- 1Department of Sustainable Crop Production, Università Cattolica del Sacro Cuore, Piacenza, Italy
- 2CEB – Centre of Biological Engineering, Micoteca da Universidade do Minho, University of Minho, Braga, Portugal
- 3Soremartec Italia S.r.l., Alba, Italy
Fungi of the genus Diaporthe have been reported as the main causative agent of hazelnut defects in the Caucasus area. This study aimed to define which fungal species are present in defective hazelnuts grown in Turkey and confirm the role of Diaporthe spp. Seven hazelnut orchards were selected, with each one located in a different Turkish Province (Düzce, Giresun, Ordu, Samsun, Sakarya, Trabzon, and Zonguldak), and hazelnuts were collected at early and full ripening. Fungal isolation and identification were performed at the genus level based on morphological characteristics. Several genera were isolated, with Diaporthe spp. being among the prevalent. This was the only genus with increasing incidence from early to full ripening, and incidence at full ripening was positively correlated both with internal (ρ = 0.86) and visible defects (ρ = 0.81), which confirmed its role as the key causative agent of hazelnut defects. The correlation of defect occurrence with rainfall, reported in previous study, was not confirmed, possibly due to the low defect incidence. A total of 86 Diaporthe monosporic strains isolated from Turkish hazelnut samples, together with 33 strains collected in the Caucasus region and 6 from Italy, were analyzed with a multi-locus phylogeny based on three genomic loci (ITS, EF1-α, and tub). The results showed that Diaporthe strains can be grouped into 7 distinct clades, with a majority of Turkish strains (95%) being placed into a single clade related with D. eres. These samples were organized into several sub-clades, which indicates the existence of genetically diverse sub-populations.
Introduction
Hazelnuts (Corylus avellana L.) are cultivated worldwide in areas of mild climate and high humidity. When considering the worldwide production of tree nuts, hazelnuts are the fifth most highly produced nut,1 at 528.07 thousand metric tons that are directly consumed or processed. Turkey is the main grower, producing approximately 72.9% of the total world supply2. In Turkey, hazelnuts are grown in different provinces, mainly in the Black Sea area (Islam, 2018).
Several defects have been reported in hazelnuts, such as the presence of blemishes, areas of discoloration, or stains in marked contrast with the rest of the kernel (Teviotdale et al., 2002). The resulting defective kernels are not compliant with the quality standards required by the market3. The incidence of defects varies between 1 and 15%, depending on the year, weather conditions, and growing area4. Thus, hazelnut defects, defined as “rotten hazelnuts” by commercial evaluation, negatively impact kernel availability on the market as well as economics. The identification of the causal agents is critical in order to define and apply preventive actions, improve hazelnut yield and quality, and thus increase the market value.
Diaporthe spp. fungi appears as necrotic spots on kernel surfaces and causes internal browning that is visible after cutting the nut in half (half-cut). In a previous study in the Caucasus region, Diaporthe spp. was identified as the crucial genus involved in causing hazelnut defects. Only three strains were identified at the species level in the study, but this suggested that D. eres was responsible for the visible brown spots on the kernel surface and the internal discoloration observable after the nut was cut in half (Battilani et al., 2018). D. eres was also recently reported by other authors as associated with hazelnut trunk cankers in Oregon (Wiman et al., 2019), while D. foeniculina was mentioned by Guerrero et al. (2019) as causing black tip and necrotic spots on hazelnut kernels in Chile and D. rudis was detected in hazelnut kernels with visible mold in Oregon (Pscheidt et al., 2019).
Diaporthe species have been frequently reported as an important group of plant pathogenic fungi, non-pathogenic endophytes, or saprobes, and are related to diseases that occur in a wide range of economically important plants (Farr et al., 2002a,b; Crous, 2005; Udayanga et al., 2011; Gomes et al., 2013; Huang et al., 2015; Chepkirui and Stadler, 2017; Guarnaccia et al., 2018).
Since their discovery, Diaporthe spp. and their asexual stage Phomopsis spp. have been identified based on morphology and host association (Uecker, 1988; Ferreira et al., 2015). However, the association between host and species is not reliable within the Diaporthe genus as an identification criterion (Gomes et al., 2013; Udayanga et al., 2014a,b). It has been observed that the same Diaporthe spp. colonizes different hosts, and the co-occurrence of different species is commonly reported in the same host (Rehner and Uecker, 1994; Mostert et al., 2001; Guarnaccia et al., 2016; Guarnaccia and Crous, 2017). Additionally, many studies have recently claimed that morphology is generally not conclusive for identification at the species level due to the high complexity of the Diaporthe genus (Santos et al., 2010; Udayanga et al., 2011; Dissanayake et al., 2017a,b). Therefore, by using molecular approaches, substantial progress regarding the identification and characterization of emerging pathogens in the Diaporthe genus has been realized (Santos and Phillips, 2009; Diogo et al., 2010; Luongo et al., 2011; Udayanga et al., 2012a,b; Thomidis et al., 2013; Gao et al., 2017; Guarnaccia and Crous, 2017). Notably, among the various techniques, multi-gene phylogenetic species delineation is becoming the most effective instrument for taxonomic studies of fungi (Taylor et al., 2000; Dettman et al., 2003). Regarding Diaporthe spp., the taxonomy of the genus is especially based on ITS (internal transcribed spacer region of ribosomal DNA), EF1-α (translation elongation factor 1-alpha gene), tub (β-tubulin), and cal (calmodulin) loci sequences (Udayanga et al., 2012a; Gomes et al., 2013).
Based on this background, the objectives of this study were to (i) investigate the fungi associated with defective hazelnuts in Turkey, with a special focus on the role of Diaporthe spp.; (ii) characterize Diaporthe strains based on molecular techniques, using multi-locus phylogenetic species identification by means of ITS, tub, and EF1-α; and (iii) identify, at the sub-genus level, the Diaporthe strains isolated from hazelnut kernels.
Materials and Methods
Culture Media
Potato Dextrose Agar (PDA). Agar, 15 g; natural potato broth obtained from 200 g potato, dextrose, 10 g, double-distilled water, 1 L; streptomycin sulfate added during cooling, 0.15 g L–1. Water Agar (WA). Agar, 15 g; double-distilled water, 1 L. Streptomycin sulfate added during cooling, 0.15 g L–1. Malt Glucose Yeast Peptone (MGYP). Malt extract, 3 g; mycological peptone, 5 g; glucose, 10 g; yeast extract, 3 g; double-distilled water, 1 L.
Molecular Biology Buffers and Solutions
Hexadecyl trimethyl-ammonium bromide 2% buffer (CTAB). Cetyl trimethylammonium bromide (CTAB), 2.0 g; 2 M Tris-HCl pH 8.4, 5.0 ml; 0.2 M ethylenediaminetetraacetic acid (EDTA), di-sodium salt, pH 8.0, 12.48 ml; NaCl, 8.18 g. The volume was adjusted to 100 ml with distilled water. 2 M Tris-HCl pH 8.0. Tris base, 12.114 g; distilled water, 50 ml. The pH was adjusted to 8.0 by adding 2 M NaOH. 0.2 M EDTA pH 8.0. Ethylenediaminetetraacetic acid, di-sodium salt, 3.7224 g; distilled water, 50 ml. The pH was adjusted to 8.4 by adding HCl. The solution was cooled to room temperature before making the final adjustments to the pH. 1% Agarose gel. Agarose, 1 g; Tris-acetate EDTA (TAE) 0.5× buffer, 100 ml; GreenSafe Premium (NZYtech, Lisbon, Portugal), 5.3 μL. 50× TAE buffer. Tris base, 242 g; acetic acid, glacial, 57.1 mL; 0.5 M EDTA pH 8.0, 100 mL; distilled water to 1 L. To make a 0.5× working solution, the concentrated stock solution was diluted at 1:100 dilution concentrated stock.
Hazelnut Orchards and Meteorological Data Collection
In 2017, 7 hazelnut-growing provinces in different Turkish locations were selected for this study (Figure 1). One orchard was considered in each province, for a total of 7 sampling points. Three orchards were located in hazelnut growing provinces in West Turkey (Düzce, Sakarya, Zonguldak), and the other orchards were located in 4 growing provinces in East Turkey (Giresun, Ordu, Samsun, and Trabzon). A mix of different hazelnut varieties were grown in the orchards sampled; Kara findik, Mincane, Çakildak and Foşa were reported in Sakarya, Düzce and Zonguldak, while Tombul, Palaz, Mincane, Çakildak, Foşa and Sivri in Samsun, Ordu, Giresun and Trabzon. Orchards were managed according to common hazelnut agricultural practices used in Turkey. At ripening, hazelnuts were hand harvested from the plant and dried on the ground for approximately one week; then, husks were mechanically removed and in shell drying continued on the ground until a final kernel humidity ≤6%. Wireless weather stations (Vantage Pro2TM Plus®, Davis Instruments) were placed close to the orchards (approximately 500 m distance; the GPS coordinates are shown in Table 1), and hourly data consisting of air temperature (T, °C), air relative humidity (%RH), and rainfall (R, mm) were recorded from January 1st to August 30th in each orchard.
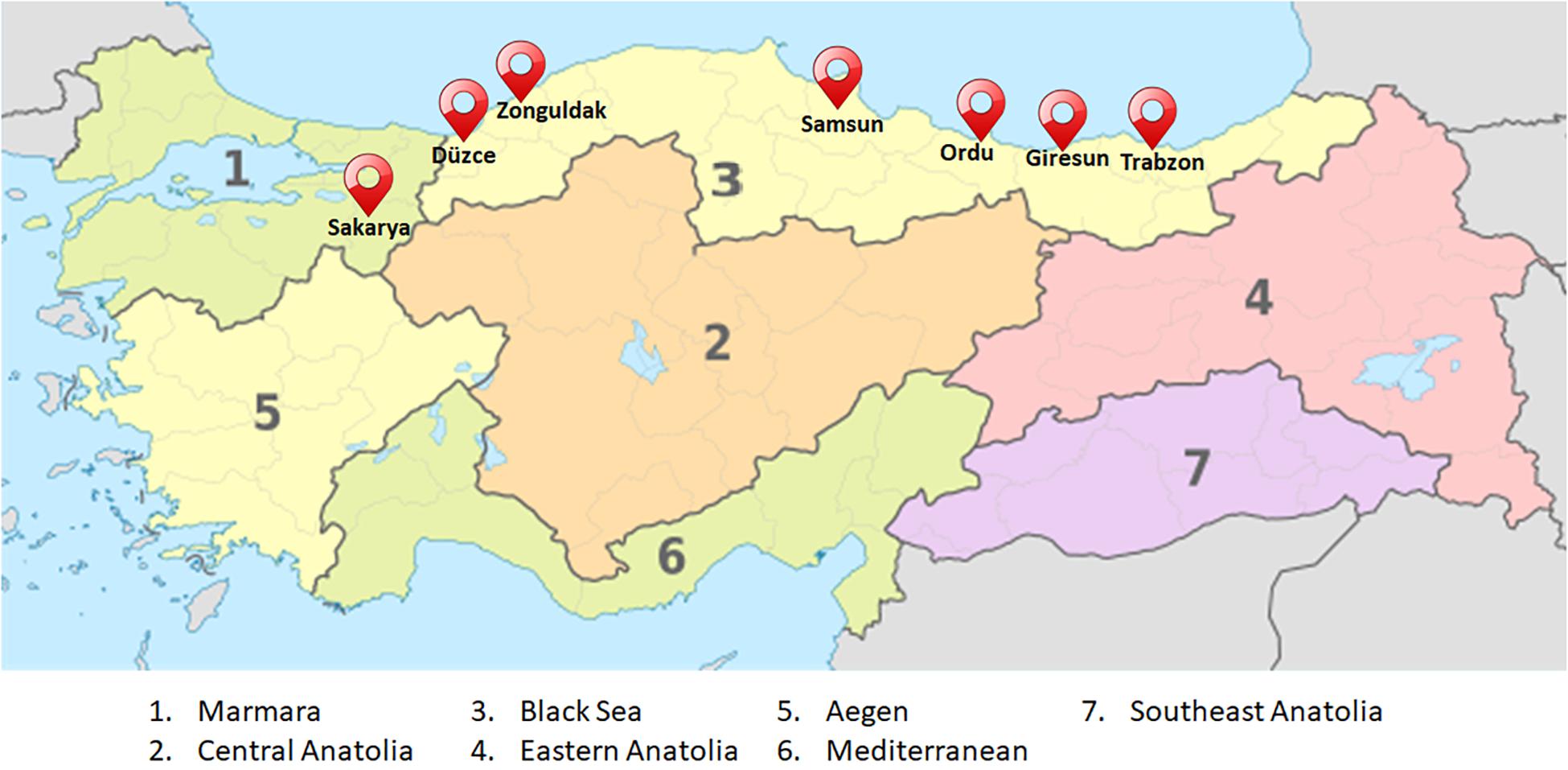
Figure 1. Distribution of the seven sampled hazelnut orchards located in different Turkish provinces (https://upload.wikimedia.org/wikipedia/commons/f/f9/Turkey_ %28regions%29%2C_administrative_divisions_-_Nmbrs_-_colored.svg adapted).
Hazelnut Sampling
Hazelnuts were collected approximately 45 days after setting, at early ripening (BBCH 81) and at full ripening (BBCH 89), according to Battilani et al. (2018). Each orchard was approximately 1,000–2,000 m2. In each orchard, 100 trees were selected along 2 diagonals of the orchard, and 30 hazelnuts per tree were randomly collected in order to obtain a total of 3,000 hazelnuts per sampling point and time. The nuts were shelled and observed for defects, both superficial and internal, the latter after the kernel was cut in half (Teserba GmbH, Rüti, Switzerland).
The hazelnuts were stored at 5°C under vacuum until they were delivered to the laboratory. Two hundred and seventy defective kernels were randomly selected from each of the 7 orchards (90 half-cut nuts, analyzed in triplicate), with some exception of smaller samples in BBCH81.
Fungi Isolation and Identification
Hazelnuts collected at early and full ripening were processed using the same protocol. Half-cut kernels were washed with running tap water for 1 min, disinfected with 1% sodium hypochlorite solution for 1 min, rinsed 3 times in sterile double-distilled water, and then dried on sterile paper under a sterile hood. Half-cut kernels were plated in 90-mm diameter Petri dishes containing WA and incubated at 25°C, with a natural photoperiod, up to 21-day. The plates were viewed twice a week for fungal growth, and visible colonies were transferred to PDA dishes and incubated at 25°C, with a natural photoperiod, until the development of reproductive structures, for a maximum of 30-day. Morphological characterization was determined with the support of a stereomicroscope (Motic) with 40× magnification, and an optical microscope (Leitz labor lux D) at 500× magnification, following taxonomic keys to identify the isolates at the genus level (Raper and Fennell, 1965; Ellis, 1971, 1976; Pitt, 1979; Sutton, 1980; Rotem, 1994; Krol, 2005; Leslie and Summerell, 2007; Udayanga et al., 2011; Gomes et al., 2013; Visagie et al., 2013; Maharachchikumbura et al., 2014; Samson et al., 2014). When available, two to three well-separated colonies of Diaporthe spp. were selected from each replicate for further studies. They were subject to conditions that resulted in obtaining monosporic cultures according to Battilani et al. (2018). Colonies grown on PDA were transferred to tube vials containing WA and stored at 4°C until use.
Of the 125 Diaporthe spp. strains used in this study for molecular characterization, 86 Diaporthe spp. strains were collected in Turkey (this study), 33 in the Caucasus region (Battilani et al., 2018), and 6 were from Italy. The nuts were gathered using the same protocol as that used to collect hazelnuts in the Caucasus region. All 125 Diaporthe spp. strains were deposited in Micoteca da Universidade do Minho (MUM) culture collection, Braga, Portugal.
Diaporthe spp. DNA Extraction
Following a previously described protocol (Rodrigues et al., 2009) with some modifications, all 125 monosporic Diaporthe spp. strains previously mentioned were inoculated into 5-mL tubes containing 3 mL of MGYP and incubated at 25°C for 14-day with rotation at 150 rev min–1. The mycelia were collected by filtration and stored at −20°C. Genomic DNA was extracted using the following protocol: 200 mg of filtrate was placed in a 2-mL tube containing 0.67 g glass beads (Sigma, 710-1, 180 μm) and 1 mL of CTAB buffer. FastPrep-24TM 5G (MP Biomedicals) was used at speed 6 for 30 s to break down the cellular membrane. After centrifugation at 14,000 × g for 8 min, 650 μL of supernatant was transferred in a new 2-mL tube, 750 μL of sodium acetate (3 M, pH 5.5) was then added, and the liquid in the tube was gently mixed by inversion and subsequently incubated at −20°C for 10 min. Then, samples were centrifuged at 14,000 × g for 10 min, and 750 μL of supernatant was transferred to a new 2-mL tube containing 750 μL of 2-propanol, gently mixed, and incubated at room temperature for 1 h. Samples were centrifuged at 14,000 × g for 10 min, and the supernatant was decanted without disturbing the pellet, which was then washed with 750 μL ice-cold 70% ethanol. The ethanol was decanted, and the last step was repeated. Residual ethanol was removed by drying in a SpeedVac concentrator (SPDIIIV, Thermo Scientific).
DNA was dissolved in 50 μL of ultrapure water, and samples were placed in a water bath at 56°C for 2 h. Next, 1 μL of RNAse (10 mg/mL) was added, and the samples were placed again in a water bath at 36°C for 1 h. The DNA was subjected to quality assessment by electrophoresis (120 V/cm for 7 min plus 80 V/cm for 30 min) on 1% (w/v) agarose in 0.5× TAE buffer gel. NZYDNA Ladder III was used as a DNA molecular weight marker. In addition, the DNA quantity and quality were measured by reading the entire absorption spectrum (220–750 nm) with a NanoDrop ND-1000 micro-spectrophotometer and calculating DNA concentration and absorbance ratio at both 260/280 and 230/260 nm. The machine was calibrated and cleaned according to the calibration check procedure.
PCR Amplification and Sequencing
DNA samples were diluted and equalized to 50 ng/μL. PCR reactions were performed in 50 μL reaction mixtures containing Taq 2x VWR Master Mix, 25 μL; genomic DNA, 1 μL; 10 μM primers, 2 μL (1 μL for each primer used); and ultrapure sterile water, 22 μL. The amplifications were performed using a Bio-Rad C1000 thermocycler. After a preliminary trial, primer pairs ITS1/ITS4 for the ITS region of the nuclear ribosomal RNA operon, EF1-728F/EF1-986R for partial EF1-α gene amplification, and Bt2a/Bt2b for partial tub gene amplification were selected and used in this study applying PCR conditions reported by the authors (Table 2). Amplified products were resolved by electrophoresis using the same conditions previously described. PCR products were purified using the E.Z.N.A. Cycle Pure kit (Omega) according to the manufacturer’s instructions and then sent to a commercial laboratory that performed Sanger sequencing (Eurofinsgenomics, Germany).
Molecular Data Analysis
Sequence files were processed using the pattern analysis software package BioEdit Sequence Alignment Editor v.7.0.5.0 (Hall, 1999). To establish the identity of the strains at the species level, combined phylogenetic analyses of the three considered loci (ITS + tub + EF1-α) were conducted against those of different species sequences selected by extensive literature review (Udayanga et al., 2011, 2014a,b, 2015; Gomes et al., 2013; Lombard et al., 2014; Huang et al., 2015; Dissanayake et al., 2017a,b; Gao et al., 2017; Santos et al., 2017a,b; Fan et al., 2018; Guarnaccia et al., 2018; Yang et al., 2018; Long et al., 2019; Manawasinghe et al., 2019; Marin-Felix et al., 2019; Ozawa et al., 2019; Zhou and Hou, 2019; Hilário et al., 2020) and retrieved from the National Center for Biotechnology Information (NCBI) database (Supplementary Table 1). Individual alignments were performed using the MUSCLE tool (Edgar, 2004) implemented in MegaX (Kumar et al., 2018). Poorly aligned positions and divergent regions were eliminated using the Gblocks v.0.91b online tool (Castresana, 2000).
In order to perform a multigene phylogeny reconstruction, datasets were concatenated using the online tool FaBox (Villesen, 2007). The most suitable substitution model was determined based on the lowest Bayesian information criterion. Maximum-likelihood trees were constructed in MegaX through 1,000 bootstrap replications (Felsenstein, 1985) based on the Tamura-Nei (Tamura and Nei, 1993) substitution model (TN93) considering non-uniformity of evolutionary rates among sites modeled using a discrete Gamma distribution (+ G) with 5 rate categories and assuming that a certain fraction of sites is evolutionarily invariable (+ I). Bayesian posterior probabilities of branches were computed in MrBayes v.3.2.7 (Ronquist et al., 2012) using settings for the best-fit model selected by the Akaike information criterion in MrModeltest v.2.4 (GTR + I + G) (Nylander, 2004). Obtained trees were edited in the iTOL v.5.6 program (Letunic and Bork, 2019).
Data Analysis
In relation to the hazelnut growing season, different meteorological parameters were computed for four different time periods (P1: 1 January-30 April; P2: 1 May-30 June; P3: 1 July-31 July; P4: 1 August-30 August). In particular, the mean air temperature (Tm;°C) was calculated as the mean of the temperature for each period; degree day (DD;°C) was computed as the sum of the mean daily T; summation of DD (°C) was obtained by adding the DD of each period; total R (mm) was computed as the sum of daily R (mm); summation of R (mm) was obtained by adding R of each period; mean RH (RHm; RH%) was calculated as the mean of the RH for each period.
SPSS software (IBM SPSS Statistics v. 24) was used for the data analysis. Analysis of variance (ANOVA) was applied to arcsine-transformed data on fungi incidence in hazelnut kernels. Tukey’s test was used to indicate statistically significant differences between mean values. Pearson correlation analysis was run between the incidence of defective hazelnuts, assessed on 3,000 hazelnuts per orchard at full ripening, and the incidence of fungi at the same sampling time, so as between the incidence of defective nuts and meteorological parameters computed for the four time-periods.
Results
Sampling and Meteorological Data
Early ripening sampling occurred on July 15–20, while the full ripening sampling was performed on August 5–10.
Similar values of Tm were recorded for the same period in the different Turkish provinces, but Sakarya and Samsun commonly had Tm that was lower and Trabzon higher than the mean (Table 3). In particular, during the time period P1, a minimum DD of 691 was recorded in Sakarya, and a maximum DD of 1041 was recorded in Trabzon. For the time period P2, the minimum DD was recorded in Samsun and the maximum in Zonguldak, with values of 934 and 1077 DD, respectively, while for the time periods P3 and P4, the minimum DD was recorded in Samsun and maximum in Trabzon, with values ranging from 632 to 723 DD in the third period and from 636 to 763 DD in P4. Further, only 70 mm of rainfall were recorded during P1 in Giresun, while more than 150 mm were recorded in all the other studied locations during the same time period, with a maximum of 282 mm in Düzce. Giresun was the province with the most abundant rainfall during the entire considered period, with ΣR of 793 mm versus a minimum of 290 mm recorded in Zonguldak.
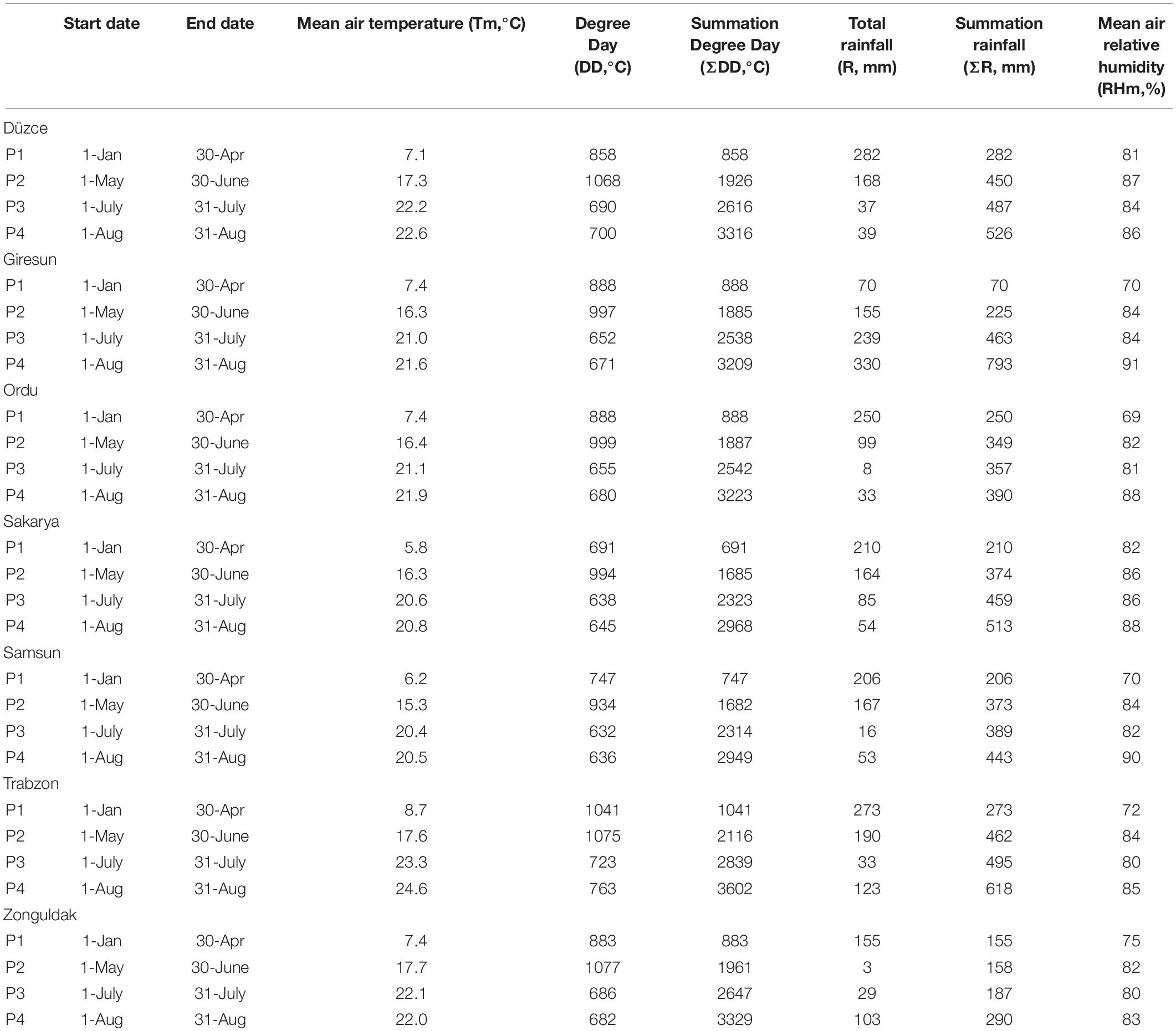
Table 3. Summary of meteorological data collected during 4 periods (P1-P4) from 1st January to 30th August in different Turkish provinces (Düzce, Giresun, Ordu, Sakarya, Samsun, Trabzon, and Zonguldak) in 2017.
Fungal Isolation and Identification
The results of fungal isolation are based on the analysis of 3,780 half-cut nuts and are reported here as incidence (%) of hazelnuts infected by each genus. Different fungi were isolated from plated half kernels; the prevalent fungi were Alternaria, Aspergillus, Botryosphaeria, Diaporthe, Fusarium, Penicillium, and Pestalotiopsis. Furthermore, Rhizopus, Cladosporium, Trichoderma, Botrytis, Trichothecium, and Mucor were also occasionally isolated, but always with incidence lower than 10%.
The ANOVA was run considering fungal incidence data collected during 2 sampling dates (early and full ripening) and in 7 Turkish provinces (Düzce, Giresun, Ordu, Sakarya, Samsun, Trabzon, and Zonguldak). Hazelnut growth stage at sampling significantly affected the incidence of Alternaria, Botryosphaeria, Diaporthe, and Fusarium. In particular, the highest incidence of Alternaria, Botryosphaeria, Diaporthe, and Fusarium was observed at full ripening. Penicillium was the most isolated genus, followed by Diaporthe. Regarding the role of geographic areas, for Alternaria spp. significant differences were only noticed between Giresun (7.2%), Trabzon (1.1%), and Ordu (2.2%). The highest incidence of Botryosphaeria was observed in Düzce, Sakarya, Samsun, and Zonguldak (mean 11.2%). The hazelnut growing province with the highest incidence of Diaporthe spp. was Samsun (26.1%), which was not significantly different from that of Giresun (19.3%). The highest incidence of Fusarium was observed in Düzce (21.3%), which was significantly different from that of Giresun (4.4%), Ordu (2.0%), and Samsun (6.5%). Penicillium incidence ranged between 68% in Ordu and 24.4% in Zonguldak. The impact of the sampling place was not significant for Aspergillus and Pestalotiopsis spp. Furthermore, the interaction between factors significantly affected the incidence of isolated fungi except for Aspergillus and Pestalotiopsis spp. (Table 4). Minor differences were noticed compared to the impact of main factors. Regarding Alternaria, no increase was observed in Düzce and Trabzon between early and full ripening, while it was reported for the main factor “location”; similarly, Fusarium spp. decreased in Giresun, Samsun and Trabzon from early to full ripening showing an opposite behavior compared to the main factor “location”. On the contrary, Penicillium commonly decreased from early to full ripening, except in Sakarya, Trabzon and Zonguldak (Figure 2).
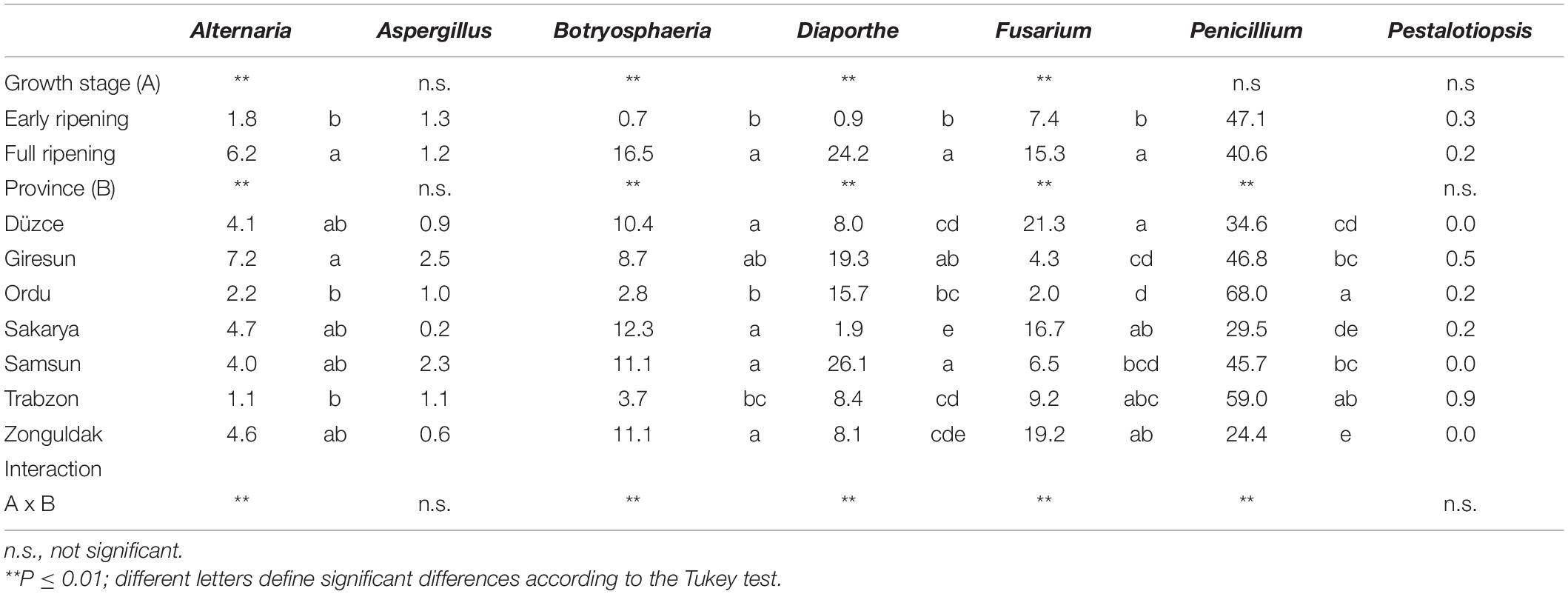
Table 4. Mean incidence of the most isolated fungal genera in hazelnuts sampled at early and full ripening in 7 Turkish provinces (Düzce, Giresun, Ordu, Sakarya, Samsun, Trabzon and Zonguldak). Growth stage and province were considered as factors in ANOVA.
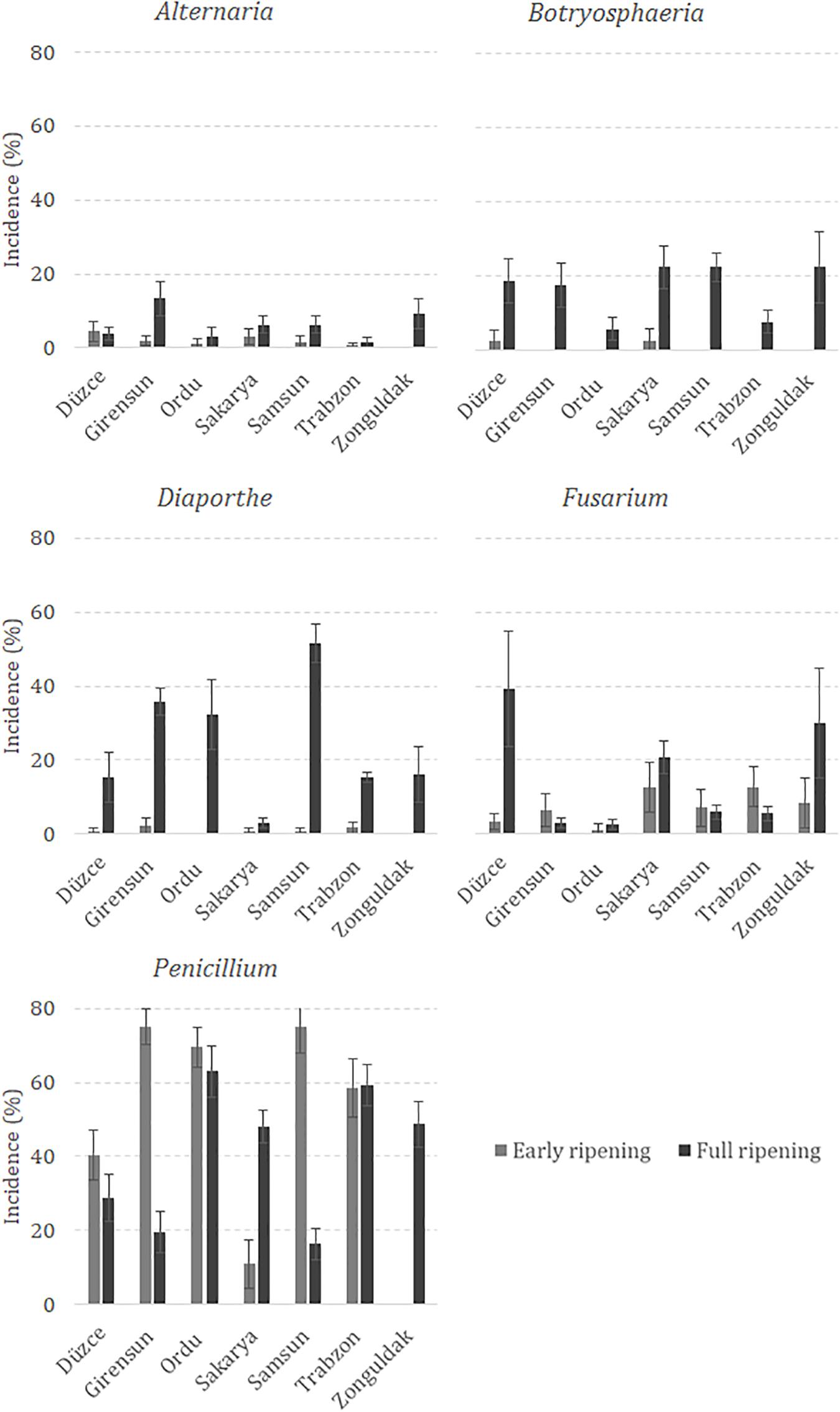
Figure 2. Mean incidence of Alternaria, Botryosphaeria, Diaporthe, Fusarium and Penicillium isolated from hazelnuts sampled in Düzce, Giresun, Ordu, Sakarya, Samsun, Trabzon and Zonguldak at early and full ripening.
Regarding the incidence of defective hazelnuts, at early ripening, the total defects were ≤0.5%, while at full ripening, they ranged between 0.3 and 4.1%. At full ripening, differences were noted between the defect incidence in East (mean 3.1%) and West (mean 0.4%) provinces, with 3, 2.3, 4.1, and 3.1% incidence observed in Giresun, Ordu, Samsun, and Trabzon, respectively (East Turkey) versus 0.6, 0.3, and 0.2% incidence scored in Düzce, Sakarya, and Zonguldak, respectively (West Turkey). The incidence of internal defects, observed after nuts are half-cut, contributed between 15 and 45% to total defects.
Pearson correlation analysis, run between the incidence of defective hazelnuts and of fungi at full ripening, proved Diaporthe as the only genus positively correlated with internal (ρ = 0.86, P ≤ 0.01) and visible defects (ρ = 0.81, P ≤ 0.05, Table 5). Negative correlation was recorded for Fusarium (ρ = −0.81 and ρ = −0.82, P ≤ 0.05 for internal and visible defects, respectively). Although not significant (P ≥ 0.05), positive related trend with both internal (ρ = 0.32) and visible (ρ = 0.46) defects was found for Aspergillus (Table 5). Regarding meteorological parameters, no significant correlation was found, neither for defects nor for fungi incidence.
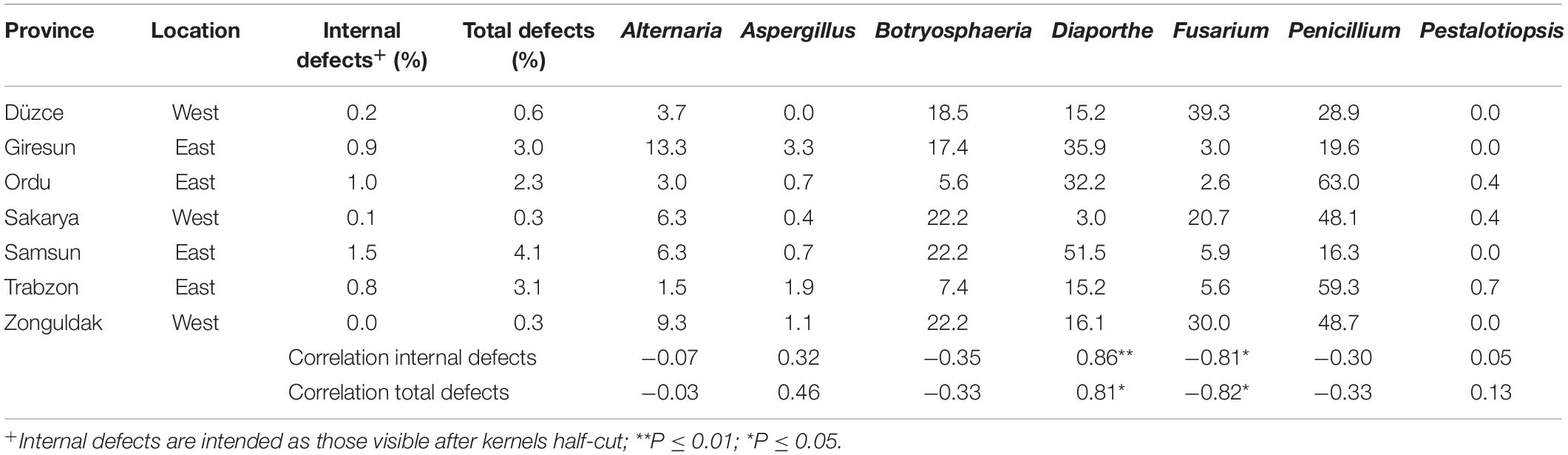
Table 5. Pearson correlation analysis, run between incidence of hazelnuts defects (internal and total) and incidence of fungi at full ripening.
Diaporthe Phylogenetic Analysis
In order to reach sub-genus identification of the Diaporthe strains isolated from Turkish, Caucasian, and Italian hazelnuts, phylogenetic analysis was first performed against a set of 280 Diaporthe spp. strains selected from the literature (Supplementary Table 1) and representing a comprehensive overview of this genus. As shown in Supplementary Figure 1, the phylogeny is highly complex, with genetic distances (branch lengths, not shown) and bootstrap support values being generally low, which is the reason why it was difficult to establish clades or species complexes in this dataset.
In order to reduce the dataset complexity and improve grouping support, a second phylogenetic tree was constructed using a set of closely related reference strains that were selected based on the grouping patterns observed in the broader phylogenetic analysis. In this case (Figure 3), it was possible to define 7 well-supported clades (bootstrap values ≥70%; Bayesian posterior probability ≥95%). Two of them, clade I and III, included 91.2% of the total number of strains, with geographic origin being a factor supporting the diversity observed. Clade I contained 76% of the total number of analyzed strains and was mainly composed of Turkish strains (82 out of 95 in the clade), with 9 Caucasian and 4 Italian strains. However, clade III included 15.2% of the analyzed samples and was mainly composed of Caucasian strains (18 out of 19) and one from Turkey. The remaining clades are most likely to represent occasional and opportunistic species.
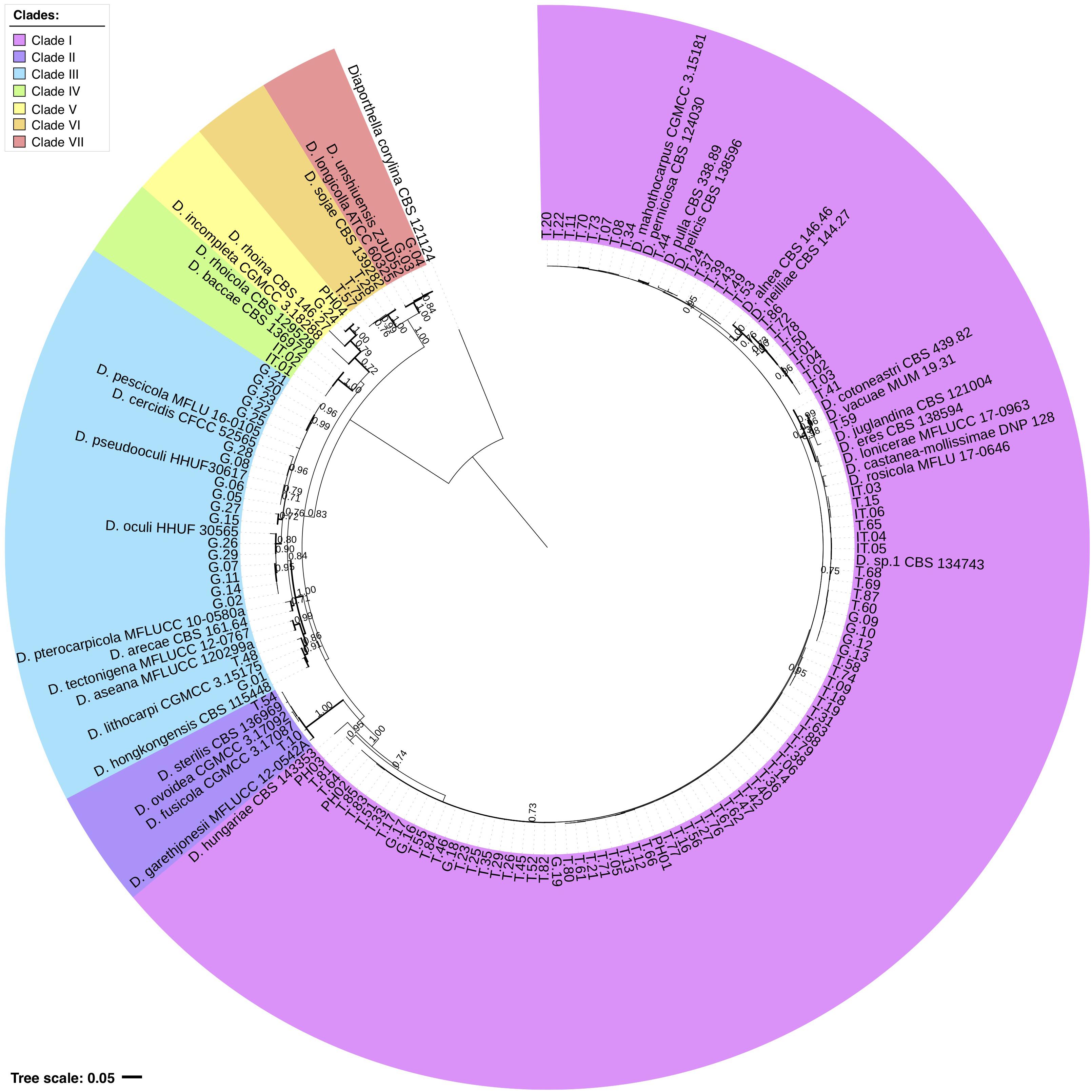
Figure 3. Combined phylogeny for the ITS, EF1-α, and tub sequence data of the 125 strains isolated from hazelnuts with other Diaporthe strains detailed in Supplementary Table 1. Diaporthella corylina CBS 121124T was used as the outgroup. Selected model: TN93 + G + I. The percentage of trees in which the associated taxa cluster together in the bootstrap test (1,000 replicates) is shown above the branches. Bold branches are supported with ≥0.95 Bayesian posterior probability. The tree is drawn to scale with branch lengths measured in the number of substitutions per site. All positions with less than 95% site coverage were eliminated. The final dataset included 162 nucleotide sequences and a total of 981 positions. The tree branch topology is shown in more detail in Supplementary Figure 2.
Discussion
Because of kernel defects, hazelnut yield loss and market value decrease are observed worldwide, and studies with the aim of understanding the origin of such defects are crucial (Garrone and Vacchetti, 1994). Several Diaporthe species have been reported as causing diseases in different types of nuts (Osmonalieva et al., 2000; Teviotdale et al., 2002; Rhouma et al., 2008; Diogo et al., 2010; Gramaje et al., 2012; Chen and Morgan, 2014; Fan et al., 2015, 2018; Annesi et al., 2016; Gao et al., 2017; Pscheidt and Ocamb, 2017; Yang et al., 2018; Eichmeier et al., 2020), including hazelnuts (Guerrero and Pérez, 2013; Guerrero et al., 2014, 2019), but only very recently, a comprehensive four-year study performed in the Caucasus region confirmed the role of this genus in causing external brown spots on nuts and also those observed after kernel half-cut (Battilani et al., 2018). In the present study, fungi associated with defective hazelnuts from Turkey, the leading hazelnut producer worldwide, were investigated to eventually confirm these results.
Several fungal genera were isolated in this survey; they may have been influenced by the hazelnut varieties, as previously stated for D. rudis (Pscheidt et al., 2019), but this factor cannot be discussed in this study because a mix of different hazelnut varieties were grown in the sampled orchards. Among the isolated fungi, the key role of Diaporthe spp. in hazelnut defects was confirmed by the observed significant differences of its incidence in kernels produced in the considered Turkish provinces. Furthermore, Diaporthe spp. is the only fungal genus positively correlated with hazelnut defects, both those on the surface and those visible after kernel half-cut. The latter are of particular interest from a commercial point of view, as internal defects cannot be found using optical sorters. Therefore, it is not easy to discharge kernels with internal defects from the commercial product. Moreover, Aspergillus spp. was positively related to hazelnut defects, even if not significantly. This should be studied deeper in order to find out eventual interaction between Diaporthe and related defects with Aspergillus; this genus includes well-known fungi that can lead to severe economical and health impacts due to mycotoxin production (Ozay et al., 2008; Kabak, 2016; Houshyarfard and Javadi, 2018). The most isolated genus was Penicillium, in agreement with Pscheidt et al. (2019), but relations between this fungus and defects were not found. Nevertheless, Penicillium also includes mycotoxin producing fungi and merit to be studied deeper for possible health issue implications.
The highest incidence of Diaporthe spp. was recorded in the provinces located in Eastern Turkey, where the highest incidence of defects was also registered. In particular, the incidence at full ripening was the highest in Samsun (51.5%), the province where the highest incidence of total defects in nuts (4.1%) was observed. The lowest incidence of Diaporthe was recorded in Western Turkey provinces (mean 11.4%), with the lowest total defect incidence (mean 0.4%). Diaporthe spp. incidence increased from early to full ripening, in agreement with a previous study (Battilani et al., 2018), and that closely corresponds to the increase in observed defects that occurs during the hazelnut ripening stage. However, defect incidence was comparable with the data from years of low defect incidence reported for Caucasian hazelnuts (incidence of 1.1–3.3%), while up to 14.3% incidence was reported in 2016 (Battilani et al., 2018). Further, the correlation with rainfall was not confirmed. The lower incidence of defective nuts compared to the Caucasian hazelnuts and the limited variation between orchards (0.25–4.13%) was insufficient to highlight the role of this or other meteorological parameters.
Although this study aimed to realize identification at the species level for the in-depth characterization of Diaporthe, this was not possible for a majority of species. As discussed by Fan et al. (2018), increased numbers of species in the alignment led to reduced accuracy in species separation, an effect that is partially dependent on the number of analyzed loci. Here, only 4 strains could be confidently grouped with two known species (D. unshiuensis and D. pseudooculi). The remaining strains were either grouped with more than one reference strain or formed clusters (with different degrees of branching support) with no known species included. Based on the phylogenetic analysis results (Figure 3), the 125 hazelnut strains were grouped into 7 clades, and some included members of known species complexes. Clade I included type strains of the D. eres species complex (D. alnea, D. eres, D. helicis, D neilliae, and D. pulla), and clades VI and VII included species from the D. sojae species complex (D. longicolla and D. sojae).
It is interesting to note that several Caucasian strains in clade III were related to D. oculi and D. pseudooculi, which are species that were initially described as human pathogens and are phylogenetically related to the D. arecae species complex (Ozawa et al., 2019). Clade III includes D. arecae and D. hongkongensis, which are also members of the D. arecae species complex (Long et al., 2019). Despite the limited power of our dataset, it is interesting to see that the sample grouping and relation to known species is mostly maintained in both the extended (Supplementary Figure 1) and reduced (Figure 3) analysis. This is of particular importance in clades I and III, as they include a large number of strains that cannot be classified at the species level, and some of them are possible taxonomic novelty candidates.
When comparing the hazelnut taxonomic composition with that of other nuts (mainly walnuts and almonds), it is interesting to see that each study reports slightly different Diaporthe species present in the considered matrix, with D. rostrata, D. amygdali, and D. eres being more recurrent. D. foeniculina has been reported in sweet chestnuts from Italy and hazelnut kernels from Chile (Annesi et al., 2016; Guerrero et al., 2019), D. rudis in hazelnut kernels from Oregon, United States (Pscheidt et al., 2019), and D. australafricana from Chilean hazelnuts (Guerrero and Pérez, 2013); among these species, only D. eres was found to be related to the hazelnut strains studied here.
The observation of poorly supported or non-monophyletic clades is a common situation within Diaporthe, probably due to ITS heterogeneity within species complexes (Udayanga et al., 2014b; Huang et al., 2015; Guarnaccia et al., 2018). This situation can be improved if a multi-locus sequences analysis approach is considered, particularly with the inclusion of EF1-α or tub gene regions (Udayanga et al., 2012a), as applied in this study. Therefore, species and species complex limits need to be carefully defined, and for that reason, no species-level classifications were performed at this point. Recent studies have successfully identified new species based on the same three loci used here (ITS, EF1-α, and tub) (Lesuthu et al., 2019; Zapata et al., 2020). Nevertheless, some studies used four or more loci (Udayanga et al., 2014a, b, 2015; Zhou and Hou, 2019), and a study from Santos et al. (2017a) showed that the most optimal Diaporthe species separation occurred when five loci were simultaneously used.
In conclusion, the present study shows that despite the heterogeneous nature of the hazelnut cultivable mycobiota, Diaporthe spp. are the only fungal species strongly associated with both internal and external defects in hazelnut kernels. A comparison of strains from different geographic origins showed that different species were responsible for similar symptoms (clade I versus clade III). Finally, a majority of Turkish Diaporthe strains are related to D. eres, a well-known plant pathogen that has been previously reported in C. avellana. Future studies focusing on improved molecular-based species classification, particularly of those strains belonging to clades I and III, will prove to be valuable to clarify their role as causative agents of hazelnut defects, assist with developing control strategies, and increase the quality and quantity of available product that meets market requirements.
Data Availability Statement
The datasets presented in this study can be found in online repositories. The names of the repository/repositories and accession number(s) can be found below: https://www.ncbi.nlm.nih.gov/search/all/?term=, MT613733–MT613857; https://www.ncbi.nlm.nih.gov/search/all/?term=, MT849830–MT849954; https://www.ncbi.nlm.nih.gov/search/all/?term=, MT840703–MT840825.
Author Contributions
PB: conceptualization and supervision. PB, RA, GCh, NL, and CSa: experiment design. RA, CSa, and CSo: experiment management. PB, RA, NL, and CSa: data analysis. CSa and RA: phylogenetic analysis. RA and CSa: original manuscript preparation. PB, GCh, NL, GCa, NS, and CSo: review and editing. PB, GCh, NL, GCa, and NS: funding acquisition. All authors contributed to the article and approved the submitted version.
Funding
This research was partially funded by the Portuguese Foundation for Science and Technology (FCT) under the scope of the strategic funding of the UIDB/04469/2020 unit and BioTecNorte operation (NORTE-01-0145-FEDER-000004) funded by the European Regional Development Fund under the scope of Norte2020-Programa Operacional Regional do Norte.
Conflict of Interest
GCa and NS were employed by the company Soremartec Italia S.r.l.
The remaining authors declare that the research was conducted in the absence of any commercial or financial relationships that could be construed as a potential conflict of interest.
Acknowledgments
RA carried out this work within the Ph.D. school Agrisystem of Università Cattolica del Sacro Cuore (Italy), and she was partially supported by Fondazione Romeo ed Enrica Invernizzi.
Supplementary Material
The Supplementary Material for this article can be found online at: https://www.frontiersin.org/articles/10.3389/fpls.2020.611655/full#supplementary-material
Supplementary Figure 1 | Tree branch topology representation of the combined phylogeny for ITS, EF1-α, and tub sequence data of the 125 strains isolated from hazelnuts with other Diaporthe strains selected from the literature and detailed in Supplementary Table 1. Diaporthella corylina CBS 121124T was used as the outgroup. Selected model: TN93 + G + I. The percentage of trees in which the associated taxa cluster together in the bootstrap test (1,000 replicates) is shown above the branches. All positions with less than 90% site coverage were eliminated. The final dataset included 406 nucleotide sequences and a total of 684 positions.
Supplementary Figure 2 | Tree branch topology representation of the tree presented in Figure 3. Combined phylogeny for ITS, EF1-α, and tub sequence data of the 125 strains isolated from hazelnuts with other Diaporthe strains selected from the results presented in Supplementary Figure 1 and detailed in Supplementary Table 1. Diaporthella corylina CBS 121124T was used as the outgroup. Selected model: TN93 + G + I. The percentage of trees in which the associated taxa cluster together in the bootstrap test (1,000 replicates) is shown above the branches. Bold branches are supported with ≥0.95 Bayesian posterior probability. All positions with less than 95% site coverage were eliminated. The final dataset included 162 nucleotide sequences and a total of 981 positions.
Supplementary Table 1 | List of strains used for the phylogenetic analyses with the corresponding ITS, EF1-α, and tub GenBank sequence accession numbers. The list includes a set of Diaporthe species selected from the literature and the 125 hazelnut strains amplified in this study.
Footnotes
- ^ http://www.statista.com
- ^ http://www.fao.org/3/x4484e/x4484e03.htm
- ^ https://www.unece.org
- ^ www.fao.org
References
Annesi, T., Luongo, L., Vitale, S., Galli, M., and Belisario, A. (2016). Characterization and pathogenicity of Phomopsis theicola anamorph of Diaporthe foeniculina causing stem and shoot cankers on sweet chestnut in Italy. J. Phytopathol. 164, 412–416. doi: 10.1111/jph.12426
Battilani, P., Chiusa, G., Arciuolo, R., Somenzi, M., Fontana, M., Castello, G., et al. (2018). Diaporthe as the main cause of hazelnut defects in the Caucasus region. Phytopathol. Mediterr. 57, 320–333. doi: 10.14601/Phytopathol_Mediterr-22872
Carbone, I., and Kohn, L. M. (1999). A method for designing primer sets for speciation studies in filamentous ascomycetes. Mycologia 91, 553–556. doi: 10.1080/00275514.1999.12061051
Castresana, J. (2000). Selection of conserved blocks from multiple alignments for their use in phylogenetic analysis. Mol. Biol. Evol. 17, 540–552. doi: 10.1093/oxfordjournals.molbev.a026334
Chen, S. F., and Morgan, D. P. (2014). Phylogeny, morphology, distribution, and pathogenicity of Botryosphaeriaceae and Diaporthaceae from English walnut in California. Plant Dis. 98, 636–652. doi: 10.1094/PDIS-07-13-0706-RE
Chepkirui, C., and Stadler, M. (2017). The genus Diaporthe: a rich source of diverse and bioactive metabolites. Mycol. Prog. 16, 477–494. doi: 10.1007/s11557-017-1288-y
Crous, P. W. (2005). Impact of molecular phylogenetics on the taxonomy and diagnostics of fungi. EPPO Bull. 35, 47–51. doi: 10.1111/j.1365-2338.2005.00811.x
Dettman, J. R., Jacobson, D. J., Turner, E., Pringle, A., and Taylor, J. W. (2003). Reproductive isolation and phylogenetic divergence in Neurospora: comparing methods of species recognition in a model eukaryote. Evolution 57, 2721–2741. doi: 10.1554/03-074
Diogo, E. L. F., Santos, J. M., and Phillips, A. J. L. (2010). Phylogeny, morphology and pathogenicity of Diaporthe and Phomopsis species on almond in Portugal. Fungal Divers. 44, 107–115. doi: 10.1007/s13225-010-0057-x
Dissanayake, A., Camporesi, E., Hyde, K. D., Zhang, W., Yan, J. Y., and Li, X. H. (2017a). Molecular phylogenetic analysis reveals seven new Diaporthe species from Italy. Mycosphere 8, 853–877. doi: 10.5943/mycosphere/8/5/4
Dissanayake, A. J., Phillips, A. J. L., Hyde, K. D., Yan, J. Y., and Li, X. H. (2017b). The current status of species in Diaporthe. Mycosphere 8, 1106–1156. doi: 10.5943/MYCOSPHERE/8/5/5
Edgar, R. C. (2004). MUSCLE: multiple sequence alignment with high accuracy and high throughput. Nucleic Acids Res. 32, 1792–1797. doi: 10.1093/nar/gkh340
Eichmeier, A., Pecenka, J., Spetik, M., Necas, T., Ondrasek, I., Armengol, J., et al. (2020). Fungal trunk pathogens associated with Juglans regia in the Czech Republic. Plant Dis. 104, 761–771. doi: 10.1094/PDIS-06-19-1308-RE
Fan, X. L., Hyde, K. D., Udayanga, D., Wu, X. Y., and Tian, C. M. (2015). Diaporthe rostrata, a novel ascomycete from Juglans mandshurica associated with walnut dieback. Mycol. Prog. 14:82.
Fan, X. L., Yang, Q., Bezerra, J. D. P., Alvarez, L. V., and Tian, C. M. (2018). Diaporthe from walnut tree (Juglans regia) in China, with insight of the Diaporthe eres complex. Mycol. Prog. 17, 841–853. doi: 10.1007/s11557-018-1395-4
Farr, D. F., Castlebury, L. A., and Rossman, A. Y. (2002a). Morphological and molecular characterization of Phomopsis vaccinii and additional isolates of Phomopsis from blueberry and cranberry in the eastern United States. Mycologia 94, 494–504. doi: 10.1080/15572536.2003.11833214
Farr, D. F., Castlebury, L. A., Rossman, A. Y., and Putnam, M. L. (2002b). A new species of Phomopsis causing twig dieback of Vaccinium vitis-idaea (lingonberry). Mycol. Res. 106, 745–752. doi: 10.1017/S095375620200583X
Felsenstein, J. (1985). Confidence limits on phylogenies: an approach using the bootstrap. Evolution 39, 783–791. doi: 10.2307/2408678
Ferreira, M. C., Vieira, M. de L. A., Zani, C. L., Alves, T. M. de A., Sales, P., Murta, S. M. F., et al. (2015). Molecular phylogeny, diversity, symbiosis and discover of bioactive compounds of endophytic fungi associated with the medicinal Amazonian plant Carapa guianensis Aublet (Meliaceae). Biochem. Syst. Ecol. 59, 36–44. doi: 10.1016/j.bse.2014.12.017
Gao, Y., Liu, F., Duan, W., Crous, P. W., and Cai, L. (2017). Diaporthe is paraphyletic. IMA Fungus 8, 153–187. doi: 10.5598/imafungus.2017.08.01.11
Garrone, W., and Vacchetti, M. (1994). La qualita’ delle nocciole in rapporto alle esigenze dell’industria dolciaria utilizzatrice [Hazelnut quality based on requests of confetionary industry]. IIIrd International Congress on Hazelnut. Acta Hortic. 351, 641–647. doi: 10.17660/actahortic.1994.351.71
Glass, N. L., and Donaldson, G. C. (1995). Development of primer sets designed for use with the PCR to amplify conserved genes from filamentous ascomycetes. Appl. Environ. Microbiol. 61, 1323–1330. doi: 10.1128/aem.61.4.1323-1330.1995
Gomes, R. R., Glienke, C., Videira, S. I. R., Lombard, L., Groenewald, J. Z., and Crous, P. W. (2013). Diaporthe: a genus of endophytic, saprobic and plant pathogenic fungi. Persoonia 31, 1–41. doi: 10.3767/003158513x666844
Gramaje, D., Agustí-Brisach, C., Pérez-Sierra, A., Moralejo, E., Olmo, D., Mostert, L., et al. (2012). Fungal trunk pathogens associated with wood decay of almond trees on Mallorca (Spain). Persoonia 28, 1–13. doi: 10.3767/003158512X626155
Guarnaccia, V., and Crous, P. W. (2017). Emerging citrus diseases in Europe caused by species of Diaporthe. IMA Fungus 8, 317–334. doi: 10.5598/imafungus.2017.08.02.07
Guarnaccia, V., Groenewald, J. Z., Woodhall, J., Armengol, J., Cinelli, T., Eichmeier, A., et al. (2018). Diaporthe diversity and pathogenicity revealed from a broad survey of grapevine diseases in Europe. Persoonia 40, 135–153. doi: 10.3767/persoonia.2018.40.06
Guarnaccia, V., Vitale, A., Cirvilleri, G., Aiello, D., Susca, A., Epifani, F., et al. (2016). Characterisation and pathogenicity of fungal species associated with branch cankers and stem-end rot of avocado in Italy. Eur. J. Plant Pathol. 146, 963–976. doi: 10.1007/s10658-016-0973-z
Guerrero, J., Galdames, R. E., and Perez, S. (2019). First report of Diaporthe foeniculina causing black tip and necrotic spot on hazelnut kernel in Chile. Plant Dis. 104:975. doi: 10.1094/PDIS-06-19-1166-PDN
Guerrero, J., and Pérez, S. (2013). First report of Diaporthe australafricana-caused stem canker and dieback in European hazelnut (Corylus avellana L.) in Chile. Plant Dis. 97:1657. doi: 10.1094/PDIS-03-13-0286-PDN
Guerrero, J. C., Pérez, S. F., Ferrada, E. Q., Cona, L. Q., and Bensch, E. T. (2014). Phytopathogens of hazelnut (Corylus avellana L.) in southern Chile. Acta Hortic. 1052, 269–274. doi: 10.17660/actahortic.2014.1052.36
Hall, T. A. (1999). BioEdit: a user-friendly biological sequence alignment editor and analysis program for windows 95/98/NT. Nucleic Acids Symp. Ser. 41, 95–98.
Hilário, S., Amaral, I. A., Gonçalves, M. F. M., Lopes, A., Santos, L., and Alves, A. (2020). Diaporthe species associated with twig blight and dieback of Vaccinium corymbosum in Portugal, with description of four new species. Mycologia 112, 293–308. doi: 10.1080/00275514.2019.1698926
Houshyarfard, M., and Javadi, D. (2018). The Aspergillus flavus susceptibility of hazelnut varieties (Corylus avellana L.) in laboratory conditions. J. Nuts 9, 181–188.
Huang, F., Udayanga, D., Wang, X., Hou, X., Mei, X., Fu, Y., et al. (2015). Endophytic Diaporthe associated with Citrus: a phylogenetic reassessment with seven new species from China. Fungal Biol. 119, 331–347. doi: 10.1016/j.funbio.2015.02.006
Islam, A. (2018). Hazelnut cultivation in Turkey. Akad. Ziraat Derg. 7, 259–266. doi: 10.29278/azd.476665
Kabak, B. (2016). Aflatoxins in hazelnuts and dried figs: occurrence and exposure assessment. Food Chem. 211, 8–16. doi: 10.1016/j.foodchem.2016.04.141
Krol, E. (2005). Identification and differentiation of Phomopsis spp. isolates from grapevine and some other plant. Phytopathol. Pol. 35, 151–156.
Kumar, S., Stecher, G., Li, M., Knyaz, C., and Tamura, K. (2018). MEGA X: molecular evolutionary genetics analysis across computing platforms. Mol. Biol. Evol. 35, 1547–1549. doi: 10.1093/molbev/msy096
Leslie, J. F., and Summerell, B. A. (2007). The Fusarium Laboratory Manual. Hoboken, NJ: Blackwell Publishing, 1–388. doi: 10.1002/9780470278376
Lesuthu, P., Mostert, L., Spies, C. F. J., Moyo, P., Regnier, T., and Halleen, F. (2019). Diaporthe nebulae sp. nov. and first report of D. cynaroidis, D. novem, and D. serafiniae on grapevines in South Africa. Plant Dis. 103, 808–817. doi: 10.1094/PDIS-03-18-0433-RE
Letunic, I., and Bork, P. (2019). Interactive tree of life (iTOL) v4: recent updates and new developments. Nucleic Acids Res. 47, 256–259. doi: 10.1093/nar/gkz239
Lombard, L., van Leeuwen, G. C. M., Guarnaccia, V., Polizzi, G., van Rijswick, P. C. J., Rosendahl, K., et al. (2014). Diaporthe species associated with Vaccinium, with specific reference to Europe. Phytopathol. Mediterr. 53, 287–299. doi: 10.14601/Phytopathol_Mediterr-14034
Long, H., Zhang, Q., Hao, Y. Y., Shao, X. Q., Wei, X. X., Hyde, K. D., et al. (2019). Diaporthe species in south-western China. MycoKeys 57, 113–127. doi: 10.3897/mycokeys.57.35448
Luongo, L., Santori, A., Riccioni, L., and Belisario, A. (2011). Phomopsis sp. associated with post-harvest fruit rot of kiwifruit in Italy. J. Plant Pathol. 93, 205–210. doi: 10.1400/169617
Maharachchikumbura, S. S. N., Hyde, K. D., Groenewald, J. Z., Xu, J., and Crous, P. W. (2014). Pestalotiopsis revisited. Stud. Mycol. 79, 121–186. doi: 10.1016/j.simyco.2014.09.005
Manawasinghe, I. S., Dissanayake, A. J., Li, X., Liu, M., Wanasinghe, D. N., Xu, J., et al. (2019). High genetic diversity and species complexity of Diaporthe associated with grapevine dieback in China. Front. Microbiol. 10:1936. doi: 10.3389/fmicb.2019.01936
Marin-Felix, Y., Hernández-Restrepo, M., Wingfield, M. J., Akulov, A., Carnegie, A. J., Cheewangkoon, R., et al. (2019). Genera of phytopathogenic fungi: GOPHY 2. Stud. Mycol. 92, 47–133. doi: 10.1016/j.simyco.2018.04.002
Mostert, L., Crous, P. W., Kang, J. C., and Phillips, A. J. L. (2001). Species of Phomopsis and a Libertella sp. occurring on grapevines with specific reference to South Africa: morphological, cultural, molecular and pathological characterization. Mycologia 93, 146–167. doi: 10.1080/00275514.2001.12061286
Nylander, J. A. A. (2004). MrModeltest v2. Program Distributed by the Author. Uppsala: Evolutionary Biology Centre, Uppsala University.
Osmonalieva, A., McNeill, D. L., Stewart, A., Klinac, D. J., and Wadia, K. D. R. (2000). Phomopsis castanea infection in chestnuts from Canterbury, New Zealand. Agron. N. Z. Proc. 30, 29–35.
Ozawa, K., Mochizuki, K., Takagi, D., Ishida, K., Sunada, A., Ohkusu, K., et al. (2019). Identification and antifungal sensitivity of two new species of Diaporthe isolated. J. Infect. Chemother. 25, 96–103. doi: 10.1016/j.jiac.2018.10.008
Ozay, G., Seyhan, F., Pembeci, C., Saklar, S., and Yilmaz, A. (2008). Factors influencing fungal and aflatoxin levels in Turkish hazelnuts (Corylus avellana L.) during growth, harvest, drying and storage: a 3-year study. Food Addit. Contam. 25, 209–218. doi: 10.1080/02652030701711016
Pitt, J. I. (1979). The Genus Penicillium and its Teleomorphic States Eupenicillium and Talaromyces. New York, NY: Academic Press.
Pscheidt, J. W., Heckert, S., Wiseman, M., and Jones, L. (2019). Fungi associated with and influence of moisture on development of kernel mold of hazelnut. Plant Dis. 103, 922–928. doi: 10.1094/PDIS-09-18-1520-RE
Pscheidt, J. W., and Ocamb, C. M. (2017). Pacific Northwest Plant Disease Management Handbook [online]. Corvallis, OR: Oregon State University, 904.
Rehner, S. A., and Uecker, F. A. (1994). Nuclear ribosomal internal transcribed spacer phylogeny and host diversity in the coelomycete Phomopsis. Can. J. Bot. 72, 1666–1674. doi: 10.1139/b94-204
Rhouma, A., Triki, M. A., Ouerteni, K., and Mezghanni, M. (2008). Chemical and biological control of Phomopsis amygdali the causal agent of constriction canker of almond in Tunisia. Tunis. J. Plant Prot. 3, 69–78.
Rodrigues, P., Venâncio, A., Kozakiewicz, Z., and Lima, N. (2009). A polyphasic approach to the identification of aflatoxigenic and non-aflatoxigenic strains of Aspergillus section Flavi isolated from Portuguese almonds. Int. J. Food Microbiol. 129, 187–193. doi: 10.1016/j.ijfoodmicro.2008.11.023
Ronquist, F., Teslenko, M., Van Der Mark, P., Ayres, D. L., Darling, A., Höhna, S., et al. (2012). Mrbayes 3.2: efficient bayesian phylogenetic inference and model choice across a large model space. Syst. Biol. 61, 539–542. doi: 10.1093/sysbio/sys029
Rotem, J. (1994). The Genus Alternaria: Biology, Epidemiology, and Pathogenicity. St. Paul, MN: APS Press.
Samson, R. A., Visagie, C. M., Houbraken, J., Hong, S. B., Hubka, V., Klaassen, C. H. W., et al. (2014). Phylogeny, identification and nomenclature of the genus Aspergillus. Stud. Mycol. 78, 141–173. doi: 10.1016/j.simyco.2014.07.004
Santos, J. M., Correia, V. G., and Phillips, A. J. L. (2010). Primers for mating-type diagnosis in Diaporthe and Phomopsis: their use in teleomorph induction in vitro and biological species definition. Fungal Biol. 114, 255–270. doi: 10.1016/j.funbio.2010.01.007
Santos, J. M., and Phillips, A. (2009). Resolving the complex of Diaporthe (Phomopsis) species occurring on Foeniculum vulgare in Portugal. Fungal Divers. 34, 111–125.
Santos, L., Alves, A., and Alves, R. (2017a). Evaluating multi-locus phylogenies for species boundaries determination in the genus Diaporthe. PeerJ 5:e3120. doi: 10.7717/peerj.3120
Santos, L., Phillips, A. J. L., Crous, P. W., and Alves, A. (2017b). Diaporthe species on Rosaceae with descriptions of D. pyracanthae sp. nov. and D. malorum sp. nov. Mycosphere 8, 485–512. doi: 10.5943/mycosphere/8/5/2
Sutton, B. C. (1980). The Coelomycetes. Fungi Imperfecti with Pycnidia Acervuli and Stromata. Surrey: Commonwealth Mycological Institute.
Tamura, K., and Nei, M. (1993). Estimation of the number of nucleotide substitutions in the control region of mitochondrial DNA in humans and chimpanzees. Mol. Biol. Evol. 10, 512–526. doi: 10.1093/oxfordjournals.molbev.a040023
Taylor, J. W., Jacobson, D. J., Kroken, S., Kasuga, T., Geiser, D. M., Hibbett, D. S., et al. (2000). Phylogenetic species recognition and species concepts in fungi. Fungal Genet. Biol. 31, 21–32. doi: 10.1006/fgbi.2000.1228
Teviotdale, B. L., Themis, J. M., and Pscheidt, J. W. (2002). Compendium of Nut Crop Diseases in Temperate Zones. St. Paul, MN: APS Press.
Thomidis, T., Exadaktylou, E., and Chen, S. F. (2013). Diaporthe neotheicola, a new threat for kiwifruit in Greece. Crop Prot. 47, 35–40. doi: 10.1016/j.cropro.2012.12.024
Udayanga, D., Castlebury, L. A., Rossman, A. Y., Chukeatirote, E., and Hyde, K. D. (2014a). Insights into the genus Diaporthe: phylogenetic species delimitation in the D. eres species complex. Fungal Divers. 67, 203–229. doi: 10.1007/s13225-014-0297-2
Udayanga, D., Castlebury, L. A., Rossman, A. Y., Chukeatirote, E., and Hyde, K. D. (2015). The Diaporthe sojae species complex: phylogenetic re-assessment of pathogens associated with soybean, cucurbits and other field crops. Fungal Biol. 119, 383–407. doi: 10.1016/j.funbio.2014.10.009
Udayanga, D., Castlebury, L. A., Rossman, A. Y., and Hyde, K. D. (2014b). Species limits in Diaporthe: molecular re-assessment of D. citri, D. cytosporella, D. foeniculina and D. rudis. Persoonia 32, 83–101. doi: 10.3767/003158514X679984
Udayanga, D., Liu, X., Crous, P. W., McKenzie, E. H. C., Chukeatirote, E., and Hyde, K. D. (2012a). A multi-locus phylogenetic evaluation of Diaporthe (Phomopsis). Fungal Divers. 56, 157–171. doi: 10.1007/s13225-012-0190-9
Udayanga, D., Liu, X., Crous, P. W., McKenzie, E. H. C., Chukeatirote, E., and Hyde, K. D. (2012b). Multi-locus phylogeny reveals three new species of Diaporthe from Thailand. Cryptogam. Mycol. 33, 295–309. doi: 10.7872/crym.v33.iss3.2012.295
Udayanga, D., Liu, X., McKenzie, E. H. C., Chukeatirote, E., Bahkali, A. H. A., and Hyde, K. D. (2011). The genus Phomopsis: biology, applications, species concepts and names of common phytopathogens. Fungal Divers. 50, 189–225. doi: 10.1007/s13225-011-0126-9
Uecker, F. A. (1988). A World List of Phomopsis Names with Notes on Nomenclature, Morphology and Biology. Contributions from the U.S. National Fungus Collection. Mycologia Memoir, 13th Edn. Berlin: Cramer Publisher.
Villesen, P. (2007). FaBox: an online toolbox for FASTA sequences. Mol. Ecol. Notes 7, 965–968. doi: 10.1111/j.1471-8286.2007.01821.x
Visagie, C. M., Houbraken, J., Rodriques, C., Silva Pereira, C., Dijksterhuis, J., Seifert, K. A., et al. (2013). Five new Penicillium species in section Sclerotiora: a tribute to the Dutch Royal family. Persoonia 31, 42–62. doi: 10.3767/003158513X667410
White, T. J., Burns, T., Lee, S., and Taylor, J. (1990). “Amplification and direct sequencing of fungal ribosomal RNA genes for phylogenetics,” in PCR Protocols: A Guide to Methods and Applications, eds M. A. Innis, D. H. Gelfand, J. J. Sninsky, and T. J. White (San Diego, CA: Academic Press), 315–322. doi: 10.1016/b978-0-12-372180-8.50042-1
Wiman, N. G., Webber, J. B., Wiseman, M., and Merlet, L. (2019). Identity and pathogenicity of some fungi associated with hazelnut (Corylus avellana L.) trunk cankers in Oregon. PLoS One 14:e0223500. doi: 10.1371/journal.pone.0223500
Yang, Q., Fan, X. L., Guarnaccia, V., and Tian, C. M. (2018). High diversity of Diaporthe species associated with dieback diseases in China, with twelve new species described. MycoKeys 39, 97–149. doi: 10.3897/mycokeys.39.26914
Zapata, M., Palma, M. A., Aninat, M. J., and Piontelli, E. (2020). Polyphasic studies of new species of Diaporthe from native forest in Chile, with descriptions of Diaporthe araucanorum sp. nov., Diaporthe foikelawen sp. nov. and Diaporthe patagonica sp. nov. Int. J. Syst. Evol. Microbiol. 70, 3379–3390. doi: 10.1099/ijsem.0.004183
Keywords: fungi, Diaporthe, Phomopsis, hazelnut, rotten, molecular phylogeny, multi-locus sequence analysis, Turkey
Citation: Arciuolo R, Santos C, Soares C, Castello G, Spigolon N, Chiusa G, Lima N and Battilani P (2020) Molecular Characterization of Diaporthe Species Associated With Hazelnut Defects. Front. Plant Sci. 11:611655. doi: 10.3389/fpls.2020.611655
Received: 29 September 2020; Accepted: 16 November 2020;
Published: 11 December 2020.
Edited by:
Valerio Cristofori, University of Tuscia, ItalyReviewed by:
Stuart James Lucas, Sabancl University, TurkeyJay Pscheidt, Oregon State University, United States
Copyright © 2020 Arciuolo, Santos, Soares, Castello, Spigolon, Chiusa, Lima and Battilani. This is an open-access article distributed under the terms of the Creative Commons Attribution License (CC BY). The use, distribution or reproduction in other forums is permitted, provided the original author(s) and the copyright owner(s) are credited and that the original publication in this journal is cited, in accordance with accepted academic practice. No use, distribution or reproduction is permitted which does not comply with these terms.
*Correspondence: Paola Battilani, paola.battilani@unicatt.it