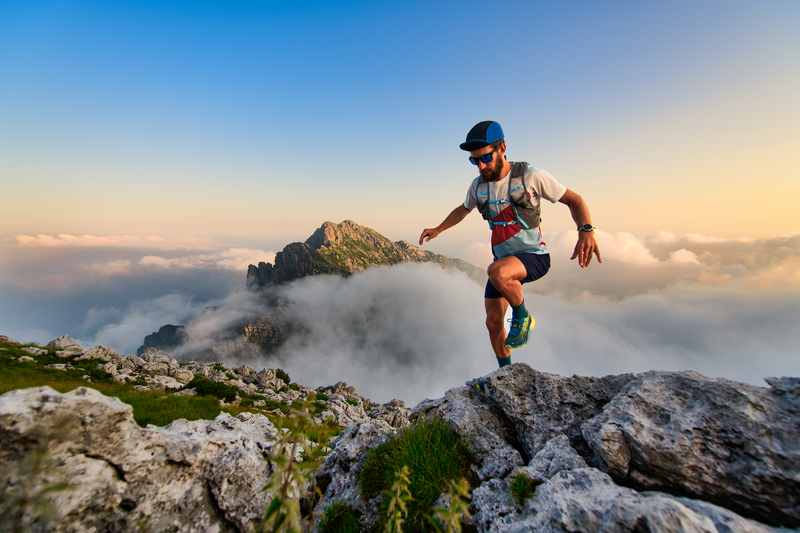
94% of researchers rate our articles as excellent or good
Learn more about the work of our research integrity team to safeguard the quality of each article we publish.
Find out more
MINI REVIEW article
Front. Plant Sci. , 25 January 2021
Sec. Plant Abiotic Stress
Volume 11 - 2020 | https://doi.org/10.3389/fpls.2020.632678
Multigene families coding for valine-glutamine (VQ) proteins have been identified in all kind of plants but chlorophytes. VQ proteins are transcriptional regulators, which often interact with WRKY transcription factors to regulate gene expression sometimes modulated by reversible phosphorylation. Different VQ-WRKY complexes regulate defense against varied pathogens as well as responses to osmotic stress and extreme temperatures. However, despite these well-known functions, new regulatory activities for VQ proteins are still to be explored. Searching public Arabidopsis thaliana transcriptome data for new potential targets of VQ-WRKY regulation allowed us identifying several VQ protein and WRKY factor encoding genes that were differentially expressed in oxygen-related processes such as responses to hypoxia or ozone-triggered oxidative stress. Moreover, some of those were also differentially regulated upon nitric oxide (NO) treatment. These subsets of VQ and WRKY proteins might combine into different VQ-WRKY complexes, thus representing a potential regulatory core of NO-modulated and O2-modulated responses. Given the increasing relevance that gasotransmitters are gaining as plant physiology regulators, and particularly considering the key roles exerted by O2 and NO in regulating the N-degron pathway-controlled stability of transcription factors, VQ and WRKY proteins could be instrumental in regulating manifold processes in plants.
A group of proteins containing the FxxxVQxxTG motif was first identified in Arabidopsis thaliana and named as valine-glutamine (VQ) proteins (Morikawa et al., 2002; Xie et al., 2010; Cheng et al., 2012). Up to 34 VQ proteins have been identified in A. thaliana (Cheng et al., 2012). The analysis of the regulatory activity of Arabidopsis VQ proteins revealed that all but five exhibited transcriptional regulatory activity, 17 activating and 12 repressing gene transcription (Li et al., 2014a). The integrity of the VQ motif seems to be essential for VQ4/MVQ1 and VQ29 regulatory activities (Li et al., 2014b; Weyhe et al., 2014) likely because their regulation often relies on the interaction with WRKY transcription factors (Cheng et al., 2012). The functional interaction of a subset of Arabidopsis VQ proteins, comprising 10 members, with WRKY transcription is modulated by reversible phosphorylation catalyzed by MAP kinases (Pecher et al., 2014; Weyhe et al., 2014). After the initial identification in Arabidopsis, VQ protein families have been also identified in a large number of plants, including rice, soybean, grapevine, Chinese cabbage, maize, banana, bamboo, strawberry, apple, tea plant, Eucalyptus, tobacco, chick pea, and alfalfa (Kim et al., 2013; Li et al., 2014a; Wang et al., 2014, 2015a, 2017; Zhang et al., 2015; Song et al., 2016; Ye et al., 2016; Zhou et al., 2016; Dong et al., 2018; Guo et al., 2018; Zhong et al., 2018; Garrido-Gala et al., 2019; Yan et al., 2019; Ling et al., 2020; Liu et al., 2020). The size of the VQ proteomes varies ranging from seven members identified in Selaginella moellendorffii to 74 in Glycine max (Jiang et al., 2018). While VQ proteins were initially thought to be plant specific proteins (Jing and Lin, 2015), recent studies on diverse genomes concluded that VQ proteins are also present in bacteria, fungi, and lower animals but not in algae (Jiang et al., 2018). The regulatory functions exerted by VQ proteins are manifold, and include defense against biotic (Xie et al., 2010; Lai et al., 2011; Wang et al., 2015b; Jiang and Yu, 2016; Chen et al., 2018; Yan et al., 2018) and abiotic stresses (Perruc et al., 2004; Hu et al., 2013b; Song et al., 2016; Cheng et al., 2020), and plant growth (Wang et al., 2010; Li et al., 2014b; Lei et al., 2017, 2018; Pan et al., 2018). Nevertheless, most of the plant VQ protein functions remain unknown.
Valine-glutamine proteins regulate developmental processes such as pollen or seed germination, plant size, photomorphogenesis, and leaf senescence. IKU1/VQ14 was characterized as a component of the so-called HAIKU pathway controlling the early growth phase of the seed endosperm (Garcia et al., 2003). iku1 mutant seeds were small and showed reduced endosperm growth (Wang et al., 2010). Chloroplast targeted VQ8 also plays a role in regulating growth as vq8-1 mutant displayed stunted-growth and pale-green leaves throughout the entire life cycle (Cheng et al., 2012). However, the over-expression of VQ17, VQ18, or VQ22, also led to highly stunted transgenic plants (Cheng et al., 2012), thus suggesting VQ proteins might promote or repress plant growth. Moreover, the over-expression of VQ29 delayed flowering time without altering vegetative growth (Cheng et al., 2012), but the expression of Arabidopsis VQ21 resulted in dwarfed and late-flowering plants (Gargul et al., 2015), thus suggesting VQ gene-specific functions may also uncouple different developmental processes. In addition, the heterologous overexpression of several soybean VQ genes in Arabidopsis led to altered leaf morphology, flowering, and seed setting (Zhou et al., 2016), thus indicating that developmental regulatory roles of VQ proteins are likely conserved across species. Moreover, the Arabidopsis vq29 mutant exhibited decreased hypocotyl elongation under low-intensity far-red and white light (Li et al., 2014b), thus pointing to VQ29 as a negative regulator of photomorphogenesis (Li et al., 2014b).
VQ20 regulates pollen development through its VQ motif by acting together with WRKY2 and WKRY34 in plant male gametogenesis (Lei et al., 2017) through the negative regulation of the expression of MYB97, MYB101, and MYB120 genes (Lei et al., 2018). Some of the development-related processes regulated by VQs are linked to phytohormone action. OsVQ13 positively regulated jasmonic acid (JA) signaling by activating the OsMPK6-OsWRKY45 signaling pathway that regulates grain size and resistance to Xanthomonas in rice (Uji et al., 2019). On the other hand, Arabidopsis seed germination seems to be controlled through the negative regulation exerted by VQ18 and VQ26 on ABI5 transcription factor-mediated ABA signaling (Pan et al., 2018). However, neither seed dormancy or leaf senescence nor ABA-regulated drought tolerance were significantly regulated by VQ18 and VQ26 (Pan et al., 2018), thus pointing to highly specific regulation. Leaf senescence is another developmental process potentially regulated by VQ proteins. The overexpression of maize ZmVQ52 in Arabidopsis accelerated premature leaf senescence (Yu et al., 2019). Figure 1A summarizes what has been reported on the involvement of VQ proteins and WRKY transcription factors in regulating different processes throughout plant life cycle.
Figure 1. Involvement of valine-glutamine (VQ) proteins and WRKY transcription factors in developmental processes throughout plant life cycle (A), in resistance of plants against biotrophic and necrotrophic pathogens as well as insects (B), and in responses to abiotic stress factors (C). VQ and WRKY proteins from different plants (At, Arabidopsis thaliana; Gm, Glycine max; Ma, Musa acuminate; Os, Oryza sativa; Pe, Phyllostachys edulis; Sl, Solanum lycopersicum; Zm, Zea mays) regulate positively (arrow lines) or negatively (blunt-ended lines).
Reports during the last decade supported the function of VQ proteins as relevant regulators of plant defense against pathogens and pests. The first report involving VQ proteins in defense against pathogens identified SIB1/VQ23 as an activator of JA-dependent salicylic acid (SA)-triggered resistance to Pseudomona syringae (Xie et al., 2010), and together with SIB2/VQ16, WRKY33, and WRKY57 also activated resistance to the necrotrophic pathogen Botrytis cinerea through the Jasmonate-zim-domain 1 (JAZ1) and 5 (JAZ5) proteins (Lai et al., 2011; Jiang and Yu, 2016). By contrast, VQ20 acted as a negative regulator of resistance to both biotrophic and necrotrophic pathogens (Cheng et al., 2012). MKS1/VQ21, in turn, positively regulated SA-mediated defense against biotrophic pathogens but it plays a negative role in JA-regulated defense against necrotrophic pathogens (Andreasson et al., 2005; Petersen et al., 2010). Regulation of resistance to Botrytis by VQ21 also requires the interaction with WRKY33 at the VQ motif domain (Petersen et al., 2010). Different VQ-WRKY complexes not only allow discriminating between different pathogens but also between defense and development. Silencing the JAV1/VQ22 gene significantly enhanced JA-regulated defense responses against necrotrophic pathogens and herbivorous insects by forming complexes with WRKY28 and WRKY51 but did not severely alter JA-mediated development (Hu et al., 2013a). Mutant or transgenic plants with double loss-of-function and gain-of-function in VQ12 and VQ29 genes were resistant and susceptible, respectively, to B. cinerea (Wang et al., 2015b), thus supporting the role of these VQ proteins as negative regulators of defense against this pathogen. Besides, the inactivation of VQ29 gene significantly increased susceptibility to Peronospora parasitica during the late stages of infection likely due to the inability to restrict the penetration and development of the oomycete (Le Berre et al., 2017). Other combinations of VQ proteins with WRKY transcription factors are also involved in modulating resistance to Botrytis. VQ10 physically interacts with WRKY8 and positively regulates plant basal resistance (Chen et al., 2018). On the other hand, strawberry homologs of Arabidopsis VQ defense proteins were all regulated in response to the ascomycete fungus Colletotrichum acutatum infection, causing anthracnose disease (Garrido-Gala et al., 2019). In tobacco, half of the 59 identified VQ protein encoding genes were significantly induced in response to Ralstonia solanacearum infection (Liu et al., 2020), thus supporting the potential extensive roles of VQ proteins in tobacco defense against this pathogen.
The complex roles of VQ genes in plant defense responses are likely due to their ability to interact with multiple WRKY proteins that in Arabidopsis were modulated through MAP Kinase-mediated phosphorylation and further degradation of VQ proteins (Pecher et al., 2014; Weyhe et al., 2014). Similar regulatory mechanisms seem to be operational also in rice (Li et al., 2014a) and Cucurbitaceae plants (Jiao et al., 2018), having an impact on regulating resistance to powdery mildew. Altogether, the involvement of VQ proteins in regulating defense against different pathogens is complex and gene-specific, likely occurring through combinatorial mechanisms involving other partners as well as functional interaction with diverse hormone-regulated pathways. These regulatory mechanisms seem to be also functional in plants attacked by insects. Injury rapidly triggers calcium influxes, calmodulin-dependent phosphorylation of JAV1/VQ22, dismantling of JAV1-JAZ8-WRKY51 complex, and activation of JA biosynthesis for plant defense (Yan et al., 2018). JAV1-associated Ubiquitin Ligase1 (JUL1) is the RING-type E3 ubiquitin ligase leading JAV1 to proteasomal degradation (Ali et al., 2019). In soybean, the down-regulation of GmVQ58 confers resistance to the common cutworm Spodoptera litura Fabricius (Li et al., 2020).
Summarizing, specific subsets of VQ proteins may regulate different pathosystems with process specificity through a complex network of interactions with WRKY transcription factors (Figure 1B). The distinct resulting complexes are often regulated through post-translational modifications (PTMs), with reversible phosphorylation being the best characterized.
Most of the information on plant VQ protein functions in responses to abiotic stress is related to osmotic stress. AtCaMBP25/VQ15 expression is induced in Arabidopsis seedlings exposed to dehydration, low temperature, or high salinity (Perruc et al., 2004). Transgenic plants overexpressing AtCaMBP25 exhibited increased sensitivity to both ionic and non-ionic osmotic stress during seed germination and seedling growth (Perruc et al., 2004). VQ9 protein acted as a repressor of the WRKY8 factor to maintain an appropriate balance of WRKY8-mediated signaling pathways and the onset of salinity stress tolerance (Hu et al., 2013b). In bamboo, PeVQ28 and WRKY83 interacted in the nucleus, and the over-expression of PeVQ28 in Arabidopsis led to increased resistance to salt stress and enhanced sensitivity to ABA (Cheng et al., 2020). Besides responses to osmotic stress, VQ proteins regulate responses to extreme temperatures. In banana fruits, MaVQ5 might act as a repressor of MaWRKY26 in activating JA biosynthesis in response to cold stress (Ye et al., 2016). On the other hand, ectopically overexpressed tomato SlVQ6 in Arabidopsis plants decreased thermotolerance (Ding et al., 2019). The main regulatory roles exerted by VQ proteins on plant responses to abiotic stress factors are summarized in Figure 1C.
An important though still mostly unexplored feature of VQ proteins is their subcellular localization. An in silico analysis of subcellular localization for Arabidopsis VQ proteins points to predominantly nuclear localization. However, VQ1 and VQ10 are potentially localized both in nuclei and cytoplasm, and others (VQ3, VQ8, VQ12, VQ19, VQ20, VQ23/SIB1, VQ16/SIB2, and VQ31) both in nuclei and chloroplasts. The nucleus/chloroplast alternative localizations of some VQ proteins may be potentially involved in plastid-nucleus retrograde and anterograde signaling (Unal et al., 2020). On the other hand, nucleo-cytoplasmic shuttling of regulatory proteins is often modulated by PTMs. Although phosphorylation of VQ proteins has been documented (Pecher et al., 2014; Weyhe et al., 2014), many other still unknown PTMs might regulate the subcellular localization and dynamics of VQ proteins. All VQ proteins but VQ3 might be potentially ubiquitinated and acetylated in K residues. In turn, only some are predicted to be sumoylated in K, palmitoylated or S-nitrosylated in C residues, and nitrated in Y. VQ6, VQ7, VQ8, VQ9, and VQ12 are predicted to be both S-nitrosylated and palmitoylated in the same C residue at the N-terminus of the proteins, thus suggesting both PTMs compete for the same sites. These alternative PTMs may be critical to determine the subcellular localization and transcriptional activity of these VQ proteins. More work will be needed to support this hypothesis and to clarify whether PTMs can determine the fate, localization, and function of VQ proteins.
Analysis of public repositories of transcriptome data allowed proposing processes potentially regulated by VQ proteins. Gene Ontology categories enrichment suggests that a significant number of Arabidopsis VQ genes were upregulated under ozone-triggered oxidative stress and differentially expressed in response to low oxygen availability. Molecular oxygen and their metabolites, mainly reactive oxygen species (ROS), have gained relevance lately as key signaling molecules in plant development and responses to stress (Van Breusegem and Dat, 2006; Suzuki etal., 2012; van Dongen and Liacausi et al., 2015; Choudhary et al., 2020; Dogra and Kim, 2020; Fichman and Mittler, 2020; Weits et al., 2020). Ozone has been used as a tool to study the role of ROS in cell death and defense signaling as well as in regulating gene expression (Vainonen and Kangasjärvi, 2015). The analysis of the differentially expressed transcriptome in ozone-treated Arabidopsis plants (Xu et al., 2015) allowed identifying that 56% of the VQ genes (19 out of 34) and 64% of the WRKY genes (48 out of 75) were upregulated by ozone (Figure 2). These data suggest that ozone seems to extensively activate VQ and WRKY genes, thus suggesting that distinct VQ-WRKY complexes might regulate plant responses to ROS.
Figure 2. Regulation of Arabidopsis VQ proteins and WRKY transcription factors encoding genes in response to ozone-triggered Reactive Oxygen Species (ROS) production (Ozone), hypoxia (Hypox.), and nitric oxide (NO) treatment (NO). Upregulated (magenta) and down-regulated (blue) transcripts identified in ozone-treated Col-0 plants (Xu et al., 2015), in response to hypoxia and re-oxygenation after hypoxia (Lee and Bailey-Serres, 2019), and in plants exposed to a NO pulse (Castillo et al., 2018; León et al., 2020). Genes marked with red asterisks are upregulated by ozone, hypoxia and NO.
Plants usually grow and develop in 21% O2 normoxic environment. However, plants are sometimes exposed to hypoxic conditions and do not have specific O2 transporters, like hemoglobin in animals, which allow transport between different plant organs or tissues. Instead, plants rely on diffusion between cells or in passive transport through vascular tissue as oxygen transport mechanisms (Armstrong et al., 2006). Importantly, plants contain tissues and organs such as root internal cells, apical meristems, or fruits, where different physical or metabolic barriers preclude oxygen diffusion, thus causing hypoxic niches (Considine et al., 2017; Weits et al., 2019; Labandera et al., 2020; Mira et al., 2020). On the other hand, hypoxia may be imposed by heavy rainfall and the subsequent flooding of lands, which maintain plants transiently submerged or waterlogged (Voesenek and Bailey-Serres, 2015). When water recedes, hypoxic plants undergo a rapid re-oxygenation that lead to the production and metabolism of ROS and NO. A combined analysis of transcriptome data on exogenous NO treatment (Castillo et al., 2018; León et al., 2020) and in response to hypoxia and re-oxygenation after hypoxia (Lee and Bailey-Serres, 2019) allows identifying a cluster of NO-regulated VQ protein encoding genes that were upregulated and downregulated by hypoxia and re-oxygenation after hypoxia, respectively (Figure 2). A similar analysis focusing on WRKY genes allowed also identifying a cluster of four WRKY genes that were upregulated by hypoxia and NO, and downregulated upon re-oxygenation (Figure 2). Five genes of that VQ cluster (VQ1, VQ10, VQ24, VQ27, and VQ32) and the four WRKY genes (WRKY18, WRKY33, WRKY40, and WRKY75) were also upregulated under ozone treatment (Figure 2). Altogether, these data suggest that some VQ proteins, likely in association to some WRKY transcription factors, may play relevant roles in responses to changes in oxygen availability, ROS and NO in plants. VQ-WRKY regulatory actions might be exerted in a combinatorial way, so that the elucidation of the dynamics and relative VQ-WRKY affinities will be essential to better know the mode of action of these regulatory complexes.
Nitric oxide might be the potential link between VQ-WRKY modules and the responses to oxidative stress, hypoxia, and other NO-regulated processes. Plants accumulate NO in response to ozone (Mahalingam et al., 2006; Ahlfors et al., 2009; Pasqualini et al., 2012; Bison et al., 2018; Li et al., 2018), and because of the mitochondrial electron chain using nitrite as electron acceptor also under oxygen limiting conditions (Gupta et al., 2018). The subset of VQ and WRKY genes that are upregulated in plants under oxidative stress, hypoxia, and treatment with NO may represent components of potential VQ-WRKY core complexes controlling downstream gene expression and metabolic alterations in a wide range of physiological processes (Figure 2). Interactions between VQ1 and VQ10 with WRKY33, VQ24 with WRKY75, WRKY18 with WRKY33 and WRKY40, have been all reported (Xu et al., 2006; Pandey et al., 2010; Arabidopsis Interactome Mapping Consortium, 2011; Cheng et al., 2012; Birkenbihl et al., 2017; Abeysinghe et al., 2019) in stress-related responses. Developmental programs such as leaf senescence are also regulated by NO and ROS, and they represent potential new targets for VQ-WRKY protein regulation. The relationship of the senescence process and the production of NO is somehow controversial as both positive or negative correlation has been reported depending upon the organ or being natural or dark-induced (Mishina et al., 2007; Ma et al., 2010; Niu and Guo, 2012; Liu and Guo, 2013; Du et al., 2014; Bruand and Meilhoc, 2019). Linked to ROS and NO action, ZmVQ52 associated to WRKY proteins regulate leaf senescence in maize (Yu et al., 2019). Moreover, around 32% of the VQ genes and more than half of the WRKY genes were upregulated in Arabidopsis senescing leaves (Schmid et al., 2005). Some of these genes were also differentially expressed in leaves in the transition from mature to senescent leaves (Woo et al., 2016).
Proteins containing the VQ motif have been studied during the last 20years with increasing attention being gained during the last decade. Despite the regulatory functions of some VQ proteins have been characterized in development and stress responses, most of the processes regulated by VQ proteins remain unknown. Importantly, the modes of action by which VQ proteins regulate these processes are still incompletely understood though their functional associations to WRKY factors seem to be important. Nevertheless, the identification of the VQ-WRKY complexes and the characterization of their affinities in different processes remain yet to be analyzed. Furthermore, the functional connection between VQ proteins and gasotransmitters such as O2 and NO opens up multiple developmental and stress-related processes potentially regulated by VQ proteins. Among them, hypoxia-triggered responses and subsequent re-oxygenation recovery are very relevant to modulate the tolerance of plants to submergence and waterlogging in flooded lands, a stressful condition becoming increasingly common in the context of climate change. On the other hand, some VQ proteins and their WRKY partners are also regulated by NO likely through NO-triggered PTMs that remain yet to be identified. Future questions that need to be also addressed include the elucidation of new WRKY-independent VQ protein regulatory functions that will benefit from the combination of genetic and omics approaches.
JL wrote the article, conceived the project and supervised co-authors draft writing. BG and M-CC had an equal contribution in collecting information. All authors contributed to the article and approved the submitted version.
Work on hypoxia in the JL laboratory is supported by grant from Ministerio de Ciencia e Innovación BIO2017-82945-P. BG holds an FPI contract (PRE2018-086290) linked to that grant.
The authors declare that the research was conducted in the absence of any commercial or financial relationships that could be construed as a potential conflict of interest.
We acknowledge support of the publication fee by the CSIC Open Access Publication Support Initiative through its Unit of Information Resources for Research (URICI).
Abeysinghe, J. K., Lam, K. M., and Ng, D. W. (2019). Differential regulation and interaction of homoeologous WRKY18 and WRKY40 in Arabidopsis allotetraploids and biotic stress responses. Plant J. 97, 352–367. doi: 10.1111/tpj.14124
Ahlfors, R., Brosche, M., Kollist, H., and Kangasjarvi, J. (2009). Nitric oxide modulates ozone-induced cell death, hormone biosynthesis and gene expression in Arabidopsis thaliana. Plant J. 58, 1–12. doi: 10.1111/j.1365-313X.2008.03756.x
Ali, M. R. M., Uemura, T., Ramadan, A., Adachi, K., Nemoto, K., Nozawa, A., et al. (2019). The Ring-type E3 ubiquitin ligase JUL1 targets the VQ-motif protein JAV1 to coordinate jasmonate signaling. Plant Physiol. 179, 1273–1284. doi: 10.1104/pp.18.00715
Andreasson, E., Jenkins, T., Brodersen, P., Thorgrimsen, S., Petersen, N. H., Zhu, S., et al. (2005). The MAP kinase substrate MKS1 is a regulator of plant defense responses. EMBO J. 24, 2579–2589. doi: 10.1038/sj.emboj.7600737
Arabidopsis Interactome Mapping Consortium (2011). Evidence for network evolution in an Arabidopsis interactome map. Science 333, 601–607. doi: 10.1126/science.1203877
Armstrong, J., Jones, R. E., and Armstrong, W. (2006). Rhizome phyllosphere oxygenation in Phragmites and other species in relation to redox potential, convective gas flow, submergence and aeration pathways. New Phytol. 172, 719–731. doi: 10.1111/j.1469-8137.2006.01878.x
Birkenbihl, R. P., Kracher, B., Roccaro, M., and Somssich, I. E. (2017). Induced genome-wide binding of three Arabidopsis WRKY transcription factors during early MAMP-triggered immunity. Plant Cell 29, 20–38. doi: 10.1105/tpc.16.00681
Bison, J. V., Cardoso-Gustavson, P., de Moraes, R. M., da Silva Pedrosa, G., Cruz, L. S., Freschi, L., et al. (2018). Volatile organic compounds and nitric oxide as responses of a Brazilian tropical species to ozone, the emission profile of young and mature leaves. Environ. Sci. Pollut. Res. 25, 3840–3848. doi: 10.1007/s11356-017-0744-1
Bruand, C., and Meilhoc, E. (2019). Nitric oxide in plants, pro- or anti-senescence. J. Exp. Bot. 70, 4419–4427. doi: 10.1093/jxb/erz117
Castillo, M. C., Coego, A., Costa-Broseta, Á., and León, J. (2018). Nitric oxide responses in Arabidopsis hypocotyls are mediated by diverse phytohormone pathways. J. Exp. Bot. 69, 5265–5278. doi: 10.1093/jxb/ery286
Chen, J., Wang, H., Li, Y., Pan, J., Hu, Y., and Yu, D. (2018). Arabidopsis VQ10 interacts with WRKY8 to modulate basal defense against Botrytis cinerea. J. Integr. Plant Biol. 60, 956–969. doi: 10.1111/jipb.12664
Cheng, X., Wang, Y., Xiong, R., Gao, Y., Yan, H., and Xiang, Y. (2020). A Moso bamboo gene VQ28 confers salt tolerance to transgenic Arabidopsis plants. Planta 251:99. doi: 10.1007/s00425-020-03391-5
Cheng, Y., Zhou, Y., Yang, Y., Chi, Y. J., Zhou, J., Chen, J. Y., et al. (2012). Structural and functional analysis of VQ motif-containing proteins in Arabidopsis as interacting proteins of WRKY transcription factors. Plant Physiol. 159, 810–825. doi: 10.1104/pp.112.196816
Choudhary, A., Kumar, A., and Kaur, N. (2020). ROS and oxidative burst, roots in plant development. Plant Divers. 42, 33–43. doi: 10.1016/j.pld.2019.10.002
Considine, M. J., Diaz-Vivancos, P., Kerchev, P., Signorelli, S., Agudelo-Romero, P., Gibbs, D. J., et al. (2017). Learning to breathe, developmental phase transitions in oxygen status. Trends Plant Sci. 22, 140–153. doi: 10.1016/j.tplants.2016.11.013
Ding, H., Yuan, G., Mo, S., Qian, Y., Wu, Y., Chen, Q., et al. (2019). Genome-wide analysis of the plant-specific VQ motif-containing proteins in tomato (Solanum lycopersicum) and characterization of SlVQ6 in thermotolerance. Plant Physiol. Biochem. 143, 29–39. doi: 10.1016/j.plaphy.2019.08.019
Dogra, V., and Kim, C. (2020). Singlet oxygen metabolism, from genesis to signaling. Front. Plant Sci. 10:1640. doi: 10.3389/fpls.2019.01640
Dong, Q., Zhao, S., Duan, D., Tian, Y., Wang, Y., Mao, K., et al. (2018). Structural and functional analyses of genes encoding VQ proteins in apple. Plant Sci. 272, 208–219. doi: 10.1016/j.plantsci.2018.04.029
Du, J., Li, M., Kong, D., Wang, L., Lv, Q., Wang, J., et al. (2014). Nitric oxide induces cotyledon senescence involving co-operation of the NES1/MAD1 and EIN2-associated ORE1 signalling pathways in Arabidopsis. J. Exp. Bot. 65, 4051–4063. doi: 10.1093/jxb/ert429
Fichman, Y., and Mittler, R. (2020). Rapid systemic signaling during abiotic and biotic stresses, is the ROS wave master of all trades? Plant J. 102, 887–896. doi: 10.1111/tpj.14685
Garcia, D., Saingery, V., Chambrier, P., Mayer, U., Jurgens, G., and Berger, F. (2003). Arabidopsis haiku mutants reveal new controls of seed size by endosperm. Plant Physiol. 131, 1661–1670. doi: 10.1104/pp.102.018762
Gargul, J. M., Mibus, H., and Serek, M. (2015). Manipulation of MKS1 gene expression affects Kalanchoë blossfeldiana and Petunia hybrida phenotypes. Plant Biotechnol. J. 13, 51–61. doi: 10.1111/pbi.12234
Garrido-Gala, J., Higuera, J. J., Muñoz-Blanco, J., Amil-Ruiz, F., and Caballero, J. L. (2019). The VQ motif-containing proteins in the diploid and octoploid strawberry. Sci. Rep. 9:4942. doi: 10.1038/s41598-019-41210-4
Guo, J., Chen, J., Yang, J., Yu, Y., Yang, Y., and Wang, W. (2018). Identification, characterization and expression analysis of the VQ motif-containing gene family in tea plant (Camellia sinensis). BMC Genomics 19:710. doi: 10.1186/s12864-018-5107-x
Gupta, K. J., Kumari, A., Florez-Sarasa, I., Fernie, A. R., and Igamberdiev, A. U. (2018). Interaction of nitric oxide with the components of the plant mitochondrial electron transport chain. J. Exp. Bot. 69, 3413–3424. doi: 10.1093/jxb/ery119
Hu, Y., Chen, L., Wang, H., Zhang, L., Wang, F., and Yu, D. (2013b). Arabidopsis transcription factor WRKY8 functions antagonistically with its interacting partner VQ9 to modulate salinity stress tolerance. Plant J. 74, 730–745. doi: 10.1111/tpj.12159
Hu, P., Zhou, W., Cheng, Z., Fan, M., Wang, L., and Xie, D. (2013a). JAV1 controls jasmonate-regulated plant defense. Mol. Cell 50, 504–515. doi: 10.1016/j.molcel.2013.04.027
Jiang, S. Y., Sevugan, M., and Ramachandran, S. (2018). Valine-glutamine (VQ) motif coding genes are ancient and non-plant-specific with comprehensive expression regulation by various biotic and abiotic stresses. BMC Genomics 19:342. doi: 10.1186/s12864-018-4733-7
Jiang, Y., and Yu, D. (2016). The WRKY57 transcription factor affects the expression of Jasmonate ZIM-domain genes transcriptionally to compromise Botrytis cinerea resistance. Plant Physiol. 171, 2771–2782. doi: 10.1104/pp.16.00747
Jiao, Z., Sun, J., Wang, C., Dong, Y., Xiao, S., Gao, X., et al. (2018). Genome-wide characterization, evolutionary analysis of WRKY genes in Cucurbitaceae species and assessment of its roles in resisting to powdery mildew disease. PLoS One 13:e0199851. doi: 10.1371/journal.pone.0199851
Jing, Y., and Lin, R. (2015). The VQ motif-containing protein family of plant-specific transcriptional regulators. Plant Physiol. 169, 371–378. doi: 10.1104/pp.15.00788
Kim, D. Y., Kwon, S. I., Choi, C., Lee, H., Ahn, I., Park, S. R., et al. (2013). Expression analysis of rice VQ genes in response to biotic and abiotic stresses. Gene 529, 208–214. doi: 10.1016/j.gene.2013.08.023
Labandera, A. M., Tedds, H. M., Bailey, M., Sprigg, C., Etherington, R. D., Akintewe, O., et al. (2020). The PRT6 N-degron pathway restricts VERNALIZATION 2 to endogenous hypoxic niches to modulate plant development. New Phytol. 229, 126–139. doi: 10.1111/nph.16477
Lai, Z., Li, Y., Wang, F., Cheng, Y., Fan, B., Yu, J. Q., et al. (2011). Arabidopsis sigma factor binding proteins are activators of the WRKY33 transcription factor in plant defense. Plant Cell 23, 3824–3841. doi: 10.1105/tpc.111.090571
Le Berre, J. Y., Gourgues, M., Samans, B., Keller, H., Panabières, F., and Attard, A. (2017). Transcriptome dynamic of Arabidopsis roots infected with Phytophthora parasitica identifies VQ29, a gene induced during the penetration and involved in the restriction of infection. PLoS One 12:e0190341. doi: 10.1371/journal.pone.0190341
Lee, T. A., and Bailey-Serres, J. (2019). Integrative analysis from the Epigenome to Translatome uncovers patterns of dominant nuclear regulation during transient stress. Plant Cell 31, 2573–2595. doi: 10.1105/tpc.19.00463
Lei, R., Li, X., Ma, Z., Lv, Y., Hu, Y., and Yu, D. (2017). Arabidopsis WRKY2 and WRKY34 transcription factors interact with VQ20 protein to modulate pollen development and function. Plant J. 91, 962–976. doi: 10.1111/tpj.13619
Lei, R., Ma, Z., and Yu, D. (2018). WRKY2/34-VQ20 modules in Arabidopsis thaliana negatively regulate expression of a trio of related MYB transcription factors during pollen development. Front. Plant Sci. 9:331. doi: 10.3389/fpls.2018.00331
León, J., Costa-Broseta, Á., and Castillo, M. C. (2020). RAP2.3 negatively regulates nitric oxide biosynthesis and related responses through a rheostat-like mechanism in Arabidopsis. J. Exp. Bot. 71, 3157–3171. doi: 10.1093/jxb/eraa069
Li, Y., Jing, Y., Li, J., Xu, G., and Lin, R. (2014b). Arabidopsis VQ MOTIF-CONTAINING PROTEIN29 represses seedling deetiolation by interacting with PHYTOCHROME-INTERACTING FACTOR1. Plant Physiol. 164, 2068–2080. doi: 10.1104/pp.113.234492
Li, N., Li, X., Xiao, J., and Wang, S. (2014a). Comprehensive analysis of VQ motif-containing gene expression in rice defense responses to three pathogens. Plant Cell Rep. 33, 1493–1505. doi: 10.1007/s00299-014-1633-4
Li, X., Qin, R., Du, Q., Cai, L., Hu, D., Du, H., et al. (2020). Knockdown of GmVQ58 encoding a VQ motif-containing protein enhances soybean resistance to the common cutworm (Spodoptera litura Fabricius). J. Exp. Bot. 71, 3198–3210. doi: 10.1093/jxb/eraa095
Li, C., Song, Y., Guo, L., Gu, X., Muminov, M. A., and Wang, T. (2018). Nitric oxide alleviates wheat yield reduction by protecting photosynthetic system from oxidation of ozone pollution. Environ. Pollut. 236, 296–303. doi: 10.1016/j.envpol.2018.01.093
Ling, L., Qu, Y., Zhu, J., Wang, D., and Guo, C. (2020). Genome-wide identification and expression analysis of the VQ gene family in Cicer arietinum and Medicago truncatula. PeerJ 8:e8471. doi: 10.7717/peerj.8471
Liu, F., and Guo, F. Q. (2013). Nitric oxide deficiency accelerates chlorophyll breakdown and stability loss of thylakoid membranes during dark-induced leaf senescence in Arabidopsis. PLoS One 8:e56345. doi: 10.1371/journal.pone.0085660
Liu, C., Liu, H., Zhou, C., and Timko, M. P. (2020). Genome-wide identification of the VQ protein gene family of tobacco (Nicotiana tabacum L.) and analysis of its expression in response to Phytohormones and abiotic and biotic stresses. Genes 11:284. doi: 10.3390/genes11030284
Ma, W., Smigel, A., Walker, R. K., Moeder, W., Yoshioka, K., and Berkowitz, G. A. (2010). Leaf senescence signaling, the Ca2+-conducting Arabidopsis cyclic nucleotide gated channel2 acts through nitric oxide to repress senescence programming. Plant Physiol. 154, 733–743. doi: 10.1104/pp.110.161356
Mahalingam, R., Jambunathan, N., Gunjan, S. K., Faustin, E., Weng, H., and Ayoubi, P. (2006). Analysis of oxidative signalling induced by ozone in Arabidopsis thaliana. Plant Cell Environ. 29, 1357–1371. doi: 10.1111/j.1365-3040.2006.01516.x
Mira, M. M., El-Khateeb, E. A., Gaafar, R. M., Igamberdiev, A. U., Hill, R. D., and Stasolla, C. (2020). Stem cell fate in hypoxic root apical meristems is influenced by phytoglobin expression. J. Exp. Bot. 71, 1350–1362. doi: 10.1093/jxb/erz410
Mishina, T. E., Lamb, C., and Zeier, J. (2007). Expression of a nitric oxide degrading enzyme induces a senescence programme in Arabidopsis. Plant Cell Environ. 30, 39–52. doi: 10.1111/j.1365-3040.2006.01604.x
Morikawa, K., Shiina, T., Murakami, S., and Toyoshima, Y. (2002). Novel nuclear encoded proteins interacting with a plastid sigma factor, Sig1, in Arabidopsis thaliana. FEBS Lett. 514, 300–304. doi: 10.1016/S0014-5793(02)02388-8
Niu, Y. H., and Guo, F. Q. (2012). Nitric oxide regulates dark-induced leaf senescence through EIN2 in Arabidopsis. J. Integr. Plant Biol. 54, 516–525. doi: 10.1111/j.1744-7909.2012.01140.x
Pan, J., Wang, H., Hu, Y., and Yu, D. (2018). Arabidopsis VQ18 and VQ26 proteins interact with ABI5 transcription factor to negatively modulate ABA response during seed germination. Plant J. 95, 529–544. doi: 10.1111/tpj.13969
Pandey, S. P., Roccaro, M., Schön, M., Logemann, E., and Somssich, I. E. (2010). Transcriptional reprogramming regulated by WRKY18 and WRKY40 facilitates powdery mildew infection of Arabidopsis. Plant J. 64, 912–923. doi: 10.1111/j.1365-313X.2010.04387.x
Pasqualini, S., Reale, L., Calderini, O., Pagiotti, R., and Ederli, L. (2012). Involvement of protein kinases and calcium in the NO-signalling cascade for defence-gene induction in ozonated tobacco plants. J. Exp. Bot. 63, 4485–4496. doi: 10.1093/jxb/ers133
Pecher, P., Eschen-Lippold, L., Herklotz, S., Kuhle, K., Naumann, K., Bethke, G., et al. (2014). The Arabidopsis thaliana mitogen-activated protein kinases MPK3 and MPK6 target a subclass of ‘VQ-motif’-containing proteins to regulate immune responses. New Phytol. 203, 592–606. doi: 10.1111/nph.12817
Perruc, E., Charpenteau, M., Ramirez, B. C., Jauneau, A., Galaud, J. P., Ranjeva, R., et al. (2004). A novel calmodulin-binding protein functions as a negative regulator of osmotic stress tolerance in Arabidopsis thaliana seedlings. Plant J. 38, 410–420. doi: 10.1111/j.1365-313X.2004.02062.x
Petersen, K., Qiu, J. L., Lütje, J., Fiil, B. K., Hansen, S., Mundy, J., et al. (2010). Arabidopsis MKS1 is involved in basal immunity and requires an intact N-terminal domain for proper function. PLoS One 5:e14364. doi: 10.1371/journal.pone.0014364
Schmid, M., Davison, T. S., Henz, S. R., Pape, U. J., Demar, M., Vingron, M., et al. (2005). A gene expression map of Arabidopsis thaliana development. Nat. Genet. 37, 501–506. doi: 10.1038/ng1543
Song, W., Zhao, H., Zhang, X., Lei, L., and Lai, J. (2016). Genome-wide identification of VQ motif-containing proteins and their expression profiles under abiotic stresses in maize. Front. Plant Sci. 6:1177. doi: 10.3389/fpls.2015.01177
Suzuki, N., Koussevitzky, S., Mittler, R., and Miller, G. (2012). ROS and redox signalling in the response of plants to abiotic stress. Plant Cell Environ. 35, 259–270. doi: 10.1111/j.1365-3040.2011.02336.x
Uji, Y., Kashihara, K., Kiyama, H., Mochizuki, S., Akimitsu, K., and Gomi, K. (2019). Jasmonic acid-induced VQ-motif-containing protein OsVQ13 influences the OsWRKY45 Signaling pathway and grain size by associating with OsMPK6 in rice. Int. J. Mol. Sci. 20:2917. doi: 10.3390/ijms20122917
Unal, D., García-Caparrós, P., Kumar, V., and Dietz, K. J. (2020). Chloroplast-associated molecular patterns as concept for fine-tuned operational retrograde signalling. Philos. Trans. R. Soc. Lond. B Biol. Sci. 375:20190443. doi: 10.1098/rstb.2019.0443
Vainonen, J. P., and Kangasjärvi, J. (2015). Plant signalling in acute ozone exposure. Plant Cell Environ. 38, 240–252. doi: 10.1111/pce.12273
Van Breusegem, F., and Dat, J. F. (2006). Reactive oxygen species in plant cell death. Plant Physiol. 141, 384–390. doi: 10.1104/pp.106.078295
van Dongen, J. T., and Licausi, F. (2015). Oxygen sensing and signaling. Annu. Rev. Plant Biol. 66, 345–367. doi: 10.1146/annurev-arplant-043014-114813
Voesenek, L. A., and Bailey-Serres, J. (2015). Flood adaptive traits and processes, an overview. New Phytol. 206, 57–73. doi: 10.1111/nph.13209
Wang, A., Garcia, D., Zhang, H., Feng, K., Chaudhury, A., Berger, F., et al. (2010). The VQ motif protein IKU1 regulates endosperm growth and seed size in Arabidopsis. Plant J. 63, 670–679. doi: 10.1111/j.1365-313X.2010.04271.x
Wang, H., Hu, Y., Pan, J., and Yu, D. (2015b). Arabidopsis VQ motif-containing proteins VQ12 and VQ29 negatively modulate basal defense against Botrytis cinerea. Sci. Rep. 5:14185. doi: 10.1038/srep14185
Wang, Y., Liu, H., Zhu, D., Gao, Y., Yan, H., and Xiang, Y. (2017). Genome-wide analysis of VQ motif-containing proteins in Moso bamboo (Phyllostachys edulis). Planta 246, 165–181. doi: 10.1007/s00425-017-2693-9
Wang, M., Vannozzi, A., Wang, G., Zhong, Y., Corso, M., Cavallini, E., et al. (2015a). A comprehensive survey of the grapevine VQ gene family and its transcriptional correlation with WRKY proteins. Front. Plant Sci. 6:417. doi: 10.3389/fpls.2015.00417
Wang, X., Zhang, H., Sun, G., Jin, Y., and Qiu, L. (2014). Identification of active VQ motif-containing genes and the expression patterns under low nitrogen treatment in soybean. Gene 543, 237–243. doi: 10.1016/j.gene.2014.04.012
Weits, D. A., Kunkowska, A. B., Kamps, N. C. W., Portz, K. M. S., Packbier, N. K., Nemec Venza, Z., et al. (2019). An apical hypoxic niche sets the pace of shoot meristem activity. Nature 569, 714–717. doi: 10.1038/s41586-019-1203-6
Weits, D. A., van Dongen, J. T., and Licausi, F. (2020). Molecular oxygen as a signaling component in plant development. New Phytol.. 229, 24–35. doi: 10.1111/nph.16424
Weyhe, M., Eschen-Lippold, L., Pecher, P., Scheel, D., and Lee, J. (2014). Ménage à trois, the complex relationships between mitogen-activated protein kinases, WRKY transcription factors, and VQ-motif-containing proteins. Plant Signal. Behav. 9:e29519. doi: 10.4161/psb.29519
Woo, H. R., Koo, H. J., Kim, J., Jeong, H., Yang, J. O., Lee, I. H., et al. (2016). Programming of plant leaf senescence with temporal and inter-organellar coordination of Transcriptome in Arabidopsis. Plant Physiol. 171, 452–467. doi: 10.1104/pp.15.01929
Xie, Y. D., Li, W., Guo, D., Dong, J., Zhang, Q., Fu, Y., et al. (2010). The Arabidopsis gene SIGMA FACTOR-BINDING PROTEIN 1 plays a role in the salicylate- and jasmonate-mediated defence responses. Plant Cell Environ. 33, 828–839. doi: 10.1111/j.1365-3040.2009.02109.x
Xu, X., Chen, C., Fan, B., and Chen, Z. (2006). Physical and functional interactions between pathogen-induced Arabidopsis WRKY18, WRKY40, and WRKY60 transcription factors. Plant Cell 18, 1310–1326. doi: 10.1105/tpc.105.037523
Xu, E., Vaahtera, L., and Brosché, M. (2015). Roles of defense hormones in the regulation of ozone-induced changes in gene expression and cell death. Mol. Plant 8, 1776–1794. doi: 10.1016/j.molp.2015.08.008
Yan, C., Fan, M., Yang, M., Zhao, J., Zhang, W., Su, Y., et al. (2018). Injury activates Ca2+/Calmodulin-dependent phosphorylation of JAV1-JAZ8-WRKY51 complex for jasmonate biosynthesis. Mol. Cell 70, 136–149.e7. doi: 10.1016/j.molcel.2018.03.013
Yan, H., Wang, Y., Hu, B., Qiu, Z., Zeng, B., and Fan, C. (2019). Genome-wide characterization, evolution, and expression profiling of VQ gene family in response to Phytohormone treatments and abiotic stress in Eucalyptus grandis. Int. J. Mol. Sci. 20:1765. doi: 10.3390/ijms20071765
Ye, Y. J., Xiao, Y. Y., Han, Y. C., Shan, W., Fan, Z. Q., Xu, Q. G., et al. (2016). Banana fruit VQ motif-containing protein5 represses cold-responsive transcription factor MaWRKY26 involved in the regulation of JA biosynthetic genes. Sci. Rep. 6:23632. doi: 10.1038/srep23632
Yu, T., Lu, X., Bai, Y., Mei, X., Guo, Z., Liu, C., et al. (2019). Overexpression of the maize transcription factor ZmVQ52 accelerates leaf senescence in Arabidopsis. PLoS One 14:e0221949. doi: 10.1371/journal.pone.0221949
Zhang, G., Wang, F., Li, J., Ding, Q., Zhang, Y., Li, H., et al. (2015). Genome-wide identification and analysis of the VQ motif-containing protein family in Chinese cabbage (Brassica rapa L. ssp. Pekinensis). Int. J. Mol. Sci. 16, 28683–28704. doi: 10.3390/ijms161226127
Zhong, Y., Guo, C., Chu, J., Liu, H., and Cheng, Z. M. (2018). Microevolution of the VQ gene family in six species of Fragaria. Genome 61, 49–57. doi: 10.1139/gen-2017-0038
Keywords: hypoxia, nitric oxide, oxidative stress, oxygen, valine-glutamine proteins, WRKY transcription factors
Citation: León J, Gayubas B and Castillo M-C (2021) Valine-Glutamine Proteins in Plant Responses to Oxygen and Nitric Oxide. Front. Plant Sci. 11:632678. doi: 10.3389/fpls.2020.632678
Received: 23 November 2020; Accepted: 31 December 2020;
Published: 25 January 2021.
Edited by:
Luisa M. Sandalio, Estación Experimental del Zaidín (EEZ), SpainReviewed by:
Alexandro Cagliari, Universidade Estadual do Rio Grande do Sul, BrazilCopyright © 2021 León, Gayubas and Castillo. This is an open-access article distributed under the terms of the Creative Commons Attribution License (CC BY). The use, distribution or reproduction in other forums is permitted, provided the original author(s) and the copyright owner(s) are credited and that the original publication in this journal is cited, in accordance with accepted academic practice. No use, distribution or reproduction is permitted which does not comply with these terms.
*Correspondence: José León, amxlb25AaWJtY3AudXB2LmVz
Disclaimer: All claims expressed in this article are solely those of the authors and do not necessarily represent those of their affiliated organizations, or those of the publisher, the editors and the reviewers. Any product that may be evaluated in this article or claim that may be made by its manufacturer is not guaranteed or endorsed by the publisher.
Research integrity at Frontiers
Learn more about the work of our research integrity team to safeguard the quality of each article we publish.