocotyl epidermal cells that lack starch granules, however, although the ESV1-GFP signal is localized to plastids, it does not form punctate signals (Supplementary Figures 6B,C). This is consistent with a previous report that ESV1 is a plastid-localized protein that can associate with starch granules.
ESV1 Acts Cell Autonomously in the Endodermis to Promote Hypocotyl Negative Gravitropism via Its Role in Starch Granule Formation
Since the endodermis is a heterotrophic tissue that obtains carbon from other tissues (José Muñoz et al., 2006), we asked if ESV1 acts cell-autonomously in the endodermis to assist in the formation of starch granules, subsequently leading to a hypocotyl negative gravitropism phenotype when it is mutated. We generated transgenic lines expressing ESV1 under the control of the endodermis-specific SCR promoter in the ESV1-2 mutant background (SCRp:ESV1/ESV1-2) and transgenic lines expressing ESV1 under the control of the epidermis-specific MERISTEM LAYER 1 (ML1) promoter in the same ESV1-2 mutant background (ML1p:ESV1/ESV1-2) (Di Laurenzio et al., 1996; Sessions et al., 1999; Heidstra et al., 2004). When we stained these seedlings with Lugol's iodine, we observed clear starch granules in the upper hypocotyl endodermis of dark-grown SCRp:ESV1/ESV1-2 lines, but not in ML1p:ESV1/ESV1-2 lines (Figure 3A). This indicates that ESV1 expressed in the endodermis but not the epidermis is able to restore starch granules in the endodermis. Consistent with this restoration of endodermal starch granules, we also observed a rescue of the hypocotyl negative gravitropism phenotype only in the SCRp:ESV1/ESV1-2 lines, not in the ML1p:ESV1/ESV1-2 lines (Figure 3B). The inability of epidermis-specific ESV1 to rescue starch granules and the hypocotyl negative gravitropism phenotype was not due to lower levels of ESV1 mRNA expression; ML1p:ESV1/ESV1-2 line expresses similar levels of ESV1 mRNA than SCRp:ESV1/ESV1-2 line (Supplementary Figure 7). Together, these results indicate ESV1 acts cell autonomously in the endodermis in the formation of starch granules, subsequently contributing to hypocotyl negative gravitropism.
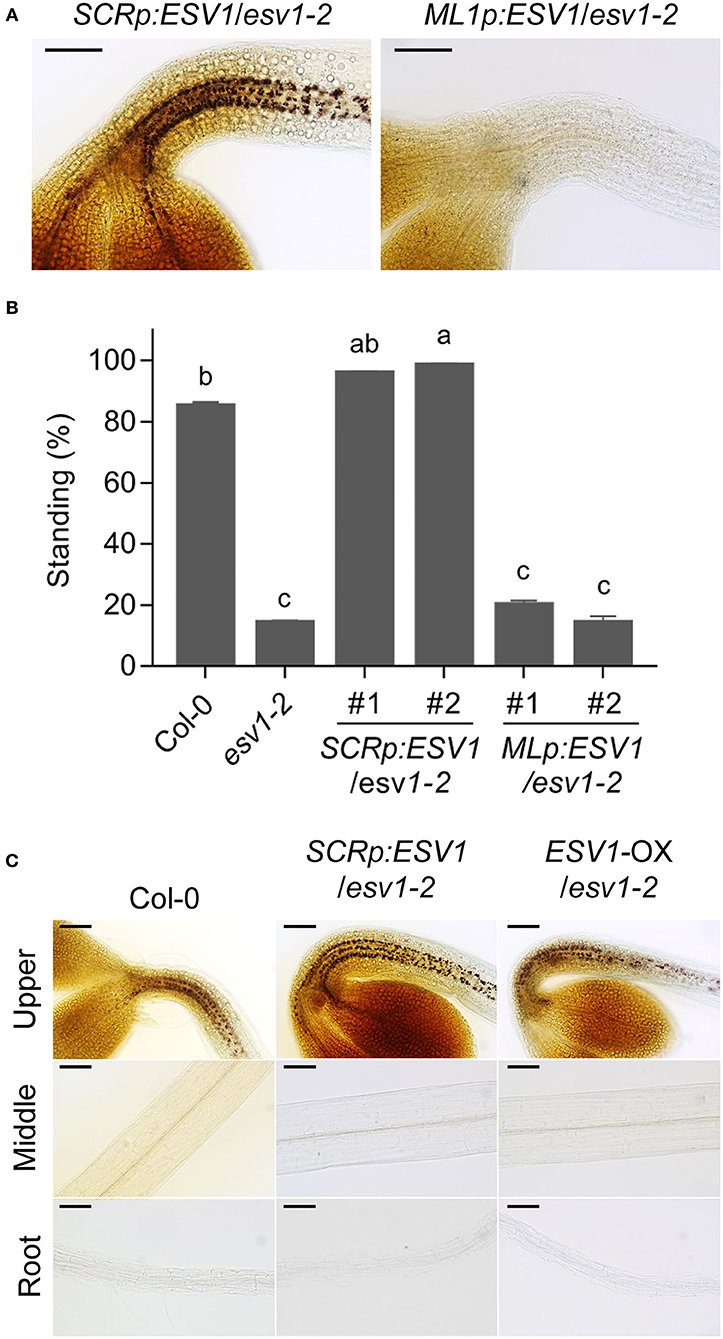
Figure 3. ESV1 promotes hypocotyl negative gravitropism in the endodermis. (A) Restoration of endodermal starch granules by endodermal but not epidermal ESV1. Endodermal starch granules were visualized by staining 3-day-old dark-grown transgenic seedlings with Lugol's iodine. SCRp:ESV1/ESV1-2: transgenic plants expressing ESV1 under the endodermis-specific SCR promoter in the ESV1-2 mutant background, ML1p:ESV1/ESV1-2: transgenic plants expressing ESV1 under the epidermis-specific ML1 promoter in the ESV1-2 mutant background. Scale bar = 100 μm. (B) Restoration of hypocotyl negative gravitropism by endodermal but not epidermal ESV1. Seedlings were grown for 3 days in the dark on horizontal plates taking the percentage of standing seedlings as an indicator of the level of negative gravitropism. Standing seedlings had cotyledons that did not touch the agar surface. Two independent transgenic lines for each tissue-specific promoter are indicated. Letters indicate statistical significance determined by an ANOVA with Tukey's HSD post-hoc test for multiple comparisons (p < 0.01). Error bars=SEM (n = 3 biological replicates, 40 seedlings each). (C) Insufficiency of ESV1 for the ectopic formation of starch granules in the lower hypocotyl and roots. Starch granules were visualized by staining 3-day-old dark-grown transgenic seedlings with Lugol's iodine. ESV1-OX/ESV1-2: transgenic plant expressing ESV1 under the 35S promoter in the ESV1-2 mutant background. Scale bar = 100 μm.
We found, however, that the ectopic expression of ESV1 is itself insufficient to induce the production of starch granules. The SCR promoter is active not only in the hypocotyl endodermis but also in the endodermis of other tissues including roots. The SCRp:ESV1/ESV1-2 line, however, forms starch granules only in the upper hypocotyl endodermis, not in the middle hypocotyl endodermis or root endodermis (Figure 3C). Neither does the expression of ESV1 under the control of the ML1 promoter induce the formation of starch granules in the epidermis (Figure 3A). Even when we expressed ESV1 ubiquitously under the control of the 35S promoter (ESV1-OX/ESV1-2), we only observed starch granule formation in the upper hypocotyl endodermis (Figure 3C). These results indicate ESV1 is necessary but insufficient for ectopic starch granule formation.
Phytochromes Do Not Remove Starch Granules by Repressing ESV1 Expression
PIFs promote hypocotyl negative gravitropism partly by maintaining starch granules in the endodermis in the dark. phyB suppresses hypocotyl negative gravitropism by inhibiting PIFs in red light (Kim et al., 2011). Since ESV1 is necessary to maintain starch granules in the endodermis, we asked if phyB and PIFs regulate starch granules through ESV1. We first determined the expression of ESV1 mRNA. In contrast to the expression of PHYTOCHROME INTERACTING FACTOR 3-LIKE 1 (PIL1), a PIF direct target gene used as a marker (Park et al., 2018), we found no evidence of red light-induced ESV1 mRNA repression in wild type seedlings or de-repression in phyB mutant seedlings (Figure 4A). This indicates phyB does not repress the expression of ESV1 mRNA in red light. We also found no evidence of ESV1 mRNA repression in pifQ mutants in the dark, confirming that PIFs do not activate the expression of ESV1 mRNA in the dark. Furthermore, red light still reduces endodermal starch granules in SCRp:ESV1/ESV1-2 seedlings (Figure 4B) expressing ESV1 mRNA under the control of the SCR promoter. These results support that phyB and PIFs do not regulate starch granules via their regulation of ESV1 mRNA expression. We next used a transgenic line expressing ESV1-GFP under the control of the 35S promoter to determine whether phyB reduces starch granules by destabilizing ESV1 protein. We found that red light does not reduce ESV1 protein levels (Figure 4C). Neither does red light reduce polysome-bound ESV1 mRNA levels (Liu et al., 2013), suggesting phytochromes do not repress the translation of ESV1 mRNA in red light. Together, these results indicate phyB removes endodermal starch granules in red light neither by repressing ESV1 mRNA levels nor by decreasing ESV1 protein levels. Auxin is known to promote starch granule accumulation in root tips by activating the expression of starch biosynthetic genes, including PGM and APS1 (Zhang et al., 2019a). Auxin does not, however, regulate the expression of ESV1 mRNA (Figure 4D). Together, our results indicate ESV1 mRNA expression is not the main point of regulation in the control of starch granule accumulation.
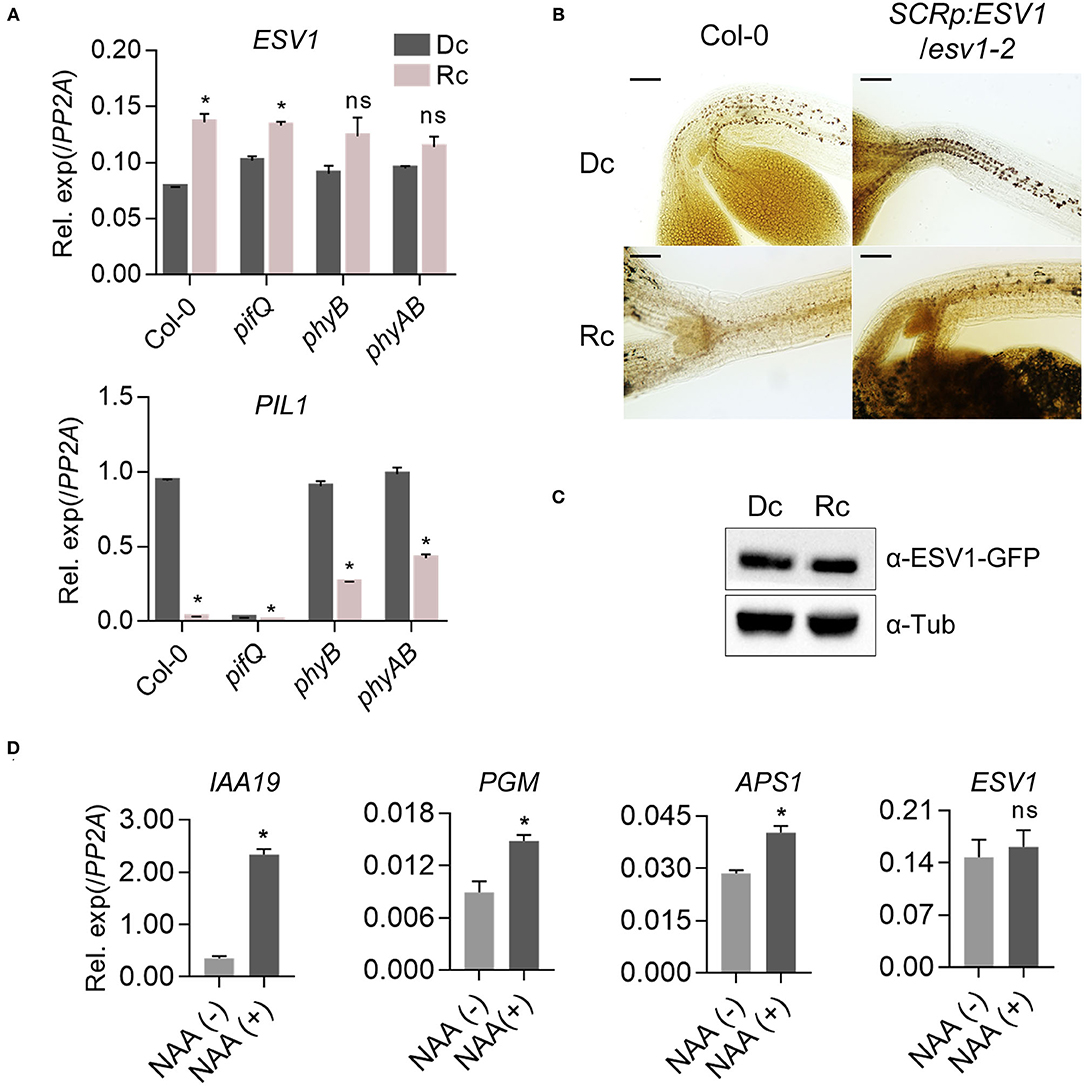
Figure 4. PhyB does not repress ESV1 to degrade endodermal starch granules. (A) No repression of ESV1 mRNA expression by red light. Three-day-old seedlings grown either in the dark (Dc) or red light (Rc) were used for mRNA expression analysis. Col-0: wild type, pifQ: pif1 pif3 pif4 pif5 mutant. phyB: phyB-9 mutant. phyAB: phyA-211 phyB-9 double mutant. Relative mRNA expression was normalized by PP2A. Asterisks indicate significant differences from dark condition (*p < 0.05; Student's t-test). n.s indicates no significant difference. Error bars=SEM (n = 3 biological replicates). (B) Degradation of starch granules by red light even in transgenic seedlings expressing ESV1 under the SCR promoter. Endodermal starch granules were visualized by staining 3-day-old seedlings grown in dark (Dc) or red light (Rc) with Lugol's iodine. Col-0: wild type, SCRp:ESV1/ESV1-2: transgenic plants expressing ESV1 by endodermis-specific SCR promoter in the ESV1-2 mutant background. Scale bar = 100 μm. (C) No destabilization of ESV1 protein by red light. Transgenic seedlings expressing ESV1-GFP under the 35S promoter in the wild type background (35Sp:ESV1-GFP) were grown for 4 days either in the dark (Dc) or under red light (Rc) and ESV1-GFP protein levels were detected by Western blot using a GFP-specific antibody (α-GFP). α-tubulin was detected using an α-tubulin-specific antibody (α-Tub). The intensity of ESV1-GFP was normalized by α-tublin (n = 3, biological replicates). (D) No activation of ESV1 mRNA expression by auxin. Seven-day-old seedlings grown in the light on a nylon mesh were transferred to media with [NAA(+)] or without [NAA(-)] auxin. At 48 h after the incubation, the root tips were removed with a blade and collected for RNA purification. Relative mRNA expression was normalized to PP2A. Asterisks indicate significant differences from NAA(-) condition (*p < 0.05; Student's t-test). ns indicates no significant difference. Error bars=SEM (n = 3 biological replicates).
ESV1 Gene From a Liverwort or Rice Can Complement Arabidopsis ESV1 Mutant
ESV1 orthologs are present in the genomes of various starch-producing eukaryotic green lineages including liverwort (Marchantia polymorpha) and rice (Oryza sativa) but not in those of cyanobacteria that do not produce starch. To investigate the relationship between ESV1's conservation and the formation of starch granules, we generated transgenic Arabidopsis seedlings expressing either liverwort ESV1 (Marchantia polymorpha, Mp-ESV1 (Mapoly0189s0007), 74% amino acid identity to At-ESV1) or rice ESV1 (Oryza sativa, Os-ESV1(LOC_Os03g04100), 88% amino acid identity to At-ESV1) under the control of the endodermis-specific SCR promoter in the ESV1 mutant background (SCRp:MpESV1/ESV1-2, SCRp:OsESV1/ESV1-2). If the ESV1 proteins from these species are functionally conserved, these transgenic seedlings should produce endodermal starch granules. We found that both Mp-ESV1 and Os-ESV1 fully rescue the production of endodermal starch granules (Figure 5A) and hypocotyl negative gravitropism in Arabidopsis ESV1 mutant seedlings (Figure 5B). Furthermore, in SCRp:MpESV1/ESV1-2 and SCRp:OsESV1/ESV1-2 seedlings, just as in SCRp:ESV1/ESV1-2 seedlings, red light inhibits the production of starch granules and hypocotyl negative gravitropism (Figures 5A,B). Together, these results indicate ESV1 proteins from liverwort and rice are functionally conserved in Arabidopsis.
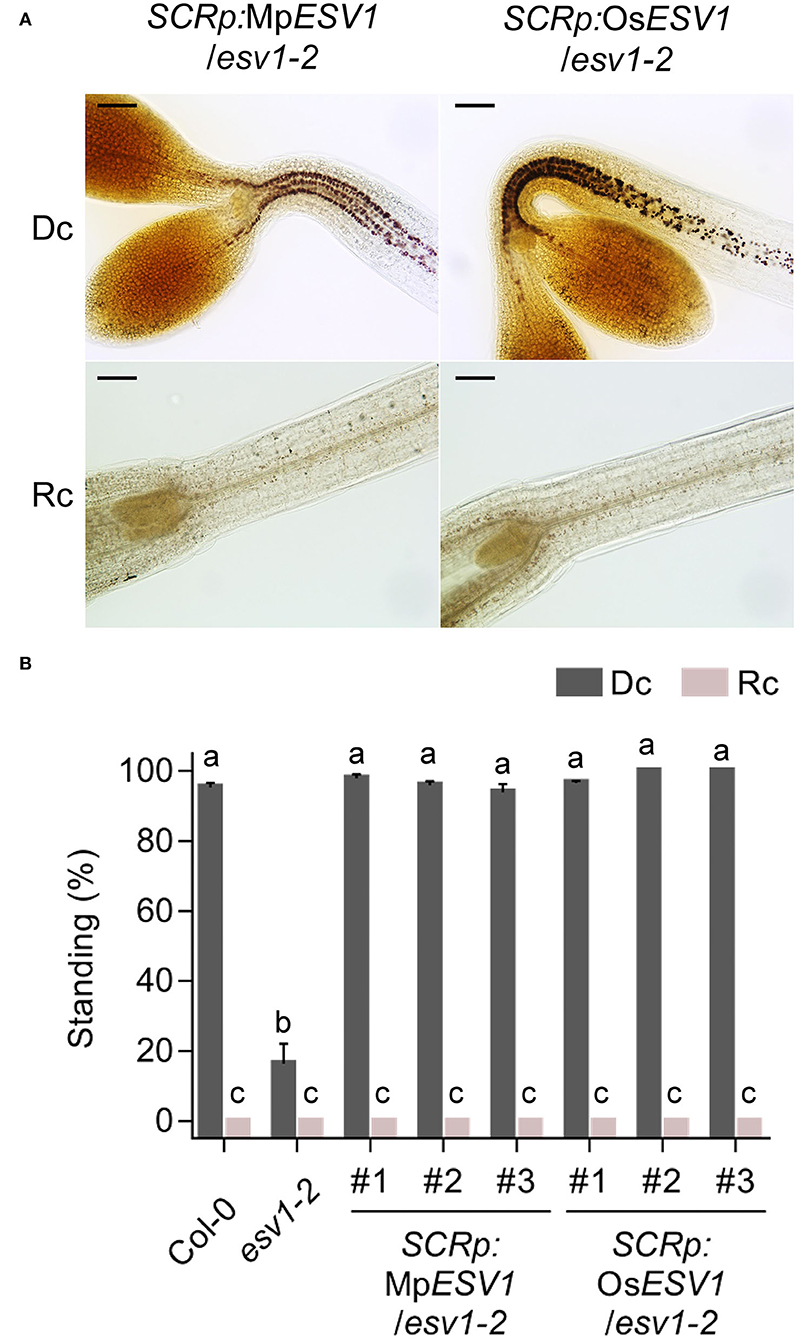
Figure 5. Liverwort and rice ESV1 genes can rescue the Arabidopsis ESV1 mutant. (A) Liverwort and rice ESV1 rescue endodermal starch granules in the Arabidopsis ESV1 mutant background. Transgenic seedlings expressing either liverwort or rice ESV1 under the SCR promoter in the ESV1-2 mutant background (SCRp:MpESV1/ESV1-2, SCRp:OsESV1/ESV1-2, were grown for 3 days either in the dark (Dc) or in red light (Rc). Then, starch granules were visualized by staining the seedlings with Lugol's iodine. Scale bar = 100 μm. (B) Liverwort and rice ESV1 rescue hypocotyl negative gravitropism in the Arabidopsis ESV1 mutant background. Seedlings were grown for 3 days in the dark (Dc) or in red light (Rc) on horizontal plates. The percentage of standing seedlings indicates the degree of negative gravitropism. Seedlings whose cotyledons did not touch the agar surface were counted as standing. The numbers indicate independent transgenic lines. Letters indicate statistical significance determined by an ANOVA with Tukey's HSD post-hoc test for multiple comparisons (p < 0.01). Error bars=SEM (n = 3 biological replicates, 40 seedlings each).
Rice ESV1 Promotes Tight Packing of Starch Granules in Grains
The fact that Mp-ESV1 and Os-ESV1 rescue Arabidopsis ESV1 mutant phenotypes suggests ESV1 is a functionally conserved protein that promotes gravitropism via its role in starch granule formation in Arabidopsis and in other plants. We therefore investigated the function of rice ESV1 by characterizing two independent rice T-DNA insertion mutants (osESV1-1, osESV1-2) in two different rice cultivars (Figure 6A). These two rice ESV1 mutants have no obvious growth defects and bear starch-filled grains in greenhouse conditions under a 14-h-light/10-h-dark photoperiod (Figure 6B). This is in contrast with the rice starch biosynthetic mutants ADP-glucose pyrophosphorylase small subunit 2 (osaps2) and ADP-glucose pyrophosphorylase large subunit 2 (osapl2) mutants, which produce shrunken grains due to a lack of starch biosynthesis in the endosperm (Lee et al., 2007), and the ospgm and ADP-glucose pyrophosphorylase large subunit 4 (osapl4) mutants, which are male sterile because of a lack of starch reserves in their pollen grains (Lee et al., 2016). These phenotypic differences suggest rice ESV1 mutants store enough starch to support grain filling and pollen survival.
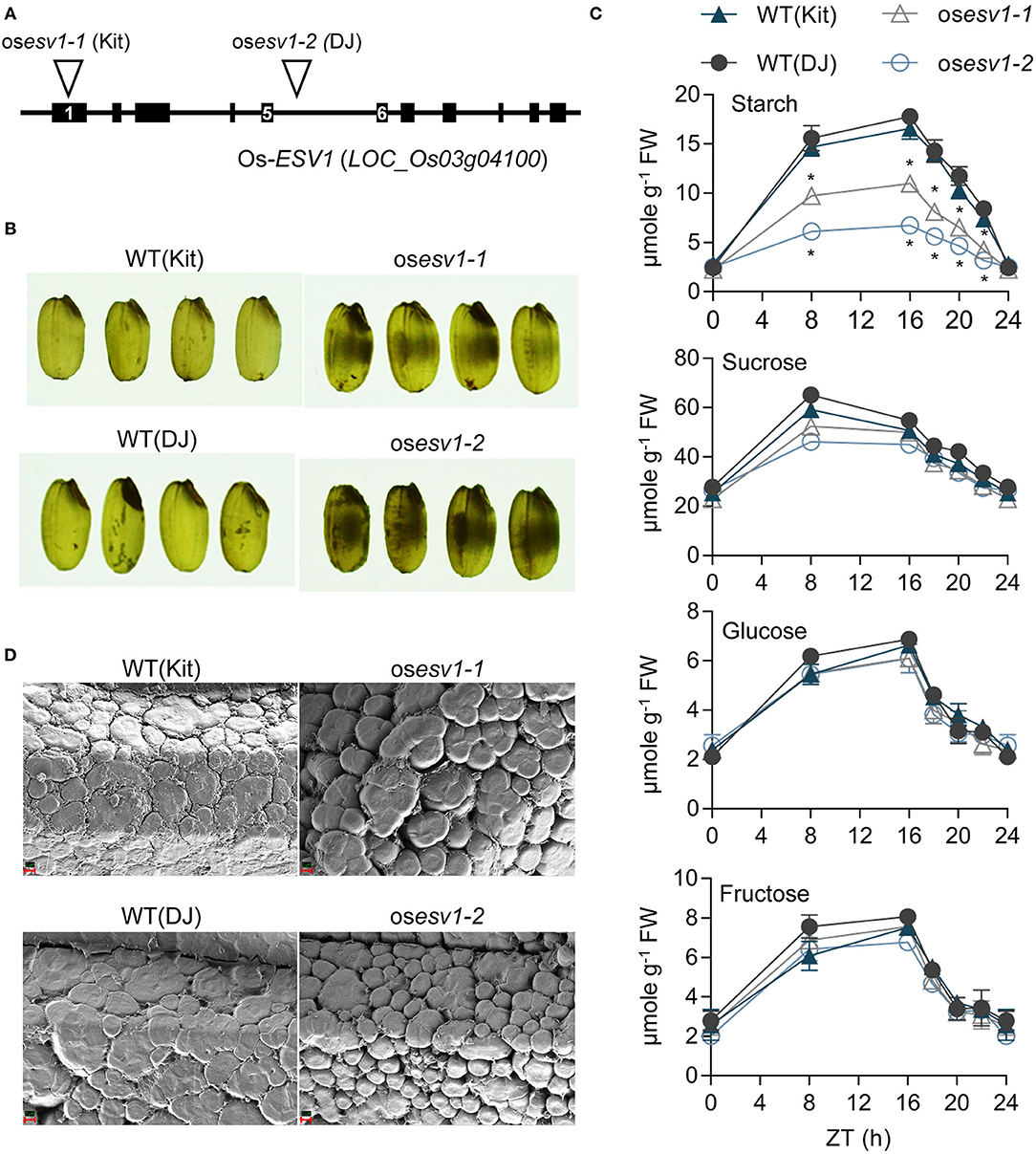
Figure 6. Rice ESV1 promotes the accumulation of starch in leaves and the tight packing of starch granules in grains. (A) A diagram showing two rice ESV1 mutant alleles. Two rice T-DNA insertion lines (osESV1-1, osESV1-2) were isolated from the rice stock center. Kitaake (Kit) and Dongjin (DJ) in parenthesis are their respective wild type cultivars. Back bars: exons, Lines: introns, numbers in black bars: exon numbers, inverted triangle: T-DNA insertion locus. (B) Chalky grains of rice ESV1 mutants. Grain images were taken on a white light box. Black patches in the grains indicate chalky areas. (C) Reduced levels of starch but not soluble sugars in rice ESV1 mutant leaves. Leaves of 6-week-old rice plants grown under a 14/10 light/dark cycle in a greenhouse were sampled at each time point and the levels of glucose, fructose, sucrose, and starch were determined. Asterisks indicate significant differences from the respective wild type (*p < 0.05; Student's t-test) (Supplementary Table 1). Error bars=SEM (n = 3 biological replicates). (D) SEM images showing loosely packed starch granules of fractured ESV1 mutant rice grains. Red scale bars appear in the lower left corners (2 μm).
In a more detailed analysis, however, we found ESV1 promotes starch granule formation also in rice (Supplementary Table 1). First, in a quantitative carbohydrate analysis, we found the two rice ESV1 mutants accumulate lower levels of leaf starch than wild type during the day but deplete starch more slowly at night, resulting in similarly low starch levels at dawn (Figure 6C). Unlike with starch, however, rice ESV1 mutants accumulate similar levels of leaf glucose, fructose, and sucrose during the day and deplete them similarly at night, indicating ESV1 is necessary in leaves specifically for high accumulation of starch during the day. Second, we noticed ESV1 mutant grains are chalky rather than translucent. Since chalky grains are often caused by altered starch granule structure, we examined these structures in high magnification scanning electron microscope images of fractured rice grains. We found that wild type grains have large, tightly packed starch granules with narrow crevices between them, whereas ESV1 mutant grains have small, loosely packed starch granules with conspicuous crevices between them (Figure 6D). It is likely the crevices between the granules that lead to the chalky appearance of ESV1 mutant grains as air cavities between the starch granules reflect and refract more light in random directions. Together, these results indicate ESV1 is necessary in rice for the accumulation of starch in leaves and to form tightly packed starch granules in rice grains.
ESV1 Promotes Root Gravitropism via Its Role in Starch Granule Formation in the Root Columella of Arabidopsis and Rice
Since Arabidopsis ESV1 mutants lack starch granules also in root columella cells, we asked whether rice ESV1 mutants show this same phenotype. After cross-sectioning and staining root tips with Lugol's iodine, we found no starch granules in the root columella cells of rice ESV1 mutants, whereas the roots of wild type seedlings are filled with starch granules and so are darkly stained (Figure 7A). Starch granules in root columella cells act as statoliths for root gravitropism. To determine whether rice ESV1 mutant roots show such gravitropism, we reoriented rice seedlings by 90 degrees and measured the bending of their root tips after 24 h of growth in the dark. While the root tips of wild type seedlings bend by more than 50 degrees by 24 h after the reorientation, the roots of rice ESV1 mutants bend downward by only about 30 degrees (Figures 7B,E). As with rice ESV1 mutants, Arabidopsis ESV1 mutants lack starch granules in their root columella cells (Figure 7C) and bend more slowly than wild type roots after a 90-degree reorientation (Figures 7D,E). The rice ESV1 mutant also show reduced shoot gravitropism (Supplementary Figure 8). Together, these results indicate ESV1 promotes gravitropism via its role in the formation of starch granules both in Arabidopsis and rice.
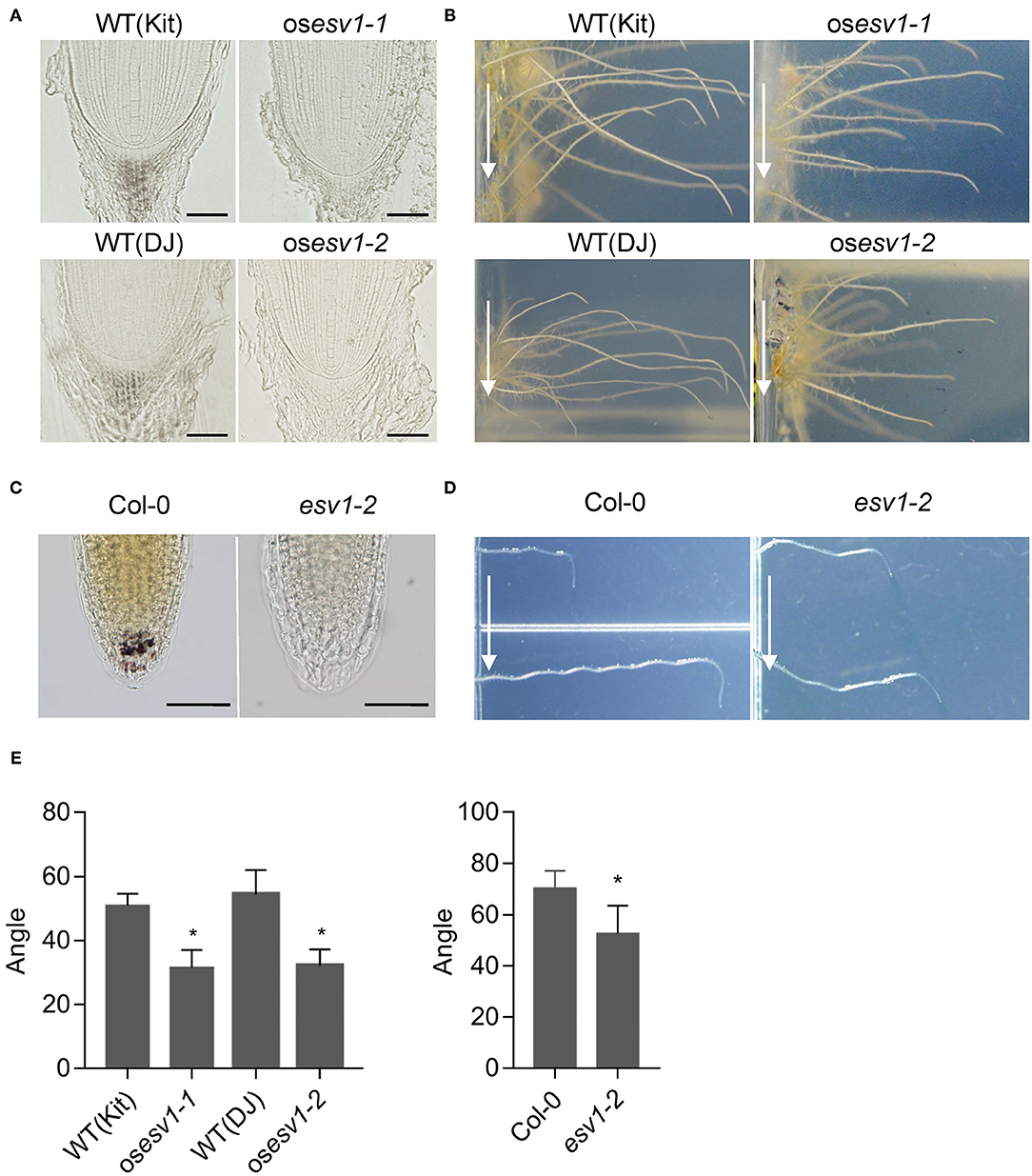
Figure 7. ESV1 promotes root gravitropism by forming root columella starch granules both in Arabidopsis and rice. (A) Absence of root columella starch granules in rice ESV1 mutants. Root tips were embedded in resin and starch granules were visualized by staining with Lugol's iodine. DJ (Dongjin) and Kit (Kitaake) in parenthesis indicate the respective rice cultivars. Scale bar = 50 μm. (B) Reduced root gravitropic responses of rice ESV1 mutants. Rice seedlings grown on MS-agar for 5 days in the dark were rotated by 90 degrees and grown one more day before taking images to measure bending angles. Arrows indicate the direction of gravity after the rotation. The degree of root gravitropism is presented as the degree of root bending. (C) Absence of root columella starch granules in Arabidopsis ESV1 mutant. Root columella starch granules were visualized by staining 3-day-old dark-grown seedlings with Lugol's iodine. Scale bar = 50 μm. (D) Reduced root gravitropic response in Arabidopsis ESV1 mutants. Arabidopsis seedlings grown on MS-agar for 5 days in the dark were rotated by 90 degrees and grown 12 more hours before taking images to measure the angle of bending. Arrows indicate the direction of gravity after the rotation. The degree of root gravitropism is presented as the degree of root bending. (E) Quantitation of root gravitropic responses in rice and Arabidopsis. Seedlings were grown as in (B,D). Asterisks indicate significant differences from wild type (*p < 0.05; Student's t-test). Error bars=SD (n = 10 for Rice, n = 100 for Arabidopsis).
Endodermal Plastids Do Not Sediment in the ESV1 Mutant
We next asked whether the reduced gravitropism of the ESV1 mutant is due to an inability of its plastids to sediment in the direction of gravity. Amyloplast sedimentation can be tracked with Lugol's iodine staining, but because endodermal plastids in the ESV1 mutant are not stained by Lugol's iodine, we generated transgenic lines expressing mCitrine fused with a chloroplast transit peptide under the control of the SCR promoter. This allowed us to track endodermal plastid sedimentation with a fluorescence microscope (Saito et al., 2005). We grew groups of seedlings on vertical plates in the dark for 3 days and determined the relative positions of the endodermal plastids within their cells either before or after inverting the growth plates. In wild type and SCRp:ESV1/ESV1-2 seedlings, we found most of the endodermal plastids located at the bottom of the endodermal cells before the inversion, whereas the endodermal plastids in ESV1-2 mutant seedlings were dispersed throughout the cells (Figures 8A,B). After the inversion, the endodermal plastids in the wild type seedlings began to move toward the new bottoms of the cells. They were dispersed in the cells at 5 min post-inversion and had moved to the new bottoms by 120 min post-inversion (Figures 8A,B). We observed a similar sedimentation pattern in SCRp:ESV1/ESV1-2 seedlings. In the ESV1-2 mutant seedlings, however, we found the endodermal plastids were dispersed widely regardless of gravity and their inversion status. These results indicate that ESV1 mutant seedlings display reduced hypocotyl negative gravitropism due to an inability of their endodermal plastids to sediment in the direction of gravity.
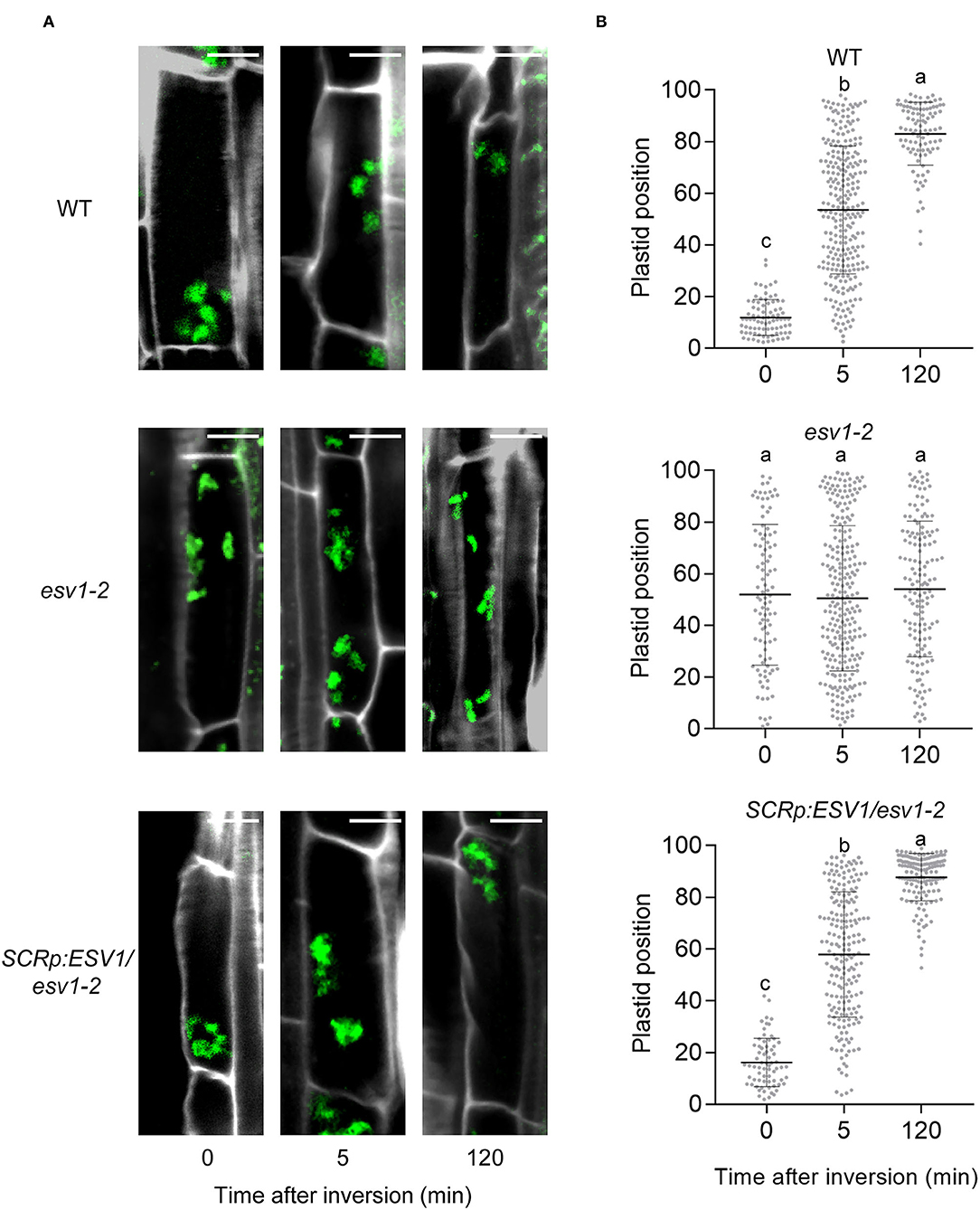
Figure 8. Endodermal plastids of the ESV1 mutant do not sediment toward the direction of gravity. (A) Confocal images showing no sedimentation of endodermal plastids in the ESV1 mutant. Three day-old dark grown seedlings expressing mCitrine fused with rubisco small subunit transit peptide were inverted in the dark. At the indicated times after inversion, the seedlings were fixed with paraformaldehyde and confocal images were taken after staining their cell walls with Calcofluor white. Cell images were arranged in a typical apical-basal orientation despite the inversion of the seedlings. Green spots indicate endodermal plastids and white lines indicate cell walls. mCitrine fused with a transit peptide was expressed in wild type (WT), the ESV1 mutant (ESV1), and the rescue line (SCRp:ESV1/ESV1) background. Scale bar = 10 μm. (B) Quantitation showing no sedimentation of endodermal plastids in the ESV1 mutant. The relative position of each endodermal plastid was calculated by assigning the initial bottom of a cell to 0 and the top of the cell to 100. Each dot indicates one endodermal plastid (n > 70). Bars indicate the average and standard deviation. Letters indicate statistical significance determined by an ANOVA with Tukey's HSD post-hoc test for multiple comparisons (p < 0.01).
Discussion
ESV1 Promotes Gravitropic Responses by Forming Starch Granules in Land Plants
Endodermis-specific expression of PIF1 restores amyloplasts and hypocotyl negative gravitropism in pifQ mutant seedlings (SCRp:PIF1/pifQ) (Kim et al., 2011). In this study, we mutagenized the SCRp:PIF1/pifQ line and isolated 30 reduced gravitropic (rgv) mutants (Figure 1). Among them, we characterized seven mutants lacking starch granules in both the hypocotyl endodermis and root columella. We determined that these seven starchless mutants belong to three complementation groups (rgv1, rgv2, rgv3). Of these, rgv2 and rgv3 are allelic to the pgm and isa1 mutants, respectively (Supplementary Figure 4C). Using whole genome sequencing, we identified in three rgv1 alleles point mutations in AT1G42430, which is also known as EARLY STARVATION 1 (ESV1) (Figure 2A). ESV1 encodes a plastid-localized tryptophan-rich protein that has been suggested to prevent hasty depletion of starch overnight either by altering starch matrix organization or by controlling glucan phosphorylation via dikinase (Feike et al., 2016; Malinova et al., 2018). We show ESV1 acts cell-autonomously in the endodermis to promote hypocotyl negative gravitropism through its role in starch granule formation (Figures 3A,B). ESV1 homologs are found in starch-accumulating green algae and land plants. We found that ESV1 orthologs from the liverwort Marchantia polymorpha and the monocot rice plant Oryza sativa can rescue endodermal starch granules and hypocotyl negative gravitropism in the Arabidopsis ESV1 mutant (Figure 5). This suggests the function of ESV1 is conserved. Furthermore, the grains of rice ESV1 mutants are filled with loosely packed starch granules (Figure 6D), and the root columella produce no starch granules at all (Figure 7A). This loss of starch granules in the root columella reduces root gravitropism both in Arabidopsis and rice (Figures 7B,D). Together, our results indicate the function of ESV1 in promoting gravitropic responses via its role in starch granule formation has been conserved in plants.
It should be noted, however, that not all green plant lineages that possess ESV1 homologs use starch-filled amyloplasts as gravity sensors. Genome sequence information indicates that ESV1 homologs are present not only in starch-producing land plants including liverwort, rice, and Arabidopsis, but also in starch-producing unicellular green algae including Chlamydomonas and starch-producing multicellular green algae including Chara. Land plants sense gravity using starch-filled amyloplasts as gravity sensors and bend toward or against the direction of gravity, displaying either positive or negative gravitropism (Zhang et al., 2019b). Unicellular Chlamydomonas also senses gravity and swims against the direction of gravity, displaying negative gravitaxis (Bean, 1977; Kam et al., 1999). Unlike land plants, however, Chlamydomonas possesses a single cup-shaped chloroplast and does not develop starch-filled amyloplasts. Instead, starch granules within the chloroplast encapsulate a matrix containing Rubisco and form a sub-organellar compartment called a pyrenoid (Ramazanov et al., 1994; Giordano et al., 2005). The exact mechanism by which Chlamydomonas senses gravity remains poorly understood, but it has been suggested that it is the whole body that senses gravity rather than just the starch-covered pyrenoid (Roberts, 2006). Similarly, the multicellular green algae Chara synthesizes starch granules but senses gravity using barium sulfate vesicles for its rhizoid gravitropism rather than starch-filled amyloplasts (Limbach et al., 2005). In contrast to green algae, the protonemata or caulonemata of bryophytes (early land plants), which include Ceratodon, Funaria, and Physcomitrella, possess sedimentable starch-filled amyloplasts in their growing tips and display negative gravitropism (Jenkins et al., 1986; Walker and Sack, 1990; Schwuchow et al., 1995). This presence of starch granule-dependent gravity sensing in bryophytes but not in green algae suggests the promotion of gravitropic responses by ESV1 via its role in starch granule formation is likely a derived feature in land plants.
ESV1 Is Insufficient for Ectopic Starch Granule Production
Although ESV1 is necessary for the formation of starch granules in the endodermis and root columella, ESV1 is neither necessary nor sufficient to induce starch granule formation in other tissues and organs. Both Arabidopsis and rice ESV1 mutants still accumulate starch granules in leaves during the day, albeit at reduced levels (Figure 6C) (Feike et al., 2016). Redundant activity of homologous genes is unlikely responsible for the formation of these residual starch granules in leaves because none of the homologous genes complement the ESV1 mutant. This suggests these homologous proteins do not have redundant functions with ESV1 and further confirms ESV1 is inessential in the accumulation of starch in Arabidopsis leaves. Our data also show that ectopic expression of ESV1 under the control of the 35S promoter rescues starch granules in the hypocotyl endodermis and root columella of ESV1 mutant seedlings, but it does not induce the formation of starch granules in other cells (Figure 3C). Even in the endodermis, ectopic ESV1 rescues starch granules only in the upper hypocotyl endodermis where starch granules are usually present in wild type. Similarly, endodermis-specific expression of ESV1 under the SCR promoter also only rescues starch granules in the upper hypocotyl endodermis but not the endodermis of other regions (Figure 3C).
It is not clear why starch granules form only in the upper hypocotyl endodermis and root columella of dark-grown Arabidopsis seedlings, but there are a few possibilities. First, since starch granules are formed from nutrients stored in seeds, dark-grown seedlings may have insufficient carbon to produce starch granules in other tissues. We noticed, however, that while wild type seedlings still form starch granules only in the upper endodermis and root columella in media supplemented with sucrose, ESV1 mutant seedlings still lack starch granules (Supplementary Figure 9). This suggests the tissue-specific formation of starch granules cannot be attributed to insufficient carbon. Second, the formation of starch granules in the hypocotyl endodermis and root columella requires plastidic PGM and plastidic AGPase but not plastidic PGI, indicating that glucose 6-phosphate must be transported into plastids to form starch granules in these tissues (Niewiadomski et al., 2005; Kunz et al., 2010; Streb and Zeeman, 2012). Thus, the tissue-specific formation of starch granules could be caused by tissue-specific expression of glucose 6-phosphate/phosphate translocator (GPT). Previous studies found that two Arabidopsis GPT genes (GPT1, GPT2) are expressed in all organs including cotyledons, hypocotyls, and roots (Niewiadomski et al., 2005), and GPT1 is expressed in various parts of roots including the epidermis, vascular tissue, the columella and surrounding cells (Birnbaum et al., 2003; Brady et al., 2007; Tsai et al., 2009). However, since starch granules form only in root columella and not the surrounding cells, it is unlikely that the tissue-specific supply of glucose 6-phosphate is the sole cause of tissue-specific starch granule formation. Third, since starch biosynthesis requires the coordinated action of many enzymes including PGM, AGPase, and SS, tissue-specific starch granule formation may require the entire set of starch biosynthetic genes rather than the tissue-specific expression of any single biosynthetic gene. Indeed, a previous study found that the entire set of starch biosynthetic genes including PGM, AGPase, and SS are expressed specifically in the root tip but not in other parts of root (Tsai et al., 2009). Thus, although the precise pattern of expression of the starch biosynthetic genes in hypocotyls is unknown, it is tempting to speculate that starch granules form only in the upper hypocotyl endodermis because only the upper hypocotyl endodermis expresses the entire set of starch biosynthetic genes, including the starch granule-stabilizing ESV1.
The Severity of ESV1 Mutant Phenotypes Is Tissue-Dependent
The starch accumulation patterns in Arabidopsis and rice ESV1 mutants show similarities and differences. Arabidopsis ESV1 mutants lack starch granules in the seedling sink tissues: the hypocotyl endodermis and the root columella (Figure 2B). Rice ESV1 mutants lack starch granules in one sink tissue, the root columella (Figure 7A), but retain loosely packed starch granules in another sink tissue, the grain endosperm (Figure 6D). Both Arabidopsis and rice ESV1 mutants accumulate starch, in a source tissue, the leaf mesophyll, during the day (Figure 6C, Supplementary Figure 5). These differences in starch accumulation patterns suggest ESV1 promotes the formation of starch granules differently depending on the plant tissue and species (Supplementary Figure 5, Supplementary Table 1).
It is unclear why starch granules in some tissues are more severely affected by ESV1 mutation than those in other tissues. It is possible that ESV1 regulates a tissue-specific metabolic process. Starch is synthesized from glucose 6-phosphate, which is transported into plastids from the cytosol through GPT, via the serial actions of PGM, AGPase, SS, and other synthetic enzymes in Arabidopsis sink tissues (Kofler et al., 2000; Fischer and Weber, 2002; Kunz et al., 2010). In photosynthetic source tissues, however, starch is synthesized from fructose 6-phosphate in the Calvin-Benson cycle via the serial action of plastidic PGI, PGM, AGPase, SS, and other synthetic enzymes (Streb and Zeeman, 2012). Similarly, starch is synthesized from fructose 6-phosphate in rice leaf mesophyll. In cereal endosperms, however, starch is synthesized from ADP-glucose molecules produced in the cytosol and then transported into plastids through BRITTLE 1 (BT1) (Cao et al., 1995; Shannon et al., 1998; Beckles et al., 2001; Jeon et al., 2010; Tuncel and Okita, 2013). Thus, the tissues lacking starch granules in the ESV1 mutants coincide with tissues using transported glucose 6-phosphate for starch biosynthesis, raising the possibility that ESV1's role in starch granule formation is associated with the transport of glucose 6-phosphate. However, this parallel does not necessarily mean that the role of ESV1 is associated with the transport of glucose 6-phosphate into plastids. We previously showed starch synthesis in rice pollen relies mainly on glucose 6-phosphate imported into amyloplasts and the reduction of starch synthesis in rice pollen causes male sterility due to reduced pollen viability (Lee et al., 2016). Rice ESV1 mutants, however, grow normally and bear seeds, indicating that their pollen grains store sufficient starch.
Alternatively, ESV1 may regulate common metabolic processes found in all tissues, but the severity of the ESV1 mutant phenotype in a given tissue depends on the ratio of starch synthesis to starch degradation in that tissue. The formation of starch granules is likely to be determined by the balance between starch synthesis and degradation rather than the synthesis alone, as supported by the expression of both synthetic and degrading genes in starch-accumulating tissues (Tsai et al., 2009; Van Harsselaar et al., 2017) or by a high co-expression coefficient for synthetic and degrading genes in deposited transcriptome data (Obayashi et al., 2017). To test this hypothesis, we estimated starch synthetic flow by analyzing the ratio of the expression of APS1 to GWD/SEX1 mRNAs using reported transcriptome data. Since APS1 encodes a subunit of an AGPase that is rate-limiting for starch synthesis (Lin et al., 1988; Crevillen et al., 2005) and GWD encodes an α-glucan water dikinase required for the first crucial step of starch degradation (Yu et al., 2001; Ritte et al., 2006), the ratio of APS1 to GWD acts as a rough estimate of the balance of starch synthetic flow in different tissues. We found in an analysis of previously reported transcriptome data that Arabidopsis leaves show higher APS1/GWD ratios than roots (Supplementary Figure 10), suggesting starch synthetic flow is stronger in leaves than roots. Since the Arabidopsis ESV1 mutation reduces starch accumulation more strongly in roots than in leaves, phenotypic severity is inversely correlated with the strength of starch synthetic flow as represented by the APS1/GWD ratio. In rice, grains have a higher APS1/GWD ratio than leaves, which suggests that grains have stronger starch synthetic flow than leaves. Since starch accumulation is more severely reduced in rice leaves than in rice grains, the severity of rice ESV1 mutant phenotype is also inversely correlated with the APS1/GWD ratio. Furthermore, Arabidopsis leaves have a higher APS1/GWD ratio than rice leaves, suggesting Arabidopsis leaves have stronger starch synthetic flow than rice leaves. Consistent with this, Arabidopsis partitions photo assimilates mainly into transient starch during the day, while rice partitions them mainly into soluble sugar (Lee et al., 2008, 2014). Together, our analysis suggests the severity of the ESV1 mutation on starch accumulation is inversely associated with tissue-specific biases toward starch synthesis over degradation.
Methods
Plant Materials and Growth
Arabidopsis plants (Arabidopsis thaliana) were grown in a growth room with a 16-h light/8-h dark cycle at 22–24°C for general growth and seed harvesting. T-DNA insertion mutants for Arabidopsis ESV1 and LESV were obtained from the Arabidopsis stock center and homozygous mutants were selected by genotyping. The corresponding stock numbers are as follows: ESV1-2 (GABI_031C11), lesv-2 (Salk_006705), lesv-3 (Salk_006697), aps1 (Salk_040155), isa1 (Salk_117080), isa2 (Salk_029442). The phot1 phot2 mutant was obtained from Eiji Gotoh at Kyushu University and the pgm-1 mutant from Samuel Zeeman at ETH Zurich.
To generate transgenic plants expressing ESV1, the ESV1 coding region was cloned into the pCAMBIA1300 or its derived vectors containing either GFP or tissue-specific promoters (ML1 promoter or SCR promoter) and introduced into Arabidopsis (35Sp:ESV1-OX/ESV1-2, 35Sp:ESV1-GFP, ML1p:ESV1/ESV1-2, SCRp:ESV1/ESV1-2). At least two independent transgenic lines were generated. Synthesized ESV1 genes from Marchantia polymorpha (Mapoly0189s0007) and Oryza sativa (LOC_Os03g04100) were also used to generate transgenic lines (SCRp:MpESV1/ESV1-2, SCRp:OsESV1/ESV1-2). To visualize endodermal plastids, a DNA fragment corresponding to the Rubisco small subunit (RBCS1A) transit peptide was cloned into a binary vector containing the mCitrine gene under the control of the SCR promoter as previously reported (Saito et al., 2005). All Arabidopsis plants used in this study were of the Columbia ecotype background.
Rice plants (Oryza sativa) were grown in a greenhouse with a 14-h light/10-h dark cycle (30/20°C). T-DNA insertion mutants of rice ESV1 were identified from the rice stock center (Jeon et al., 2000; Jeong et al., 2006). Homozygous mutants were selected by PCR genotyping with gene-specific and T-DNA-specific primers. The corresponding stock numbers are as follows: osESV1-1 (PFG_K-03747) and osESV1-2 (PFG_1A-07022). Their parental lines are Kitaake and Dongjin, respectively. The primers used for cloning are described in Supplementary Table 3.
Identification of Starchless rgv Loci
A total of 10,000 seeds from an Arabidopsis transgenic line expressing PIF1 under the control of the endodermis-specific SCR promoter (SCRp:PIF1/pifQ) were mutagenized with EMS. M2 seedlings that did not grow upward in the dark were selected and mutants displaying stable phenotypes over generations were selected as reduced gravitropic (rgv) mutants. For complementation grouping, seven starchless rgv mutants were each crossed with one another and the endodermal starch granules of the F1 seedlings were examined with Lugol's iodine. To determine if the starchless mutants are allelic to known starchless biosynthetic mutants, rgv1-1, rgv2-1, and rgv3-1, representing three complementation groups, were crossed with pgm, aps1, isa1, and isa2 mutants and the endodermal starch granules of the resulting F1 seedlings were examined with Lugol's iodine. Simultaneously, the genomic regions of PGM, APS1, and ISA1 were amplified and sequenced from the seven starchless mutants. To identify the mutated loci in the three rgv1 mutant alleles (rgv1-1, rgv1-2, rgv1-3), each mutant was backcrossed to the parental line three times and then used for whole genome sequencing analysis. The whole-genome sequencing was performed by Illumina NextSeq and the results were mapped to the Arabidopsis reference genome (Arabidopsis.org; TAIR10). High quality homozygous single nucleotide polymorphisms (SNPs) caused by G to A or C to T substitutions were identified and, by comparing the SNPs in the three rgv1 alleles with the parental line, SNPs were identified in AT1G42430 in all three rgv1 alleles but not in the parental line. To further support the hypothesis that rgv1 mutants are caused by mutations in this gene, a T-DNA insertion line of AT1G42430 was obtained from the Arabidopsis stock center. After confirming that the rgv1 mutants were caused by mutations in AT1G42430, the rgv1 mutant was renamed to the ESV1 mutant.
Lugol's Iodine Staining of Starch Granules
Lugol's iodine staining was used to visualize starch granules in seedlings. Arabidopsis seedlings were fixed in FAA solution (5% Formaldehyde, 5% Acetic acid, 45% EtOH) for 24 h at 4°C. After fixation, the seedlings were washed with 50% ethanol once, and stained with I2-KI solution (2% w/v Iodine, 5% w/v Potassium iodide, 20% v/v Trichloroacetic acid) for 1 min. Seedlings were de-stained with a clearing solution (Trichloroacetic acid: phenol: lactate = 2: 1: 1) for 1 min and mounted on slide glass with fresh clearing solution for microscopy. For rice, samples were fixed in 0.05 M sodium-phosphate buffer (pH 7.4) containing 2% paraformaldehyde for 4 h at 4°C. After fixation, the tissues were washed overnight in phosphate-buffered saline (PBS, pH 7.4) containing 6.8% sucrose, dehydrated in an ethanol gradient series, and embedded in Technovit 8100 resin (Kulzer, Frankfurt, Germany). Tissues were then sliced into 10 μm sections with a rotary microtome (RM2165; Leica, Wetzlar, Germany) and stained with 10% diluted I2-KI solution (Sigma-Aldrich, St. Louis, MO, USA) for 1 min.
Seedling Gravitropism
Hypocotyl negative gravitropism was determined by growing seedlings for 3 days on horizontal plates in different light conditions and counting the number of standing seedlings. For the assay, surface-sterilized seeds were plated on half Murashige and Skoog (MS) media (1/2 MS, 0.8% phytoagar, and 0.05% MES, pH 5.7) and stratified for 3 days in the dark at 4°C. After the stratification, the seeds were incubated under white light (50 μmol/m2s) for 6 h at 22°C to induce germination. Germination-induced seeds were then incubated for 3 days in the dark or in red light (20 μmol/m2s) at 22°C. Seedlings were counted as standing if their cotyledons did not touch the agar surface. Total 40 of seedlings were used for each experiment (n = 3, biological replicates). Phototropic seedlings were similarly determined after growing them on horizontal plates under blue light (1 μmol/m2s) for 4 days. To determine Arabidopsis root gravitropic responses, seedlings were grown for 5 days on vertical plates under white light (50 μmol/m2s). Then, they were grown for an additional 12 h after rotating the plates by 90 degrees. The angles formed by the bent root were measured and supplementary angles were used to indicate the degree of root gravitropism. To determine rice root gravitropic responses, surface-sterilized seeds were germinated on half MS media (1/2 MS, 3% phytoagar, pH 5.7) in Magenta vessels (Sigma-Aldrich, St. Louis, MO, USA) for 7 days in the dark at 25°C until the shoots and roots established a vertical extension of seedling growth. These were then further incubated for 1 day after rotating the vessels by 90 degrees.
Sedimentation of Endodermal Plastids
Seedlings were grown on vertical plates in the dark for 3 days before being inverted. For the ESV1 mutant, seedlings were rearranged vertically at day 2 under a safety green light and allowed to grow 1 more day in the dark before inversion. At the indicated times after inversion, the seedlings were decapitated with a blade, transferred to 4% paraformaldehyde + 0.1% triton X-100 solution, fixed under vacuum for 1 h, and washed twice with PBS buffer. The seedlings were then transferred to 0.1% Calcofluor white solution for 1 h to stain the cell walls (Fluorescent Brightener 28, MP Biomedicals). After two more washes with PBS buffer, the seedlings were mounted on a slide glass with anti-fade mounting solution (VECTASHIELD Antifade Mounting Medium H-1000). Fluorescence images were taken with a confocal microscope (LSM780, ZEISS). The relative position of each plastid was calculated by assigning the initial bottom of a cell to 0 and the top of the cell to 100.
Protein and mRNA Expression Analysis
After the induction of seed germination, seedlings were grown on half MS media with 1% sucrose either in the dark or in red light (20 μmol/m2s) for 3 days and whole seedlings were harvested for RNA or protein expression analysis. For mRNA expression analysis, total RNAs were extracted using the Spectrum plant total RNA kit (Sigma-Aldrich) and converted to cDNA using MMLV-RTase (Promega). Relative transcript levels were determined by quantitative PCR (CFX connect, Bio-rad) and normalized to the level of PP2A. All experiments were performed with biological triplicates.
For protein expression analysis, harvested seedlings were ground in liquid nitrogen and further homogenized in a denaturing buffer (100 mM NaH2PO4, pH 8.0, 10 mM Tris-Cl, 8 M Urea, 1 mM phenylmethylsulfonyl fluoride (PMSF), and protease inhibitor cocktail). After removing the debris by centrifugation, the supernatant protein extract was mixed with 5X SDS sample buffer [50 mM Tris-Cl, pH 6.8, 10% glycerol, 2% sodium dodecyl sulfate (SDS), 0.05% bromophenol blue, and 100 mM dithiothreitol (DTT)] and boiled at 100°C for 5 min. Proteins were then detected by immunoblot analysis using an anti-GFP antibody for ESV1-GFP and an anti-α-tubulin antibody for α-tubulin. For the quantitative analysis, the intensity of ESV1-GFP was normalized by α-tubulin (n = 3, biological replicates).
Determination of Soluble Sugars and Starch
Approximately 100 mg of leaves were sampled from 6-week-old rice plants grown under a 14/10 light cycle in a greenhouse. Each sample was harvested at zeitgeber (ZT) 0, 8, 16, 18, 20, and 22. Glucose, fructose, sucrose, and starch were measured in the soluble and insoluble fractions of ethanol-water extracts using enzymatic methods (Lee et al., 2005). The measured metabolite contents were normalized to fresh weights (n = 3, biological replicates).
Scanning Electron Microscopy
Scanning Electron Microscope (SEM) analysis were carried out with Carl Zeiss MERLIN™ FE-SEM operated at low accelerating voltage of 1 kV. The fractured surface morphologies of wild type and mutant rice grains were observed without any coating materials.
Accession Numbers
Arabidopsis Thaliana
APS1: AT5G48300, GWD: AT1G10760, IAA19: AT3G15540, ISA1: AT2G39930, ISA2: AT1G03310, ML1: AT4G21750, PGM: AT5G51820, PHOT1: AT3G45780, PHOT2: AT5G58140, PHYA: AT1G09570, PHYB: AT2G18790, PIF1: AT2G20180, PIF3: AT1G09530, PIF4: AT2G43010, PIF5: AT3G59060, PIL1: AT2G46970, PP2A: AT1G13320, RBCS1A: AT1G67090, ESV1: AT1G42430, LESV: AT3G55760, and SCR: AT3G54220.
Oryza Sativa
Os-ESV1: LOC_Os03g04100, APS1: LOC_Os09g12660, GWD: LOC_Os06g30310, APS2: LOC_Os08g25734, APL2: LOC_Os01g44220, PGM: LOC_Os10g11140, APL4: LOC_Os07g13980.
Marchantia Polymorpha
Mp-ESV1: Mapoly0189s0007.
Data Availability Statement
The raw data supporting the conclusions of this article will be made available by the authors, without undue reservation.
Author Contributions
KS, D-WL, JeK, J-SJ, and GC designed the experiments. KS, D-WL, JeK, JaK, HG, and KK performed the experiments. KS, D-WL, J-SJ, and GC wrote the paper. All authors contributed to the article and approved the submitted version.
Funding
This work was supported by the National Research Foundation of Korea (NRF-2018R1A3B1052617 to GC) and the Mid-Career Researcher program, National Research Foundation of Korea (Project No. 2020R1A2C2012976 to J-SJ).
Conflict of Interest
The authors declare that the research was conducted in the absence of any commercial or financial relationships that could be construed as a potential conflict of interest.
Publisher's Note
All claims expressed in this article are solely those of the authors and do not necessarily represent those of their affiliated organizations, or those of the publisher, the editors and the reviewers. Any product that may be evaluated in this article, or claim that may be made by its manufacturer, is not guaranteed or endorsed by the publisher.
Supplementary Material
The Supplementary Material for this article can be found online at: https://www.frontiersin.org/articles/10.3389/fpls.2021.628948/full#supplementary-material
References
Baunsgaard, L., Lutken, H., Mikkelsen, R., Glaring, M. A., Pham, T. T., and Blennow, A. (2005). A novel isoform of glucan, water dikinase phosphorylates pre-phosphorylated alpha-glucans and is involved in starch degradation in Arabidopsis. Plant J. 41, 595–605. doi: 10.1111/j.1365-313X.2004.02322.x
Bean (1977). Geotactiv behavior of Chlamydomonas. J. Protozool. 24, 394–401. doi: 10.1111/j.1550-7408.1977.tb04759.x
Beckles, D. M., Smith, A. M., and Ap Rees, T. (2001). A cytosolic ADP-glucose pyrophosphorylase is a feature of graminaceous endosperms, but not of other starch-storing organs. Plant Physiol. 125, 818–827. doi: 10.1104/pp.125.2.818
Birnbaum, K., Shasha, D. E., Wang, J. Y., Jung, J. W., Lambert, G. M., Galbraith, D. W., et al. (2003). A gene expression map of the arabidopsis root. Science 302:1956. doi: 10.1126/science.1090022
Brady, S. M., Orlando, D. A., Lee, J.-Y., Wang, J. Y., Koch, J., Dinneny, J. R., et al. (2007). A high-resolution root spatiotemporal map reveals dominant expression patterns. Science 318:801. doi: 10.1126/science.1146265
Cao, H., Sullivan, T. D., Boyer, C. D., and Shannon, J. C. (1995). Btl, a structural gene for the major 39-44 kDa amyloplast membrane polypeptides. Physiol. Plant. 95, 176–186. doi: 10.1111/j.1399-3054.1995.tb00825.x
Caspar, T., Huber, S. C., and Somerville, C. (1985). Alterations in growth, photosynthesis, and respiration in a starchless mutant of Arabidopsis thaliana (L.) deficient in chloroplast phosphoglucomutase activity. Plant Physiol. 79:11. doi: 10.1104/pp.79.1.11
Crevillen, P., Ventriglia, T., Pinto, F., Orea, A., Merida, A., and Romero, J. M. (2005). Differential pattern of expression and sugar regulation of Arabidopsis thaliana ADP-glucose pyrophosphorylase-encoding genes. J. Biol. Chem. 280, 8143–8149. doi: 10.1074/jbc.M411713200
Di Laurenzio, L., Wysocka-Diller, J., Malamy, J. E., Pysh, L., Helariutta, Y., Freshour, G., et al. (1996). The SCARECROW gene regulates an asymmetric cell division that is essential for generating the radial organization of the arabidopsis root. Cell 86, 423–433. doi: 10.1016/S0092-8674(00)80115-4
Feike, D., Seung, D., Graf, A., Bischof, S., Ellick, T., Coiro, M., et al. (2016). The starch granule-associated protein EARLY STARVATION1 is required for the control of starch degradation in Arabidopsis thaliana leaves. Plant Cell 28, 1472–1489. doi: 10.1105/tpc.16.00011
Fischer, K., and Weber, A. (2002). Transport of carbon in non-green plastids. Trends Plant Sci. 7, 345–351. doi: 10.1016/S1360-1385(02)02291-4
Friml, J., Wisniewska, J., Benkova, E., Mendgen, K., and Palme, K. (2002). Lateral relocation of auxin efflux regulator PIN3 mediates tropism in Arabidopsis. Nature 415, 806–809. doi: 10.1038/415806a
Giordano, M., Beardall, J., and Raven, J. A. (2005). CO2 concentrating mechanisms in algae: mechanisms, environmental modulation, and evolution. Annu. Rev. Plant Biol. 56, 99–131. doi: 10.1146/annurev.arplant.56.032604.144052
Harrison, B. R., and Masson, P. H. (2008). ARL2, ARG1 and PIN3 define a gravity signal transduction pathway in root statocytes. Plant J. 53, 380–392. doi: 10.1111/j.1365-313X.2007.03351.x
Hashiguchi, Y., Tasaka, M., and Morita, M. T. (2013). Mechanism of higher plant gravity sensing. Am. J. Bot. 100, 91–100. doi: 10.3732/ajb.1200315
Hashiguchi, Y., Yano, D., Nagafusa, K., Kato, T., Saito, C., Uemura, T., et al. (2014). A unique HEAT repeat-containing protein SHOOT GRAVITROPISM6 is involved in vacuolar membrane dynamics in gravity-sensing cells of Arabidopsis inflorescence stem. Plant Cell Physiol. 55, 811–822. doi: 10.1093/pcp/pcu020
Heidstra, R., Welch, D., and Scheres, B. (2004). Mosaic analyses using marked activation and deletion clones dissect Arabidopsis SCARECROW action in asymmetric cell division. Genes Dev. 18, 1964–1969. doi: 10.1101/gad.305504
Hejazi, M., Fettke, J., Haebel, S., Edner, C., Paris, O., Frohberg, C., et al. (2008). Glucan, water dikinase phosphorylates crystalline maltodextrins and thereby initiates solubilization. Plant J. 55, 323–334. doi: 10.1111/j.1365-313X.2008.03513.x
Hejazi, M., Fettke, J., Paris, O., and Steup, M. (2009). The two plastidial starch-related dikinases sequentially phosphorylate glucosyl residues at the surface of both the A- and B-type allomorphs of crystallized maltodextrins but the mode of action differs. Plant Physiol. 150, 962–976. doi: 10.1104/pp.109.138750
Jenkins, G. I., Courtice, G. R., and Cove, D. J. (1986). Gravitropic responses of wild-type and mutant strains of the moss Physcomitrella patens. Plant Cell Environ. 9, 637–644. doi: 10.1111/j.1365-3040.1986.tb01621.x
Jeon, J.-S., Lee, S., Jung, K.-H., Jun, S.-H., Jeong, D.-H., Lee, J., et al. (2000). T-DNA insertional mutagenesis for functional genomics in rice. Plant J. 22, 561–570. doi: 10.1046/j.1365-313x.2000.00767.x
Jeon, J. S., Ryoo, N., Hahn, T. R., Walia, H., and Nakamura, Y. (2010). Starch biosynthesis in cereal endosperm. Plant Physiol. Biochem. 48, 383–392. doi: 10.1016/j.plaphy.2010.03.006
Jeong, D. H., An, S., Park, S., Kang, H. G., Park, G. G., Kim, S. R., et al. (2006). Generation of a flanking sequence-tag database for activation-tagging lines in japonica rice. Plant J. 45, 123–132. doi: 10.1111/j.1365-313X.2005.02610.x
José Muñoz, F., Teresa Morán Zorzano, M., Alonso-Casajús, N., Baroja-Fernández, E., Etxeberria, E., and Pozueta-Romero, J. (2006). New enzymes, new pathways and an alternative view on starch biosynthesis in both photosynthetic and heterotrophic tissues of plants. Biocatal. Biotransform. 24, 63–76. doi: 10.1080/10242420500518839
Kam, V., Moseyko, N., Nemson, J., and Feldman, L. J. (1999). Gravitaxis in Chlamydomonas reinhardtii: characterization using video microscopy and computer analysis. Int. J. Plant Sci. 160, 1093–1098. doi: 10.1086/314205
Kato, T., Morita, M. T., Fukaki, H., Yamauchi, Y., Uehara, M., Niihama, M., et al. (2002). SGR2, a phospholipase-like protein, and ZIG/SGR4, a SNARE, are involved in the shoot gravitropism of Arabidopsis. Plant Cell 14, 33–46. doi: 10.1105/tpc.010215
Kim, K., Shin, J., Lee, S. H., Kweon, H. S., Maloof, J. N., and Choi, G. (2011). Phytochromes inhibit hypocotyl negative gravitropism by regulating the development of endodermal amyloplasts through phytochrome-interacting factors. Proc. Natl. Acad. Sci. U.S.A. 108, 1729–1734. doi: 10.1073/pnas.1011066108
Kiss, J. Z. (2000). Mechanisms of the early phases of plant gravitropism. CRC Crit. Rev. Plant Sci. 19, 551–573. doi: 10.1080/07352680091139295
Kleine-Vehn, J., Ding, Z., Jones, A. R., Tasaka, M., Morita, M. T., and Friml, J. (2010). Gravity-induced PIN transcytosis for polarization of auxin fluxes in gravity-sensing root cells. Proc. Natl. Acad. Sci. U.S.A. 107, 22344–22349. doi: 10.1073/pnas.1013145107
Knight, T. A. (1806). On the direction of the radicle and Germen during the vegetation of seeds. Philos. Transact. R. Soc. Lond. 96, 99–108. doi: 10.1098/rstl.1806.0006
Kofler, H., Hausler, R. E., Schulz, B., Groner, F., Flugge, U. I., and Weber, A. (2000). Molecular characterisation of a new mutant allele of the plastid phosphoglucomutase in Arabidopsis, and complementation of the mutant with the wild-type cDNA. Mol. Gen. Genet. 263, 978–986. doi: 10.1007/PL00008698
Kotting, O., Pusch, K., Tiessen, A., Geigenberger, P., Steup, M., and Ritte, G. (2005). Identification of a novel enzyme required for starch metabolism in Arabidopsis leaves. The phosphoglucan, water dikinase. Plant Physiol. 137, 242–252. doi: 10.1104/pp.104.055954
Kunz, H. H., Hausler, R. E., Fettke, J., Herbst, K., Niewiadomski, P., Gierth, M., et al. (2010). The role of plastidial glucose-6-phosphate/phosphate translocators in vegetative tissues of Arabidopsis thaliana mutants impaired in starch biosynthesis. Plant Biol. 12(Suppl. 1), 115–128. doi: 10.1111/j.1438-8677.2010.00349.x
Lee, J. W., Lee, D. S., Bhoo, S. H., Jeon, J. S., Lee, Y. H., and Hahn, T. R. (2005). Transgenic Arabidopsis plants expressing Escherichia coli pyrophosphatase display both altered carbon partitioning in their source leaves and reduced photosynthetic activity. Plant Cell Rep. 24, 374–382. doi: 10.1007/s00299-005-0951-y
Lee, N., and Choi, G. (2017). Phytochrome-interacting factor from Arabidopsis to liverwort. Curr. Opin. Plant Biol. 35, 54–60. doi: 10.1016/j.pbi.2016.11.004
Lee, S. K., Eom, J. S., Hwang, S. K., Shin, D., An, G., Okita, T. W., et al. (2016). Plastidic phosphoglucomutase and ADP-glucose pyrophosphorylase mutants impair starch synthesis in rice pollen grains and cause male sterility. J. Exp. Bot. 67, 5557–5569. doi: 10.1093/jxb/erw324
Lee, S. K., Eom, J. S., Voll, L. M., Prasch, C. M., Park, Y. I., Hahn, T. R., et al. (2014). Analysis of a triose phosphate/phosphate translocator-deficient mutant reveals a limited capacity for starch synthesis in rice leaves. Mol. Plant 7, 1705–1708. doi: 10.1093/mp/ssu082
Lee, S. K., Hwang, S. K., Han, M., Eom, J. S., Kang, H. G., Han, Y., et al. (2007). Identification of the ADP-glucose pyrophosphorylase isoforms essential for starch synthesis in the leaf and seed endosperm of rice (Oryza sativa L.). Plant Mol. Biol. 65, 531–546. doi: 10.1007/s11103-007-9153-z
Lee, S. K., Jeon, J. S., Bornke, F., Voll, L., Cho, J. I., Goh, C. H., et al. (2008). Loss of cytosolic fructose-1,6-bisphosphatase limits photosynthetic sucrose synthesis and causes severe growth retardations in rice (Oryza sativa). Plant Cell Environ. 31, 1851–1863. doi: 10.1111/j.1365-3040.2008.01890.x
Legris, M., Ince, Y. É., and Fankhauser, C. (2019). Molecular mechanisms underlying phytochrome-controlled morphogenesis in plants. Nat. Commun. 10:5219. doi: 10.1038/s41467-019-13045-0
Leivar, P., and Quail, P. H. (2011). PIFs: pivotal components in a cellular signaling hub. Trends Plant Sci. 16, 19–28. doi: 10.1016/j.tplants.2010.08.003
Limbach, C., Hauslage, J., Schäfer, C., and Braun, M. (2005). How to activate a plant gravireceptor. Early mechanisms of gravity sensing studied in characean rhizoids during parabolic flights. Plant Physiol. 139:1030. doi: 10.1104/pp.105.068106
Lin, T.-P., Caspar, T., Somerville, C., and Preiss, J. (1988). Isolation and Characterization of a Starchless Mutant of Arabidopsis thaliana (L.) Heynh Lacking ADPglucose Pyrophosphorylase Activity. Plant Physiol. 86, 1131. doi: 10.1104/pp.86.4.1131
Liu, M. J., Wu, S. H., Wu, J. F., Lin, W. D., Wu, Y. C., Tsai, T. Y., et al. (2013). Translational landscape of photomorphogenic Arabidopsis. Plant Cell 25, 3699–3710. doi: 10.1105/tpc.113.114769
Lorberth, R., Ritte, G., Willmitzer, L., and Kossmann, J. (1998). Inhibition of a starch-granule-bound protein leads to modified starch and repression of cold sweetening. Nat. Biotechnol. 16, 473–477. doi: 10.1038/nbt0598-473
Malinova, I., Mahto, H., Brandt, F., Al-Rawi, S., Qasim, H., Brust, H., et al. (2018). EARLY STARVATION1 specifically affects the phosphorylation action of starch-related dikinases. Plant J. 95, 126–137. doi: 10.1111/tpj.13937
Morita, M. T. (2010). Directional gravity sensing in gravitropism. Annu. Rev. Plant Biol. 61, 705–720. doi: 10.1146/annurev.arplant.043008.092042
Morita, M. T., Kato, T., Nagafusa, K., Saito, C., Ueda, T., Nakano, A., et al. (2002). Involvement of the vacuoles of the endodermis in the early process of shoot gravitropism in Arabidopsis. Plant Cell 14, 47–56. doi: 10.1105/tpc.010216
Morita, M. T., and Tasaka, M. (2004). Gravity sensing and signaling. Curr. Opin. Plant Biol. 7, 712–718. doi: 10.1016/j.pbi.2004.09.001
Nakamura, M., Nishimura, T., and Morita, M. T. (2019). Gravity sensing and signal conversion in plant gravitropism. J. Exp. Bot. 70, 3495–3506. doi: 10.1093/jxb/erz158
Niewiadomski, P., Knappe, S., Geimer, S., Fischer, K., Schulz, B., Unte, U. S., et al. (2005). The Arabidopsis plastidic glucose 6-phosphate/phosphate translocator GPT1 is essential for pollen maturation and embryo sac development. Plant Cell 17, 760–775. doi: 10.1105/tpc.104.029124
Obayashi, T., Aoki, Y., Tadaka, S., Kagaya, Y., and Kinoshita, K. (2017). ATTED-II in 2018: a plant coexpression database based on investigation of the statistical property of the mutual rank index. Plant Cell Physiol. 59:e3. doi: 10.1093/pcp/pcx191
Park, E., Kim, Y., and Choi, G. (2018). Phytochrome B requires PIF degradation and sequestration to induce light responses across a wide range of light conditions. Plant Cell 30, 1277–1292. doi: 10.1105/tpc.17.00913
Pham, V. N., Kathare, P. K., and Huq, E. (2018). Phytochromes and phytochrome interacting factors. Plant Physiol. 176, 1025–1038. doi: 10.1104/pp.17.01384
Rakusova, H., Gallego-Bartolome, J., Vanstraelen, M., Robert, H. S., Alabadi, D., Blazquez, M. A., et al. (2011). Polarization of PIN3-dependent auxin transport for hypocotyl gravitropic response in Arabidopsis thaliana. Plant J. 67, 817–826. doi: 10.1111/j.1365-313X.2011.04636.x
Ramazanov, Z., Rawat, M., Henk, M. C., Mason, C. B., Matthews, S. W., and Moroney, J. V. (1994). The induction of the CO2-concentrating mechanism is correlated with the formation of the starch sheath around the pyrenoid of Chlamydomonas reinhardtii. Planta 195, 210–216. doi: 10.1007/BF00199681
Ritte, G., Heydenreich, M., Mahlow, S., Haebel, S., Kotting, O., and Steup, M. (2006). Phosphorylation of C6- and C3-positions of glucosyl residues in starch is catalysed by distinct dikinases. FEBS Lett. 580, 4872–4876. doi: 10.1016/j.febslet.2006.07.085
Ritte, G., Lloyd, J. R., Eckermann, N., Rottmann, A., Kossmann, J., and Steup, M. (2002). The starch-related R1 protein is an alpha -glucan, water dikinase. Proc. Natl. Acad. Sci. U.S.A. 99, 7166–7171. doi: 10.1073/pnas.062053099
Roberts, A. M. (2006). Mechanisms of gravitaxis in Chlamydomonas. Biol. Bull. 210, 78–80. doi: 10.2307/4134597
Sack, F. D. (1991). Plant gravity sensing. Int. Rev. Cytol. Survey Cell Biol. 127, 193–252. doi: 10.1016/S0074-7696(08)60695-6
Saito, C., Morita, M. T., Kato, T., and Tasaka, M. (2005). Amyloplasts and vacuolar membrane dynamics in the living graviperceptive cell of the Arabidopsis inflorescence stem. Plant Cell 17, 548–558. doi: 10.1105/tpc.104.026138
Schwuchow, J. M., Kim, D., and Sack, F. D. (1995). Caulonemal gravitropism and amyloplast sedimentation in the moss Funaria. Can. J. Bot. 73, 1029–1035. doi: 10.1139/b95-112
Sessions, A., Weigel, D., and Yanofsky, M. F. (1999). The Arabidopsis thaliana MERISTEM LAYER 1 promoter specifies epidermal expression in meristems and young primordia. Plant J. 20, 259–263. doi: 10.1046/j.1365-313x.1999.00594.x
Shannon, J. C., Pien, F.-M., Cao, H., and Liu, K.-C. (1998). Brittle-1, an adenylate translocator, facilitates transfer of extraplastidial synthesized ADP-glucose into amyloplasts of maize endosperms. Plant Physiol. 117:1235. doi: 10.1104/pp.117.4.1235
Shin, J., Kim, K., Kang, H., Zulfugarov, I. S., Bae, G., Lee, C. H., et al. (2009). Phytochromes promote seedling light responses by inhibiting four negatively-acting phytochrome-interacting factors. Proc. Natl. Acad. Sci. U.S.A. 106, 7660–7665. doi: 10.1073/pnas.0812219106
Slewinski, T. L., and Braun, D. M. (2010). Current perspectives on the regulation of whole-plant carbohydrate partitioning. Plant Sci. 178, 341–349. doi: 10.1016/j.plantsci.2010.01.010
Smith, A. M., Zeeman, S. C., and Smith, S. M. (2005). Starch degradation. Annu. Rev. Plant Biol. 56, 73–98. doi: 10.1146/annurev.arplant.56.032604.144257
Streb, S., and Zeeman, S. C. (2012). Starch metabolism in Arabidopsis. Arabidopsis Book 10:e0160. doi: 10.1199/tab.0160
Taniguchi, M., Furutani, M., Nishimura, T., Nakamura, M., Fushita, T., Iijima, K., et al. (2017). The Arabidopsis LAZY1 family plays a key role in gravity signaling within statocytes and in branch angle control of roots and shoots. Plant Cell 29, 1984–1999. doi: 10.1105/tpc.16.00575
Toyota, M., Ikeda, N., Sawai-Toyota, S., Kato, T., Gilroy, S., Tasaka, M., et al. (2013). Amyloplast displacement is necessary for gravisensing in Arabidopsis shoots as revealed by a centrifuge microscope. Plant J. 76, 648–660. doi: 10.1111/tpj.12324
Tsai, H. L., Lue, W. L., Lu, K. J., Hsieh, M. H., Wang, S. M., and Chen, J. (2009). Starch synthesis in Arabidopsis is achieved by spatial cotranscription of core starch metabolism genes. Plant Physiol. 151, 1582–1595. doi: 10.1104/pp.109.144196
Tuncel, A., and Okita, T. W. (2013). Improving starch yield in cereals by over-expression of ADPglucose pyrophosphorylase: expectations and unanticipated outcomes. Plant Sci. 211, 52–60. doi: 10.1016/j.plantsci.2013.06.009
Van Harsselaar, J. K., Lorenz, J., Senning, M., Sonnewald, U., and Sonnewald, S. (2017). Genome-wide analysis of starch metabolism genes in potato (Solanum tuberosum L.). BMC Genomics 18:37. doi: 10.1186/s12864-016-3381-z
Walker, L. M., and Sack, F. D. (1990). Amyloplasts as possible statoliths in gravitropic protonemata of the moss Ceratodon purpureus. Planta 181, 71–77. doi: 10.1007/BF00202326
Yano, D., Sato, M., Saito, C., Sato, M. H., Morita, M. T., and Tasaka, M. (2003). A SNARE complex containing SGR3/AtVAM3 and ZIG/VTI11 in gravity-sensing cells is important for Arabidopsis shoot gravitropism. Proc. Natl. Acad. Sci. U.S.A. 100, 8589–8594. doi: 10.1073/pnas.1430749100
Yoshihara, T., and Iino, M. (2007). Identification of the gravitropism-related rice gene LAZY1 and elucidation of LAZY1-dependent and -independent gravity signaling pathways. Plant Cell Physiol. 48, 678–688. doi: 10.1093/pcp/pcm042
Yoshihara, T., and Spalding, E. P. (2017). LAZY genes mediate the effects of gravity on auxin gradients and plant architecture. Plant Physiol. 175, 959–969. doi: 10.1104/pp.17.00942
Yoshihara, T., Spalding, E. P., and Iino, M. (2013). AtLAZY1 is a signaling component required for gravitropism of the Arabidopsis thaliana inflorescence. Plant J. 74, 267–279. doi: 10.1111/tpj.12118
Yu, T.-S., Kofler, H., Häusler, R. E., Hille, D., Flügge, U.-I., Zeeman, S. C., et al. (2001). The Arabidopsis sex1 mutant is defective in the R1 protein, a general regulator of starch degradation in plants, and not in the chloroplast hexose transporter. Plant Cell 13:1907. doi: 10.2307/3871327
Yu, T.-S., Lue, W.-L., Wang, S.-M., and Chen, J. (2000). Mutation of Arabidopsis plastid phosphoglucose isomerase affects leaf starch synthesis and floral initiation. Plant Physiol. 123:319. doi: 10.1104/pp.123.1.319
Zeeman, S. C., Kossmann, J., and Smith, A. M. (2010). Starch: its metabolism, evolution, and biotechnological modification in plants. Annu. Rev. Plant Biol. 61, 209–234. doi: 10.1146/annurev-arplant-042809-112301
Zhang, Y., He, P., Ma, X., Yang, Z., Pang, C., Yu, J., et al. (2019a). Auxin-mediated statolith production for root gravitropism. New Phytol. 224, 761–774. doi: 10.1111/nph.15932
Keywords: Arabidopsis, gravitropism, liverwort, rice, starch, EARLY STARVATION 1
Citation: Song K, Lee D-W, Kim J, Kim J, Guim H, Kim K, Jeon J-S and Choi G (2021) EARLY STARVATION 1 Is a Functionally Conserved Protein Promoting Gravitropic Responses in Plants by Forming Starch Granules. Front. Plant Sci. 12:628948. doi: 10.3389/fpls.2021.628948
Received: 13 November 2020; Accepted: 29 June 2021;
Published: 23 July 2021.
Edited by:
Rujin Chen, Lanzhou University, ChinaReviewed by:
Haodong Chen, Tsinghua University, ChinaUte Hoecker, University of Cologne, Germany
Dmitry Suslov, Saint Petersburg State University, Russia
Copyright © 2021 Song, Lee, Kim, Kim, Guim, Kim, Jeon and Choi. This is an open-access article distributed under the terms of the Creative Commons Attribution License (CC BY). The use, distribution or reproduction in other forums is permitted, provided the original author(s) and the copyright owner(s) are credited and that the original publication in this journal is cited, in accordance with accepted academic practice. No use, distribution or reproduction is permitted which does not comply with these terms.
*Correspondence: Giltsu Choi, Z2Nob2lAa2Fpc3QuYWMua3I=; Jong-Seong Jeon, amplb25Aa2h1LmFjLmty
†These authors have contributed equally to this work