- 1Department of Bioenergy Science and Technology, Chonnam National University, Gwangju, South Korea
- 2Bioenergy Crop Research Institute, National Institute of Crop Science, Rural Development Administration, Muan, South Korea
Rare cold inducible 2 (RCI2) proteins are small hydrophobic membrane proteins in plants, and it has been widely reported that RCI2 expressions are dramatically induced by salt, cold, and drought stresses in many species. The RCI2 proteins have been shown to regulate plasma membrane (PM) potential and enhance abiotic stress tolerance when over-expressed in plants. RCI2 protein structures contain two transmembrane domains that are thought to be PM intrinsic proteins and have been observed at the PM and endomembranes. However, cellular trafficking of RCI2s are not fully understood. In this review, we discussed (i) general properties of RCI2s characterized in many species, (ii) the uses of RCI2s as a tracer in live cell imaging analyses and when they are fused to fluorescence proteins during investigations into vesicle trafficking, and (iii) RCI2 functionalities such as their involvement in rapid diffusion, endocytosis, and protein interactions. Consequently, the connection between physiological characteristics of RCI2s and traffic of RCI2s interacting membrane proteins might be helpful to understand role of RCI2s contributing abiotic stresses tolerance.
Introduction
It is impossible for plants to avoid abiotic stresses because they are sessile organisms. Therefore, plants have genetically evolved toward enhanced stress tolerance against abiotic stresses through the use of complex mechanisms. The stress tolerance properties of plants vary depending on their native location and environmental conditions, and understanding the genetic and physiological mechanisms that underlie stress tolerance will improve abiotic stress tolerance in plants and help improve crop yields.
The rare cold inducible 2 (RCI2s) are putative membrane proteins that are considerably induced by environmental stresses, such as salt, cold, and drought, in most plant species, microorganisms, and nematodes, but not in higher animals (Medina et al., 2007; Kim et al., 2016; Rocha, 2016). RCI2 names, such as low temperature inducible 6 (LTI6), plasma membrane proteolipid 3 (PMP3), and sensitive to Na+ (SNA) can vary depending on the species. The first RCI2 to be identified was early salt stress induced 3 (ESI3) in wheatgrass under salt stress (Gulick et al., 1994). The RCI2 characteristics in Arabidopsis were reported by Capel et al. (1997) who investigated RCI2A and RCI2B. The unique structure of the AtRCI2 family and their subcellular localization in the plasma and inner membranes were reported by Medina et al. (2007), and these reports have been used as a basis to investigate the expression and functional analysis of RCI2s in many different species. The multifunctionality of RCI2s in various plant species was reviewed by Rocha (2016). The study concluded that RCI2 functions were associated with the mechanism involved in defense against major abiotic stresses, such as cold, drought, and salt. Ectopical expression of RCI2s enhanced abiotic stress tolerance in many species (Feng et al., 2009; Long et al., 2015; Sivankalyani et al., 2015; Yu et al., 2016; Ben Romdhane et al., 2017), but an absence of RCI2s caused sensitive responses to the stresses (Mitsuya et al., 2005, 2006). The role of RCI2s during abiotic stress defense has been well reviewed. However, the precise mechanism by which small hydrophobic RCI2s contribute to abiotic stress tolerance remains unknown.
If the detailed function of RCI2s is to be determined, then it is important to understand the unique structures of these small hydrophobic proteolipids. The RCI2s have two transmembrane domains (TMDs), and almost half the RCI2s have a hydrophilic C-terminal tail. The eight AtRCI2s in Arabidopsis can be divided into two types based on the presence (AtRCI2D/E/F/G) or absence (AtRCI2A/B/C/H) of the hydrophilic C-terminal tails attached to their second TMD (Medina et al., 2007). The bioenergy crop, camelina (Camelina sativa L), belongs to the Brassicaceae family, and the CsRCI2s in camelina are reported to be highly hydrophobic proteins compared to those in other plant species (Supplementary Figure 1). Furthermore, the CsRCI2 homologies have identities that are similar to those of AtRCI2s (Supplementary Table 1), and phylogenetic tree analyses have shown that there is a close relationship between CsRCI2s and AtRCI2s when they are grouped based on their structural similarity (Supplementary Figure 1B). The protein sequence alignment and phylogenetic tree for RCI2s clearly showed that they were divided by their structural properties (Supplementary Figure 1). Additionally, several studies have reported that the over-expression of RCI2 proteins which do not contain the C-terminal hydrophilic tail contributed physiological changes and enhance abiotic stress tolerance (Feng et al., 2009; Kim et al., 2016, 2019). However, little is known about the function of tail-type RCI2 proteins when plants are subjected to cold, drought, and salt stress.
The two TMD structure is a common property of RCI2s. In most reports, subcellular localization of RCI2s was observed in the PM and other membranes, such as the Golgi apparatus, endoplasmic reticulum (ER), and intracellular vesicles, when they were fused to fluorescence proteins (Rocha, 2016). Therefore, the structures associated with RCI2s enable them to localize in cellular membranes within the cell. However, RCI2s are small size (∼55–76 amino acids long) protein that it is not yet determined to have a transport activity contributing abiotic stress tolerance. Previous study suggested that the functions of RCI2s were not provided by its single expression but through their interaction with functional proteins related to the abiotic stresses defense mechanism (Kim et al., 2019). From this result, it was speculated that the function of RCI2 is related to protein interactions important for cellular homeostasis through vesicle trafficking, such as endocytosis.
Changes to RCI2 Expression in Response to Abiotic Stresses
The RCI2 expressions are markedly induced by cold stress, drought, and saline stress (Medina et al., 2007; Rocha, 2016). Many reports have suggested that osmotic unbalance and water deficiency induce RCI2 expression in plant cells. The expression profiles of the RCI2/PMP3 responses to abiotic stresses in various species have been reviewed by Rocha (2016). These include Arabidopsis (Capel et al., 1997; Medina et al., 2001, 2007; Nylander et al., 2001), maize (Fu et al., 2012; Zhao et al., 2014), barley (Goddard et al., 1993; Ueda et al., 2004), alfalfa and barrel medic (Long et al., 2015), wheatgrass (Gulick and Dvořák, 1992; Galvez et al., 1993), strawberry (NDong et al., 1997), Physcomitrella patens (Kroemer et al., 2004), sheepgrass (Inada et al., 2005), rice (Morsy et al., 2005; Kim et al., 2007; Chang-Qing et al., 2008), wheat (Imai et al., 2005), alkali grass (Chang-Qing et al., 2008), mandarin (Gimeno et al., 2009), plantain (Feng et al., 2009; Liu et al., 2012), red sage (Wang D. et al., 2013), smooth cordgrass (Baisakh et al., 2008; Baisakh and Subudhi, 2009), and Aulacomnium turgidum (Liu et al., 2010). Additionally, the RCI2/PMP3 gene expression profiles have been updated for camelina (Kim et al., 2016, 2020), pearl millet (Yeshvekar et al., 2017), and cucumber (Zhou et al., 2020).
In Arabidopsis, AtRCI2 expressions were considerably induced by cold, abscisic acid, dehydration, and NaCl, whereas the AtRCI2C and AtRCI2H level was not significantly different from that of the control (Medina et al., 2007). The expressions of CsRCI2A/E in camelina, which have high homology to AtRCI2s were considerably induced by cold, drought, and NaCl (Figure 1). The expressions of ten ZmRCI2 members, which are involved in the maize response to drought stress, either increased (ZmRCI2-1, 2, 6, 7) or decreased (ZmRCI2-3, 5, 8, 9, 10) (Zhao et al., 2014). These results indicated that the expression of each RCI2 in the plant species had individual characteristic responses to its corresponding abiotic stresses. The RCI2s may not respond to all abiotic stresses, but may react to a specific stress type depending on their promoter. Upstream region of RCI2 was reported to be regulated by C-repeat binding factor (CBF) in rice (Kim et al., 2007), but this needs to be confirmed by investigating the transcription factor that regulates the expression of RCI2s under salt stress.
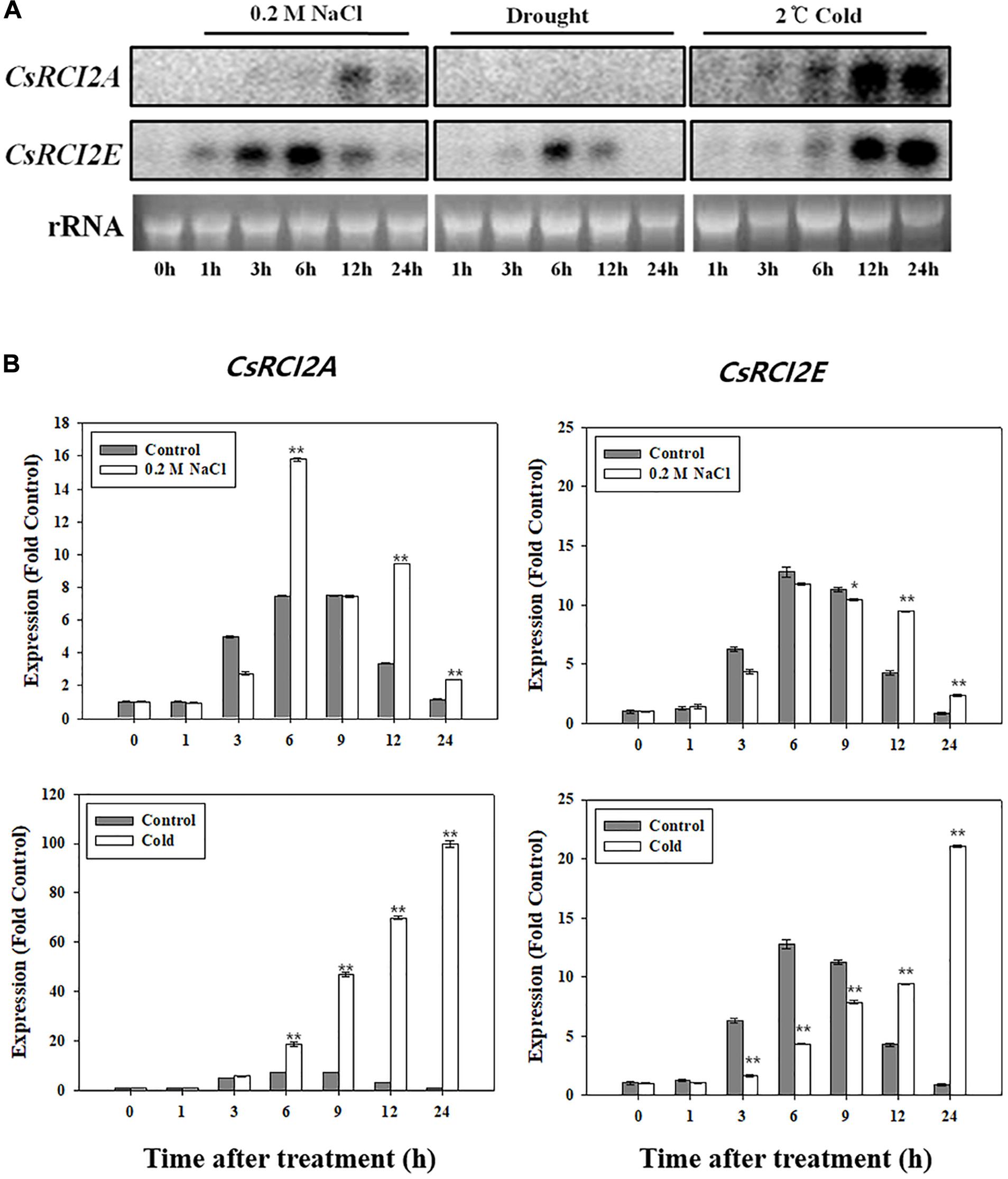
Figure 1. Expression changes in CsRCI2A and CsRCI2E under NaCl, cold, and mannitol stress. The expression of genes were analyzed by RNA blot (A) and quantitative RT-PCR analysis (B). The CsRCI2A and CsRCI2E revealed typical expression of RCI2s induced by cold and NaCl stresses over time (Kim et al., 2016).
Medina et al. (2007) reported that AtRCI2s showed different expressions depending on the tissue examined, such as leaves, stems, roots, flowers, and seeds. Additionally, modification of the RCI2 promoter changed the localization range in xylem and phloem (Chidambaram et al., 2015). This suggested that the expression and localization of RCI2s were dependent on the promoter. At the germination stage, the expression of AtRCI2A and AtRCI2B had increased at days 1 and 2, but returned to the control level at 3 days after germination according to a GUS analysis using the native promoter for each RCI2 in Arabidopsis (Medina et al., 2001). Therefore, the RCI2 expressions may vary depending on the development stage and their responses to environmental stimulation. Furthermore, the regulation of each RCI2 expression may be combined with tissue specific expression and that this combination contributes to cell homeostasis under various abiotic stresses.
The gene expression of RCI2s has been widely reported. However, few protein accumulation analyses have been performed by western blotting. Furthermore, it is difficult to synthesize antibodies for the no-tail type RCI2s because of their hydrophobic properties. However, the accumulations of a few RCI2s, such as CsRCI2E and CsRCI2F in camelina, have been determined by analysis of the tail types (Kim et al., 2019). Both CsRCI2E and CsRCI2F accumulation at the PM was induced under NaCl stress in a time- and dose-dependent manner. The CsRCI2E accumulation was more significantly induced by NaCl concentration compared to CsRCI2F. The different RCI2 protein accumulation levels might be caused by their differing gene expression characteristics. The diverse gene and protein expression properties among RCI2 members suggest that they may have different roles in the maintenance of cell homeostasis and the plant response to various abiotic stresses.
Properties of Plasma Membrane RCI2 Proteins
Most of the previous RCI2s studies investigated the hydrophobic two TMD structure. Many prediction results have shown that the RCI2 protein structure has two regions that are predicted to be TMDs, but several proteins have hydrophilic N- or C-terminal residues that resemble a tail (Supplementary Figure 2). The properties of RCI2/PMP3 have classified it as a proteolipid. Rocha (2016) grouped the RCI2/PMP3 family into groups Ia (hydrophobic end), Ib (charged end), and II (hydrophilic C-terminal tail) based on hydropathy and extension of the C-terminal tail. However, a few proteins, such as SNA3/4 in yeast and CsaRCI2C in cucumber, have an N-terminal tail. These types of proteins were subdivided into PMP3-like proteins by Kwok et al. (2020).
The best known functions of the C-terminal tail in RCI2s are related to PMP3 (SNA1) complementation of NaCl sensitivity in knockout mutant yeast. Navarre and Goffeau (2000) reported that the function of RCI2s was to modulate membrane potential, which is related to NaCl sensitivity in yeast. Other reports suggested that if the RCI2s had a tail at the C-terminus, then Δpmp3 yeast did not recover NaCl tolerance, but C-terminal tail truncated RCI2s could recover their NaCl tolerance (Medina et al., 2007). In camelina, CsRCI2s also exhibited functional complementation of Δpmp3 yeast if they did not have a tail (Kim et al., 2016). However, when a C-terminal tail was added to a no-tail type, then it could not complement NaCl tolerance when expressed in Δpmp3. In Escherichia coli, YqaE has been identified as an RCI2 homolog. Unlike yeast PMP3, mutant E. coli that lacked YqaE were sensitive to both deficiency and excess concentration of NaCl (Kwok et al., 2020). Interestingly, this sensitivity to NaCl deficiency in prokaryote E. coli was complemented by expression of other RCI2 homologs, such as MFP1a from marine dinoflagellates belong into eukaryote. Additionally, YqaE expression decreased when E. coli was incubated in 200 mM KCl, but yeast PMP3 and dinoflagellate MFP1a expression increased. These results suggest that RCI2s have individual response to Na+ and K+ sensitivity for each kingdom. In plant kingdom, atrci2a knockout mutant Arabidopsis showed NaCl sensitivity than wild type (WT) but recovered by over-expression of MpRCI from Musa paradisiac. Interestingly, MpRCI over-expressed atrci2a plant showed increased KCl sensitivity while atrci2a knockout mutant was tolerant to 150 mM KCl stress than WT (Liu et al., 2012). This suggest that RCI2 proteins played important roles in Na+ and K+ cellular ionic balance in each kingdom. Therefore, investigation of cross-linkage between RCI2s and transporter proteins related to Na+/K+ balance will be important for further studies.
The deletion of the C-terminal tail on SNA2 in yeast meant that SNA2 became localized on the PM rather than the ER (Renard et al., 2010). This suggested that the C-terminal tail might have a key destination sequence that allowed the protein to be sent to the right location. However, truncation of the RCI2 N-terminal tail was not investigated in the Δpmp3 complementation experiment. A future study using C-terminal tail-deleted RCI2-over-expressing plants is required if the different functions of the tail in RCI2s are to be fully understood.
Subcellular Localization and Endocytic Trafficking of RCI2 Proteins
Recent studies have shown that most of the RCI2 proteins are localized at the PM in Arabidopsis, maize, alfalfa, barrel medic, rice, and wheat (Medina et al., 2007; Rocha, 2016). In camelina, the GFP or YFP fused RCI2s, such as CsRCI2A/E/H, have been reported to localized at the PM (Figure 2; Kim et al., 2016, 2020). However, these CsRCI2s, including CsRCI2A, CsRCI2B, CsRCI2C, CsRCI2E, CsRCI2F, and CsRCI2G, were also found on endomembrane vesicles (Supplementary Videos 1–6). These RCI2s-vesicles were also observed around the PM and vacuoles in cells when transiently expressed in tobacco leaf. Furthermore, several vesicles emerged from the PM and then moved to the intracellular space, which is similar to endocytosis. However, the reason of occurrence of RCI2-vesicle trafficking has not been properly reviewed in plants. The unique two TMD structure of RCI2s has been shown to be due to an integral PM protein. Therefore, methods in previous studies involved fusion of LTI6a (AtRCI2A) and LTI6b (AtRCI2B) with non-polar PM marker proteins that could be used as tracers in high-resolution live imaging systems (Grebe et al., 2003; Maizel et al., 2011; Hosy et al., 2015). This tracer system has been used to investigate RCI2-associated endocytic trafficking in studies pertaining to the endocytosis of transporter proteins (Table 1). The RCI2s are considered to be PM proteins bound to phospholipid, and GFP-fused LTI6a and LTI6b-over-expressing Arabidopsis plants have helped to elucidate endocytic trafficking of PIN2 and aquaporins (Kleine-Vehn et al., 2011; Li et al., 2011; Wudick et al., 2015). When the LTI6s were treated with brefeldin A [BFA, aggregation reagent for endocytic vesicles trapped in the trans-Golgi network and early endosomes (EEs)], the results showed that they were localized to the early endosomes (EEs). When Arabidopsis roots were treated with cycloheximide (CHX) for 15 and 30 min to limit protein synthesis, the Lti6a-GFP protein clearly internalized to BFA bodies (Wang C. et al., 2013). Also, GFP-LTI6b expression was observed at the vacuole in Arabidopsis under NaCl stress (Ueda et al., 2016). Yeast that lacked the PMP3 protein showed delayed endocytosis of lipid-specific dye FM4-64 in their cells. Furthermore, ΔPMP3 yeast showed decreased expression of membrane proteins and increased expression of endocytosis-related proteins in ΔPMP3 compared to the WT (De Block et al., 2015). These results suggested that RCI2s could be localized at the PM, EEs, or vacuoles through the endocytic vesicle trafficking pathway.
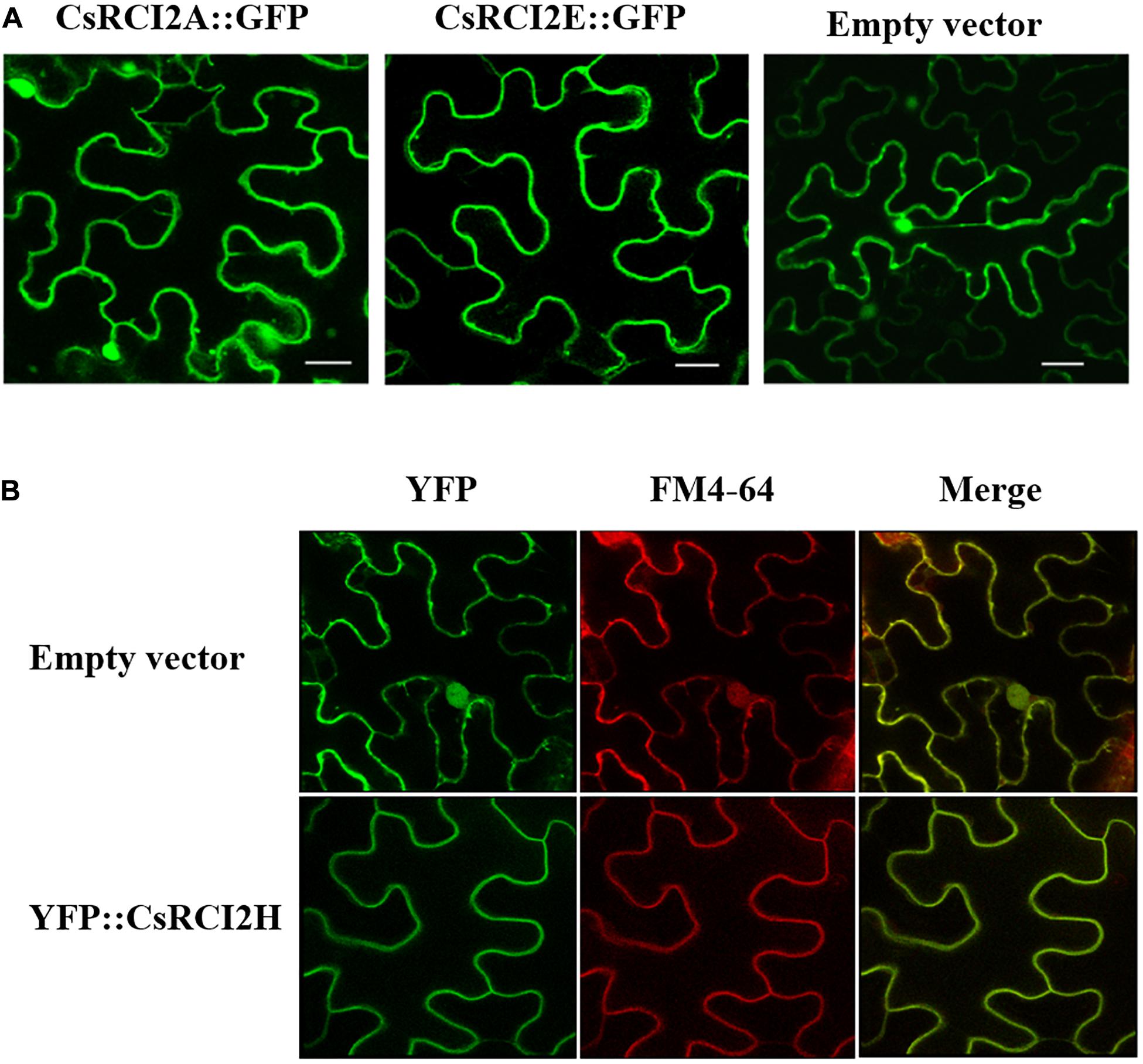
Figure 2. Subcellular localization of CsRCI2A/E-GFP and YFP-CsRCI2H proteins in tobacco. Confocal laser scanning microscope images of tobacco cells expressing GFP and YFP fused to CsRCI2A/E (A) (Kim et al., 2016) and CsRCI2H (B) (Kim et al., 2020) proteins, respectively. The red fluorescence signal represent FM4-64 stained lipids and PM in cell. White scale bars represent 20 μm.
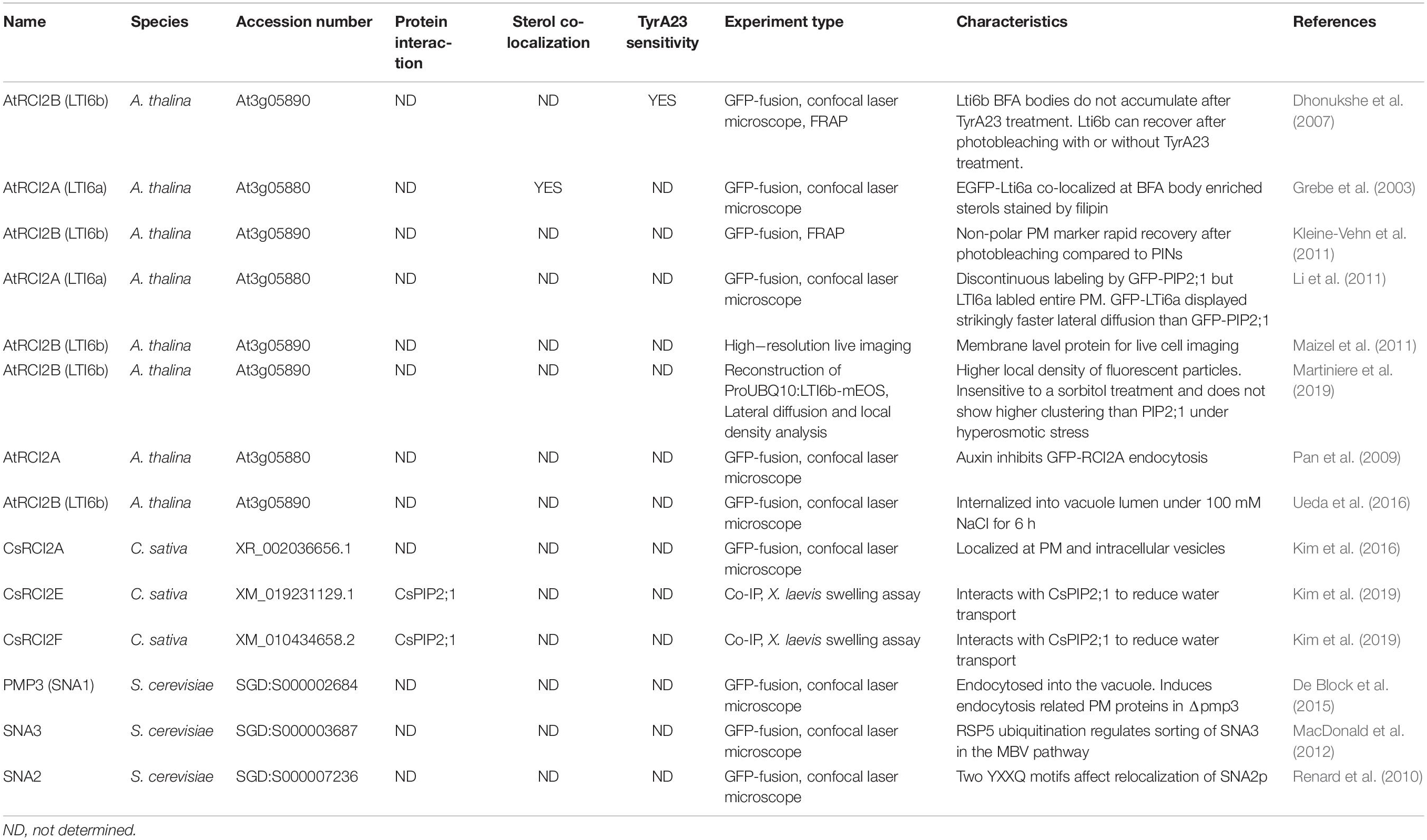
Table 1. Subcellular localization or endomembrane trafficking of RCI2/PMP3 proteins established by recent studies.
Endocytosis is a basic metabolism in living organism that participating remove, recycle, and degrade of proteins from PM (Rodriguez-Furlan et al., 2019). It maintains cell homeostasis by fine-tuning the PM system to adapt to biotic and abiotic stresses in plants (Wang et al., 2020). Internalization of major PM transporter compounds, such as water transporter aquaporins and auxin transporter PIN2, has been reported to regulate water homeostasis and cell development, respectively (Kleine-Vehn et al., 2011; Li et al., 2011; Luu et al., 2012). The endocytosis of PIP2s has been shown to be internalized through both clathrin-mediated endocytosis (CME) and the sterol-raft system under severe osmotic stress, including NaCl shock (Li et al., 2011; Pou et al., 2016). However, it has also been reported that the PIN2 pathway is only regulated by CME as evidenced by a pharmacological test using tyrphostin A23 (TyrA23), which inhibits CME (Dhonukshe et al., 2007). Interestingly, a study on PIP2 and PIN2 endocytosis also tested LTI6b as a reference PM protein (Wang C. et al., 2013). In their results, expression of GFP-LTI6b revealed endocytic trafficking and CME features after treatment with BFA. These results suggested that RCI2 proteins could be internalized into the endomembranes. Additionally, the accumulation of LTI6a and LTI6b BFA bodies was inhibited by treatment with auxin and TyrA23 (Dhonukshe et al., 2007; Pan et al., 2009). This implied that LTI6a and LTI6b-associated endocytosis was regulated by hormone signals and CME. The RCI2/PMP3 dynamics were confirmed by other studies when the LTI6s were introduced as PM marker proteins. The density results for GFP-LTI6b fluorescence protein particles after they had been recovered following photo bleaching showed that they had a higher velocity than that of GFP-AtPIP2;1 (Dhonukshe et al., 2007). Furthermore, LTI6b had a greater rapid diffusion co-efficiency compare than other PM proteins, such as PIP2;1 and AHA2, when a FRAP (fluorescence recovery after photobleaching) test was carried out (Martiniere et al., 2019). Additionally, the velocity of LTI6b re-localization was faster than that for PIP2;1 and PIN2, but slower than total lipid stained by FM4-64 (Boutté et al., 2010). This information suggests that the RCI2 proteins have characteristics that would support rapid trafficking. However, LTI6a/b are typical no-tail type RCI2 proteins, which suggests that future studies should characterize tail-type RCI2 proteins. The function of tail-type RCI2s is not well-established in plant species, but significant results have been revealed by several reports on yeast. In yeast, four PMP3 protein families can be divided into a no-tail (PMP3/SNA1) family and tail type families (SNA2, SNA3, and SNA4). Deletion of PMP3 increases NaCl sensitivity, but it is reduced by RCI2 homologs that have no tails. PMP3 has been considered to be a membrane potential regulator because Na+ uptake increased when PMP3 was deleted (Nylander et al., 2001). De Block et al. (2015) reported the role played by PMP3 in yeast PM proteins. They found that yeast that lacked PMP3 had an altered PM protein composition that led to increased amounts of endocytosis-related proteins compared to the WT. They also reported that the Δpmp3 yeast showed delayed endocytosis of FM4-64 stained vesicles compared to the WT, and that PMP3 protein could bind to phosphorylated phosphoinositides, phosphatidic acid, and sphingomyelin. The growth of Δpmp3 yeast was affected because it could not metabolize sphingolipid. These results suggest that PMP3 plays an important role in the endocytic trafficking pathway and that it interacts with inositides and sphingolipid.
Yeast SNA2 is a tail type RCI2/PMP3 protein that has a hydrophilic tail in the C-terminal region. Renard et al. (2010) reported that point mutation of the YXXQ motif in C-terminal residues changed the destination of SNA2 protein during the re-localization process. Furthermore, their report suggested that two tyrosine-based sorting motifs in SNA2 played a key role in the localization pathway. SNA3 belongs to the PMP3-like proteins, which have relatively long C-terminal tails compared to other tail type RCI2s. SNA3 has been reported to interact with RSP5, a major ligase responsible for ubiquitination of proteins (Stawiecka-Mirota et al., 2007; Lauwers et al., 2010; MacDonald et al., 2012). It is an adapter protein for RSP5 which acts via the PY (PPXY) motif in the C-terminal residue and is sorted in multiple vesicular bodies. Additionally, it has been found that SNA3 protein lacks the lysine residues found in the cytosolic region of K19 (N-terminal) and K125 (C-terminal), which affects SNA3 protein trafficking to the vacuole (MacDonald et al., 2012). These results indicated that the PMP3-like structure might be involved in specialist protein binding and could induce endomembrane sorting. Furthermore, reports have suggested that the C-terminal peptide properties of RCI2/PMP3 may contribute to the final destination of vesicles containing RCI2/PMP3.
Function of RCI2-Induced Vesicle Trafficking
Previous reports have suggested that NaCl or osmotic stress induces endocytosis of PIP2s (Li et al., 2011; Chevalier and Chaumont, 2015; Pou et al., 2016). Under hyperosmotic conditions, PIP2s are internalized into the intracellular region by CME or sterol-raft induced endocytosis. These mechanisms are known to maintain water homeostasis under osmotic stress. The PIP2s contribute to water transportation into cells, and they are also involved in the removal of compounds from cells, which is based on the outside osmolarity of the cell. The water transport activities of PIPs are regulated by phosphorylation, voltage-sense gating, and internalization into cells. Additionally, the Na+ transport activity of AtPIP2;1 is regulated by its interaction with AtPIP1;2 (Byrt et al., 2017). The PIP2;1 channel ion transport pathway needs to be inactivated if cell homeostasis is to be maintained under serious NaCl stress. Internalized PIP2s are sorted in vesicles and vacuoles for recycling or degradation (Ueda et al., 2016). The protein interactions between tail-type CsRCI2E/F and CsPIP2;1 have been analyzed using co-immunoprecipitation and a Xenopus laevis oocyte swelling assay in camelina (Kim et al., 2019). Purified PM protein pull-down assay using CsRCI2E and CsRCI2F antibodies showed a CsPIP2s band after immunoblotting with PIP2s antibodies. Expression of CsRCI2E and CsRCI2F reduced the water transport activity of CsPIP2;1 when co-expressed in the X. laevis oocyte. Furthermore, CsPIP2;1 abundance at the oocyte membrane decreased after co-expression with CsRCI2E and CsRCI2F. This suggests that deactivation of CsPIP2;1 by internalization can reduce Na+ influxes into cells under NaCl stress.
The H+-ATPase in the PM is a key regulator of NaCl tolerance because it generates a proton driving force for Na+/H+ exchange by SOS1 (Shi et al., 2000; Zhu, 2003). Moreover, increased PM H+-ATPase activity has also been reported to contribute to cold stress tolerance in many plant species (Janicka-Russak et al., 2012). In Arabidopsis atrci2a mutant complemented by MpRCI2 over-expression, the PM H+-ATPase activity was increased under NaCl stress than WT but the function for RCI2-induced H+-ATPase activation was not concluded (Liu et al., 2012). The activation of PM H+-ATPase regulated by several conditions such as phosphorylation of penultimate C-terminal threonine residue, interaction with 14-3-3 proteins, and abundance of phosphatase PP2C in cytosol (Spartz et al., 2014). Moreover, hormonal endocytic trafficking of PM H+-ATPase have been reported by Xia et al. (2019). In the result, SYP132-induced endocytic trafficking of PM H+-ATPase from PM was inhibited by auxin, but promoted by ABA (abscisic acid). Meanwhile, auxin treatment causes inhibition of RCI2s endocytosis but ABA treatment can induce RCI2 expression (Medina et al., 2007; Pan et al., 2009). It means that both auxin and ABA can affect PM H+-ATPase activity and/or endocytosis of RCI2s. However, the relationship between RCI2 and PM H+-ATPase requires additional analysis of the RC12 type when it is subjected to various stress conditions. Also, status of RCI2s and PM H+-ATPase trafficking should be considered in further study.
The expression of most RCI2 proteins are rapidly induced by abiotic stresses, and high RCI2 expression levels under abiotic stresses have been confirmed by many reports (Nylander et al., 2001; Medina et al., 2007; Kim et al., 2016; Rocha, 2016). It has been suggested that RCI2 regulates membrane potential under cold, drought, and salt stress (Kim et al., 2016; Kwok et al., 2020). To date, other independent functions related to RCI2 proteins have not been adequately investigated, but their characteristics imply that they can be used to explore cell functioning. Therefore, they are often used as a PM reference protein during live cell imaging when fused with fluorescence proteins (Li et al., 2011). Many reports have indicated that RCI2 proteins can travel from the PM to the ER or EE via the endocytic trafficking pathway. It has also been shown that RCI2B (Lti6b) treated with BFA can mediate the localization of RCI2B at BFA bodies, but it has been reported to be inhibited by TyrA23 (Dhonukshe et al., 2007). This result indicated that endocytic trafficking of RCI2A was part of the CME pathway because TyrA23 is a known inhibitor of CME. In addition, Wang C. et al. (2013) concluded that endocytosis of RCI2A-GFP is clathrin-dependent because the numbers of RCI2A-GFP labeled BFA bodies were changed in clathrin light chain double knockout (clc2-1 and clc3-1) Arabidopsis. However, EGFP-RCI2B was observed in the BFA body along with sterols stained by the sterol-binding dye filipin (Grebe et al., 2003). Plant cells have a sterol-raft endocytic pathway and AtPIP2;1 has been reported to be internalized by both the CME and the sterol-raft pathway (Li et al., 2011). Interestingly, PIP2;1 has been shown to interact with CsRCI2E and CsRCI2F in camelina (Kim et al., 2019). This suggested that RCI2 might be related to the sterol-raft pathway. The evidence suggests that RCI2s are involved in both the CME and Sterol-raft pathways, but only results for a few of RCI2A and RCI2B are currently reported. Therefore, focusing on endocytic trafficking of unlabeled RCI2 members related to CME or sterol-raft pathway are required for further study.
Concluding Remarks
Many plant species have RCI2 family (Rocha, 2016). Unlike animal, survival of plants depends on its natural born stress tolerance or dynamic adaptation from various environmental conditions. The RCI2s exhibit dynamic expression, cellular diffusion, and endocytic trafficking (Medina et al., 2007; Kim et al., 2019; Martiniere et al., 2019). Most of RCI2s studies concluded that RCI2s participate wide range of stress tolerance (Liu et al., 2012; Kim et al., 2016, 2020). However, the published studies of RCI2s in plants did not make a linkage with cellular functions despite the several RCI2s were already been used to tracking membrane proteins redistribution through live cell imaging (Li et al., 2011; Maizel et al., 2011; Hosy et al., 2015). Moreover, recent reports of RCI2s live cell imaging was carried out through pharmacology process such as FM4-64 and NaCl even the RCI2s have individual characteristics to temperature stress (Ueda et al., 2016; Kim et al., 2020). Therefore, exploring RCI2s dynamics under various stresses such as cold and heat might be provided novel mechanisms of RCI2-dependent stress tolerances.
The RCI2s have unique structure containing two TMD and hydrophilic C-terminal tail and can divide into subgroups by composition of N- or C-terminal extension (Kwok et al., 2020). C-terminus studies of SNA2 and SNA3 in yeast revealed that RCI2s have motifs to binding other proteins or molecules which is guide to final destination (Stawiecka-Mirota et al., 2007; Renard et al., 2010; MacDonald et al., 2012). Also, CsRCI2E and CsRCI2F in camelina as a tail type showed protein interaction with PIP2 which is participate PM abundance of PIP2 (Kim et al., 2019). From these evidences, C-terminus of RCI2s might have functional motifs for cellular redistribution. However, no-tail type RCI2s can travel through endocytic pathway, especially in RCI2A and RCI2B (Pan et al., 2009; Li et al., 2011; Maizel et al., 2011; Ueda et al., 2016). These evidences make question to possible binding affinity from two TMD structure. Therefore, characterization of motif-specific RCI2s traffic regulation might be a tool for regulation of membrane proteins distribution for crop of the desired form.
In conclusion, the results indicated that the expression of RCI2s proteins was related to internalization of PM proteins, and that the regulation of major transporter protein activity altered the strategy used to maintain cell homeostasis under abiotic stresses (Figure 3). However, a number of aspects about the RCI2 properties need to be investigated. The aspects are: (i) does RCI2 protein directly mediate vesicle formation? (ii) What is the function of RCI2 under temperature stress; and (iii) which PM protein binding motifs regulate RCI2 binding? Furthermore, internalization of RCI2 has not been fully elucidated in relation with CME or sterol-raft endocytosis, and has not been investigated for all subgroups of RCI2s. The expressions of the RCI2 genes showed that their individual transcriptions were affected by the type, intensity, and duration of the abiotic stresses. Therefore, focusing on the individual roles of RCI2 members under abiotic stress will improve our understanding of the internalization of PM proteins, their effects on cell homeostasis, and the mechanisms that help cells adapt to adverse conditions.
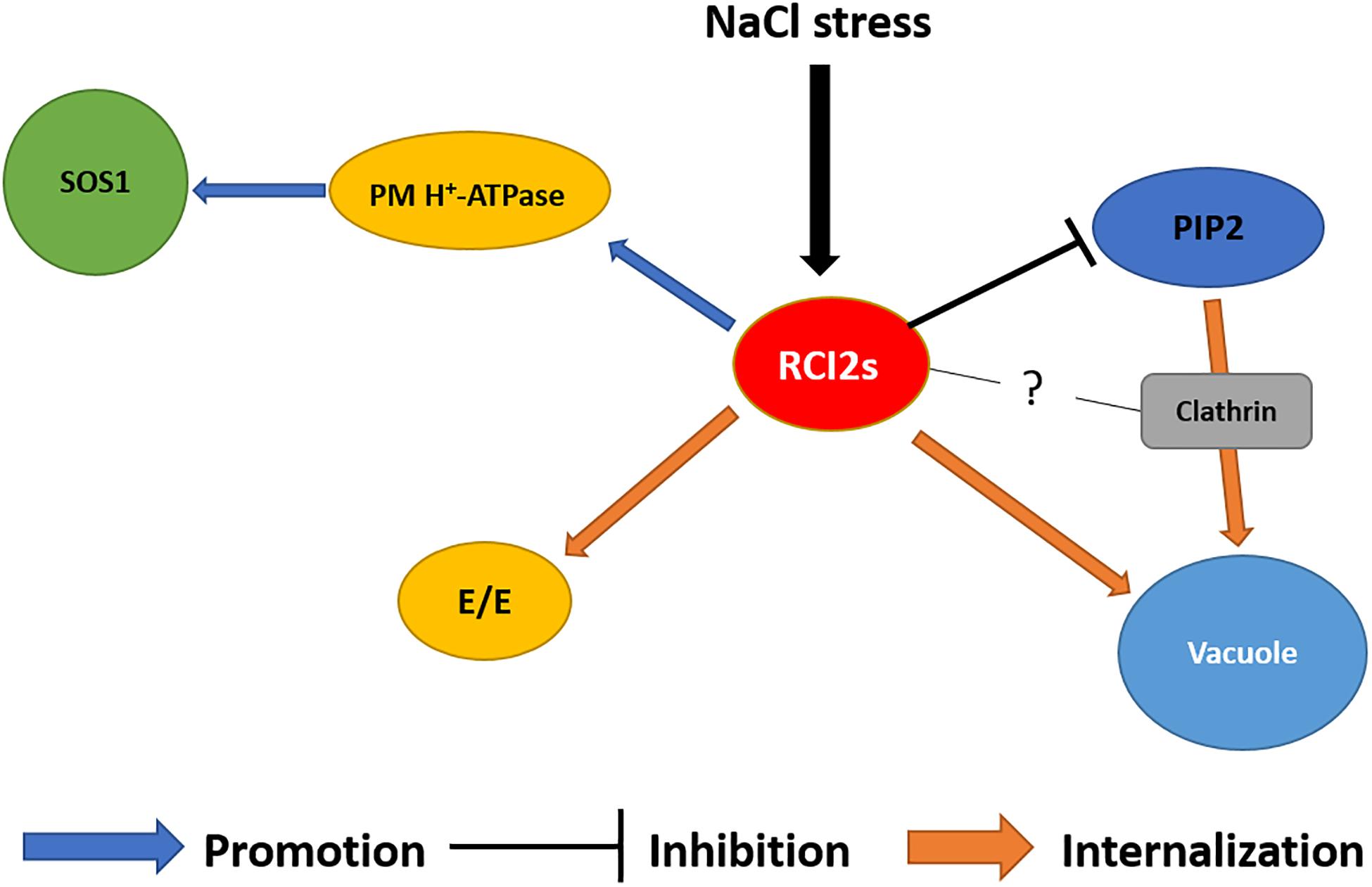
Figure 3. Schematic diagram of the RCI2/PMP3-related NaCl stress tolerance mechanism. Expression of RCI2s promotes PM H+-ATPase activity to generate a proton driving force for the Na+/H+ exchanger SOS1. The NaCl-induced RCI2s inhibit water transport activity of aquaporin PIP2, which transports Na+ into the cell. Under NaCl stress, PIP2s were internalized into the vacuole via CME. Subcellular localization of RCI2s was observed in the PM and endomembranes, including early endosomes (EEs) and the vacuole. The NaCl-induced RCI2s contribute to the NaCl stress defense mechanism by regulating the activity and reorganization of PM proteins, which helps maintain ion homeostasis in the plant cell.
Author Contributions
H-SK and WP contributed to the review conception, design, and writing of the manuscript. H-SL and J-HS assisted information and data arrange from literature. S-JA supervised the entire study including manuscript editing. All authors contributed to the completed manuscript.
Funding
This research was supported by the Basic Science Research Program through the National Research Foundation of Korea (NRF), grant funded by the Korea government (MSIT) (2019R1A6A3A01096311) and Water Management Research Program funded by the Ministry of Land, Infrastructure and Transport of the Korean Government (18AWMP-B114119-03).
Conflict of Interest
The authors declare that the research was conducted in the absence of any commercial or financial relationships that could be construed as a potential conflict of interest.
Supplementary Material
The Supplementary Material for this article can be found online at: https://www.frontiersin.org/articles/10.3389/fpls.2020.610251/full#supplementary-material
References
Baisakh, N., and Subudhi, P. K. (2009). Heat stress alters the expression of salt stress induced genes in smooth cordgrass (Spartina alterniflora L.). Plant Physiol. Biochem. 47, 232–235. doi: 10.1016/j.plaphy.2008.11.010
Baisakh, N., Subudhi, P. K., and Varadwaj, P. (2008). Primary responses to salt stress in a halophyte, smooth cordgrass (Spartina alterniflora Loisel.). Funct. Integr. Genomic 8, 287–300. doi: 10.1007/s10142-008-0075-x
Ben Romdhane, W., Ben-Saad, R., Meynard, D., Verdeil, J.-L., Azaza, J., Zouari, N., et al. (2017). Ectopic expression of Aeluropus littoralis plasma membrane protein gene AlTMP1 confers abiotic stress tolerance in transgenic tobacco by improving water status and cation homeostasis. Int. J. Mol. Sci. 18:692. doi: 10.3390/ijms18040692
Boutté, Y., Frescatada−Rosa, M., Men, S., Chow, C. M., Ebine, K., Gustavsson, A., et al. (2010). Endocytosis restricts Arabidopsis KNOLLE syntaxin to the cell division plane during late cytokinesis. EMBO J. 29, 546–558. doi: 10.1038/emboj.2009.363
Byrt, C. S., Zhao, M., Kourghi, M., Bose, J., Henderson, S. W., Qiu, J., et al. (2017). Non−selective cation channel activity of aquaporin AtPIP2; 1 regulated by Ca2+ and pH. Plant Cell Environ. 40, 802–815. doi: 10.1111/pce.12832
Capel, J., Jarillo, J. A., Salinas, J., and Martinez-Zapater, J. M. (1997). Two homologous low-temperature-inducible genes from Arabidopsis encode highly hydrophobic proteins. Plant Physiol. 115, 569–576. doi: 10.1104/pp.115.2.569
Chang-Qing, Z., Shunsaku, N., Shenkui, L., and Tetsuo, T. (2008). Characterization of two plasma membrane protein 3 genes (PutPMP3) from the alkali grass, Puccinellia tenuiflora, and functional comparison of the rice homologues, OsLti6a/b from rice. BMB Rep. 41, 448–454. doi: 10.5483/bmbrep.2008.41.6.448
Chevalier, A. S., and Chaumont, F. (2015). Trafficking of plant plasma membrane aquaporins: multiple regulation levels and complex sorting signals. Plant Cell Physiol. 56, 819–829. doi: 10.1093/pcp/pcu203
Chidambaram, R., Venkataraman, G., and Parida, A. (2015). Analysis of transcriptional regulation and tissue-specific expression of Avicennia marina plasma membrane protein 3 suggests it contributes to Na+ transport and homoeostasis in A. marina. Plant Sci. 236, 89–102. doi: 10.1016/j.plantsci.2015.03.013
De Block, J., Szopinska, A., Guerriat, B., Dodzian, J., Villers, J., Hochstenbach, J.-F., et al. (2015). Yeast Pmp3p has an important role in plasma membrane organization. J. Cell Sci. 128, 3646–3659. doi: 10.1242/jcs.173211
Dhonukshe, P., Aniento, F., Hwang, I., Robinson, D. G., Mravec, J., Stierhof, Y.-D., et al. (2007). Clathrin-mediated constitutive endocytosis of PIN auxin efflux carriers in Arabidopsis. Curr. Biol. 17, 520–527. doi: 10.1016/j.cub.2007.01.052
Feng, D.-R., Liu, B., Li, W.-Y., He, Y.-M., Qi, K.-B., Wang, H.-B., et al. (2009). Over-expression of a cold-induced plasma membrane protein gene (MpRCI) from plantain enhances low temperature-resistance in transgenic tobacco. Environ. Exp. Bot. 65, 395–402. doi: 10.1016/j.envexpbot.2008.12.009
Fu, J., Zhang, D.-F., Liu, Y.-H., Ying, S., Shi, Y.-S., Song, Y.-C., et al. (2012). Isolation and characterization of maize PMP3 genes involved in salt stress tolerance. PLoS One 7:e31101. doi: 10.1371/journal.pone.0031101
Galvez, A. F., Gulick, P. J., and Dvorak, J. (1993). Characterization of the early stages of genetic salt-stress responses in salt-tolerant Lophopyrum elongatum, salt-sensitive wheat, and their amphiploid. Plant Physiol. 103, 257–265. doi: 10.1104/pp.103.1.257
Gimeno, J., Gadea, J., Forment, J., Pérez-Valle, J., Santiago, J., Martinez-Godoy, M. A., et al. (2009). Shared and novel molecular responses of mandarin to drought. Plant Mol. Biol. 70, 403–420. doi: 10.1007/s11103-009-9481-2
Goddard, N., Dunn, M., Zhang, L., White, A., Jack, P., and Hughes, M. (1993). Molecular analysis and spatial expression pattern of a low-temperature-specific barley gene, blt101. Plant Mol. Biol. 23, 871–879. doi: 10.1007/bf00021541
Grebe, M., Xu, J., Möbius, W., Ueda, T., Nakano, A., Geuze, H. J., et al. (2003). Arabidopsis sterol endocytosis involves actin-mediated trafficking via ARA6-positive early endosomes. Curr. Biol. 13, 1378–1387. doi: 10.1016/s0960-9822(03)00538-4
Gulick, P. J., and Dvořák, J. (1992). Coordinate gene response to salt stress in Lophopyrum elongatum. Plant Physiol. 100, 1384–1388. doi: 10.1104/pp.100.3.1384
Gulick, P. J., Shen, W., and An, H. (1994). ESI3, a stress-induced gene from Lophopyrum elongatum. Plant Physiol. 104:799. doi: 10.1104/pp.104.2.799
Hosy, E., Martinière, A., Choquet, D., Maurel, C., and Luu, D.-T. (2015). Super-resolved and dynamic imaging of membrane proteins in plant cells reveal contrasting kinetic profiles and multiple confinement mechanisms. Mol. Plant. 8, 339–342. doi: 10.1016/j.molp.2014.10.006
Imai, R., Koike, M., Sutoh, K., Kawakami, A., Torada, A., and Oono, K. (2005). Molecular characterization of a cold-induced plasma membrane protein gene from wheat. Mol. Genet. Genomics 274, 445–453. doi: 10.1007/s00438-005-0050-3
Inada, M., Ueda, A., Shi, W., and Takabe, T. (2005). A stress-inducible plasma membrane protein 3 (AcPMP3) in a monocotyledonous halophyte, Aneurolepidium chinense, regulates cellular Na+ and K+ accumulation under salt stress. Planta 220, 395–402. doi: 10.1007/s00425-004-1358-7
Janicka-Russak, M., Kabała, K., Wdowikowska, A., and Kłobus, G. (2012). Response of plasma membrane H+-ATPase to low temperature in cucumber roots. J. Plant Res. 125, 291–300. doi: 10.1007/s10265-011-0438-6
Kim, H.-S., Lee, J.-E., Jang, H.-Y., Kwak, K.-J., and Ahn, S.-J. (2016). CsRCI2A and CsRCI2E genes show opposite salt sensitivity reaction due to membrane potential control. Acta Physiol. Plant 38:50.
Kim, H.-S., Park, W., Lim, H.-G., Eom, S., Lee, J.-H., Carlson, J. E., et al. (2019). NaCl-induced CsRCI2E and CsRCI2F interact with aquaporin CsPIP2; 1 to reduce water transport in Camelina sativa L. Biochem. Biophys. Res. Commun. 513, 213–218. doi: 10.1016/j.bbrc.2019.03.208
Kim, S.-H., Kim, J.-Y., Kim, S.-J., An, K.-S., An, G., and Kim, S.-R. (2007). Isolation of cold stress-responsive genes in the reproductive organs, and characterization of the OsLti6b gene from rice (Oryza sativa L.). Plant Cell Rep. 26, 1097–1110. doi: 10.1007/s00299-006-0297-0
Kim, Y.-O., Lim, H.-G., Kim, H.-S., and Ahn, S.-J. (2020). Overexpression of Cs RCI2H enhances salt tolerance in Camelina sativa (L.). Plant Biotechnol. Rep. 14, 439–449. doi: 10.1007/s11816-020-00622-9
Kleine-Vehn, J., Wabnik, K., Martiniere, A., Łangowski, Ł, Willig, K., Naramoto, S., et al. (2011). Recycling, clustering, and endocytosis jointly maintain PIN auxin carrier polarity at the plasma membrane. Mol. Syst. Biol. 7:540. doi: 10.1038/msb.2011.72
Kroemer, K., Reski, R., and Frank, W. (2004). Abiotic stress response in the moss Physcomitrella patens: evidence for an evolutionary alteration in signaling pathways in land plants. Plant Cell Rep. 22, 864–870. doi: 10.1007/s00299-004-0785-z
Kwok, A. C., Zhang, F., Ma, Z., Chan, W. S., Yu, V. C., Tsang, J. S., et al. (2020). Functional responses between PMP3 small membrane proteins and membrane potential. Environ. Microbiol. 22, 3066–3080. doi: 10.1111/1462-2920.15027
Lauwers, E., Erpapazoglou, Z., Haguenauer-Tsapis, R., and André, B. (2010). The ubiquitin code of yeast permease trafficking. Trends Cell Biol. 20, 196–204. doi: 10.1016/j.tcb.2010.01.004
Li, X., Wang, X., Yang, Y., Li, R., He, Q., Fang, X., et al. (2011). Single-molecule analysis of PIP2; 1 dynamics and partitioning reveals multiple modes of Arabidopsis plasma membrane aquaporin regulation. Plant Cell 23, 3780–3797.
Liu, B., Feng, D., Zhang, B., Mu, P., Zhang, Y., He, Y., et al. (2012). Musa paradisica RCI complements AtRCI and confers Na+ tolerance and K+ sensitivity in Arabidopsis. Plant Sci. 184, 102–111. doi: 10.1016/j.plantsci.2011.12.004
Liu, S., Lee, H., Kang, P.-S., Huang, X., Yim, J. H., Lee, H. K., et al. (2010). Complementary DNA library construction and expressed sequence tag analysis of an Arctic moss, Aulacomnium turgidum. Polar Biol. 33, 617–626. doi: 10.1007/s00300-009-0737-8
Long, R., Zhang, F., Li, Z., Li, M., Cong, L., Kang, J., et al. (2015). Isolation and functional characterization of salt-stress induced RCI2-like genes from Medicago sativa and Medicago truncatula. J. Plant Res. 128, 697–707. doi: 10.1007/s10265-015-0715-x
Luu, D. T., Martiniere, A., Sorieul, M., Runions, J., and Maurel, C. (2012). Fluorescence recovery after photobleaching reveals high cycling dynamics of plasma membrane aquaporins in Arabidopsis roots under salt stress. Plant J. 69, 894–905. doi: 10.1111/j.1365-313x.2011.04841.x
MacDonald, C., Stringer, D. K., and Piper, R. C. (2012). Sna 3 Is an Rsp 5 adaptor protein that relies on ubiquitination for its MVB sorting. Traffic 13, 586–598. doi: 10.1111/j.1600-0854.2011.01326.x
Maizel, A., von Wangenheim, D., Federici, F., Haseloff, J., and Stelzer, E. H. K. (2011). High-resolution live imaging of plant growth in near physiological bright conditions using light sheet fluorescence microscopy. Plant J. 68, 377–385. doi: 10.1111/j.1365-313x.2011.04692.x
Martiniere, A., Fiche, J. B., Smokvarska, M., Mari, S., Alcon, C., Dumont, X., et al. (2019). Osmotic stress activates two reactive oxygen species pathways with distinct effects on protein nanodomains and diffusion. Plant Physiol. 179, 1581–1593. doi: 10.1104/pp.18.01065
Medina, J., Ballesteros, M. L., and Salinas, J. (2007). Phylogenetic and functional analysis of Arabidopsis RCI2 genes. J. Exp. Bot. 58, 4333–4346. doi: 10.1093/jxb/erm285
Medina, J., Catalá, R., and Salinas, J. (2001). Developmental and stress regulation of RCI2A andRCI2B, two cold-inducible genes of Arabidopsis encoding highly conserved hydrophobic proteins. Plant Physiol. 125, 1655–1666. doi: 10.1104/pp.125.4.1655
Mitsuya, S., Taniguchi, M., Miyake, H., and Takabe, T. (2005). Disruption of RCI2A leads to over-accumulation of Na+ and increased salt sensitivity in Arabidopsis thaliana plants. Planta 222:1001. doi: 10.1007/s00425-005-0043-9
Mitsuya, S., Taniguchi, M., Miyake, H., and Takabe, T. (2006). Overexpression of RCI2A decreases Na+ uptake and mitigates salinity−induced damages in Arabidopsis thaliana plants. Physiol. Plant 128, 95–102. doi: 10.1111/j.1399-3054.2006.00714.x
Morsy, M. R., Almutairi, A. M., Gibbons, J., Yun, S. J., and Benildo, G. (2005). The OsLti6 genes encoding low-molecular-weight membrane proteins are differentially expressed in rice cultivars with contrasting sensitivity to low temperature. Gene 344, 171–180. doi: 10.1016/j.gene.2004.09.033
Navarre, C., and Goffeau, A. (2000). Membrane hyperpolarization and salt sensitivity induced by deletion of PMP3, a highly conserved small protein of yeast plasma membrane. EMBO J. 19, 2515–2524. doi: 10.1093/emboj/19.11.2515
NDong, C., Ouellet, F., Houde, M., and Sarhan, F. (1997). Gene expression during cold acclimation in strawberry. Plant Cell Physiol. 38, 863–870. doi: 10.1093/oxfordjournals.pcp.a029245
Nylander, M., Heino, P., Helenius, E., Palva, E. T., Ronne, H., and Welin, B. V. (2001). The low-temperature-and salt-induced RCI2A gene of Arabidopsis complements the sodium sensitivity caused by a deletion of the homologous yeast gene SNA1. Plant Mol. Biol. 45, 341–352.
Pan, J., Fujioka, S., Peng, J., Chen, J., Li, G., and Chen, R. (2009). The E3 ubiquitin ligase SCFTIR1/AFB and membrane sterols play key roles in auxin regulation of endocytosis, recycling, and plasma membrane accumulation of the auxin efflux transporter PIN2 in Arabidopsis thaliana. Plant Cell 21, 568–580. doi: 10.1105/tpc.108.061465
Pou, A., Jeanguenin, L., Milhiet, T., Batoko, H., Chaumont, F., and Hachez, C. (2016). Salinity-mediated transcriptional and post-translational regulation of the Arabidopsis aquaporin PIP2; 7. Plant Mol. Biol. 92, 731–744. doi: 10.1007/s11103-016-0542-z
Renard, H. F., Demaegd, D., Guerriat, B., and Morsomme, P. (2010). Efficient ER exit and vacuole targeting of yeast Sna2p require two tyrosine−based sorting motifs. Traffic 11, 931–946. doi: 10.1111/j.1600-0854.2010.01070.x
Rocha, P. S. (2016). Plant abiotic stress-related RCI2/PMP3s: multigenes for multiple roles. Planta 243, 1–12. doi: 10.1007/s00425-015-2386-1
Rodriguez-Furlan, C., Minina, E. A., and Hicks, G. R. (2019). Remove, recycle, degrade: regulating plasma membrane protein accumulation. Plant Cell. 31, 2833–2854. doi: 10.1105/tpc.19.00433
Shi, H., Ishitani, M., Kim, C., and Zhu, J.-K. (2000). The Arabidopsis thaliana salt tolerance gene SOS1 encodes a putative Na+/H+ antiporter. Proc. Natl. Acad. Sci. U.S.A. 97, 6896–6901. doi: 10.1073/pnas.120170197
Sivankalyani, V., Geetha, M., Subramanyam, K., and Girija, S. (2015). Ectopic expression of Arabidopsis RCI2A gene contributes to cold tolerance in tomato. Transgenic Res. 24, 237–251. doi: 10.1007/s11248-014-9840-x
Spartz, A. K., Ren, H., Park, M. Y., Grandt, K. N., Lee, S. H., Murphy, A. S., et al. (2014). SAUR inhibition of PP2C-D phosphatases activates plasma membrane H+-ATPases to promote cell expansion in Arabidopsis. Plant Cell 26, 2129–2142. doi: 10.1105/tpc.114.126037
Stawiecka-Mirota, M., Pokrzywa, W., Morvan, J., Zoladek, T., Haguenauer-Tsapis, R., Urban-Grimal, D., et al. (2007). Targeting of Sna3p to the endosomal pathway depends on its interaction with Rsp5p and multivesicular body sorting on its ubiquitylation. Traffic 8, 1280–1296. doi: 10.1111/j.1600-0854.2007.00610.x
Ueda, A., Kathiresan, A., Inada, M., Narita, Y., Nakamura, T., Shi, W., et al. (2004). Osmotic stress in barley regulates expression of a different set of genes than salt stress does. J. Exp. Bot. 55, 2213–2218. doi: 10.1093/jxb/erh242
Ueda, M., Tsutsumi, N., and Fujimoto, M. (2016). Salt stress induces internalization of plasma membrane aquaporin into the vacuole in Arabidopsis thaliana. Biochem. Biophys. Res. Commun. 474, 742–746. doi: 10.1016/j.bbrc.2016.05.028
Wang, C., Yan, X., Chen, Q., Jiang, N., Fu, W., Ma, B., et al. (2013). Clathrin light chains regulate clathrin-mediated trafficking, auxin signaling, and development in Arabidopsis. Plant Cell 25, 499–516. doi: 10.1105/tpc.112.108373
Wang, D., Chen, Y., Wang, Y., and Wang, Z. (2013). Molecular cloning and expression of two plasma membrane protein 3 (SmPMP3) genes from Salvia miltiorrhiza. Russ. J. Plant Physiol. 60, 155–164. doi: 10.1134/s1021443712060179
Wang, X., Xu, M., Gao, C., Zeng, Y., Cui, Y., Shen, W., et al. (2020). The roles of endomembrane trafficking in plant abiotic stress responses. J. Integr. Plant Biol. 62, 55–69. doi: 10.1111/jipb.12895
Wudick, M. M., Li, X., Valentini, V., Geldner, N., Chory, J., Lin, J., et al. (2015). Subcellular redistribution of root aquaporins induced by hydrogen peroxide. Mol. Plant 8, 1103–1114. doi: 10.1016/j.molp.2015.02.017
Xia, L., Marquès-Bueno, M. M., Bruce, C. G., and Karnik, R. (2019). Unusual roles of secretory SNARE SYP132 in plasma membrane H+-ATPase traffic and vegetative plant growth. Plant Physiol. 180, 837–858. doi: 10.1104/pp.19.00266
Yeshvekar, R. K., Nitnavare, R. B., Chakradhar, T., Bhatnagar-Mathur, P., Reddy, M. K., and Reddy, P. S. (2017). Molecular characterization and expression analysis of pearl millet plasma membrane proteolipid 3 (Pmp3) genes in response to abiotic stress conditions. Plant Gene 10, 37–44. doi: 10.1016/j.plgene.2017.05.002
Yu, Y., Cui, Y., Ren, C., Rocha, P., Peng, M., Xu, G., et al. (2016). Transgenic rice expressing a cassava (Manihot esculenta Crantz) plasma membrane gene MePMP3-2 exhibits enhanced tolerance to salt and drought stresses. Genet. Mol. Res. 15:15017336.
Zhao, Y., Tong, H., Cai, R., Peng, X., Li, X., Gan, D., et al. (2014). Identification and characterization of the RCI2 gene family in maize (Zea mays). J. Genet. 93, 655–666. doi: 10.1007/s12041-014-0421-9
Zhou, Y., Ge, L., Li, G., He, P., Yang, Y., and Liu, S. (2020). In silico identification and expression analysis of Rare Cold Inducible 2 (RCI2) gene family in cucumber. J. Plant Biochem. Biotechnol. 29, 56–66. doi: 10.1007/s13562-019-00510-6
Keywords: RCI2, PMP3, NaCl stress, endocytosis, plasma membrane, protein interaction
Citation: Kim H-S, Park W, Lee H-S, Shin J-H and Ahn S-J (2021) Subcellular Journey of Rare Cold Inducible 2 Protein in Plant Under Stressful Condition. Front. Plant Sci. 11:610251. doi: 10.3389/fpls.2020.610251
Received: 25 September 2020; Accepted: 16 December 2020;
Published: 12 January 2021.
Edited by:
Sergey Shabala, University of Tasmania, AustraliaReviewed by:
Doan Trung Luu, Centre National de la Recherche Scientifique (CNRS), FranceRucha Karnik, University of Glasgow, United Kingdom
Copyright © 2021 Kim, Park, Lee, Shin and Ahn. This is an open-access article distributed under the terms of the Creative Commons Attribution License (CC BY). The use, distribution or reproduction in other forums is permitted, provided the original author(s) and the copyright owner(s) are credited and that the original publication in this journal is cited, in accordance with accepted academic practice. No use, distribution or reproduction is permitted which does not comply with these terms.
*Correspondence: Sung-Ju Ahn, YXNqc3VzZUBqbnUuYWMua3I=