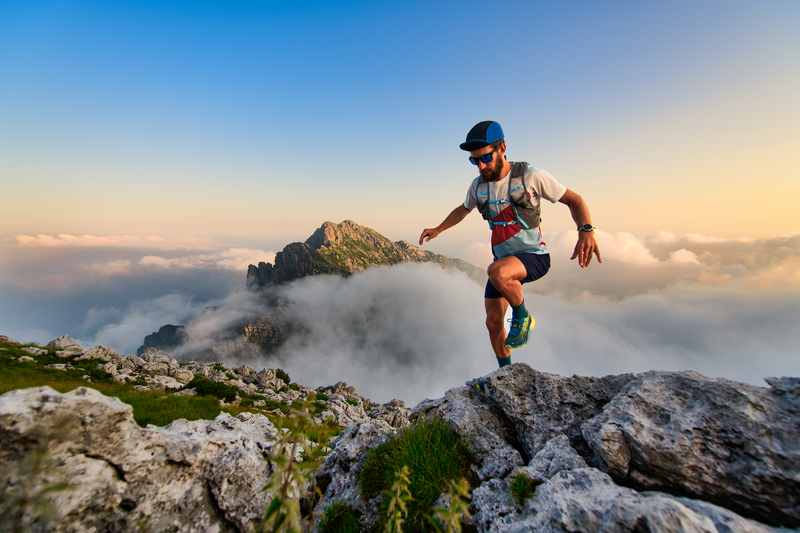
95% of researchers rate our articles as excellent or good
Learn more about the work of our research integrity team to safeguard the quality of each article we publish.
Find out more
REVIEW article
Front. Plant Sci. , 12 January 2021
Sec. Crop and Product Physiology
Volume 11 - 2020 | https://doi.org/10.3389/fpls.2020.613004
This article is part of the Research Topic Physiological and Molecular Aspects of Plant Rootstock-Scion Interactions View all 24 articles
Plant grafting is an ancient agricultural practice widely employed in crops such as woody fruit trees, grapes, and vegetables, in order to improve plant performance. Successful grafting requires the interaction of compatible scion and rootstock genotypes. This involves an intricate network of molecular mechanisms operating at the graft junction and associated with the development and the physiology of the scion, ultimately leading to improved agricultural characteristics such as fruit quality and increased tolerance/resistance to abiotic and biotic factors. Bidirectional transfer of molecular signals such as hormones, nutrients, proteins, and nucleic acids from the rootstock to the scion and vice versa have been well documented. In recent years, studies on rootstock-scion interactions have proposed the existence of an epigenetic component in grafting reactions. Epigenetic changes such as DNA methylation, histone modification, and the action of small RNA molecules are known to modulate chromatin architecture, leading to gene expression changes and impacting cellular function. Mobile small RNAs (siRNAs) migrating across the graft union from the rootstock to the scion and vice versa mediate modifications in the DNA methylation pattern of the recipient partner, leading to altered chromatin structure and transcriptional reprogramming. Moreover, graft-induced DNA methylation changes and gene expression shifts in the scion have been associated with variations in graft performance. If these changes are heritable they can lead to stably altered phenotypes and affect important agricultural traits, making grafting an alternative to breeding for the production of superior plants with improved traits. However, most reviews on the molecular mechanisms underlying this process comprise studies related to vegetable grafting. In this review we will provide a comprehensive presentation of the current knowledge on the epigenetic changes and transcriptional reprogramming associated with the rootstock–scion interaction focusing on woody plant species, including the recent findings arising from the employment of advanced—omics technologies as well as transgrafting methodologies and their potential exploitation for generating superior quality grafts in woody species. Furthermore, will discuss graft—induced heritable epigenetic changes leading to novel plant phenotypes and their implication to woody crop improvement for yield, quality, and stress resilience, within the context of climate change.
Grafting is an ancient agricultural propagation technique widely used to improve plant performance, in terms of yield, quality and resilience to abiotic and biotic stresses. It involves the merging of two genetically different plant parts, the rootstock, and the scion, in such a manner that the two parts join and grow as a single plant. Grafting is essentially dependent on the fundamental ability of wound healing in plants (Goldschmidt, 2014). Grafting is commonly used to improve production in woody fruit, nut crops (Warschefsky et al., 2016) as well as in non-woody vegetable crops (Abd Al-Razaq, 2019) and in order to influence scion performance in forest tree species (Jayawickrama et al., 1997) and in ornamental plants (Ambros et al., 2016). Important grafting applications include using rootstocks (a) to propagate plants that cannot be grown from seeds or cuttings (plants that do not set seed or have poor rooting ability), like certain cultivars of Olea europaea (Ayoub and Qrunfleh, 2006), (b) to control the juvenile period of the seedling (e.g., the prolonged delay in starting to bearing fruit observed in many Citrus genotypes (Warschefsky et al., 2016), (c) to replace the existing non-productive cultivar, or an old individual, with an improved one, capable of reliable and steady yield (Mudge et al., 2009). (d) To control canopy size and scion vigor, a practice widely used in apple cultivation (Fazio et al., 2015), (e) to repair rapidly a tree that has experienced significant damage either from natural causes or from management practices, and (f) to improve abiotic and biotic stress resistance characteristics (e.g., tristeza virus in citrus, Phytophthora on apple, Meloidogyne on peach, drought or phyloxera tolerance in grape).
Despite its widespread use, the molecular mechanisms underlying grafting are not fully understood although progress has been achieved in recent years in model and vegetable plants and recently in woody species as well. Transcriptional reprogramming and epigenetic changes seem to play crucial roles in the molecular mechanisms regulating rootstock-scion interactions and the development of the grafted plant. Epigenetics refers to stable and heritable changes in chromatin architecture that do not involve changes in the underlying DNA sequence but profoundly affect gene expression and impact cellular function. Epigenetic alterations are attained by three epigenetic mechanisms, namely, DNA methylation, post-translational histone modification and the action of non-coding RNA molecules which are either small RNAs (small interfering RNAs-siRNAs and micro RNAs-miRNAs) or long non-coding RNAs (long ncRNAs). Epigenetic regulation plays a major role in all aspects of plant development such as proper vegetative growth, successful reproduction, fruit development, yield, fruit quality, and tolerance to environmental stresses (Fujimoto et al., 2012; Kapazoglou et al., 2018). Moreover, understanding the vis ‘a-vis comparison of climate change impacts in relation to the impact of mitigation measures for agricultural and forestry and other land use is of significant importance in order to predict future food and energy sustainability (Van Meijl et al., 2018). For example, climatic changes are expected to exacerbate the negative impact of biotic stresses such as pathogens and pests. Losses in major crops and the role of crop protection has to be considered as a global strategy in order to safeguard future food needs (Oerke and Dehne, 2004). For instance, rising temperatures and rainfall changes are expected to increase water demand during the vegetative period, and in addition photodamage induced by solar radiation stress and high UV-B doses would be more detrimental for the Mediterranean crops (Doupis et al., 2011, 2013; Fares et al., 2017). Consequently, the effective abiotic stress assessment should focus on the development and evaluation of rootstocks that can influence scion growth and productivity under drought and light stress; particularly those rootstocks that can increase water conservation and those that can avoid photo bleaching by changes in leaf orientation and canopy structure. Considering the aforementioned biotic and abiotic constrains, emergence for better adaptation and selection as well as for reproduction of superior genotypes (e.g., through grafting or in vivo techniques) will have a significant impact in the future. Indeed, various studies nowadays focus on adaptation through grafting approaches for example cucumber salinity tolerance of cucumber (Elsheery et al., 2020), while a comprehensive recent review points out the significance of grafting for disease control, productivity and fruit quality (Belmonte-Urena et al., 2020). Creation through grafting of new superior genotypes will have a significant impact in order to achieve sustainable agricultural production and food security under the climatic changes which are evident nowadays. Understanding the molecular mechanisms underlying grafting with focus on the rootstock-scion interactions in woody species and the knowledge accumulated, thus far, with respect to the transcriptional and epigenetic effects associated with this process and the implications for woody crop improvement is an important task toward this direction.
Most of our knowledge on the molecular mechanisms of grafting has arisen from studies with model and vegetable species but in recent years studies on woody plants have been reported as well. Successful grafting requires a compatible and highly intricate interaction between the two parts of the graft, the scion, and the rootstock, which involves the concerted action of nutrient, hormonal, metabolic, transcriptional, and epigenetic pathways (Figures 1, 2). Establishment of a proper graft junction is a multistep process and hormones such as auxin, cytokinin, ethylene, gibberellin, and jasmonic acid play crucial roles in the scion-rootstock interaction (Melnyk and Meyerowitz, 2015; Melnyk et al., 2018; Nanda and Melnyk, 2018; Gautier et al., 2019; Sharma and Zheng, 2019). Homografts (same genotype used as scion and rootstock) present the highest grafting capacity but heterografts (different genotypes from the same species or different species) can also be successful depending on the phylogenetic distance between species and the particular genotype combinations. Accumulating evidence indicates short- or long-distance trafficking of signal molecules such as hormones, nutrients, proteins, and nucleic acids across the graft junction at cells adjacent to the graft interphase, or long range, at distant recipient tissue, with important implications to the success of the grafting and the development and performance of the scion (Albacete et al., 2015; Wang et al., 2017; Nanda and Melnyk, 2018; Gaut et al., 2019; Sharma and Zheng, 2019; Thomas and Frank, 2019).
Figure 1. Simple diagram presentation of graft union formation. Establishment of a proper graft junction is a complex process which involves the secretion of pectin from graft cells to serve as an adherent for the two parts, cell de-differentiation and formation of callus at the grafting site to form a bridge between graft partners, and cambium differentiation and production of secondary xylem and phloem that enables vascular reconnection between the scion and the rootstock. Hormones such as auxin and cytokinin regulate the grafting process. Auxin accumulation takes place at the graft site above the graft junction and genes related to auxin biosynthesis, auxin transport, and signaling are induced during the grafting process in order to promote vascular development at the graft union. Similarly, gene networks involved in cytokinin biosynthesis and signaling as well as hormonal cross talk are activated toward efficient vascular formation, tissue reconnection, and graft development.
Figure 2. Molecular effects of grafting in vegetables and woody species. Grafting in vegetable plants involves active and rapidly grown tissues provided soon after germination whereas in woody species grafting involves dormant tissues. This implies that signaling and rootstock-scion interaction may also exhibit differences between the two (Gautier et al., 2019) at least in the initial stages of graft formation and development Transfer of coding (mRNA) and non-coding (miRNAs, siRNAs) RNAs along the graft, induction of transcript accumulation at gene-specific and genome-wide level and DNA methylation changes in the scions have been observed for both grafted vegetables and woody species. However, it not yet known if the same mobile molecules, for instance the same small RNA molecules and accompanying proteins, are transferred through the phloem in vegetable grafts and woody species grafts and under which conditions. In addition, graft-induced DNA methylation changes have not been shown, thus far, in woody species rootstocks whereas graft-induced epigenetic changes such as histone modifications have not been reported for either vegetable or woody species, as yet, although histone modification related genes have been found to display altered expression profiles. In addition, it is not known how these epigenetic modifications may vary between vegetables and woody plants species and the cross talk with hormonal networks in each case needs to be elucidated. Furthermore, it should be noted that unlike vegetables that are propagated by sexual reproduction, woody plants are clonally propagated by asexual reproduction. This would favor stable trans-generational transmission of graft-induced epigenetic changes such as DNA methylation, in woody crops, which may lead to phenotypic variation and ultimately to novel varieties with improved traits. The aspects described above suggest that although a plethora of information has been obtained regarding the molecular basis of grafting, the exact mechanisms underlying signaling and communication between rootstock and scion and graft-induced effects in either vegetables or woody plants remain unclear and present exciting challenges for future research.
Short-distance movement of DNA molecules was shown in early studies by Stegemann and Bock (2009). By employing transgenic and transplastomic tobacco grafting systems these authors were able to show movement of both nuclear and plastid DNA in cells adjacent to the graft interface at either side of the graft (Stegemann and Bock, 2009). Utilizing a similar system, movement of entire plastid genomes was demonstrated in reciprocal grafts of Nicotiana tabacum, N. benthamiana, and N. glauca (tree tobacco) and most notably, the transferred plastid DNA was stably inherited in regenerated plants pointing to evolutionary and agronomical implications (Stegemann et al., 2012). Nuclear genome transfer between rootstock and scion was also demonstrated in tobacco grafts (Fuentes et al., 2014).
Bi-directional transport of RNA molecules over long distances via the phloem of grafted as well as non-grafted plants has been well established over the last two decades (Lucas et al., 2001; Lough and Lucas, 2006; Mermigka et al., 2006; Kalantidis et al., 2008; Melnyk et al., 2011b; Haroldsen et al., 2012; Melnyk et al., 2018; Morris, 2018; Tamiru et al., 2018; Thomas and Frank, 2019; Wang et al., 2020). Protein-coding RNA movement across grafting partners was first reported in a Cucurbitaceae heterograft for CmNACP, a Cucurbita maxima (pumpkin) transcript (Ruiz-Medrano et al., 1999). CmNACP encodes a NAC-domain transcription factor involved in meristem formation and crucial for controlling organ boundaries and organ development. CmNACP mRNA was detected in the cucumber part of a cucumber (scion)-pumpkin (rootstock) graft, indicating graft–induced transfer from the pumpkin rootstock via the phloem to the cucumber scion (Ruiz-Medrano et al., 1999). In a similar manner, Xoconostle-Cázares et al. (1999) demonstrated that a mRNA molecule encoding the phloem protein CmPP16 moved along with its protein across the graft union and into the phloem of the cucumber scion which was detected also in stems, leaves, and floral scion tissues (Xoconostle-Cázares et al., 1999).
The physiological relevance of mRNA transport was rather obscure at first and started being delineated in later studies showing that movement of certain mRNA molecules from source to sink or the other way around is linked to important biological functions. For example, translocation of a homeobox fusion transcript from rootstock to scion caused changes in scion leaf morphology in tomato grafts (Kim et al., 2001). Transport of BEL1 transcription factor transcripts (encoding regulators of tuber formation), from scion to roots enhanced tuber production in potato grafts (Banerjee et al., 2006) whereas a similar movement of BEL11 and BEL29 transcripts suppressed tuber growth in potato roots (Ghate et al., 2017). Similarly, movement of AUX/IAA transcripts (encoding regulators of auxin transport) to the root tip, modified tobacco root architecture in Arabidopsis/tobacco hetero-grafts (Notaguchi et al., 2012).
Massive long-distance transport of mRNA molecules in a series of homograft or heterograft systems also has been described. Utilizing Arabidopsis ecotype-specific SNPs, thousands of mRNAs were found to be transported between Arabidopsis graft partners and were differentially detected in response to nutritional conditions (Thieme et al., 2015). Over 3,000 transcripts moved from cucumber scion leaves to watermelon sink tissues in a tissue-specific manner as an early response to phosphate deficiency stress (Zhang et al., 2016), 138 transcripts moved from Arabidopsis rootstock to Nicotiana benthamiana scion (Notaguchi et al., 2015) and 183 transcripts migrated from N. benthamiana scions to tomato roots (Xia et al., 2018). It is thought that these migrating mRNAs attain a tRNA-like structure that confers stability, mobility, and translational capacity (Morris, 2018). Exciting new work has shown that cytosine methylation of mRNAs is an essential element for transport of mobile transcripts from shoot to root in Arabidopsis grafts and the translocation of a methylated TCPT transcript to root tissue had an effect on root growth (Yang et al., 2019). The physiological relevance of mobile transcriptomes along the graft partners has just started to be addressed (discussed in following sections) and it is expected to be further elucidated in future research (Morris, 2018).
Apart from protein-coded mRNA movement a plethora of studies during the last two decades has focused on the transport of non-coding small RNAs (short interfering RNAs-siRNAs and micro RNA-miRNAs) across graft segments. Small RNAs comprise a category of 21–24 nucleotides (nt) RNAs of different origins that are directed either to chromatin DNA loci in the nucleus, or target homologous mRNA molecules in the cytoplasm. Extensive studies have demonstrated the essential and instrumental role of small RNAs in all aspects of plant growth and development and in both abiotic and biotic stress responses (reviewed in Gohlke and Mosher, 2015; Lewsey et al., 2016; Li and Zhang, 2016; Banerjee et al., 2017; Kumar et al., 2017; Kumar and Sathishkumar, 2017; Li et al., 2017; Poltronieri et al., 2020; Wang et al., 2020).
Migration of 21-nt and mostly 24-nt small RNAs in homografted and heterografted plants had been demonstrated originally mostly in Arabidopsis, tobacco, Solanaceae, and Cucurbitaceae species (Molnar et al., 2010; Melnyk et al., 2011a; Haroldsen et al., 2012). Most importantly mobile small RNA molecules were found to exert a transcriptional or post-transcriptional gene silencing effect in graft partners either through RNA-directed DNA methylation (RdDNA) on targeted genomic loci (mainly for siRNAs) or via degradation of the corresponding mRNA target molecule (mainly for miRNAs) (Molnar et al., 2010; Melnyk et al., 2011a; Tamiru et al., 2018). Molnar et al. (2010) reported movement of both transgene-derived and endogenous small RNAs across the graft union in a shoot-to-root direction in Arabidopsis grafts and showed that the 24-nt siRNAs were able to direct DNA methylation at three sites in the genome of the recipient cells (Molnar et al., 2010). Furthermore, in a similar study, Lewsey et al. (2016) showed that the mobile siRNA signal migrating to the roots of an Arabidopsis graft, guided genome-wide DNA methylation events at thousands of loci of the recipient root genome predominately targeting transposable elements (TE) (Lewsey et al., 2016). These silencing effects have the potential to trigger gene expression changes impacting cellular functions ultimately leading to changes in the morphology and physiology of the grafted plant. Several reports have described long distance movement of miRNAs and their potential effects. In Arabidopsis miR399, miR827, miR2111 travel long-distance from shoot to root as a response to phosphate starvation (Huen et al., 2017). In Lotus japonicus, miR2111 translocates in a shoot-to-root direction to control rhizobial infection through post-transcriptional regulation of a key suppressor of symbiosis promoting increased nodulation (Tsikou et al., 2018).
In addition, graft-induced protein-coding RNAs and non-coding small RNAs have been detected in the scion or rootstock of vegetables (Ren et al., 2018; Miao et al., 2019; Wang et al., 2019a,b; Zhang et al., 2019a,b; Aslam et al., 2020; Garcia-Lozano et al., 2020; Spanò et al., 2020) as well as woody species grafts (Cookson et al., 2013; Corso et al., 2015; Kaja et al., 2015; Li et al., 2016; Chitarra et al., 2017; Pagliarani et al., 2017). Such graft-induced transcripts have a putative role in graft development, yield, fruit quality of scions and in response to abiotic or biotic factors (Ren et al., 2018; Zhang et al., 2019a,b; Garcia-Lozano et al., 2020; Spanò et al., 2020) (described in following sections).
In several other studies epigenetic effects have been reported in scions of homografts or hetero-grafts, although RNA movement has not been investigated in these systems, as yet. Utilizing methylation sensitive amplified polymorphism (MSAP) analysis in a Cucurbitaceae inter-species graft system, a significant DNA methylation increase was observed both in melon and cucumber scions heterografted onto pumpkin, as compared to the seed plants (Avramidou et al., 2014). Similarly, graft-induced alterations in global DNA methylation were evidenced in tomato, eggplant and pepper scions in a Solanaceae inter-species grafting system (Wu et al., 2013). Importantly, the DNA methylation changes could be inherited in the self-pollinating progeny, pointing to stable transfer of graft-induced epigenetic changes to the next generation (Wu et al., 2013). Furthermore, intra-species grafting in Cucurbitaceae induced significant DNA methylation changes in pumpkin scions which were associated with altered fruit morphology and metabolic profiles (Xanthopoulou et al., 2019). Recently such graft-induced epigenetic alterations were reported also in woody species. DNA methylation profiles in Hevea brasiliensis (rubber tree) heterografts were significantly altered depending on rootstock and rootstock-scion genotype combinations (Uthup et al., 2018) and DNA methylation polymorphisms were found between juvenile seedlings, grafts, and adult trees, in apple (Perrin et al., 2020). All the aforementioned studies indicate that grafting can trigger changes in epigenetic factors such as DNA methylation and small RNAs, potentially impacting important traits of agronomical relevance.
Progress has been achieved in recent years with respect to the effects of grafting on the improvement of woody plants and the mechanisms underlying this process at the physiological, genetic, and epigenetic level. Employment of advanced—omics technologies has contributed greatly on the genome-wide aspects of rootstock-scion interactions, genotype compatibility, and graft-induced transcriptional and epigenetic effects. Some examples describing these investigations in woody species of high economic value are presented below and are summarized in Tables 1, 2.
Table 1. Examples of graft-induced transcriptional and epigenetic changes and physiological effects in woody species.
Viticulture is a vital sector of agriculture in many countries around the world. Global production of grape and wine amounts to 73.3 million tons of grape berry and 279 million hl of wine, annually (OIV statistical report on world vitiviniculture-http://www.oiv.int).
Grapevine grafting has been used widely to improve yield and quality and cope with the threat of diseases. The use of rootstocks is a common practice in most viticultural areas since the second half of nineteenth century in order to control infestation by phylloxera (Daktulosphaira vitifoliae), a soil-borne aphid that destroyed around four million of own-rooted vineyard hectares. The common commercial rootstocks currently used around the world were developed from native American Vitis species that have co-evolved with phylloxera and, as a result, they display a higher level of resistance (Mudge et al., 2009; Corso and Bonghi, 2014). Grapevine yield and quality of berry and wine depends on a variety of parameters such as genotype, rootstock, abiotic, and biotic factors, soil, and agricultural practices. A major factor affecting grapevine characteristics in relation to the environment is grafting onto appropriate rootstocks (Koundouras et al., 2008; Corso and Bonghi, 2014; Corso et al., 2015, 2016; Warschefsky et al., 2016; Peccoux et al., 2018; Wang et al., 2019b). A number of investigations have focused on the potential effects of scion/rootstock RNA movement along grapevine grafts as well as graft-induced transcriptional reprogramming with respect to rootstock specificity and plant performance. Using diagnostic SNPs from high throughput genome analyses to distinguish between scion and rootstock sequences more than 3,000 transcripts were found transported across graft junctions in field-or greenhouse-grown grafted grapevines (Yang et al., 2015). A large number of these sequences was associated with diverse biological functions and was highly enriched for genes involved in signal transduction cascades, metabolic activities, and stress responses implying they most likely influence whole plant development and physiology.
Graft-induced transcriptional reprogramming of mRNAs and miRNAs linked to particular scion/rootstock combinations have been described in several reports although their mobility aspect has not been characterized thus far. Graft-induced differential gene expression was reported in the shoot apex tissue of cv. Cabernet Sauvignon scions grafted onto either Riparia Gloire de Montpellier (RG) or Paulsen 1,103 rootstocks (Cookson and Ollat, 2013). Interestingly, these rootstocks conferred vigor effects and biomass accumulation to the scion which may be associated with the profound alterations in gene expression observed in scion shoots. The rootstocks induced expression of a multitude of genes which were enriched for chromatin modification genes (DNA methyltransferases and histone modifying genes), cell organization, hormonal signaling, and defense responses (Cookson and Ollat, 2013). Likewise, differential transcriptional responses related to secondary and jasmonate metabolism were reported for field-grown cv. Pinot Noir grafted onto a drought-sensitive rootstock (125AA) and a drought-tolerant rootstock (110R) upon drought stress. Berries of drought exposed Pinot Noir grafted on the drought-susceptible rootstock displayed increased induction of genes that are associated with the biosynthesis of primary and secondary metabolites affecting berry composition and wine quality (Berdeja et al., 2015). In addition, analysis of the effect of rootstock on Cabernet Sauvignon berries demonstrated that modulation of auxin-related genes and the rate of ripening varies according to the rootstock used in the graft (Corso et al., 2016).
Pagliarani et al. (2017) reported differential and drought stress-specific accumulation of miRNAs in auto-grafts and reciprocal heterografts of Cabernet Sauvignon and M4, a drought tolerant hybrid. Differential expression of novel and conserved miRNAs evidenced in all grafts depended on genotype combinations, tissue type, and drought stress conditions and graft directionality (Pagliarani et al., 2017).
An integrative physiological, metabolomics, and transcriptomic analysis using hetero-grafts of the southern Italian grapevine variety “Gaglioppo” highlighted the multiple effects of different rootstocks on the physiology, gene expression and metabolic pathways of the grafted plants (Chitarra et al., 2017). Significant differences in gene expression were evidenced in the leaves of “Gaglioppo” scions depending on rootstock genotype. Differentially Expressed Genes (DEGs) associated with photosynthetic processes, secondary metabolism, hormonal, stress, and signal transduction processes were upregulated in the scions of a Gaglioppo scions grafted on 41B rootstocks. Moreover, although less productive and less vigorous, Gaglioppo/41B exhibited increased resistance to pathogen infection by Plasmopara viticola as compared to the susceptible combination Gaglioppo/1103P. Interestingly, the 41B rootstock induced biotic response-related genes such as NBS and NBS-LRR type transcription factors and triggered a remarkable increase of stilbene synthase transcripts associated with ROS scavenging activity and oxidative stress prevention (Chitarra et al., 2017). Thus, specific rootstocks may induce biotic stress responses and confer resistance to disease through genome-wide modulation of the scion transcriptome.
Although most investigations have focused on the effect of rootstock on scion transcriptomes, studies on the effect of scion inducing gene expression changes in the rootstock remain limited. A very recent study by Gautier et al. (2020) investigated the control exerted by scions of grafted grapevine on rootstock transcriptomes and in particular under conditions of low phosphate. By employing an RNA-seq approach these authors examined rootstock transcriptome responses in different scion-rootstock genotype combinations of grapevine grafts in relation to low phosphate treatment and demonstrated differential transcriptome responses upon low phosphate imposition that depended on the particular rootstock-scion combinations (Gautier et al., 2020).
Notably, aside from transcripts, protein abundance differences depending on rootstock have also been reported. Increased salinity in grapevine cv. Thompson Seedless grafted onto a salt-tolerant rootstock (110R) is associated with differential protein accumulation involving photosynthesis, chlorophyll biosynthesis, amino acid metabolism, and precursor metabolite biosynthesis, as compared to the own-rooted plants (Patil et al., 2020).
Finally, molecular factors determining the success of graft healing and union formation in relation to graft compatibility/incompatibility have been investigated in grapevine. During the process of union formation in Cabernet-Sauvignon heterografts, CS/RG, and CS/1103 Paulsen, transcriptional changes were detected at the graft interphases of both combinations and these were associated to cell wall modification, wound responses, hormonal signaling, and stress responses, all playing a role in the complex mechanism of graft healing and development (Cookson et al., 2014). A very recent study utilized a transcriptomic approach with compatible and incompatible grapevine grafts of Touriga Nacional scions onto Richter 110 rootstocks to identify master regulators governing graft union formation. Grafts were analyzed in a nursery context at 21 and 80 days post-grafting (Assunção et al., 2019). Genes associated with hormone and metabolic signaling were markedly induced in the tissues of the graft zone in the compatible combination at the early stage whereas genes engaging in oxidative stress and wound healing showed higher induction in the less compatible combination at the later stage implying an inability of the later to cope with excess stress. In addition, reduced expression of specific miRNAs and concomitant increased expression of their target genes encoding transcription factors, which regulate cambium maintenance and vascular tissue differentiation was evident in the compatible grafts at the later stage (Assunção et al., 2019). Thus, the regulation of specific miRNA-target gene modules appears to be of high importance for proper graft union formation and could be of potential use for molecular prediction of graft success.
Grafting in fruit trees serves various purposes such as yield increase, control of growth, early transition to reproductive stage, improved economic quality, and tolerance to biotic and abiotic stresses. Grafting is widely used within the Rosaceae family members such as apple, pear, peach, and cherry which greatly contribute to a balanced and healthy diet (Farinati et al., 2017).
The underlying molecular mechanisms by which the rootstock controls scion growth and properties in Rosaceae remain largely unknown. Research has focused mainly on anatomical features, hormonal interactions, and nutrient transport across graft union (Sorce et al., 2002; Zamorskyi, 2011; Valverdi et al., 2019). Some preliminary studies aimed to identify miRNAs from vascular tissue and phloem sap that are possibly involved in long-distance signaling and modulation of expression and movement of mRNA targets (Varkonyi-Gasic et al., 2010). In grafted apple, the first reports on long distance RNA movement came in 2010 mainly focusing on their implications to hormone signaling (Kanehira et al., 2010). These authors revealed the transport of MpSLR/IAA14 transcripts from the wild rootstock (Malus var. ringo Asami Mo84-A) to the scion of apple cv. Fuji by in situ hybridization in the phloem. The same year the transport of gibberellic acid insensitive mRNA transcripts (GAI) was detected in both directions (upward and downward) from the graft union (Xu et al., 2010). In a similar study, the transport of Gibberellic acid insensitive transcripts was demonstrated across the graft union from a wild Pyrus betulaefolia cv. Bunge (rootstock) to a traditional local Chinese pear cultivar, Pyrus bretschneideri cv. Yali (scion) in a distance up to 50 cm (2 years old grafting tree) (Zhang et al., 2012). Moreover, the transmission capacity of Pyrus GAI transcript was successfully tested by grafting a 35S:pear -GAI transgenic tobacco (Nicotiana tabacum L. cv. Samson.) to wild-type tobacco and detecting transmission of GAI mRNA through the graft union occurred soon as 15 days after grafting. Findings in arabidopsis demonstrate that trafficking of GAI RNA transcripts is mediated by specific RNA motifs (Huang and Yu, 2009); it remains to be verified whether these motifs are responsible for long distance signaling in fruit trees as well.
Few works have pointed out the involvement of long-distance signal molecules to biotic stress tolerance. Investigation of resistance mechanisms of three apple rootstocks to wooly apple aphid, showed that the resistance factors exist in the phloem tissue, implying the modulation of resistance via a long-distance signal (Sandanayaka et al., 2003). Kaja et al. (2015) studied the expression levels of miRNAs on four different apple rootstocks (two resistant and two susceptible in fire blight) by deep sequencing of 12 small RNA libraries. Their results together with stem loop qPCR analysis revealed four apple miRNAs that could be potentially involved in fire blight resistance (mdm-miR169a, mdm-miR160e, mdm-miR167b-g, and mdm-miR168a,b) (Kaja et al., 2015). Thus, they assumed that the miRNA expression profiles in scion could influence rootstock characteristics, such as resistance to this bacterial pathogen.
Recent experiments have aimed to unravel the role of transmissible signals and epigenetic mechanisms on flower induction. In a recent study, it was demonstrated that a PbWoxT1 from pear (Pyrus betulaefolia) interacts with a polypyrimidine tract binding protein PbPTB3 assisting in long distance transport in the phloem and possibly controlling flower development and growth (Duan et al., 2016). Fan et al. (2018) investigated the role of 12 histone modification (HMs) genes in flower induction in two apple varieties with contrasting flowering characteristics. Their expression patterns indicated that their up- or down-regulation contributed to different aspects of flowering. Although the two varieties were both grafted to the same rootstock, the relationship of grafting to the differential expression of the HMs was not investigated. An et al. (2018) studied the miRNA expression in self-rooted and grafted Fuji apple trees by high-throughput sequencing in relation to flowering rate. Some of them that were differentially regulated in response to grafting (miR156, miR172, miR159, miR171) target genes encoding transcription factors that regulate flower formation, and suggested that there is a regulatory network between miRNAs and mRNAs that is generated by grafting. Mir156 and mir172 are suggested to act as mobile signals regulating flower formation in grafted arabidopsis plants as well (Zhu and Helliwell, 2011; Wang, 2014).
Inversely, the role of scions onto rootstocks has been examined in a study by Li et al. (2016). Combined morphological, metabolic, hormonal, and root transcriptome analysis of M. robusta rootstock grafted with scions of wild-type (WT) apple (M. spectabilis) and a more-branching (MB) mutant at the branching stage revealed that sugar metabolism and auxin and cytokinin signaling exert major effects on root growth and development of grafted apple (Li et al., 2016).
Future work should enable to extend our limited so far knowledge about the implication of mobile signals in controlling agronomically important traits in grafted trees as well as to find commonalities and differences regarding their role in herbaceaus and woody grafted plants (Thomas and Frank, 2019).
Studies with citrus grafts have shown differential DNA methylation in different scion/rootstock combinations. Valencia orange (VO) scion variety grafted on two rootstocks with different soil water extraction capacities, Rangpur Lime (RL) and Sunki Maravilha (SM), displayed differential graft-induced DNA methylation which were associated with enhanced acclimatization responses upon recurrent drought stress for the VO/SM combination (Neves et al., 2017). Similarly, “Tahiti acid lime” (TAL) scions grafted on RL and SM exhibited hypermethylation and hypomethylation, respectively, upon water deficit treatments, however, physiological responses were similar (Santos et al., 2020).
Ahsan et al. (2019) provided the first evidence of avocado inter-graft regulation of miR156 and miR172 that control transition from juvenile to flowering phase by mediating the expression of the SQUAMOSA promoter binding protein-like (SPL) gene using different combinations (scions from shoot of young seedlings or mature scion wood grafted onto both seedlings or clonal rootstocks). Results demonstrated that scion age significantly influenced grafted tree maturity through the control of miR156-SPL4-miR172 regulatory network. They also showed that the existence of leaves on cutting rootstocks provided grafting success and influence the abundance of miR156 and miR172 in the scion (Ahsan et al., 2019).
An interesting study conducted by Khaldun et al. (2016) examined the miRNA regulation and expression patterns of tomato plants grafted onto Goji Berry (Lycium chinense Mill.) to reveal their implication to diverse biological pathways within a distant-grafting system by using Illumina sequencing technology. Significant differences in miRNA expression of shoots and fruits were revealed comparing non-grafted to grafted tomato plants. The setting of fruits was found to be the stage with the highest abundance of miRNA transcripts (123 were up-regulated). Potential targets of differentially expressed miRNAs identified by in silico analysis were found to be mainly transcription factors involved in diverse metabolic and regulatory pathways. Global transcriptome changes have been detected also between compatible and incompatible graft combinations in Litchi chinensis (Chen et al., 2017) and during graft union development in pecan (Carya illinoinensis) (Mo et al., 2018).
Grafting has been used empirically also in clonal forestry section as a tool which primary targets transfer of juvenility properties to mature materials by establishing them onto young rootstocks in nurseries (Huang et al., 1992). Phenotypic traits (such as wood density, tree height, biomass production) in superior forest trees are linked to economic benefits but limitations such as time needed for maturity to be reached and ineffective vegetative propagation limits the production of this material. Furthermore, recent findings showed that epigenetic modifications which can act through grafting procedures can be responsible for phase change from juvenility to maturity (von Aderkas and Bonga, 2000).
In a recent work with Spanish red cedar (Cedrela odorata L.) authors showed the significant effect of elite genotype grafting in juvenility induction in mature material (Robert et al., 2020). Interestingly, this phase change requires a particular balance and distribution of plant growth regulators among specific cell layers in meristematic tissues, where a “nurse tissue” condition is observed upon grafting of juvenile cells on scion tissues (Wendling et al., 2014). Those effects are hard to detect due to the fact that probably they involve the participation of epigenetic shifts mediated by siRNAs (Molnar et al., 2010) and require further research.
In another study conducted for Hevea brasilensis methylation differences were detected between buds from a single own rooted polyembryony-derived seedling grafted to genetically divergent rootstock (Uthup et al., 2018). MSAP and bisulfite sequencing techniques revealed significant alterations in DNA methylation profiles between heterografts and the levels of those epigenetic rearrangements was found to depend on the degree of incompatibility between the rootstock and the scion.
In grafted trees, the long-distance transport of signaling molecules through phloem and xylem, has proven as a major communication mechanism that fine-tunes tree architecture, developmental transitions, abiotic and biotic stress tolerance and cold-hardiness (Thomas and Frank, 2019; Figure 3). T he term trans-grafting is used when only one part (more often rootstock rather than scion) is transgenic with the other part untransformed (Haroldsen et al., 2012; Limera et al., 2017). The interaction of transgenic and non-transgenic parts in trans-grafted plants through the translocation of mRNA and RNAi (small RNA interference) molecules via the vascular system is the core of trans-grafting technique (Haroldsen et al., 2012). Consequently, and particularly in fruit crops, trans-grafting provides the potential improvement of woody tree species without the need of long-lasting biosafety controls normally required for traditional genetically modified plants (Song et al., 2019a).
Figure 3. Examples of plant traits that were altered by transgrafting on woody plants (transgenic rootstock/non-transgenic scion) by known (left) and unknown (right) transmissible signals.
To date, several studies focused on movement of RNAi- based silencing molecules from transgenic rootstock to non-transgenic scions to enhance pathogen resistance in perennial woody plants (Lemgo et al., 2013). The first study investigating the impact of long-distance-moving signals on conferring disease resistance was conducted by Dutt et al. (2007), where they identified that Shiva-1, a small lytic peptide, is transmitted from transgenic rootstocks to scions in grapevine and subsequently control the spreading of the xylem limited bacteria Xylella fastidiosa, which also protects from Pierce’s disease (PD). Transgenic cassava rootstocks expressing sRNAs in order to trigger RNA silencing in non-transgenic scions have successfully prevented the spreading of pomovirus Cassava brown streak disease (CBSD) (Yadav et al., 2011). Moreover, transgenic cherry rootstocks containing a hairpin RNAi vector were capable of combating Prunus necrotic ringspot virus (PNRSV) (Song et al., 2013). Later on, it was demonstrated that long-distance transportation from transgenic rootstock to non-transgenic sweet cherry scions of small interfering RNAs (siRNAs) derived from short hairpin RNAs of PNRSV induced systemic silencing and improved protection against PNRSV (Zhao and Song, 2014).
However not all of RNAi-based rootstocks can efficiently transfer the silencing molecules to non-transformed scions and confer resistance. In another study, hairpin siRNA derived from transgenic rootstocks of lime plants did not confer resistance to tristeza tree virus (CTV) in the non-transgenic scions, mainly due to the fact that the biggest fraction of siRNA was very quickly degraded (Lopez et al., 2010).
Grafting of transgenic rootstocks produced mRNA and siRNA signals and was also tested for speeding-up transition to flowering stage and for controlling tree size, characteristics that are useful for agriculture (McGarry and Kragler, 2013; Song et al., 2015). Interestingly, none of these studies confirmed the transmission of a long-distance mRNA or siRNA signal. In the work described by Flachowsky et al. (2012) attempted to downregulate the Terminal Flower1 (MdTFL1) gene, in order to induce early transition from juvenile to flowering stage in apple trees. However, when dsRNAiMdTFL1 transgenic rootstocks were grafted onto non-transgenic apple genotype “PinS,” the flower-inducing signal obtained after silencing of MdTFL1 gene was not transmittable (Flachowsky et al., 2012). A study by Smolka et al. (2010) aimed to reduce vegetative growth of apple trees by using transgenic rootstocks carrying the root-inducing rolB gene of Agrobacterium rhizogenes. Although these transgenic apple rootstocks significantly altered the characteristics of the scion when cultivated in nursery, no transmission of big molecules such as rolB gene or its mRNA was detectable in the scion cultivars. Moreover, overexpression of a peach CBF (PpCBF1) gene in a transgenic apple rootstock, responsible for enhanced freezing tolerance and growth inhibition, provoked reduced scion growth and delayed flowering with no evidence of phloem-transmissible PpCBF1 mRNA (Artlip et al., 2016). A transgenic apple rootstock variety, Malus prunifolia, integrating the AtGAI gene reduced moderately the growth rate of the Malus cultivar “Orin” (Xu et al., 2013).
A very interesting study by Song et al. (2019b) showed that transgenic blueberry rootstocks overexpressing flowering locus T gene (VcFT) induced early flowering in non-transgenic scions and the mobile signal responsible for this was a transmissible phytohormonal signal. Finally, in some woody tree species such as nut crops transgrafting has been applied very recently but with encouraging results, confirming its emerging role in tree breeding (Liu et al., 2017).
It is anticipated that in coming years plants will face major environmental challenges such as rapid rise in temperature, increased drought conditions and extreme weather phenomena. The impact of biotic stresses will be exacerbated owing to regional climate changes favoring a variety of pathogens. These factors are expected to have considerable impacts in crop productivity and food security. Therefore, the development of new agricultural practices or the capitalization of existing technologies is an imperative for confronting the above threats and imparting crops with resilience to unfavorable climatic conditions.
Grafting is an ancient agricultural practice originally used to counteract soil borne diseases, and ameliorate the performance of woody fruit species, enhancing yield, fruit quality, and tolerance to external stressors. Despite its widespread use worldwide the molecular mechanisms governing grafting and scion-rootstock communication are still not well understood. Progress has been achieved by research in model and vegetable plants that has contributed to a better insight into graft regulation at the molecular level. Lately this research has been extended to woody species. It is more and more evident that graft success and proper development of the grafted plant resides on scion-rootstock communication that involves epigenetic interactions and transcriptional modulation. mRNAs, miRNAs, and proteins can act as potential short- and long-distance signals exerting a regulatory role in the receiving graft partner which impacts scion but also whole plant performance. In grapevine, apple and avocado, miRNA and mRNA molecules as well as induction of transcriptional reprogramming in scions has been associated with physiological and metabolic pathways affecting traits of agronomical relevance (Cookson et al., 2014; Kaja et al., 2015; Corso et al., 2016; Li et al., 2016; Chitarra et al., 2017; Pagliarani et al., 2017; An et al., 2018). Gaining a deeper insight into the molecular mechanisms underlying these processes could direct the development of suitable molecular markers to be used for generating and selecting superior grafts with improved yield, quality, and tolerance to abiotic and biotic stresses.
Furthermore, graft compatibility is a prerequisite for graft success. Studies have started to explore the molecular regulators determining rootstock-scion compatibility (Cookson et al., 2014; Mo et al., 2018; Assunção et al., 2019; Notaguchi et al., 2020).
This information can be utilized to develop molecular markers for detecting successful grafts at early stages of graft development. Such diagnostic biomarker tools would be of great value to nurseries for early prediction of successful grafting and rapid and efficient selection of superior graft combinations, something particularly important for woody species where incompatibility may not be apparent before a period of several years.
Moreover, trans-grafting technology has enabled the grafting of wild type scions onto genetically engineered rootstocks carrying desirable traits, circumventing genetic modification of the scion. Thus, the transgene is contained in the rootstock and provides its beneficial effects such as resistance to viruses and other detrimental disease as well as tolerance to abiotic stressors, without genetic modification of the product or edible fruit (Zhao and Song, 2014; Artlip et al., 2016; Song et al., 2019b). This promising technology holds great potential as a targeted approach in woody plant improvement.
Finally, epigenetic factors may play an important role in improvement of woody plants through grafting. Epigenetic changes such as DNA methylation, histone modifications and the action of small RNAs can result in gene expression changes without affecting the underlying DNA sequence, with major impacts in cellular function and subsequent plant development and performance. In certain occasions these changes may be transmitted to the next generation. Stably inherited natural, environmental or graft-induced epigenetic changes have the potential to generate epi-alleles and lead to phenotypic variation (Gallusci et al., 2017; Kapazoglou et al., 2018; Varotto et al., 2020). In the context of changing environments, transgenerational effects, transgenerational memory, or transgenerational inheritance is the phenomenon that is defined as the “memory” at molecular level of the environmental conditions that an organism experienced which leads to modification of progeny phenotype (Tricker, 2015; Bilichak and Kovalchuk, 2016). This means that plants remember the stress they have experienced during their lifetime and transfer this memory to their offspring. As a result, offspring presents with increased resilience to stress exposure as compared to their parents. To date, the molecular base of this phenomenon is not fully understood. It seems that during gametogenesis some epigenetic marks escape the process of epigenetic reprogramming and resetting and subsequently are passed on to the offspring (Bilichak and Kovalchuk, 2016). The research about the transgenerational effects in plants has been focused mostly on annual plant species (Suter and Widmer, 2013; Zheng et al., 2013; Herman and Sultan, 2016) whereas studies in herbaceous perennial species reported that they might adopt a sexual reproductive strategy (Latzel et al., 2014) or an asexual reproductive strategy (Ren et al., 2017; Rendina González et al., 2018). Inversely, very limited information is available about the woody long-lived perennial species and especially those whose commercial varieties are maintained by vegetative (clonal) propagation. Long-lived perennial species potentially can accumulate epigenetic modifications during their lifetime that may be transferred to their offspring. Unlike sexually reproduced species where epigenetic modifications could be lost during meiosis as a result of erasure and resetting of epigenetic marks the possibility of stable transmission of epigenetic changes may be increased in asexually propagated plants as only mitotic cell divisions are involved (Verhoeven and Preite, 2014; He and Li, 2018).
In this regard, a recent report described putative transgenerational effects at the DNA methylation level as a result of temperature and photoperiod experienced by mother plants on the bud phenology of asexually produced offspring of Populus deltoides and Populus trichocarpa (Dewan et al., 2018). These authors underlined the importance of this effect on the potential response of plants to climatic changes. In another study, Perrin et al. (2020) compared the DNA methylation pattern in apples (Malus domestica) between adult trees and seedlings from selfing as well as between mature donor trees and newly grafted trees (Perrin et al., 2020). According to their results, in the former comparison the DNA methylation pattern did not differ at whole genome level but clearly varied in regions with genes related to photosynthesis. On the other hand, in the latter comparison, the majority of DNA methylation patterns was transferred from donor trees to newly grafted trees. They concluded that from a physiological, transcriptomic, and epigenomic point of view, newly grafted plants are in a phase between an adult tree and a seedling (Perrin et al., 2020). The above findings together with the fact that epigenetic variation contributes significantly to phenotypic plasticity, especially in species with limited genetic variation, points to the benefits of exploiting the potential of epigenetic variability. Further understanding of the molecular mechanisms regulating epigenetic changes such as DNA methylation in woody plants will contribute to the development of diagnostic molecular epi-markers for selection of superior genotypes and grafts with advantageous traits of agronomic relevance.
The ongoing climate change and rapid expansion of global population growth call for concerted efforts by diverse fields in order to mitigate the effects of adverse climatic conditions, promote crop sustainability, ensure food security and enable preservation of biodiversity.
In this context, woody species grafting is a promising agriculture technology for generating improved woody plants that can face environmental challenges without major compromise in yield and quality and with low input requirements. Despite its widespread use and the wealth of knowledge concerning woody species grafting at the morpho-physiological level, little is known about the molecular mechanisms governing this process. Nevertheless, research has now begun to address the molecular aspects associated with woody species scion-rootstock compatibility and successful graft development.
For some species like grapevine and apple, progress has been accomplished in terms of the graft-triggered transport of small RNAs or mRNAs long-distance between the graft partners and the characteristics they impart on the scion or whole grafted plant. In addition, RNA seq technologies have revealed graft-induced transcriptome reprogramming in scions with potential impact on phenotype. Epigenetic changes such as altered DNA methylation or histone modifications in woody species, is an area largely unexplored but in some cases like apple, citrus, avocado, and rubber tree investigations have started on DNA methylation alterations associated to grafting. There are no reports thus far on graft-induced histone modifications which is another crucial epigenetic mark that regulates cell function and plant development. Investigations on DNA methylation and histone modifications should be the focus of future studies in order to delineate further the molecular mechanism of grafting.
Moreover, trans-grafting technology has allowed targeted manipulation of rootstock genes affecting important scion traits, but without transferring the modified gene to the scion. This technology is gaining ground and holds a lot of potential toward generating grafts of agronomically important woody crops with improved qualities.
Challenges ahead include deeper understanding of the genetic and epigenetic molecular mechanisms underlying graft compatibility and proper growth and resilience of the grafted woody plants. This will contribute significantly to our knowledge on the molecular factors regulating these processes. Consequently, it will direct the development of molecular markers and epi-markers for rapid and efficient detection of graft success and compatible graft combinations at early stages as well as selection of superior grafts with improved adaptability to environmental challenges.
Finally, adaptive transgenerational plasticity and the molecular mechanisms governing this phenomenon is an under-explored area with great potential for agricultural application in woody plants. Graft induced-epigenetic variants (epi-allelles) are more likely to be stably inherited across generations in woody perennials than vegetables due to their way of clonal propagation (asexual reproduction) which favors the stable transmission of new characters. It is anticipated that in coming years research will expand greatly in uncovering mechanisms associated with woody plant grafting and transgenerational heritable changes. This knowledge would be of utmost importance for generating and selecting superior grafts for woody crop improvement and for transmitting graft-induced beneficial traits to subsequent generations, in order to meet sustainability demands in a changing environment.
AK coordinated author contributions, contributed with specific parts, and edited the manuscript. ET, EVA, EMA, MG, SM, and GD contributed with specific parts each. AD had the idea and edited the manuscript. All authors contributed to the article and approved the submitted version.
This work was part of the 221419.243 research project (acronym “DADA”) and co-financed by the Prefecture of Crete.
The authors declare that the research was conducted in the absence of any commercial or financial relationships that could be construed as a potential conflict of interest.
Abd Al-Razaq, A. H. (2019). Grafting techniques in vegetables crops: a review. Plant Arch. 19, 49–51.
Ahsan, M. U., Hayward, A., Alam, M., Bandaralage, J. H., Topp, B., Beveridge, C. A., et al. (2019). Scion control of miRNA abundance and tree maturity in grafted avocado. BMC Plant Biol. 19:382. doi: 10.1186/s12870-019-1994-1995
Albacete, A., Martínez-Andújar, C., Martínez-Pérez, A., Thompson, A. J., Dodd, I. C., and Pérez-Alfocea, F. (2015). Unravelling rootstock×scion interactions to improve food security. J. Exp. Bot. 66, 2211–2226.
Ambros, E. V., Vasilyeva, O. Y., and Novikova, T. (2016). Effects of in vitro propagation on ontogeny of rosa canina L. micropropagated plants as a promising rootstock for ornamental roses. Plant Cell Biotechnol. Mol. Biol. 17, 72–78.
An, N., Fan, S., Yang, Y., Chen, X., Dong, F., Wang, Y., et al. (2018). Identification and characterization of miRNAs in self-rooted and grafted malus reveals critical networks associated with flowering. Intl. J. Mol. Sci. 19:2384. doi: 10.3390/ijms19082384
Artlip, T. S., Wisniewski, M. E., Arora, R., and Norelli, J. L. (2016). An apple rootstock overexpressing a peach CBF gene alters growth and flowering in the scion but does not impact cold hardiness or dormancy. Hortic. Res. 3:16006.
Aslam, A., Zhao, S., Azam, M., Lu, X., He, N., Li, B., et al. (2020). Comparative analysis of primary metabolites and transcriptome changes between ungrafted and pumpkin-grafted watermelon during fruit development. PeerJ 8:e8259. doi: 10.7717/peerj.8259
Assunção, M., Santos, C., Brazão, J., Eiras-Dias, J., and Fevereiro, P. (2019). Understanding the molecular mechanisms underlying graft success in grapevine. BMC Plant Biol. 19:396. doi: 10.1186/s12870-019-1967-1968
Avramidou, E., Kapazoglou, A., Aravanopoulos, F. A., Xanthopoulou, A., Ganopoulos, I., Tsaballa, A., et al. (2014). Global DNA methylation changes in Cucurbitaceae inter-species grafting. Crop Breed. Appl. Biotechnol. 15, 112–116. doi: 10.1590/1984-70332015v15n2n20
Ayoub, S., and Qrunfleh, M. M. (2006). “A study on some physiological and anatomical aspects of rooting’Nabali’and’Raseei’olive semi-hardwood stem cuttings,” in Proceedings of the XXVII International Horticultural Congress-IHC2006: International Symposium on Citrus and Other Tropical and Subtropical, (Leuven: ISHS), 221–226. doi: 10.17660/actahortic.2008.773.32
Banerjee, A. K., Chatterjee, M., Yu, Y., Suh, S.-G., Miller, W. A., and Hannapel, D. J. (2006). Dynamics of a mobile RNA of potato involved in a long-distance signaling pathway. Plant Cell 18, 3443–3457. doi: 10.1105/tpc.106.042473
Banerjee, S., Banerjee, A., Gill, S. S., Gupta, O. P., Dahuja, A., Jain, P. K., et al. (2017). RNA Interference: a novel source of resistance to combat plant parasitic nematodes. Front. Plant Sci. 8:834. doi: 10.3389/fpls.2017.00834
Belmonte-Urena, L. J., Garrido-Cardenas, J. A., and Camacho-Ferre, F. (2020). Analysis of World Research on grafting in horticultural plants. HortScience 55, 112–120. doi: 10.21273/hortsci14533-19
Berdeja, M., Nicolas, P., Kappel, C., Dai, Z. W., Hilbert, G., Peccoux, A., et al. (2015). Water limitation and rootstock genotype interact to alter grape berry metabolism through transcriptome reprogramming. Hortic. Res. 2:15012.
Bilichak, A., and Kovalchuk, I. (2016). Transgenerational response to stress in plants and its application for breeding. J. Exp. Bot. 67, 2081–2092. doi: 10.1093/jxb/erw066
Chen, Z., Zhao, J., Hu, F., Qin, Y., Wang, X., and Hu, G. (2017). Transcriptome changes between compatible and incompatible graft combination of Litchi chinensis by digital gene expression profile. Sci. Rep. 7:3954.
Chitarra, W., Perrone, I., Avanzato, C. G., Minio, A., Boccacci, P., Santini, D., et al. (2017). Grapevine grafting: scion transcript profiling and defense-related metabolites induced by rootstocks. Front. Plant Sci. 8:654.
Cookson, S. J., and Ollat, N. (2013). Grafting with rootstocks induces extensive transcriptional re-programming in the shoot apical meristem of grapevine. BMC Plant Biol. 13:147. doi: 10.1186/1471-2229-13-147
Cookson, S. J., Clemente Moreno, M. J., Hevin, C., Nyamba Mendome, L. Z., Delrot, S., Trossat-Magnin, C., et al. (2013). Graft union formation in grapevine induces transcriptional changes related to cell wall modification, wounding, hormone signalling, and secondary metabolism. J. Exp. Bot. 64, 2997–3008. doi: 10.1093/jxb/ert144
Cookson, S., Clemente Moreno, M., Hevin, C., Nyamba Mendome, L., Delrot, S., Magnin, N., et al. (2014). Heterografting with non-self rootstocks induces genes involved in stress responses at the graft interface when compared with autografted controls. J. Exp. Bot. 65, 2473–2481. doi: 10.1093/jxb/eru145
Corso, M., and Bonghi, C. (2014). Grapevine rootstock effects on abiotic stress tolerance. Plant Sci. Today 1, 108–113. doi: 10.14719/pst.2014.1.3.64
Corso, M., Vannozzi, A., Maza, E., Vitulo, N., Meggio, F., Pitacco, A., et al. (2015). Comprehensive transcript profiling of two grapevine rootstock genotypes contrasting in drought susceptibility links the phenylpropanoid pathway to enhanced tolerance. J. Exp. Bot. 66, 5739–5752. doi: 10.1093/jxb/erv274
Corso, M., Vannozzi, A., Ziliotto, F., Zouine, M., Maza, E., Nicolato, T., et al. (2016). Grapevine rootstocks differentially affect the rate of ripening and modulate auxin-related genes in Cabernet Sauvignon berries. Front. Plant Sci. 7:69.
Dewan, S., De Frenne, P., Broeck, A. V., Steenackers, M., Vander Mijnsbrugge, K., and Verheyen, K. (2018). Transgenerational effects in asexually reproduced offspring of Populus. PLoS One 13:e0208591. doi: 10.1038/s41598-019-56934-56936
Doupis, G., Bertaki, M., Psarras, G., Kasapakis, I., and Chartzoulakis, K. (2013). Water relations, physiological behavior and antioxidant defence mechanism of olive plants subjected to different irrigation regimes. Sci. Hortic. 153, 150–156. doi: 10.1016/j.scienta.2013.02.010
Doupis, G., Chartzoulakis, K., Beis, A., and Patakas, A. (2011). Allometric and biochemical responses of grapevines subjected to drought and enhanced ultraviolet-B radiation. Aust. J. Grape Wine Res. 17, 36–42. doi: 10.1111/j.1755-0238.2010.00114.x
Duan, X. W., Zhang, W. N., Huang, J., Hao, L., Wang, S. N., Wang, A. D., et al. (2016). PbWoxT1 mRNA from pear (Pyrus betulaefolia) undergoes long-distance transport assisted by a polypyrimidine tract binding protein. New Phytol. 210, 511–524. doi: 10.1111/nph.13793
Dutt, M., Li, Z. T., Kelley, K. T., Dhekney, S. A., Van Aman, M., Tattersall, J., et al. (2007). Trangenic rootstock protein transmisison in grapevines. Acta Hortic. 738, 749–754. doi: 10.17660/actahortic.2007.738.99
Elsheery, N. I., Helaly, M. N., Omar, S. A., John, S. V., Zabochnicka-Swia̧tek, M., Kalaji, H. M., et al. (2020). Physiological and molecular mechanisms of salinity tolerance in grafted cucumber. South Afr. J. Bot. 130, 90–102. doi: 10.1016/j.sajb.2019.12.014
Fan, S., Wang, J., Lei, C., Gao, C., Yang, Y., Li, Y. M., et al. (2018). Identification and characterization of histone modification gene family reveal their critical responses to flower induction in apple. BMC Plant Biol. 18:173. doi: 10.1186/s12870-018-1388-1380
Fares, A., Bayabil, H. K., Zekri, M., Mattos-Jr, D., and Awal, R. (2017). Potential climate change impacts on citrus water requirement across major producing areas in the world. J. Water Climate Change 8, 576–592. doi: 10.2166/wcc.2017.182
Farinati, S., Rasori, A., Varotto, S., and Bonghi, C. (2017). Rosaceae fruit development, ripening and post-harvest: an epigenetic perspective. Front. Plant Sci. 8:1247. doi: 10.3389/fpls.2017.01247
Fazio, G., Robinson, T. L., and Aldwinckle, H. S. (2015). The Geneva apple rootstock breeding program. Plant Breed. Rev. 39, 379–424. doi: 10.1002/9781119107743.ch08
Flachowsky, H., Szankowski, I., Waidmann, S., Peil, A., Tränkner, C., and Hanke, M.-V. (2012). The MdTFL1 gene of apple (Malus?×?domestica Borkh.) reduces vegetative growth and generation time. Tree Physiol. 32, 1288–1301. doi: 10.1093/treephys/tps080
Fuentes, I., Stegemann, S., Golczyk, H., Karcher, D., and Bock, R. (2014). Horizontal genome transfer as an asexual path to the formation of new species. Nature 511, 232–235. doi: 10.1038/nature13291
Fujimoto, R., Sasaki, T., Ishikawa, R., Osabe, K., Kawanabe, T., and Dennis, E. S. (2012). Molecular mechanisms of epigenetic variation in plants. Intl. J. Mol. Sci. 13, 9900–9922. doi: 10.3390/ijms13089900
Gallusci, P., Dai, Z., Génard, M., Gauffretau, A., Leblanc-Fournier, N., Richard-Molard, C. L., et al. (2017). Epigenetics for plant improvement: current knowledge and modeling avenues. Trends Plant Sci. 22, 610–623. doi: 10.1016/j.tplants.2017.04.009
Garcia-Lozano, M., Dutta, S. K., Natarajan, P., Tomason, Y. R., Lopez, C., Katam, R., et al. (2020). Transcriptome changes in reciprocal grafts involving watermelon and bottle gourd reveal molecular mechanisms involved in increase of the fruit size, rind toughness and soluble solids. Plant Mol. Biol. 102, 213–223. doi: 10.1007/s11103-019-00942-7
Gaut, B. S., Miller, A. J., and Seymour, D. K. (2019). Living with two genomes: grafting and its implications for plant genome-to-genome interactions. Phenotypic Variation and Evolution. Annu. Rev. Genet. 53, 195–215. doi: 10.1146/annurev-genet-112618-043545
Gautier, A. T., Chambaud, C., Brocard, L., Ollat, N., Gambetta, G. A., Delrot, S., et al. (2019). Merging genotypes: graft union formation and scion-rootstock interactions. J. Exp. Bot. 70, 747–755. doi: 10.1093/jxb/ery422
Gautier, A. T., Cochetel, N., Merlin, I., Hevin, C., Lauvergeat, V., Vivin, P., et al. (2020). Scion genotypes exert long distance control over rootstock transcriptome responses to low phosphate in grafted grapevine. BMC Plant Biol. 20:367. doi: 10.1186/s12870-020-02578-y
Ghate, T. H., Sharma, P., Kondhare, K. R., Hannapel, D. J., and Banerjee, A. K. (2017). The mobile RNAs, StBEL11 and StBEL29, suppress growth of tubers in potato. Plant Mol. Biol. 93, 563–578. doi: 10.1007/s11103-016-0582-4
Gohlke, J., and Mosher, R. A. (2015). Exploiting mobile RNA silencing for crop improvement. Am. J. Bot. 102, 1399–1400. doi: 10.3732/ajb.1500173
Goldschmidt, E. E. (2014). Plant grafting: new mechanisms, evolutionary implications. Front. Plant Sci. 5:727.
Haroldsen, V. M., Szczerba, M. W., Aktas, H., Lopez-Baltazar, J., Odias, M. J., Chi-Ham, C. L., et al. (2012). Mobility of transgenic nucleic acids and proteins within grafted rootstocks for agricultural improvement. Front. Plant Sci. 3:39.
He, Y. H., and Li, Z. C. (2018). Epigenetic environmental memories in plants: establishment, maintenance, and reprogramming. Trends Genet. 34, 856–866. doi: 10.1016/j.tig.2018.07.006
Herman, J. J., and Sultan, S. E. (2016). DNA methylation mediates genetic variation for adaptive transgenerational plasticity. Proc. R. Soc. B Biol. Sci. 283:20160988. doi: 10.1098/rspb.2016.0988
Huang, L.-C., Lius, S., Huang, B.-L., Murashige, T., El Fatih, M. M., and Van Gundy, R. (1992). Rejuvenation of Sequoia sempervirens by repeated grafting of shoot tips onto juvenile rootstocks in vitro: model for phase reversal of trees. Plant Physiol. 98, 166–173. doi: 10.1104/pp.98.1.166
Huang, N.-C., and Yu, T.-S. (2009). The sequences of Arabidopsis GA-INSENSITIVE RNA constitute the motifs that are necessary and sufficient for RNA long-distance trafficking. Plant J. 59, 921–929. doi: 10.1111/j.1365-313X.2009.03918.x
Huen, A., Rodriguez-Medina, C., Ho, A., Atkins, C., and Smith, P. (2017). Long-distance movement of phosphate starvation-responsive microRNAs in Arabidopsis. Plant Biol. 19, 643–649. doi: 10.1111/plb.12568
Jayawickrama, K., McKeand, S., and Jett, J. (1997). Rootstock effects on scion growth and reproduction in 8-year-old grafted loblolly pine. Can. J. For. Res. 27, 1781–1787. doi: 10.1139/x97-152
Kaja, E., Szcześniak, M. W., Jensen, P. J., Axtell, M. J., McNellis, T., and Makałowska, I. (2015). Identification of apple miRNAs and their potential role in fire blight resistance. Tree Genet. Genomes 11:812.
Kalantidis, K., Schumacher, H. T., Alexiadis, T., and Helm, J. M. (2008). RNA silencing movement in plants. Biol. Cell 100, 13–26. doi: 10.1042/bc20070079
Kanehira, A., Yamada, K., Iwaya, T., Tsuwamoto, R., Kasai, A., Nakazono, M., et al. (2010). Apple phloem cells contain some mRNAs transported over long distances. Tree Genet. Genomes 6, 635–642. doi: 10.1007/s11295-010-0279-9
Kapazoglou, A., Ganopoulos, I., Tani, E., and Tsaftaris, A. (2018). Epigenetics, epigenomics and crop improvement. Adv. Bot. Res. 86, 287–324. doi: 10.1016/bs.abr.2017.11.007
Khaldun, A. B. M., Huang, W., Lv, H., Liao, S., Zeng, S., and Wang, Y. (2016). Comparative profiling of mirnas and target gene identification in distant-grafting between tomato and lycium (Goji Berry). Front. Plat J. 7:1475. doi: 10.3389/fpls.2016.01475
Kim, M., Canio, W., Kessler, S., and Sinha, N. (2001). Developmental changes due to long-distance movement of a homeobox fusion transcript in tomato. Science 293, 287–289. doi: 10.1126/science.1059805
Koundouras, S., Tsialtas, I. T., Zioziou, E., and Nikolaou, N. (2008). Rootstock effects on the adaptive strategies of grapevine (Vitis vinifera L.) cv. Cabernet–Sauvignon) under contrasting water status: leaf physiological and structural responses. Agricu. Ecosyst. Environ. 128, 86–96. doi: 10.1016/j.agee.2008.05.006
Kumar, S. R., and Sathishkumar, R. (2017). ““Small RNAs: master regulators of epigenetic silencing in plants,” in Plant Epigenetics eds N. Rajewsky, S. Jurga, and J. Barciszewski, (Cham: Springer), 89–106. doi: 10.1007/978-3-319-55520-1_5
Kumar, S., Verma, S., and Trivedi, P. K. (2017). Involvement of Small RNAs in Phosphorus and sulfur sensing, signaling and stress: current update. Front. Plant Sci. 8:285.
Latzel, V., Janeèek, Š, Doležal, J., Klimešová, J., and Bossdorf, O. (2014). Adaptive transgenerational plasticity in the perennial Plantago lanceolata. Oikos 123, 41–46. doi: 10.1111/j.1600-0706.2013.00537.x
Lemgo, G. N. Y., Sabbadini, S., Pandolfini, T., and Mezzetti, B. (2013). Biosafety considerations of RNAi-mediated virus resistance in fruit-tree cultivars and in rootstock. Transgenic Res. 22, 1073–1088. doi: 10.1007/s11248-013-9728-9721
Lewsey, M. G., Hardcastle, T. J., Melnyk, C. W., Molnar, A., Valli, A., Urich, M. A., et al. (2016). Mobile small RNAs regulate genome-wide DNA methylation. Proc. Natl. Acad. Sci. U.S.A. 113, E801–E810.
Li, C., and Zhang, B. (2016). MicroRNAs in control of plant development. J. Cell. Physiol. 231, 303–313. doi: 10.1002/jcp.25125
Li, G., Ma, J., Tan, M., Mao, J., An, N., Sha, G., et al. (2016). Transcriptome analysis reveals the effects of sugar metabolism and auxin and cytokinin signaling pathways on root growth and development of grafted apple. BMC Genom. 17:150. doi: 10.1186/s12864-016-2484-x
Li, S., Castillo-Gonzalez, C., Yu, B., and Zhang, X. (2017). The functions of plant small RNAs in development and in stress responses. Plant J. 90, 654–670. doi: 10.1111/tpj.13444
Limera, C., Sabbadini, S., Sweet, J. B., and Mezzetti, B. (2017). New biotechnological tools for the genetic improvement of major woody fruit species. Front. Plant Sci. 8:1418. doi: 10.3389/fpls.2017.01418
Liu, X., Walawage, S. L., Leslie, C. A., Dandekar, A. M., Tricoli, D. M., Hu, H., et al. (2017). In vitro gene expression and mRNA translocation from transformed walnut (Juglans regia) rootstocks expressing DsRED fluorescent protein to wild-type scions. Plant Cell Rep. 36, 877–885. doi: 10.1007/s00299-017-2116-1
Lopez, C., Cervera, M., Fagoaga, C., Moreno, P., Navarro, L., Flores, R., et al. (2010). Accumulation of transgene-derived siRNAs is not sufficient for RNAi-mediated protection against Citrus tristeza virus in transgenic Mexican lime. Mol. Plant Pathol. 11, 33–41. doi: 10.1111/j.1364-3703.2009.00566.X
Lough, T. J., and Lucas, W. J. (2006). Integrative plant biology: role of phloem long-distance macromolecular trafficking. Annu. Rev. Plant Biol. 57, 203–232. doi: 10.1146/annurev.arplant.56.032604.144145
Lucas, W. J., Yoo, B.-C., and Kragler, F. (2001). RNA as a long-distance information macromolecule in plants. Nat. Rev. Mol. Cell Biol. 2, 849–857. doi: 10.1038/35099096
McGarry, R. C., and Kragler, F. (2013). Phloem-mobile signals affecting flowers: applications for crop breeding. Trends Plant Sci. 18, 198–206. doi: 10.1016/j.tplants.2013.01.004
Melnyk, C. W., Gabel, A., Hardcastle, T. J., Robinson, S., Miyashima, S., Grosse, I., et al. (2018). Transcriptome dynamics at Arabidopsis graft junctions reveal an intertissue recognition mechanism that activates vascular regeneration. Proc. Natl. Acad. Sci. U.S.A. 115, E2447–E2456.
Melnyk, C. W., Molnar, A., and Baulcombe, D. C. (2011b). Intercellular and systemic movement of RNA silencing signals. EMBO J. 30, 3553–3563. doi: 10.1038/emboj.2011.274
Melnyk, C. W., Molnar, A., Bassett, A., and Baulcombe, D. C. (2011a). Mobile 24 nt small RNAs direct transcriptional gene silencing in the root meristems of Arabidopsis thaliana. Curr. Biol. 21, 1678–1683. doi: 10.1016/j.cub.2011.08.065
Mermigka, G., Verret, F., and Kalantidis, K. (2006). RNA silencing movement in plants. Rev. Cell Biol. Mol. Med. 1, 96–129.
Miao, L., Di, Q., Sun, T., Li, Y., Duan, Y., Wang, J., et al. (2019). Integrated metabolome and transcriptome analysis provide insights into the effects of grafting on fruit flavor of cucumber with different rootstocks. Intl. J. Mol. Sci. 20:3592. doi: 10.3390/ijms20143592
Mo, Z., Feng, G., Su, W., Liu, Z., and Peng, F. (2018). Transcriptomic analysis provides insights into grafting union development in pecan (Carya illinoinensis). Genes 9:71. doi: 10.3390/genes9020071
Molnar, A., Melnyk, C. W., Bassett, A., Hardcastle, T. J., Dunn, R., and Baulcombe, D. C. (2010). Small silencing RNAs in plants are mobile and direct epigenetic modification in recipient cells. Science 328, 872–875. doi: 10.1126/science.1187959
Morris, R. J. (2018). On the selectivity, specificity and signalling potential of the long-distance movement of messenger RNA. Curr. Opin. Plant Biol. 43, 1–7. doi: 10.1016/j.pbi.2017.11.001
Mudge, K., Janick, J., Scofield, S., and Goldschmidt, E. E. (2009). “A history of grafting,” in Horticultural Reviews, ed. J. Janick (Honoken, NJ: John Wiley & Sons, Inc), 437–493.
Nanda, A. K., and Melnyk, C. W. (2018). The role of plant hormones during grafting. J. Plant Res. 131, 49–58. doi: 10.1007/s10265-017-0994-5
Neves, D. M., Almeida, L. A. D., Santana-Vieira, D. D. S., Freschi, L., Ferreira, C. F., Soares, W. D. S., et al. (2017). Recurrent water deficit causes epigenetic and hormonal changes in citrus plants. Sci. Rep. 7:13684. doi: 10.1038/s41598-017-14161-x
Notaguchi, M., Higashiyama, T., and Suzuki, T. (2015). Identification of mRNAs that move over long distances using an RNA-Seq analysis of Arabidopsis/Nicotiana benthamiana heterografts. Plant Cell Physiol. 56, 311–321. doi: 10.1093/pcp/pcu210
Notaguchi, M., Kurotani, K., Sato, Y., Tabata, R., Kawakatsu, Y., Okayasu, K., et al. (2020). Cell-cell adhesion in plant grafting is facilitated by beta-1,4-glucanases. Science 369, 698–702. doi: 10.1126/science.abc3710
Notaguchi, M., Wolf, S., and Lucas, W. J. (2012). Phloem-Mobile Aux/IAA transcripts target to the root tip and modify root architectureF. J. Integr. Plant Biol. 54, 760–772. doi: 10.1111/j.1744-7909.2012.01155.x
Oerke, E.-C., and Dehne, H.-W. (2004). Safeguarding production—losses in major crops and the role of crop protection. Crop Prot. 23, 275–285. doi: 10.1016/j.cropro.2003.10.001
Pagliarani, C., Vitali, M., Ferrero, M., Vitulo, N., Incarbone, M., Lovisolo, C., et al. (2017). The accumulation of miRNAs differentially modulated by drought stress is affected by grafting in grapevine. Plant Physiol. 173, 2180–2195. doi: 10.1104/pp.16.01119
Patil, S., Shinde, M., Prashant, R., Kadoo, N., Upadhyay, A., and Gupta, V. (2020). Comparative proteomics unravels the differences in salt stress response of own-rooted and 110R-grafted thompson seedless grapevines. J. Proteome Res. 19, 583–599. doi: 10.1021/acs.jproteome.9b00420
Peccoux, A., Loveys, B., Zhu, J., Gambetta, G. A., Delrot, S., Vivin, P., et al. (2018). Dissecting the rootstock control of scion transpiration using model-assisted analyses in grapevine. Tree Physiol. 38, 1026–1040. doi: 10.1093/treephys/tpx153
Perrin, A., Daccord, N., Roquis, D., Celton, J.-M., Vergne, E., and Bucher, E. (2020). Divergent DNA methylation signatures of juvenile seedlings, grafts and adult apple trees. Epigenomes 4:4. doi: 10.3390/epigenomes4010004
Poltronieri, P., Marrazzo, M. T., and Cipriani, G. (2020). “Grapevine: resistance genes, sRNAs and immunity,” in Applied Plant Biotechnology for Improving Resistance to Biotic Stress, eds Y. Hong and P. Poltronieri. (Amsterdam: Elsevier), 151–179. doi: 10.1016/b978-0-12-816030-5.00007-0
Ren, W., Hu, N., Hou, X., Zhang, J., Guo, H., Liu, Z., et al. (2017). Long-term overgrazing-induced memory decreases photosynthesis of clonal offspring in a perennial grassland plant. Front. Plant Sci. 8:419.
Ren, Y., Xu, Q., Wang, L., Guo, S., Shu, S., Lu, N., et al. (2018). Involvement of metabolic, physiological and hormonal responses in the graft-compatible process of cucumber/pumpkin combinations was revealed through the integrative analysis of mRNA and miRNA expression. Plant Physiol. Biochem. 129, 368–380. doi: 10.1016/j.plaphy.2018.06.021
Rendina González, A. P., Preite, V., Verhoeven, K. J., and Latzel, V. (2018). Transgenerational effects and epigenetic memory in the clonal plant Trifolium repens. Front. Plant Sci. 9:1677.
Robert, M. L., Juárez-Gómez, J., Chaires-Pacheco, M., and Peña-Ramírez, Y. J. (2020). Successive grafting confers juvenility traits to adult Spanish red cedar (Cedrela odorata Linnaeus): a tool for the rescue of selected materials. New For. 51, 335–347. doi: 10.1007/s11056-019-09736-7
Ruiz-Medrano, R., Xoconostle-Cázares, B., and Lucas, W. J. (1999). Phloem long-distance transport of CmNACP mRNA: implications for supracellular regulation in plants. Development 126, 4405–4419.
Sandanayaka, W. R. M., Bus, V. G. M., Connolly, P., and Newcomb, R. (2003). Characteristics associated with Woolly Apple Aphid Eriosoma lanigerum, resistance of three apple rootstocks. Entomologia Experimentalis et Applicata 109, 63–72. doi: 10.1046/j.1570-7458.2003.00095.x
Santos, A. S., Neves, D. M., Santana-Vieira, D. D. S., Almeida, L. A. H., Costa, M. G. C., Soares Filho, W. S., et al. (2020). Citrus scion and rootstock combinations show changes in DNA methylation profiles and ABA insensitivity under recurrent drought conditions. Sci. Hortic. 267:109313. doi: 10.3389/fpls.2016.01475
Sharma, A., and Zheng, B. (2019). Molecular responses during plant grafting and its regulation by auxins, cytokinins, and gibberellins. Biomolecules 9:397. doi: 10.3390/biom9090397
Smolka, A., Li, X.-Y., Heikelt, C., Welander, M., and Zhu, L.-H. (2010). Effects of transgenic rootstocks on growth and development of non-transgenic scion cultivars in apple. Transgenic Res. 19, 933–948. doi: 10.1007/s11248-010-9370-9370
Song, G.-Q., Prieto, H., and Orbovic, V. (2019a). Agrobacterium-mediated transformation of tree fruit crops: methods, progress, and challenges. Front. Plant Sci. 10:226. doi: 10.3389/fpls.2019.00226
Song, G.-Q., Sink, K. C., Walworth, A. E., Cook, M. A., Allison, R. F., and Lang, G. A. (2013). Engineering cherry rootstocks with resistance to Prunus necrotic ring spot virus through RNAi-mediated silencing. Plant Biotechnol. J. 11, 702–708. doi: 10.1111/pbi.12060
Song, G.-Q., Walworth, A. E., and Loescher, W. H. (2015). Grafting of genetically engineered plants. Am. Soc. Hortic. Sci. 140, 203–213. doi: 10.21273/jashs.140.3.203
Song, G.-Q., Walworth, A., Lin, T., Chen, Q., Han, X., Zaharia, L. I., et al. (2019b). VcFT-induced mobile florigenic signals in transgenic and transgrafted blueberries. Hortic. Res. 6:105.
Sorce, C., Massai, R., Picciarelli, P., and Lorenzi, R. (2002). Hormonal relationships in xylem sap of grafted and ungrafted Prunus rootstocks. Sci. Hortic. 93, 333–342. doi: 10.1016/s0304-4238(01)00338-7
Spanò, R., Ferrara, M., Montemurro, C., Mulè, G., Gallitelli, D., and Mascia, T. (2020). Grafting alters tomato transcriptome and enhances tolerance to an airborne virus infection. Sci. Rep. 10:2538. doi: 10.1038/s41598-020-59421-59425
Stegemann, S., and Bock, R. (2009). Exchange of genetic material between cells in plant tissue grafts. Science 324, 649–651. doi: 10.1126/science.1170397
Stegemann, S., Keuthe, M., Greiner, S., and Bock, R. (2012). Horizontal transfer of chloroplast genomes between plant species. Proc. Natl. Acad. Sci. U. S. A. 109, 2434–2438. doi: 10.1073/pnas.1114076109
Suter, L., and Widmer, A. (2013). Environmental heat and salt stress induce transgenerational phenotypic changes in Arabidopsis thaliana. PLoS One 8:e60364. doi: 10.1371/journal.pone.0060364
Tamiru, M., Hardcastle, T. J., and Lewsey, M. G. (2018). Regulation of genome-wide DNA methylation by mobile small RNAs. New Phytol. 217, 540–546. doi: 10.1111/nph.14874
Thieme, C. J., Rojas-Triana, M., Stecyk, E., Schudoma, C., Zhang, W., Yang, L., et al. (2015). Endogenous Arabidopsis messenger RNAs transported to distant tissues. Nat. Plants 1:15025.
Thomas, H. R., and Frank, M. H. (2019). Connecting the pieces: uncovering the molecular basis for long-distance communication through plant grafting. New Phytol. 223, 582–589. doi: 10.1111/nph.15772
Tricker, P. J. (2015). Transgenerational inheritance or resetting of stress-induced epigenetic modifications: two sides of the same coin. Front. Plant Sci. 6:699.
Tsikou, D., Yan, Z., Holt, D. B., Abel, N. B., Reid, D. E., Madsen, L. H., et al. (2018). Systemic control of legume susceptibility to rhizobial infection by a mobile microRNA. Science 362, 233–236. doi: 10.1126/science.aat6907
Uthup, T. K., Karumamkandathil, R., Ravindran, M., and Saha, T. (2018). Heterografting induced DNA methylation polymorphisms in Hevea brasiliensis. Planta 248, 579–589. doi: 10.1007/s00425-018-2918-6
Valverdi, N. A., Cheng, L., and Kalcsits, L. (2019). Apple scion and rootstock contribute to nutrient uptake and partitioning under different belowground environments. Agronomy 9:415. doi: 10.3390/agronomy9080415
Van Meijl, H., Havlik, P., Lotze-Campen, H., Stehfest, E., Witzke, P., Domínguez, I. P., et al. (2018). Comparing impacts of climate change and mitigation on global agriculture by 2050. Environ. Res. Lett. 13:064021.
Varkonyi-Gasic, E., Gould, N., Sandanayaka, M., Sutherland, P., and MacDiarmid, R. M. (2010). Characterisation of microRNAs from apple (Malus domestica’Royal Gala’) vascular tissue and phloem sap. BMC Plant Biol. 10:159. doi: 10.1186/1471-2229-10-159
Varotto, S., Tani, E., Abraham, E., Krugman, T., Kapazoglou, A., Melzer, R., et al. (2020). Epigenetics: possible applications in climate-smart crop breeding. J. Exp. Bot. 71, 5223–5236. doi: 10.1093/jxb/eraa188
Verhoeven, K. J., and Preite, V. (2014). Epigenetic variation in asexually reproducing organisms. Evolution 68, 644–655. doi: 10.1111/evo.12320
von Aderkas, P., and Bonga, J. M. (2000). Influencing micropropagation and somatic embryogenesis in mature trees by manipulation of phase change, stress and culture environment. Tree Physiol. 20, 921–928. doi: 10.1093/treephys/20.14.921
Wang, H., Zhou, P., Zhu, W., and Wang, F. (2019a). De novo comparative transcriptome analysis of genes differentially expressed in the scion of homografted and heterografted tomato seedlings. Sci. Rep. 9:20240.
Wang, J. W. (2014). Regulation of flowering time by the miR156-mediated age pathway. J. Exp. Bot. 65, 4723–4730. doi: 10.1093/jxb/eru246
Wang, J., Jiang, L., and Wu, R. (2017). Plant grafting: how genetic exchange promotes vascular reconnection. New Phytol. 214, 56–65. doi: 10.1111/nph.14383
Wang, W.-Q., Allan, A. C., and Yin, X.-R. (2020). Small RNAs with a big impact on horticultural traits. Crit. Rev. Plant Sci. 39, 30–43. doi: 10.1080/07352689.2020.1741923
Wang, Y., Chen, W.-K., Gao, X.-T., He, L., Yang, X.-H., He, F., et al. (2019b). Rootstock-mediated effects on cabernet sauvignon performance: vine growth, berry ripening, flavonoids, and aromatic profiles. Intl. J. Mol. Sci. 20:401. doi: 10.3390/ijms20020401
Warschefsky, E. J., Klein, L. L., Frank, M. H., Chitwood, D. H., Londo, J. P., von Wettberg, E. J., et al. (2016). Rootstocks: diversity, domestication, and impacts on shoot phenotypes. Trends Plant Sci. 21, 418–437. doi: 10.1016/j.tplants.2015.11.008
Wendling, I., Trueman, S. J., and Xavier, A. (2014). Maturation and related aspects in clonal forestry—part II: reinvigoration, rejuvenation and juvenility maintenance. New For. 45, 473–486. doi: 10.1007/s11056-014-9415-y
Wu, R., Wang, X., Lin, Y., Ma, Y., Liu, G., Yu, X., et al. (2013). Inter-species grafting caused extensive and heritable alterations of DNA methylation in solanaceae plants. PLoS One 8:e61995. doi: 10.1371/journal.pone.0061995
Xanthopoulou, A., Tsaballa, A., Ganopoulos, I., Kapazoglou, A., Avramidou, E., Aravanopoulos, F. A., et al. (2019). I ntra-species grafting induces epigenetic and metabolic changes accompanied by alterations in fruit size and shape of Cucurbita pepo L. Plant Growth Regul. 87, 93–108. doi: 10.1007/s10725-018-0456-7
Xia, C., Zheng, Y., Huang, J., Zhou, X., Li, R., Zha, M., et al. (2018). Elucidation of the mechanisms of long-distance mRNA movement in a Nicotiana benthamiana/tomato heterograft system. Plant Physiol. 177, 745–758. doi: 10.1104/pp.17.01836
Xoconostle-Cázares, B., Xiang, Y., Ruiz-Medrano, R., Wang, H.-L., Monzer, J., Yoo, B.-C., et al. (1999). Plant paralog to viral movement protein that potentiates transport of mRNA into the phloem. Science 283, 94–98. doi: 10.1126/science.283.5398.94
Xu, H. Y., Iwashiro, R., Li, T. Z., and Harada, T. (2013). Long-distance transport of gibberellic acid insensitive mRNA in Nicotiana benthamiana. BMC Plant Biol. 13:165. doi: 10.1186/1471-2229-13-165
Xu, H., Zhang, W., Li, M., Harada, T., Han, Z., and Li, T. (2010). Gibberellic acid insensitive mRNA transport in both directions between stock and scion in Malus. Tree Genet. Genomes 6, 1013–1019. doi: 10.1007/s11295-010-0309-7
Yadav, J. S., Ogwok, E., Wagaba, H., Patil, B. L., Bagewadi, B., Alicai, T., et al. (2011). RNAi-mediated resistance to cassava brown streak Uganda virus in transgenic cassava. Mol. Plant Pathol. 12, 677–687. doi: 10.1111/j.1364-3703.2010.00700.X
Yang, Y., Mao, L., Jittayasothorn, Y., Kang, Y., Jiao, C., Fei, Z., et al. (2015). Messenger RNA exchange between scions and rootstocks in grafted grapevines. BMC Plant Biol. 15:251.
Yang, L., Perrera, V., Saplaoura, E., Apelt, F., Bahin, M., Kramdi, A., et al. (2019). m5C methylation guides systemic transport of messenger RNA over graft junctions in plants. Curr. Biol. 29, 2465–2476. doi: 10.1016/j.cub.2019.06.042
Zamorskyi, V. (2011). Anatomic Features of the Young Grafted Apple Trees. Leuven: International Society for Horticultural Science, 897–902.
Zhang, J., Yang, J., Yang, Y., Luo, J., Zheng, X., Wen, C., et al. (2019a). Transcription factor CsWIN1 regulates pericarp wax biosynthesis in cucumber grafted on pumpkin. Front. Plant Sci. 10:1564.
Zhang, W.-N., Gong, L., Ma, C., Xu, H.-Y., Hu, J.-F., Harada, T., et al. (2012). Gibberellic acid-insensitive mRNA transport in Pyrus. Plant Mol. Biol. Rep. 30, 614–623. doi: 10.1007/s11105-011-0365-7
Zhang, Z., Cao, B., Li, N., Chen, Z., and Xu, K. (2019b). Comparative transcriptome analysis of the regulation of ABA signaling genes in different rootstock grafted tomato seedlings under drought stress. Environ. Exp. Bot. 166:103814. doi: 10.1016/j.envexpbot.2019.103814
Zhang, Z., Zheng, Y., Ham, B.-K., Chen, J., Yoshida, A., Kochian, L. V., et al. (2016). Vascular-mediated signalling involved in early phosphate stress response in plants. Nat. Plants 2:16033.
Zhao, D., and Song, G. Q. (2014). Rootstock-to-scion transfer of transgene-derived small interfering RNA s and their effect on virus resistance in nontransgenic sweet cherry. Plant Biotechnol. J. 12, 1319–1328. doi: 10.1111/pbi.12243
Zheng, X., Chen, L., Li, M., Lou, Q., Xia, H., Wang, P., et al. (2013). Transgenerational variations in DNA methylation induced by drought stress in two rice varieties with distinguished difference to drought resistance. PLoS One 8:e80253. doi: 10.1371/journal.pone.0080253
Keywords: grafting, rootstock, scion, woody species, epigenetics, transcriptional reprogramming, climate change, transgenerational inheritance
Citation: Kapazoglou A, Tani E, Avramidou EV, Abraham EM, Gerakari M, Megariti S, Doupis G and Doulis AG (2021) Epigenetic Changes and Transcriptional Reprogramming Upon Woody Plant Grafting for Crop Sustainability in a Changing Environment. Front. Plant Sci. 11:613004. doi: 10.3389/fpls.2020.613004
Received: 01 October 2020; Accepted: 10 December 2020;
Published: 12 January 2021.
Edited by:
Rosario Paolo Mauro, University of Catania, ItalyReviewed by:
Yuan Huang, Huazhong Agricultural University, ChinaCopyright © 2021 Kapazoglou, Tani, Avramidou, Abraham, Gerakari, Megariti, Doupis and Doulis. This is an open-access article distributed under the terms of the Creative Commons Attribution License (CC BY). The use, distribution or reproduction in other forums is permitted, provided the original author(s) and the copyright owner(s) are credited and that the original publication in this journal is cited, in accordance with accepted academic practice. No use, distribution or reproduction is permitted which does not comply with these terms.
*Correspondence: Aliki Kapazoglou, YWthcGF6b2dsb3VAZ21haWwuY29t; Andreas G. Doulis, YW5kcmVhcy5kb3VsaXNAbmFncmVmLWhlci5ncg==
Disclaimer: All claims expressed in this article are solely those of the authors and do not necessarily represent those of their affiliated organizations, or those of the publisher, the editors and the reviewers. Any product that may be evaluated in this article or claim that may be made by its manufacturer is not guaranteed or endorsed by the publisher.
Research integrity at Frontiers
Learn more about the work of our research integrity team to safeguard the quality of each article we publish.