- State Key Laboratory of Crop Stress Biology for Arid Areas/Shaanxi Key Laboratory of Apple, College of Horticulture, Northwest A&F University, Xianyang, China
Tyrosine is decarboxylated to tyramine by TYDC (Tyrosine decarboxylase) and then hydroxylated to dopamine, which is involved in plant response to abiotic stress. However, little is known about the function of MdTyDc in response to alkaline stress in plants. In our study, it was found that the expression of MdTyDc was induced by alkaline stress. Therefore, the apple plants overexpressing MdTyDc was treated with alkali stress, and we found that MdTyDc played an important role in apple plants’ resistance to alkali stress. Our results showed that the restriction on the growth, the decrease of membrane permeability and the accumulation of Na+ were alleviated to various degrees in MdTyDc transgenic plants under alkali stress. In addition, overexpression of MdTyDc enhanced the root activity and photosynthetic capacity, and improved the enzyme activity related to N metabolism, thus promoting N absorption. It is noteworthy that the dopamine content of these three transgenic lines is significantly higher than that of WT. In summary, these findings indicated that MdTyDc may enhance alkaline tolerance of apples by mediating dopamine content, mainly by maintaining high photosynthetic capacity, normal ion homeostasis and strong nitrogen absorption capacity.
Introduction
Soil salinization is one of the key abiotic stresses that inhibit plant growth; it significantly restricts agricultural production, especially in arid and semi-arid areas (Rozema and Flowers, 2008; Zhang et al., 2010). Alkaline stress (the stress caused by basic salts, primarily Na2CO3, and NaHCO3) includes components of osmotic stress, ion toxicity, and high pH stress; it greatly influences root function, photosynthesis, biological membranes, ion homeostasis, and nutrient metabolism, thereby strongly inhibiting plant growth (Yang et al., 2009; Guo et al., 2010, 2015a; Wang et al., 2015a; Sun et al., 2016; Liu et al., 2019a). Therefore, it is very important to improve the capacity of plants to resist alkaline stress.
Photosynthesis is an important physiological and biochemical process that supports plant growth and development. However, the photosynthetic apparatus are vulnerable to alkaline stress, leading to severe inhibition of photosynthesis (Xu et al., 2013; Li et al., 2020b). Alkaline stress hinders photosynthetic electron transfer, causing a decrease in apparent quantum yield and light energy conversion efficiency, thereby inhibiting PSII activity (Gorbe and Calatayud, 2012). In addition, alkaline stress can cause chlorophyll degradation, reduce chlorophyll content in thylakoid membranes, and inhibit the function of pigment–protein complexes (Yang et al., 2011).
The maintenance of intracellular ion balance and pH stability are necessary to ensure normal metabolism and energy conversion in plants. Alkaline stress significantly increases the content of sodium ions in the root zone, thereby causing serious osmotic stress to plants (Guo et al., 2010). Plants can respond to multiple ion stresses such as high Na+, low K+, high Mg2+, and high pH through specific salt overly sensitive (SOS) signal transduction pathways (Zhu, 2016). The known SOS system has four components: SOS1, SOS2, SOS3, and NHX (Zhu, 2016). The SOS1 gene encodes a Na+/H+ antiporter (NHX) in the cell membrane that is responsible for sensing and expelling Na+ from the root (ElMahi et al., 2019). The SOS2/SOS3 complex negatively regulates the activity of NHX and high affinity K+ channels (HKT), positively regulates the activity of low affinity K channels (AKT), and promotes the absorption of K+ by root cells (Zhu, 2003). Under alkaline stress, plants can also absorb anions (primarily Cl–, SO42–, NO3–, and organic acids) to balance the excess accumulation of cations in stems and leaves and maintain a stable pH (Yang et al., 2008). In addition, plants can alleviate osmotic stress by increasing their intracellular proline content and soluble sugar content (Flowers and Colmer, 2008; Dai et al., 2018).
Nitrogen (N) is one of the main elements that limit plant growth and crop yield, but plant N absorption can be significantly inhibited by low osmotic stress (Botella et al., 1997). Higher plants acquire N from both organic and inorganic sources, and the latter include NO3– and NH4+ (Britto and Kronzucker, 2002; Bloom, 2015). Nitrate reductase (NR), nitrite reductase (NiR), glutamine synthetase (GS), and glutamic acid synthase (GOGAT) are key enzymes in the process of plant N uptake, transport, and assimilation (Luo et al., 2013). Previous work has shown that the damage caused by alkaline stress perturbs plant ion balance, impairing the activity of NR in cells, and thus inhibiting N absorption (Fujiyama, 2005). In addition, studies on N absorption and assimilation have been performed at the molecular and cellular levels, including studies of NH4+ transport by the ammonium transporter (AMT) protein family and NO3– transport by the nitrate transporter (NRT) protein family (Wang et al., 2018). Moreover, the expression of NRT and AMT genes was shown to change in response to alkaline stress in rice (Wang et al., 2012a). In Arabidopsis NRT1, AtNRT1.1, and AtNRT1.2 are mainly responsible for NO3– uptake in roots (Orsel et al., 2007). OsNRT1.1 and OsNRT1.2 were up-regulated in old leaves under alkaline stress, suggesting that these two genes play an important role in NO3– accumulation. AtNRT2.4 is expressed in leaves and roots and is mainly involved in NO3– transport from phloem to leaves (Kiba et al., 2012). AtNRT2.5 is mainly located in the root epidermis and cortex, and its transcription is strongly induced by N starvation (Lezhneva et al., 2015). AtNRT2.7 is located in the vacuolar membrane and its function is mainly to mediate NO3– transport in the vacuole (Chopin et al., 2007). Huang et al. (2018) reported that members of the NRT family (MdNRT1.1, MdNRT2.4, MdNRT2.5, MdNRT2.7) were involved in the response to PEG-induced drought stress in apples. The roots of plants mainly absorb NH4+ through AMT. AtAMT1.1, AtAMT1.2, AtAMT1.5, AtAMT2.1, and AtAMT3.1 are mainly expressed in root and aboveground parts (Sohlenkamp et al., 2000; Yuan et al., 2009). AtAMT1.1 plays a leading role in the transport of NH4+ under N deficiency, and AtAMT1.2 is mainly expressed in the endodermis of roots and is responsible for transporting NH4+ from roots to aboveground parts (Gazzarrini et al., 1999; Yuan et al., 2007). PtrAMT1.5 and PtrAMT1.6 play a certain role in plant reproductive development in Populus trichocarpa (Couturier et al., 2007). AtAMT2.1 and AtAMT2.2 has the function of high affinity ammonium transport in Arabidopsis (Sohlenkamp et al., 2002). The expression levels of MdAMT3.1 and MdAMT4.2 and MdAMT4.3 in apple roots increased significantly with the decrease of N supply level (Huang et al., 2018).
Dopamine is a type of catecholamine that plays a vital role in plants, and various studies have shown that it has an important relationship with plant stress resistance. Li et al. (2015) demonstrated that dopamine relieved salt stress not only at the level of antioxidant defense but also through mechanisms such as the maintenance of ion balance. Exogenous dopamine improved the salt tolerance of apple by promoting its symbiosis with arbuscular mycorrhizal fungi (Gao et al., 2020a). In addition, dopamine has been shown to alleviate drought stress in apple seedlings through a variety of physiological mechanisms (Gao et al., 2020b). Liu et al. (2020) found that the absorption of mineral nutrients in apple seedlings was upregulated by exogenous dopamine, thereby promoting resistance to low N stress. Tyrosine decarboxylase (TYDC) is a key enzyme in the plant dopamine synthesis pathway (Nagatsu et al., 1972; Kong et al., 1998) and is involved in many plant secondary metabolism and defense processes. For example, RcTyDc can promote salidroside biosynthesis (Lan et al., 2013). Overexpression of TYDC in potato promoted the synthesis of tyrosine and effectively improved disease resistance (Landtag et al., 2002). Lehmann and Pollmann (2009) showed that the expression of TYDC in Arabidopsis was induced by drought stress and injury.
Previously, we found that dopamine content was increased by the overexpression of MdTyDc in apple seedlings and calli, and apple salt tolerance was improved by its overexpression in apple plants (Wang et al., 2020). Moreover, previous studies in our lab showed that the expression of N-metabolism-related genes in apple seedlings was up-regulated by exogenous dopamine under alkaline stress, permitting better adaptation to this stress (Jiao et al., 2019). Although the effects of TYDC on plant growth and resistance to biotic and abiotic stress have been described, the mechanism by which TYDC influences plant response to alkaline stress is not fully understood. Here, we found that overexpression of MdTyDc improved the ability of apples to cope with alkaline stress. This study adds to our understanding of the mechanisms by which MdTyDc functions in response to alkaline stress.
Materials and Methods
Plant Materials and Alkaline Treatments
Our experiments were carried out at the Northwest A&F University, Yangling (34°20′ N, 108°24′ E) in Shaanxi, China. WT and transgenic GL-3 (Malus domestica cv. Gala) plants were obtained from the previous research in our laboratory (Wang et al., 2020). After subculturing and rooting, the apple plants were transplanted to plastic pots (12 × 12 cm) filled with soil/perlite/vermiculite (4:1:1, v:v:v) and placed in a growth chamber. WT and transgenic apple plants at the same growth stage (7–9 true leaves) were transferred to plastic containers (52 × 37 × 15 cm3) that were wrapped in black plastic and contained 13 L half-strength Hoagland’s nutrient solution. The pH of the nutrient solution was adjusted to 6.0 ± 0.2 with H3PO4 or KOH, and it was changed every 5 days. Our hydroponic culture system was designed and used as described in Li et al. (2012). The apple plants were cultivated in a growthchamber where the growth conditions included a 14 h photoperiod (the light intensity was 160 μmol m–2 s–1), 24 ± 2°C/16 ± 2°C day/night and 60 ± 5% relative humidity. After 12 days of pre-cultivation, WT and transgenic GL-3 plants of uniform size were selected and randomly divided into two groups: (1) the control group (CK) that received half-strength Hoagland’s nutrient solution with a pH of 6.0 ± 0.2 and (2) the alkaline stress group (AL) that received half-strength Hoagland’s nutrient solution with a pH of 9.0 ± 0.2. The solution pH was adjusted with 1 M NaHCO3 and 1 M Na2CO3 (1:1), and the treatment lasted for 15 days.
Measurement of Dopamine Contents
A 0.1 g sample of fresh leaf and root tissues was weighed and placed in a 2 mL centrifuge tube, and 1 mL acidic methanol (hydrochloric acid:methanol = 1:9, v/v) was added. After mixing well, all samples were centrifuged at 3,500 rpm for 5 min. The supernatant was filtered through a 0.45 μm membrane and analyzed by high-performance liquid chromatography (HPLC, LC-2010, Shimadzu, Japan).
Growth Measurements
At the beginning and end of the alkaline stress treatment, the growth indices of all plants were measured. The stem height (SH) was measured with a plastic ruler, and the leaf number (LN) was counted. All the harvested plants were divided into three parts: roots, stems, and leaves. Total fresh weight (TFW), total dry weight (TDW), root–shoot ratio (RSR), and relative growth rate (RGR) were calculated as described previously (Liang et al., 2017).
Root Architecture Measurements
Root systems were carefully cleaned without damaging the roots. After the roots had been flattened and spread out, a SNAPSCAN 310 scanner was used to obtain their images. Finally, root system parameters were analyzed with WinRHIZO image analysis software (V4.1c; Regent Instruments, Quebec City, QC, Canada).
Measurement of Root Activity and Relative Electrolyte Leakage
Root vital staining was performed using the triphenyl tetrazolium chloride (TTC) method of Joseph et al. (2010), and the dehydrogenase activity (mg g–1 FW h–1) was used to represent root activity. Relative electrolyte leakage (REL) was measured by the method of Dionisio-Sese and Tobita (1998).
Quantification of Photosynthetic Characteristics
The net photosynthesis rate (Pn), intercellular CO2 concentration (Ci), stomatal conductance (Gs), and transpiration rate (Tr) were recorded from 9:00 to 11:00 a.m. with a CIRAS-3 portable photosynthesis system (CIRAS-3, PP Systems, Amesbury, United States). All photosynthetic characteristics were measured at 1,000 μmol photons m–2 s–1 and a constant 500 μmol s–1 airflow rate. The CO2 concentration was 400 μmol CO2 mol–1 air. For each treatment, photosynthetic parameters were measured from 10 fully exposed and mature leaves at the same position.
Chlorophyll Content and Fv/Fm Measurements
According to the method of Arnon (1949), the cleaned leaves were cut into filaments of about 0.1 cm with scissors. 0.1 g of the mixed filaments were weighed and loaded into a 10 mL centrifuge tube containing 8 mL 80% acetone. After shaking evenly, they were placed in a dark place (room temperature, 24 h) and shaken 3–4 times during the period. The optical density values at the wavelengths of 663, 645, and 470 nm were determined by UV-2250 spectrophotometer (Shimadzu, Kyoto, Japan).
For chlorophyll fluorescence measurements, black cloth was used to cover fresh, mature leaves at the same position on plants from each treatment for 30 min. Fv/Fm (the maximum quantum efficiency of photosystem II) was measured using a three-dimensional chlorophyll fluorescence imaging system (FC800, PSI, Czech Republic). The parameters of this system were set as follows: the shutter value was 1; the light source was flashes; and the sensitivity value was 70%.
Measurements of Inorganic Ion and Proline Content
The apple plants were washed with tap water, distilled water and double distilled water successively, and the water on the surface of plants was carefully dried with filter paper. Then the plants were divided into root, stem and leaf parts. After 30 min of high temperature treatment at 105°C, the plants were placed at 80°C for at least 72 h to constant weight. After drying, the samples were broken and bagged with a pulverizer for the determination of mineral element content. 0.1 g of dry sample was weighed and placed into a centrifuge tube with 20 mL of deionized water. Then it was extracted at 100°C for 20 min for the determination of ions contents. The contents of Na+ and K+ were determined by flame photometry (FP6410 flame photometer). The contents of nitrate, chloride, sulfate, dihydrogen phosphate, and oxalic acid Cl–, SO42–, and NO3– were determined by ion chromatography (Thermo Fisher Scientific DIONEX ICS-1100). 0.1 g of dry sample was weighed and placed into a desiccating tube containing 5 mL concentrated sulfuric acid (H2SO4, AR, 98%). H2O2 was added for deboiling according to the digestion method of plant sample. After disboiling, the digestion solution was filled with deionized water at constant volume to 100 mL, and the supernatant was to be measured. The content of N was determined by AA3 continuous flow analyzer.
Proline (Pro) was extracted and measured according to the method of Jin et al. (2019), and Pro content was analyzed using liquid chromatography–mass spectrometry (LC–MS, LC: AC, ExionLC; MS:Q-trap5500, AB Sciex Pret., Ltd., Framingham, MA, United States). Methanol and 0.1% methanolic acid were used as mobile phases A and B, respectively. The flow rate was set to 0.3 mL min–1. The injection volume is 10 μl. The retention time of Pro was 12 min, and the content of Pro was calculated using the peak area of the standard curve.
Measurement of Enzyme Activities
Three apple plants were randomly sampled from each treatment on the 15th day after alkaline stress treatment. They were washed with distilled water and dried with filter paper. After the leaves and roots of each plant were separated, they were mixed separately and immediately put into liquid nitrogen. Then they were placed in the −80°C refrigerator for testing. The activity of nitrate reductase (NR) was determined by the method of Högberg et al. (1986), the activity of nitrite reductase (NiR) was measured according the previous study (Seith et al., 1994), the activity of glutamine synthase (GS) was analyzed by spectrophotometry (Wang et al., 2008) and the activity of glutamate synthase (GOGAT) by was tested based on the method of Lin and Kao (1996).
qRT–PCR Analysis
Total RNA was extracted from leaf and root samples using the Wolact plant RNA extraction kit (Vicband, Hong Kong, China). Complementary DNA was then reverse transcribed using the PrimeScript RT reagent Kit with gDNA Eraser (Perfect Real Time). The real-time quantitative PCR (qRT-PCR) reactions were carried out using the SYBR Green qPCR kit (TaKaRa, Tokyo, Japan) and the iQ5 Multicolor Detection System (Bio-Rad Laboratories, Hercules, United States). Perini et al. (2014) showed that MdMDH (malate dehydrogenase gene) were found to be the most stable and suitable normalizers for all apple tissue expression analyses by RT-qPCR. Therefore, MDH was used as an internal standard. The specific primer sequences used for qRT–PCR are provided in Supplementary Table S1. At 0, 3, 12, 24 h, 3, 5, 10, and 15 days after alkali stress treatment, leaves and roots were randomly selected for measurement.
Statistical Analysis
The results are presented as means ± SD. The differences between treatments were evaluated by Tukey’s test after a one-way ANOVA (P < 0.05) with SPSS 25.0 for Windows.
Results
Overexpression of MdTyDc Alleviates Alkaline Stress in Apple
MdTyDc expression was induced by alkaline treatment of GL-3 apple plants (Figure 1A), and three overexpressing (OE) apple lines were used to investigate the role of MdTyDc under alkaline stress. In OE-2, OE-3, and OE-5 lines, MdTyDc transcripts were elevated 28. 81-, 47. 89-, and 70.87-fold compared with WT (Figure 1B).
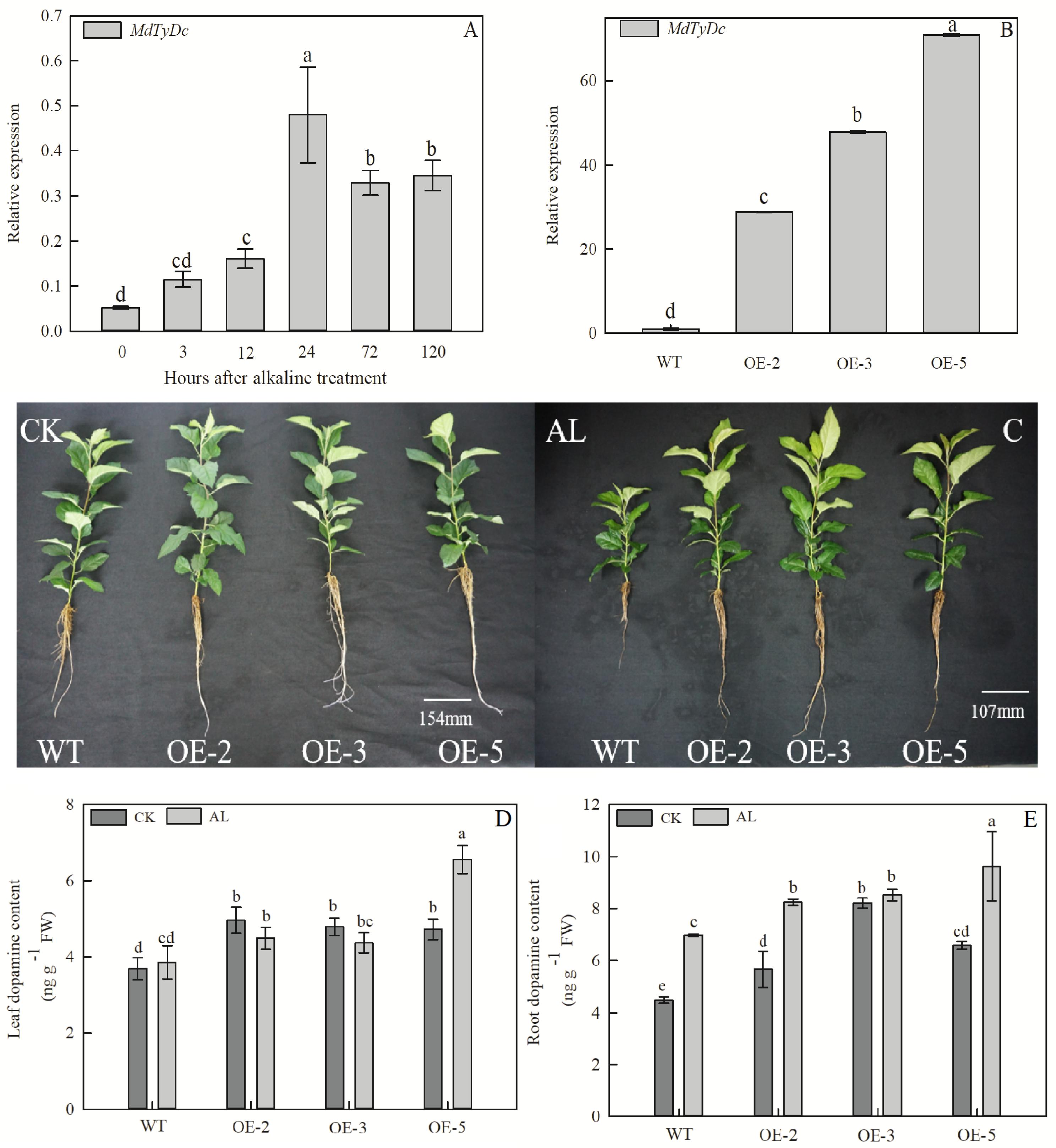
Figure 1. Overexpression of MdTyDc confers enhanced alkaline tolerance to apple. (A) The expression of MdTyDc after alkaline treatment in GL-3 apple; (B) qRT–PCR analysis of MdTyDc transcripts in lines WT, OE-2, OE-3, and OE-5; (C) the phenotype and dopamine content of MdTyDc-overexpressing apple plants in (D) leaves and (E) roots after 15 days under control and alkaline conditions. The data are presented as means ± SD (n = 3). Significant differences between WT and MdTyDc overexpression lines are indicated by different lowercase letters based on Tukey’s multi-range test (P < 0.05). WT, wild type. OE-2, MdTyDc overexpression line 2. OE-3, MdTyDc overexpression line 3. OE-5, MdTyDc overexpression line 5.
The growth of apple plants was severely inhibited after 15 days of alkaline stress; however, OE lines were more tolerant to alkaline stress than WT (Figure 1C). Plant height and leaf number were significantly higher in OE lines than in WT under alkaline conditions (Table 1). The TFW, TDW, and RGR of all genotypes were not significantly different under normal hydroponics conditions. However, these parameters were notably lower in WT plants than OE lines under alkaline stress (Table 1). In addition, the WT plants showed clear increases in RSR compared with OE plants under stress treatment (Table 1). All these results indicated that MdTyDc plays an important role in the response to alkaline stress.
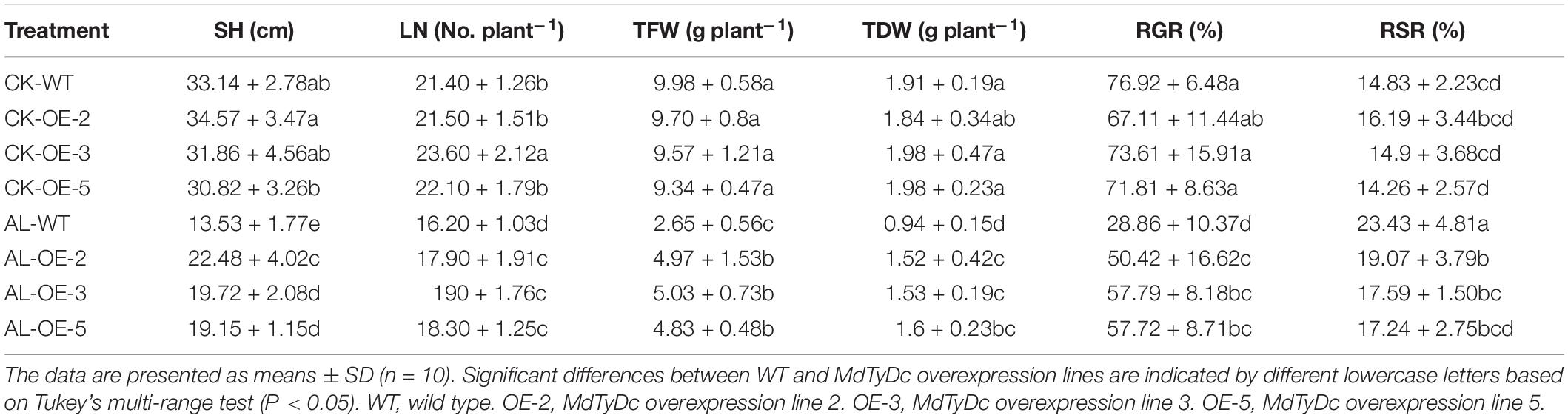
Table 1. Stem height (SH), leaf number (LN), total fresh weight (TFW), total dry weight (TDW), relative growth rate (RGR), and root stem ratio (RSR) of MdTyDc-overexpressing apple plants after 15 days under control and alkaline conditions.
Overexpression of MdTyDc Increased Dopamine Content
Because tyrosine decarboxylase is a vital enzyme in the plant dopamine synthesis pathway, plant dopamine contents were measured. The content of dopamine in leaves and roots of OE lines was significantly higher than that of WT lines under both normal and alkaline stress conditions (Figures 1D,E and Supplementary Figure S1). Moreover, after 5 days of alkaline treatment, the dopamine content in the leaves and roots of WT and OE plants was markedly increased due to the damage caused by alkaline stress (Supplementary Figure S1). These data showed that the overexpression of MdTyDc increases dopamine content in apple plants under both normal and alkaline conditions.
Overexpression of MdTyDc Affected Root Development, REL, and Proline Content Under Alkaline Conditions
After 15 days of alkaline treatment, the root architecture significantly changed, although WT and OE lines showed no significant differences under normal hydroponics conditions. The root length, surface area, number of root tips, and number of root forks were less affected by alkaline stress in transgenic apple plants than in WT plants (Table 2). Furthermore, under alkaline stress, the average root diameter of WT plants was noticeably smaller than that of OE-2 and OE-3 plants, and the root volume of OE-2 and OE-5 plants was significantly higher than that of WT plants (Table 2).
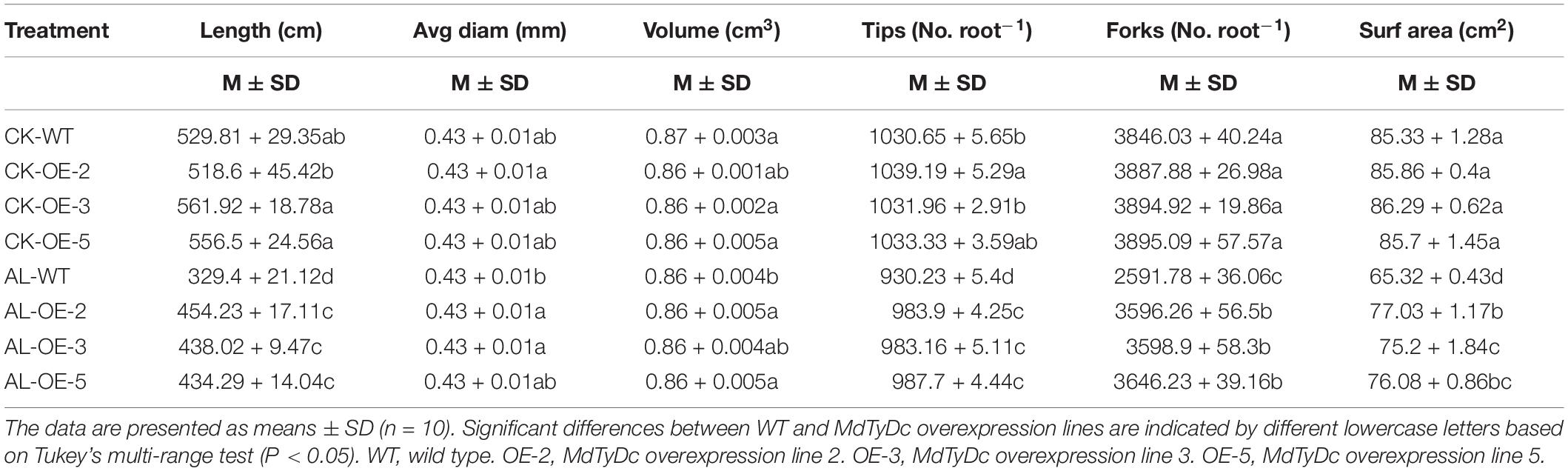
Table 2. Root system architecture of MdTyDc-overexpression apple plants after 15 days under control and alkaline conditions.
TTC reduction was used as an indicator of root activity. TTC reduction decreased in WT plants after 15 days of alkaline treatment, but this decrease was noticeably mitigated in OE plants. TTC reduction measurements from OE-2, OE-3, and OE-5 plants were 32.01, 28.27, and 27.16% higher than those of WT plants (Figure 2A).
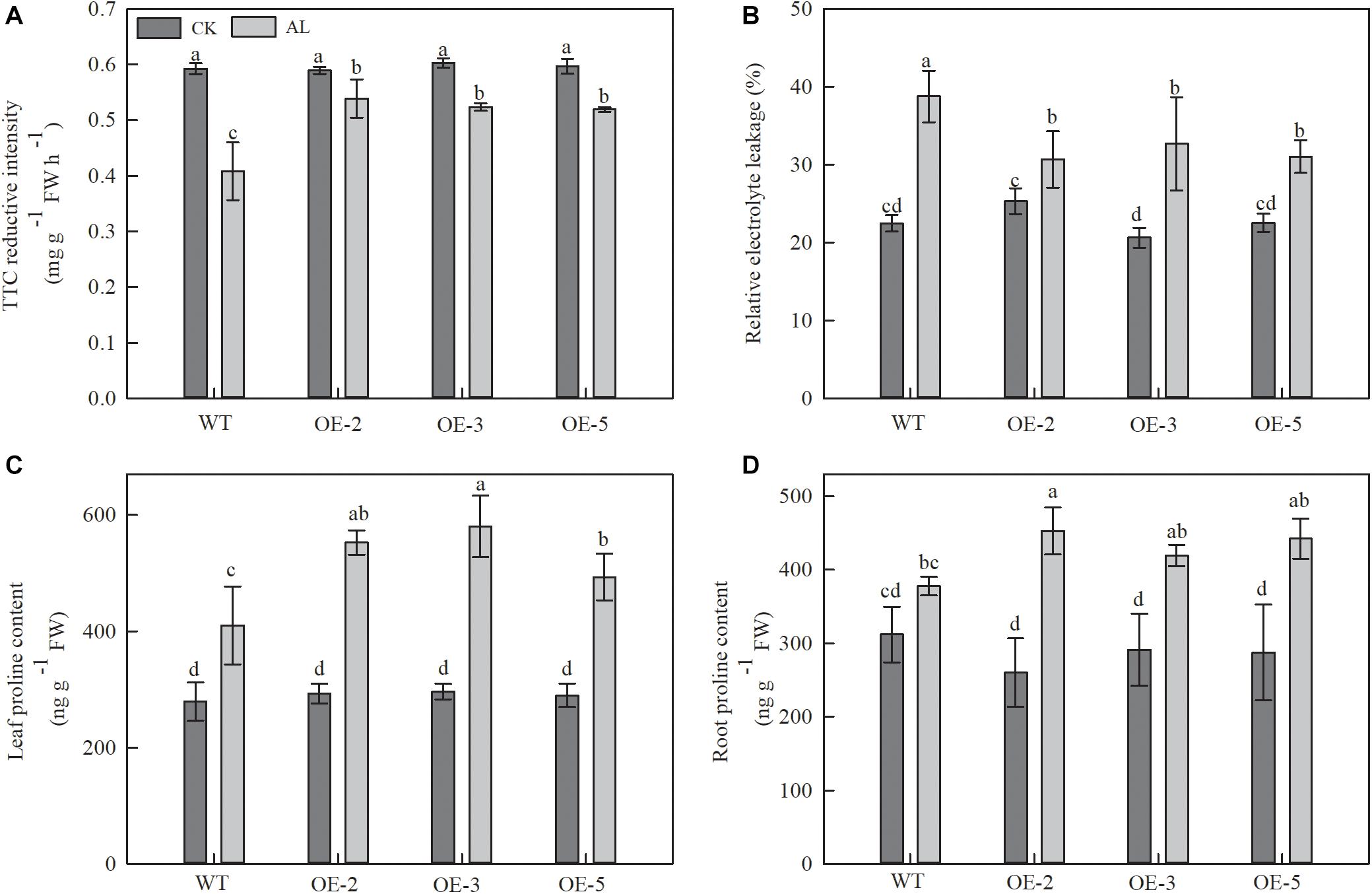
Figure 2. Overexpression of MdTyDc had an effect on the root development, REL and proline content after 15 days under control and alkaline conditions. (A) The root activity, (B) leaf relative electrolyte leakage (REL), (C) leaf proline content, and (D) root proline content. The data are presented as means ± SD (n = 3). Significant differences between WT and MdTyDc overexpression lines are indicated by different lowercase letters based on Tukey’s multi-range test (P < 0.05). WT, wild type. OE-2, MdTyDc overexpression line 2. OE-3, MdTyDc overexpression line 3. OE-5, MdTyDc overexpression line 5.
Relative electrolyte leakage (REL) from leaves was used to assess the influence of alkaline stress on leaf membrane permeability. Under alkaline treatment, REL was 15.67–20.82% lower in the OE lines than in the WT (Figure 2B). These findings suggested that MdTyDc transgenic lines had stronger root systems and leaf membrane systems than WT lines under alkaline stress.
We also measured the content of proline, which is considered to be the main osmotic regulator. Proline content increased in all apple plants under alkaline stress (Figures 2C,D), but that of OE plants was noticeably higher than that of WT plants (Figure 2C). A similar trend was observed for root proline contents under alkaline stress. Although the differences did not reach the threshold for statistical significance, the root proline content of OE-2, OE-3, and OE-5 lines was 19.88, 11.03, and 17.07% higher than that of WT lines (Figure 2D). These results showed that the tolerance of MdTyDc transgenic lines to alkaline stress was related to balanced ion homeostasis and increased proline accumulation.
Apple Lines That Overexpressed MdTyDc Maintained a Stronger Photosynthetic System Under Alkaline Stress
Net photosynthetic rate is another key index of plant growth. The WT and transgenic lines showed no conspicuous differences in Pn, Ci, Gs, or Tr under normal growing conditions (Figures 3A,C,E,G). Pn declined rapidly from the fifth day of alkaline treatment, but the overexpression of MdTyDc slowed this decline noticeably (Figure 3B). The Ci values were markedly higher for OE plants than for WT plants under alkaline conditions (Figure 3D), and Gs and Tr showed a trend similar to that of Pn (Figures 3F,H). Chlorophyll a, chlorophyll b, carotenoid, and total chlorophyll contents also decreased in response to alkaline stress, but this reduction was mitigated in the transgenic lines (Figures 4A–D).
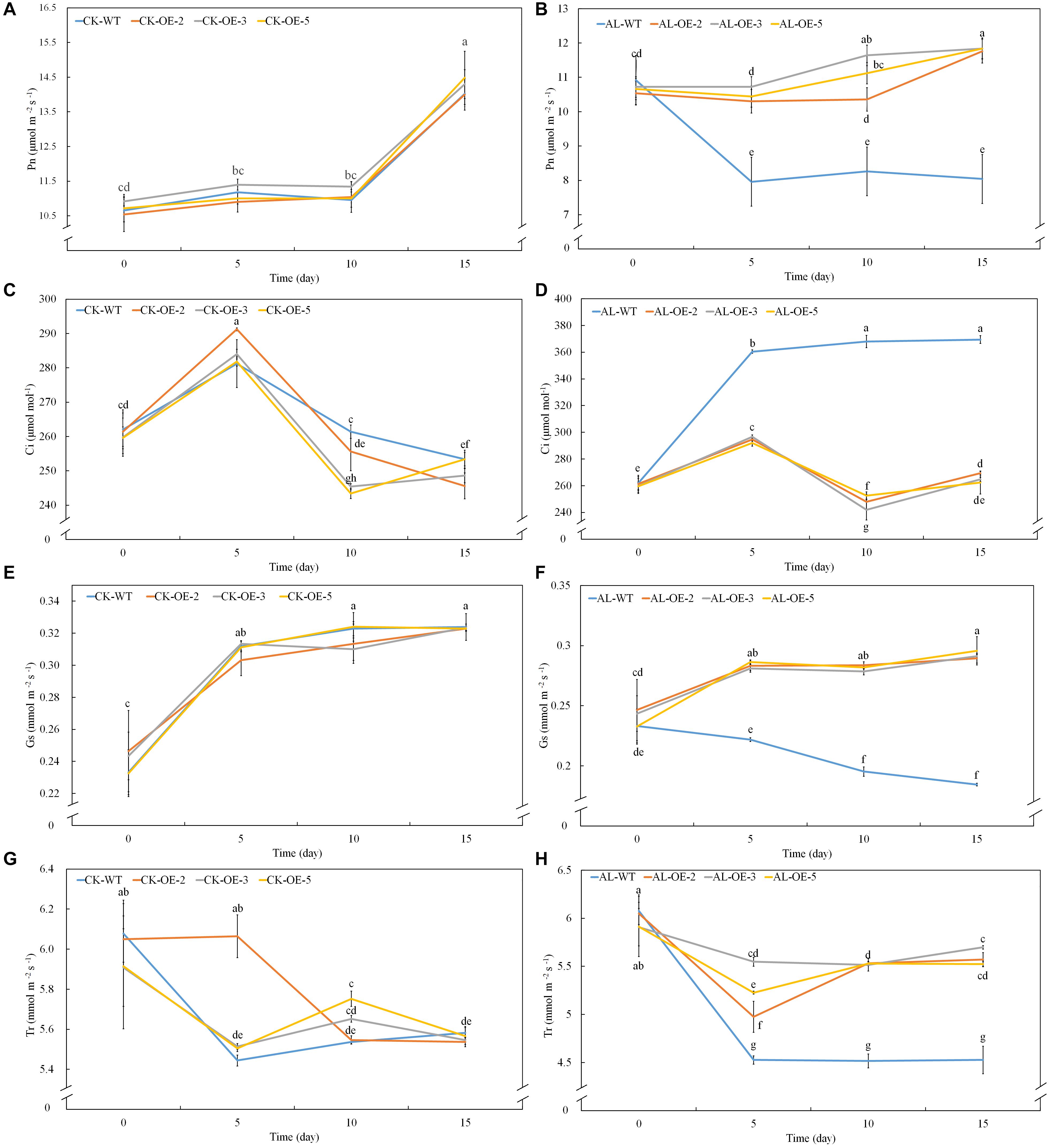
Figure 3. Apple lines overexpressing MdTyDc maintain a stronger photosynthetic system after 15 days under control and alkaline conditions. (A,B) Net photosynthesis (Pn) under (A) control and (B) alkaline conditions, (C,D) intercellular CO2 concentration (Ci) under (C) control and (D) alkaline conditions, (E,F) stomatal conductance (Gs) under (E) control and (F) alkaline conditions, and (G,H) transpiration rate (Tr) under (G) control and (H) alkaline conditions. The data are presented as means ± SD (n = 5). Significant differences between WT and MdTyDc overexpression lines are indicated by different lowercase letters based on Tukey’s multi-range test (P < 0.05). WT, wild type. OE-2, MdTyDc overexpression line 2. OE-3, MdTyDc overexpression line 3. OE-5, MdTyDc overexpression line 5.
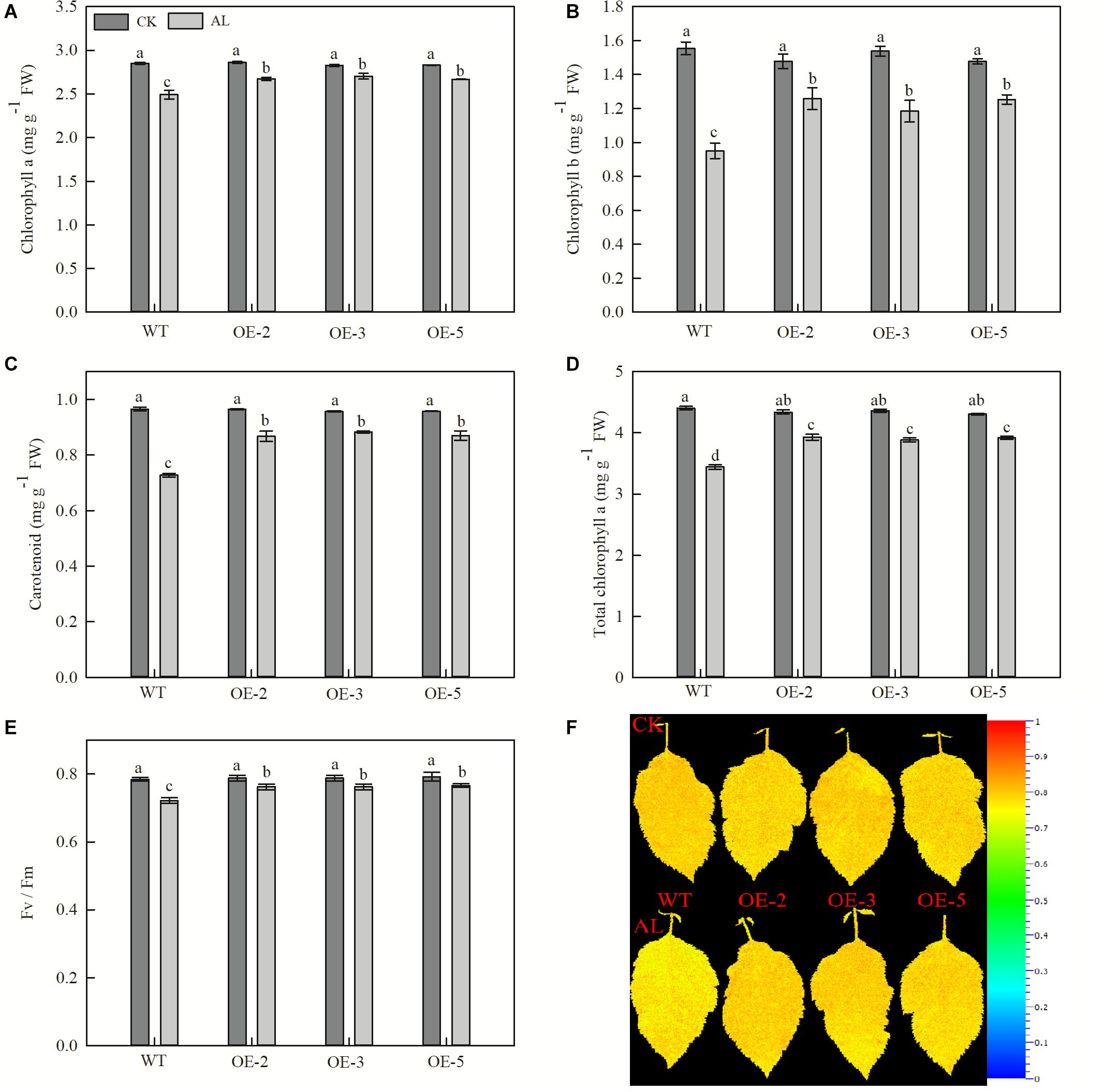
Figure 4. Overexpression of MdTyDc enhanced the chlorophyll concentrations and Fv/Fm after 15 days under control and alkaline conditions. (A) Chlorophyll a, (B) chlorophyll b, (C) carotenoids, (D) total chlorophyll, (E) Fv/Fm, and (F) Fv/Fm image obtained from chlorophyll fluorescence imaging. The data are presented as means ± SD (n = 5). Significant differences between WT and MdTyDc overexpression lines are indicated by different lowercase letters based on Tukey’s multi-range test (P < 0.05). WT, wild type. OE-2, MdTyDc overexpression line 2. OE-3, MdTyDc overexpression line 3. OE-5, MdTyDc overexpression line 5.
The physiological activity of PSII influences chlorophyll fluorescence, and we therefore measured Fv/Fm, which represents the maximum photochemical efficiency of PSII photochemistry. After 15 days of alkaline stress, Fv/Fm decreased by 7.91% in WT plants and by ∼3.29% in the three OE lines (Figures 4E,F). Together, these data indicated that the overexpression of MdTyDc enhanced photosynthetic activity, thereby increasing plant resistance to alkaline stress.
MdTyDc Overexpression Changed Inorganic Ion Content
Na+ content of leaves, shoots, and roots was strongly increased after 15 days of alkaline treatment. However, the Na+ content of these tissues in apple MdTyDc OE lines was significantly lower than that of WT plants (Figures 5A–C). Leaf and root K+ content decreased after alkaline treatment, but this decrease was considerably smaller in the three OE lines than in WT plants, and there was no significant difference in stem K+ content between WT and OE lines (Figures 5D–F). Changes in the Na+/K+ ratio of leaves, stems, and roots followed a trend similar to that of Na+ content (Figures 5G–I).
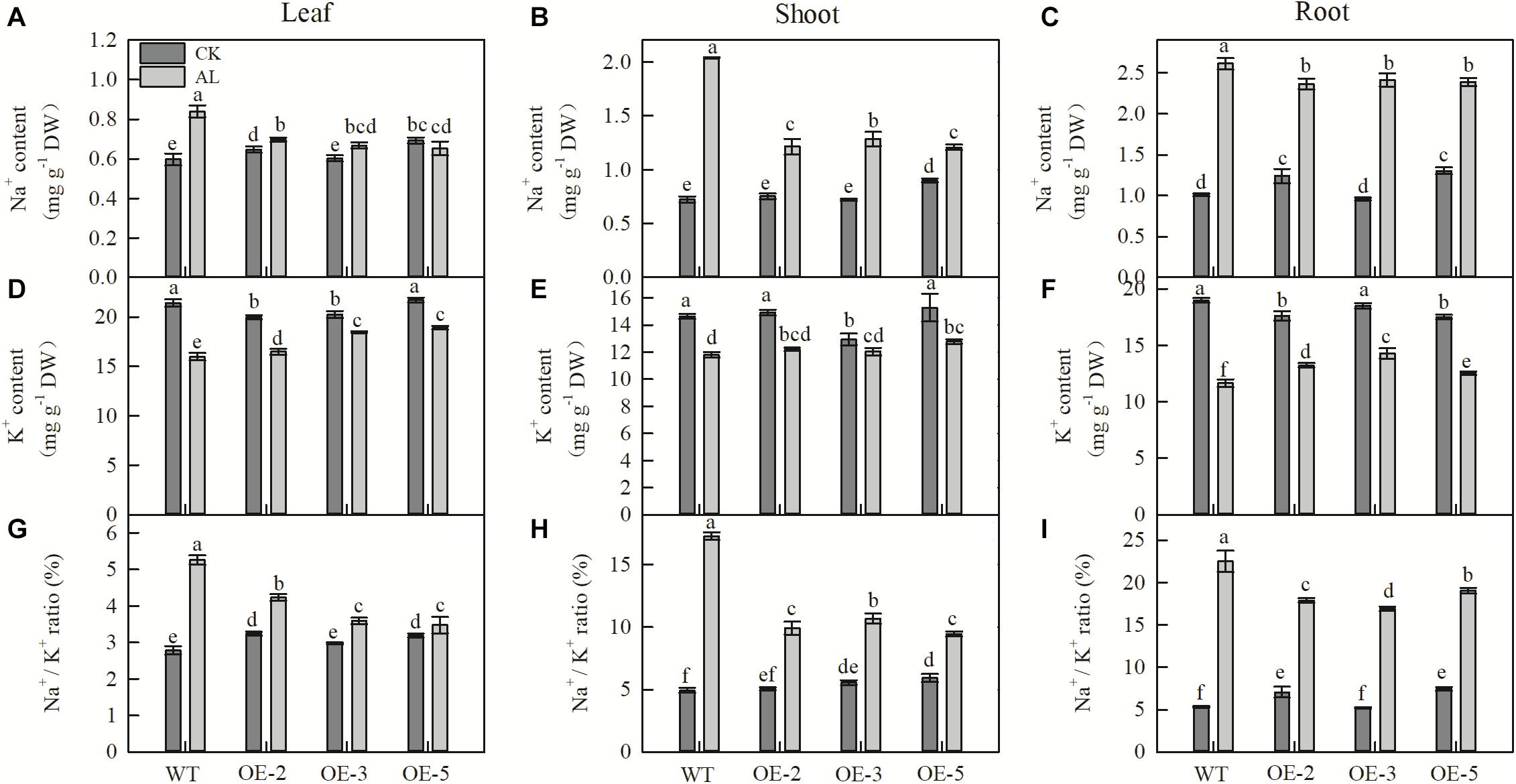
Figure 5. MdTyDc overexpression changed the Na+and K+ content in apple plants after 15 days under control and alkaline conditions. (A) Leaf Na+ content, (B) shoot Na+ content, (C) root Na+ content, (D) leaf K+ content, (E) shoot K+ content, (F) root K+ content, (G) leaf Na+/K+ ratio, (H) shoot Na+/K+ ratio, and (I) root Na+/K+ ratio. The data are presented as means ± SD (n = 3). Significant differences between WT and MdTyDc overexpression lines are indicated by different lowercase letters based on Tukey’s multi-range test (P < 0.05). WT, wild type. OE-2, MdTyDc overexpression line 2. OE-3, MdTyDc overexpression line 3. OE-5, MdTyDc overexpression line 5.
Fifteen days of alkaline treatment reduced the leaf, stem, and root Cl– content of WT plants (Figures 6A–D). However, under alkaline stress, leaf, root, and whole-plant Cl– contents were significantly higher in OE lines than in WT (Figures 6A,C,D). Although the shoot Cl– content of WT plants was higher than that of OE plants under normal and stress conditions, the Cl– content of WT plants decreased significantly under stress conditions, whereas that of OE plants increased significantly (Figure 6B). The SO42– content of WT plants showed results similar to those for Cl– content (Figures 6E–H). Under alkaline stress, there was no significant difference in leaf SO42– content between WT and OE plants (Figure 6E), and trends in shoot SO42– content of the genotypes were similar to those observed for Cl– (Figure 6F). However, under alkaline conditions, the root and whole-plant SO42– contents of OE plants were considerably higher than those of WT plants (Figures 6G,H).
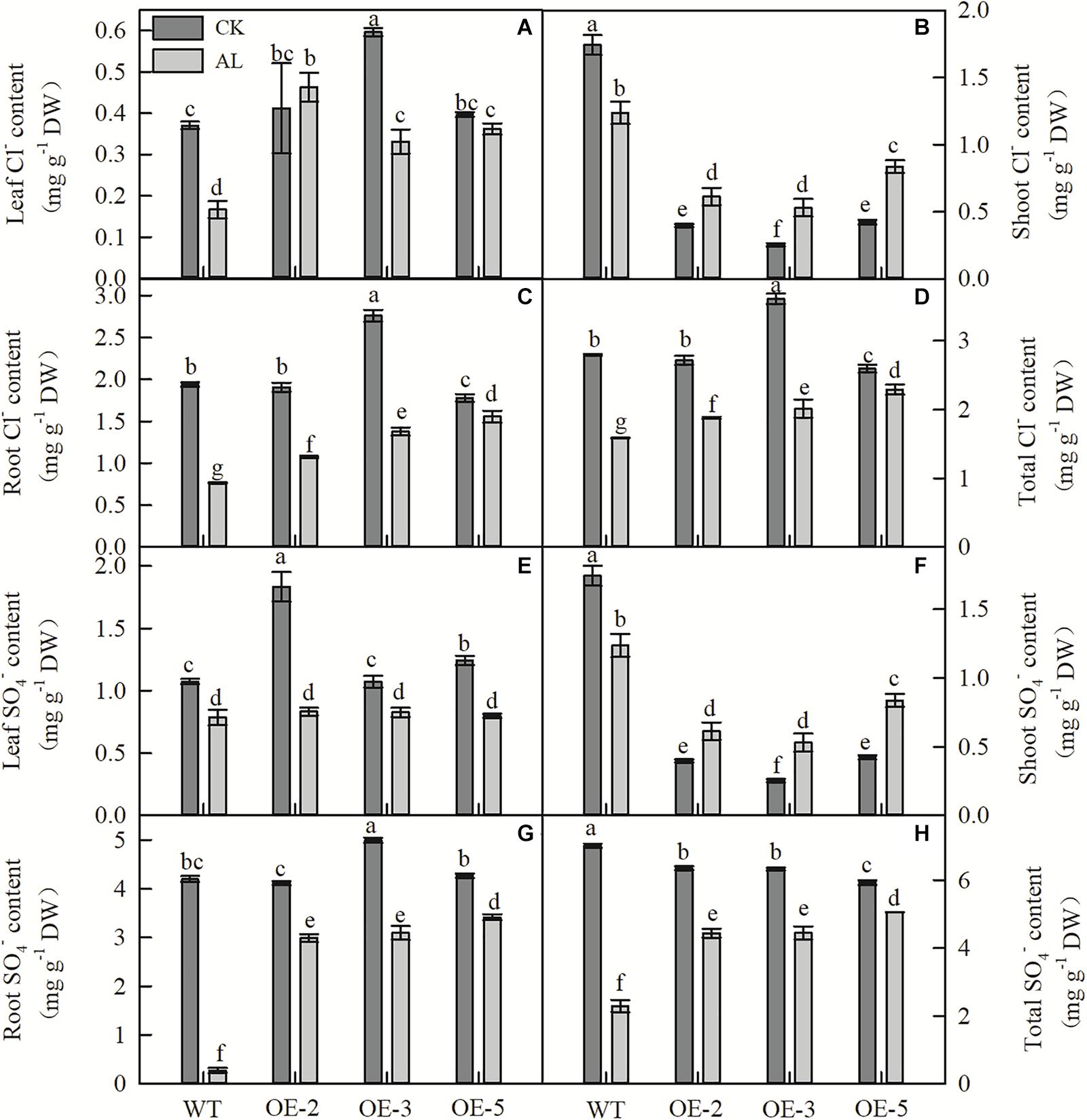
Figure 6. MdTyDc overexpression changed the Cl–and SO42– content in apple plants after 15 days under control and alkaline conditions. (A) Leaf Cl– content, (B) shoot Cl– content, (C) root Cl– content, (D) total Cl– content, (E) leaf SO42– content, (F) shoot SO42– content, (G) root SO42– content, and (H) total SO42– content. The data are presented as means ± SD (n = 3). Significant differences between WT and MdTyDc overexpression lines are indicated by different lowercase letters based on Tukey’s multi-range test (P < 0.05). WT, wild type. OE-2, MdTyDc overexpression line 2. OE-3, MdTyDc overexpression line 3. OE-5, MdTyDc overexpression line 5.
MdTyDc Overexpression Improved N Metabolism Under Alkaline Stress
Because N metabolism is one of the most important metabolic activities in plant life, we measured the N content and the activities of enzymes related to N metabolism. Compared with normal growth conditions, growth under alkaline stress considerably reduced the N content in all apple plants (Figures 7A–D). However, overexpression of MdTyDc markedly enhanced leaf, root, and whole-plant N content of OE lines under stress conditions (Figures 7A,C,D). It is worth noting that the shoot N contents of OE-2 and OE-5 lines were 10.27 and 12.32% higher than that of WT under alkaline stress, but the shoot N content of OE-3 was 11.79% lower (Figure 7B).
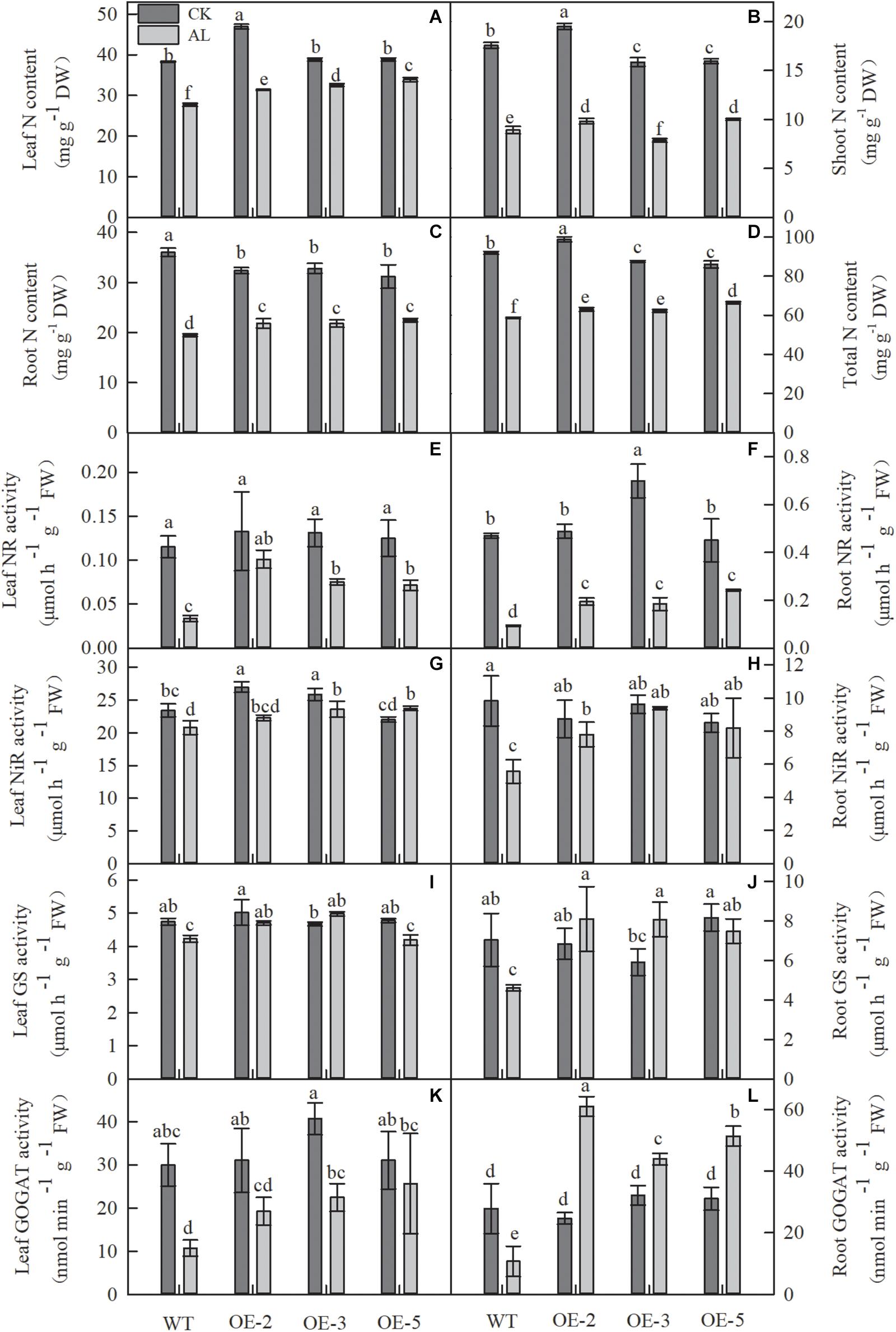
Figure 7. Alkaline stress caused less damage to the nitrogen content and the activity of enzymes involved in N metabolism in MdTyDc OE apples than in WT apples. (A) Leaf N content, (B) shoot N content, (C) root N content, and (D) total N content, (E) the activity of leaf NR, (F) root NR, (G) leaf NiR, (H) root NiR, (I) leaf GS, (J) root GS, (K) leaf GOGAT, and (L) root GOGAT. The data are presented as means ± SD (n = 3). Significant differences between WT and MdTyDc overexpression lines are indicated by different lowercase letters based on Tukey’s multi-range test (P < 0.05). WT, wild type. OE-2, MdTyDc overexpression line 2. OE-3, MdTyDc overexpression line 3. OE-5, MdTyDc overexpression line 5.
After 15 days of alkaline treatment, the activities of NR, NiR, GS, and GOGAT were markedly lower than those measured under normal hydroponic conditions. Nevertheless, all these enzyme activities were considerably improved by the overexpression of MdTyDc under alkaline stress (Figures 7E–L). Interestingly, root GOGAT activity of all three OE lines was dramatically increased under alkaline stress compared with normal conditions (Figure 7L). These data indicated that MdTyDc overexpression in apple improved N metabolism under alkaline treatment, promoting the resistance of apple to alkaline stress.
Overexpression of MdTyDc Affected the Expression of Genes Involved in Ion Transport, N Absorption and C Metabolism
To further investigate the effect of MdTyDc overexpression on apple growth under alkaline stress, we measured the expression of genes related to ion transport and N absorption (Figure 8).
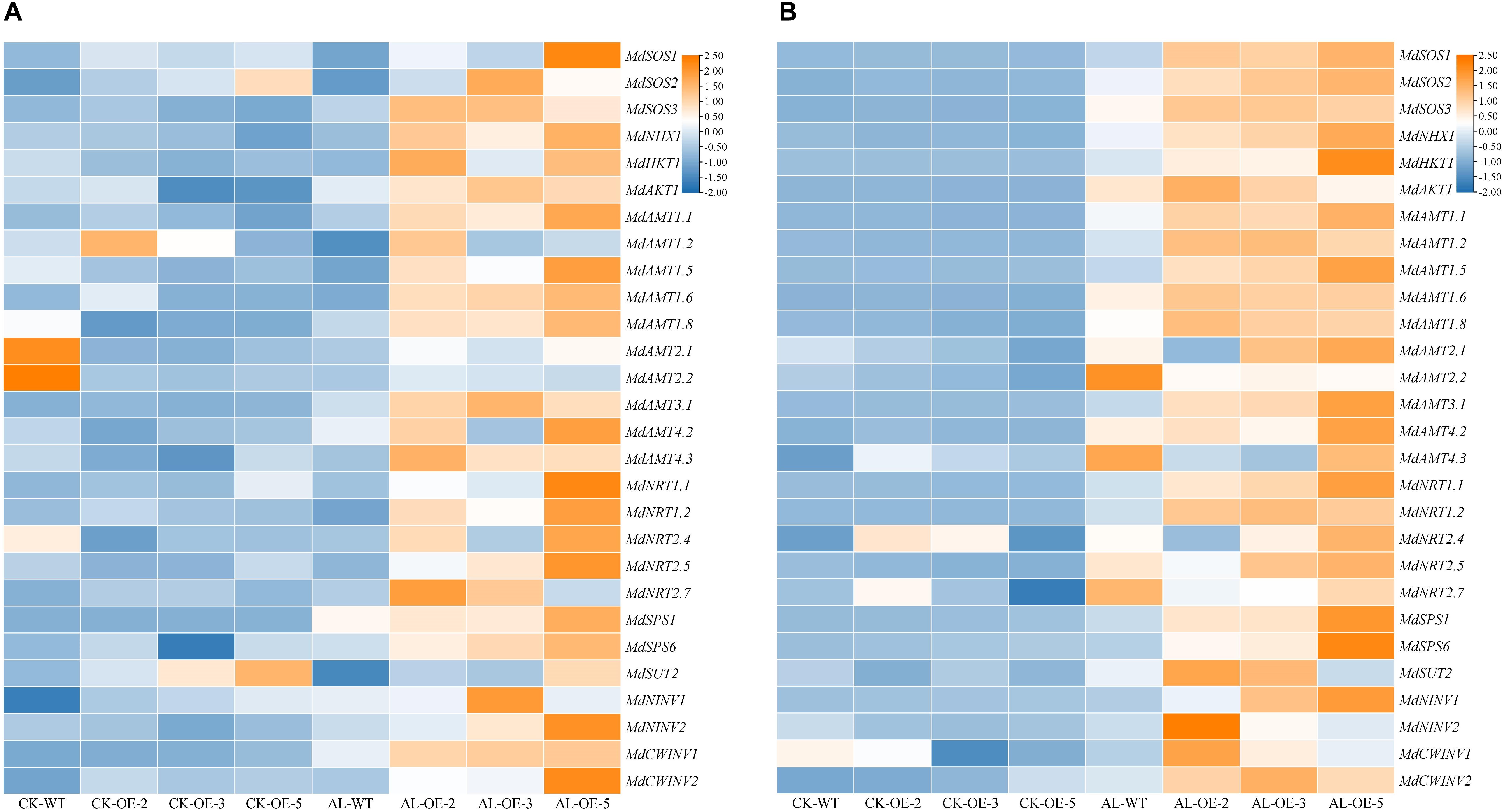
Figure 8. Heatmap presenting expression levels of 6 ion-transport-related genes (MdSOS1, MdSOS2, MdSOS3, MdNHX1, MdHKT1, and MdAKT1), 15 N-absorption-related genes (MdAMT1.1, MdAMT1.2, MdAMT1.5, MdAMT1.6, MdAMT1.8, MdAMT2.1, MdAMT2.2, MdAMT3.1, MdAMT4.2, MdAMT4.3, MdNRT1.1, MdNRT1.2, MdNRT2.4, MdNRT2.5, and MdNRT2.7) and 7 C-metabolism-related genes (MdSPS1, MdSPS6, MdSUT2, MdNINV1, MdNINV2, MdCWINV1 and MdCWINV2) in (A) leaves and (B) roots of MdTyDc-overexpressing apple plants after 15 days under control and alkaline conditions. The data are presented as means ± SD (n = 3). Significant differences between WT and MdTyDc overexpression lines are indicated by different lowercase letters based on Tukey’s multi-range test (P < 0.05). WT, wild type. OE-2, MdTyDc overexpression line 2. OE-3, MdTyDc overexpression line 3. OE-5, MdTyDc overexpression line 5.
In leaves (Figure 8A), the expression of genes from the SOS signaling system (e.g., MdSOS1, MdSOS2, MdSOS3, and MdNHX1) was higher in at least two OE lines compared with WT plants under alkaline stress. The expression patterns of other ion-transport-related genes such as MdHKT1 and MdAKT1 were similar to those of MdSOS3. Moreover, with the exceptions of MdAMT1.2, MdAMT2.1, and MdAMT2.2, the transcript levels of other N-absorption-related genes (i.e., MdAMT1.1, MdAMT1.5, MdAMT1.6, MdAMT1.8, MdAMT3.1, MdAMT4.2, MdAMT4.3, MdNRT1.1, MdNRT1.2, MdNRT2.4, MdNRT2.5, and MdNRT2.7) were higher in OE lines than in WT lines after 15 days of alkaline stress. In addition, under alkaline stress, the expression of genes involed in C metabolism (e.g., MdSPS1, MdSPS6, MdNINV2, and MdCWINV1) was higher in OE plants than that in WT plants.
In roots (Figure 8B), the transcript levels of all ion-transport-related genes and N-absorption-related genes were up-regulated in WT plants under alkaline stress compared with normal growth conditions. With the exceptions of MdAMT2.2, MdAMT4.2, MdAMT4.3, MdNRT2.4, and MdNRT2.7, whose expression was lower in at least two OE lines than in WT lines after alkaline treatment, the other measured genes were all significantly upregulated in at least two OE lines. Furthermore, the MdSPS1, MdSPS6, MdSUT2, MdNINV1, MdNINV2, MdCWINV1, and MdCWINV2 genes in at least two OE plants were more strongly induced by alkaline stress than those in WT.
Discussion
Tyrosine decarboxylase (EC 4.1.1.25) is a 5’-pyridoxal phosphate (PLP)-dependent decarboxylase. Many studies have shown that it plays an active role in plant growth and development (Kang et al., 2007), secondary metabolite synthesis (Lan et al., 2013), and resistance to biotic and abiotic stresses (Landtag et al., 2002; Wang et al., 2020). Tyrosine is decarboxylated to tyramine by TYDC and then hydroxylated to dopamine, which is involved in plant response to salt stress (Li et al., 2015; Gao et al., 2020a), drought stress (Gao et al., 2020b), and low N stress (Liu et al., 2020). However, little is known about the function of MdTyDc in response to alkaline stress in plants. We found that MdTyDc expression was induced by alkaline stress (Figure 1A) and analyzed its role in the alkaline resistance mechanisms of apple using a hydroponic alkaline treatment.
As found previously, the inhibitory effect of alkaline stress on apple seedlings was significantly alleviated by the application of exogenous dopamine (Jiao et al., 2019). Here, we found that MdTyDc overexpressing apple lines, which showed higher dopamine content under both normal and alkaline conditions, exhibited enhanced alkalinity tolerance (Figures 1C–E and Table 1). For example, compared with WT plants under normal conditions, WT and OE plants grown under alkaline stress showed notable reductions in all growth parameters (shoot height, leaf number, fresh weight, dry weight, and RGR) (Table 1). However, the overexpression of MdTyDc clearly mitigated these declines. The root-shoot ratio of plants tends to increase in adverse environments, representing plants’ self-adaptation to the environment (Xia et al., 2010). We observed that MdTyDc overexpressing plants grown under alkaline stress had RSRs closer to those observed under normal conditions (Table 1). These findings indicate that the overexpression of MdTyDc may increase the dopamine content, improving plant growth and reducing the damage caused by alkaline stress.
The root system is a vital plant part that directly senses adversity signals, and measuring root system architecture is an effective way to quantify root development (Zhao et al., 2016). Alkaline stress can cause root growth retardation and directly or indirectly inhibit aboveground growth (Hu et al., 2012; Fan et al., 2015). Our experiment confirmed previous research in which almost all root system indices were seriously affected by alkaline stress (Table 2). Nevertheless, overexpression of MdTyDc could alleviate this inhibitory effect (Table 2). We also found that root activity was higher in OE plants than in WT plants (Figure 2A); root activity also reflects root growth and directly affects plant stress tolerance (Liu et al., 2019b). We therefore concluded that the root systems of plants that overexpressed MdTyDc showed better development under alkaline stress.
Numerous studies have shown that plant photosynthetic ability decreases under alkaline stress and that this decrease is proportional to the degree of stress (Liu et al., 2010; Liu and Shi, 2010). Here, Pn, Gs, and Tr of all genotypes decreased significantly from the 5th day of alkaline stress treatment, but this decrease was not significant in the three OE lines (Figures 3B,F,H). Measurements of Ci showed the opposite trend (Figure 3D), and this result suggests that the photosynthetic decline was due mainly to non-stomatal limitation (Huo et al., 2020). Furthermore, the decrease in photosynthetic ability caused by alkaline stress directly affects the photosynthetic apparatus by reducing chlorophyll content and Fv/Fm (Li et al., 2020a). Chlorophyll fluorescence, which can be quantified by Fv/Fm, reflects the true photosynthetic behavior of whole plants under adversity (Baker, 2008; Huang et al., 2020). Successive measurements during alkaline treatment showed that OE lines had higher chlorophyll contents and Fv/Fm values than WT lines under stress conditions (Figure 4). Many studies have shown that dopamine has a significant role in the regulation of oxygen reduction and the promotion of energy conversion during photosynthesis (Kanazawa and Sakakibara, 2000). Dopamine can also mitigate salt-induced photosynthetic limitations directly by reducing non-stomatal limitations associated with photosynthetic metabolism or indirectly by alleviating oxidative stress (Li et al., 2015). Considering the dramatic changes in photosynthesis on the fifth day after alkaline stress treatment, we hypothesized that there might be a significant change in dopamine content at this time. Therefore, we measured dopamine content in leaves and roots of apple plants after 5 days of alkaline stress treatment. Consistent with the study of Wang et al. (2020), dopamine content increased when MdTyDc was overexpressed in apples (Figures 1D,E and Supplementary Figure S1). In addition, dopamine content in OE plants under alkaline stress was higher than that under normal conditions. We therefore speculated that the overexpression of MdTyDc promoted the synthesis of dopamine, thereby alleviating damage to the photosynthetic system caused by alkaline stress in apple.
Guo et al. (2015b) found that the effect of alkaline stress on plant membranes was mainly manifested by an increase in REL. Therefore, we measured leaf REL and discovered that the overexpression of MdTyDc contributed to lower REL and maintained appropriate cell membrane permeability in apple under alkaline stress (Figure 2B). Proline, a compatible osmolyte present in the cytoplasm, can protect macromolecules and scavenge free radicals (Gong et al., 2014). Our research showed that MdTyDc overexpression promoted the accumulation of proline under alkaline stress (Figures 2C,D). This is consistent with previous research in which improved plant osmotic regulation helped to prevent the damage associated with alkaline stress (Ashraf and Foolad, 2007).
Excess Na+ accumulation under alkaline stress can damage membrane structure and alter membrane function, thereby increasing plasma membrane permeability and causing the exosmosis of intracellular potassium, phosphorus, and organic solutes (Tuna et al., 2007). Numerous studies have shown that changes in Na+ and K+ concentrations are a physiological means by which plants respond to alkaline stress (Shuyskaya et al., 2014; Abbas and Mobin, 2016). Here, apple plants that overexpressed MdTyDc maintained lower leaf, stem, and root Na+/K+ ratios than WT plants under alkaline stress (Figure 5). Therefore, plants under alkaline stress can effectively control the absorption and transport of Na+ and K+ and maintain Na+/K+ balance, which is important for adaptation to alkaline stress (Guo et al., 2016; Gresh et al., 2017). It has been reported that a sudden increase in Na+ in plant tissues under alkaline stress may also be related to the interference with the SOS signaling system (Türkan and Demiral, 2009). We found that the expression of SOS signal system genes (MdSOS1, MdSOS2, MdSOS3, and MdNHX1) was upregulated by MdTyDc overexpression under alkaline conditions (Figure 8). We also analyzed the expression of genes encoding potassium ion transporters or channels (MdHKT1 and MdAKT1) and found that overexpression of MdTyDc upregulated their expression under alkaline stress (Figure 8). Previously, Li et al. (2015) found that dopamine regulated the expression of MdSOS1, MdSOS2, MdSOS3, MdNHX1, and MdHKT1 under salt stress, helping to maintain a high K+/Na+ ratio and alleviating the damage caused by salt stress. Because Na+–K+ equilibrium is thought to be the ultimate manifestation of plant resistance to alkalinity (Qi and Spalding, 2004; Song et al., 2015; Wang et al., 2015b; Zhang et al., 2015), we hypothesized that MdTyDc overexpression would help to maintain a normal Na+/K+ ratio in cells by regulating the expression of genes related to Na+ and K+ transport.
The maintenance of intracellular ion balance and pH stability are necessary to ensure the normal progress of various metabolic processes (Yang et al., 2007). Here, we observed that more Cl– and SO42– accumulated in OE lines than in WT lines under alkaline stress (Figures 6A–H). As previous studies have shown, plants absorb inorganic anions such as Cl– and SO42– to balance the accumulation of excess cations under alkaline stress, thereby maintaining a stable pH (Ghoulam et al., 2002; Yang et al., 2007). Our results suggest that MdTyDc plays a role in plant ion balance and osmotic regulation.
Previous studies have shown that an adequate N supply helps to compensate for plant nutrient imbalances caused by alkaline stress (Ehlting et al., 2007; Wang et al., 2012a). The growth rate of trees leveled out when soil nitrogen concentration was highest (Vestin et al., 2013). Yang et al. (2007) showed that NO3– absorption by an herbaceous halophyte was reduced due to alkaline stress, and we demonstrated that MdTyDc overexpression significantly alleviated reduced N content in leaves and roots (Figures 7A–D). Decreased N content under alkaline stress may be due to inhibition of N absorption and ammonium assimilation (Guo et al., 2015b). We therefore examined the activities of N metabolism enzymes and found that MdTyDc overexpression noticeably increased the activities of NR, NiR, GS, and GOGAT in leaves and roots (Figures 7E–L). Plant N uptake depends on the activity of corresponding carrier proteins (Munns and Tester, 2008). We measured transcript levels of genes related to N absorption and found that almost all N metabolism genes were induced by alkaline treatment in the roots of all genotypes (Figure 8B). This is consistent with the findings of Wang et al. (2012b), in which the OsAMT and OsNRT gene families played an important role in nitrate accumulation in old and new rice leaves under alkaline stress. Moreover, the expression of those genes was higher in plants that overexpressed MdTyDc than in WT (Figure 8). Taken together, our results suggest that MdTyDc overexpression increases the activities of enzymes related to nitrogen metabolism by regulating genes associated with N absorption and assimilation, thereby improving plant N content under alkaline stress.
It is well known that plants can accumulate and synthesize compatible small molecules such as sugars, alcohols, quaternary amines, proline and betaine in response to osmotic stress during saline-alkali stress (Flowers and Colmer, 2008). Coincidentally, previous studies have shown that overexpression of dopamine synthesizing genes in potatoes increases soluble sugar content (Swiedrych et al., 2004). The overexpression of dopamine receptors in potatoes increases the content of catecholamines, along with the content of sucrose, glucose, and fructose (Skirycz et al., 2005). In plants, sucrose can be converted to fructose and glucose by sucrose phosphate synthase (SPS), cell wall invertase (CWINV), and neutral invertase (NINV) (Diana et al., 2008; Hirose et al., 2008). Therefore, we examined the expression levels of several carbon metabolism-related genes (MdSPS1, MdSPS6, MdSUT2, MdNINV1, MdNINV2, MdCWINV1, and MdCWINV2) in leaves and roots. We found that the expression of MdSPS1and MdSPS6 in the leaves and roots of OE plants were more strongly induced by alkaline stress than those in WT (Figure 8). Therefore, we hypothesized that MdTyDc overexpression may increase glucose and fructose synthesis by increasing the expression of MdSPS1/6 thus improving the osmotic regulation ability of plants to resist alkali stress.
In conclusion, our study demonstrated that apple plants overexpressing MdTyDc showed enhanced tolerance to alkaline stress. As shown in Figure 9, the exposure of MdTyDc overexpressing apple lines to alkaline stress led to higher dopamine content, which appeared to enhance photosynthetic capacity and maintain intracellular ion homeostasis. In addition, overexpression of MdTyDc promoted root development and increased the activities of enzymes related to N metabolism, which may have contributed to better plant N absorption under alkaline stress. Our study provides evidence for dopamine mediation of alkalinity tolerance and has important applications for promoting the growth of horticultural crops in saline and alkaline soils.
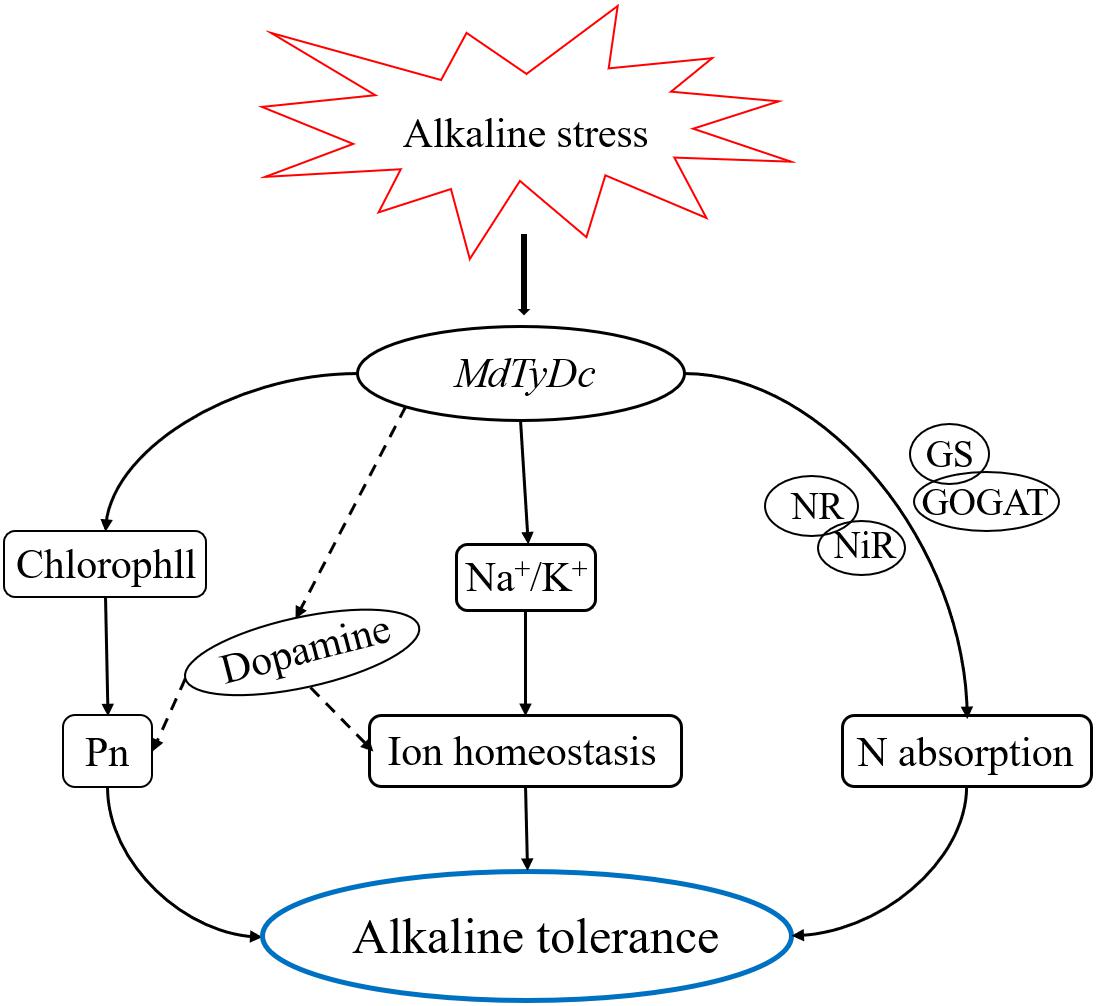
Figure 9. A model to explain the regulatory effect of MdTyDc on apple’s alkaline stress response. Under alkaline stress, apple lines overexpressing MdTyDc showed increased photosynthetic pigment content and inhibited the increase of sodium ions, thus the photosynthetic capacity was enhanced and intracellular ion homeostasis was maintained, which may be realized by increasing dopamine content; In addition, overexpression of MdTyDc also improved the enzyme activity related to nitrogen metabolism, thus promoting nitrogen absorption. Therefore, the alkaline tolerance of transgenic apple plants was increased.
Data Availability Statement
The original contributions presented in the study are included in the article/Supplementary Material, further inquiries can be directed to the corresponding author/s.
Author Contributions
XL contributed to the study conception and performed most of the experiments. YJ and KT prepared the materials and obtained the experimental data. JZ, TG, ZZ, and YZ analyzed the data. FM contributed to the study design. CL provided all financial support, critical intellectual input in study design, and manuscript preparation. XL wrote the first draft of the manuscript. All authors commented on previous versions of the manuscript, read and approved the final manuscript.
Funding
This work was supported by the National Key Research and Development Program of China (2018YFD1000303), the National Natural Science Foundation of China (31972389), Tang Scholar, and the China Postdoctoral Science Foundation (2017M613224).
Conflict of Interest
The authors declare that the research was conducted in the absence of any commercial or financial relationships that could be construed as a potential conflict of interest.
Acknowledgments
We are grateful to Dr. Zhihong Zhang of Shenyang Agricultural University for his donation of the “Gala” apple (M. domestica cv. Gala) plants.
Supplementary Material
The Supplementary Material for this article can be found online at: https://www.frontiersin.org/articles/10.3389/fpls.2021.625890/full#supplementary-material
Supplementary Figure | Dopamine content of MdTyDc-overexpressing apple plants after 5 days under control and alkaline conditions. (A) Dopamine content in leaves and (B) dopamine content in roots. The data are presented as means ± SD (n = 3). Significant differences between WT and MdTyDc overexpression lines are indicated by different lowercase letters based on Tukey’s multi-range test (P < 0.05). WT, wild type. OE-2, MdTyDc overexpression line 2. OE-3, MdTyDc overexpression line 3. OE-5, MdTyDc overexpression line 5.
References
Abbas, Z. K., and Mobin, M. (2016). Comparative growth and physiological responses of two wheat (Triticum aestivum L.) cultivars differing in salt tolerance to salinity and cyclic drought stress. Arch. Agron. Soil Sci. 62, 745–758. doi: 10.1080/03650340.2015.1083553
Arnon, D. I. (1949). Copper enzymes in isolated chloroplasts. Polyphenoloxidase in beta vulgaris. Plant Physiol. 24, 1–15. doi: 10.1104/pp.24.1.1
Ashraf, M., and Foolad, M. R. (2007). Roles of glycine betaine and proline in improving plant abiotic stress resistance. Environ. Exp. Bot. 59, 206–216. doi: 10.1016/j.envexpbot.2005.12.006
Baker, N. R. (2008). Chlorophyll fluorescence, a probe of photosynthesis in vivo. Annu. Rev. Plant Biol. 59, 89–113. doi: 10.1146/annurev.arplant.59.032607.092759
Bloom, A. J. (2015). The increasing importance of distinguishing among plant nitrogen sources. Curr. Opin. Plant Biol. 25, 10–16. doi: 10.1016/j.pbi.2015.03.002
Botella, M. A., Martínezb, V., Nieves, M., and Cerdáa, A. (1997). Effect of salinity on the growth and nitrogen uptake by wheat seedlings. J. Plant Nutr. 20, 793–804. doi: 10.1080/01904169709365295
Britto, D. T., and Kronzucker, H. J. (2002). NH4+ toxicity in higher plants: a critical review. J. Plant Physiol. 159, 567–584. doi: 10.1078/0176-1617-0774
Chopin, F., Orsel, M., Dorbe, M. F., Chardon, F., Truong, H. N., Miller, A. J., et al. (2007). The Arabidopsis ATNRT2.7 nitrate transporter controls nitrate content in seeds. Plant Cell Online 19, 1590–1602. doi: 10.1105/tpc.107.050542
Couturier, J., Montanini, B., Martin, F., Brun, A., Blaudez, D., and Chalot, M. (2007). The expanded family of ammonium transporters in the perennial poplar plant. N. Phytol. 174, 137–150. doi: 10.1111/j.1469-8137.2007.01992.x
Dai, W. S., Wang, M., Gong, X. Q., and Liu, J. H. (2018). The transcription factor FcWRKY40 of Fortunella crassifolia functions positively in salt tolerance through modulation of ion homeostasis and proline biosynthesis by directly regulating SOS2 and P5CS1 homologs. N. Phytol. 219, 972–989. doi: 10.1111/nph.15240
Diana, P., Giampiero, C., Cecilia, D. C., Claudia, F., Willemse, M. T. M., and Mauro, C. (2008). Sucrose synthase is associated with the cell wall of tobacco pollen tubes. Plant Physiol. 147, 1603–1618. doi: 10.1104/pp.108.115956
Dionisio-Sese, M. L., and Tobita, S. (1998). Antioxidant responses of rice seedings to salinity stress. Plant Sci. 135, 1–9. doi: 10.1016/s0168-9452(98)00025-9
Ehlting, B., Dluzniewska, P., Dietrich, H., Selle, A., Teuber, M., Hansch, R., et al. (2007). Interaction of nitrogen nutrition and salinity in Grey poplar (Populus tremula×alba). Plant Cell Environ. 30, 796–811. doi: 10.1111/j.1365-3040.2007.01668.x
ElMahi, H., Perez-Hormaeche, J., DeLuca, A., Villalta, I., Espartero, J., Gamez-Arjona, F., et al. (2019). A critical role of sodium flux via the plasma membrane Na+/H+ exchanger SOS1 in the salt tolerance of rice. Plant Physiol. 180, 1046–1065. doi: 10.1104/pp.19.00324
Fan, J. W., Du, Y. L., Turner, N. C., Wang, B. R., Fang, Y., Xi, Y., et al. (2015). Changes in root morphology and physiology to limited phosphorus and moisture in a locally-selected cultivar and an introduced cultivar of Medicago sativa L. growing in alkaline soil. Plant Soil 392, 215–226. doi: 10.1007/s11104-015-2454-0
Flowers, T. J., and Colmer, T. D. (2008). Salinity tolerance in halophytes. N. Phytol. 179, 945–963. doi: 10.1111/j.1469-8137.2008.02531.x
Fujiyama, H. (2005). Characteristics of nitrate uptake by plants under salinity. J. Plant Nutr. 28, 33–46. doi: 10.1081/pln-200042156
Gao, T. T., Liu, X. M., Shan, L., Wu, Q., and Li, C. (2020a). Dopamine and arbuscular mycorrhizal fungi act synergistically to promote apple growth under salt stress. Environ. Exp. Bot. 178:104159. doi: 10.1016/j.envexpbot.2020.104159
Gao, T. T., Zhang, Z. J., Liu, X. M., Wu, Q., Chen, Q., Liu, Q. W., et al. (2020b). Physiological and transcriptome analyses of the effects of exogenous dopamine on drought tolerance in apple. Plant Physiol. Biochem. 148, 260–272. doi: 10.1016/j.plaphy.2020.01.022
Gazzarrini, S., Lejay, L., Gojon, A., Ninnemann, O., Frommer, W. B., and Wirén, V. (1999). Three functional transporters for constitutive, diurnally regulated, and starvation-induced uptake of ammonium into Arabidopsis roots. Plant Cell 11, 937–947. doi: 10.2307/3870826
Ghoulam, C., Foursy, A., and Fares, K. (2002). Effects of salt stress on growth, inorganic ions and proline accumulation in relation to osmotic adjustment in five sugar beet cultivars. Environ. Exp. Bot. 47, 39–50. doi: 10.1016/s0098-8472(01)00109-5
Gong, B., Zhang, C., and Li, X. (2014). Identification of NaCl and NaHCO3 stress responsive proteins in tomato roots using iTRAQ based analysis. Biochem. Biophys. Res. Commun. 446, 417–422. doi: 10.1016/j.bbrc.2014.03.005
Gorbe, E., and Calatayud, A. (2012). Applications of chlorophyll fluorescence imaging technique in horticultural research: A review. Sci. Hortic. 138, 24–35. doi: 10.1016/j.scienta.2012.02.002
Gresh, N., Naseemkhan, S., Lagardère, L., Piquemal, J. P., Sponer, J. E., and Sponer, J. (2017). Channeling through two stacked guanine quartets of one and two alkali cations in the Li+, Na+, K+ and Rb+ series. Assessment of the accuracy of the SIBFA anisotropic polarizable molecular mechanics potential. J. Phys. Chem. B 121, 3997–4014. doi: 10.1021/acs.jpcb.7b01836
Guo, C. Y., Wang, X. Z., Chen, L., Ma, L. N., and Wang, R. Z. (2015a). Physiological and biochemical responses to saline-alkaline stress in two halophytic grass species with different photosynthetic pathways. Photosynthetica 53, 128–135. doi: 10.1007/s11099-015-0094-5
Guo, L. Q., Shi, D. C., and Wang, D. L. (2010). The key physiological response to alkali stress by the alkali-resistant halophyte Puccinellia tenuiflora is the accumulation of large quantities of organic acids and into the rhyzosphere. J. Agron. Crop Sci. 196, 123–135. doi: 10.1111/j.1439-037x.2009.00397.x
Guo, R., Feng, L. I., Zhou, J., Li, H. R., Xia, X., and Liu, Q. (2016). Ecophysiological responses of linseed (Linum usitatissimum) to salt and alkali stresses. Chin. J. Plant Ecol. 40, 69–79.
Guo, R., Yang, Z., Li, F., Yan, C., Zhong, X., Liu, Q., et al. (2015b). Comparative metabolic responses and adaptive strategies of wheat (Triticum aestivum) to salt and alkali stress. BMC Plant Biol. 15:170. doi: 10.1186/s12870-015-0546-x
Hirose, T., Scofield, G. N., and Terao, T. (2008). An expression analysis profile for the entire sucrose synthase gene family in rice. Plant Sci. 174, 534–543. doi: 10.1016/j.plantsci.2008.02.009
Högberg, P., Granström, A., Johansson, T., Lundmark-Thelin, A., and Näsholm, T. (1986). Plant nitrate reductase activity as an indicator of availability of nitrate in forest soils. Ottawa: NRC Research Press.
Hu, X. H., Zhang, Y., Shi, Y., Zhang, Z., Zou, Z. R., Zhang, H., et al. (2012). Effect of exogenous spermidine on polyamine content and metabolism in tomato exposed to salinity-alkalinity mixed stress. Plant Physiol. Biochem. 57, 200–209. doi: 10.1016/j.plaphy.2012.05.015
Huang, D., Wang, Q., Jing, G. Q., Ma, M. N., Li, C., and Ma, F. W. (2020). Overexpression of MdIAA24 improves apple drought resistance by positively regulating strigolactone biosynthesis and mycorrhization. Tree Physiol. 41, 134–146.
Huang, L. L., Li, M. J., Yun, S., Sun, T. T., and Li, C. Y. (2018). Ammonium uptake increases in response to PEG-induced drought stress in Malus hupehensis Rehd. Environ. Exp. Bot. 151, 32–42. doi: 10.1016/j.envexpbot.2018.04.007
Huo, L. Q., Sun, X., Guo, Z. J., Jia, X., Che, R. M., Sun, Y. M., et al. (2020). MdATG18a overexpression improves basal thermotolerance in transgenic apple by decreasing damage to chloroplasts. Hortic. Res. Engl. 7:21.
Jiao, X. Y., Li, Y. X., Zhang, X. Z., Liu, C. L., Liang, W., Li, C., et al. (2019). Exogenous dopamine application promotes alkali tolerance of apple seedlings. Plants 8:580. doi: 10.3390/plants8120580
Jin, X. Q., Liu, T., Xu, J. J., Gao, Z. X., and Hu, X. H. (2019). Exogenous GABA enhances muskmelon tolerance to salinity-alkalinity stress by regulating redox balance and chlorophyll biosynthesis. BMC Plant Biol. 1:19. doi: 10.1186/s12870-019-1660-y
Joseph, A. B., David, J. B., and Peter, J. F. (2010). Stomata: key players in the earth system, past and present. Curr. Opin. Plant Biol. 13, 232–239. doi: 10.1016/j.pbi.2010.04.013
Kanazawa, K., and Sakakibara, H. (2000). High content of dopamine, a strong antioxidant, in cavendish banana. J. Agric. Food Chem. 48, 844–848. doi: 10.1021/jf9909860
Kang, S., Kang, K., Lee, K., and Back, K. (2007). Characterization of rice tryptophan decarboxylases and their direct involvement in serotonin biosynthesis in transgenic rice. Planta 227, 263–272. doi: 10.1007/s00425-007-0614-z
Kiba, T., Feria-Bourrellier, A. B., Lafouge, F., Lezhneva, L., Boutet-Mercey, S., Orsel, M., et al. (2012). The Arabidopsis nitrate transporter NRT2.4 plays a double role in roots and shoots of nitrogen-straved plants. Plant Cell 24, 245–258. doi: 10.1105/tpc.111.092221
Kong, K. H., Lee, J. L., Park, H. J., and Cho, S. H. (1998). Purification and characterization of the tyrosinase isozymes of pine needles. IUBMB Life 45, 717–724. doi: 10.1080/15216549800203122
Lan, X., Chang, K., Zeng, L., Liu, X., Qiu, F., Zheng, W. L., et al. (2013). Engineering salidroside biosynthetic pathway in hairy root cultures of rhodiola crenulata based on metabolic characterization of tyrosine decarboxylase. PLoS One 8:e75459. doi: 10.1371/journal.pone.0075459
Landtag, J., Baumert, A., Degenkolb, T., Schmidt, J., Wray, V., Scheel, D., et al. (2002). Accumulation of tyrosol glucoside in transgenic potato plants expressing a parsley tyrosine decarboxylase. Phytochemistry 60, 683–689. doi: 10.1016/s0031-9422(02)00161-9
Lehmann, T., and Pollmann, S. (2009). Gene expression and characterization of a stress-induced tyrosine decarboxylase from Arabidopsis thaliana. FEBS Lett. 583, 1895–1900. doi: 10.1016/j.febslet.2009.05.017
Lezhneva, L., Kiba, T., Feriabourrelier, A. B., Lafouge, F., Boutetmercey, S., Zoufan, P., et al. (2015). The Arabidopsis nitrate transporter NRT2.5 plays a role in nitrate acquisition and remobilization in nitrogen-starved plants. Plant J. 80, 230–241. doi: 10.1111/tpj.12626
Li, C., Sun, X. K., Chang, C., Jia, D. F., Wei, Z. W., Li, C. Y., et al. (2015). Dopamine alleviates salt-induced stress in Malus hupehensis. Physiol. Plant. 153, 584–602. doi: 10.1111/ppl.12264
Li, C., Wang, P., Wei, Z. W., Liang, D., and Ma, F. W. (2012). The mitigation effects of exogenous melatonin on salinity-induced stress in Malus hupehensis. J. Pineal Res. 53, 298–306. doi: 10.1111/j.1600-079x.2012.00999.x
Li, Y. X., Liu, B. Y., Peng, Y. X., Liu, C. L., Zhang, X. Z., Zhang, Z. J., et al. (2020a). Exogenous GABA alleviates alkaline stress in Malus hupehensis by regulating the accumulation of organic acids. Sci. Hortic. 261:108982. doi: 10.1016/j.scienta.2019.108982
Li, Y. X., Liu, C. L., Sun, X., Liu, B. Y., Zhang, X. Z., Liang, W., et al. (2020b). Overexpression of MdATG18a enhances alkaline tolerance and GABA shunt in apple through increased autophagy under alkaline conditions. Tree Physiol. 40, 1509–1519. doi: 10.1093/treephys/tpaa075
Liang, B. W., Li, C. Y., Ma, C. Q., Wei, Z. W., Wang, Q., Huang, D., et al. (2017). Dopamine alleviates nutrient deficiency-induced stress in Malus hupehensis. Plant Physiol. Biochem. 119, 346–359. doi: 10.1016/j.plaphy.2017.09.012
Lin, C. C., and Kao, C. H. (1996). Disturbed ammonium assimilation is associated with growth inhibition of roots in rice seedlings caused by NaCl. Plant Growth Regul. 18, 233–238. doi: 10.1007/bf00024387
Liu, J., and Shi, D. C. (2010). Photosynthesis, chlorophyll fluorescence, inorganic ion and organic acid accumulations of sunflower in responses to salt and salt-alkaline mixed stress. Photosynthetica 48, 127–134. doi: 10.1007/s11099-010-0017-4
Liu, J., Guo, W. Q., and Shi, D. C. (2010). Seed germination, seedling survival and physiological response of sunflowers under saline and alkaline conditions. Photosynthetica 48, 278–286. doi: 10.1007/s11099-010-0034-3
Liu, X. L., Zhang, H., Jin, Y. Y., Wang, M. M., Yang, H. Y., Ma, H. Y., et al. (2019a). Abscisic acid primes rice seedlings for enhanced tolerance to alkaline stress by upregulating antioxidant defense and stress tolerance-related genes. Plant Soil 438, 39–55. doi: 10.1007/s11104-019-03992-4
Liu, X. M., Gao, T. T., Zhang, Z. J., Tan, K. X., Jin, Y. B., Zhao, Y. J., et al. (2020). The mitigation effects of exogenous dopamine on low nitrogen stress in Malus hupehensis. J. Integr. Agric. 19, 2709–2724. doi: 10.1016/s2095-3119(20)63344-5
Liu, X., Liang, W., Li, Y. X., Li, M. J., Ma, B. Q., Liu, C. H., et al. (2019b). Transcriptome analysis reveals the effects of alkali stress on root system architecture and endogenous hormones in apple rootstocks. J. Integr. Agric. 18, 2264–2271. doi: 10.1016/s2095-3119(19)62706-1
Luo, J., Li, H., Liu, T. X., Polle, A., Peng, C. H., and Luo, Z. B. (2013). Nitrogen metabolism of two contrasting poplar species during acclimation to limiting nitrogen availability. J. Exp. Bot. 64, 4207–4224. doi: 10.1093/jxb/ert234
Munns, R., and Tester, M. (2008). Mechanisms of salinity tolerance. Annu. Rev. Plant Biol. 59, 651–681.
Nagatsu, I., Sudo, Y., and Nagatsu, T. (1972). Tyrosine hydroxylation in the banana plant. Enzymologia 43, 25–31.
Orsel, M., Chopin, F., Leleu, O., Smith, S. J., Krapp, A., Daniel-Vedele, F., et al. (2007). Nitrate signaling and the two component high affinity uptake system in Arabidopsis. Plant Signal. Behav. 2, 260–262. doi: 10.4161/psb.2.4.3870
Perini, P., Pasquali, G., Margis-Pinheiro, M., De Oliviera, P. R. D., and Revers, L. F. (2014). Reference genes for transcriptional analysis of flowering and fruit ripening stages in apple (Malus × domestica Borkh.). Mol. Breed. 34, 829–842. doi: 10.1007/s11032-014-0078-3
Qi, Z., and Spalding, E. P. (2004). Protection of plasma membrane K+ transport by the salt overly sensitive1 Na+-H+ antiporter during salinity stress. Plant Physiol. 136:3849.
Rozema, J., and Flowers, T. J. (2008). Crops for a salinized world. Science 322, 1478–1480. doi: 10.1126/science.1168572
Seith, B., Setzer, B., Flaig, H., and Mohr, H. (1994). Appearance of nitrate reductase, nitrite reductase and glutamine synthetase in different organs of the Scots pine (Pinus sylvestris) seedling as affected by light, nitrate and ammonium. Physiol. Plant. 91, 419–426. doi: 10.1034/j.1399-3054.1994.910310.x
Shuyskaya, E. V., Li, E. V., Rahmankulova, Z. F., Kuznetsova, N. A., Toderich, K. N., and Voronin, P. Y. (2014). Morphophysiological adaptation aspects of different Haloxylon aphyllum (Chenopodiaceae) genotypes along a salinity gradient. Russ. J. Ecol. 45, 181–187. doi: 10.1134/s1067413614030114
Skirycz, A., Widrych, A., and Szopa, J. (2005). Expression of human dopamine receptor in potato (Solanum tuberosum) results in altered tuber carbon metabolism. BMC Plant Biol. 5:1. doi: 10.1186/1471-2229-5-1
Sohlenkamp, C., Shelden, M., Howitt, S., and Udvardi, M. (2000). Characterization of Arabidopsis AtAMT2, a novel ammonium transporter in plants. Febs Lett. 467, 273–278. doi: 10.1016/s0014-5793(00)01153-4
Sohlenkamp, C., Wood, C. C., Roeb, G. W., and Udvardi, M. K. (2002). Characterization of Arabidopsis AtAMT2, a high-affinity ammonium transporter of the plasma membrane. Plant Physiol. 130, 1788–1796. doi: 10.1104/pp.008599
Song, M. L., Chai, Q., Li, X. Z., Yao, X., Li, C. J., Christensen, M. J., et al. (2015). An asexual Epichloë, endophyte modifies the nutrient stoichiometry of wild barley (Hordeum brevisubulatum) under salt stress. Plant Soil 387, 153–165. doi: 10.1007/s11104-014-2289-0
Sun, M., Jia, B., Cui, N., Wen, Y. D., Duanmu, H. Z., Yu, Q. Y., et al. (2016). Functional characterization of a Glycine soja Ca2+ ATPase in salt-alkaline stress responses. Plant Mol. Biol. 90, 419–434. doi: 10.1007/s11103-015-0426-7
Swiedrych, A., Stachowiak, J., and Szopa, J. (2004). The catecholamine potentiates starch mobilization in transgenic potato tubers. Plant Physiol. Biochem. 42, 103–109. doi: 10.1016/j.plaphy.2003.11.002
Tuna, A. L., Kaya, C., Ashraf, M., Altunlu, H., Yokas, I., and Yagmur, B. (2007). The effects of calcium sulphate on growth, membrane stability and nutrient uptake of tomato plants grown under salt stress. Environ. Exp. Bot. 59, 173–178. doi: 10.1016/j.envexpbot.2005.12.007
Türkan, I., and Demiral, T. (2009). Recent developments in understanding salinity tolerance. Environ. Exp. Bot. 67, 2–9. doi: 10.1016/j.envexpbot.2009.05.008
Vestin, J. L. K., Söderberg, U., Bylund, D., Nambu, K., van Hees, P. A. W., Haslinger, E., et al. (2013). The influence of alkaline and non-alkaline parent material on Norway spruce tree chemical composition and growth rate. Plant Soil 370, 103–113. doi: 10.1007/s11104-013-1615-2
Wang, H., Ahan, J., Wu, Z. H., Shi, D. C., Liu, B., and Yang, C. W. (2012a). Alteration of nitrogen metabolism in rice variety ‘Nipponbare’ induced by alkali stress. Plant Soil 355, 131–147. doi: 10.1007/s11104-011-1086-2
Wang, H., Wu, Z. H., Han, J. Y., and Yang, C. W. (2012b). Comparison of ion balance and nitrogen metabolism in old and young leaves of alkali-stressed rice plants. PLoS One 7:e37817. doi: 10.1371/journal.pone.0037817
Wang, L. X., Fang, C., and Wang, K. (2015a). Physiological responses of to long-term salt, alkali and mixed salt-alkali stresses. J. Plant Nutr. 38, 526–540. doi: 10.1080/01904167.2014.937874
Wang, L., Zhou, Q., Ding, L., and Sun, Y. (2008). Effect of cadmium toxicity on nitrogen metabolism in leaves of Solanum nigrum L. as a newly found cadmium hyperaccumulator. J. Hazard. Mater. 154, 818–825. doi: 10.1016/j.jhazmat.2007.10.097
Wang, Q., Guan, C., Wang, P., Lv, M. L., Ma, Q., Wu, G. Q., et al. (2015b). AtHKT1.1 and AtHAK5 mediate low-affinity Na+, uptake in Arabidopsis thaliana, under mild salt stress. Plant Growth Regul. 75, 615–623. doi: 10.1007/s10725-014-9964-2
Wang, Y. P., Gao, T. T., Zhang, Z. J., Yuan, X., Chen, Q., Zheng, J. Z., et al. (2020). Overexpression of the tyrosine decarboxylase gene MdTyDC confers salt tolerance in apple. Environ. Exp. Bot. 180:104244. doi: 10.1016/j.envexpbot.2020.104244
Wang, Y. Y., Cheng, Y. H., Chen, K. E., and Tsay, Y. F. (2018). Nitrate transport, signaling, and use efficiency. Annu. Rev. Plant Biol. 69, 85–122. doi: 10.1146/annurev-arplant-042817-040056
Xia, F. S., Dong, Q. L., and Dong, K. H. (2010). Effects of alkali stress on the growth characteristics of Saussurea runcinata. J. Grassl. Forage Sci. 5, 15–18.
Xu, G., Huang, T. F., Zhang, X. L., and Duan, B. L. (2013). Significance of mesophyll conductance for photosynthetic capacity and water-use efficiency in response to alkaline stress in Populus cathayana seedlings. Photosynthetica 51, 438–444. doi: 10.1007/s11099-013-0043-0
Yang, C. W., Xu, H. H., Wang, L. L., Liu, J., Shi, D. C., and Wang, D. L. (2009). Comparative effects of salt-stress and alkali-stress on the growth, photosynthesis, solute accumulation, and ion balance of barley plants. Photosynthetica 47, 79–86. doi: 10.1007/s11099-009-0013-8
Yang, C., Chong, J., Li, C., Kim, C., Shi, D., and Wang, D. (2007). Osmotic adjustment and ion balance traits of an alkali resistant halophyte kochia sieversiana during adaptation to salt and alkali conditions. Plant Soil 294, 263–276. doi: 10.1007/s11104-007-9251-3
Yang, C., Shi, D., and Wang, D. (2008). Comparative effects of salt and alkali stresses on growth, osmotic adjustment and ionic balance of an alkali-resistant halophyte Suaeda glauca (Bge.). Plant Growth Regul. 56, 179–190. doi: 10.1007/s10725-008-9299-y
Yang, J. Y., Zheng, W., Tian, Y., Wu, Y., and Zhou, D. W. (2011). Effects of various mixed salt-alkaline stresses on growth, photosynthesis, and photosynthetic pigment concentrations of Medicago ruthenica seedlings. Photosynthetica 49, 275–284. doi: 10.1007/s11099-011-0037-8
Yuan, L. X., Graff, L., Loque, D., Kojima, S., and Tsuchiya, Y. (2009). AtAMT1;4, a pollen-specific high-affinity ammonium transporter of the plasma membrane in Arabidopsis. Plant Cell Physiol. 50, 13–25.
Yuan, L., Loqué, D., Ye, F. H., Frommer, W. B., and von Wirén, N. (2007). Nitrogen-dependent posttranscriptional regulation of the ammonium transporter AtAMT1;1. Plant Physiol. 143, 732–744. doi: 10.1104/pp.106.093237
Zhang, J. L., Flowers, T. J., and Wang, S. M. (2010). Mechanisms of sodium uptake by roots of higher plants. Plant Soil 326:45. doi: 10.1007/s11104-009-0076-0
Zhang, Y. M., Zhang, H. M., and Liu, Z. H. (2015). The wheat NHX antiporter gene TaNHX2, confers salt tolerance in transgenic alfalfa by increasing the retention capacity of intracellular potassium. Plant Mol. Biol. 87, 317–327. doi: 10.1007/s11103-014-0278-6
Zhao, X. H., Yu, H. Q., Wen, J., Wang, X. G., Du, Q., Wang, J., et al. (2016). Response of root morphology, physiology and endogenous hormones in maize (Zea mays L.) to potassium deficiency. J. Integr. Agric. 15, 785–794. doi: 10.1016/s2095-3119(15)61246-1
Zhu, J. K. (2003). Regulation of ion homeostasis under salt stress. Curr. Opin. Plant Biol. 6, 441–445. doi: 10.1016/s1369-5266(03)00085-2
Keywords: MdTyDc, alkaline stress, dopamine, ion homeostasis, N metabolism
Citation: Liu X, Jin Y, Tan K, Zheng J, Gao T, Zhang Z, Zhao Y, Ma F and Li C (2021) MdTyDc Overexpression Improves Alkalinity Tolerance in Malus domestica. Front. Plant Sci. 12:625890. doi: 10.3389/fpls.2021.625890
Received: 10 December 2020; Accepted: 27 January 2021;
Published: 16 February 2021.
Edited by:
Bao-Sheng Qiu, Central China Normal University, ChinaReviewed by:
Mingcai Zhang, China Agricultural University, ChinaJing Zhang, Nanjing Agricultural University, China
Copyright © 2021 Liu, Jin, Tan, Zheng, Gao, Zhang, Zhao, Ma and Li. This is an open-access article distributed under the terms of the Creative Commons Attribution License (CC BY). The use, distribution or reproduction in other forums is permitted, provided the original author(s) and the copyright owner(s) are credited and that the original publication in this journal is cited, in accordance with accepted academic practice. No use, distribution or reproduction is permitted which does not comply with these terms.
*Correspondence: Fengwang Ma, ZndtNjRAbndzdWFmLmVkdS5jbg==; ZndtNjRAc2luYS5jb20=; Chao Li, bGM0NTNAMTYzLmNvbQ==