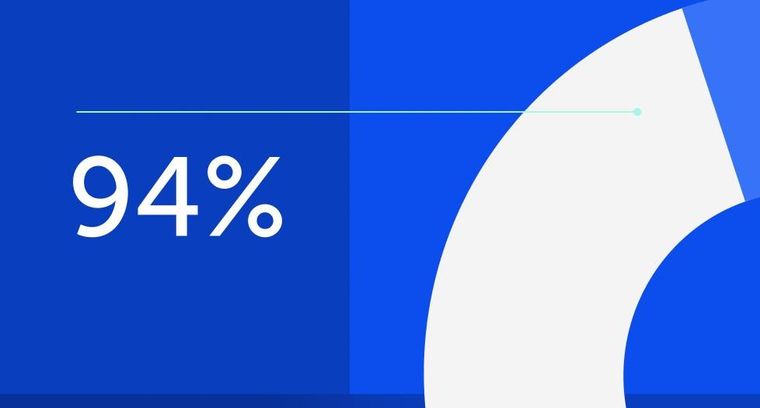
94% of researchers rate our articles as excellent or good
Learn more about the work of our research integrity team to safeguard the quality of each article we publish.
Find out more
ORIGINAL RESEARCH article
Front. Plant Sci., 28 January 2021
Sec. Plant Development and EvoDevo
Volume 12 - 2021 | https://doi.org/10.3389/fpls.2021.620325
This article is part of the Research TopicMolecular Mechanisms of Flowering Plant ReproductionView all 19 articles
Protein farnesylation is a post-translational modification regulated by the ERA1 (Enhanced Response to ABA 1) gene encoding the β-subunit of the protein farnesyltransferase in Arabidopsis. The era1 mutants have been described for over two decades and exhibit severe pleiotropic phenotypes, affecting vegetative and flower development. We further investigated the development and quality of era1 seeds. While the era1 ovary contains numerous ovules, the plant produces fewer seeds but larger and heavier, with higher protein contents and a modified fatty acid distribution. Furthermore, era1 pollen grains show lower germination rates and, at flower opening, the pistils are immature and the ovules require one additional day to complete the embryo sac. Hand pollinated flowers confirmed that pollination is a major obstacle to era1 seed phenotypes, and a near wild-type seed morphology was thus restored. Still, era1 seeds conserved peculiar storage protein contents and altered fatty acid distributions. The multiplicity of era1 phenotypes reflects the diversity of proteins targeted by the farnesyltransferase. Our work highlights the involvement of protein farnesylation in seed development and in the control of traits of agronomic interest.
Post-translational processes are commonly used to control activity, stability, sub-cellular localization and/or protein interaction through modifications of biochemical properties of proteins. Among them protein isoprenylation is widely distributed in Eukaryotes and relies on the covalent attachment of a C15 farnesyl or a C20 geranylgeranyl isoprenoid onto a specific CaaX-box located at the C-terminal end of polypeptide chains (Zhang and Casey, 1996; Galichet and Gruissem, 2003; Hemsley, 2015). This is mediated by the CaaX-protein prenyltransferases (CaaX-PTases) (i.e., protein farnesyltransferase (PFT) and protein geranylgeranyltransferase type I (PGGT-I)). Both enzymes are heterodimers composed of a common α-subunit and a specific β-subunit (Lane and Beese, 2006). The identification of targets of CaaX-PTases is still poorly documented in plants. Among the 120 putative farnesylatable proteins predicted in silico in the Arabidopsis thaliana genome (Galichet and Gruissem, 2003), less than 15 have been functionally characterized. These proteins have wide-ranging functions, being involved in chaperone pathways, in phytohormone biosynthesis, in ubiquitin-mediated protein degradation or in gene expression regulation (Barth et al., 2009; Crowell and Huizinga, 2009; Dutilleul et al., 2016; Northey et al., 2016; Barghetti et al., 2017; Sjögren et al., 2018). For most of them, isoprenylation results in membrane anchoring or in modified subcellular location, promoting or not interactions with other proteins (Running, 2014; Hemsley, 2015).
The biological relevance of CaaX-protein isoprenylation in plant has been uncovered 20 years ago through the study of mutants compromised in one or both CaaX-PTases activities. Studies dedicated to Arabidopsis ggb (geranylgeranyltransferase β-subunit) mutant characterization highlighted few developmental phenotypes but suggested PGGT-I involvement in phytohormone signaling and in some aspects of plant defense (Johnson et al., 2005; Goritschnig et al., 2008). The Arabidopsis era1 (enhanced response to abscisic acid1) mutant, impaired in the β-subunit of PFT, has been extensively studied due to its multifaceted phenotypes. era1 has been discovered in a genetic screen designed to isolate mutants with altered ABA sensitivity at germination (Cutler et al., 1996). era1-mediated ABA sensitivity have been also associated to stomatal movements and to the resistance to hydric stress (Pei et al., 1998). Since this phenotype relies on traits of agronomic interests, numerous works have been undertaken to better understand the link between ABA and protein farnesylation. Hence, era1-mediated drought tolerance and increased seed dormancy are observed in crops such as wheat and rapeseed, as well (Wang et al., 2009; Manmathan et al., 2013). Several developmental defects characterize era1 plants that display increased seed dormancy, delayed growth, longer lifespan, enlarged meristems, altered phyllotaxis and root architecture, as well as supernumerary floral organs (Cutler et al., 1996; Running et al., 1998, 2004; Yalovsky et al., 2000b; Ziegelhoffer et al., 2000; Brady et al., 2003). In addition, era1 displays an enhanced susceptibility to pathogens and an increased tolerance to heat, indicating that farnesylation also participates to plant responses to both biotic and abiotic stress (Goritschnig et al., 2008; Wu et al., 2017).
The era1 mutant has been extensively described through ABA signaling and for its developmental phenotypes, ranging from seed germination to flower morphology. According to the Arabidopsis eFP Browser atlas1 ERA1 (At5g40280) is widely expressed in plant (data not shown). With in situ hybridization studies, Ziegelhoffer et al. (2000) revealed that ERA1 is expressed during flower and embryo developments. Nevertheless, era1 seed features (i.e., morphology and content) have not been documented so far, while ABA signaling largely contributes to seed development (Baud et al., 2008). Seed development is a complex process that starts by embryo morphogenesis and then proceeds by seed maturation during which accumulation of storage compounds occurs in the embryo. Many seed traits are also heavily dependent on ovule development maternal traits, especially those that have maternal sporophytic origin (Baud and Lepiniec, 2010; Nakashima and Yamaguchi-Shinozaki, 2013; Shu et al., 2016). In this complex process, ABA acts upstream of key transcriptional factors that control a wide range of seed-specific traits and play a critical role in seed quality, including lipid and protein contents (Baud et al., 2008). Among these, ABI3 (Abscisic acid Insensitive 3) is a major actor of the ABA-dependent seed maturation process and it participates in the control of storage compound biosynthesis (Giraudat et al., 1992; Roscoe et al., 2015). ABI3 acts downstream of ERA1 in the seed ABA responsiveness (Brady et al., 2003). Thus, one would expect era1 plants to be affected in the seed maturation process.
In the present work, we characterized the seeds produced by Arabidopsis era1-8 and ggb-2 mutants. Our data show that protein farnesylation, but not geranylgeranylation, is engaged in seed size determination and in the production of seed storage compounds (i.e., protein and lipid contents). Moreover, ω3 and ω6 fatty acid (FA) repartition is altered in era1 seeds. Further investigation linked the era1 low seed production to an inefficient self-pollination and explained some era1 seed peculiarities. Hence, our results reveal the functional plurality of protein farnesylation in the control of flower and seed development.
An heapmap generated by recovering the expression pattern of ERA1, GGB and PLP genes during eight stages of seed maturation (Winter et al., 2007) reveals that the genes encoding the subunits of protein isoprenylation enzymes are expressed during the early stages of seed development, then decrease until the green cotyledon stage (Figure 1A). In order to assess the impact of protein farnesylation or geranylgeranylation on seed production, we first investigated the seed production of the protein isoprenylation mutants era1-8 (Goritschnig et al., 2008) and ggb-2 (Running et al., 2004). Plants were grown under short day conditions and, as previously described (Bonetta et al., 2000), under these growth conditions, era1 plants showed severe developmental phenotypes, whereas ggb plants developed similarly to WTs, without any obvious developmental phenotype. At first sight, isoprenylation mutants do not produce altered seeds in morphology nor color (Figure 1B). Nevertheless, while era1-8 plants appear to be in poor shape, seed size measurements revealed that era1-8 seeds are significantly longer (Figure 1C) and wider (Figure 1D) than WT. The inferred volume confirms that era1-8 seeds are larger than those of the WT by 25% (Figure 1E). The size phenotype is supported by an increased seed weight in era1-8, by 25% too (Figure 1F). No difference could be detected for ggb-2 seeds based on these criteria. A close-up view to embryo cells highlights that the surface of embryo cells increases in era1-8 (Figure 2). Although seed size also relies on the size of the tegument cells, as well as endosperm development, the increased seed size of era1-8 may be associated to bigger embryo cells in this mutant. Enlarged meristems have been already reported in era1 and it is related to increased cell size too (Running et al., 1998; Yalovsky et al., 2000b). Hence, the farnesylation-dependent mechanisms that control meristem cell size might be extended to embryo cells.
Figure 1. Seed morphological features of Arabidopsis prenylation mutants. (A) Heatmap of ERA1, GGB and PLP gene expression during seed development (data collected from Winter et al., 2007). (B) Representative mature seeds from WT, era1-8 and ggb-2 mutants. Scale bar, 500 μm. (C) and (D) Length and width correspond to an average of >250 measurements (i.e., >50 seeds from 5 independent biological replicates for each genotype) using ImageJ software and microscopy pictures. (E) The volume was calculated according to Riefler et al. (2006) using (C) and (D) data. (F) Seed mass was estimated by weighting 500 seeds of five different plants for each genotype with three technical replicates. Data represent mean ± SE. ***p-value < 0.001 (Student’s t-test).
Figure 2. Embryo cell size. (A) Representative pictures of embryo (up) and embryo cells (down) from WT, era1-8 and ggb-2. (B) Box-plot of embryo cell areas from WT, era1-8 and ggb-2. Cell surface embryo were measured using differential interference contrast microscopy pictures and ImageJ software. Gray boxes represent 50% of measured cell areas, horizontal lines are the medians, the top and the bottom whiskers represent 25% of the measurements (n > 200). Scale bar, 250 μm for embryo pictures and 10 μm for embryo cells. ∗∗∗P value < 0.001 (Student’s t-test).
Near-infrared spectroscopy (NIRS) is a nondestructive and accurate method developed to assess carbon, nitrogen, lipid and protein in Arabidopsis seeds and allows the detection of seed filling modifications (Jasinski et al., 2016). NIRS quantification reveals that era1-8 seeds contain a lower percentage of carbon and a higher percentage of nitrogen compared to WT and ggb-2 (Figures 3A,B). According to NIRS predictive equations (Jasinski et al., 2016), era1-8 seeds look enriched in proteins and depleted in lipids (Supplementary Figure 2A), nevertheless, when considering a single seed (heavier in era1-8, Figure 1F), lipid content is comparable in the three genotypes whereas protein content is higher in era1-8 than in WT and ggb-2 (Figure 3C). This suggests that era1-8 seed weight is increased by an extra protein filling while the overall lipid quantity is not altered in this mutant.
Figure 3. Near-infrared spectroscopy to assess seed carbon, nitrogen, protein and lipid contents. Graphs showing carbon (A) and nitrogen (B) contents of WT, era1-8, ggb-2 seeds. (C) Graphs showing predicted seed protein and lipid contents (μg) expressed per seed. Data represent mean ± SE. ***p-value < 0.001 (Student’s t-test).
NIRS protein quantification was further strengthened by Bradford protein assays (Bradford, 1976) and confirmed that the era1-8 seeds accumulate more proteins than WT and ggb-2 (Figures 4A,B). Arabidopsis seeds contain two predominant types of storage proteins, 12S globulins and 2S albumins (Heath et al., 1986). They represent more than 80% of total seed proteins (Higashi et al., 2006) and constitute the main source of nitrogen and sulfur during the seed germination (Tabe et al., 2002). When a quantity of protein equivalent to one seed is separated on SDS-PAGE, era1-8 displays a global pattern with more intense bands than WT and ggb-2 (Figure 4C). When the same amount of protein (i.e., 5 μg) is loaded in each lane, all three patterns appear more balanced (Figure 3D). Because era1-8 produces larger and heavier seeds, we could expect higher protein content in these seeds, but 1 mg of era1-8 seeds contains more protein than 1 mg of WT (and ggb-2) seeds (Figures 3C, 4A), which would mean that era1-8 seeds have somehow enriched protein content. Quantification of the band intensities performed after gel scanning (Di Berardino et al., 2018) shows that high molecular weight proteins (HW, above 37 kDa) are more abundant in WT than era1-8 seeds, while low molecular-weight proteins (LW, below 37 kDa, mainly 12Sα and 12Sβ globulins and 2S albumins) are more abundant in era1-8 than in WT seeds (Figure 4D, graph), especially the lowest 2S albumin band. Beside an increased seed size that accumulates more protein in seed, those results indicate that storage protein profiles is altered in era1-8 and it affects 2S albumins rather than 12S globulins.
Figure 4. Qualitative analysis of protein contents in Arabidopsis prenylation mutant seeds. Quantification of total protein extracts from mature seeds expressed (A) as μg mg– 1 of seeds or (B) as μg seed– 1 using Bradford’s method (1976). Data present mean ± SE of 5 replicates. *** indicates a p-value < 0.001 (Student’s t-test). (C) SDS-PAGE loaded with the amount of proteins equivalent to one seed (silver nitrate staining), 12Sα and 12Sβ correspond to globulins, 2S corresponds to albumins. (D) SDS-PAGE loaded with 5 μg of total seed protein in each lane (silver nitrate staining). The graph on the right corresponds to WT and era1-8 ImageJ plot profiles. HW and LW correspond to high-weight (>37 kDa) and low-weight (<37 kDa) proteins, respectively [according to Di Berardino et al. (2018)].
Near-infrared spectroscopy experiments revealed comparable lipid contents in the seeds of the three genotypes (Figure 3C) but this approach cannot distinguish the different forms of lipids. So, to complement the NIRS data, seed lipid compositions were investigated through HPTLC analyses. WT, era1-8 and ggb-2 contain comparable quantities of phospholipids per mg of seeds (Supplementary Figure 2B), but individual seeds of era1-8 display 30% more phospholipids (Figure 5A). This is consistent with the larger size of seeds and embryo cells observed in era1-8. Since Arabidopsis is an oleaginous plant, carbon reserves are mainly stored as triacylglycerols (TAGs) in specific lipid bodies of embryo cells (Murphy, 1993). TAGs consist of a glycerol bound to three fatty acids (FAs) and represent more than 90% of seed total lipids in Arabidopsis (Baud et al., 2008). So, we assume that NIRS analyses reflect TAG contents (7–7.9 μg per seed, Figure 5B) rather than minor seed lipids such as phospholipids (3.7–4.2 μg per seed, Figure 5A), explaining why the difference in phospholipid contents is only observed with HPTLC analyses. One mg of era1-8 seeds contains slightly less TAGs than WT and ggb-2 (Supplementary Figure 2C). However, although era1-8 seeds are larger, one era1-8 seed contains an equal quantity of TAGs as WT or ggb-2 seeds (Figure 5B). We then investigated FA distribution in the 3 genotypes. Gas chromatography analysis reveals that era1-8 has an altered FA distribution while ggb-2 resembles to that of WT. Notably, era1-8 seeds accumulate more C18:1 and C18:2, and display a lower C18:3 content (Figure 5C). Repartition of C18:0, C20:2 and C22:1 is also altered with less pronounced variations (Figure 5C). Furthermore, TAGs are enclosed within lipid bodies that consist of a monolayer of phospholipids and structural proteins, mainly steroleosin and oleosins (Jolivet et al., 2004). Consistent with the similar quantity of TAGs observed in the three genotypes, WT, era1-8 and ggb-2 seeds display comparable lipid body-associated protein patterns (Figure 5C, inset).
Figure 5. Comparison of lipid contents in WT, era1-8 and ggb-2 seeds. (A) Total phospholipids contents per seed. (B) TAG contents per seed. (C) FAs distribution in seeds (%). Inset shows lipid body protein patterns prepared from 25 mg of dry seeds (see section “Materials and Methods”); S, steroleosin and O, oleosins [according to Jolivet et al. (2004)]. Values are the mean ± SE of five independent replicates each composed of 10 mg of seeds. *** indicates a p-value < 0,001 (Student’s t-test).
All these data indicate that protein farnesylation, but not geranylgeranylation, may control seed size determination and the production of seed storage compounds (i.e., protein content and FA distribution).
In Arabidopsis, pollination and fertilization follow the flower opening and then, embryo development and seed maturation take place. Until silique dehiscence, this process occurs within 16 days for WT and ggb-2 plants (Figure 6A). Silique development is dramatically delayed in era1-8. At day four, whereas WT and ggb-2 siliques start to elongate, era1-8 siliques remain shorter and the tip starts to crook. Yellowing of siliques that corresponds to the end of the seed maturation, is observed at day 29 for era1-8, instead of day 16 for WT and ggb-2. Silique dehiscence is delayed by 13 days in era1-8 (Figure 6A). Moreover, era1-8 mature siliques are significantly smaller than WT and ggb-2 (Figure 6A and Supplementary Figure 1), distorted and display a crooked tip (Figure 6A). Moreover, at DAF0, era1-8 stigma does not display fully developed papillae as WT (Figure 6B). Under our growth conditions (i.e., short days), most of era1-8 gynoecium are constituted by three carpels and develop numerous ovules compared to WT (Figure 6B). Variation in carpel number was observed in three other alleles of era1 (i.e., wig-1, wig-2, and wig-3 corresponding to WIGGUM, a former name of ERA1, Running et al., 1998), nevertheless this phenotype is more developed under short day growth conditions than long days (Yalovsky et al., 2000b). Quantification of ovule production reveals that era1-8 produces about twice more ovules than WT (Figure 6C), which represents around 24 and 31 ovules per carpel for WT (2 carpels) and era1-8 (3 carpels), respectively. Surprisingly, era1-8 mature siliques contain few seeds (Figure 6D). WT plants produce frequently around 45–50 seeds per silique whereas it is highly variable in era1-8 and the median production is restricted to 12 (Figure 6E).
Figure 6. Silique development and seed production. (A) Kinetic of silique development of WT, era1-8 and ggb-2. (B) Representative pictures of ovules within open ovaries of WT and era1-8 at DAF0. (C) Quantification of ovules in WT and era1-8 ovaries at DAF0 (Student’s t-test, n = 10). (D) Open mature siliques of WT and era1-8. (E) Quantification of seed production in WT and era1-8 mature siliques (ANOVA, n = 30). DAF, Day after flowering. Scale bar in 6B and 6D is 1 mm. *** indicates a p-value < 0,001.
To understand why most of era1-8 ovules do not develop into seeds, we scrutinized the fate of era1-8 ovules at flower opening and the following days. Observations of ovules collected from WT and era1-8 ovaries at flower opening (i.e., DAF0, Day after flowering #0) reveal that era1-8 plants produce proper peripheral ovules tissues consisting of outer and inner integuments, endothelium, funiculus and micropyle as observed in WT (Figure 7A). However, era1-8 embryo sac is not fully developed at DAF0 whereas WT ovule exhibits a large embryo sac (Figure 7A). At DAF2, no embryo is visible in era1-8 ovules whereas WT ones already display globular embryos (Figure 7B). At DAF4 and DAF7, a developing embryo is visible in WT ovules at heart and green mature embryo stages, respectively (Figure 7B). In era1-8 ovules, the globular embryo stage is observed at DAF4 and the heart stage at DAF7, the green mature embryo stage is reached at DAF10. Actually, embryo development from globular embryo stage to green mature embryo stage takes five to six days in era1-8, as observed for WT. This indicates that, once the ovules are mature (i.e., with embryo sac), after fertilization, era1-8 embryo development is similar to WT. According to expression data (Figure 1A), ERA1 expression level is higher in the globular stage and then deceases during the seed development, which suggests that protein farnesylation may be a determinant process for embryo early development. Moreover, as exemplified (Figure 7B, lane era1-8†, DAF2-10), although era1-8 ovules display a fully developed embryo sac at DAF2, most of them do not develop embryo and end up degenerating (DAF10). This is consistent with the low seed production observed in era1-8 siliques (Figures 6D,E).
Figure 7. Comparison of WT and era1-8 ovules. (A) Detailed views of DAF0 unfertilized ovules of WT (with embryo sac) and era1-8 (without embryo sac). (B) Developmental kinetic of WT and era1-8 embryos (dashed lines) at indicated time. † corresponds to unfertilized era1-8 ovules. Dotted rectangle represented proper ovules observed at DAF2 in era1-8 with different futures. oi, outer integuments; ii, inner integuments; en, endothelium; m, micropyle; f, funiculus; ES, Embryo Sac; N, Nucellus [according to Yu et al. (2005)]. Scale bar 250 μm in panel (A) and 50 μm in panel (B). DAF, Day after flowering.
Because era1-8 plant produces proper ovules that may be fertilized and able to develop embryos, ovule degeneration can arise from a deficiency in fertilization or a spontaneous abortion. We then investigated era1-8 flower abilities to perform pollination. As previously described (Northey et al., 2016), era1-8 flower exhibits a protruding pistil already observable in flower buds (Supplementary Figure 3), i.e., before anthesis. At flower opening (Figure 8A, DAF0), WT harbors two short stamens and four long stamens that are in contact with the stigma, which promotes the pollination. In this way, in the Arabidopsis Col-0 accession, fertilization further occurs mainly thanks to self-pollination (Nasrallah et al., 2004). In era1-8 flowers, all stamens appear below the stigma (Figure 8A) and remain below after flowering. This should significantly reduce self-pollination and lower seed production. Moreover, at DAF0, papillae that are required for pollen recruitment on stigma are fully developed in WT (Figure 8B). A close-up view to era1-8 stigma reveals that at DAF0, papillae are clearly shorter (Figure 8B, DAF0) and require one additional day to acquire a comparable appearance to those of WT (Figure 8B, DAF1). The delay in papillae expansion may thus further reduce pollen capture and pollination efficiency in era1-8.
Figure 8. Gynoecium defects and pollination in era1-8. (A) Anther and stigma relative positioning at flower opening at indicated time. Black and white arrowheads indicate the top of stamens and stigmas, respectively. (B) Close-up views of flower’s stigmas and papillae shown in panel (A). (C) Transversal cross-sections of young WT and era1-8 siliques stained with neutral red and Alcian blue (scale bar, 50 μm). The corresponding ovary organization is drawn below (green, carpel; violet, replum; orange, septum; blue, transmitting tract). (D) WT and era1-8 freshly harvested pollen (scale bar, 50 μm). (E) Estimated pollen volumes. (F) In vitro pollen germination assays. (G) Quantification of germinated pollen grains ± SE (Student t-test, n = 6). DAF, Day after flowering.
Arabidopsis WT ovary is bilocular and consists of two valves (open carpels) connected by two replums. An additional transversal wall, the septum, links the two replums and supports the transmitting tract that facilitates pollen tube growth (Lennon et al., 1998). Because of callose deposition, Alcian blue specifically stains the transmitting tracts among ovary tissues (Reyes-Olalde et al., 2013). Cross sections of WT and era1-8 siliques reveal that, in most cases, era1-8 has 3-carpels ovaries with a disturbed organization (Figure 8C and Supplementary Figure 4A). The septum does not link the replums and the transmitting tracts appear free in the unilocular ovary (Figure 8C and Supplementary Figure 4A).
According to Bonetta et al. (2000), era1 pollen release is not delayed but microsporogenesis is strongly perturbed in era1. Indeed, the microsporocyte meiosis is not synchronous and may produce aberrant tetrads and misshaped or degenerated microspores, reducing the quality of the pollen production. Although era1-8 mature pollen grains display a comparable shape with that of WT (Figure 8D), they are significantly larger (Figure 8E and Supplementary Figure 4B). Pollen germination capacity, assessed through in vitro assays, highlighted that in our experimental conditions, WT pollen grains reach up to 60% germination whereas era1-8 ones are limited to 30% (Figures 8F,G).
In order to decipher whether pollination is critical for era1-8 seed phenotypes, hand pollinations were performed on WT and era1-8 flowers. Stamens where removed from flowers before opening and pollen was directly applied on pistils. As shown in Figure 9A, era1-8 pistils pollinated by WT pollen produce siliques with a comparable shape of WT ones (WT × WT). A reciprocal cross (WT ovules vs era1-8 pollen) achieves the same result. era1-8 vs era1-8 hand pollination restores a WT silique phenotype, as well, without a crooked tip (Figure 9A). This indicates distortion of era1-8 tips during silique development (Figure 6A) is correlated with the low seed content. Besides, average seed production is partially restored regardless of the crossing made (Figure 9B). era1-8 pollen applied on WT pistils leads to a seed production close to that of WT x WT hand crosses. Nevertheless, the pollination of era1-8 pistils by either WT or era1-8 pollens results in numerous non-developing seeds (Supplementary Figure 5) and era1-8 show a highly variable seed production among the siliques (Figure 9B). Whatever the pollination method applied and the pollen genotypes, pollination and fertilization success of era1-8 is difficult to control because of the delayed pistil maturity and its variable structural organization.
Figure 9. Phenotyping of seeds obtained after hand pollination. (A) Pictures of representative siliques obtained after handmade pollination with indicated crosses. (B) Average of seed number within siliques from (a) (n = 5 for WT and 20 for era1-8, see section “Materials and Methods”). (C) Volume of seeds obtained from WT x WT (WT) or era1-8 x era1-8 (era1-8) handmade crosses. (D) Seed weight. (E) Seed protein content. (F) Seed total lipids. (G) SDS-PAGE protein pattern. (H) Repartition (%) of indicated FAs. Error bars are ± SE.
Morphology and biochemical characterization of seeds produced through era1-8 x era1-8 hand pollinations displays a near-WT phenotype: volume, weight, protein content and total lipid content were indeed restored (Figures 9C–F), suggesting that these characteristics rely on the number of seeds developing in the siliques. However, the lower 2S storage protein remains more abundant in era1-8 x era1-8 protein pattern (Figure 9G and Supplementary Figure 6A) and the distribution of C18:1, C18:2 and C18:3 is still altered, as observed when era1-8 plants self-pollinate (Figure 9H and Supplementary Figure 6B). This further support the role of protein farnesylation in these biochemical seed traits.
Two decades ago, ERA1 was involved in flower development thanks to thorough descriptions of era1 mutants by Running et al. (1998) and Yalovsky et al. (2000a,b). The authors described enlarged floral meristems, late flowering, homeotic transformations of flowers and supernumerary organs in floral whorls. This organ number phenotype correlated with specific size changes in the early floral meristem and authors suggested that ERA1 controls cell division and differentiation in the floral meristem. This would also explain why era1 flowers often fail to develop (Yalovsky et al., 2000b). Besides era1 floral phenotypes, our work highlights noticeable morphological and biochemical phenotypes of siliques and seeds that we specifically associated to the protein farnesylation (era1-8) but not to the protein geranylgeranylation (ggb-2). Overall, ggb plants are barely affected by the mutation (Johnson et al., 2005) compared to era1 for which the phenotypes are probably based on specifically farnesylated CaaX-proteins. Nevertheless, this discrepancy can also be explained through the specific activities of PFT and PGGT-I. Indeed, PFT is less specific than PGGT-I for CaaX-box sequences, PFT can therefore compensate for loss of PGGT-I in ggb mutants more effectively than PGGT-I can compensate for loss of PFT in era1-8 mutants (Andrews et al., 2010). Consequently, as described for vegetative developmental traits and the flower shape, seed production and quality are also more altered in era1-8 than in ggb-2.
Nevertheless, some of the era1-8 seed phenotypes that we describe can be, at least partially, attributed to CaaX-proteins cited in the literature.
era1-8 produces twice more ovules than WT plants. Although hand pollination improves era1-8 seed production to a comparable level to that of WT, it does not lead to the full development of all era1-8 ovules into seeds. So, era1-8 low seed production can be attributed to both spontaneous ovule abortions and low self-pollination efficiency (Figures 8, 9). Indeed, a protruding pistil seems incompatible with self-pollination and it may explain the low seed production in era1-8. Northey et al. (2016) described protruding pistils in the flowers of cyp85a2 mutants. Interestingly, CYP85A2 gene encodes a CaaX-protein, belonging to the cytochrome P450 family, involved in brassinosteroid synthesis. Loss of either CYP85A2 or CYP85A2 farnesylation (mutated CaaX-box) resulted in reduced brassinolide accumulation (Northey et al., 2016). In era1, protruding pistils can thus originate from malfunctions of non-farnesylated CYP85A2. In addition, brassinosteroids are involved in various plant developmental process and play a major role in the control of pollen germination and growth (Ye et al., 2010; Vogler et al., 2014). Because CYP85A2 is highly expressed in pollen tubes (Supplementary Figure 7A), we can speculate that involvement of CYP85A2 in reproductive traits may not only concern the protruding pistils but also concerns the pollen germination and/or tube growth. However, era1 pollination faces several problems: protruding pistils, low germinating pollen grains, improper ovary transmitting tracts, delayed stigma papillae extension and delayed proper ovule formation (Figure 8). Because those traits are handled by different physiological pathways (Lord and Russell, 2002; Ma, 2005; Browse and Wallis, 2019), we can suspect that besides CYP85A2 several farnesylatable CaaX-proteins participate in the successful completion of pollination in WT Arabidopsis plants.
In addition to pollination defect, a closer view to hand pollinated era1-8 siliques shows that numerous ovules do not develop into seeds, even when hand pollination is performed with WT pollen (Supplementary Figure 5). Indeed, WT and era1-8 plants produce around 45 and 90 ovules per silique, respectively (Figure 6C). Hand-pollination of WT pistils with WT or era1-8 pollens leads to the development of 38–45 seeds (Figure 9B) suggesting that era1-8 pollen is efficient enough to ensure the fertilization by this way. When era1-8 pistils are hand-pollinated, among the ∼90 ovules present in era1-8 siliques, around 49–56 develop into seeds (Figure 9B and Supplementary Figure 5). According to our observations, it is difficult to assess whether non-developing seeds in era1-8 result from (i) spontaneous ovule abortion, (ii) abortion due to no fertilization (because of pollen quality and/or carpel alterations), and (iii) ovule abortion at zygotic to pre-globular stages. Since the protein farnesyl transferase has hundreds of targets, in era1-8, non-developing seeds may result from overlapping malfunctions of CaaX-proteins involved in ovule/seed development. This may engage the MEE56 (Maternal Effect Embryo arrest 56) gene, coding for a CaaX-protein, that we tracked in a list of 130 loci resulting from a large-scale screen of Arabidopsis mutants with defects in female gametophyte development (Pagnussat et al., 2005). MEE56 is the only CaaX-protein we identified in Pagnussat et al. (2005). Interestingly, MEE mutants may arrest embryo development at one-cell stage (i.e., zygote). They are also characterized by a slight general delay in embryo sac development and fertilization. Genetic approaches indicated these phenotypes rely on female gametophyte malfunctions (Pagnussat et al., 2005). MEE56 belongs to the HIPP (Heavy metal-associated Isoprenylated Plant Protein) family (45 members in Arabidopsis; MEE56 is HIPP18), which are all equipped with a C-terminal CaaX-box (de Abreu-Neto et al., 2013). Although HIPP molecular function has not been clearly established, isoprenylation affects their subcellular localization and protein-protein interactions (Cowan et al., 2018). mee56 siliques bear some aborted seeds (Pagnussat et al., 2005), which may correspond to those observed in era1-8 siliques that also rely on maternal traits (Supplementary Figure 5, ♀era1-8 x ♂WT). According to Le et al. (2010) microarray experiments, MEE56 expression is restricted to the chalazal endosperm (Supplementary Figure 7B), which allows the flow of nutrients from the phloem to the egg sac through the endosperm (Sager and Lee, 2014). A lack of farnesylation may disrupt MEE56 function or localization, and would lead to seed abortion in era1, as described in mee56 siliques (Pagnussat et al., 2005).
The high ovule production observed in era1-8 may be related to the increased number of carpels (Figures 6, 8). In Arabidopsis WT flowers, the number of carpels (i.e., two) is highly conserved whereas in the other whorls, the number of flower organs may be slightly variable (Running et al., 1998). In era1, supernumerary flower organs are often observed, including the carpel whorl (Running et al., 1998). Number of floral organs relies on floral meristem homeostasis that is determined by a complex interplay involving the WUSCHEL/CLAVATA (WUS/CLV) pathway, hormonal controls and dozens of genes (Somssich et al., 2016; Conti, 2017; Lee et al., 2019). Among these, the two homologs AtJ2/AtJ3 (A. thaliana DnaJ homologue 2 or 3) encode CaaX-proteins that may partially explain era1 floral-related phenotypes. Indeed, the atj2/atj3 double mutant complemented with a non-farnesylatable form of AtJ3 (AtJ3C417S) displays enlarged meristems similarly to era1 and produces flowers with supernumerary petals. Nevertheless, only 16% of AtJ3C417S flowers display supernumerary petals when it reaches 66% in era1, and no alteration of the number of carpels nor stamens has been reported in AtJ3C417S (Barghetti et al., 2017). WUS is required for stem cell identity and CLV promotes organ initiation (Schoof et al., 2000). clv mutants harbor enlarged meristems and give rise to supernumerary stamens and carpels, but they maintain a WT-like sepal/petal number (Schoof et al., 2000). We can therefore suspect that, although farnesylation of AtJ3 is required for the determinism of petal number, era1 floral phenotypes rely on other CaaX-proteins related to WUS/CVL pathway that control the determinism of the number of carpels. For instance, APETALA1 (AP1) is a well known transcription factor involved in the early floral meristem identity (Yalovsky et al., 2000a). ap1 mutant develops flowers with carpeloid sepals and stamenoid petals. AP1 and its paralog CAULIFLOWER (CAL) have farnesylatable CaaX-boxes. Together, they participate in the transition of inflorescence meristem into floral meristem (Ye et al., 2016). AP1 is mainly expressed during flower development but its expression is also detected in ovary as for CAL (Supplementary Figure 7C). ap1, cal and ap1/cal knock-out plants display more severe floral phenotype than era1 suggesting that the non-farnesylated proteins present in era1 flower maintain some functionality. Because, AP1 is also involved in flower organ number determination (Monniaux et al., 2018) and its actions are enhanced through CAL (Ye et al., 2016), the lack of farnesylation of both proteins may lead, in cooperation with AtJ2/AtJ3, to the abnormal number of carpels observed in era1-8. Investigating Arabidopsis transgenic ap1/cal/AtJ2/AtJ3 plants co-expressing non-farnesylatable forms of AP1, CAL, AtJ2 and AtJ3 (i.e., mutated CaaX-boxes) with their specific transcriptional promoters may unravel the involvement of protein farnesylation in carpel number determination, nevertheless we cannot exclude that other unidentified CaaX-proteins make more complex the mechanism leading to this era1-8 singular phenotype.
Interestingly, era1-8 produces larger seeds than the WT, with different storage contents. They accumulate more proteins and have a different FA distribution. The control of seed size depends on genetic, environmental and physiological factors (Gnan et al., 2014; Orozco-Arroyo et al., 2015). Because hand pollination of era1-8 flowers restores WT-like size and most of the biochemical phenotypes (Figure 9), the seed enlargement observed in era1-8 may be a consequence of the low seed yield (Herridge et al., 2011). Nutrients provided by era1-8 plants toward the flowers are distributed in few developing seeds, which consequently accumulate more storage compounds (Patrick and Offler, 2001; Zhang et al., 2007). Depending on mechanical constraints (Rolletschek et al., 2020), seed enlargement as well as silique impairments may also contribute to modify the accumulation of storage compounds observed in self-pollinated era1-8 seeds. However, hand pollination do not restore the enhanced accumulation of 2S albumin (Figure 9G and Supplementary Figure 6A) nor the differential FA distribution observed in era1-8 seeds (Figure 9H and Supplementary Figure 6B). Seed maturation involves master regulators such as ABI3, FUS3 (Fusca 3), LEC1 and LEC2 (Leafy Cotyledon 1 and 2) that govern embryo development and storage compound accumulation. It also involves hormonal regulations, mainly relying on ABA signaling (Baud et al., 2008; Kanno et al., 2010; Gao et al., 2012). Although the relation between ERA1 and ABA-signaling has not been fully elucidated, ABA enhanced sensitivity of era1-8 may also perturb the control of storage compounds accumulation in seeds.
Beside an increased overall protein content in era1-8 seeds, the 2S albumin accumulation is discernibly modified (Figure 4D). In Arabidopsis, five genes encode the 2S albumins (At2S1-5) (Gruis et al., 2002). Albumins are synthesized as precursors that are cleaved post-translationally by vacuolar processing enzymes (Otegui et al., 2006). Although the 2S albumin gene expression follows the embryo maturation process, albumins can accumulate differentially depending on nutrient intake (Higashi et al., 2006). For instance, sulfur modulates At2S3 accumulation but not its transcript level suggesting that albumin accumulation is regulated at the post-translational step rather than transcriptional level (Naito et al., 1994; Higashi et al., 2006). Furthermore, according to amino acid sequence analysis, the 2S albumin atomic composition is three time richer in sulfur compared to the 12S globin one, whereas the other atoms (i.e., C, H, N, and O) are similar (Supplementary Figure 8) which suggests that era1-8 seeds have an overall enhanced sulfur content. Albumins are not farnesylated (no CaaX-box on precursors nor mature albumins; Shimada et al., 2003; Higashi et al., 2006), thus ERA1 action on albumin accumulation may stand on unidentified CaaX-proteins involved in nutrient perception or albumin post-translational cleavages during seed maturation.
Finally, era1-8 seed phenotypes also deal with altered FA distribution. The major changes concern the increase of C18:1 and C18:2, and a decrease in C18:3 (Figure 5C). The C18:2/C18:3 balance (related to ω6 and ω3) is essential for human fitness and animal feed (Okuyama et al., 2007), and it became an important trait for seed oil selection. In Arabidopsis seeds, FA distribution relies on well-characterized regulatory network and biosynthetic pathway (Baud et al., 2008). These include ω-6-fatty acid desaturase2 (FAD2), ω-3-fatty acid desaturase3 (FAD3), fatty acid elongase1 (FAE1) and diacylglycerol acyltransferase1 (DGAT1) (To et al., 2012), which are critical for determining the composition and/or quantity of seed storage oil in Arabidopsis (Li-Beisson et al., 2013). C18:1, C18:2 and C18:3 distributions are tightly linked by their biosynthetic pathway: C18:1 desaturation involves FAD2 to produce C18:2 and then FAD3 to produce C18:3 (Baud et al., 2008). FAD3 gene expression requires a transcriptional complex that engages L1L (Leafy cotyledon1-Like), NF-YC2 subunit and βZIP67 (Mendes et al., 2013). Interestingly, l1l, nf-yc2 and βzip67 mutants have an altered fatty acid composition and l1l C18:1/C18:2/C18:3 balance resembles to that of era1-8. None of these proteins are farnesylatable, nevertheless Dutilleul et al. (2016) described ASG2 (Altered Seed Germination 2), a farnesylated CaaX-protein involved in FA metabolism (Ducos et al., 2017). ASG2 belongs to a E3 ubiquitin ligase complex and interacts with histone deacetylase when farnesylated. Seeds of asg2 mutants have a higher level of C18:1 and a reduced C18:3 content, suggesting that ASG2 may control FAD2 and/or FAD3 expression through histone modifications, as described for the histone acetyltransferase GCN5 (General Control Non−repressed protein 5) (Wang et al., 2016). Although the FA phenotype of asg2 only partially overlap with era1-8, the lack of farnesylation of ASG2 might contribute to era1-8 FA peculiarities.
Major agronomic traits, such as enlarged seed and protein content, rely on self-pollination deficiency and cannot thus be exploited for further agronomic purposes. However, quality of FA composition in era1-8, as well as, 2S albumins accumulation would disserve more attention to improve seed nutritional quality.
Figure 10 summarizes new phenotypes developed by the era1-8 mutant that we identified. Several phenotypes are related to gynoecium impairments: carpel number and architecture, delayed papillae extension, pollen quality and ovule maturation. Other phenotypes rely on the seed morphology (i.e., size, weight) and storage compounds (i.e., ω3/ω6 FAs, 2S albumins). We assume that these various phenotypes are the consequence of dysfunctions of several CaaX-proteins in era1-8. To date, most of the prenylated protein functions have been established through the characterization of knocked-out plants for the corresponding genes. Thus, the phenotypes observed do not correspond to a deficiency in isoprenylation but rather to a total absence of the functional protein in the plant, which is different of what happens in era1. Investigating the role of protein farnesylation requires the production of the non-farnesylatable protein in plant without the wild-type form. This can be achieved through the utilization of genome editing technologies allowing specific modifications of the CaaX-box encoding sequence for a unique farnesylatable protein. This strategy could lead to understand the role of isoprenylation on CaaX-protein behaviors and to decipher the era1 intricate phenotypes.
Figure 10. Diagram of an era1-8 flower summarizing farnesylation-related phenotypes. ERA1 with the PLP α-subunit constitute the active farnesyltransferase that performs CaaX-protein farnesylation. The picture summarizes novel era1 phenotypes involving unidentified CaaX-proteins.
Experiments were performed using A. thaliana accession Columbia (Col-0), the T-DNA insertion mutant era1-8 [stock name SALK_110517 described in Goritschnig et al. (2008)] and the T-DNA insertion mutant ggb-2 [stock name SALK_040904C described in Running et al. (2004)]. Plants were grown in a growth chamber (24°C at 70% humidity) under a 12 h light/12 h dark photoperiod and were exposed to an 80–100 μmol.m–2.s–1 irradiance. Once harvested, seeds were maintained in the dark at 4°C.
Expression data were retrieved from the Bio-Analytic Resource (BAR) Expression browser2 by querying the database with the Seed and Silique Development filter. Raw absolute expression data were centered and scaled per gene and the heatmap was generated with Excel software. Accession numbers: ERA1 (Enhanced Response to ABA1), At5g40280; GGB (GeranylGeranyl transferase Beta subunit), At2g39550; PLP (PLuriPetala), At3g59380.
Length and width of 50 dry seeds from five independent biological replicates (i.e., five plants) for each genotype were determined using the ImageJ software3 and microscopy pictures. The volume of the seeds was calculated by equating the seed shape to a spheroid, according to Riefler et al. (2006). A similar approach was used to determine pollen grain sizes and volumes.
To determine the Arabidopsis seed weight, 500 dry mature seeds (stored in dark at 4°C for 2 months after harvest) were weighted with five independent biological replicates for each genotype with three technic replicates.
NIRS method and predictive equations developed by Jasinski et al. (2016) were used to determine carbon, nitrogen, protein and lipid contents in Arabidopsis seeds. Seed samples (about 300 μl of seeds) were placed in a 9 mm diameter clear glass bottle (Agilent, 5182-0714) on 4 mm height for NIRS spectra acquisition and were analyzed as intact (without any treatment). Spectra acquisition was performed with a Fourier transform near-infrared (FTNIR) analyzer (Antaris II spectrometer; Thermofisher Scientific, France). Spectra were collected as described by Jasinski et al. (2016). The spectral data provide useful information about the organic signature of the Arabidopsis samples.
Seed total protein extracts were prepared as described in Rajjou et al. (2008) with minor changes. 50 dry mature seeds were hand-grinded using mortar and pestle at 4°C in 200 μL of extraction buffer consisting of 18 mM Tris-base, 14 mM Tris–HCl, 7 M urea, 2 M thiourea, 4% CHAPS, 0.2% Triton X-100, 1 mM PMSF (Harder et al., 1999). Samples were left on ice for 10 min. After the addition of 14 mM dithiothreitol, samples were incubated for 20 min at 4°C with shaking and clarified by centrifugation for 20 min at 20.000 g at 4°C. Protein concentration was determined in the supernatant using the Bradford method (Bradford, 1976). Protein extracts were analyzed by 12% SDS-PAGE and proteins were revealed by silver staining.
Proteins of seed lipid bodies were prepared from 25 mg of seeds using sucrose flotation techniques including successive NaCl, Tween 20 and urea treatments in order to remove non-specifically trapped proteins, as described by Jolivet et al. (2004). After an overnight acetone precipitation, dry pellets were dissolved in Laemmli sample buffer and directly loaded on 15% SDS-PAGE. Proteins were then stained by Coomassie blue procedure.
Triacylglycerol (TAG) and total phospholipid quantifications were performed by High-Performance Thin-Layer Chromatography (HPTLC). Lipids were extracted by grinding 10 mg of dry seeds in 1 ml of dichloromethane:chloroform (2:1, v:v) 5 times during 1 min in a Mixer Mill MM400 (Retsch) at 30 hz. Samples were cooled on ice 30 s during each grinding cycle. Lysates were filtrated and lipid extract collected according to Bligh and Dyer (1959). Lipid extracts were spotted on HPTLC precoated silica gel glass plates (60F254 Merck, Darmstadt, Germany) using a CAMAG autosampler ATS4 sample applicator (CAMAG, Muttenz Switzerland). Before loading, plates were pre-developed once in chloroform:methanol (1:1, v:v), air-dried, and activated at 110°C for 20 min. Samples were applied in the form of 10-mm band shapes, 1.5 cm from the bottom of the silica plate at a constant flow rate of 150 nl.s–1 under nitrogen flow. Development was carried out with a mobile phase composed of hexane:ether:acetic acid (70:30:1, v:v:v). HPTLC plates were then air-dried for 90 min, and treated for 1 min in an immersion tank containing a 50% perchloric acid solution. After a 2-h drying period at room temperature, plates were heated for 16 min at 160°C to allow carbonization for the staining reaction. Densitometric analysis was performed using a TLC/HPTLC video-densitometer (TLC Visualizer 2 CAMAG, with Videoscan software, Camag, Muttenz, Switzerland). TAG and total phospholipids quantifications were performed using standard calibration curves obtained by spotting standards of known lipid composition.
For fatty acid analysis, TAG were separated by one-dimensional TLC (LK5, 20 × 20 cm, Carlo Erba, Val de Reuil, France) using hexane:diethyl ether:acetic acid (60:40:1, v:v:v) as mobile phase. TAG spots were scraped and collected in screw-cap glass tubes. TAG were prepared as fatty acids methyl esters before gas chromatography analysis (GC-2010plus, Shimadzu Scientific instruments, Noisiel, France) using a BPX70 capillary column (60 m, SGE, Chromoptic SAS, Courtaboeuf, France). Hydrogen was used as carrier gas with a constant pressure (120 kPa). After an on-column injection of sample at 60°C, oven temperature increased from 60°C to 220°C. Fatty acids methyl esters were detected by a FID at 255°C and identified by comparison of their retention times with commercial standards (Supelco 37, Fatty Acid Methyl Ester mix, Sigma-Aldrich, France, St Louis, MO, United States). Fatty acids levels were expressed as the percentage of total integrated peaks area using the GC Solutions software (Shimadzu, Noisiel, France).
For Arabidopsis crossing, one day prior to pollination, mature siliques, open flowers and buds having a white tip were removed from the plant. Immature buds were delicately opened with a needle under a binocular and stamens were removed with forceps. Two to three flowers were emasculated per inflorescence. After 1 day, stamens of freshly opened flowers of the desired plant were brushed against the emasculated flower stigmas. In order to ensure a maximum pollination rate, the pollination was repeated twice during 2 days on the same flowers after anthesis. Pollen presence on anthers was carefully checked with binocular magnifier.
For hand pollination of era1-8 flowers, one stamen of a freshly opened flower was removed delicately and then brushed against the stigma of the same flower. The process was repeated until the presence of pollen on the stigma could be assessed under the binocular. Five experiments were conducted when WT pistils were used (n = 5 in Figure 9). Because era1-8 pistils gave highly variable number of seeds, the experiment was repeated 20 times (n = 20 in Figure 9).
Selected flowers were marked by a colored knot on the day of flowering and siliques were further collected at the desired day. Siliques were opened delicately under a binocular and ovules or developing seeds were incubated between microscopic slides overnight at room temperature in the dark in 50 μl of Hoyer clarification medium (24 g chloral hydrate, 3 ml glycerol and 9 ml Milli-Q® water; Feng and Ma, 2017). Samples were then directly observed with an Olympus BX51 microscope equipped with a differential interference contrast (DIC) optic and an Olympus DP71 digital camera.
Flowers were incubated in FAA (50% ethanol, 5% acetic acid, and 5% formaldehyde) 16 h at 4°C. After ethanol and tert-butanol series, the samples were incubated overnight at 60°C, first in Paraplast®Plus:tert-butanol (1:1) and then in pure Paraplast®Plus (Leica Biosystems, Richmond, VA, United States). The Paraplast-embedded samples were sectioned to a thickness of 10 μm by using a rotary microtome. Sections were spread on slides pretreated with 2% 3-aminopropyltriethoxysilane (Sigma-Aldrich) in acetone (v:v), dried for 24 h at 40°C. Two 15-min incubations in xylene were used to remove paraffin from the samples, and an ethanol series up to water was used to rehydrate the sections. Sections were then stained for 2 h with Alcian blue (Sigma-Aldrich) to visualize acidic polysaccharides, such as callose, a major component of the transmitting tract (Crawford et al., 2007) and then with neutral red (to visualize cell walls) for 15 s. Samples were then observed on microscope.
Pollen germination assays were performed according to Li (2011). About ten open flowers (DAF1 to DAF3) for each genotype were collected and dried for 30 min at room temperature. After addition of 500 μL of germination medium (5 mM MES-Tris, 1 mM mM KCl, 0.5 mM MgSO4, 1.5 mM Boric acid, 10 mM CaCl2, 5% sucrose (w:v) and 15% PEG4000 (w:v), pH 5.8; Fan et al., 2001), flowers were shaken and pollen grains were collected by centrifugation at 500 g for 5 min. Pellet of pollen was suspended in 500 μl of germination medium. Pollen grains were germinated at 28°C overnight by placing 100 μl of the pollen suspensions in a 96 well plate (BioLite Thermo Fisher Scientific). Microscopy pictures of germinating pollen were obtained as described for ovules. The procedure was conducted six times for each genotype (n = 6).
The original contributions presented in the study are included in the article/Supplementary Material, further inquiries can be directed to the corresponding author/s.
VV and CD performed plant experiments, microscopy observations, and analyzed data. BG, BC, AL, and LR performed NIRS experiments and protein analyses. CG, MP, and SC performed seed lipid analyses. ED and CD planned and designed the research. CD, NG-G, and ED wrote the manuscript. All authors contributed to the article and approved the submitted version.
EA2106 laboratory was financially supported by the “Enseignement supérieur, de la Recherche et de l’Innovation” French Ministry and the Région Centre-Val de Loire. Institut Jean-Pierre Bourgin (IJPB) benefits from the support of the LABEX Saclay Plant Sciences-SPS (ANR-10-LABX-0040-SPS).
The authors declare that the research was conducted in the absence of any commercial or financial relationships that could be construed as a potential conflict of interest.
We thank O. Pichon from the University of Tours for critical reading. M-A Marquet (University of Tours) for her technical expertise in plant culture.
The Supplementary Material for this article can be found online at: https://www.frontiersin.org/articles/10.3389/fpls.2021.620325/full#supplementary-material
Andrews, M., Huizinga, D. H., and Crowell, D. N. (2010). The CaaX specificities of Arabidopsis protein prenyltransferases explain era1 and ggb phenotypes. BMC Plant Biol. 10:118. doi: 10.1186/1471-2229-10-118
Barghetti, A., Sjögren, L., Floris, M., Paredes, E. B., Wenkel, S., and Brodersen, P. (2017). Heat-shock protein 40 is the key farnesylation target in meristem size control, abscisic acid signaling, and drought resistance. Gene Dev. 31, 2282–2295. doi: 10.1101/gad.301242.117
Barth, O., Vogt, S., Uhlemann, R., Zschiesche, W., and Humbeck, K. (2009). Stress induced and nuclear localized HIPP26 from Arabidopsis thaliana interacts via its heavy metal associated domain with the drought stress related zinc finger transcription factor ATHB29. Plant Mol. Biol. 69, 213–226. doi: 10.1007/s11103-008-9419-0
Baud, S., Dubreucq, B., Miquel, M., Rochat, C., and Lepiniec, L. (2008). Storage reserve accumulation in Arabidopsis: metabolic and developmental control of seed filling. Arabidopsis Book 6:e0113. doi: 10.1199/tab.0113
Baud, S., and Lepiniec, L. (2010). Physiological and developmental regulation of seed oil production. Prog. Lipid Res. 49, 235–249. doi: 10.1016/j.plipres.2010.01.001
Bligh, E. G., and Dyer, W. J. (1959). A rapid method of total lipid extraction and purification. Can. J. Biochem. Physiol. 37, 911–917. doi: 10.1139/y59-099
Bonetta, D., Bayliss, P., Sun, S., Sage, T., and McCourt, P. (2000). Farnesylation is involved in meristem organization in Arabidopsis. Planta 211, 182–190. doi: 10.1007/s004250000283
Bradford, M. M. (1976). A rapid and sensitive method for the quantitation of microgram quantities of protein utilizing the principle of protein-dye binding. Anal. Biochem. 72, 248–254. doi: 10.1016/0003-2697(76)90527-3
Brady, S. M., Sarkar, S. F., Bonetta, D., and McCourt, P. (2003). The ABSCISIC ACID INSENSITIVE 3 (ABI3) gene is modulated by farnesylation and is involved in auxin signaling and lateral root development in Arabidopsis. Plant J. 34, 67–75. doi: 10.1046/j.1365-313x.2003.01707.x
Browse, J., and Wallis, J. G. (2019). Arabidopsis flowers unlocked the mechanism of jasmonate signaling. Plants 8:285. doi: 10.3390/plants8080285
Conti, L. (2017). Hormonal control of the floral transition: Can one catch them all? Dev. Biol. 430, 288–301. doi: 10.1016/j.ydbio.2017.03.024
Cowan, G. H., Roberts, A. G., Jones, S., Kumar, P., Kalyandurg, P. B., Gil, J. F., et al. (2018). Potato mop-top virus co-opts the stress sensor HIPP26 for long-distance movement. Plant Physiol. 76, 2052–2070. doi: 10.1104/pp.17.01698
Crawford, B. C., Ditta, G., and Yanofsky, M. F. (2007). The NTT gene is required for transmitting-tract development in carpels of Arabidopsis thaliana. Curr. Biol. 17, 1101–1108. doi: 10.1016/j.cub.2007.05.079
Crowell, D. N., and Huizinga, D. H. (2009). Protein isoprenylation: the fat of the matter. Trends Plant Sci. 14, 163–170. doi: 10.1016/j.tplants.2008.12.001
Cutler, S., Ghassemian, M., Bonetta, D., Cooney, S., and McCourt, P. (1996). A protein farnesyl transferase involved in abscisic acid signal transduction in Arabidopsis. Science 273, 1239–1241. doi: 10.1126/science.273.5279.1239
de Abreu-Neto, J. B., Turchetto-Zolet, A. C., de Oliveira, L. F., Zanettini, M. H., and Margis-Pinheiro, M. (2013). Heavy metal-associated isoprenylated plant protein (HIPP): characterization of a family of proteins exclusive to plants. FEBS J. 280, 1604–1616. doi: 10.1111/febs.12159
Di Berardino, J., Marmagne, A., Berger, A., Yoshimoto, K., Cueff, G., Chardon, F., et al. (2018). Autophagy controls resource allocation and protein storage accumulation in Arabidopsis seeds. J. Exp. Bot. 69, 1403–1414. doi: 10.1093/jxb/ery012
Ducos, E., Vergès, V., Dugé de Bernonville, T., Blanc, N., Giglioli-Guivarc’h, N., and Dutilleul, C. (2017). Remarkable evolutionary conservation of antiobesity ADIPOSE/WDTC1 homologs in animals and plants. Genetics 207, 153–162. doi: 10.1534/genetics.116.198382
Dutilleul, C., Ribeiro, I., Blanc, N., Nezames, C. D., Deng, X. W., Zglobicki, P., et al. (2016). ASG2 is a farnesylated DWD protein that acts as ABA negative regulator in Arabidopsis. Plant Cell Environ. 39, 185–198. doi: 10.1111/pce.12605
Fan, L. M., Wang, Y. F., Wang, H., and Wu, W. H. (2001). In vitro Arabidopsis pollen germination and characterization of the inward potassium currents in Arabidopsis pollen grain protoplasts. J. Exp. Bot. 52, 1603–1614. doi: 10.1093/jxb/52.361.1603
Feng, J., and Ma, L. (2017). A method for characterizing embryogenesis in Arabidopsis. J. Vis. Exp. 126:55969.
Galichet, A., and Gruissem, W. (2003). Protein farnesylation in plants - conserved mechanisms but different targets. Curr. Opin. Plant Biol. 6, 530–535. doi: 10.1016/j.pbi.2003.09.005
Gao, M.-J., Gropp, G., Wei, S., Hegedus, D. D., and Lydiate, D. J. (2012). “Combinatorial networks regulating seed development and seed filling,” in Embryogenesis, ed. K.-I. Sato (Rijeka: InTech), 189–228.
Giraudat, J., Hauge, B. M., Valon, C., Smalle, J., Parcy, F., and Goodman, H. M. (1992). Isolation of the Arabidopsis ABI3 gene by positional cloning. Plant Cell 4, 1251–1261. doi: 10.2307/3869411
Gnan, S., Priest, A., and Kover, P. X. (2014). The genetic basis of natural variation in seed size and seed number and their trade-off using Arabidopsis thaliana MAGIC lines. Genetics 198, 1751–1758. doi: 10.1534/genetics.114.170746
Goritschnig, S., Weihmann, T., Zhang, Y., Fobert, P., McCourt, P., and Li, X. (2008). A novel role for protein farnesylation in plant innate immunity. Plant Physiol. 148, 348–357. doi: 10.1104/pp.108.117663
Gruis, D. F., Selinger, D. A., Curran, J. M., and Jung, R. (2002). Redundant proteolytic mechanisms process seed storage proteins in the absence of seed-type members of the vacuolar processing enzyme family of cysteine proteases. Plant Cell 14, 2863–2882. doi: 10.1105/tpc.005009
Harder, A., Wildgruber, R., Nawrocki, A., Fey, S. J., Larsen, P. M., and Görg, A. (1999). Comparison of yeast cell protein solubilization procedures for two-dimensional electrophoresis. Electrophoresis 20, 826–829. doi: 10.1002/(sici)1522-2683(19990101)20:4/5<826::aid-elps826>3.0.co;2-a
Heath, J. D., Weldon, R., Monnot, C., and Meinke, D. W. (1986). Analysis of storage proteins in normal and aborted seeds from embryo-lethal mutants of Arabidopsis thaliana. Planta 169, 304–312. doi: 10.1007/bf00392124
Hemsley, P. A. (2015). The importance of lipid modified proteins in plants. New Phytol. 205, 476–489. doi: 10.1111/nph.13085
Herridge, R. P., Day, R. C., Baldwin, S., and Macknight, R. C. (2011). Rapid analysis of seed size in Arabidopsis for mutant and QTL discovery. Plant Methods 7:3. doi: 10.1186/1746-4811-7-3
Higashi, Y., Hirai, M. Y., Fujiwara, T., Naito, S., Noji, M., and Saito, K. (2006). Proteomic and transcriptomic analysis of Arabidopsis seeds: molecular evidence for successive processing of seed proteins and its implication in the stress response to sulfur nutrition. Plant J. 48, 557–571. doi: 10.1111/j.1365-313x.2006.02900.x
Jasinski, S., Lécureuil, A., Durandet, M., Bernard-Moulin, P., and Guerche, P. (2016). Arabidopsis seed content QTL mapping using high-throughput phenotyping: the assets of near infrared spectroscopy. Front. Plant Sci. 7:1682. doi: 10.3389/fpls.2016.01682
Johnson, C. D., Chary, S. N., Chernoff, E. A., Zeng, Q., Running, M. P., and Crowell, D. N. (2005). Protein geranylgeranyltransferase I is involved in specific aspects of abscisic acid and auxin signaling in Arabidopsis. Plant Physiol. 139, 722–733. doi: 10.1104/pp.105.065045
Jolivet, P., Roux, E., D’Andrea, S., Davanture, M., Negroni, L., Zivy, M., et al. (2004). Protein composition of oil bodies in Arabidopsis thaliana ecotype WS. Plant Physiol. Biochem. 42, 501–509. doi: 10.1016/j.plaphy.2004.04.006
Kanno, Y., Jikumaru, Y., Hanada, A., Nambara, E., Abrams, S. R., Kamiya, Y., et al. (2010). Comprehensive hormone profiling in developing Arabidopsis seeds: examination of the site of ABA biosynthesis, ABA transport and hormone interactions. Plant Cell Physiol. 51, 1988–2001. doi: 10.1093/pcp/pcq158
Lane, K. T., and Beese, L. S. (2006). Thematic review series: lipid posttranslational modifications. Structural biology of protein farnesyltransferase and geranylgeranyltransferase type I. J. Lipid Res. 47, 681–699. doi: 10.1194/jlr.r600002-jlr200
Le, B. H., Cheng, C., Bui, A. Q., Wagmaister, J. A., Henry, K. F., Pelletier, J., et al. (2010). Global analysis of gene activity during Arabidopsis seed development and identification of seed-specific transcription factors. PNAS USA 107, 8063–8070. doi: 10.1073/pnas.1003530107
Lee, Z. H., Hirakawa, T., Yamaguchi, N., and Ito, T. (2019). The roles of plant hormones and their interactions with regulatory genes in determining meristem activity. Int. J. Mol. Sci. 20:4065. doi: 10.3390/ijms20164065
Lennon, K. A., Roy, S., Peter, K., Hepler, P. K., and Lord, E. M. (1998). The structure of the transmitting tissue of Arabidopsis thaliana (L.) and the path of pollen tube growth. Sex. Plant Reprod. 11, 49–59. doi: 10.1007/s004970050120
Li-Beisson, Y., Shorrosh, B., Beisson, F., Andersson, M. X., Arondel, V., Bates, P. D., et al. (2013). Acyl-lipid metabolism. Arabidopsis Book 11:e0161.
Lord, E. M., and Russell, S. D. (2002). The mechanisms of pollination and fertilization in plants. Annu. Rev. Cell Dev. Biol. 18, 81–105. doi: 10.1146/annurev.cellbio.18.012502.083438
Ma, H. (2005). Molecular genetic analyses of microsporogenesis and microgametogenesis in flowering plants. Annu. Rev. Cell Dev. Biol. 56, 393–434. doi: 10.1146/annurev.arplant.55.031903.141717
Manmathan, H., Shaner, D., Snelling, J., Tisserat, N., and Lapitan, N. (2013). Virus induced gene silencing of Arabidopsis thaliana gene homologues in wheat identifies genes conferring improved drought tolerance. J. Exp. Bot. 64, 1381–1392. doi: 10.1093/jxb/ert003
Mendes, A., Kelly, A. A., van Erp, H., Shaw, E., Powers, S. J., Kurup, S., et al. (2013). bZIP67 regulates the omega-3 fatty acid content of Arabidopsis seed oil by activating fatty acid desaturase3. Plant Cell 25, 3104–3016. doi: 10.1105/tpc.113.116343
Monniaux, M., Pieper, B., McKim, S. M., Routier-Kierzkowska, A. L., Kierzkowski, D., Smith, R. S., et al. (2018). The role of APETALA1 in petal number robustness. eLIFE 7:e39399.
Murphy, D. J. (1993). Structure, function and biogenesis of storage lipid bodies and oleosins in plants. Prog. Lipid Res. 32, 247–280. doi: 10.1016/0163-7827(93)90009-l
Naito, S., Hirai, M. Y., Chino, M., and Komeda, Y. (1994). Expression of a soybean (Glycine max [L.] Merr.) seed storage protein gene in transgenic Arabidopsis thaliana and its response to nutritional stress and to abscisic acid mutations. Plant Physiol. 104, 497–503. doi: 10.1104/pp.104.2.497
Nakashima, K., and Yamaguchi-Shinozaki, K. (2013). ABA signaling in stress-response and seed development. Plant Cell Rep. 32, 959–970. doi: 10.1007/s00299-013-1418-1
Nasrallah, M. E., Liu, P., Sherman-Broyles, S., Boggs, N. A., and Nasrallah, J. B. (2004). Natural variation in expression of self-incompatibility in Arabidopsis thaliana: Implications for the evolution of selfing. PNAS USA 101, 16070–16074. doi: 10.1073/pnas.0406970101
Northey, J. G., Liang, S., Jamshed, M., Deb, S., Foo, E., Reid, J. B., et al. (2016). Farnesylation mediates brassinosteroid biosynthesis to regulate abscisic acid responses. Nat. Plants 2:16114.
Okuyama, H., Yamada, K., Miyazawa, D., Yasui, Y., and Ohara, N. (2007). Dietary lipids impacts on healthy ageing. Lipids 42, 821–825. doi: 10.1007/s11745-007-3073-1
Orozco-Arroyo, G., Paolo, D., Ezquer, I., and Colombo, L. (2015). Networks controlling seed size in Arabidopsis. Plant Reprod. 28, 17–32. doi: 10.1007/s00497-015-0255-5
Otegui, M. S., Herder, R., Schulze, J., Jung, R., and Staehelin, L. A. (2006). The proteolytic processing of seed storage proteins in Arabidopsis embryo cells starts in the multivesicular bodies. Plant Cell 18, 2567–2581. doi: 10.1105/tpc.106.040931
Pagnussat, G. C., Yu, H. J., Ngo, Q. A., Rajani, S., Mayalagu, S., Johnson, C. S., et al. (2005). Genetic and molecular identification of genes required for female gametophyte development and function in Arabidopsis. Development 132, 603–614. doi: 10.1242/dev.01595
Patrick, J. W., and Offler, C. E. (2001). Compartmentation of transport and transfer events in developing seeds. J. Exp. Bot. 52, 551–564. doi: 10.1093/jxb/52.356.551
Pei, Z. M., Ghassemian, M., Kwak, C. M., McCourt, P., and Schroeder, J. I. (1998). Role of farnesyltransferase in ABA regulation of guard cell anion channels and plant water loss. Science 282, 287–290. doi: 10.1126/science.282.5387.287
Rajjou, L., Lovigny, Y., Groot, S. P., Belghazi, M., Job, C., and Job, D. (2008). Proteome-wide characterization of seed aging in Arabidopsis: a comparison between artificial and natural aging protocols. Plant Physiol. 148, 620–641. doi: 10.1104/pp.108.123141
Reyes-Olalde, J. I., Zuñiga-Mayo, V. M., Chávez Montes, R. A., Marsch-Martínez, N., and de Folter, S. (2013). Inside the gynoecium: at the carpel margin. Trends Plant Sci. 18, 644–655. doi: 10.1016/j.tplants.2013.08.002
Riefler, M., Novak, O., Strnad, M., and Schmülling, T. (2006). Arabidopsis cytokinin receptor mutants reveal functions in shoot growth, leaf senescence, seed size, germination, root development, and cytokinin metabolism. Plant Cell 18, 40–54. doi: 10.1105/tpc.105.037796
Rolletschek, H., Muszynska, A., and Borisjuk, L. (2020). The process of seed maturation is influenced by mechanical constraints. New Phytol. 229, 19–23. doi: 10.1111/nph.16815
Roscoe, T. T., Guilleminot, J., Bessoule, J. J., Berger, F., and Devic, M. (2015). Complementation of seed maturation phenotypes by ectopic expression of ABSCISIC ACID INSENSITIVE3, FUSCA3 and LEAFY COTYLEDON2 in Arabidopsis. Plant Cell Physiol. 56, 1215–1228. doi: 10.1093/pcp/pcv049
Running, M. P. (2014). The role of lipid post-translational modification in plant developmental processes. Front. Plant Sci. 5:50. doi: 10.3389/fpls.2014.00050
Running, M. P., Fletcher, J. C., and Meyerowitz, E. M. (1998). The WIGGUM gene is required for proper regulation of floral meristem size in Arabidopsis. Development 125, 2545–2553.
Running, M. P., Lavy, M., Sternberg, H., Galichet, A., Gruissem, W., Hake, S., et al. (2004). Enlarged meristems and delayed growth in plp mutants result from lack of CaaX prenyltransferases. PNAS USA 101, 7815–7820. doi: 10.1073/pnas.0402385101
Sager, R., and Lee, J. Y. (2014). Plasmodesmata in integrated cell signalling: insights from development and environmental signals and stresses. J. Exp. Bot. 65, 6337–6358. doi: 10.1093/jxb/eru365
Schoof, H., Lenhard, M., Haecker, A., Mayer, K. F., Jürgens, G., and Laux, T. (2000). The stem cell population of Arabidopsis shoot meristems is maintained by a regulatory loop between the CLAVATA and WUSCHEL genes. Cell 10, 635–644. doi: 10.1016/s0092-8674(00)80700-x
Shimada, T., Yamada, K., Kataoka, M., Nakaune, S., Koumoto, Y., Kuroyanagi, M., et al. (2003). Vacuolar processing enzymes are essential for proper processing of seed storage proteins in Arabidopsis thaliana. J. Biol. Chem. 278, 32292–32299. doi: 10.1074/jbc.m305740200
Shu, K., Chen, Q., Wu, Y., Liu, R., Zhang, H., Wang, P., et al. (2016). ABI4 mediates antagonistic effects of abscisic acid and gibberellins at transcript and protein levels. Plant J. 85, 348–361. doi: 10.1111/tpj.13109
Sjögren, L., Floris, M., Barghetti, A., Völlmy, F., Linding, R., and Brodersen, P. (2018). Farnesylated heat shock protein 40 is a component of membrane-bound RISC in Arabidopsis. J. Biol. Chem. 293, 16608–16622.
Somssich, M., Je, B. I., Simon, R., and Jackson, D. (2016). CLAVATA-WUSCHEL signaling in the shoot meristem. Development 143, 3238–3248.
Tabe, L., Hagan, N., and Higgins, T. J. (2002). Plasticity of seed protein composition in response to nitrogen and sulfur availability. Curr. Opin. Plant Biol. 5, 212–217.
To, A., Joubès, J., Barthole, G., Lécureuil, A., Scagnelli, A., Jasinski, S., et al. (2012). WRINKLED transcription factors orchestrate tissue-specific regulation of fatty acid biosynthesis in Arabidopsis. Plant Cell 24, 5007–5023.
Vogler, F., Schmalzl, C., Englhart, M., Bircheneder, M., and Sprunck, S. (2014). Brassinosteroids promote Arabidopsis pollen germination and growth. Plant Reprod. 27, 153–167.
Wang, T., Xing, J., Liu, X., Liu, Z., Yao, Y., Hu, Z., et al. (2016). Histone acetyltransferase general control non-repressed protein 5 (GCN5) affects the fatty acid composition of Arabidopsis thaliana seeds by acetylating fatty acid desaturase3 (FAD3). Plant J. 88, 794–808.
Wang, Y., Beaith, M., Chalifoux, M., Ying, J., Uchacz, T., Sarvas, C., et al. (2009). Shoot-specific down-regulation of protein farnesyltransferase (alpha-subunit) for yield protection against drought in canola. Mol. Plant. 2, 191–200.
Winter, D., Vinegar, B., Nahal, H., Ammar, R., Wilson, G. V., and Provart, N. J. (2007). An “Electronic Fluorescent Pictograph” Browser for Exploring and Analyzing Large-Scale Biological Data Sets. PLoS One 2:e718. doi: 10.1371/journal.pone.0000718
Wu, J. R., Wang, L. C., Lin, Y. R., Weng, C. P., Yeh, C. H., and Wu, S. J. (2017). The Arabidopsis heat-intolerant 5 (hit5)/enhanced response to aba 1 (era1) mutant reveals the crucial role of protein farnesylation in plant responses to heat stress. New Phytol. 213, 1181–1193.
Yalovsky, S., Rodríguez-Concepción, M., Bracha, K., Toledo-Ortiz, G., and Gruissem, W. (2000b). Prenylation of the floral transcription factor APETALA1 modulates its function. Plant Cell 12, 1257–1266.
Yalovsky, S., Kulukian, A., Rodríguez-Concepción, M., Young, C. A., and Gruissem, W. (2000a). Functional requirement of plant farnesyltransferase during development in Arabidopsis. Plant Cell 12, 1267–1278.
Ye, L., Wang, B., Zhang, W., Shan, H., and Kong, H. (2016). Gains and losses of cis-regulatory elements led to divergence of the Arabidopsis APETALA1 and CAULIFLOWER duplicate genes in the time, space, and level of expression and regulation of one paralog by the other. Plant Physiol. 171, 1055–1069.
Ye, Q., Zhu, W., Li, L., Zhang, S., Yin, Y., Ma, H., et al. (2010). Brassinosteroids control male fertility by regulating the expression of key genes involved in Arabidopsis anther and pollen development. PNAS USA 107, 6100–6105.
Yu, H.-J., Hogan, P., and Venkatesan Sundaresan, V. (2005). Analysis of the female gametophyte transcriptome of Arabidopsis by comparative expression profiling. Plant Physiol. 139, 1853–1869.
Zhang, F. L., and Casey, P. J. (1996). Influence of metal ions on substrate binding and catalytic activity of mammalian protein geranylgeranyltransferase type-I. Biochem. J. 320, 925–932.
Zhang, W.-H., Zhou, Y., Dibley, K. E., Tyerman, S. D., Furbank, R. T., and Patrick, J. W. (2007). Nutrient loading of developing seeds. Funct. Plant Biol. 346, 314–331.
Keywords: ERA1, protein farnesylation, plant reproduction, fatty acid, gynoecium, pollination
Citation: Vergès V, Dutilleul C, Godin B, Collet B, Lecureuil A, Rajjou L, Guimaraes C, Pinault M, Chevalier S, Giglioli-Guivarc’h N and Ducos E (2021) Protein Farnesylation Takes Part in Arabidopsis Seed Development. Front. Plant Sci. 12:620325. doi: 10.3389/fpls.2021.620325
Received: 22 October 2020; Accepted: 08 January 2021;
Published: 28 January 2021.
Edited by:
Natalia Pabón-Mora, University of Antioquia, ColombiaReviewed by:
Raffaella Battaglia, Council for Agricultural and Economics Research (CREA), ItalyCopyright © 2021 Vergès, Dutilleul, Godin, Collet, Lecureuil, Rajjou, Guimaraes, Pinault, Chevalier, Giglioli-Guivarc’h and Ducos. This is an open-access article distributed under the terms of the Creative Commons Attribution License (CC BY). The use, distribution or reproduction in other forums is permitted, provided the original author(s) and the copyright owner(s) are credited and that the original publication in this journal is cited, in accordance with accepted academic practice. No use, distribution or reproduction is permitted which does not comply with these terms.
*Correspondence: Eric Ducos, ZXJpYy5kdWNvc0B1bml2LXRvdXJzLmZy
Disclaimer: All claims expressed in this article are solely those of the authors and do not necessarily represent those of their affiliated organizations, or those of the publisher, the editors and the reviewers. Any product that may be evaluated in this article or claim that may be made by its manufacturer is not guaranteed or endorsed by the publisher.
Research integrity at Frontiers
Learn more about the work of our research integrity team to safeguard the quality of each article we publish.