- Department of Cell Biology, Centre of the Region Haná for Biotechnological and Agricultural Research, Faculty of Science, Palacký University, Olomouc, Czechia
Reactive oxygen species (ROS) are signaling molecules essential for plant responses to abiotic and biotic stimuli as well as for multiple developmental processes. They are produced as byproducts of aerobic metabolism and are affected by adverse environmental conditions. The ROS content is controlled on the side of their production but also by scavenging machinery. Antioxidant enzymes represent a major ROS-scavenging force and are crucial for stress tolerance in plants. Enzymatic antioxidant defense occurs as a series of redox reactions for ROS elimination. Therefore, the deregulation of the antioxidant machinery may lead to the overaccumulation of ROS in plants, with negative consequences both in terms of plant development and resistance to environmental challenges. The transcriptional activation of antioxidant enzymes accompanies the long-term exposure of plants to unfavorable environmental conditions. Fast ROS production requires the immediate mobilization of the antioxidant defense system, which may occur via retrograde signaling, redox-based modifications, and the phosphorylation of ROS detoxifying enzymes. This review aimed to summarize the current knowledge on signaling processes regulating the enzymatic antioxidant capacity of plants.
Introduction
Reactive oxygen species (ROS), as unavoidable byproducts of metabolism, have important signaling roles in living organisms under optimal and adverse environmental conditions (Apel and Hirt, 2004; Baxter et al., 2014; Waszczak et al., 2018). ROS are produced from atmospheric oxygen by its partial monovalent reduction, which occurs in the presence of electron donors. Plant ROS are generated mainly by electron transport chains in chloroplasts (Pospíšil, 2016; Foyer, 2018) and mitochondria (Gleason et al., 2011) as well as during photorespiration in peroxisomes (del Río et al., 2006; del Río and López-Huertas, 2016). Apoplastic ROS are produced by plasma membrane-localized NADPH oxidase [NOX, in plants encoded by RESPIRATORY BURST OXIDASE HOMOLOG (RBOH) genes; Sagi and Fluhr, 2006], oxalate oxidase (Voothuluru and Sharp, 2013), or by the degradation of spermidine by polyamine oxidase (Geilfus et al., 2015). Apoplastic peroxidases also possess ROS generating capacity (Bindschedler et al., 2006). Only four ROS, namely singlet oxygen (1O2), superoxide (O2·−), hydrogen peroxide (H2O2), and hydroxyl radical (OH·), are more abundant and stable. They quickly interconvert, thus providing a high level of functional variability. However, they differ in their stability, reactivity, and ability to be transported across membranes. H2O2 is the most stable ROS transported actively across membranes by aquaporins (Miller et al., 2010; Mittler, 2017; Smirnoff and Arnaud, 2019).
Depending on the generated ROS concentration, severity of stress, antioxidant capacity, and cellular energetic status, different cellular and physiological outcomes may be obtained. In small concentrations, ROS exert signaling functions, leading to the expression of stress-responsive genes. Upon extensive accumulation, ROS reactivity may cause damaging oxidative effects on lipids, nucleic acids, and proteins, eventually resulting in cell death. Moreover, ROS are involved in strictly regulated programmed cell death (Lehmann et al., 2015; Petrov et al., 2015). A very important feature of ROS signaling is that it can be propagated from cell to cell and transduce signals for long distances in a process known as the ROS wave (Mittler et al., 2011). This is mediated by the interplay between NOX, calcium (Ca2+) channels, and oxidative stress-induced Ca2+ fluxes (Gilroy et al., 2016). The ROS wave, together with other hormone- or electric signal-mediated signaling mechanisms, is implicated in systemic acquired acclimation, in which the locally generated stress signal is transported into sites not being directly affected by the stress. Systemic acquired acclimation is accompanied by rapid and dramatic transcriptional reprogramming (Zandalinas et al., 2019, 2020).
In addition to plant stress responses, ROS are widely involved in developmental processes (Considine and Foyer, 2014; Xia et al., 2015; Mhamdi and Van Breusegem, 2018), regulating morphogenetic processes in the close interplay of phytohormones such as auxins and cytokinins (Xia et al., 2015; Zwack et al., 2016). Interestingly, auxin and abscisic acid (ABA) can promote ROS production by activating NOX (Joo et al., 2001; Schopfer et al., 2002; Pasternak et al., 2007). In turn, ROS may affect auxin levels (Takáč et al., 2016a) and homeostasis leading to altered shoot branching and leaf rosette shapes (Tognetti et al., 2010).
This review aimed to summarize recent findings on the regulation of major ROS-decomposing enzymes. First, we introduce the major antioxidant enzymes and provide an overview of the mechanisms by which ROS affect signaling in plants. Next, we summarize recent knowledge on the redox regulation of major antioxidant enzymes and elaborate on their modulation by mitogen-activated protein kinase (MAPK) and Ca2+ signaling pathways. The transcriptional activation of antioxidant enzymes, including metabolite-driven retrograde signaling, is discussed as well. Finally, we point out the importance of their post-translational regulation by reversible phosphorylation and reactive nitrogen species (RNS).
Characterization of Key Antioxidant Enzymes
Generally, enzymatic antioxidant capacity inevitably contributes to plant survival in adverse conditions, especially when the stress pressure exceeds the mechanisms preventing ROS overaccumulation. The significance of antioxidant enzymes has been documented many times by genetic studies reporting on the positive correlation between the expression of these enzymes and plant stress tolerance. Contrarily, the deregulation of these enzymes is connected with plant hypersensitivity to stress and programmed cell death (De Pinto et al., 2012).
ROS scavenging is performed enzymatic or via non-enzymatic antioxidant defense pathways, which control the regulation of ROS levels through strict compartmentalization (Mignolet-Spruyt et al., 2016; Noctor et al., 2017; Foyer and Noctor, 2020). Non-enzymatic antioxidant defense is mainly mediated by low molecular-weight metabolites such as ascorbate, glutathione, α-tocopherol, carotenoids, and flavonoids (Locato et al., 2017; Smirnoff, 2018; Zechmann, 2018; Muñoz and Munné-Bosch, 2019; Foyer and Noctor, 2020). Superoxide dismutases (SODs), catalases (CATs), ascorbate peroxidases (APXs), dehydroascorbate reductases (DHARs), monodehydroascorbate reductases (MDHARs), and glutathione reductases (GRs) are among the main antioxidant enzyme classes. Furthermore, glutathione peroxidases, peroxidases, and thio-, gluta-, and peroxiredoxins are potent ROS scavengers as well (Dietz, 2011; Kang et al., 2019; Foyer and Noctor, 2020). Within this section, we briefly characterize the key enzymatic antioxidants in plants.
Major enzymatic antioxidants are plastidic, cytosolic, mitochondrial, and peroxisomal SODs, which decompose O2.− to H2O2 (Kliebenstein et al., 1998; Alscher et al., 2002; Pilon et al., 2011). Based on the presence of metal cofactors in their active site, four different SODs are recognized in living organisms, namely FeSOD, MnSOD, NiSOD (not present in higher plants), and Cu/ZnSOD. In the Arabidopsis thaliana genome, three FeSOD (FSD1, FSD2, and FSD3), one MnSOD (MSD1), and three Cu/ZnSOD (CSD1, CSD2, and CSD3; Kliebenstein et al., 1998; Pilon et al., 2011) genes have been identified. Individual SOD isozymes are compartmentalized into mitochondria (MSD1; Morgan et al., 2008), peroxisomes (CSD3; Kliebenstein et al., 1998), cytosol (CSD1 and FSD1; Kliebenstein et al., 1998, Dvořák et al., 2020), the chloroplast stroma (FSD1; Kuo et al., 2013; Dvořák et al., 2020), and thylakoids (CSD2, FSD2, and FSD3; Kliebenstein et al., 1998; Myouga et al., 2008). Recently, it was discovered that FSD1 is also localized to the nucleus (Dvořák et al., 2020). In addition to their antioxidative role during salt, oxidative (Myouga et al., 2008; Shafi et al., 2015; Dvořák et al., 2020), and photooxidative stresses (Myouga et al., 2008; Xing et al., 2013; Gallie and Chen, 2019), SODs also have developmental functions during lateral root growth (Morgan et al., 2008; Dvořák et al., 2020), germination (Dvořák et al., 2020), chloroplast development, and flowering (Rizhsky et al., 2003; Myouga et al., 2008).
CATs are responsible for the detoxification of the overproduced H2O2, which occurs owing to their kinetic properties (Tuzet et al., 2019). As iron-containing homotetrameric proteins, CATs catalyze the decomposition of H2O2 to H2O and O2, predominantly produced during photorespiration. Three genes (CAT1, CAT2, and CAT3) encoding CATs have been found in the Arabidopsis genome. CAT isozymes are localized in peroxisomes (Frugoli et al., 1996; Du et al., 2008) and play important roles under unfavorable conditions for plants. For example, all three CAT isoforms are required for the plant response to photooxidative stress (Vandenabeele et al., 2004; Bueso et al., 2007; Zhang S. et al., 2020). CAT2 is involved in plant responses to heat, heavy metal (Corpas and Barroso, 2017; Ono et al., 2020), cold, and salt stresses (Bueso et al., 2007). CAT3 participates in the drought stress response (Zou et al., 2015), whereas CAT1 is implicated in the drought and salt stress responses (Xing et al., 2007). Surprisingly, H2O2-induced CAT2, likely together with CAT1 and CAT3, generates a signal promoting autophagy-dependent cell death during plant immune responses (Hackenberg et al., 2013; Teh and Hofius, 2014). In addition, tobacco NbCAT1 is relocalized to nuclei after interaction with CRINKLING- AND NECROSIS-INDUCING PROTEIN 63 (CRN63) secreted by Phytophthora sojae under attack as found in the transient assay. This mechanism was reported to regulate pathogen-induced cell death in tobacco (Zhang M. et al., 2015). CATs are involved in root growth (Yang et al., 2019), leaf development and senescence (Mhamdi et al., 2010; Zhang Y. et al., 2020), as well as shoot, ovule, pollen, and seed development (Sharma and Ahmad, 2014; Su et al., 2018; Palma et al., 2020).
Balance in cellular H2O2 levels is also maintained by enzymes of the ascorbate–glutathione cycle, such as APX, MDHAR, DHAR, and GR. APXs, as heme-containing peroxidases, detoxify H2O2 via the electron transfer from ascorbate to form monodehydroascorbate (MDHA) and H2O. The presence of nine putative APX genes has been described in the Arabidopsis genome; nevertheless, the APX4 gene product is lacking H2O2 decomposing activity, and APX7 is annotated as a pseudogene (Granlund et al., 2009). Cytosolic (APX1, APX2 and APX6), chloroplast (stromal sAPX and thylakoid tAPX), peroxisomal (APX3 and APX5) APXs have been recognized in Arabidopsis (Maruta et al., 2012, 2016), while sAPX is targeted also to mitochondria (Chew et al., 2003). Chloroplastic APX isozymes are involved in the water-water cycle, which decomposes H2O2 generated by O2.− dismutation (Huang et al., 2019). They are therefore crucial for photoprotection (Murgia et al., 2004; Kangasjärvi et al., 2008; Maruta et al., 2010). Remarkably, the chloroplastic H2O2 detoxification turns to be inactive in plants depleted of cytosolic APX1 (Davletova et al., 2005a, Pnueli et al., 2003). APX1 is also involved in plant responses to heat and drought stress (Koussevitzky et al., 2008; Vanderauwera et al., 2011) and to wounding (Maruta et al., 2012). The importance of cytosolic APX2 was shown during high light, heat, salinity, and drought stresses using apx2 and apx1/apx2 mutants (Rossel et al., 2006; Suzuki et al., 2013). Additionally, both cytosolic APXs play important roles during cold stress (van Buer et al., 2016). Mutants in peroxisomal APX3 do not display any phenotype upon salt treatment and exposure to low or high temperature (Narendra et al., 2006). Finally, APXs also play essential roles as enzymatic regulators of H2O2 signaling during plant development (Chen et al., 2014; Pandey et al., 2017; Chin et al., 2019).
The reverse reduction of MDHA to ascorbate, catalyzed by MDHAR, occurs in the presence of NAD(P)H as a reductant (Foyer and Noctor, 2011). Overall, five Arabidopsis genes encode six functional proteins of MDHARs (Obara et al., 2002). Cytosolic localization is confirmed for MDHAR2 and 3, whereas MDHAR1 has been found also in peroxisomes. MDHAR4 is located in peroxisomal membrane (Lisenbee et al., 2005; Eubel et al., 2008; Kaur and Hu, 2011). The MDHAR6 gene is expressed in two splicing variants, producing two protein products localized either in mitochondria (MDHAR5) or chloroplasts (MDHAR6; Obara et al., 2002). The overexpression of Arabidopsis MDHAR1 in tobacco leads to increased tolerance to ozone, salt, and osmotic stresses (Eltayeb et al., 2007). The overexpression of cytosolic Acanthus ebracteatus MDHAR in rice confers increased resistance to salt stress and higher germination rate and grain weight (Sultana et al., 2012). A study exploiting the genetic manipulation of MDHAR4 suggests that it is implicated in plant germination, post-germination growth, and possibly in senescence (Eastmond, 2007). MDHAR2 and MDHAR5 play important roles during the interaction of Arabidopsis with plant growth-promoting endophyte Piriformospora indica (Vadassery et al., 2009).
Dehydroascorbate (DHA) is enzymatically reduced by DHAR by using glutathione as an electron donor, which is oxidized to glutathione disulfide. DHARs are soluble monomeric enzymes, and their thiol group participates in the catalyzed reaction. Three functional genes are present in the Arabidopsis genome. Their protein products are localized either in the cytosol (DHAR1, DHAR2) or chloroplasts (DHAR3; Rahantaniaina et al., 2017). Recently, their role in the regulation of ascorbate and glutathione homeostasis was described during plant developmental processes (reviewed in Ding et al., 2020). The overexpression of DHAR1 protects Arabidopsis from methyl viologen-induced oxidative, high temperature, and high light stresses (Ushimaru et al., 2006; Wang et al., 2010; Noshi et al., 2017). DHAR2 has an antioxidant role in plant responses to ozone (Yoshida et al., 2006), drought, salt, and polyethylene glycol (Yin et al., 2010; Eltayeb et al., 2011), whereas DHAR3 is involved in the high light response (Noshi et al., 2016).
The pool of reduced glutathione consumed by DHAR activity is recovered by GR in a NADPH-dependent reaction, which is essential for glutathione homeostasis. Structurally, the GR protein contains a FAD-binding domain, a dimerization domain, and a NADPH-binding domain, crucial for proper enzymatic activity (Berkholz et al., 2008). Two isozymes were described in Arabidopsis, showing dual localization in the cytosol and peroxisomes for GR1 and in chloroplasts and mitochondria for GR2 (Kataya and Reumann, 2010; Marty et al., 2019). GR1 is involved in the tolerance of Arabidopsis to high light (Müller-Schüssele et al., 2020), heavy metals (Guo et al., 2016; Yin et al., 2017), and salt stress (Csiszár et al., 2018). GR2 is involved in methyl viologen-induced oxidative stress (Wang et al., 2019), chilling stress (Kornyeyev et al., 2003), and high light stress (Karpinski et al., 1997) but also in developmental processes such as root growth, root apical meristem maintenance (Yu et al., 2013), embryo development (Marty et al., 2019), and seed germination (Sumugat et al., 2010). In addition, the knockout mutation of GR3 confers salt stress sensitivity in rice (Wu et al., 2015).
Regulation of Antioxidant Enzymes
Plants can percept, transduce, and then translate the ROS signal into appropriate cellular responses. The key consequence of ROS accumulation is the modification of the potential signaling targets [e.g., kinases, transcription factors (TFs), and stress response-related proteins] by their oxidizing properties. ROS can modulate signaling through their capability to affect the protein redox status via the oxidation of methionine residues and thiol groups of cysteines. This leads to the activation/deactivation, structure alteration, and loss-/gain-of-function of ROS targets (Waszczak et al., 2015). Redox-related processes are strictly regulated by such proteins as thio- and glutaredoxins, which can undergo reversible oxidation/reduction and can be activated/inactivated in response to the cellular redox state (Waszczak et al., 2015, 2018). Recently, a redox-based sensing mechanism was introduced for H2O2, including cell-surface H2O2 receptor capable of transducing signal from extracellularly produced ROS into intracellular signaling cascades (Wu et al., 2020). HYDROGEN-PEROXIDE-INDUCED Ca2+ INCREASES 1 (HPCA1) is a membrane-spanning enzyme belonging to a protein family of leucine-rich repeat (LRR) receptor kinases, which percepts apoplastic H2O2 via the oxidation of two pairs of cysteine residues in its extracellular domain, leading to its autophosphorylation. This promotes the acceleration of Ca2+ influx through Ca2+-channels and the subsequent closure of stomata (Wu et al., 2020).
ROS can also cause carbonylation, a type of protein oxidation, where the carbonyl groups (aldehydes and ketones) are attached to protein side chains at proline, arginine, lysine and threonine residues (Yalcinkaya et al., 2019). Carbonylation alters protein stability and might enhance their susceptibility to proteolysis (Dalle-Donne et al., 2003; Suzuki et al., 2010; Ciacka et al., 2020).
Redox perturbations, driven by ROS produced in chloroplasts and mitochondria, are transduced by metabolic signals to activate rapid adaptive mechanisms by retrograde signaling (Chan et al., 2016; Cui et al., 2019). As of late, ROS were also introduced as mediators of retrograde signaling directed from the plastids to the nucleus. Thus, H2O2 generated in plastids is transported to the nucleus to activate the defense gene expression (Exposito-Rodriguez et al., 2017).
In addition, ROS can cross talk with other key secondary messengers, such as Ca2+ and RNS. Owing to their strong oxidation potential, ROS interact with such ubiquitous messengers as nitric oxide (NO), leading to the formation of RNS, including radical nitric oxide (NO·), nitric dioxide (), and nitrate radical () as well as non-radical peroxynitrite (ONOO−), nitrosonium cation (NO+), nitroxyl anion (NO−), nitrous acid (HNO2), and other NOx species involved in plant development, metabolism, (a)biotic stress responses, or stomatal closure (del Río, 2015; Lindermayr and Durner, 2015; Piterková et al., 2015; Farnese et al., 2016; Niu and Liao, 2016). The first evidence of NO and H2O2 interplay was related to cytotoxic effects during plant hypersensitive responses (Delledonne et al., 2001). Generally, the ROS cross talk with RNS is concentration-dependent and organelle- and even microcompartment-specific and might have beneficial or deleterious effects on plant cells (Kohli et al., 2019).
In general, an increased production of ROS caused by various environmental cues rapidly triggers antioxidant defense by multiple mechanisms, including retrograde signaling, transcriptional control, post-transcriptional regulation, post-translational redox modifications or phosphorylation, and protein–protein interactions.
Redox Regulation
The cellular antioxidant capacity is tightly coupled with the maintenance of redox homeostasis by redox buffers such as ascorbate and glutathione (Karpinski et al., 1997; Foyer and Noctor, 2011). Both compounds may directly decompose ROS and are essential for preserving the ROS content at physiological levels. In addition, they serve as co-substrates for enzymes of the ascorbate–glutathione cycle. The high reduction state of both compounds is connected to enhanced plant tolerance to adverse stress conditions and increased antioxidant capacity (Foyer and Noctor, 2011). The flexibility and rapid response of antioxidant enzymes to changing external conditions are primarily controlled by the redox state of thiol groups in their amino acid sequences, regulated by thio-, peroxi-, and glutaredoxins or other oxidoreductases (Meyer et al., in press). One of them, NUCLEOREDOXIN 1 (NRX1), can target several important antioxidant enzymes, including CAT1, CAT2, and CAT3, GLUTATHIONE S-TRANSFERASE (GST), APX1, GLUTAREDOXIN FAMILY PROTEIN, and METHIONINE SULFOXIDE REDUCTASE (MSR) B2. The expression of antioxidant enzymes depends on the proper functioning of NRX1 that has an impact on plant oxidative stress tolerance (Kneeshaw et al., 2017). A similar proteomic elucidation of thioredoxin mitochondrial targets using affinity chromatography revealed that mitochondrial MSD1, thio- and peroxiredoxins, MSR isoforms, and GLUTATHIONE PEROXIDASE 6 act in a thioredoxin-dependent manner (Yoshida et al., 2013). Multiple antioxidant enzymes including chloroplastic CSD2, CAT3, MDHAR6, PEROXIREDOXIN TPx1, GST isoforms, and MSR-LIKE PROTEIN have also been detected as targets of THIOREDOXIN y1, a plastidic thioredoxin isoform in roots (Marchand et al., 2010). These data indicate that the redox regulation of antioxidant plant defense is quite complex and requires both spatial and temporal coordination.
Mitogen-Activated Protein Kinase Signaling, Reactive Oxygen Species, and Antioxidants
Reactive Oxygen Species-Induced Mitogen-Activated Protein Kinase Signaling Pathways
A very significant feature of ROS is their capability to activate MAPKs, representing key signal transduction proteins triggered by a plethora of environmental and developmental factors (Colcombet and Hirt, 2008; Šamajová et al., 2013; Smékalová et al., 2014, Komis et al., 2018). MAPK signaling cascades consist of MAPKKKs (MAP3Ks), MAPKKs (MAP2Ks), and MAPKs, which are consecutively phosphorylated, leading to the activation/inactivation of a wide range of target proteins, including TFs (Liu and He, 2017). It is well-known that MAPK signaling pathways are activated by ROS accumulated during plant responses to either abiotic stresses or pathogen attack. So far, two kinds of MAP3Ks were identified to be activated by ROS, namely ARABIDOPSIS HOMOLOGS OF NUCLEUS AND PHRAGMOPLAST LOCALIZED KINASES (ANPs) and MITOGEN-ACTIVATED PROTEIN KINASE KINASE KINASE 1 (MAPKKK1 or MEKK1). ANPs are required for the plant immune response (Kovtun et al., 2000; Savatin et al., 2014), whereas the ROS-triggered signal is further transduced via MITOGEN-ACTIVATED PROTEIN KINASE 3 (MPK3) and MPK6 (Kovtun et al., 2000; Nakagami et al., 2006). The full activation of MPK3 and MPK6 is preconditioned by the presence of OXIDATIVE SIGNAL INDUCIBLE 1 kinase (OXI1), which is an essential component of this signal transduction pathway (Rentel et al., 2004). Another ROS-activated MAPK cascade consists of MEKK1, MKK1/2, and MPK4 (Nakagami et al., 2006; Pitzschke et al., 2009). This pathway is also important for basal plant defense against pathogen attack (Zhang et al., 2012). Moreover, a pathogen-induced oxidative burst activates the MPK7 downstream of MKK3 (MAP2K), thus triggering the expression of pathogenesis-related (PR) genes, independently of flagellin receptor FLAGELLIN SENSING 2 (Dóczi et al., 2007).
Furthermore, MPK1 and MPK2 are activated by oxidative stress and jasmonic acid (JA), ABA, and wounding (Ortiz-Masia et al., 2007). In turn, ABA and ROS-activated MPK9 and MPK12 act upstream of anion channels in guard cells, thus regulating stomatal closure (Jammes et al., 2009). MPK3 was found as another principal player in guard cell signaling via ABA and H2O2 perception in guard cells, which leads to stomatal closure (Gudesblat et al., 2007). Thus, MAPK signaling activated by ABA-induced ROS accumulation is generally implicated in stomatal movements (Danquah et al., 2014; Sierla et al., 2016).
MAPKs also respond to ROS produced in chloroplasts and mitochondria. In this respect, MPK6 is implicated in chloroplast to nucleus-directed retrograde signaling upon intense light exposure. The activation of MPK6 under such conditions is preceded by the export of Calvin–Benson cycle intermediate dihydroxyacetone phosphate (DHAP) from chloroplasts to the cytosol. These events lead to the rapid (within several minutes) expression of APETALA2/ETHYLENE-RESPONSIVE ELEMENT BINDING FACTOR (AP2/ERF) TFs and other downstream genes, such as CHLOROPLAST PROTEIN KINASE LIKE (ChlPK-like), CHITINASE FAMILY PROTEIN (CHFP), HEAT SHOCK PROTEIN 20 LIKE (HSP20-like), and PR1 (Vogel et al., 2014). Similarly, MPK4 orchestrates plastid retrograde signaling in a salicylic acid-dependent manner (Gawroński et al., 2014). Mitochondrial ROS production induced by oxygen deprivation activates MPK6 and subsequent retrograde signaling toward the nucleus, leading to transcriptional reprogramming and triggering plant defense mechanisms (Chang et al., 2012).
Several studies report on the regulation of MAPKs by direct interactions with ROS. Waszczak et al. (2014) identified MPK2, MPK4, and MPK7 as capable of being sulfenylated in an H2O2-dependent manner. Another example pertains to Brassica napus BnMPK4, an ortholog of Arabidopsis MPK4, activated by H2O2 and undergoes aggregation upon the H2O2-dependent oxidation of the Cys232 residue (Zhang T. et al., 2015). Thus, it is obvious that MAPKs could be modified on cysteine residues by direct oxidation, affecting their stability, aggregation, and probably protein–protein interactions. It is noteworthy that the redox regulation of MAPKs may lead either to their activation or inactivation. The oxidation of kinase amino acid residues was reported to interfere with ATP binding and cause inactivation (Diao et al., 2010) but may also lead to their super-activation state (Corcoran and Cotter, 2013).
Regulation of Antioxidant Enzymes by Mitogen-Activated Protein Kinase Signaling
MAPKs appear as essential regulators of antioxidant defense, as their genetic modifications can alter the expression profile of many antioxidant enzymes under diverse environmental conditions.
Under high light intensity (Xing et al., 2013) and hypersalinity (Xing et al., 2015), the expression of Arabidopsis SODs is regulated by MKK5 (MAP2K). The expression of CSD1 and CSD2 increases under enhanced light exposure. Interestingly, the transcript levels of both CSD genes remain unchanged under these conditions in a transgenic Arabidopsis line with downregulated MKK5, which is hypersensitive to high light. In contrast, a transgenic Arabidopsis line overexpressing MKK5 is resistant to high light stress and shows the increased activity of both CSDs. Moreover, the downregulation of MKK5 negatively affects the activation of MPK3 and MPK6 under these conditions, thereby implying that these two MAPKs act downstream of MKK5 (Xing et al., 2013). It is worth mentioning that MKK5, acting downstream of MEKK1 and upstream of MPK6, is also essential for the expression of chloroplastic FSD2 and FSD3 during salt stress (Xing et al., 2015). In addition, MKK1 mediates the transcriptional activation of CAT1, but not of CAT2 and CAT3, during salt stress, and after drought and ABA treatments (Xing et al., 2007). CAT1 is important for the regulation of ABA-mediated H2O2 production, whereas its expression is activated by MPK6 operating downstream of MKK1 (Xing et al., 2008).
A MAPK cascade activated by ROS includes ANPs, MPK3, and MPK6 (Kovtun et al., 2000). A shotgun proteomic analysis of Arabidopsis anp2/anp3 double mutant revealed the overabundance and/or increased activity of several proteins important for plant antioxidative defense, including FSD1, MSD1, and enzymes of the ascorbate–glutathione cycle, such as APX and DHAR. Consequently, this double mutant showed enhanced resistance to the methyl viologen-induced oxidative stress. Thus, it might be concluded that ANPs negatively regulate Arabidopsis tolerance to oxidative stress (Takáč et al., 2014). ANPs are likely master regulators of plant antioxidant defense. Contrarily, they also confer resistance to Arabidopsis against necrotrophic fungus Botrytis cinerea (Savatin et al., 2014), suggesting the functional divergence of ANPs in Arabidopsis.
The genetic modification of the MEKK1-MKK1/MKK2-MPK4 signaling pathway also deregulates the expression of several antioxidant enzymes. Notably, Arabidopsis mutants in genes encoding individual constituents of this cascade show diverse patterns of CSD1, APX1, GR, CAT1, and CAT2 expression. The upregulation of CAT1 and CAT3 in mekk1 and mkk1/mkk2 mutants, but not in the mpk4 mutant points, to the complexity of MAPK signaling toward antioxidant genes suggests possible cross talk of diverse MAPK signaling pathways (Pitzschke et al., 2009). According to another study, MPK4 is required for the homeostasis of ROS scavenging proteins in a salicylic acid-dependent manner (Gawroński et al., 2014). Apart from its signaling role during pathogen defense, salicylic acid positively regulates the expression of diverse abiotic stress-related proteins, including antioxidant enzymes, thus contributing to plant stress tolerance (Horváth et al., 2007; Khan et al., 2015). The double mutant in MPK4 and ISOCHRISMATE SYNTHASE 1, encoding an enzyme involved in the synthesis of salicylic acid, results in the deregulated expression of FSDs, CSD2, GR2, and APXs. Therefore, the cross talk of salicylic acid and MAPK signaling is important for the expression of enzymes with antioxidant functions (Gawroński et al., 2014).
Thus, MEKK1 appears as an important regulator of antioxidant defense, capable of activation of MKK1/2 and MKK5 and subsequently MPK3/6 and MPK4. According to the current knowledge, MAPKs acting downstream of MEKK1 (MKK1/2/5; MPK3/4/6) may serve as proteins providing signal specificity toward individual antioxidant enzymes (Figure 1).
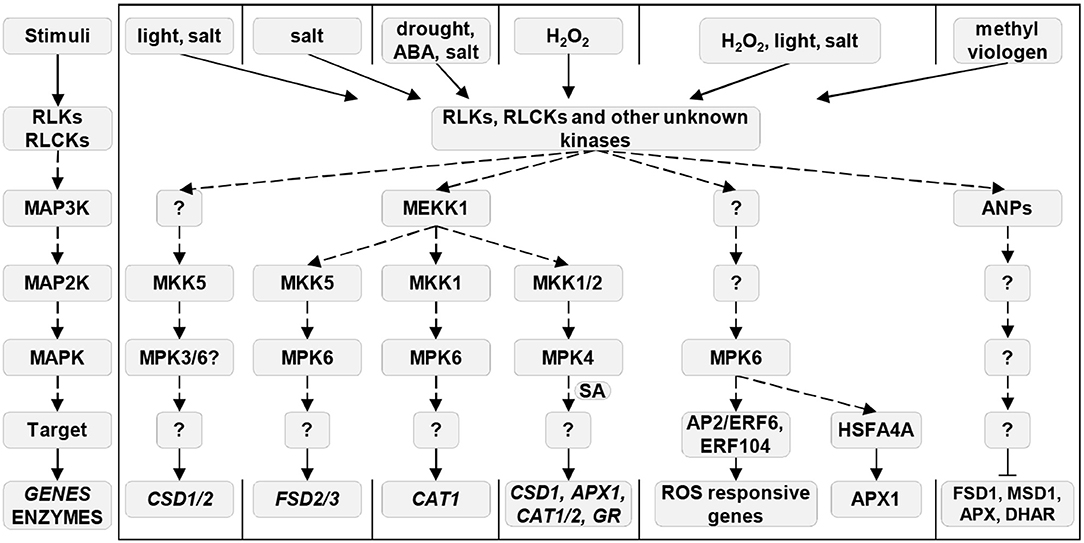
Figure 1. MAPK-dependent regulation of antioxidant enzymes during plant stress responses. Most left pathway shows the universal order of a MAP3K/MAP2K/MAPK cascade. Solid arrows indicate induction, dashed arrows show phosphorylation, and ⊥ indicates negative regulation. Question mark means an unknown component of the pathway. ABA, abscisic acid; ANPs, Arabidopsis nucleus- and phragmoplast-localized kinases; AP2/ERF6, Apetala2/Ethylene-responsive element binding factor 6; APX, ascorbate peroxidase; CAT, catalase; CSD, Cu/Zn superoxide dismutase; DHAR, dehydroascorbate reductase; ERF104, Ethylene-responsive element binding factor 104; FSD, Fe superoxide dismutase; GR, glutathione reductase; HSFA4A, Heat shock transcription factor A4A; MAPK, mitogen-activated protein kinase; MAP2K, mitogen-activated protein kinase kinase; MAP3K, mitogen-activated protein kinase kinase kinase; MSD, Mn superoxide dismutase; RLCKs, receptor-like cytoplasmic kinases; RLKs, receptor-like kinases; ROS, reactive oxygen species; SA, salicylic acid.
The MAPK-mediated transcriptional remodeling of oxidative stress-related genes occurs via the phosphorylation of TFs. For example, a TF called MYB44 (Persak and Pitzschke, 2013, 2014) and HEAT SHOCK TRANSCRIPTION FACTOR A4A (HSFA4A; Pérez-Salamó et al., 2014) are phosphorylated by MPK3 or MPK6. Heat shock TFs are known to play important roles during plant responses to several abiotic stresses. Thus, the overexpression of HSFA4A reduces the H2O2 content and lipid peroxidation, whereas it increases the activity of APX1 after salt treatment. Moreover, it leads to transcriptional changes in a large set of genes responsive to oxidative stress. The same study also confirmed that HSFA4A is involved in the transcriptional activation of genes encoding other TFs, such as WRKY30, ZAT12, CRK13, HSP17.6A, ZAT6, and CTP1, which are known to play essential roles in plant responses to biotic and abiotic stresses (Pérez-Salamó et al., 2014). Furthermore, MPK6 phosphorylates AP2/ERF6 (Wang et al., 2013a; Vogel et al., 2014) and AP2/ERF104 (Bethke et al., 2009) during oxidative stress. After activation, ERF6 specifically binds to the ROS-responsive cis-acting element 7 (ROSE7/GCC box), thus inducing the expression of ROS-responsive genes under intense illumination. ROSE are sorted into seven groups according to their cis-acting motives and core sequences showing different responses to stress conditions (Wang et al., 2013a).
Thus, MAPKs can activate an array of TFs controlling the expression of antioxidant enzymes. Modern bioinformatics provides an opportunity to broaden the list of potential TF-regulating antioxidants, which show altered expression (transcriptomics) or abundance (proteomics) in transgenic lines with a modified or missing expression of particular MAPK genes. This may be carried out by integrating four different parameters: (1) the presence of cis-element(s) in the promoter sequence of the gene encoding the target antioxidant enzyme (predicted by AthaMap; Hehl et al., 2016), (2) TFs co-expressed with target antioxidants and MAPKs (determined by ATTED II; Obayashi et al., 2018), (3) TFs containing a MAPK-specific phosphorylation site [S(p)P or S(p)T; evaluated by PhosPhat 4.0 and GPS 3.0; Xue et al., 2005; Zulawski et al., 2013], and (4) the presence of a MAPK-specific docking site in the amino acid sequence of the TF (evaluated by ELM; Kumar et al., 2020). As an example, we provide a list of TFs potentially responsible for the expression of FSD1, DHAR1, and APX1 under the regulation of MPK3, MPK4, and MPK6 (Figure 2; Supplementary Tables 1–3). Previously, these three enzymes showed significant changes in their abundance in mpk4 (Takáč et al., 2016b) and anp2/anp3 mutants (Takáč et al., 2014). Some predicted TFs have already been reported as regulators of antioxidant enzymes, including SPL7 regulating FSD1. The data set also includes HSFB2A, which has been predicted as a general TF for all three examined enzymes (Supplementary Table 1).
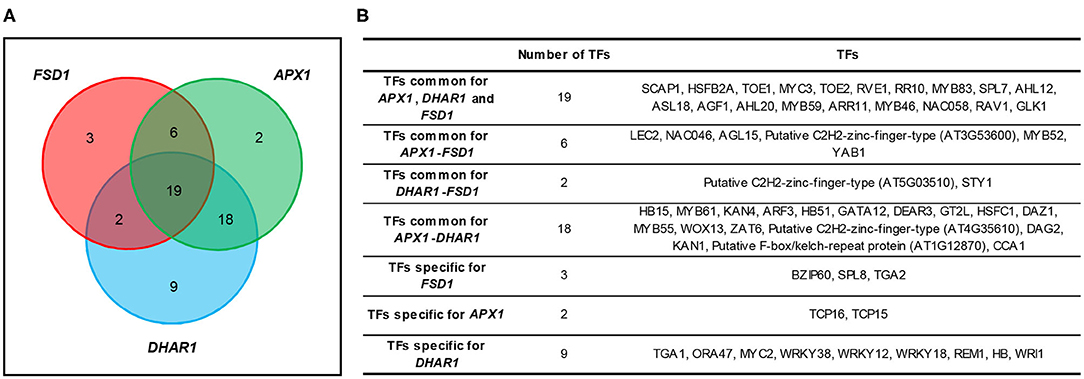
Figure 2. Graphical (A) and tabular (B) overview of common transcription factors (TFs) predicted to regulate the expression of FSD1, APX1, and DHAR1 under the regulation of mitogen-activated protein kinases.
Finally, it should be noted that MAPK signaling can also affect the expression of NOXs. MEK2 cascade phosphorylates WRKY TFs (WRKY7/WRKY8/WRKY9/WRKY11), binding to the cis-element of the RBOHB promoter sequence during the exposure of Nicotiana benthamiana to bacterial protein elicitor INF1 and effector R3a/AVR3a (Adachi et al., 2015). In Zea mays, the activation of ZmMAPK5 is related to the increased expression of NOX and apoplastic ROS production in response to brassinosteroid and ABA treatments (Lin et al., 2009; Zhang et al., 2010). Nevertheless, the precise mechanism controlling the homeostasis between MAPK-induced ROS production and decomposition remains unknown.
Ca2+ Signaling, Reactive Oxygen Species, and Antioxidants
Cross Talk of Reactive Oxygen Species With Ca2+ Signaling
Cytosolic Ca2+ is an important secondary messenger functioning in intra- and extracellular signaling networks involved in abiotic stress responses and plant innate immunity (Schulz et al., 2013; Demidchik et al., 2018; Tian et al., 2020). The rapidly fluctuating cytosolic content of Ca2+ is regulated by environmental cues, which activate Ca2+ channels, ion pumps, or plasma membrane- or organelle-membrane-embedded Ca2+ transporters (Chmielowska-Bak et al., 2014; Tian et al., 2020), as well as by Ca2+-binding proteins, such as calmodulin, calcineurin B-like proteins (CBL), and various Ca2+-dependent protein kinases (CPKs or CDPKs; Gilroy et al., 2014; Zhang et al., 2018). Plant- and protozoan-specific Ser-/Thr-CDPKs are key players of stress-mediated signaling networks, represented by a large number of members in the multigene family in diverse plant species [34 in Arabidopsis, 29 in rice, 20 in wheat, and 30 in poplar and Brachypodium distachyon (Zhang et al., 2018; reviewed in Atif et al., 2019; Wen et al., 2020)].
The interplay between Ca2+ and ROS is mutual because the cytosolic Ca2+ content is regulated by ROS, and vice versa, Ca2+ is crucial for ROS production (Choi et al., 2014; Gaupels et al., 2017). Therefore, Ca2+-dependent ROS signaling through NOXs as key signaling hubs and ROS-dependent Ca2+ signaling through the direct regulation of Ca2+ channels and sensors amplify each other during plant immune responses (reviewed by Marcec et al., 2019). The auto-propagating ROS wave generated by Ca2+-dependent NOX is directly linked to the Ca2+ wave during the systemic response of plants to pathogen infection, as CDPKs modulate the activity of RBOHD (reviewed by Gilroy et al., 2016). Both Ca2+ and ROS waves may be integrated via RBOHs, Ca2+ channel TWO-PORE CHANNEL 1, and CDPKs such as CPK/CBL-CIPKs (CBL-interacting protein kinases; Choi et al., 2014; Gilroy et al., 2016). Moreover, NOXs possess a special hydrophilic N-terminal Ca2+-binding site, the so called EF-hand motif, activated by Ca2+ (reviewed by Hu et al., 2020).
Under drought stress, ion channels in the plasma membrane are activated by ROS (Pei et al., 2000; Dodd et al., 2010), whereas H2O2 can induce the channel activity of Arabidopsis ANNEXIN 1 (AtANN1) and STELAR K+ OUTWARD RECTIFIER (SKOR; Richards et al., 2014; reviewed by Demidchik, 2018). In turn, CDPKs regulate the production of ROS by the phosphorylation of serine residues at the C-terminus of NOXs in a Ca2+-dependent manner (Kobayashi et al., 2007).
Moreover, Arabidopsis CPK5 and RBOHD are key components of a self-propagating activation circuit mediating cell-to-cell communication in plant immunity responses (Dubiella et al., 2013). Further, a plasma membrane anchored AtCPK27 is required for the plant response to salt stress, as its disruption causes oxidative burst and H2O2 accumulation in primary roots (Zhao et al., 2015). CPK4, CPK5, CPK6, and CPK11 regulate ROS production in Arabidopsis, possibly by the direct phosphorylation of RBOHB (Boudsocq et al., 2010). Finally, endoplasmic reticulum membrane-localized B. napus CPK2 interacts with RBOHD during cell death, accompanied by ROS accumulation (Wang et al., 2018).
Therefore, reciprocal ROS and Ca2+ signaling pathways orchestrate the production and accumulation of these secondary messengers and play vital roles in plant adaptation to adverse environmental conditions.
Modulation of Antioxidant Enzymes by Ca2+ Signaling
The impact of Ca2+ signaling on antioxidant enzymes was demonstrated by experiments with exogenous Ca2+ or the genetic manipulation of Ca2+ transporters. For example, CaCl2 enhances the tolerance of rice seedlings to arsenic stress by the reduction of ROS content and the stimulation of MDHAR, DHAR, CAT, GLUTATHIONE PEROXIDASE, and SOD (Rahman et al., 2015). Ca2+-dependent ATPases are Ca2+ transporters involved in multiple stress signaling pathways, physiological processes such as stomatal closure and programmed cell death, and ROS homeostasis (reviewed by Marcec et al., 2019). Furthermore, OsACA6, a Ca2+-ATPase from rice, can modulate ROS levels under salinity and drought stresses by the upregulation of CAT, APX, and GR activities, whereas plants overexpressing OsACA6 show enhanced tolerance to these abiotic stress factors (Huda et al., 2013).
It is known that CDPKs/CPKs are crucial for the regulation of both ROS generation and metabolism under both abiotic and biotic stress conditions and are closely related to enhanced antioxidant enzyme activities in plants, e.g., under the attack of herbivores or microbial pathogens (Romeis and Herde, 2014; Marcec et al., 2019). For instance, AtCPK8 is a component of the ABA- and Ca2+-mediated signaling pathway, which phosphorylates and activates CAT3 at Ser261 in stomatal guard cells during drought stress (Zou et al., 2015). The CPK-mediated regulation of CAT activity was reported under salt stress conditions, namely CPK12 controls ion homeostasis and ROS accumulation via CAT, APX, and SOD activities, thereby impacting Arabidopsis salt stress tolerance (Zhang et al., 2018). CPKs also affect other components of the ROS-decomposing machinery. Thus, OsCPK12 from rice enhances salt stress tolerance through decreasing the ROS content, owing to the elevated expression of OsAPX2, OsAPX8, and reduced expression of NOX called OsBOHI but, at the same time, negatively modulates blast disease resistance (Asano et al., 2012). Ca2+-regulated expression of APX was also observed in maize, as Ca2+ activation of ZmCCaMK (Ca2+/calmodulin-dependent protein kinase from Z. mays) is required for the expression and activation of APX2 (and also SOD4) in response to brassinosteroids (Yan et al., 2015).
Hence, Ca2+ homeostasis and subsequent Ca2+ signal transduction via CDPKs (Figure 3) play an inevitable role in the regulation of antioxidant protection machinery by enhancing plant plasticity and resistance to environmental challenges.
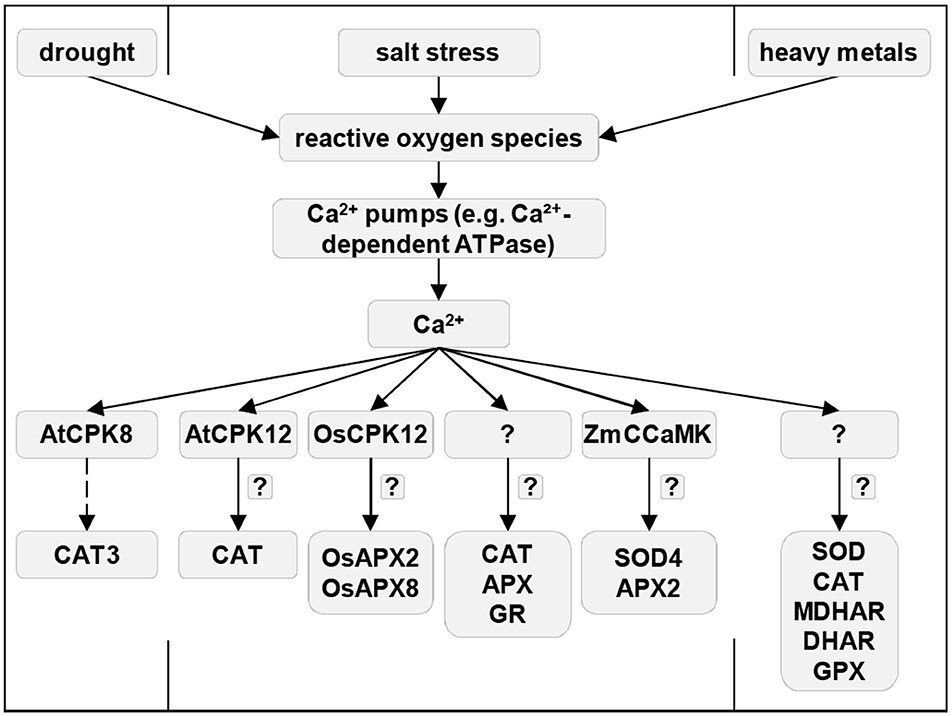
Figure 3. Mechanisms of Ca2+-mediated regulation of antioxidant enzymes during stress in plants. Solid arrows indicate induction, and dashed arrows show phosphorylation. Question mark means an unknown regulation. APX, ascorbate peroxidases; CAT, catalase; CPK, Ca2+-dependent protein kinases; DHAR, dehydroascorbate reductase; GPX, glutathione peroxidase; GR, glutathione reductase; MDHAR, monodehydroascorbate reductase; SOD, superoxide dismutase; ZmCCaM, Ca2+/calmodulin-dependent protein kinase from Zea mays.
Reactive Nitrogen Species-Mediated Regulation of Antioxidant Enzymes
RNS interplay with ROS-signaling pathway by both direct and indirect modulation of antioxidant enzymes activity (Lindermayr and Durner, 2015; Farnese et al., 2016). Thus, exogenous NO donor sodium nitroprusside (SNP) alleviates the detrimental effects of arsenic stress by stimulating the SOD, CAT, GST, and APX activities (Shukla and Singh, 2015). Potentiated action of SNP and silicon (Si) significantly increased the activities of SOD, guaiacol peroxidase, APX, GR, and GST in arsenic-stressed B. juncea plants (Ahmad et al., 2020). SNP also alleviates cadmium (Cd2+)-induced oxidative damage in O. sativa by the pronounced enhancement of the SOD, APX, guaiacol peroxidase, and CAT activities (He et al., 2014). A similar mechanism of the protective effects of SNP under nickel stress by upregulating the transcript levels of CAT, guaiacol peroxidase, APX, GR, and SOD genes was described (Rizwan et al., 2018). Both NO and H2O2 are involved in the regulation of ascorbate and glutathione metabolism by JA in Agropyron cristatum leaves, as JA stimulates the production of both secondary messengers and enhances the activities of APX, GR, MDHAR, DHAR, and enzymes of ascorbate biosynthesis (Shan and Yang, 2017). The link between NO and glutathione exists as well, as SNP modulates glutathione synthesis in Medicago truncatula roots by upregulation of GAMMA-GLUTAMYLCYSTEINE SYNTHETASE and GLUTATHIONE SYNTHETASE but not HOMOGLUTATHIONE SYNTHETASE (Innocenti et al., 2007).
Covalent loss- and/or gain-of-function NO-induced post-translational modifications (PTM) of antioxidant enzymes such as S-nitrosylation and tyrosine nitration reciprocally regulate ROS homeostasis, thereby preserving the balance between ROS production and scavenging in plant cells under natural and stress conditions (Yang et al., 2015; Romero-Puertas and Sandalio, 2016; Kohli et al., 2019). Thus, S-nitrosylation at Cys32 increases the activity of cytosolic APX1 under salinity stress (Begara-Morales et al., 2014), enhances the plant resistance to methyl viologen, but negatively modulates the plant immune response triggered by flagellin elicitor peptide flg22 (Yang et al., 2015). ROS reduces the degree of S-nitrosylation through inhibition of S-nitrosoglutathione reductase (GSNOR), a Zn2+-dependent class III alcohol dehydrogenase enzyme controlling the pool of S-nitrosoglutathione (GSNO)-the storage and long-distance transport NO form. This mechanism leads to higher glutathione levels, transcripts, and activities of glutathione-dependent antioxidant enzymes (Kovacs et al., 2016). This PTM at Cys230 inhibits CAT1 activity in response to Cd2+ in pea leaves (Ortega-Galisteo et al., 2012). In turn, another NO-induced PTM, namely ONOO−-induced tyrosine nitration, inhibits the activity of APX (Begara-Morales et al., 2014), MDHAR (Begara-Morales et al., 2015), CAT (Chaki et al., 2015), MSD1, peroxisomal CSD3, and chloroplastic FSD3 (Holzmeister et al., 2015). It is noteworthy that CAT activity is affected not only by S-nitrosylation but also by H2S-induced persulfidation (Palma et al., 2020).
GSNOR is apparently a significant convergence point between ROS- and RNS-signaling, as ROS inhibits GSNOR activity by oxidation or the Zn2+-release from the GSNOR structure (Tichá et al., 2017; Lindermayr, 2018). The activity and stability of GSNOR1 are also regulated by ROG1 (REPRESSOR OF GSNOR1)-mediated transnitrosylation at Cys10, which, by this mechanism, affects NO-based redox signaling in plants. Surprisingly, ROG1 is identical to CAT3 (Chen et al., 2020), assigning unexpected roles to this antioxidant enzyme. Last but not least, ROS and RNS cooperatively regulate MAPK signaling in a broad range of abiotic stresses, such as heavy metal exposure as well as drought and osmotic stress (reviewed by Farnese et al., 2016).
In summary, the expression and activity of antioxidant enzymes indispensably depend on their RNS mediated PTMs (Figure 4).
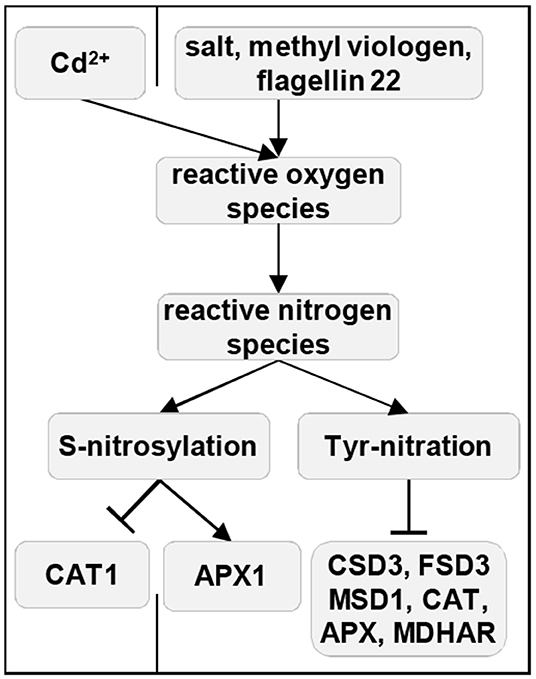
Figure 4. Reactive nitrogen species-mediated direct regulation of antioxidant enzymes during plant stress responses. Solid arrows indicate induction, and ⊥ indicates negative regulation. APX, ascorbate peroxidase; CAT, catalase; FSD, Fe superoxide dismutase; MDHAR, monodehydroacorbate reductase; MSD, Mn superoxide dismutase.
Transcriptional and Post-transcriptional Regulation of Antioxidant Enzymes
Antioxidant enzymes are also regulated at the level of transcription, which is mediated by diverse TFs, not necessarily acting downstream of MAPKs. They are rapidly activated by redox perturbations in photosynthetic electron transport during retrograde signaling from plastid to nucleus encompassing metabolites (e.g., phosphoadenosines, tetrapyrroles, carotenoid oxidation products, carbohydrate metabolites, and isoprenoid precursors) and ROS (Chan et al., 2016; Exposito-Rodriguez et al., 2017). The rapid activation of MAPK cascades conditions this signaling process. Furthermore, TFs alone might be controlled by redox modifications (Dietz, 2014).
Compelling evidence suggests the transcriptional remodeling of ROS-responsive genes (reviewed in He et al., 2018; Li et al., 2018). Gadjev et al. (2006) performed a microarray transcriptomic analysis focused on the expression of exogenous ROS-induced TFs. More than 500 annotated Arabidopsis TFs showed a distinct expression pattern upon ROS, and some of them displayed the ability to modulate the expression of antioxidant enzymes (Gadjev et al., 2006). TF families most often connected to the transcriptional control of antioxidants in response to ROS include WRKY, Zinc finger (Znf), NAC, AP2/ERF, DREB, MYB, or bZIP (Khedia et al., 2019).
In general, numerous reports demonstrate the altered expression of antioxidant enzymes in transgenic or mutant plants with modified TF expression. Contrarily, only a few studies demonstrate the direct binding of TFs to the promoter sequences of antioxidant enzymes. It is known that FSD1 and CSDs are transcriptionally orchestrated via a TF called SQUAMOSA PROMOTER BINDING PROTEIN-LIKE 7 (SPL7; Yamasaki et al., 2007, 2009; Garcia-Molina et al., 2014) in a Cu-dependent manner. Under copper deficiency, SPL7 binds to an SPL-specific SBP (SQUAMOSA PROMOTER BINDING PROTEIN DOMAIN) promoter sequence and induces the expression of FSD1, leading to increased abundance and activity of the FSD1 enzyme. At the same time, CSD1, CSD2, and COPPER CHAPERONE FOR SOD (CCS) are post-transcriptionally downregulated by miR398, which is induced by the binding of SPL7 to its promoter sequence (Cohu et al., 2009; Yamasaki et al., 2009). Therefore, SPL7 is an important modulator of copper balance via Cu-responsive proteins and miRNAs (reviewed by Pilon et al., 2011; Pilon, 2017; Araki et al., 2018). In turn, miR398 suppresses the mRNA of CSD1 and CSD2 after treatment with high sucrose content in the culture medium (Dugas and Bartel, 2008). Importantly, miR398 levels are downregulated to allow the post-transcriptional CSD1 and CSD2 mRNA accumulation leading to elevated (oxidative) stress tolerance (Sunkar et al., 2006; Khraiwesh et al., 2012). SPL7 is also implicated in the circadian regulation of FSD1 expression (Perea-García et al., 2016). Further, CSD1 and CSD2 expression is also regulated by miR408 (Ma et al., 2015), illustrating that the post-transcriptional control of SOD expression is quite complex.
The equilibrium between CSDs and FSD1 is influenced by ZAT12 (Znf protein) because its overexpression leads to the increased expression of CSD1, CSD2, and CCS, while FSD1 is downregulated (Davletova et al., 2005b). The induction of ZAT12 expression is reported as an abiotic stress marker during high light exposure, low temperature, oxidative stress (triggered by H2O2 and methyl viologen), and osmotic and salinity stress (Kreps et al., 2002; Rizhsky et al., 2004a; Davletova et al., 2005b; Vogel et al., 2005; Xu et al., 2017). Furthermore, the expression of FSD1, APX1, and APX2 depends on ZAT10 (Mittler et al., 2006) and RELATED TO APETALA-2.6L (RAP2.6L; Liu et al., 2012), while this positive regulation determines the resistance of Arabidopsis to abiotic stresses.
MYB49 has been reported to be an important TF for plant responses to drought, salt, and heavy metal stresses (Cui et al., 2018; Zhang et al., 2019; Zhang P. et al., 2020). In this respect, a transgenic tomato line overexpressing MYB49 showed higher resistance to drought and salt stresses and increased its SOD and peroxidase activity (Cui et al., 2018). Additionally, an Arabidopsis transgenic line overexpressing functional repressor of MYB49, a chimeric AtMYB49-SRDX called SRDX49, showed downregulation of CSD2 and several peroxidases (Zhang P. et al., 2020). Nevertheless, the binding of the TFs of ZAT, RAP, or MYB families to the promoter sequence of SODs has not been confirmed yet.
The expression patterns of CATs are closely correlated with plant senescence, and they contribute to the redox control of this process (Zimmermann et al., 2006; Mhamdi et al., 2012). The transcriptional regulation of CATs is complex and requires several TFs. CATs are directly controlled by WRKY53, which works downstream of redox-sensitive regulatory factor WRKY25 during senescence and oxidative stress response (Miao et al., 2004; Doll et al., 2020). Arabidopsis wrky25/cat2 double mutant exhibit upregulated H2O2 levels in comparison with the wild-type or cat2 single mutant (Doll et al., 2020). This ROS inducible WRKY25-WRKY53-CAT signaling hub can cross talk with MEKK1, which interacts with and phosphorylates WRKY53 (Miao et al., 2007). In addition, the CAT2 promoter interacts with WRKY75, leading to CAT2 downregulation depending on plant age, senescence, salicylic acid, H2O2, and multiple plant hormones (Guo et al., 2017). The CAT3 expression is directly induced by two isoforms of AP2/ERF4 family TFs, having a different impact on plant senescence according to the alternative polyadenylation of pre-mRNAs. The age-dependent expression of CAT3 and plant senescence are controlled by the expression ratio of ERF4-A (inducer) and ERF4-R (repressor) isoforms (Riester et al., 2019). Recently, MYC2 was experimentally approved to bind to the promoter of Arabidopsis CAT2, leading to its downregulation during leaf senescence in a JA-dependent manner. Such downregulation promotes H2O2 accumulation and the activation of senescence-associated genes (Zhang Y. et al., 2020). Finally, CAT2 expression is also regulated by CDF4 (CYCLING DOF FACTOR 4) during senescence (Xu et al., 2020).
The ascorbate–glutathione cycle represents a very sensitive and efficient system for H2O2 decomposition, requiring not only strict redox control but also the synchronized expression of respective enzymes. APXs are major components of the ascorbate–glutathione cycle and comprise chloroplastic, peroxisomal, mitochondrial, and cytosolic isoforms, which efficiently eliminate excessive H2O2 by using ascorbate as an electron donor (Foyer and Noctor, 2011). The transcriptional control of APXs relies on multiple TFs, whereas RAP2s (also known as ERF-VIIs) exhibit the highest affinity toward both chloroplastic and cytosolic APXs. Chloroplastic stromal and thylakoid APX isoforms (Rudnik et al., 2017) and 2-Cys PEROXIREDOXIN A (Shaikhali et al., 2008; Rudnik et al., 2017) are regulated by redox-sensitive RAP2.4a directly interacting with their promoters under photooxidative stress and increased ROS levels. Seven additional members of the RAP2.4 family also demonstrate an ability to bind to the promoters of the genes mentioned earlier and to fine-tune their expression (Rudnik et al., 2017). In addition, RADICAL-INDUCED CELL DEATH 1 (RCD1), a protein integrating mitochondrial and chloroplastic ROS signals with pleiotropic functions (Shapiguzov et al., 2019), interacts with RAP2.4 under mild and severe oxidative stress and promotes the activation of downstream genes (Hiltscher et al., 2014). The preference of RAP2 for APXs regulation has also been demonstrated for RAP2.6L because the overexpression of this TF causes the overexpression of cytosolic APX1 and improves plant tolerance against waterlogging stress (Liu et al., 2012). Besides RAP2.6L, ZAT12, ZAT7, and WRKY25 are also required for the proper expression of cytosolic APX1 during oxidative stress (H2O2, methyl viologen, heat shock, or wounding; Rizhsky et al., 2004b). APX2 and APX7 are expressed under the control of HSFA3, downstream of a TF named DEHYDRATION-RESPONSIVE ELEMENT-BINDING 2C (DREB2C), having an impact on plant resistance to oxidative stress (Hwang et al., 2012; Song et al., 2016). The induction of APX2 transcription as a response to high light initiates photosynthetic redox homeostasis alterations occurring upon the nuclear accumulation and activation of HSFA1D (Jung et al., 2013). C2H2-type zinc finger protein ZFP36 is among the important APX regulators, as this TF binds to the promoter sequence and activates the OsAPX1 gene in rice. This activation is enhanced by LATE EMBRYOGENESIS ABUNDANT 5 (OsLEA5), a protein interacting with ZFP36, which regulates OsAPX1 expression during seed germination (Huang et al., 2018). In addition, ZFP36 was found to be important during rice responses to oxidative and abiotic stresses (Zhang et al., 2014). Another TF called ANAC089 has been reported as a negative regulator of stromal APX in Arabidopsis and can play different roles during plant acclimation to low, normal, and high light conditions. ANAC089 decreases the expression of stromal APX under highly reducing conditions induced by a DTT treatment (Klein et al., 2012).
Knowledge on the transcriptional control of DHARs, MHARs, and GRs, encoding enzymes implicated in ascorbate regeneration during the ascorbate–glutathione cycle, is limited. The mutant analysis of an AP2/ERF domain-containing TF showed the upregulation of DHAR1 and the downregulation of MDHAR3 in response to H2O2 treatment (Sewelam et al., 2013). Recently, R2R3-type MYB from Pyrus betulaefolia (PbrMYB5) was reported as TF important for the expression of PbrDHAR2. The genetic manipulation of PbrMYB5 in tobacco positively correlates with chilling when overexpressor lines show a higher expression of NtDHAR2 and elevated levels of ascorbate (Xing et al., 2019). Finally, regulation by miRNA (PN-2013) interference was reported for wheat MDHAR (Feng et al., 2014).
Phosphorylation of Antioxidant Enzymes
PTMs of proteins represent versatile, dynamic, and flexible regulatory mechanisms for the reprogramming of a wide range of cellular functions. For example, these processes ensure the fast and targeted activation of plant immune responses upon pathogen attacks. Generally, PTMs affect enzymatic activities, subcellular localization, protein interactions, and stability (reviewed in Withers and Dong, 2017; Ruiz-May et al., 2019; Vu et al., 2018;). Among PTMs, the reversible phosphorylation of proteins represents a driving force of signaling processes during plant development and stress challenges (Arsova et al., 2018). Several antioxidant proteins are modified by direct phosphorylation, mainly by proteomic approaches (Table 1).
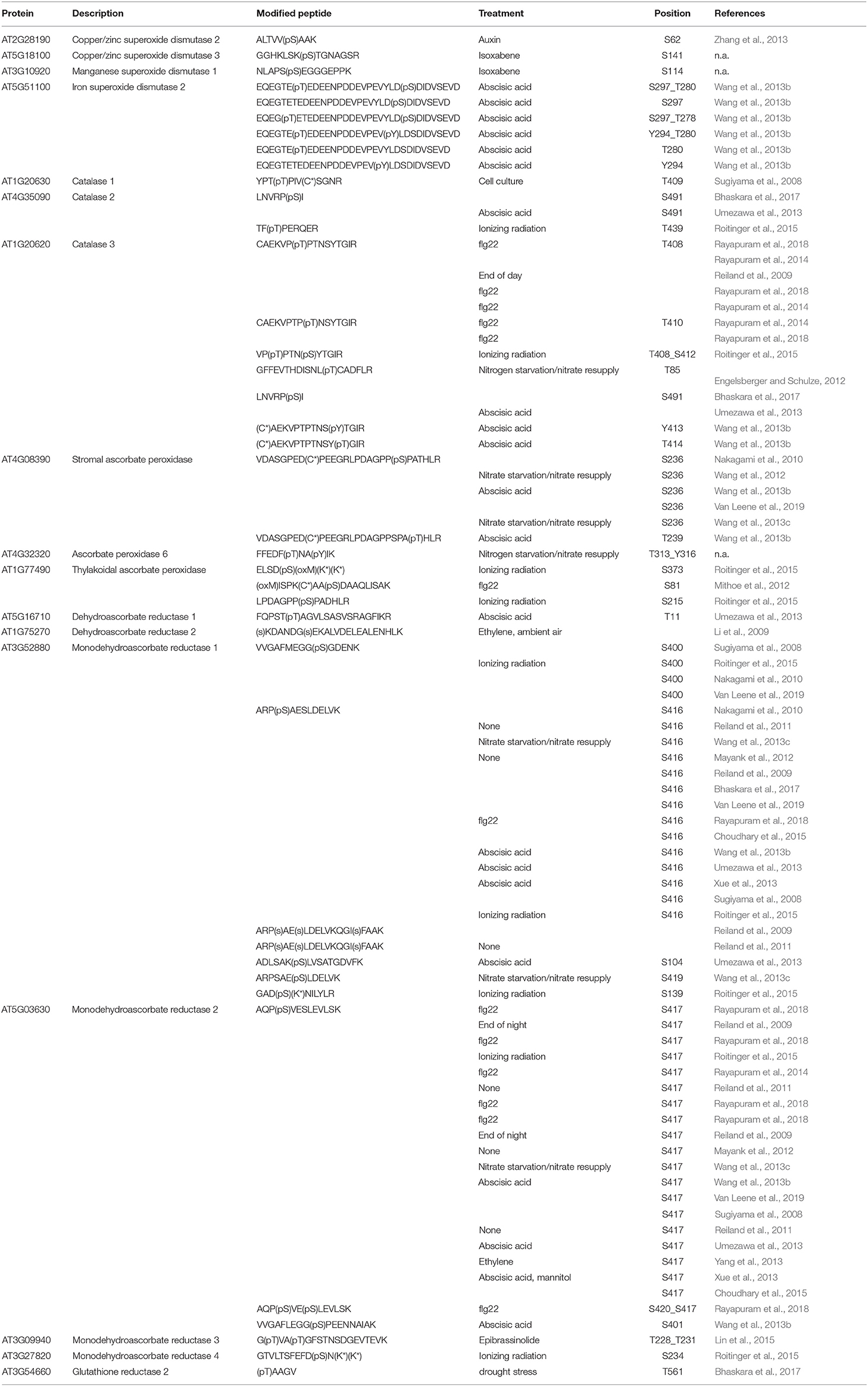
Table 1. Phosphorylation sites experimentally found in antioxidant enzymes by mass spectrometry, retrieved from PhosPhat database.
Although the phosphorylation of SODs has been documented for human SOD1 (Fay et al., 2016; Tsang et al., 2018) and SOD2 (Candas et al., 2013; Jin et al., 2015), mouse SOD2 (Candas et al., 2013), or yeast SOD1 (Leitch et al., 2012; Tsang et al., 2014), the phosphorylation of SOD isoforms in plants has not been approved by genetic means so far. Mammalian SOD1 is phosphorylated at Thr2 (Fay et al., 2016) and Thr40 (Tsang et al., 2018), having a pronounced impact on its activity. For example, the mammalian target of rapamycin complex 1 (mTORC1) is a negative regulator of SOD1 activity under nutrient-rich conditions by reversible phosphorylation at Thr40 (Tsang et al., 2018). Yeast SOD1 is phosphorylated at Ser38 under low oxygen conditions, which allows its interaction with CCS and, consequently, proper folding and activation (Leitch et al., 2012). Contrarily, the double phosphorylation of yeast SOD1 at Ser60 and Ser99 by a DNA damage checkpoint kinase Dun1 during oxidative stress leads to the translocation of this SOD to the nucleus, where it binds to the promoters and activates ROS-responsive and DNA repair genes (Tsang et al., 2014). Recently, the first in planta evidence of the nuclear localization of SODs was reported in plants (Dvořák et al., 2020). Thus, in addition to well-known protective functions during oxidative stress, plant SODs might have similar functions in the nucleus as yeast SOD1 (Figure 5). However, this working hypothesis needs to be experimentally tested in the future. Rarely, phosphoproteomic studies have reported on the detection of phosphorylated amino acid residues in plant SODs. A gel-based study on mitochondrial phosphoproteomes identified phosphorylated MSD1 (Bykova et al., 2003). In addition, CSD2 and CSD3 were found to be phosphorylated in response to auxin and isoxaben, respectively (Zhang et al., 2013; Table 1). Multiple phosphorylation sites were also detected in FSD2 after ABA treatment (Wang et al., 2013b). These findings demonstrate that the phosphorylation of plant SODs may have some important functions that need to be elucidated in future studies.
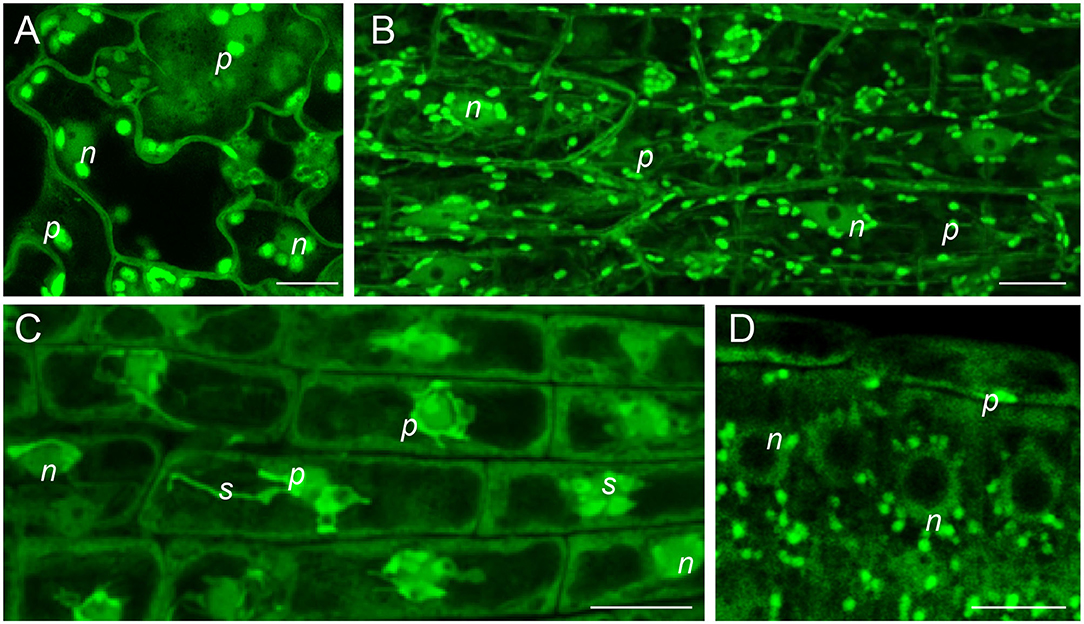
Figure 5. In vivo subcellular localization of FSD1-GFP in plastids, nuclei and cytoplasm of 4-day-old Arabidopsis fsd1-1 mutant harboring proFSD1::FSD1:GFP construct (Dvořák et al., 2020) as revealed by confocal laser scanning microscope equipped with an Airyscan detector. (A) Pavement cells of leaf epidermis; (B) epidermal cells of hypocotyl; (C) lateral root cap cells; (D) root meristematic cells. n, nucleus; p, plastid; s, stromule. Scale bars: (A, C, D) 10 μm; (B) 20 μm.
On the other side, there is quite rich phosphoproteomic evidence on the phosphorylation of CAT isoforms in plants (Table 1). CAT phosphorylation has been broadly documented in human research (Kumar et al., 2010; Rafikov et al., 2014). A recent study suggested that high light-induced H2O2 is regulated by the interaction between CAT isoforms and BRASSINOSTEROID-INSENSITIVE 1 ASSOCIATED RECEPTOR KINASE 1 (BAK1), leading to CAT1 phosphorylation and activation (Zhang S. et al., 2020). CAT3 activity is enhanced through phosphorylation by CPK8, leading to H2O2 decomposition under drought stress (Zou et al., 2015). In rice, the CATC isoform is phosphorylated by SALT TOLERANCE RECEPTOR-LIKE CYTOPLASMIC KINASE 1 (STRK1), which acts as a positive regulator of salt and oxidative stress tolerance (Zhou et al., 2018). STRK1 is anchored in the plasma membrane via palmitoylation, where it interacts and phosphorylates the CATC isoform at Tyr210 (Zhou et al., 2018).
Notably, H2O2 decomposition is controlled by phosphorylation also within the ascorbate–glutathione cycle. Based on phosphoproteomic data, the stromal and thylakoid APX and MDAR1, MDAR2, and GR2 are enzymes prone to phosphorylation (Table 1). Thylakoid APX is negatively regulated by WHEAT KINASE START1 (WKS1)-dependent phosphorylation in wheat infected by stripe rust-inducing fungi Puccinia striiformis f. sp. tritici. Such decreased activity of thylakoid APX leads to the enhanced accumulation of peroxides, causing cell death upon pathogen attacks (Gou et al., 2015).
Conclusion and Future Prospects
In summary, the expression and activities of antioxidant enzymes are controlled both directly and indirectly at multiple levels with the involvement of ubiquitous secondary messengers (ROS, RNS, and Ca2+), PTMs (phosphorylation and redox-dependent ones), TFs, and other precise mechanisms (Figure 6). Currently, data on the coordination of the transcriptional and post-translational regulation of these enzymes are scarce. Moreover, the impact of protein–protein interactions on the functionality of antioxidant enzymes should not be underestimated. The complexity is increased by the requirement for rapid and spatially-specific antioxidant activation, which has to occur in subcellular- and tissue-dependent manners.
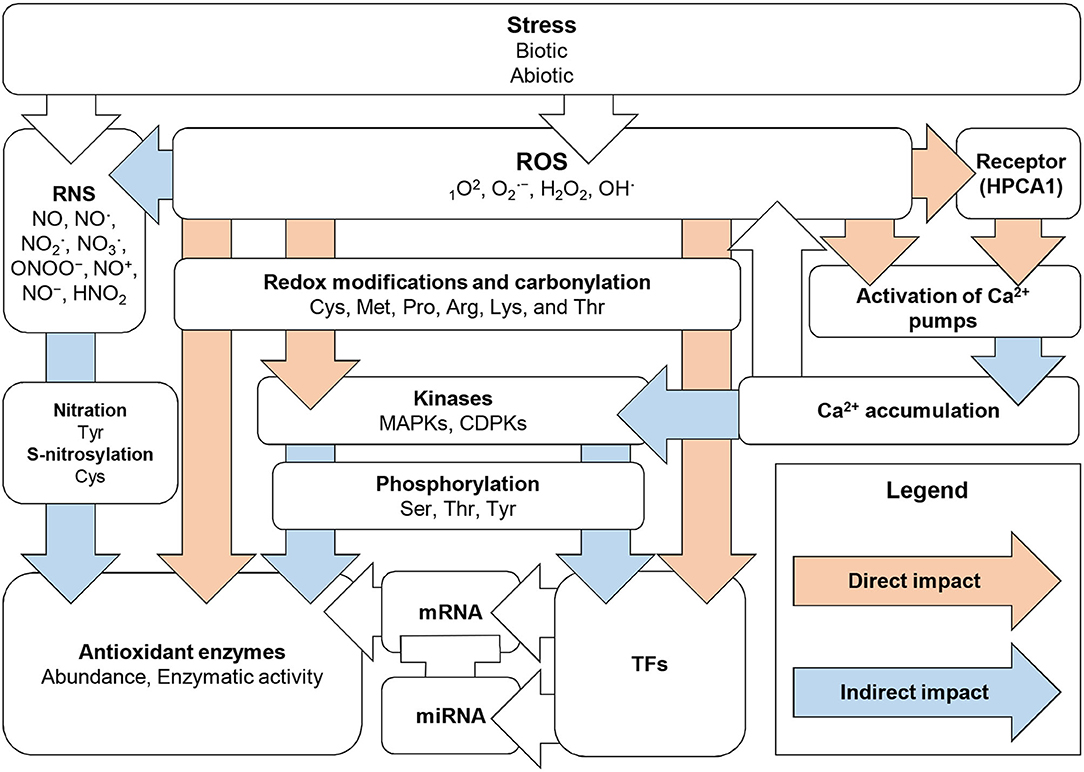
Figure 6. Overview of direct and indirect mechanisms of antioxidant enzyme regulation in response to stress-induced ROS generation.
Further proteomic, genetic, cell, and molecular biology integrative investigations are necessary to uncover precise spatiotemporal regulations of individual antioxidant enzymes during plant development and stress responses. In addition, up-to-date proteomic, phospho- and redox-proteomic approaches might uncover new MAPK and CDPK targets modulating antioxidant defense during oxidative stress. The use of these techniques on transgenic plant lines with modified abundances of certain antioxidant enzymes may significantly contribute to the elucidation of their developmental and stress-related functions.
Author Contributions
PD and AZ performed the bioinformatic prediction. PD and YK drafted the manuscript, which was revised and edited by TT and JŠ. All authors approved the final version of the manuscript.
Funding
This research was funded by grant no. 19-00598S from the Czech Science Foundation GACR and by the ERDF project Plants as a tool for sustainable global development (No. CZ.02.1.01/0.0/0.0/16_019/0000827).
Conflict of Interest
The authors declare that the research was conducted in the absence of any commercial or financial relationships that could be construed as a potential conflict of interest.
Supplementary Material
The Supplementary Material for this article can be found online at: https://www.frontiersin.org/articles/10.3389/fpls.2020.618835/full#supplementary-material
References
Adachi, H., Nakano, T., Miyagawa, N., Ishihama, N., Yoshioka, M., Katou, Y., et al. (2015). WRKY transcription factors phosphorylated by MAPK regulate a plant immune NADPH oxidase in Nicotiana benthamiana. Plant Cell 27, 2645–2663. doi: 10.1105/tpc.15.00213
Ahmad, A., Khan, W. U., Shah, A. A., Yasin, N. A., Naz, S., Ali, A., et al. (2020). Synergistic effects of nitric oxide and silicon on promoting plant growth, oxidative stress tolerance and reduction of arsenic uptake in Brassica juncea. Chemosphere 262:128384. doi: 10.1016/j.chemosphere.2020.128384
Alscher, R. G., Erturk, N., and Heath, L. S. (2002). Role of superoxide dismutases (SODs) in controlling oxidative stress in plants. J. Exp. Bot. 53, 1331–1341. doi: 10.1093/jexbot/53.372.1331
Apel, K., and Hirt, H. (2004). REACTIVE OXYGEN SPECIES: metabolism, oxidative stress, and signal transduction. Annu. Rev. Plant Biol. 55, 373–399. doi: 10.1146/annurev.arplant.55.031903.141701
Araki, R., Mermod, M., Yamasaki, H., Kamiya, T., Fujiwara, T., and Shikanai, T. (2018). SPL7 locally regulates copper-homeostasis-related genes in Arabidopsis. J. Plant Physiol. 224–225, 137–143. doi: 10.1016/j.jplph.2018.03.014
Arsova, B., Watt, M., and Usadel, B. (2018). Monitoring of plant protein post-translational modifications using targeted proteomics. Front. Plant Sci. 9:1168. doi: 10.3389/fpls.2018.01168
Asano, T., Hayashi, N., Kobayashi, M., Aoki, N., Miyao, A., Mitsuhara, I., et al. (2012). A rice calcium-dependent protein kinase OsCPK12 oppositely modulates salt-stress tolerance and blast disease resistance. Plant J. 69, 26–36. doi: 10.1111/j.1365-313X.2011.04766.x
Atif, R. M., Shahid, L., Waqas, M., Ali, B., Rashid, M. A. R., Azeem, F., et al. (2019). Insights on calcium-dependent protein kinases (CPKs) signaling for abiotic stress tolerance in plants. Int. J. Mol. Sci. 20:5298. doi: 10.3390/ijms20215298
Baxter, A., Mittler, R., and Suzuki, N. (2014). ROS as key players in plant stress signalling. J. Exp. Bot. 65, 1229–1240. doi: 10.1093/jxb/ert375
Begara-Morales, J. C., Sánchez-Calvo, B., Chaki, M., Mata-Pérez, C., Valderrama, R., Padilla, M. N., et al. (2015). Differential molecular response of monodehydroascorbate reductase and glutathione reductase by nitration and S-nitrosylation. J. Exp. Bot. 66, 5983–5996. doi: 10.1093/jxb/erv306
Begara-Morales, J. C., Sánchez-Calvo, B., Chaki, M., Valderrama, R., Mata-Pérez, C., López-Jaramillo, J., et al. (2014). Dual regulation of cytosolic ascorbate peroxidase (APX) by tyrosine nitration and S-nitrosylation. J. Exp. Bot. 65, 527–538. doi: 10.1093/jxb/ert396
Berkholz, D. S., Faber, H. R., Savvides, S. N., and Karplus, P. A. (2008). Catalytic cycle of human glutathione reductase near 1 A resolution. J. Mol. Biol. 382, 371–384. doi: 10.1016/j.jmb.2008.06.083
Bethke, G., Unthan, T., Uhrig, J. F., Pöschl, Y., Gust, A. A., Scheel, D., et al. (2009). Flg22 regulates the release of an ethylene response factor substrate from MAP kinase 6 in Arabidopsis thaliana via ethylene signaling. Proc. Natl. Acad. Sci. U.S.A. 106, 8067–8072. doi: 10.1073/pnas.0810206106
Bhaskara, G. B., Wen, T. N., Nguyen, T. T., and Verslues, P. E. (2017). Protein phosphatase 2Cs and Microtubule-Associated Stress Protein 1 control microtubule stability, plant growth, and drought response. Plant Cell 29, 169–191. doi: 10.1105/tpc.16.00847
Bindschedler, L. V., Dewdney, J., Blee, K. A., Stone, J. M., Asai, T., Plotnikov, J., et al. (2006). Peroxidase-dependent apoplastic oxidative burst in Arabidopsis required for pathogen resistance. Plant J. 47, 851–863. doi: 10.1111/j.1365-313X.2006.02837.x
Boudsocq, M., Willmann, M. R., McCormack, M., Lee, H., Shan, L., He, P., et al. (2010). Differential innate immune signalling via Ca2+ sensor protein kinases. Nature 464, 418–422. doi: 10.1038/nature08794
Bueso, E., Alejandro, S., Carbonell, P., Perez-Amador, M. A., Fayos, J., Bellés, J. M., et al. (2007). The lithium tolerance of the Arabidopsis cat2 mutant reveals a cross-talk between oxidative stress and ethylene. Plant J. 52, 1052–1065. doi: 10.1111/j.1365-313X.2007.03305.x
Bykova, N. V., Egsgaard, H., and Møller, I. M. (2003). Identification of 14 new phosphoproteins involved in important plant mitochondrial processes. FEBS Lett. 540, 141–146. doi: 10.1016/s0014-5793(03)00250-3
Candas, D., Fan, M., Nantajit, D., Vaughan, A. T., Murley, J. S., Woloschak, et al. (2013). CyclinB1/Cdk1 phosphorylates mitochondrial antioxidant MnSOD in cell adaptive response to radiation stress. J. Mol. Cell Biol. 5, 166–175. doi: 10.1093/jmcb/mjs062
Chaki, M., Álvarez de Morales, P., Ruiz, C., Begara-Morales, J. C., Barroso, J. B., Corpas, F. J., et al. (2015). Ripening of pepper (Capsicum annuum) fruit is characterized by an enhancement of protein tyrosine nitration. Ann. Bot. 116, 637–647. doi: 10.1093/aob/mcv016
Chan, K. X., Phua, S. Y., Crisp, P., McQuinn, R., and Pogson, B. J. (2016). Learning the languages of the chloroplast: retrograde signaling and beyond. Annu. Rev. Plant Biol. 67, 25–53. doi: 10.1146/annurev-arplant-043015-111854
Chang, R., Jang, C. J., Branco-Price, C., Nghiem, P., and Bailey-Serres, J. (2012). Transient MPK6 activation in response to oxygen deprivation and reoxygenation is mediated by mitochondria and aids seedling survival in Arabidopsis. Plant Mol. Biol. 78, 109–122. doi: 10.1007/s11103-011-9850-5
Chen, C., Letnik, I., Hacham, Y., Dobrev, P., Ben-Daniel, B. H., Vanková, R., et al. (2014). ASCORBATE PEROXIDASE6 protects Arabidopsis desiccating and germinating seeds from stress and mediates cross talk between reactive oxygen species, abscisic acid, and auxin. Plant Physiol. 166, 370–383. doi: 10.1104/pp.114.245324
Chen, L., Wu, R., Feng, J., Feng, T., Wang, C., Hu, J., et al. (2020). Transnitrosylation mediated by the non-canonical catalase ROG1 regulates nitric oxide signaling in plants. Dev. Cell 53, 444–457.e5. doi: 10.1016/j.devcel.2020.03.020
Chew, O., Whelan, J., and Millar, A. H. (2003). Molecular definition of the ascorbate-glutathione cycle in Arabidopsis mitochondria reveals dual targeting of antioxidant defenses in plants. J. Biol. Chem. 278, 46869–46877. doi: 10.1074/jbc.M307525200
Chin, D. C., Senthil Kumar, R., Suen, C. S., Chien, C. Y., Hwang, M. J., Hsu, C. H., et al. (2019). Plant cytosolic ascorbate peroxidase with dual catalytic activity modulates abiotic stress tolerances. iScience 16, 31–49. doi: 10.1016/j.isci.2019.05.014
Chmielowska-Bak, J., Gzyl, J., Rucińska-Sobkowiak, R., Arasimowicz-Jelonek, M., and Deckert, J. (2014). The new insights into cadmium sensing. Front. Plant Sci. 5:245. doi: 10.3389/fpls.2014.00245
Choi, W. G., Toyota, M., Kim, S. H., Hilleary, R., and Gilroy, S. (2014). Salt stress-induced Ca2+ waves are associated with rapid, long-distance root-to-shoot signaling in plants. Proc. Natl. Acad. Sci. U.S.A. 111, 6497–6502. doi: 10.1073/pnas.1319955111
Choudhary, M. K., Nomura, Y., Wang, L., Nakagami, H., and Somers, D. E. (2015). Quantitative circadian phosphoproteomic analysis of Arabidopsis reveals extensive clock control of key components in physiological, metabolic, and signaling pathways. Mol. Cell Proteomics 14, 2243–2260. doi: 10.1074/mcp.M114.047183
Ciacka, K., Tymiński, M., Gniazdowska, A., and Krasuska, U. (2020). Carbonylation of proteins-an element of plant ageing. Planta 252:12. doi: 10.1007/s00425-020-03414-1
Cohu, C. M., Abdel-Ghany, S. E., Gogolin Reynolds, K. A., Onofrio, A. M., Bodecker, J. R., Kimbrel, J. A., et al. (2009). Copper delivery by the copper chaperone for chloroplast and cytosolic copper/zinc-superoxide dismutases: regulation and unexpected phenotypes in an Arabidopsis mutant. Mol. Plant. 2, 1336–1350. doi: 10.1093/mp/ssp084
Colcombet, J., and Hirt, H. (2008). Arabidopsis MAPKs: a complex signalling network involved in multiple biological processes. Biochem. J. 413, 217–226. doi: 10.1042/BJ20080625
Considine, M. J., and Foyer, C. H. (2014). Redox regulation of plant development. Antioxid. Redox Signal. 21, 1305–1326. doi: 10.1089/ars.2013.5665
Corcoran, A., and Cotter, T. G. (2013). Redox regulation of protein kinases. FEBS J. 280, 1944–1965. doi: 10.1111/febs.12224
Corpas, F. J., and Barroso, J. B. (2017). Lead-induced stress, which triggers the production of nitric oxide (NO) and superoxide anion (O2·−) in Arabidopsis peroxisomes, affects catalase activity. Nitric Oxide 68, 103–110. doi: 10.1016/j.niox.2016.12.010
Csiszár, J., Brunner, S., Horváth, E., Bela, K., Ködmön, P., Riyazuddin, R., et al. (2018). Exogenously applied salicylic acid maintains redox homeostasis in salt-stressed Arabidopsis gr1 mutants expressing cytosolic roGFP1. Plant Growth Regul. 86, 181–194. doi: 10.1007/s10725-018-0420-6
Cui, F., Brosché, M., Shapiguzov, A., He, X. Q., Vainonen, J. P., Leppälä, J., et al. (2019). Interaction of methyl viologen-induced chloroplast and mitochondrial signalling in Arabidopsis. Free Radic. Biol. Med. 134, 555–566. doi: 10.1016/j.freeradbiomed.2019.02.006
Cui, J., Jiang, N., Zhou, X., Hou, X., Yang, G., Meng, J., et al. (2018). Tomato MYB49 enhances resistance to Phytophthora infestans and tolerance to water deficit and salt stress. Planta 248, 1487–1503. doi: 10.1007/s00425-018-2987-6
Dalle-Donne, I., Rossi, R., Giustarini, D., Milzani, A., and Colombo, R. (2003). Protein carbonyl groups as biomarkers of oxidative stress. Clin. Chim. Acta 329, 23–38. doi: 10.1016/s0009-8981(03)00003-2
Danquah, A., de Zelicourt, A., Colcombet, J., and Hirt, H. (2014). The role of ABA and MAPK signaling pathways in plant abiotic stress responses. Biotechnol. Adv. 32, 40–52. doi: 10.1016/j.biotechadv.2013.09.006
Davletova, S., Rizhsky, L., Liang, H., Shengqiang, Z., Oliver, D. J., Coutu, J., et al. (2005a). Cytosolic ascorbate peroxidase 1 is a central component of the reactive oxygen gene network of Arabidopsis. Plant Cell 17, 268–281. doi: 10.1105/tpc.104.026971
Davletova, S., Schlauch, K., Coutu, J., and Mittler, R. (2005b). The zinc-finger protein Zat12 plays a central role in reactive oxygen and abiotic stress signaling in Arabidopsis. Plant Physiol. 139, 847–856. doi: 10.1104/pp.105.068254
De Pinto, M. C., Locato, V., and De Gara, L. (2012). Redox regulation in plant programmed cell death: redox regulation in plant PCD. Plant Cell Environ. 35, 234–244. doi: 10.1111/j.1365-3040.2011.02387.x
del Río, L. A. (2015). ROS and RNS in plant physiology: an overview. J. Exp. Bot. 66, 2827–2837. doi: 10.1093/jxb/erv099
del Río, L. A., and López-Huertas, E. (2016). ROS Generation in peroxisomes and its role in cell signaling. Plant Cell Physiol. 57, 1364–1376. doi: 10.1093/pcp/pcw076
del Río, L. A., Sandalio, L. M., Corpas, F. J., Palma, J. M., and Barroso, J. B. (2006). Reactive oxygen species and reactive nitrogen species in peroxisomes. Production, scavenging, and role in cell signaling. Plant Physiol. 141, 330–335. doi: 10.1104/pp.106.078204
Delledonne, M., Zeier, J., Marocco, A., and Lamb, C. (2001). Signal interactions between nitric oxide and reactive oxygen intermediates in the plant hypersensitive disease resistance response. Proc. Natl. Acad. Sci. U.S.A. 98, 13454–13459. doi: 10.1073/pnas.231178298
Demidchik, V. (2018). ROS-Activated ion channels in plants: biophysical characteristics, physiological functions and molecular nature. Int. J. Mol. Sci. 19, 1263. doi: 10.3390/ijms19041263
Demidchik, V., Shabala, S., Isayenkov, S., Cuin, T. A., and Pottosin, I. (2018). Calcium transport across plant membranes: mechanisms and functions. New Phytol. 220, 49–69. doi: 10.1111/nph.15266
Diao, Y., Liu, W., Wong, C. C. L., Wang, X., Lee, K., Cheung, P.-Y., et al. (2010). Oxidation-induced intramolecular disulfide bond inactivates mitogen-activated protein kinase 6 by inhibiting ATP binding. Proc. Natl. Acad. Sci. U.S.A. 107, 20974–20979. doi: 10.1073/pnas.1007225107
Dietz, K.-J. (2011). Peroxiredoxins in plants and cyanobacteria. Antioxid. Redox Signal. 15, 1129–1159. doi: 10.1089/ars.2010.3657
Dietz, K.-J. (2014). Redox regulation of transcription factors in plant stress acclimation and development. Antioxid. Redox Signal. 21, 1356–1372. doi: 10.1089/ars.2013.5672
Ding, H., Wang, B., Han, Y., and Li, S. (2020). The pivotal function of dehydroascorbate reductase in glutathione homeostasis in plants. J. Exp. Bot. 71, 3405–3416. doi: 10.1093/jxb/eraa107
Dóczi, R., Brader, G., Pettkó-Szandtner, A., Rajh, I., Djamei, A., Pitzschke, A., et al. (2007). The Arabidopsis mitogen-activated protein kinase kinase MKK3 is upstream of group C mitogen-activated protein kinases and participates in pathogen signaling. Plant Cell 19, 3266–3279. doi: 10.1105/tpc.106.050039
Dodd, A. N., Kudla, J., and Sanders, D. (2010). The language of calcium signaling. Annu. Rev. Plant Biol. 61, 593–620. doi: 10.1146/annurev-arplant-070109-104628
Doll, J., Muth, M., Riester, L., Nebel, S., Bresson, J., Lee, H. C., et al. (2020). Arabidopsis thaliana WRKY25 transcription factor mediates oxidative stress tolerance and regulates senescence in a redox-dependent manner. Front. Plant Sci. 10:1734. doi: 10.3389/fpls.2019.01734
Du, Y. Y., Wang, P. C., Chen, J., and Song, C. P. (2008). Comprehensive functional analysis of the catalase gene family in Arabidopsis thaliana. J. Integr. Plant Biol. 50, 1318–1326. doi: 10.1111/j.1744-7909.2008.00741.x
Dubiella, U., Seybold, H., Durian, G., Komander, E., Lassig, R., Witte, C.-P., et al. (2013). Calcium-dependent protein kinase/NADPH oxidase activation circuit is required for rapid defense signal propagation. Proc. Natl. Acad. Sci. U.S.A. 110, 8744–8749. doi: 10.1073/pnas.1221294110
Dugas, D. V., and Bartel, B. (2008). Sucrose induction of Arabidopsis miR398 represses two Cu/Zn superoxide dismutases. Plant Mol. Biol. 67, 403–417. doi: 10.1007/s11103-008-9329-1
Dvořák, P., Krasylenko, Y., Ovečka, M., Basheer, J., Zapletalová, V., Šamaj, J., et al. (2020). In-vivo light-sheet microscopy resolves localisation patterns of FSD1, a superoxide dismutase with function in root development and osmoprotection. Plant Cell Environ. 44, 68–87. doi: 10.1111/pce.13894
Eastmond, P. J. (2007). MONODEHYROASCORBATE REDUCTASE4 is required for seed storage oil hydrolysis and postgerminative growth in Arabidopsis. Plant Cell 19, 1376–1387. doi: 10.1105/tpc.106.043992
Eltayeb, A. E., Kawano, N., Badawi, G. H., Kaminaka, H., Sanekata, T., Shibahara, T., et al. (2007). Overexpression of monodehydroascorbate reductase in transgenic tobacco confers enhanced tolerance to ozone, salt and polyethylene glycol stresses. Planta 225, 1255–1264. doi: 10.1007/s00425-006-0417-7
Eltayeb, A. E., Yamamoto, S., Habora, M. E. E., Yin, L., Tsujimoto, H., and Tanaka, K. (2011). Transgenic potato overexpressing Arabidopsis cytosolic AtDHAR1 showed higher tolerance to herbicide, drought and salt stresses. Breed. Sci. 61, 3–10. doi: 10.1270/jsbbs.61.3
Engelsberger, W. R., and Schulze, W. X. (2012). Nitrate and ammonium lead to distinct global dynamic phosphorylation patterns when resupplied to nitrogen-starved Arabidopsis seedlings. Plant J. 69, 978–995. doi: 10.1111/j.1365-313X.2011.04848.x
Eubel, H., Meyer, E. H., Taylor, N. L., Bussell, J. D., O'Toole, N., Heazlewood, J. L., et al. (2008). Novel proteins, putative membrane transporters, and an integrated metabolic network are revealed by quantitative proteomic analysis of Arabidopsis cell culture peroxisomes. Plant Physiol. 148, 1809–1829. doi: 10.1104/pp.108.129999
Exposito-Rodriguez, M., Laissue, P. P., Yvon-Durocher, G., Smirnoff, N., and Mullineaux, P. M. (2017). Photosynthesis-dependent H2O2 transfer from chloroplasts to nuclei provides a high-light signalling mechanism. Nat. Commun. 8:49. doi: 10.1038/s41467-017-00074-w
Farnese, F. S., Menezes-Silva, P. E., Gusman, G. S., and Oliveira, J. A. (2016). When bad guys become good ones: the key role of reactive oxygen species and nitric oxide in the plant responses to abiotic stress. Front. Plant Sci. 7:471. doi: 10.3389/fpls.2016.00471
Fay, J. M., Zhu, C., Proctor, E. A., Tao, Y., Cui, W., Ke, H., et al. (2016). A phosphomimetic mutation stabilizes SOD1 and rescues cell viability in the context of an ALS-associated mutation. Structure 24, 1898–1906. doi: 10.1016/j.str.2016.08.011
Feng, H., Wang, X., Zhang, Q., Fu, Y., Feng, C., Wang, B., et al. (2014). Monodehydroascorbate reductase gene, regulated by the wheat PN-2013 miRNA, contributes to adult wheat plant resistance to stripe rust through ROS metabolism. Biochim. Biophys. Acta 1839, 1–12. doi: 10.1016/j.bbagrm.2013.11.001
Foyer, C. H. (2018). Reactive oxygen species, oxidative signaling and the regulation of photosynthesis. Environ. Exp. Bot. 154, 134–142. doi: 10.1016/j.envexpbot.2018.05.003
Foyer, C. H., and Noctor, G. (2011). Ascorbate and glutathione: the heart of the redox hub. Plant Physiol. 155, 2–18. doi: 10.1104/pp.110.167569
Foyer, C. H., and Noctor, G. (2020). Redox homeostasis and signaling in a higher-CO2 world. Annu. Rev. Plant Biol. 71, 157–182. doi: 10.1146/annurev-arplant-050718-095955
Frugoli, J. A., Zhong, H. H., Nuccio, M. L., McCourt, P., McPeek, M. A., Thomas, T. L., et al. (1996). Catalase is encoded by a multigene family in Arabidopsis thaliana (L.) Heynh. Plant Physiol. 112, 327–336. doi: 10.1104/pp.112.1.327
Gadjev, I., Vanderauwera, S., Gechev, T. S., Laloi, C., Minkov, I. N., Shulaev, V., et al. (2006). Transcriptomic footprints disclose specificity of reactive oxygen species signaling in Arabidopsis. Plant Physiol. 141, 436–445. doi: 10.1104/pp.106.078717
Gallie, D. R., and Chen, Z. (2019). Chloroplast-localized iron superoxide dismutases FSD2 and FSD3 are functionally distinct in Arabidopsis. PLoS ONE 14:e0220078. doi: 10.1371/journal.pone.0220078
Garcia-Molina, A., Xing, S., and Huijser, P. (2014). Functional characterisation of Arabidopsis SPL7 conserved protein domains suggests novel regulatory mechanisms in the Cu deficiency response. BMC Plant Biol. 14:231. doi: 10.1186/s12870-014-0231-5
Gaupels, F., Durner, J., and Kogel, K.-H. (2017). Production, amplification and systemic propagation of redox messengers in plants? The phloem can do it all! New Phytol. 214, 554–560. doi: 10.1111/nph.14399
Gawroński, P., Witoń, D., Vashutina, K., Bederska, M., Betliński, B., Rusaczonek, A., et al. (2014). Mitogen-activated protein kinase 4 is a salicylic acid-independent regulator of growth but not of photosynthesis in Arabidopsis. Mol. Plant. 7, 1151–1166. doi: 10.1093/mp/ssu060
Geilfus, C.-M., Niehaus, K., Gödde, V., Hasler, M., Zörb, C., Gorzolka, K., et al. (2015). Fast responses of metabolites in Vicia faba L. to moderate NaCl stress. Plant Physiol. Biochem. 92, 19–29. doi: 10.1016/j.plaphy.2015.04.008
Gilroy, S., Białasek, M., Suzuki, N., Górecka, M., Devireddy, A. R., Karpiński, S., et al. (2016). ROS, calcium, and electric signals: key mediators of rapid systemic signaling in plants. Plant Physiol. 171, 1606–1615. doi: 10.1104/pp.16.00434
Gilroy, S., Suzuki, N., Miller, G., Choi, W.-G., Toyota, M., Devireddy, A. R., et al. (2014). A tidal wave of signals: calcium and ROS at the forefront of rapid systemic signaling. Trends Plant Sci. 19, 623–630. doi: 10.1016/j.tplants.2014.06.013
Gleason, C., Huang, S., Thatcher, L. F., Foley, R. C., Anderson, C. R., Carroll, A. J., et al. (2011). Mitochondrial complex II has a key role in mitochondrial-derived reactive oxygen species influence on plant stress gene regulation and defense. Proc. Natl. Acad. Sci. U.S.A. 108, 10768–10773. doi: 10.1073/pnas.1016060108
Gou, J. Y., Li, K., Wu, K., Wang, X., Lin, H., Cantu, D., et al. (2015). Wheat stripe rust resistance protein WKS1 reduces the ability of the thylakoid-associated ascorbate peroxidase to detoxify reactive oxygen species. Plant Cell 27, 1755–1770. doi: 10.1105/tpc.114.134296
Granlund, I., Storm, P., Schubert, M., García-Cerdán, J. G., Funk, C., and Schröder, W. P. (2009). The TL29 protein is lumen located, associated with PSII and not an ascorbate peroxidase. Plant Cell Physiol. 50, 1898–1910. doi: 10.1093/pcp/pcp134
Gudesblat, G. E., Iusem, N. D., and Morris, P. C. (2007). Guard cell-specific inhibition of Arabidopsis MPK3 expression causes abnormal stomatal responses to abscisic acid and hydrogen peroxide. New Phytol. 173, 713–721. doi: 10.1111/j.1469-8137.2006.01953.x
Guo, B., Liu, C., Li, H., Yi, K., Ding, N., Li, N., et al. (2016). Endogenous salicylic acid is required for promoting cadmium tolerance of Arabidopsis by modulating glutathione metabolisms. J. Hazard. Mater. 316, 77–86. doi: 10.1016/j.jhazmat.2016.05.032
Guo, P., Li, Z., Huang, P., Li, B., Fang, S., Chu, J., et al. (2017). A tripartite amplification loop involving the transcription factor WRKY75, salicylic acid, and reactive oxygen species accelerates leaf senescence. Plant Cell 29, 2854–2870. doi: 10.1105/tpc.17.00438
Hackenberg, T., Juul, T., Auzina, A., Gwizdz, S., Malolepszy, A., Van Der Kelen, K., et al. (2013). Catalase and NO CATALASE ACTIVITY1 promote autophagy-dependent cell death in Arabidopsis. Plant Cell 25, 4616–4626. doi: 10.1105/tpc.113.117192
He, H., Van Breusegem, F., and Mhamdi, A. (2018). Redox-dependent control of nuclear transcription in plants. J. Exp. Bot. 69, 3359–3372. doi: 10.1093/jxb/ery130
He, J., Ren, Y., Chen, X., and Chen, H. (2014). Protective roles of nitric oxide on seed germination and seedling growth of rice (Oryza sativa L.) under cadmium stress. Ecotoxicol. Environ. Saf. 108, 114–119. doi: 10.1016/j.ecoenv.2014.05.021
Hehl, R., Norval, L., Romanov, A., and Bülow, L. (2016). Boosting AthaMap database content with data from protein binding microarrays. Plant Cell Physiol. 57:e4. doi: 10.1093/pcp/pcv156
Hiltscher, H., Rudnik, R., Shaikhali, J., Heiber, I., Mellenthin, M., Duarte, I. M., et al. (2014). The radical induced cell death protein 1 (RCD1) supports transcriptional activation of genes for chloroplast antioxidant enzymes. Front. Plant Sci. 5:475. doi: 10.3389/fpls.2014.00475
Holzmeister, C., Gaupels, F., Geerlof, A., Sarioglu, H., Sattler, M., Durner, J., et al. (2015). Differential inhibition of Arabidopsis superoxide dismutases by peroxynitrite-mediated tyrosine nitration. J. Exp. Bot. 66, 989–999. doi: 10.1093/jxb/eru458
Horváth, E., Szalai, G., and Janda, T. (2007). Induction of abiotic stress tolerance by salicylic acid signaling. J. Plant Growth Regul. 26, 290–300. doi: 10.1007/s00344-007-9017-4
Hu, C.-H., Wang, P.-Q., Zhang, P.-P., Nie, X.-M., Li, B.-B., Tai, L., et al. (2020). NADPH Oxidases: the vital performers and center hubs during plant growth and signaling. Cells 9:437. doi: 10.3390/cells9020437
Huang, L., Jia, J., Zhao, X., Zhang, M., Huang, X., Ji, E., et al. (2018). The ascorbate peroxidase APX1 is a direct target of a zinc finger transcription factor ZFP36 and a late embryogenesis abundant protein OsLEA5 interacts with ZFP36 to co-regulate OsAPX1 in seed germination in rice. Biochem. Biophys. Res. Commun. 495, 339–345. doi: 10.1016/j.bbrc.2017.10.128
Huang, W., Yang, Y.-J., and Zhang, S.-B. (2019). The role of water-water cycle in regulating the redox state of photosystem I under fluctuating light. Biochim. Biophys. Acta 1860, 383–390. doi: 10.1016/j.bbabio.2019.03.007
Huda, K. M. K., Banu, M. S. A., Garg, B., Tula, S., Tuteja, R., and Tuteja, N. (2013). OsACA6, a P-type IIB Ca2+ATPase promotes salinity and drought stress tolerance in tobacco by ROS scavenging and enhancing the expression of stress-responsive genes. Plant J. 76, 997–1015. doi: 10.1111/tpj.12352
Hwang, J. E., Lim, C. J., Chen, H., Je, J., Song, C., and Lim, C. O. (2012). Overexpression of Arabidopsis dehydration- responsive element-binding protein 2C confers tolerance to oxidative stress. Mol. Cells 33, 135–140. doi: 10.1007/s10059-012-2188-2
Innocenti, G., Pucciariello, C., Le Gleuher, M., Hopkins, J., de Stefano, M., Delledonne, M., et al. (2007). Glutathione synthesis is regulated by nitric oxide in Medicago truncatula roots. Planta 225, 1597–1602. doi: 10.1007/s00425-006-0461-3
Jammes, F., Song, C., Shin, D., Munemasa, S., Takeda, K., Gu, D., et al. (2009). MAP kinases MPK9 and MPK12 are preferentially expressed in guard cells and positively regulate ROS-mediated ABA signaling. Proc. Natl. Acad. Sci. U.S.A. 106, 20520–20525. doi: 10.1073/pnas.0907205106
Jin, C., Qin, L., Shi, Y., Candas, D., Fan, M., Lu, C. L., et al. (2015). CDK4-mediated MnSOD activation and mitochondrial homeostasis in radioadaptive protection. Free Radic. Biol. Med. 81, 77–87. doi: 10.1016/j.freeradbiomed.2014.12.026
Joo, J. H., Bae, Y. S., and Lee, J. S. (2001). Role of auxin-induced reactive oxygen species in root gravitropism. Plant Physiol. 126, 1055–1060. doi: 10.1104/pp.126.3.1055
Jung, H. S., Crisp, P. A., Estavillo, G. M., Cole, B., Hong, F., Mockler, T. C., et al. (2013). Subset of heat-shock transcription factors required for the early response of Arabidopsis to excess light. Proc. Natl. Acad. Sci. U.S.A. 110, 14474–14479. doi: 10.1073/pnas.1311632110
Kang, Z., Qin, T., and Zhao, Z. (2019). Thioredoxins and thioredoxin reductase in chloroplasts: a review. Gene 706, 32–42. doi: 10.1016/j.gene.2019.04.041
Kangasjärvi, S., Lepistö, A., Hännikäinen, K., Piippo, M., Luomala, E. M., Aro, E. M., et al. (2008). Diverse roles for chloroplast stromal and thylakoid-bound ascorbate peroxidases in plant stress responses. Biochem. J. 412, 275–285. doi: 10.1042/BJ20080030
Karpinski, S., Escobar, C., Karpinska, B., Creissen, G., and Mullineaux, P. M. (1997). Photosynthetic electron transport regulates the expression of cytosolic ascorbate peroxidase genes in Arabidopsis during excess light stress. Plant Cell 9, 627–640. doi: 10.1105/tpc.9.4.627
Kataya, A. R., and Reumann, S. (2010). Arabidopsis glutathione reductase 1 is dually targeted to peroxisomes and the cytosol. Plant Signal. Behav. 5, 171–175. doi: 10.4161/psb.5.2.10527
Kaur, N., and Hu, J. (2011). Defining the plant peroxisomal proteome: from Arabidopsis to rice. Front. Plant Sci. 2:103. doi: 10.3389/fpls.2011.00103
Khan, M. I., Fatma, M., Per, T. S., Anjum, N. A., and Khan, N. A. (2015). Salicylic acid-induced abiotic stress tolerance and underlying mechanisms in plants. Front. Plant Sci. 6:462. doi: 10.3389/fpls.2015.00462
Khedia, J., Agarwal, P., and Agarwal, P. K. (2019). Deciphering hydrogen peroxide-induced signalling towards stress tolerance in plants. 3 Biotech 9, 395. doi: 10.1007/s13205-019-1924-0
Khraiwesh, B., Zhu, J. K., and Zhu, J. (2012). Role of miRNAs and siRNAs in biotic and abiotic stress responses of plants. Biochim. Biophys. Acta 1819, 137–148. doi: 10.1016/j.bbagrm.2011.05.001
Klein, P., Seidel, T., Stöcker, B., and Dietz, K. J. (2012). The membrane-tethered transcription factor ANAC089 serves as redox-dependent suppressor of stromal ascorbate peroxidase gene expression. Front. Plant Sci. 3:247. doi: 10.3389/fpls.2012.00247
Kliebenstein, D. J., Monde, R. A., and Last, R. L. (1998). Superoxide dismutase in Arabidopsis: an eclectic enzyme family with disparate regulation and protein localization. Plant Physiol. 118, 637–650. doi: 10.1104/pp.118.2.637
Kneeshaw, S., Keyani, R., Delorme-Hinoux, V., Imrie, L., Loake, G. J., Le Bihan, T., et al. (2017). Nucleoredoxin guards against oxidative stress by protecting antioxidant enzymes. Proc. Natl. Acad. Sci. U.S.A. 114, 8414–8419. doi: 10.1073/pnas.1703344114
Kobayashi, M., Ohura, I., Kawakita, K., Yokota, N., Fujiwara, M., Shimamoto, K., et al. (2007). Calcium-dependent protein kinases regulate the production of reactive oxygen species by potato NADPH oxidase. Plant Cell 19, 1065–1080. doi: 10.1105/tpc.106.048884
Kohli, S. K., Khanna, K., Bhardwaj, R., Abd Allah, E. F., Ahmad, P., and Corpas, F. J. (2019). Assessment of subcellular ROS and NO metabolism in higher plants: multifunctional signaling molecules. Antioxidants 8:641. doi: 10.3390/antiox8120641
Komis, G., Šamajová, O., Ovečka, M., and Šamaj, J. (2018). Cell and developmental biology of plant mitogen-activated protein kinases. Annu. Rev. Plant Biol. 69, 237–265. doi: 10.1146/annurev-arplant-042817-040314
Kornyeyev, D., Logan, B. A., Payton, P. R., Allen, R. D., and Holaday, A. S. (2003). Elevated chloroplastic glutathione reductase activities decrease chilling-induced photoinhibition by increasing rates of photochemistry, but not thermal energy dissipation, in transgenic cotton. Funct. Plant Biol. 30, 101–110. doi: 10.1071/FP02144
Koussevitzky, S., Suzuki, N., Huntington, S., Armijo, L., Sha, W., Cortes, D., et al. (2008). Ascorbate peroxidase 1 plays a key role in the response of Arabidopsis thaliana to stress combination. J. Biol. Chem. 283, 34197–34203. doi: 10.1074/jbc.M806337200
Kovacs, I., Holzmeister, C., Wirtz, M., Geerlof, A., Fröhlich, T., Römling, G., et al. (2016). ROS-mediated inhibition of S-nitrosoglutathione reductase contributes to the activation of anti-oxidative Mechanisms. Front. Plant Sci. 7:1669. doi: 10.3389/fpls.2016.01669
Kovtun, Y., Chiu, W. L., Tena, G., and Sheen, J. (2000). Functional analysis of oxidative stress-activated mitogen-activated protein kinase cascade in plants. Proc. Natl. Acad. Sci. U.S.A. 97, 2940–2945. doi: 10.1073/pnas.97.6.2940
Kreps, J. A., Wu, Y., Chang, H. S., Zhu, T., Wang, X., and Harper, J. F. (2002). Transcriptome changes for Arabidopsis in response to salt, osmotic, and cold stress. Plant Physiol. 130, 2129–2141. doi: 10.1104/pp.008532
Kumar, M., Gouw, M., Michael, S., Sámano-Sánchez, H., Pancsa, R., Glavina, J., et al. (2020). ELM-the eukaryotic linear motif resource in 2020. Nucleic Acids Res. 48, D296–D306. doi: 10.1093/nar/gkz1030
Kumar, S., Sud, N., Fonseca, F. V., Hou, Y., and Black, S. M. (2010). Shear stress stimulates nitric oxide signaling in pulmonary arterial endothelial cells via a reduction in catalase activity: role of protein kinase C delta. Am. J. Physiol. Lung Cell Mol. Physiol. 298, L105–L116. doi: 10.1152/ajplung.00290.2009
Kuo, W. Y., Huang, C. H., Liu, A. C., Cheng, C. P., Li, S. H., Chang, W. C., et al. (2013). CHAPERONIN 20 mediates iron superoxide dismutase (FeSOD) activity independent of its co-chaperonin role in Arabidopsis chloroplasts. New Phytol. 197, 99–110. doi: 10.1111/j.1469-8137.2012.04369.x
Lehmann, S., Serrano, M., L'Haridon, F., Tjamos, S. E., and Metraux, J.-P. (2015). Reactive oxygen species and plant resistance to fungal pathogens. Phytochemistry 112, 54–62. doi: 10.1016/j.phytochem.2014.08.027
Leitch, J. M., Li, C. X., Baron, J. A., Matthews, L. M., Cao, X., Hart, P. J., et al. (2012). Post-translational modification of Cu/Zn superoxide dismutase under anaerobic conditions. Biochemistry 51, 677–685. doi: 10.1021/bi201353y
Li, H., Wong, W. S., Zhu, L., Guo, H. W., Ecker, J., and Li, N. (2009). Phosphoproteomic analysis of ethylene-regulated protein phosphorylation in etiolated seedlings of Arabidopsis mutant ein2 using two-dimensional separations coupled with a hybrid quadrupole time-of-flight mass spectrometer. Proteomics 9, 1646–1661. doi: 10.1002/pmic.200800420
Li, X., Makavitskaya, M., Samokhina, V., Mackievic, V., Navaselsky, I., Hryvusevich, P., et al. (2018). Effects of exogenously-applied L-ascorbic acid on root expansive growth and viability of the border-like cells. Plant Signal. Behav. 13:e1514895. doi: 10.1080/15592324.2018.1514895
Lin, F., Ding, H., Wang, J., Zhang, H., Zhang, A., Zhang, Y., et al. (2009). Positive feedback regulation of maize NADPH oxidase by mitogen-activated protein kinase cascade in abscisic acid signalling. J. Exp. Bot. 60, 3221–3238. doi: 10.1093/jxb/erp157
Lin, L. L., Hsu, C. L., Hu, C. W., Ko, S. Y., Hsieh, H. L., Huang, H. C., et al. (2015). Integrating phosphoproteomics and bioinformatics to study brassinosteroid-regulated phosphorylation dynamics in Arabidopsis. BMC Genom. 16, 533. doi: 10.1186/s12864-015-1753-4
Lindermayr, C. (2018). Crosstalk between reactive oxygen species and nitric oxide in plants: key role of S-nitrosoglutathione reductase. Free Radic. Biol. Med. 122, 110–115. doi: 10.1016/j.freeradbiomed.2017.11.027
Lindermayr, C., and Durner, J. (2015). Interplay of reactive oxygen species and nitric oxide: nitric oxide coordinates reactive oxygen species homeostasis. Plant Physiol. 167, 1209–1210. doi: 10.1104/pp.15.00293
Lisenbee, C. S., Lingard, M. J., and Trelease, R. N. (2005). Arabidopsis peroxisomes possess functionally redundant membrane and matrix isoforms of monodehydroascorbate reductase. Plant J. 43, 900–914. doi: 10.1111/j.1365-313X.2005.02503.x
Liu, P., Sun, F., Gao, R., and Dong, H. (2012). RAP2.6L overexpression delays waterlogging induced premature senescence by increasing stomatal closure more than antioxidant enzyme activity. Plant Mol. Biol. 79, 609–622. doi: 10.1007/s11103-012-9936-8
Liu, Y., and He, C. (2017). A review of redox signaling and the control of MAP kinase pathway in plants. Redox Biol. 11, 192–204. doi: 10.1016/j.redox.2016.12.009
Locato, V., Cimini, S., and De Gara, L. (2017). “Glutathione as a key player in plant abiotic stress responses and tolerance,” in Glutathione in Plant Growth, Development, and Stress Tolerance, eds M. A. Hossain, M. G. Mostofa, P. Diaz-Vivancos, D. J. Burritt, M. Fujita, and L.-S. P. Tran (Cham: Springer International Publishing), 127–145. doi: 10.1007/978-3-319-66682-2_6
Ma, C., Burd, S., and Lers, A. (2015). miR408 is involved in abiotic stress responses in Arabidopsis. Plant J. 84, 169–187. doi: 10.1111/tpj.12999
Marcec, M. J., Gilroy, S., Poovaiah, B. W., and Tanaka, K. (2019). Mutual interplay of Ca2+ and ROS signaling in plant immune response. Plant Sci. 283, 343–354. doi: 10.1016/j.plantsci.2019.03.004
Marchand, C. H., Vanacker, H., Collin, V., Issakidis-Bourguet, E., Maréchal, P. L., and Decottignies, P. (2010). Thioredoxin targets in Arabidopsis roots. Proteomics 10, 2418–2428. doi: 10.1002/pmic.200900835
Marty, L., Bausewein, D., Müller, C., Bangash, S., Moseler, A., Schwarzländer, M., et al. (2019). Arabidopsis glutathione reductase 2 is indispensable in plastids, while mitochondrial glutathione is safeguarded by additional reduction and transport systems. New Phytol. 224, 1569–1584. doi: 10.1111/nph.16086
Maruta, T., Inoue, T., Noshi, M., Tamoi, M., Yabuta, Y., Yoshimura, K., et al. (2012). Cytosolic ascorbate peroxidase 1 protects organelles against oxidative stress by wounding- and jasmonate-induced H2O2 in Arabidopsis plants. Biochim. Biophys. Acta 1820, 1901–1907. doi: 10.1016/j.bbagen.2012.08.003
Maruta, T., Sawa, Y., Shigeoka, S., and Ishikawa, T. (2016). Diversity and evolution of ascorbate peroxidase functions in chloroplasts: more than just a classical antioxidant enzyme? Plant Cell Physiol. 57, 1377–1386. doi: 10.1093/pcp/pcv203
Maruta, T., Tanouchi, A., Tamoi, M., Yabuta, Y., Yoshimura, K., Ishikawa, T., et al. (2010). Arabidopsis chloroplastic ascorbate peroxidase isoenzymes play a dual role in photoprotection and gene regulation under photooxidative stress. Plant Cell Physiol. 51, 190–200. doi: 10.1093/pcp/pcp177
Mayank, P., Grossman, J., Wuest, S., Boisson-Dernier, A., Roschitzki, B., Nanni, P., et al. (2012). Characterization of the phosphoproteome of mature Arabidopsis pollen. Plant J. 72, 89–101. doi: 10.1111/j.1365-313X.2012.05061.x
Meyer, A. J., Dreyer, A., Ugalde, J. M., Feitosa-Araujo, E., Dietz, K.-J., and Schwarzländer, M. (in press). Shifting paradigms novel players in Cys-based redox regulation ROS signaling in plants - where to go next. Biol. Chem. doi: 10.1515/hsz-2020-0291
Mhamdi, A., Noctor, G., and Baker, A. (2012). Plant catalases: peroxisomal redox guardians. Arch. Biochem. Biophys. 525, 181–194. doi: 10.1016/j.abb.2012.04.015
Mhamdi, A., Queval, G., Chaouch, S., Vanderauwera, S., Van Breusegem, F., and Noctor, G. (2010). Catalase function in plants: a focus on Arabidopsis mutants as stress-mimic models. J. Exp. Bot. 61, 4197–4220. doi: 10.1093/jxb/erq282
Mhamdi, A., and Van Breusegem, F. (2018). Reactive oxygen species in plant development. Development 145:dev164376. doi: 10.1242/dev.164376
Miao, Y., Laun, T., Zimmermann, P., and Zentgraf, U. (2004). Targets of the WRKY53 transcription factor and its role during leaf senescence in Arabidopsis. Plant Mol. Biol. 55, 853–867. doi: 10.1007/s11103-004-2142-6
Miao, Y., Laun, T. M., Smykowski, A., and Zentgraf, U. (2007). Arabidopsis MEKK1 can take a short cut: it can directly interact with senescence-related WRKY53 transcription factor on the protein level and can bind to its promoter. Plant Mol. Biol. 65, 63–76. doi: 10.1007/s11103-007-9198-z
Mignolet-Spruyt, L., Xu, E., Idänheimo, N., Hoeberichts, F. A., Mühlenbock, P., Brosché, M., et al. (2016). Spreading the news: subcellular and organellar reactive oxygen species production and signalling. J. Exp. Bot. 67, 3831–3844. doi: 10.1093/jxb/erw080
Miller, E. W., Dickinson, B. C., and Chang, C. J. (2010). Aquaporin-3 mediates hydrogen peroxide uptake to regulate downstream intracellular signaling. Proc. Natl. Acad. Sci. U.S.A. 107, 15681–15686. doi: 10.1073/pnas.1005776107
Mithoe, S. C., Boersema, P. J., Berke, L., Snel, B., Heck, A. J., and Menke, F. L. (2012). Targeted quantitative phosphoproteomics approach for the detection of phospho-tyrosine signaling in plants. J. Proteome Res. 11, 438–448. doi: 10.1021/pr200893k
Mittler, R., Kim, Y., Song, L., Coutu, J., Coutu, A., Ciftci-Yilmaz, S., et al. (2006). Gain- and loss-of-function mutations in Zat10 enhance the tolerance of plants to abiotic stress. FEBS Lett. 580, 6537–6542. doi: 10.1016/j.febslet.2006.11.002
Mittler, R., Vanderauwera, S., Suzuki, N., Miller, G., Tognetti, V. B., Vandepoele, K., et al. (2011). ROS signaling: the new wave? Trends Plant Sci. 16, 300–309. doi: 10.1016/j.tplants.2011.03.007
Morgan, M. J., Lehmann, M., Schwarzländer, M., Baxter, C. J., Sienkiewicz-Porzucek, A., Williams, T. C., et al. (2008). Decrease in manganese superoxide dismutase leads to reduced root growth and affects tricarboxylic acid cycle flux and mitochondrial redox homeostasis. Plant Physiol. 147, 101–114. doi: 10.1104/pp.107.113613
Müller-Schüssele, S. J., Wang, R., Gütle, D. D., Romer, J., Rodriguez-Franco, M., Scholz, M., et al. (2020). Chloroplasts require glutathione reductase to balance reactive oxygen species and maintain efficient photosynthesis. Plant J. 103, 1140–1154. doi: 10.1111/tpj.14791
Muñoz, P., and Munné-Bosch, S. (2019). Vitamin E in plants: biosynthesis, transport, and function. Trends Plant Sci. 24, 1040–1051. doi: 10.1016/j.tplants.2019.08.006
Murgia, I., Tarantino, D., Vannini, C., Bracale, M., Carravieri, S., and Soave, C. (2004). Arabidopsis thaliana plants overexpressing thylakoidal ascorbate peroxidase show increased resistance to Paraquat-induced photooxidative stress and to nitric oxide-induced cell death. Plant J. 38, 940–953. doi: 10.1111/j.1365-313X.2004.02092.x
Myouga, F., Hosoda, C., Umezawa, T., Iizumi, H., Kuromori, T., Motohashi, R., et al. (2008). A heterocomplex of iron superoxide dismutases defends chloroplast nucleoids against oxidative stress and is essential for chloroplast development in Arabidopsis. Plant Cell 20, 3148–3162. doi: 10.1105/tpc.108.061341
Nakagami, H., Soukupová, H., Schikora, A., Zárský, V., and Hirt, H. (2006). A Mitogen-activated protein kinase kinase kinase mediates reactive oxygen species homeostasis in Arabidopsis. J. Biol. Chem. 281, 38697–38704. doi: 10.1074/jbc.M605293200
Nakagami, H., Sugiyama, N., Mochida, K., Daudi, A., Yoshida, Y., Toyoda, T., et al. (2010). Large-scale comparative phosphoproteomics identifies conserved phosphorylation sites in plants. J. Plant Physiol. 153, 1161–1174. doi: 10.1104/pp.110.157347
Narendra, S., Venkataramani, S., Shen, G., Wang, J., Pasapula, V., Lin, Y., et al. (2006). The Arabidopsis ascorbate peroxidase 3 is a peroxisomal membrane-bound antioxidant enzyme and is dispensable for Arabidopsis growth and development. J. Exp. Bot. 57, 3033–3042. doi: 10.1093/jxb/erl060
Niu, L., and Liao, W. (2016). Hydrogen peroxide signaling in plant development and abiotic responses: crosstalk with nitric oxide and calcium. Front Plant Sci. 7:230. doi: 10.3389/fpls.2016.00230
Noctor, G., Reichheld, J.-P., and Foyer, C. H. (2017). ROS-related redox regulation and signaling in plants. Cell Dev. Biol. 80, 3–12. doi: 10.1016/j.semcdb.2017.07.013
Noshi, M., Hatanaka, R., Tanabe, N., Terai, Y., Maruta, T., and Shigeoka, S. (2016). Redox regulation of ascorbate and glutathione by a chloroplastic dehydroascorbate reductase is required for high-light stress tolerance in Arabidopsis. Biosci. Biotechnol. Biochem. 80, 870–877. doi: 10.1080/09168451.2015.1135042
Noshi, M., Yamada, H., Hatanaka, R., Tanabe, N., Tamoi, M., and Shigeoka, S. (2017). Arabidopsis dehydroascorbate reductase 1 and 2 modulate redox states of ascorbate-glutathione cycle in the cytosol in response to photooxidative stress. Biosci. Biotechnol. Biochem. 81, 523–533. doi: 10.1080/09168451.2016.1256759
Obara, K., Sumi, K., and Fukuda, H. (2002). The use of multiple transcription starts causes the dual targeting of Arabidopsis putative monodehydroascorbate reductase to both mitochondria and chloroplasts. Plant Cell Physiol. 43, 697–705. doi: 10.1093/pcp/pcf103
Obayashi, T., Aoki, Y., Tadaka, S., Kagaya, Y., and Kinoshita, K. (2018). ATTED-II in 2018: a plant coexpression database based on investigation of the statistical property of the mutual rank index. Plant Cell Physiol. 59:e3. doi: 10.1093/pcp/pcx191
Ono, M., Isono, K., Sakata, Y., and Taji, T. (2020). CATALASE2 plays a crucial role in long-term heat tolerance of Arabidopsis thaliana. Biochem. Biophys. Res. Commun. 534, 747–751. doi: 10.1016/j.bbrc.2020.11.006
Ortega-Galisteo, A. P., Rodríguez-Serrano, M., Pazmiño, D. M., Gupta, D. K., Sandalio, L. M., and Romero-Puertas, M. C. (2012). S-Nitrosylated proteins in pea (Pisum sativum L.) leaf peroxisomes: changes under abiotic stress. J. Exp. Bot. 63, 2089–2103. doi: 10.1093/jxb/err414
Ortiz-Masia, D., Perez-Amador, M. A., Carbonell, J., and Marcote, M. J. (2007). Diverse stress signals activate the C1 subgroup MAP kinases of Arabidopsis. FEBS Lett. 581, 1834–1840. doi: 10.1016/j.febslet.2007.03.075
Palma, J. M., Mateos, R. M., López-Jaramillo, J., Rodríguez-Ruiz, M., González-Gordo, S., Lechuga-Sancho, A. M., et al. (2020). Plant catalases as NO and H2S targets. Redox Biol. 34:101525. doi: 10.1016/j.redox.2020.101525
Pandey, S., Fartyal, D., Agarwal, A., Shukla, T., James, D., Kaul, T., et al. (2017). Abiotic stress tolerance in plants: myriad roles of ascorbate peroxidase. Front. Plant Sci. 8:581. doi: 10.3389/fpls.2017.00581
Pasternak, T. P., Ötvös, K., Domoki, M., and Fehér, A. (2007). Linked activation of cell division and oxidative stress defense in alfalfa leaf protoplast-derived cells is dependent on exogenous auxin. Plant Growth Regul. 51, 109–117. doi: 10.1007/s10725-006-9152-0
Pei, Z.-M., Murata, Y., Benning, G., Thomine, S., Klüsener, B., Allen, G. J., et al. (2000). Calcium channels activated by hydrogen peroxide mediate abscisic acid signalling in guard cells. Nature 406, 731–734. doi: 10.1038/35021067
Perea-García, A., Andrés-Bordería, A., Mayo de Andrés, S., Sanz, A., Davis, A. M., Davis, S., et al. (2016). Modulation of copper deficiency responses by diurnal and circadian rhythms in Arabidopsis thaliana. J. Exp. Bot. 67, 391–403. doi: 10.1093/jxb/erv474
Pérez-Salamó, I., Papdi, C., Rigó, G., Zsigmond, L., Vilela, B., Lumbreras, V., et al. (2014). The heat shock factor A4A confers salt tolerance and is regulated by oxidative stress and the mitogen-activated protein kinases MPK3 and MPK6. Plant Physiol. 165, 319–334. doi: 10.1104/pp.114.237891
Persak, H., and Pitzschke, A. (2013). Tight interconnection and multi-level control of Arabidopsis MYB44 in MAPK cascade signalling. PLoS ONE 8:e57547. doi: 10.1371/journal.pone.0057547
Persak, H., and Pitzschke, A. (2014). Dominant repression by Arabidopsis transcription factor MYB44 causes oxidative damage and hypersensitivity to abiotic stress. Int. J. Mol. Sci. 15, 2517–2537. doi: 10.3390/ijms15022517
Petrov, V., Hille, J., Mueller-Roeber, B., and Gechev, T. S. (2015). ROS-mediated abiotic stress-induced programmed cell death in plants. Front. Plant Sci. 6:69. doi: 10.3389/fpls.2015.00069
Pilon, M., Ravet, K., and Tapken, W. (2011). The biogenesis and physiological function of chloroplast superoxide dismutases. Biochim. Biophys. Acta 1807, 989–998. doi: 10.1016/j.bbabio.2010.11.002
Piterková, J., Luhová, L., Navrátilová, B., Sedlárová, M., and Petrivalsky, M. (2015). Early and long-term responses of cucumber cells to high cadmium concentration are modulated by nitric oxide and reactive oxygen species. Acta Physiol. Plant 37:19. doi: 10.1007/s11738-014-1756-9
Pitzschke, A., Djamei, A., Bitton, F., and Hirt, H. (2009). A major role of the MEKK1-MKK1/2-MPK4 pathway in ROS signalling. Mol. Plant 2, 120–137. doi: 10.1093/mp/ssn079
Pnueli, L., Liang, H., Rozenberg, M., and Mittler, R. (2003). Growth suppression, altered stomatal responses, and augmented induction of heat shock proteins in cytosolic ascorbate peroxidase (Apx1)-deficient Arabidopsis plants. Plant J. 34, 187–203. doi: 10.1046/j.1365-313x.2003.01715.x
Pospíšil, P. (2016). Production of reactive oxygen species by photosystem II as a response to light and temperature stress. Front. Plant Sci. 7:1950. doi: 10.3389/fpls.2016.01950
Rafikov, R., Kumar, S., Aggarwal, S., Hou, Y., Kangath, A., Pardo, D., et al. (2014). Endothelin-1 stimulates catalase activity through the PKCδ-mediated phosphorylation of serine 167. Free Radic. Biol. Med. 67, 255–264. doi: 10.1016/j.freeradbiomed.2013.10.814
Rahantaniaina, M.-S., Li, S., Chatel-Innocenti, G., Tuzet, A., Issakidis-Bourguet, E., Mhamdi, A., et al. (2017). Cytosolic and chloroplastic DHARs cooperate in oxidative stress-driven activation of the salicylic acid pathway. Plant Physiol. 174, 956–971. doi: 10.1104/pp.17.00317
Rahman, A., Mostofa, M. G., Alam, M. M., Nahar, K., Hasanuzzaman, M., and Fujita, M. (2015). Calcium mitigates arsenic toxicity in rice seedlings by reducing arsenic uptake and modulating the antioxidant defense and glyoxalase systems and stress markers. Biomed Res. Int. 2015:340812. doi: 10.1155/2015/340812
Rayapuram, N., Bigeard, J., Alhoraibi, H., Bonhomme, L., Hesse, A. M., Vinh, J., et al. (2018). Quantitative phosphoproteomic analysis reveals shared and specific targets of Arabidopsis mitogen-activated protein kinases (MAPKs) MPK3, MPK4, and MPK6. Mol. Cell. Proteom. 17, 61–80. doi: 10.1074/mcp.RA117.000135
Rayapuram, N., Bonhomme, L., Bigeard, J., Haddadou, K., Przybylski, C., Hirt, H., et al. (2014). Identification of novel PAMP-triggered phosphorylation and dephosphorylation events in Arabidopsis thaliana by quantitative phosphoproteomic analysis. J. Proteome Res. 13, 2137–2151. doi: 10.1021/pr401268v
Reiland, S., Finazzi, G., Endler, A., Willig, A., Baerenfaller, K., Grossmann, J., et al. (2011). Comparative phosphoproteome profiling reveals a function of the STN8 kinase in fine-tuning of cyclic electron flow (CEF). Proc. Natl. Acad. Sci. U.S.A. 108, 12955–12960. doi: 10.1073/pnas.1104734108
Reiland, S., Messerli, G., Baerenfaller, K., Gerrits, B., Endler, A., Grossmann, J., et al. (2009). Large-scale Arabidopsis phosphoproteome profiling reveals novel chloroplast kinase substrates and phosphorylation networks. Plant Physiol. 150, 889–903. doi: 10.1104/pp.109.138677
Rentel, M. C., Lecourieux, D., Ouaked, F., Usher, S. L., Petersen, L., Okamoto, H., et al. (2004). OXI1 kinase is necessary for oxidative burst-mediated signalling in Arabidopsis. Nature 427, 858–861. doi: 10.1038/nature02353
Richards, S. L., Laohavisit, A., Mortimer, J. C., Shabala, L., Swarbreck, S. M., Shabala, S., et al. (2014). Annexin 1 regulates the H2O2-induced calcium signature in Arabidopsis thaliana roots. Plant J. 77, 136–145. doi: 10.1111/tpj.12372
Riester, L., Köster-Hofmann, S., Doll, J., Berendzen, K. W., and Zentgraf, U. (2019). Impact of alternatively polyadenylated isoforms of ETHYLENE RESPONSE FACTOR4 with activator and repressor function on senescence in Arabidopsis thaliana L. Genes 10:91. doi: 10.3390/genes10020091
Rizhsky, L., Davletova, S., Liang, H., and Mittler, R. (2004b). The zinc finger protein Zat12 is required for cytosolic ascorbate peroxidase 1 expression during oxidative stress in Arabidopsis. J. Biol. Chem. 279, 11736–11743. doi: 10.1074/jbc.M313350200
Rizhsky, L., Liang, H., and Mittler, R. (2003). The water-water cycle is essential for chloroplast protection in the absence of stress. J. Biol. Chem. 278, 38921–38925. doi: 10.1074/jbc.M304987200
Rizhsky, L., Liang, H., Shuman, J., Shulaev, V., Davletova, S., and Mittler, R. (2004a). When defense pathways collide. The response of Arabidopsis to a combination of drought and heat stress. Plant Physiol. 134, 1683–1696. doi: 10.1104/pp.103.033431
Rizwan, M., Mostofa, M. G., Ahmad, M. Z., Imtiaz, M., Mehmood, S., Adeel, M., et al. (2018). Nitric oxide induces rice tolerance to excessive nickel by regulating nickel uptake, reactive oxygen species detoxification and defense-related gene expression. Chemosphere 191, 23–35. doi: 10.1016/j.chemosphere.2017.09.068
Roitinger, E., Hofer, M., Köcher, T., Pichler, P., Novatchkova, M., Yang, J., et al. (2015). Quantitative phosphoproteomics of the ataxia telangiectasia-mutated (ATM) and ataxia telangiectasia-mutated and rad3-related (ATR) dependent DNA damage response in Arabidopsis thaliana. Mol. Cell Proteomics 14, 556–571. doi: 10.1074/mcp.M114.040352
Romeis, T., and Herde, M. (2014). From local to global: CDPKs in systemic defense signaling upon microbial and herbivore attack. Curr. Opin. Plant Biol. 20, 1–10. doi: 10.1016/j.pbi.2014.03.002
Romero-Puertas, M. C., and Sandalio, L. M. (2016). Nitric oxide level is self-regulating and also regulates its ROS partners. Front. Plant Sci. 7:316. doi: 10.3389/fpls.2016.00316
Rossel, J. B., Walter, P. B., Hendrickson, L., Chow, W. S., Poole, A., Mullineaux, P. M., et al. (2006). A mutation affecting ASCORBATE PEROXIDASE 2 gene expression reveals a link between responses to high light and drought tolerance. Plant Cell Environ. 29, 269–281. doi: 10.1111/j.1365-3040.2005.01419.x
Rudnik, R., Bulcha, J. T., Reifschneider, E., Ellersiek, U., and Baier, M. (2017). Specificity versus redundancy in the RAP2.4 transcription factor family of Arabidopsis thaliana: transcriptional regulation of genes for chloroplast peroxidases. BMC Plant Biol. 17:144. doi: 10.1186/s12870-017-1092-5
Ruiz-May, E., Segura-Cabrera, A., Elizalde-Contreras, J. M., Shannon, L. M., and Loyola-Vargas, V. M. (2019). A recent advance in the intracellular and extracellular redox post-translational modification of proteins in plants. J. Mol. Recogn. 32:e2754. doi: 10.1002/jmr.2754
Sagi, M., and Fluhr, R. (2006). Production of reactive oxygen species by plant NADPH oxidases. Plant Physiol. 141, 336–340. doi: 10.1104/pp.106.078089
Šamajová, O., Plíhal, O., Al-Yousif, M., Hirt, H., and Šamaj, J. (2013). Improvement of stress tolerance in plants by genetic manipulation of mitogen-activated protein kinases. Biotechnol. Adv. 31, 118–128. doi: 10.1016/j.biotechadv.2011.12.002
Savatin, D. V., Bisceglia, N. G., Marti, L., Fabbri, C., Cervone, F., and De Lorenzo, G. (2014). The Arabidopsis NUCLEUS- AND PHRAGMOPLAST-LOCALIZED KINASE1-related protein kinases are required for elicitor-induced oxidative burst and immunity. Plant Physiol. 165, 1188–1202. doi: 10.1104/pp.114.236901
Schopfer, P., Liszkay, A., Bechtold, M., Frahry, G., and Wagner, A. (2002). Evidence that hydroxyl radicals mediate auxin-induced extension growth. Planta 214, 821–828. doi: 10.1007/s00425-001-0699-8
Schulz, P., Herde, M., and Romeis, T. (2013). Calcium-dependent protein kinases: hubs in plant stress signaling and development. Plant Physiol. 163, 523–530. doi: 10.1104/pp.113.222539
Sewelam, N., Kazan, K., Thomas-Hall, S. R., Kidd, B. N., Manners, J. M., and Schenk, P. M. (2013). Ethylene response factor 6 is a regulator of reactive oxygen species signaling in Arabidopsis. PLoS ONE 8:e70289. doi: 10.1371/journal.pone.0070289
Shafi, A., Chauhan, R., Gill, T., Swarnkar, M. K., Sreenivasulu, Y., Kumar, S., et al. (2015). Expression of SOD and APX genes positively regulates secondary cell wall biosynthesis and promotes plant growth and yield in Arabidopsis under salt stress. Plant Mol. Biol. 87, 615–631. doi: 10.1007/s11103-015-0301-6
Shaikhali, J., Heiber, I., Seidel, T., Ströher, E., Hiltscher, H., Birkmann, S., et al. (2008). The redox-sensitive transcription factor Rap2.4a controls nuclear expression of 2-Cys peroxiredoxin A and other chloroplast antioxidant enzymes. BMC Plant Biol. 8:48. doi: 10.1186/1471-2229-8-48
Shan, C., and Yang, T. (2017). Nitric oxide acts downstream of hydrogen peroxide in the regulation of ascorbate and glutathione metabolism by jasmonic acid in Agropyron cristatum leaves. Biol. Plant. 61, 779–784. doi: 10.1007/s10535-017-0708-9
Shapiguzov, A., Vainonen, J. P., Hunter, K., Tossavainen, H., Tiwari, A., Järvi, S., et al. (2019). Arabidopsis RCD1 coordinates chloroplast and mitochondrial functions through interaction with ANAC transcription factors. Elife 8:e43284. doi: 10.7554/eLife.43284
Sharma, I., and Ahmad, P. (2014). “Catalase: a versatile antioxidant in plants,” in Oxidative Damage to Plants, ed P. Ahmad (Srinagar: S.P. College), 131–148.
Shukla, P., and Singh, A. K. (2015). Nitric oxide mitigates arsenic-induced oxidative stress and genotoxicity in Vicia faba L. Environ. Sci. Pollut. Res. 22, 13881–13891. doi: 10.1007/s11356-015-4501-z
Sierla, M., Waszczak, C., Vahisalu, T., and Kangasjärvi, J. (2016). Reactive oxygen species in the regulation of stomatal movements. Plant Physiol. 171, 1569–1580. doi: 10.1104/pp.16.00328
Smékalová, V., Doskočilová, A., Komis, G., and Šamaj, J. (2014). Crosstalk between secondary messengers, hormones and MAPK modules during abiotic stress signalling in plants. Biotechnol. Adv. 32, 2–11. doi: 10.1016/j.biotechadv.2013.07.009
Smirnoff, N. (2018). Ascorbic acid metabolism and functions: a comparison of plants and mammals. Free Radic. Biol. Med. 122, 116–129. doi: 10.1016/j.freeradbiomed.2018.03.033
Smirnoff, N., and Arnaud, D. (2019). Hydrogen peroxide metabolism and functions in plants. New Phytol. 221, 1197–1214. doi: 10.1111/nph.15488
Song, C., Chung, W. S., and Lim, C. O. (2016). Overexpression of heat shock factor gene HsfA3 increases galactinol levels and oxidative stress tolerance in Arabidopsis. Mol. Cells 39, 477–483. doi: 10.14348/molcells.2016.0027
Su, T., Wang, P., Li, H., Zhao, Y., Lu, Y., Dai, P., et al. (2018). The Arabidopsis catalase triple mutant reveals important roles of catalases and peroxisome-derived signaling in plant development. J. Integr. Plant Biol. 60, 591–607. doi: 10.1111/jipb.12649
Sugiyama, N., Nakagami, H., Mochida, K., Daudi, A., Tomita, M., Shirasu, K., et al. (2008). Large-scale phosphorylation mapping reveals the extent of tyrosine phosphorylation in Arabidopsis. Mol. Syst. Biol. 4:193. doi: 10.1038/msb.2008.32
Sultana, S., Khew, C. Y., Morshed, M. M., Namasivayam, P., Napis, S., and Ho, C. L. (2012). Overexpression of monodehydroascorbate reductase from a mangrove plant (AeMDHAR) confers salt tolerance on rice. J. Plant Physiol. 169, 311–318. doi: 10.1016/j.jplph.2011.09.004
Sumugat, M. R., Donahue, J. L., Cortes, D. F., Stromberg, V. K., Grene, R., Shulaev, V., et al. (2010). Seed development and germination in an Arabidopsis thaliana line antisense to glutathione reductase 2. J. New Seeds 11, 104–126. doi: 10.1080/15228861003776175
Sunkar, R., Kapoor, A., and Zhu, J. K. (2006). Posttranscriptional induction of two Cu/Zn superoxide dismutase genes in Arabidopsis is mediated by downregulation of miR398 and important for oxidative stress tolerance. Plant Cell 18, 2051–2065. doi: 10.1105/tpc.106.041673
Suzuki, N., Miller, G., Sejima, H., Harper, J., and Mittler, R. (2013). Enhanced seed production under prolonged heat stress conditions in Arabidopsis thaliana plants deficient in cytosolic ascorbate peroxidase 2. J. Exp. Bot. 64, 253–263. doi: 10.1093/jxb/ers335
Suzuki, Y. J., Carini, M., and Butterfield, D. A. (2010). Protein carbonylation. Antioxid. Redox Signal. 12, 323–325. doi: 10.1089/ars.2009.2887
Takáč, T., Obert, B., Rolčík, J., and Šamaj, J. (2016a). Improvement of adventitious root formation in flax using hydrogen peroxide. N. Biotechnol. 33, 728–734. doi: 10.1016/j.nbt.2016.02.008
Takáč, T., Šamajová, O., Vadovič, P., Pechan, T., Košútová, P., Ovečka, M., et al. (2014). Proteomic and biochemical analyses show a functional network of proteins involved in antioxidant defense of the Arabidopsis anp2anp3 double mutant. J. Proteome Res. 13, 5347–5361. doi: 10.1021/pr500588c
Takáč, T., Vadovič, P., Pechan, T., Luptovčiak, I., Šamajová, O., and Šamaj, J. (2016b). Comparative proteomic study of Arabidopsis mutants mpk4 and mpk6. Sci. Rep. 6:28306. doi: 10.1038/srep28306
Teh, O.-K., and Hofius, D. (2014). Membrane trafficking and autophagy in pathogen-triggered cell death and immunity. J. Exp. Bot. 65, 1297–1312. doi: 10.1093/jxb/ert441
Tian, W., Wang, C., Gao, Q., Li, L., and Luan, S. (2020). Calcium spikes, waves and oscillations in plant development and biotic interactions. Nat. Plants 6, 750–759. doi: 10.1038/s41477-020-0667-6
Tichá, T., Lochman, J., Cinčalová, L., Luhová, L., and Petrivalský, M. (2017). Redox regulation of plant S-nitrosoglutathione reductase activity through post-translational modifications of cysteine residues. Biochem. Biophys. Res. Commun. 494, 27–33. doi: 10.1016/j.bbrc.2017.10.090
Tognetti, V. B., Van Aken, O., Morreel, K., Vandenbroucke, K., van de Cotte, B., De Clercq, I., et al. (2010). Perturbation of indole-3-butyric acid homeostasis by the UDP-glucosyltransferase UGT74E2 modulates Arabidopsis architecture and water stress tolerance. Plant Cell 22, 2660–2679. doi: 10.1105/tpc.109.071316
Tsang, C. K., Chen, M., Cheng, X., Qi, Y., Chen, Y., Das, I., et al. (2018). SOD1 phosphorylation by mTORC1 couples nutrient sensing and redox regulation. Mol. Cell 70, 502–515.e8. doi: 10.1016/j.molcel.2018.03.029
Tsang, C. K., Liu, Y., Thomas, J., Zhang, Y., and Zheng, X. F. (2014). Superoxide dismutase 1 acts as a nuclear transcription factor to regulate oxidative stress resistance. Nat. Commun. 5:3446. doi: 10.1038/ncomms4446
Tuzet, A., Rahantaniaina, M. S., and Noctor, G. (2019). Analyzing the function of catalase and the ascorbate-glutathione pathway in H2O2 processing: insights from an experimentally constrained kinetic model. Antioxid. Redox Signal. 30, 1238–1268. doi: 10.1089/ars.2018.7601
Umezawa, T., Sugiyama, N., Takahashi, F., Anderson, J. C., Ishihama, Y., Peck, S. C., et al. (2013). Genetics and phosphoproteomics reveal a protein phosphorylation network in the abscisic acid signaling pathway in Arabidopsis thaliana. Sci. Signal. 6:rs8. doi: 10.1126/scisignal.2003509
Ushimaru, T., Nakagawa, T., Fujioka, Y., Daicho, K., Naito, M., Yamauchi, Y., et al. (2006). Transgenic Arabidopsis plants expressing the rice dehydroascorbate reductase gene are resistant to salt stress. J. Plant Physiol. 163, 1179–1184. doi: 10.1016/j.jplph.2005.10.002
Vadassery, J., Tripathi, S., Prasad, R., Varma, A., and Oelmüller, R. (2009). Monodehydroascorbate reductase 2 and dehydroascorbate reductase 5 are crucial for a mutualistic interaction between Piriformospora indica and Arabidopsis. J. Plant Physiol. 166, 1263–1274. doi: 10.1016/j.jplph.2008.12.016
van Buer, J., Cvetkovic, J., and Baier, M. (2016). Cold regulation of plastid ascorbate peroxidases serves as a priming hub controlling ROS signaling in Arabidopsis thaliana. BMC Plant Biol. 16:163. doi: 10.1186/s12870-016-0856-7
Van Leene, J., Han, C., Gadeyne, A., Eeckhout, D., Matthijs, C., Cannoot, B., et al. (2019). Capturing the phosphorylation and protein interaction landscape of the plant TOR kinase. Nat. Plants 5, 316–327. doi: 10.1038/s41477-019-0378-z
Vandenabeele, S., Vanderauwera, S., Vuylsteke, M., Rombauts, S., Langebartels, C., Seidlitz, H. K., et al. (2004). Catalase deficiency drastically affects gene expression induced by high light in Arabidopsis thaliana. Plant J. 39, 45–58. doi: 10.1111/j.1365-313X.2004.02105.x
Vanderauwera, S., Suzuki, N., Miller, G., van de Cotte, B., Morsa, S., Ravanat, J. L., et al. (2011). Extranuclear protection of chromosomal DNA from oxidative stress. Proc. Natl. Acad. Sci. U.S.A. 108, 1711–1716. doi: 10.1073/pnas.101835910
Vogel, J. T., Zarka, D. G., Van Buskirk, H. A., Fowler, S. G., and Thomashow, M. F. (2005). Roles of the CBF2 and ZAT12 transcription factors in configuring the low temperature transcriptome of Arabidopsis. Plant J. 41, 195–211. doi: 10.1111/j.1365-313X.2004.02288.x
Vogel, M. O., Moore, M., König, K., Pecher, P., Alsharafa, K., Lee, J., et al. (2014). Fast retrograde signaling in response to high light involves metabolite export, MITOGEN-ACTIVATED PROTEIN KINASE6, and AP2/ERF transcription factors in Arabidopsis. Plant cell 26, 1151–1165. doi: 10.1105/tpc.113.121061
Voothuluru, P., and Sharp, R. E. (2013). Apoplastic hydrogen peroxide in the growth zone of the maize primary root under water stress. I. Increased levels are specific to the apical region of growth maintenance. J. Exp. Bot. 64, 1223–1233. doi: 10.1093/jxb/ers277
Vu, L. D., Gevaert, K., and De Smet, I. (2018). Protein language: post-translational modifications talking to each other. Trends Plant Sci. 23, 1068–1080. doi: 10.1016/j.tplants.2018.09.004
Wang, B., Ding, H., Chen, Q., Ouyang, L., Li, S., and Zhang, J. (2019). Enhanced tolerance to methyl viologen-mediated oxidative stress via AtGR2 expression from chloroplast genome. Front. Plant Sci. 10:1178. doi: 10.3389/fpls.2019.01178
Wang, P., Du, Y., Zhao, X., Miao, Y., and Song, C. P. (2013a). The MPK6-ERF6-ROS-responsive cis-acting Element7/GCC box complex modulates oxidative gene transcription and the oxidative response in Arabidopsis. Plant Physiol. 161, 1392–1408. doi: 10.1104/pp.112.210724
Wang, P., Xue, L., Batelli, G., Lee, S., Hou, Y. J., Van Oosten, M. J., et al. (2013b). Quantitative phosphoproteomics identifies SnRK2 protein kinase substrates and reveals the effectors of abscisic acid action. Proc. Natl. Acad. Sci. U.S.A. 110, 11205–11210. doi: 10.1073/pnas.1308974110
Wang, W., Zhang, H., Wei, X., Yang, L., Yang, B., Zhang, L., et al. (2018). Functional characterization of calcium-dependent protein kinase (CPK) 2 gene from oilseed rape (Brassica napus L.) in regulating reactive oxygen species signaling and cell death control. Gene 651, 49–56. doi: 10.1016/j.gene.2018.02.006
Wang, X., Bian, Y., Cheng, K., Gu, L. F., Ye, M., Zou, H., et al. (2013c). A large-scale protein phosphorylation analysis reveals novel phosphorylation motifs and phosphoregulatory networks in Arabidopsis. J. Proteom. 78, 486–498. doi: 10.1016/j.jprot.2012.10.018
Wang, X., Bian, Y., Cheng, K., Zou, H., Sun, S. S., and He, J. X. (2012). A comprehensive differential proteomic study of nitrate deprivation in Arabidopsis reveals complex regulatory networks of plant nitrogen responses. J. Proteome Res. 11, 2301–2315. doi: 10.1021/pr2010764
Wang, Z., Xiao, Y., Chen, W., Tang, K., and Zhang, L. (2010). Increased vitamin C content accompanied by an enhanced recycling pathway confers oxidative stress tolerance in Arabidopsis. J. Integr. Plant Biol. 52, 400–409. doi: 10.1111/j.1744-7909.2010.00921.x
Waszczak, C., Akter, S., Eeckhout, D., Persiau, G., Wahni, K., Bodra, N., et al. (2014). Sulfenome mining in Arabidopsis thaliana. Proc. Natl. Acad. Sci. U.S.A. 111, 11545–11550. doi: 10.1073/pnas.1411607111
Waszczak, C., Akter, S., Jacques, S., Huang, J., Messens, J., and Van Breusegem, F. (2015). Oxidative post-translational modifications of cysteine residues in plant signal transduction. J. Exp. Bot. 66, 2923–2934. doi: 10.1093/jxb/erv084
Waszczak, C., Carmody, M., and Kangasjärvi, J. (2018). Reactive oxygen species in plant signaling. Annu. Rev. Plant Biol. 69, 209–236. doi: 10.1146/annurev-arplant-042817-040322
Wen, F., Ye, F., Xiao, Z., Liao, L., Li, T., Jia, M., et al. (2020). Genome-wide survey and expression analysis of calcium-dependent protein kinase (CDPK) in grass Brachypodium distachyon. BMC Genomics 21:53. doi: 10.1186/s12864-020-6475-6
Withers, J., and Dong, X. (2017). Post-translational regulation of plant immunity. Curr. Opin. Plant Biol. 38, 124–132. doi: 10.1016/j.pbi.2017.05.004
Wu, F., Chi, Y., Jiang, Z., Xu, Y., Xie, L., Huang, F., et al. (2020). Hydrogen peroxide sensor HPCA1 is an LRR receptor kinase in Arabidopsis. Nature 578, 577–581. doi: 10.1038/s41586-020-2032-3
Wu, T. M., Lin, W. R., Kao, C. H., and Hong, C. Y. (2015). Gene knockout of glutathione reductase 3 results in increased sensitivity to salt stress in rice. Plant Mol. Biol. 87, 555–564. doi: 10.1007/s11103-015-0290-5
Xia, X.-J., Zhou, Y.-H., Shi, K., Zhou, J., Foyer, C. H., and Yu, J.-Q. (2015). Interplay between reactive oxygen species and hormones in the control of plant development and stress tolerance. J. Exp. Bot. 66, 2839–2856. doi: 10.1093/jxb/erv089
Xing, C., Liu, Y., Zhao, L., Zhang, S., and Huang, X. (2019). A novel MYB transcription factor regulates ascorbic acid synthesis and affects cold tolerance. Plant Cell Environ. 42, 832–845. doi: 10.1111/pce.13387
Xing, Y., Cao, Q., Zhang, Q., Qin, L., Jia, W., and Zhang, J. (2013). MKK5 regulates high light-induced gene expression of Cu/Zn superoxide dismutase 1 and 2 in Arabidopsis. Plant Cell Physiol. 54, 1217–1227. doi: 10.1093/pcp/pct072
Xing, Y., Chen, W. H., Jia, W., and Zhang, J. (2015). Mitogen-activated protein kinase kinase 5 (MKK5)-mediated signalling cascade regulates expression of iron superoxide dismutase gene in Arabidopsis under salinity stress. J. Exp. Bot. 66, 5971–5981. doi: 10.1093/jxb/erv305
Xing, Y., Jia, W., and Zhang, J. (2007). AtMEK1 mediates stress-induced gene expression of CAT1 catalase by triggering H2O2 production in Arabidopsis. J. Exp. Bot. 58, 2969–2981. doi: 10.1093/jxb/erm144
Xing, Y., Jia, W., and Zhang, J. (2008). AtMKK1 mediates ABA-induced CAT1 expression and H2O2 production via AtMPK6-coupled signaling in Arabidopsis. Plant J. 54, 440–451. doi: 10.1111/j.1365-313X.2008.03433.x
Xu, J., Tran, T., Padilla Marcia, C. S., Braun, D. M., and Goggin, F. L. (2017). Superoxide-responsive gene expression in Arabidopsis thaliana and Zea mays. Plant Physiol. Biochem. 117, 51–60. doi: 10.1016/j.plaphy.2017.05.018
Xu, P., Chen, H., and Cai, W. (2020). Transcription factor CDF4 promotes leaf senescence and floral organ abscission by regulating abscisic acid and reactive oxygen species pathways in Arabidopsis. EMBO Rep. 21:e48967. doi: 10.15252/embr.201948967
Xue, L., Wang, P., Wang, L., Renzi, E., Radivojac, P., Tang, H., et al. (2013). Quantitative measurement of phosphoproteome response to osmotic stress in Arabidopsis based on Library-Assisted eXtracted Ion Chromatogram (LAXIC). Mol. Cell. Proteomics 12, 2354–2369. doi: 10.1074/mcp.O113.027284
Xue, Y., Zhou, F., Zhu, M., Ahmed, K., Chen, G., and Yao, X. (2005). GPS: a comprehensive www server for phosphorylation sites prediction. Nucleic Acids Res. 33, W184–W187. doi: 10.1093/nar/gki393
Yalcinkaya, T., Uzilday, B., Ozgur, R., Turkan, I., and Mano, J. (2019). Lipid peroxidation-derived reactive carbonyl species (RCS): their interaction with ROS and cellular redox during environmental stresses. Environ. Exp. Bot. 165, 139–149. doi: 10.1016/j.envexpbot.2019.06.004
Yamasaki, H., Abdel-Ghany, S. E., Cohu, C. M., Kobayashi, Y., Shikanai, T., and Pilon, M. (2007). Regulation of copper homeostasis by micro-RNA in Arabidopsis. J. Biol. Chem. 282, 16369–16378. doi: 10.1074/jbc.M700138200
Yamasaki, H., Hayashi, M., Fukazawa, M., Kobayashi, Y., and Shikanai, T. (2009). SQUAMOSA promoter binding protein-like7 is a central regulator for copper homeostasis in Arabidopsis. Plant Cell 21, 347–361. doi: 10.1105/tpc.108.060137
Yan, J., Guan, L., Sun, Y., Zhu, Y., Liu, L., Lu, R., et al. (2015). Calcium and ZmCCaMK are involved in brassinosteroid-induced antioxidant defense in maize leaves. Plant Cell Physiol. 56, 883–896. doi: 10.1093/pcp/pcv014
Yang, H., Mu, J., Chen, L., Feng, J., Hu, J., Li, L., et al. (2015). S-Nitrosylation positively regulates ascorbate peroxidase activity during plant stress responses. Plant Physiol. 167, 1604–1615. doi: 10.1104/pp.114.255216
Yang, Z., Guo, G., Zhang, M., Liu, C. Y., Hu, Q., Lam, H., et al. (2013). Stable isotope metabolic labeling-based quantitative phosphoproteomic analysis of Arabidopsis mutants reveals ethylene-regulated time-dependent phosphoproteins and putative substrates of constitutive triple response 1 kinase. Mol. Cell. Proteom. 12, 3559–3582. doi: 10.1074/mcp.M113.031633
Yang, Z., Mhamdi, A., and Noctor, G. (2019). Analysis of catalase mutants underscores the essential role of CATALASE2 for plant growth and day length-dependent oxidative signalling. Plant Cell Environ. 42, 688–700. doi: 10.1111/pce.13453
Yin, L., Mano, J., Tanaka, K., Wang, S., Zhang, M., Deng, X., et al. (2017). High level of reduced glutathione contributes to detoxification of lipid peroxide-derived reactive carbonyl species in transgenic Arabidopsis overexpressing glutathione reductase under aluminum stress. Physiol. Plant. 161, 211–223. doi: 10.1111/ppl.12583
Yin, L., Wang, S., Eltayeb, A. E., Uddin, M. I., Yamamoto, Y., Tsuji, W., et al. (2010). Overexpression of dehydroascorbate reductase, but not monodehydroascorbate reductase, confers tolerance to aluminum stress in transgenic tobacco. Planta 231, 609–621. doi: 10.1007/s00425-009-1075-3
Yoshida, K., Noguchi, K., Motohashi, K., and Hisabori, T. (2013). Systematic exploration of thioredoxin target proteins in plant mitochondria. Plant Cell Physiol. 54, 875–892. doi: 10.1093/pcp/pct037
Yoshida, S., Tamaoki, M., Shikano, T., Nakajima, N., Ogawa, D., Ioki, M., et al. (2006). Cytosolic dehydroascorbate reductase is important for ozone tolerance in Arabidopsis thaliana. Plant Cell Physiol. 47, 304–308. doi: 10.1093/pcp/pci246
Yu, X., Pasternak, T., Eiblmeier, M., Ditengou, F., Kochersperger, P., Sun, J., et al. (2013). Plastid-localized glutathione reductase2-regulated glutathione redox status is essential for Arabidopsis root apical meristem maintenance. Plant Cell 25, 4451–4468. doi: 10.1105/tpc.113.11702
Zandalinas, S. I., Fichman, Y., Devireddy, A. R., Sengupta, S., Azad, R. K., and Mittler, R. (2020). Systemic signaling during abiotic stress combination in plants. Proc. Natl. Acad. Sci. U.S.A. 117, 13810–13820. doi: 10.1073/pnas.2005077117
Zandalinas, S. I., Sengupta, S., Burks, D., Azad, R. K., and Mittler, R. (2019). Identification and characterization of a core set of ROS wave-associated transcripts involved in the systemic acquired acclimation response of Arabidopsis to excess light. Plant J. 98, 126–141. doi: 10.1111/tpj.14205
Zechmann, B. (2018). Compartment-specific importance of ascorbate during environmental stress in plants. Antioxid. Redox Signal. 29, 1488–1501. doi: 10.1089/ars.2017.7232
Zhang, A., Zhang, J., Ye, N., Cao, J., Tan, M., Zhang, J., et al. (2010). ZmMPK5 is required for the NADPH oxidase-mediated self-propagation of apoplastic H2O2 in brassinosteroid-induced antioxidant defence in leaves of maize. J. Exp. Bot. 61, 4399–4411. doi: 10.1093/jxb/erq243
Zhang, H., Liu, Y., Wen, F., Yao, D., Wang, L., Guo, J., et al. (2014). A novel rice C2H2-type zinc finger protein, ZFP36, is a key player involved in abscisic acid-induced antioxidant defence and oxidative stress tolerance in rice. J. Exp. Bot. 65, 5795–5809. doi: 10.1093/jxb/eru313
Zhang, H., Zhang, Y., Deng, C., Deng, S., Li, N., Zhao, C., et al. (2018). The Arabidopsis Ca2+-dependent protein kinase CPK12 is involved in plant response to salt stress. Int. J. Mol. Sci. 19:4062. doi: 10.3390/ijms19124062
Zhang, H., Zhou, H., Berke, L., Heck, A. J., Mohammed, S., Scheres, B., et al. (2013). Quantitative phosphoproteomics after auxin-stimulated lateral root induction identifies an SNX1 protein phosphorylation site required for growth. Mol. Cell. Proteomics 12, 1158–1169. doi: 10.1074/mcp.M112.021220
Zhang, M., Li, Q., Liu, T., Liu, L., Shen, D., Zhu, Y., et al. (2015). Two cytoplasmic effectors of Phytophthora sojae regulate plant cell death via interactions with plant catalases. Plant Physiol. 167, 164–175. doi: 10.1104/pp.114.252437
Zhang, P., Wang, R., Ju, Q., Li, W., Tran, L. P., and Xu, J. (2019). The R2R3-MYB transcription factor MYB49 regulates cadmium accumulation. Plant Physiol. 180, 529–542. doi: 10.1104/pp.18.01380
Zhang, P., Wang, R., Yang, X., Ju, Q., Li, W., Lü, S., et al. (2020). The R2R3-MYB transcription factor AtMYB49 modulates salt tolerance in Arabidopsis by modulating the cuticle formation and antioxidant defence. Plant Cell Environ. 43, 1925–1943. doi: 10.1111/pce.13784
Zhang, S., Li, C., Ren, H., Zhao, T., Li, Q., Wang, S., et al. (2020). BAK1 mediates light intensity to phosphorylate and activate catalases to regulate plant growth and development. Int. J. Mol. Sci. 21:1437. doi: 10.3390/ijms21041437
Zhang, T., Zhu, M., Song, W. Y., Harmon, A. C., and Chen, S. (2015). Oxidation and phosphorylation of MAP kinase 4 cause protein aggregation. Biochim. Biophys. Acta 1854, 156–165. doi: 10.1016/j.bbapap.2014.11.006
Zhang, Y., Ji, T. T., Li, T. T., Tian, Y. Y., Wang, L. F., and Liu, W. C. (2020). Jasmonic acid promotes leaf senescence through MYC2-mediated repression of CATALASE2 expression in Arabidopsis. Plant Sci. 299:110604. doi: 10.1016/j.plantsci.2020.110604
Zhang, Z., Wu, Y., Gao, M., Zhang, J., Kong, Q., Liu, Y., et al. (2012). Disruption of PAMP-induced MAP kinase cascade by a Pseudomonas syringae effector activates plant immunity mediated by the NB-LRR protein SUMM2. Cell Host Microbe 11, 253–263. doi: 10.1016/j.chom.2012.01.015
Zhao, R., Sun, H., Zhao, N., Jing, X., Shen, X., and Chen, S. (2015). The Arabidopsis Ca2+-dependent protein kinase CPK27 is required for plant response to salt-stress. Gene 563, 203–214. doi: 10.1016/j.gene.2015.03.024
Zhou, Y. B., Liu, C., Tang, D. Y., Yan, L., Wang, D., Yang, Y. Z., et al. (2018). The receptor-like cytoplasmic kinase STRK1 phosphorylates and activates CatC, thereby regulating H2O2 homeostasis and improving salt tolerance in rice. Plant Cell 30, 1100–1118. doi: 10.1105/tpc.17.01000
Zimmermann, P., Heinlein, C., Orendi, G., and Zentgraf, U. (2006). Senescence-specific regulation of catalases in Arabidopsis thaliana (L.) Heynh. Plant Cell Environ. 29, 1049–1060. doi: 10.1111/j.1365-3040.2005.01459.x
Zou, J.-J., Li, X.-D., Ratnasekera, D., Wang, C., Liu, W.-X., Song, L.-F., et al. (2015). Arabidopsis CALCIUM-DEPENDENT PROTEIN KINASE8 and CATALASE3 function in abscisic acid-mediated signaling and H2O2 homeostasis in stomatal guard cells under drought stress. Plant Cell 27, 1445–1460. doi: 10.1105/tpc.15.00144
Zulawski, M., Braginets, R., and Schulze, W. X. (2013). PhosPhAt goes kinases-searchable protein kinase target information in the plant phosphorylation site database PhosPhAt. Nucleic Acids Res. 41, D1176–D1184. doi: 10.1093/nar/gks1081
Keywords: signaling, stress, oxidative stress, plants, mitogen-activated protein kinases, calcium, antioxidant enzymes, reactive oxygen species
Citation: Dvořák P, Krasylenko Y, Zeiner A, Šamaj J and Takáč T (2021) Signaling Toward Reactive Oxygen Species-Scavenging Enzymes in Plants. Front. Plant Sci. 11:618835. doi: 10.3389/fpls.2020.618835
Received: 18 October 2020; Accepted: 11 December 2020;
Published: 01 February 2021.
Edited by:
Luisa M. Sandalio, Departamento de Bioquímica, Biología Celular y Molecular de Plantas, Estación Experimental del Zaidín (EEZ), SpainReviewed by:
Rosa M. Rivero, Spanish National Research Council, SpainMirza Hasanuzzaman, Sher-e-Bangla Agricultural University, Bangladesh
Copyright © 2021 Dvořák, Krasylenko, Zeiner, Šamaj and Takáč. This is an open-access article distributed under the terms of the Creative Commons Attribution License (CC BY). The use, distribution or reproduction in other forums is permitted, provided the original author(s) and the copyright owner(s) are credited and that the original publication in this journal is cited, in accordance with accepted academic practice. No use, distribution or reproduction is permitted which does not comply with these terms.
*Correspondence: Tomáš Takáč, dG9tYXMudGFrYWNAdXBvbC5jeg==