- 1UNIROUEN, Laboratoire Glyco-MEV EA4358, Normandie Université, Rouen, France
- 2Unité de Glycobiologie Structurale et Fonctionnelle (UGSF), UMR 8576, CNRS, Université de Lille, Lille, France
The term microalga refers to various unicellular and photosynthetic organisms representing a polyphyletic group. It gathers numerous species, which can be found in cyanobacteria (i.e., Arthrospira) as well as in distinct eukaryotic groups, such as Chlorophytes (i.e., Chlamydomonas or Chlorella) and Heterokonts (i.e., diatoms). This phylogenetic diversity results in an extraordinary variety of metabolic pathways, offering large possibilities for the production of natural compounds like pigments or lipids that can explain the ever-growing interest of industrials for these organisms since the middle of the last century. More recently, several species have received particular attention as biofactories for the production of recombinant proteins. Indeed, microalgae are easy to grow, safe and cheap making them attractive alternatives as heterologous expression systems. In this last scope of applications, the glycosylation capacity of these organisms must be considered as this post-translational modification of proteins impacts their structural and biological features. Although these mechanisms are well known in various Eukaryotes like mammals, plants or insects, only a few studies have been undertaken for the investigation of the protein glycosylation in microalgae. Recently, significant progresses have been made especially regarding protein N-glycosylation, while O-glycosylation remain poorly known. This review aims at summarizing the recent data in order to assess the state-of-the art knowledge in glycosylation processing in microalgae.
Introduction
All microalgae share two common features: they are unicellular and photosynthetic organisms. According to the literature, more than thirty thousand organisms fall into this definition (Guiry, 2012; Rumin et al., 2020). Beside these common features, microalgae species exhibit a broad diversity of morphology, size (ranging from a few to one hundred micrometers), physiology and metabolism. This diversity results from various adaptation strategies allowing them to colonize very different habitats going from freshwaters and oceans to terrestrial environments (Brodie et al., 2017). Microalgae represent a polyphyletic group meaning that they spread in distinct phyla ranging from Cyanobacteria to Eukaryotes (Burki et al., 2020). As far as eukaryotic species are concerned, most of them are distributed in two supergroups: the Archaeplastida and the Chromalveolata lineages arising from series of endosymbiotic events leading to various photosynthetic organisms (Gould et al., 2008). The first endosymbiosis is thought to have arisen between 1 and 1.5 billion years ago. During this event, a cyanobacterium was engulfed by an eukaryotic host cell that gave birth to three photosynthetic lineages: the Chlorophytes, the Rhodophytes and the Glaucophytes, in which cells are characterized by the presence of primary plastids corresponding to the ancestral cyanobacterium. These three photosynthetic lineages form together the Archaeplastida supergroup, also named the “green lineage” (Rodríguez-Ezpeleta et al., 2005). Then, during secondary endosymbiotic events, some of these eukaryotic cells containing a primary plastid were engulfed by another eukaryotic cell, leading to new photosynthetic cells in which photosynthesis occurs in secondary plastids. Organisms that derived from a secondary endosymbiosis involving a rhodophyte as a host cell belongs to the Chromalveolata lineage (Cavalier-Smith, 1999; Keeling, 2009). In addition, a few photosynthetic unicellular organisms are belonging to other Eukaryotic supergroups. For example, the Euglenid group, belonging to the Excavate supergroup, encompasses several freshwater and marine or brackish phototrophic species that are spread out in Euglenales and Eutreptiales, respectively (Vesteg et al., 2019). These phototrophic species are thought to have emerged recently (about 600 million years ago) as the result of a secondary endosymbiosis between an Euglenid host cell and a prasinophyte green alga (Jackson et al., 2018).
Taking advantage of this huge diversity, industrials used microalgae since the 1950’s in various applications ranging from food industry (e.g., pigments extraction; Novoveská et al., 2019) to biofuel production or wastewaters treatments (Oey et al., 2016; Gilmour, 2019; Li et al., 2019). However, the diversity of microalgae metabolisms and the remaining number of unknown species, still represent an untapped potential. This is illustrated through the exponential increase of publications regarding microalgae during the last 10 years: more than 5,700 papers dealing with microalgae have been published in 2018 worldwide representing twice the number of publications in 2010 (Rumin et al., 2020). Furthermore, the advances in genome sequencing technologies allow now access to numerous microalgae genomes (Fu et al., 2019), that facilitate the development of molecular tools for studying metabolic processes in these organisms. Currently, DNA recombinant technology and transgenesis have been successfully implemented in some microalgae. In this context, microalgae have been investigated as emerging industrial platforms for the production of high value-added biopharmaceuticals (Barolo et al., 2020; Dehghani et al., 2020; Rosales-Mendoza et al., 2020). Indeed, microalgae are easy and fast to grow, safe and cheap making them attractive expression systems for the production of therapeutic proteins (Hempel and Maier, 2016; Rosales-Mendoza et al., 2020). Nowadays, the most biological expression systems used for the production of recombinant proteins are bacteria, yeast and mammalian cells (Walsh, 2014). One of the critical issues for the choice of an heterologous system is its capacity of protein glycosylation that is required for the biopharmaceutical biological activity. Apart from efforts to engineer and humanize the N-glycosylation pathway in plants, mammalian cells are currently the only system able to synthesize proteins bearing glycan structures close to the human ones, even if differences might subsist. For example, the sialic acids present in the terminal position of CHO N-glycan structures are linked in α(2, 3) whereas they are linked in α(2, 6) in human N-glycans (Bragonzi et al., 2000). Despite this difference, most of the glycosylated biopharmaceuticals are to date produced in Chinese Hamster Ovary (CHO) cells (O’Flaherty et al., 2017).
The term glycosylation refers to the processes leading to the synthesis of oligosaccharides that are then attached to another molecule like a protein. Glycosylation pathways comprise numerous distinct steps, starting with the cytosolic synthesis of nucleotide-sugars that are used in the Golgi apparatus as donor substrates by specific glycosyltransferases involved in the synthesis of the oligosaccharide moiety. In Eukaryotes, glycoproteins can be distinguished according to the site of glycan attachment on the protein. The attachment of oligosaccharide occurs either on the amide group of an asparagine (Asn) residue (N-glycosylation) or on the hydroxyl group of a serine (Ser), a threonine (Thr), or an hydroxyproline residue (O-glycosylation). Glycans attached to proteins regulate fundamental biological functions such as cell adhesion, molecular trafficking, control of growth, morphogenesis, adaptation to biotic and abiotic stresses and receptor activation (Schjoldager and Clausen, 2012; Varki, 2017). Moreover, glycosylation of proteins is crucial for their half-life, stability, immunogenicity, secretion and biological activity (Lingg et al., 2012; Van Beers and Bardor, 2012; Zhang et al., 2013).
Although these mechanisms are well described in various Eukaryotes like vertebrates (Moremen et al., 2012; Stanley et al., 2017), plants (Nguema-Ona et al., 2014; Strasser, 2016; Schoberer and Strasser, 2018) or insects (Walski et al., 2017), only few studies have been undertaken to investigate the protein glycosylation pathways in microalgae. Recently, significant progresses have been made regarding especially the N-glycosylation in microalgae, while O-glycosylation remain poorly known. This review reports on recent findings and summarizes the current knowledge in the N- and O-glycosylation pathways in microalgae.
N-Glycosylation
General Features of Eukaryotic N-Glycosylation
In Eukaryotes, protein N-glycosylation process can be divided in three major steps: the synthesis of the oligosaccharide moiety on a lipid carrier, called the lipid-linked oligosaccharide (LLO), the transfer of this oligosaccharide precursor on the target protein and the maturation of the protein N-linked glycans. The two first steps occur in the Endoplasmic Reticulum (ER) while the maturation and further elongation of the protein N-glycans take place in the Golgi apparatus.
The assembly of the oligosaccharide moiety requires several enzymes called Asparagine-linked glycosylation (ALG) that act according to well-established sequential steps. It starts on the cytosolic side of the ER with the addition of two N-acetylglucosamine (GlcNAc) residues on a dolichol pyrophosphate (PP-Dol) lipid carrier that is embedded in the ER membrane. A first GlcNAc residue is transferred from UDP-GlcNAc to the PP-Dol by the GlcNAc-1-phosphotransferase ALG7 (also called DPAGT1 in mammals) (Bretthauer, 2009) and then the ALG13/ALG14 complex adds the second GlcNAc residue to form GlcNAc2-PP-Dol (Bickel et al., 2005; Gao et al., 2005) in which the two GlcNAc linked in β(1,4) correspond to the chitobiose core unit (Figure 1). Thereafter, the oligosaccharide is extended sequentially under the activity of several enzymes which add overall five mannose (Man) residues on the chitobiose to form Man5GlcNAc2-PP-Dol. This intermediate structure is translocated across the ER membrane by a flip-flop mechanism involving flippases like RFT1 (Frank et al., 2008). Then, the synthesis continues within the ER luminal compartment with the addition of four other Man residues leading to an oligosaccharide lipid precursor Man9GlcNAc2-PP-Dol thanks to the respective action of ALG3, ALG9, and ALG12. Finally, the glucosyltransferases ALG6, ALG8, and ALG10 transfer three glucose (Glc) residues to build the final structure Glc3Man9GlcNAc2-PP-Dol (Burda and Aebi, 1998; Farid et al., 2011; Bloch et al., 2020; Figure 1A). Afterward, the oligosaccharide moiety is transferred “en bloc” from the precursor onto a specific asparagine residue belonging to the N-glycosylation consensus site Asn-X-Ser/Thr/Cys (where X cannot be a proline) of a newly synthesized protein (Matsui et al., 2011; Schwarz and Aebi, 2011). The transfer occurs either co- or post-translationally.
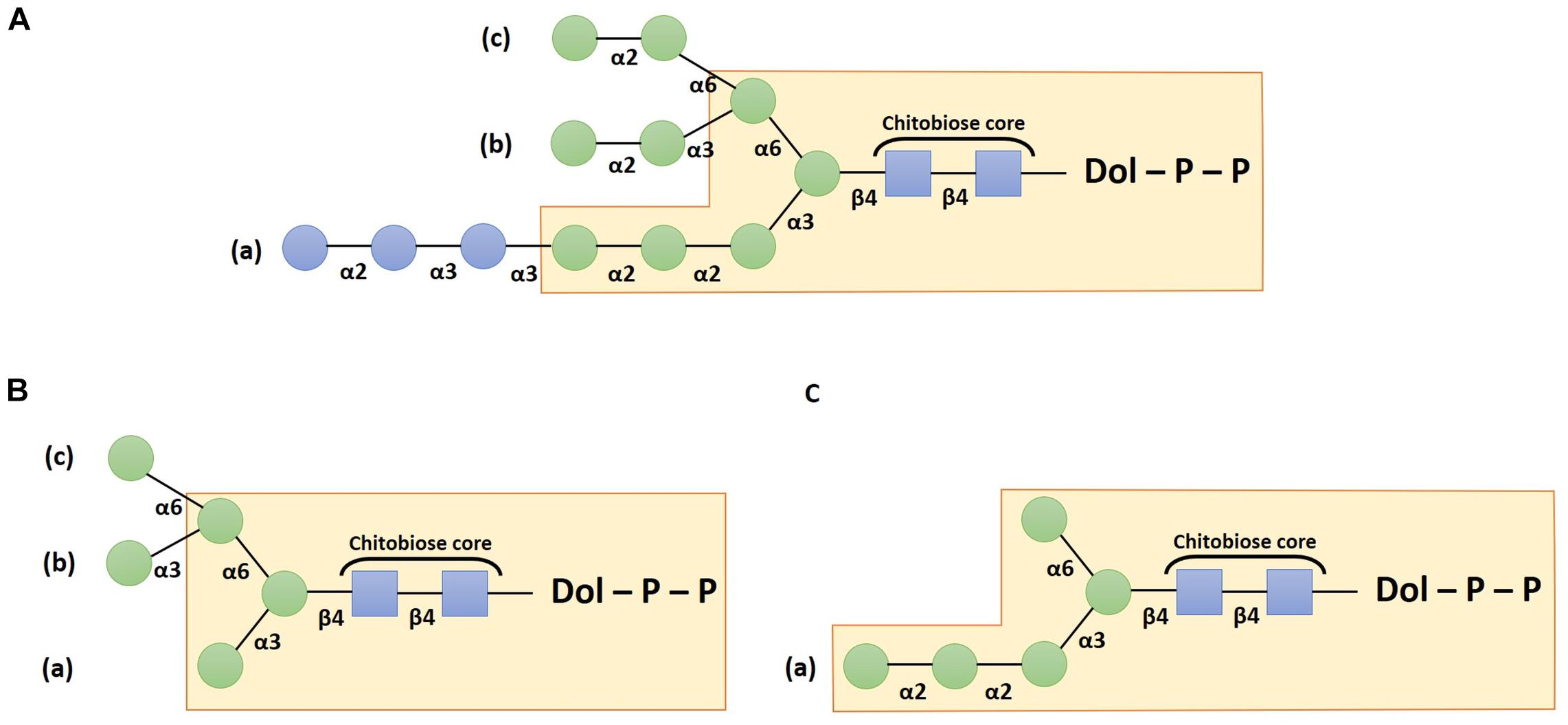
Figure 1. Schemes depicting the structure of the dolichol pyrophosphate oligosaccharide precursor (A), the canonical Man5GlcNAc2 structure (B) and the non-canonical Man5GlcNAc2 structure (C). Structures are drawn according to the Symbol Nomenclature For Glycans (SNFG) (Neelamegham et al., 2019). Blue squares: N-acetylglucosamine residues; green circles: mannose and blue circles: glucose residues.
Once the oligosaccharide has been transferred on the target protein, the two terminal Glc residues are trimmed by the α-glucosidases I and II. The resulting Glc1Man9GlcNAc2 structure is then involved in the quality control by interacting with calnexin or calreticulin chaperone that contribute to glycoprotein folding (for recent reviews please refer to Strasser, 2018; Adams et al., 2019). Thus, the involvement of this N-glycan precursor in the protein quality control cycle justifies that ER processing steps of protein N-linked glycans are highly conserved in most of the Eukaryotes. However, exceptions have been described, especially in parasitic species that lack some ER luminal ALG (Samuelson et al., 2005). For example, in Toxoplasma gondii, Cryptosporidium parvum, and Tetrahymena pyriformis, the luminal ALG responsible for the addition of the four last Man residues are absent. Thus, the synthesis of the oligosaccharide precursor stops prematurely leading to a structure harboring only five mannose residues (Yagodnik et al., 1987; Garénaux et al., 2008; Haserick et al., 2017). Moreover, in Cryptococcus neoformans, Trypanosoma brucei, and Trypanosoma cruzi, ALG6, ALG8 and ALG10 are missing, resulting in a non-glucosylated precursor Man9GlcNAc2-PP-Dol (De La Canal and Parodi, 1987; Parodi, 1993).
Subsequently, correctly folded glycoproteins leave the ER and transit through the Golgi apparatus where α(1,2)-Man residues are first removed (Benyair et al., 2015). This process involves several isoforms of α-mannosidases I and leads to glycoproteins bearing Man5GlcNAc2 structures (Figure 1B). Whereas these early Golgi steps are common in most Eukaryotes, following maturation steps greatly differ according to the Golgi enzyme repertoire, giving rise to various distinct structures between species (Wang et al., 2017). The synthesis of the complex N-glycan structures depends especially on the activity of the β(1,2)-N-acetylglucosaminyltransferase I (GnT I), a key enzyme that transfers a GlcNAc residue on the α(1,3)-Man attached to the chitobiose core. In organisms where N-glycosylation is GnT I-dependent (i.e., plants, insects or mammals), the attachment of the GlcNAc residue is followed by the removing of two outer terminal Man residues by the α-mannosidase II (Rose, 2012). Then, a β(1,2)-N-acetylglucosaminyltransferase II (GnT II) adds another GlcNAc residue on the α(1,6)-Man attached to the N-glycan chitobiose core. The resulting GlcNAc2Man3GlcNAc2 structures can be further “decorated” by diverse glycosyltransferases such as fucosyltransferases, xylosyltransferases, galactosyltransferases, or sialyltransferases. In mammals, supplemental N-acetylglucosaminyltransferases add a third, a fourth and sometimes a fifth GlcNAc leading to the formation of tri- and tetra-antennary N-glycan structures (Wang et al., 2017).
N-Glycosylation Pathways in Microalgae
In microalgae, current knowledge regarding the N-glycosylation processes is available essentially in species belonging to the Chlorophytes (Table 1). Among these, the Chlorophyceae Chlamydomonas reinhardtii and the Trebouxiophycea Chlorella vulgaris have been the most investigated microalgae so far (Mathieu-Rivet et al., 2013; Vanier et al., 2017; Lucas et al., 2018, 2020; Schulze et al., 2018; Mócsai et al., 2019, 2020a, b; Oltmanns et al., 2020). Concerning microalgae with secondary plastids, the structural data available regarding N-glycans have been obtained from the diatom Phaeodactylum tricornutum (Baïet et al., 2011; Vanier et al., 2015; Lucas et al., 2018) and from the Euglenoid Euglena gracilis (De La Canal and Parodi, 1985; O’Neill et al., 2017).
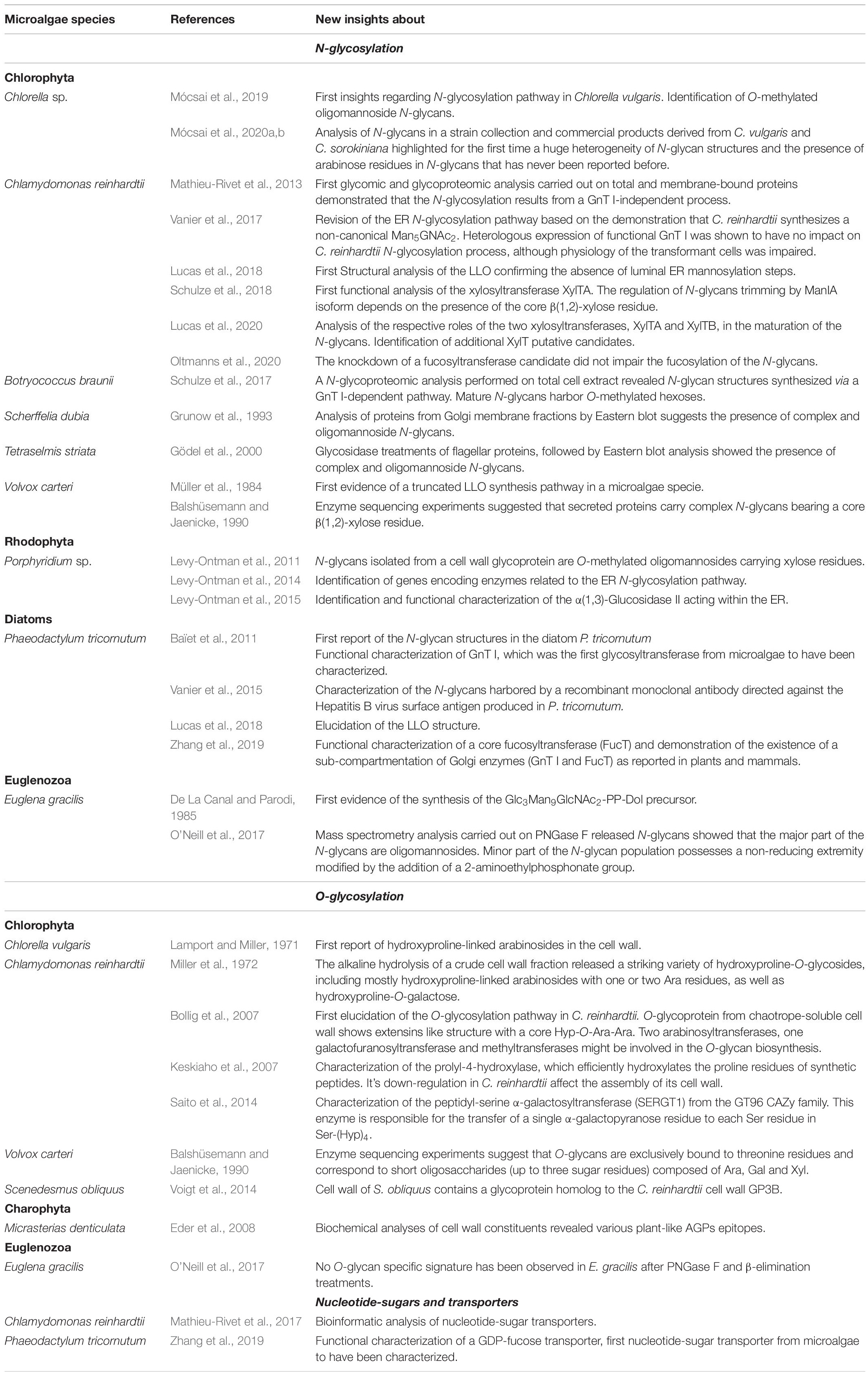
Table 1. Table summarizing published results regarding the N- and O-glycosylation processes in microalgae species.
Synthesis of the Precursor in the ER
In C. reinhardtii, the synthesis of the LLO in the ER stops prematurely as this organism lacks the luminal mannosyltransferases ALG9 and ALG12, as well as the glucosyltransferase ALG10 (Mathieu-Rivet et al., 2013; Vanier et al., 2017). In addition, a LLO-released oligosaccharide Glc3Man5GlcNAc2 moiety has been identified by multistage tandem mass spectrometry (Lucas et al., 2018). This oligosaccharide is transferred on proteins via the oligosaccharyltranferase (OST) complex for which seven homolog subunits have been predicted based on the genome analysis (Mathieu-Rivet et al., 2013). Then, glycosylated proteins are submitted to the control quality cycle that involves α-glucosidases I and II as well as the calnexin and calreticulin chaperones. As a consequence, glycoproteins that exit the ER harbor a non-canonical Man5GlcNAc2 N-glycan exhibiting a linear trimannosyl sequence linked to the β-Man residue (Figure 1C) instead of the canonical Man5GlcNAc2 (Figure 1B). A first study carried out previously in the colonial microalgae Volvox carteri, which is phylogenetically closely related to C. reinhardtii, also highlighted the absence of ER luminal mannosylation steps (Müller et al., 1984). Thus, regarding these features, ER steps in both C. reinhardtii and V. carteri appear to be similar to those described in T. gondii (Garénaux et al., 2008), C. parvum (Haserick et al., 2017) and T. pyriformis (Yagodnik et al., 1987; Table 1).
In contrast, data reported in other microalgae species suggest that the oligosaccharide precursor is synthesized according to a more conventional process. Thus, ER pathways in C. vulgaris and Botryococcus braunii appears to be similar to those described in plants since these microalgae synthesizes oligomannosides ranging from Man5GlcNAc2 to Man9GlcNAc2 (Schulze et al., 2017; Mócsai et al., 2019). In addition, the structural analysis of a cell wall glycoprotein from the red microalgae Porphyridium sp. has revealed the presence of N-glycans containing eight to nine Man residues (Levy-Ontman et al., 2011). These results are consistent with the bioinformatic prediction of genes encoding for ER enzymes (Levy-Ontman et al., 2014). In E. gracilis, labeling assays of protein-linked oligosaccharides have demonstrated that Glc3Man9GlcNAc2-PP-Dol is synthesized before transfer of the carbohydrate moiety on proteins (De La Canal and Parodi, 1985). More recently, O’Neill et al. (2017) have shown using mass spectrometry that major N-glycans in this specie correspond to oligomannoside structures. Proteins from P. tricornutum also carry oligomannoside N-glycans having five to nine Man residues (Baïet et al., 2011). In agreement with this N-glycan profile, P. tricornutum LLO oligosaccharide has been identified as being Glc2Man9GlcNAc2 that is missing the terminal α(1,2)Glc residue (Lucas et al., 2018).
GnT I: To Have or Not to Have
In most Eukaryotes, the Golgi maturation steps depends on the transfer by GnT I of a GlcNAc residue on the arm (a) of the canonical Man5GlcNAc2 (Figure 1B), thus opening the door to the formation of complex-type N-glycans. This key step does not seem to be a general rule on the microalgae that have been studied so far. In P. tricornutum, although no structure harboring terminal GlcNAc residues has been detected in PNGase-released N-glycans, a genomic sequence encoding for a GnT I has been shown to efficiently restore the CHO Lec1 cell line that is deficient for this enzyme activity. This result demonstrated that the paucimannosidic fucosylated structures Man3FucGlcNAc2 identified in the protein N-glycan profiles of P. tricornutum likely results from a GnT I-dependent process (Baïet et al., 2011). In contrast, glycoproteins from C. reinhardtii are processed through a GnT I-independent pathway (Mathieu-Rivet et al., 2013; Vanier et al., 2017). No gene candidate has been identified in the genome by search for sequence homology using functional GnT I from others species as queries. Furthermore, the heterologous expression of GnT I from Arabidopsis thaliana or P. tricornutum did not impact the N-glycan profile of C. reinhardtii proteins. This is consistent with the fact that this green microalga synthesized a non-canonical Man5GlcNAc2 (Figure 1C) that is not an acceptor substrate for GnT I (Vanier et al., 2017). Thus, glycoproteins harboring the non-canonical Man5GlcNAc2 are submitted in the Golgi apparatus to the action of glycosyltransferases responsible for the addition of decorations. Mass spectrometry analyses carried out on C. reinhardtii secreted and membrane-bound proteins have shown that mature N-glycans are partially O-methylated Man3GlcNAc2 to Man5GlcNAc2 substituted by one or two Xyl residues (Mathieu-Rivet et al., 2013), and for a minor part by one fucose residue (Oltmanns et al., 2020). Recently, Schulze et al. (2018) and Lucas et al. (2020) have showed that the first Xyl is linked in β(1,2) to the β-Man via the action of the xylosyltransferase A (XylTA) similarly to the plant xylosylation process. In contrast, the xylosyltransferase B (XylTB) is responsible for the transfer of a second residue on the linear trimannosyl branch of the Man5GlcNAc2 structure. However, although it is clearly established that these two XylT play a major role in the N-glycan xylosylation processing, the remaining presence of structures containing Xyl residues in a double knockdown mutant XylTA × XylTB has suggested that other uncharacterized enzymes could also contribute to the N-glycan xylosylation in C. reinhardtii. The fucosylation mechanism remains uncleared as the analysis of an insertional mutant in which the candidate gene encoding for a putative FucT was disrupted, did not affect N-glycans harboring Fuc residues (Oltmanns et al., 2020). In addition, a bioinformatic analysis of other microalgae genomes showed that other Chlorophyta species like Ostreococcus lucimarinus, Ostreococcus tauri, or V. carteri would lack GnT I enzymatic activity (Mathieu-Rivet et al., 2014), which suggest that the GnT I-independent process described in C. reinhardtii would not be an exception. However, recent structural data obtained in other species indicate that the absence of GnT I is not a common feature in Chlorophyta (Table 1). Indeed, in B. braunii, traces amount of Man5GlcNAc2 bearing a terminal GlcNAc at the non-reducing end has been detected, in addition to the presence of a genomic sequence sharing a strong homology with A. thaliana GnT I (Schulze et al., 2017). In C. vulgaris, an in vitro GlcNAc-transferase assay on N-glycans showed that Man5GlcNAc2 was converted into GlcNAcMan5GlcNAc2 (Mócsai et al., 2019). Moreover, it was shown that the GlcNAcMan5GlcNAc2 synthesized by C. vulgaris was substrate for core 6-fucosyltransferase, which depends on the presence of terminal GlcNAc (Mócsai et al., 2020b). Altogether, this favors the existence of a GnT I-dependent processing of the N-glycans in the Golgi apparatus of these species. In addition, the study of two strain collections from C. vulgaris and Chlorella sorokiniana also revealed heterogeneous N-glycan structures with both arabinose and galactose occurring as furanose as well as pyranose forms (Mócsai et al., 2020a, b), that constitute an unprecedented discovery among the Eukaryotes.
O-Glycosylation
General Features of Eukaryotic O-Glycosylation
As for N-glycosylation, several families of enzymes orchestrate O-glycosylation pathways. Unlike N-glycosylation in which the first ER steps are conserved in most Eukaryotes, the O-glycosylation of proteins encompasses various distinct processes. Some of them start in the ER and continue in the Golgi apparatus, while others occur exclusively in the Golgi apparatus.
O-glycosylation involves an oxygen-carbon bond between the hydroxyl group of a Ser or a Thr residue of the protein and the oligosaccharide chain in mammals (Bennett et al., 2012) while in plants, O-glycosylation occurs essentially in hydroxyproline residue (Hyp; Nguema-Ona et al., 2014; Seifert, 2020). In most eukaryotes including humans, O-glycans do not present a common structure or a consensus sequence. For example, O-glycans in yeasts are composed of multiple Man residues attached to a Ser or a Thr (Schoberer and Strasser, 2018; Barolo et al., 2020). In mammals, most of O-glycans were found on mucins. Mucins represent large glycoproteins with three domains: (i) a cytoplasmic tail; (ii) a single transmembrane spanning region and (iii) an extracellular domain. The extracellular domain contains a repeating peptide motif with numerous proline (Pro), Ser and Thr residues. The first monosaccharide attached to the mucin is usually β-GalNAc but can also be β-GlcNAc, α-GalNAc, α-Man or other monosaccharides (Bennett et al., 2012; Schoberer and Strasser, 2018). More than 20 different UDP-GalNAc polypeptide N-acetylgalactosaminyltransferases can be involved in the GalNAc attachment. This GalNAc is further modified by the stepwise attachment of different monosaccharides such as galactose (Gal), GlcNAc, sialic acid and fucose giving rise to diverse mucin-type core O-glycans that play crucial roles in many biological processes (Schjoldager and Clausen, 2012; Mewono et al., 2015).
In plants, the main O-glycosylated proteins belong to a large group of glycoproteins known as Hydroxyproline-rich-glycoproteins (HRGPs). HRGPs are involved in many aspects of plant growth and development. They consist in a superfamily of plant cell wall proteins that are divided into three major multigene families: the highly glycosylated arabinogalactan proteins (AGPs), the moderately glycosylated extensins (EXTs) and the low glycosylated proline-rich proteins. The O-glycosylation of HRGPs results from two consecutive post-translational modifications involving the hydroxylation of Pro (Hyp) residues by prolyl 4-hydroxylases in the ER and the subsequent O-glycosylation in the Golgi apparatus of some, but not all, Hyp residues by glycosyltransferases before being transported to their final location within or outside the cell (Nguema-Ona et al., 2014; Seifert, 2020). Overall, O-glycan cores in plants present a Gal residue attached to a Ser or an unique arabinose (Ara) residue attached to an Hyp. The monosaccharide being incorporated and the level of glycosylation depends on the glycoproteins families (AGPs, EXTs or proline-rich proteins). The O-glycans of AGPs are composed of short oligoarabinoside chains containing up to four residues and of a larger β(1,3)-linked galactan backbone with β(1,6)-linked side chains containing galactose, arabinose and, sometime fucose, rhamnose, or glucuronic acid. The structure of arabinogalactan chains varies between plant species (Nguema-Ona et al., 2014). EXTs contain several Ser-(Hyp)4 repeats usually O-glycosylated with oligosaccharide chains of up to five arabinose units on each Hyp (Velasquez et al., 2011, 2015; Ogawa-Ohnishi et al., 2013) and a unique galactose on the Ser residue (Saito et al., 2014). O-glycosylated Ser-(Hyp)4 repeat sequences have also been identified in several other EXT-like chimeras and hybrid EXT glycoproteins, such as arabinogalactan protein-EXTs, Pro-rich protein-EXTs, Leu-rich repeat-EXTs, Pro-rich kinases and formins with an extracellular EXT domain (Velasquez et al., 2015). Moreover, Hyp-O-arabinosylation also occurs in single Hyp units in small secreted glycopeptide hormones with up to three arabinose units (Ohyama et al., 2009; Shinohara and Matsubayashi, 2010).
Concerning the enzyme machinery involved in the HRGP synthesis, three groups of arabinosyltransferases (AraTs) have been identified: hydroxyproline O-arabinosyltransferase 1 (HPAT1 to HPAT3), reduced residual arabinose (RRA1 to RRA3) and xyloglucanase 113 (XEG113). These transferases have been demonstrated to be responsible for the sequential addition of the innermost three arabinose residues (Egelund et al., 2007; Ogawa-Ohnishi et al., 2013).
O-Glycosylation in Microalgae
Very few articles related to the O-glycosylation pathways in microalgae are available to date. So far, C. reinhardtii is the main microalga that has been investigated regarding protein O-glycosylation.
Extensin-Like O-Glycoproteins
Bollig et al. (2007) have investigated the structure of linear glycans O-linked to Hyp residues of C. reinhardtii proteins, showing some similarities with plant O-glycans. Indeed, they identified a O-glycan core Hyp-O-Ara-Ara, which is consistent with previous results reported by Miller and coworkers (Miller et al., 1972). This suggests a certain level of conservation of the extensin structures within the green lineage.
O-glycosylated Hyp residues have been identified in chaotrope-soluble glycoproteins, which constitute the vegetative outer cell wall in C. reinhardtii (Bollig et al., 2007). Mass spectrometry and NMR analyses have indicated the presence of mainly Ara and Gal, followed by Glc, Xyl and Man residues (Bollig et al., 2007). Bollig et al. (2007) have demonstrated that glycans O-linked to Hyp residue are composed of a β(1-2)-linked L-Ara disaccharide substituted with galactofuranose (Galf) residues and O-methylation, two modifications not reported in plants (Bollig et al., 2007; Mathieu-Rivet et al., 2017; Barolo et al., 2020; Figure 2). Little information is available concerning the enzymes involved in this process in C. reinhardtii. However, a prolyl-4-hydroxylase has been characterized (Keskiaho et al., 2007). This enzyme efficiently hydroxylates the Pro residues of synthetic peptides and its down-regulation affect the assembly of a proper cell wall, which is consistent with the role of hydroxyproline residues in the attachment of the oligosaccharide moiety (Keskiaho et al., 2007).
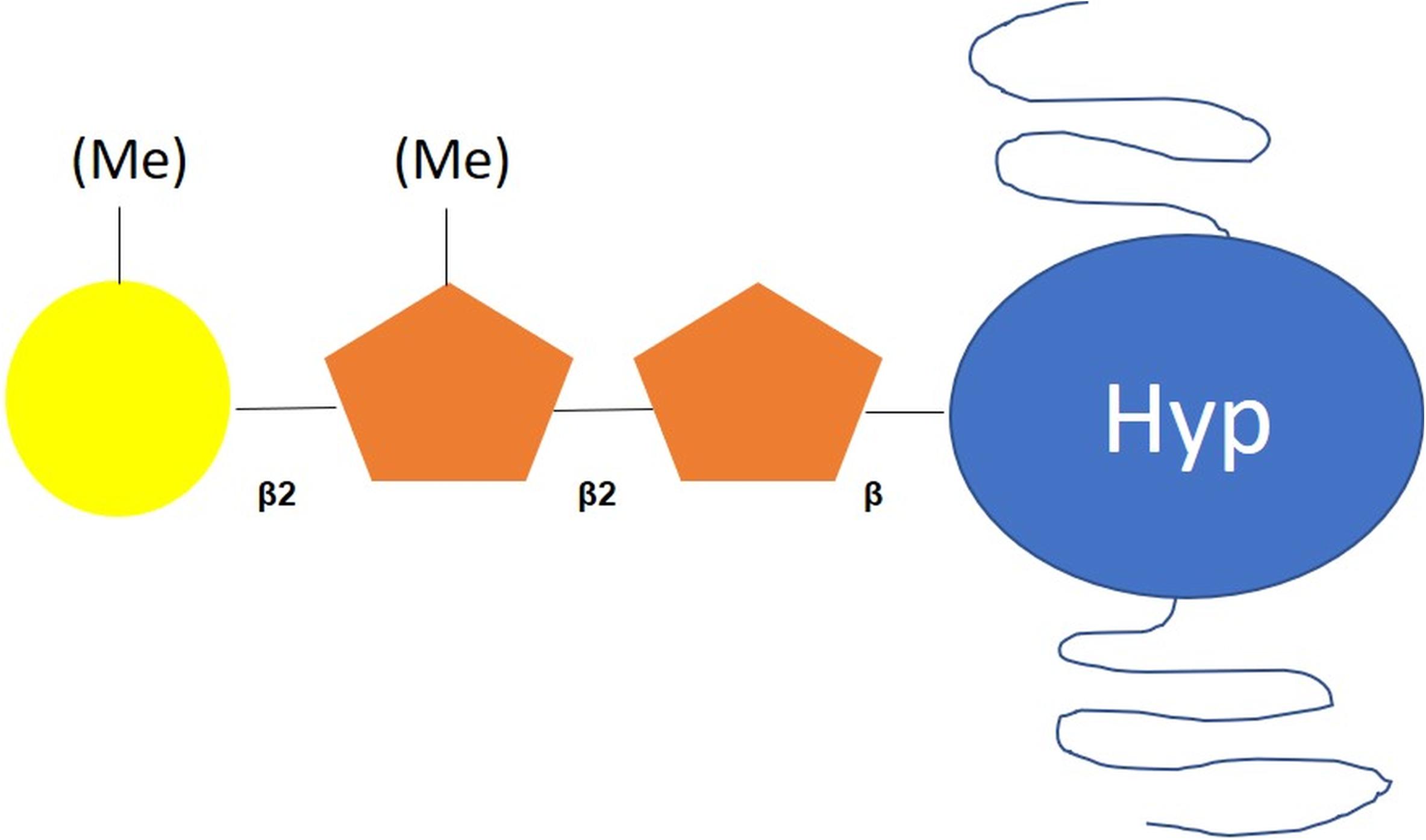
Figure 2. Scheme of O-glycan motif harbored by proteins in C. reinhardtii. According to Bollig et al. (2007), O-glycans attached to C. reinhardtii proteins are composed of a Hyp-O-Ara-Ara core substituted with methylated galactofuranose residues. Hyp: hydroxyproline; orange pentagon: arabinofuranose; yellow circle: galactofuranose, Me: methyl group.
In addition, few papers have reported data regarding the composition of cell wall glycoproteins of others microalgae. Hyp-linked arabinosides have been reported in the green alga C. vulgaris (Lamport and Miller, 1971). Balshüsemann and Jaenicke (1990) have described O-linked oligosaccharides in the glycoprotein pheromones of V. carteri. These short oligosaccharide chains (up to three residues) are composed of Ara, Gal and Xyl bound to Thr residues (Balshüsemann and Jaenicke, 1990). Eder et al. (2008) have detected in the cell wall of the green alga Micrasterias denticulata various plant-like AGPs epitopes by combination of cell imaging and biochemical approaches. Cell-wall glycoprotein number 1, 2, and 3 (GP1, GP2, and GP3, respectively) are hydroxyproline-rich glycoproteins that co-polymerize to form the W6 layer of C. reinhardtii cell wall. W6 layer is one of the three major outer layers of the C. reinhardtii cell wall that can be solubilized from living cells with chaotropes (Monk, 1988). Voigt and collaborators have studied the ultrastructure of cell wall glycoproteins of V. carteri and the green alga Scenedesmus obliquus. A multi-layered cell wall similar to the GP3 of C. reinhardtii has been reported although its proportion in Hyp is considerably lower (Voigt et al., 2014). Glycoproteins similar to GP1 have also been found in other Chlamydomonas species (eugametos and incerta) (Goodenough et al., 1986) but are absent in Volvovaceae Gonium pectoral and V. carteri (Voigt et al., 2014). The chaotrope-soluble cell wall glycoprotein GP1 is the only polypeptide with an even higher proportion of Hyp (35%) occurring in vegetative C. reinhardtii cells (Voigt et al., 2009). In contrast, GP2 and GP3 have been found in all studied Volvovaceae species. Putative homologs of GP3 have also been detected on the cell walls of some Zygnematales using a polyclonal antibody raised against the glycosylated GP3B isoform of C. reinhardtii (Voigt et al., 2014).
Arabinosylation of O-Glycans in Microalgae
Bollig et al. (2007) have proposed that two arabinosyltransferases are responsible for the addition of the first two Ara residues onto Hyp followed by the action of a galactofuranosyltransferase (GalfT) in C. reinhardtii (Figure 2 and Table 1). These two arabinoses are arabinofuranosyl (Araf) rather than arabinopyranosyl (Arap) residues. UDP-L-Arap is first synthesized in the cytosol from UDP-Xyl and is then converted into UDP-L-Araf through the action of a specific mutase (Bollig et al., 2007). An UDP-L-Arap mutase sharing 78% of identity with AtRGP1 that catalyzes the conversion of UDP-L-Arap into UDP-L-Araf in A. thaliana (Rautengarten et al., 2011) has been purified from the cytosol of C. reinhardtii. UDP-L-Arap mutase activity has also been detected in microsomal fraction of C. reinhardtii (Kotani et al., 2013).
Three putative arabinosyltransferases have been predicted in C. reinhardtii’s genome. The Hyp O-arabinosyltransferase HPAT, belonging to the CAZy GT95 family, performs the transfer of a β-linked L-Ara to Hyp. Genes encoding homologous transferases have also been found in the genomes of V. carteri (Ogawa-Ohnishi et al., 2013), B. braunii and C. vulgaris (Barolo et al., 2020). The second arabinosyltransferase, RRA (CAZy GT77), transfers L-Ara residues linked in β(1-2) to the Hyp-linked Ara (Velasquez et al., 2015). Based on the recent in silico analysis reported by Barolo et al. (2020), this putative enzyme is predicted in the genomes of Porphyridium purpureum and C. vulgaris. The third one is XEG113, a xyloglucanase that acts as an arabinosyltransferase. XEG113 homolog sequences have been identified in O. lucimarinus and O. tauri, suggesting the synthesis of closely related extensins in these microalgae (Roycewicz and Malamy, 2014).
Galactosylation of O-Glycans in Microalgae
UDP-Gal is synthesized from UDP-Glc via the epimerization of the C4 hydroxyl group. Whereas several isoforms of UDP-Gal-4-epimerase (UGE) have been found in plants, only one single sequence encoding for a putative GME has been identified in C. reinhardtii (Cre04.g214502, CrGME; Rösti et al., 2007). Moreover, it has been shown that the Gal residues present in C. reinhardtii O-glycans exhibits the unusual furanose conformation (Galf) (Bollig et al., 2007). Bollig et al. (2007) have proposed that the UDP-Galf residues result from the activity of an UDP-galactopyranose mutase (UGM), which is able to convert UDP-galactopyranose (UDP-Galp) into UDP-Galf. UGMs were found in prokaryotes and a few eukaryotes such as C. neoformans or T. cruzi. One gene sequence encoding for a putative UGM is predicted in the genome of C. reinhardtii (Cre06.g272900). This putative C. reinhardtii UGM shares 60% of identity with the UGM from C. neoformans (Beverley et al., 2005). In addition, a gene sequence encoding for a putative GalfT (Cre02.g108200) is predicted in C. reinhardtii genome (Hung et al., 2016). The presence of Galf has also been reported in the glycosylated toxin “prymnesium” extracted from a red tide microalga Prymnesium parvum (Binzer et al., 2019). Genes encoding putative UGM are predicted in JGI phytozome 13 in other microalgae, such as V. carteri, B. braunii (Chlorococcales), Coccomyxa subellipsoidea (Chlorophytes), Chromochloris zofingiensis, and Dunaliella salina, suggesting the presence of Galf residues on the glycans of these microalgae (unpublished data, personal communication).
One the other hand, a peptidyl-serine α-galactosyltransferase, named SERGT1, has been characterized in C. reinhardtii by Saito et al. (2014). This enzyme has been purified from an endosomal fraction and its galactosyltransferase activity has been confirmed by an in vitro assay. These results revealed that SERGT1 transfer the single α-galactopyranose residue to Ser residues in Ser-(Hyp)4 motifs of EXT, suggesting that O-glycosylation of Ser residues can occur in C. reinhardtii.
Other O-Glycosylation Types in Microalgae
Other O-glycosylation types might exist in microalgae. Recently, using computational analysis of available microalgae genomes, Barolo and collaborators have searched for putative candidates involved in protein O-glycosylation by comparison with genes encoding enzymes of O-glycan pathways in both humans (Homo sapiens) and plants (A. thaliana) (Barolo et al., 2020). Through this work, it was shown that C. reinhardtii exhibits an enzyme repertoire that possess a putative O-fucosyltransferase (POFUT 1) that could be involved in protein O-fucosylation. This enzyme is also predicted in other microalgae such as P. tricornutum, P. purpureum, Nannochloropsis gaditana, B. braunii, and C. vulgaris (Barolo et al., 2020). These authors have also highlighted the presence of two putative O-mannosyltransferase 1 and 2 (POMT1 and POMT2) activities in P. purpureum genome suggesting that O-mannosylation of proteins occurs in this microalga as reported in humans and in the yeast, Saccharomyces cerevisiae (Barolo et al., 2020). In addition, a putative xylosyltransferase 1 (XylT 1) was found in the genomes of P. tricornutum and N. gaditana, although the enzymatic activity was not confirmed experimentally. This suggests a possible O-xylosylation in these two microalgae (Barolo et al., 2020).
Methylation of Glycans in Microalgae
Methylation of glycans has been found in the animal kingdom only in worms and mollusks, whereas it is more frequently present in some species of bacteria, fungi and algae (Staudacher, 2012). Methylation has been reported in both N- and O-glycans in microalgae. Indeed, O-methylation of N-glycans appears to be a common feature in microalgae species from Archaeplastidae, although it has never been reported in plants. Indeed, Mócsai et al. (2019) have shown that oligomannosidic structures are O-methylated in C. vulgaris (Mócsai et al., 2019). In addition, O-methylated N-glycans have also been detected in B. braunii (Schulze et al., 2017) and C. reinhardtii (Mathieu-Rivet et al., 2013; Vanier et al., 2017; Lucas et al., 2020). Moreover, the biochemical analysis of the 66 kDa cell wall glycoprotein of the Rhodophyta Porphyridium sp. revealed the presence of methylated N-glycans (Levy-Ontman et al., 2011).
As far as O-glycans in microalgae are concerned, Bollig et al. (2007) proposed that two methytransferases specific to C. reinhardtii perform methylation of some Gal and Ara residues, which corresponds to the final modification of the protein O-glycans in this organism. To the best of our knowledge, none of the enzymes involved in the methylation process has been characterized and the role of the methylation in both N- and O-glycans remains unknown. In this context, authors have suggested that methylation can confer a protective role to the mature glycans. For example, Wohlschlager et al. (2014) have suggested that O-methylated glycans constitute a conserved epitope for the fungal and animal innate immune system. As glycans carrying this modification are present in bacteria, worms, and mollusks, this epitope represents a hitherto unknown target that is recognized by the immune system. Recently, Mócsai et al. (2019) also highlighted that O-methylated N-glycans are possibly immunogenic. Therefore, this has to be taken into account if pharmaceutical glycoproteins are produced using chlorophytes such as C. vulgaris as a cell biofactory. To solve this issue, the authors proposed to identify the O-methyltransferase acting on terminal mannose residues and to knockout this enzyme in the future (Mócsai et al., 2019). Such knockout lines could also be used to answer the scientific question of the biological purpose of N-glycan methylation.
Conclusion and Perspectives
To date, little information is available regarding the N- and O-glycosylation pathways and their regulation in microalgae. Even if the knowledge regarding these protein post-translational modifications has been extended recently, significant efforts remain to be done to characterize these processes in microalgae, especially in the context of using microalgae as cell biofactories where N- and O-glycosylation pathways remain essential for the biological activities and stability of recombinant proteins (Lingg et al., 2012; Zhang et al., 2013).
In the context of using microalgae as a biopharmaceutical platform for the production of recombinant proteins dedicated to therapeutic applications in humans, it will be crucial to unravel the protein glycosylation pathways and then optimize them in order to mimic human-type N- and O-glycans through metabolic engineering (Dumontier et al., 2018; Barolo et al., 2020; Rosales-Mendoza et al., 2020). This represents an important challenge for the next decades. However, it would benefit from the recent development of genome-editing tools in microalgae (Daboussi et al., 2014; Mussgnug, 2015; Baek et al., 2016; Nymark et al., 2016; Shin et al., 2016; Wang et al., 2016; Huang and Daboussi, 2017; Dumontier et al., 2018; Slattery et al., 2018; Guzmán-Zapata et al., 2019; Fabris et al., 2020; Moosburner et al., 2020; Park et al., 2020). Moreover, several recent studies carried out in plants have highlighted the feasibility of N- and O-glycosylation metabolic engineering for the production of humanized recombinant N- and O-glycoproteins in transgenic plants including for example the production of recombinant IgA1 with defined human-type N- and O-linked glycans (Bakker et al., 2001; Paccalet et al., 2007; Vézina et al., 2009; Castilho et al., 2010; Castilho and Steinkellner, 2012; Yang et al., 2012; Dicker et al., 2016; Kallolimath et al., 2016).
Author Contributions
NM-B, EM-R, and MB conceptualized and wrote the manuscript. M-LW-B and PL corrected the manuscript. MB coordinated the work. All authors have read and agreed on the manuscript prior to its submission.
Funding
Current work is funded by the European Union’s Horizon 2020 Research and Innovation Program under Grant Agreement 774078 (Pharma-Factory). M-LW-B’s Ph.D. fellowship is granted by the European Union’s Horizon 2020 Research and Innovation Program under Grant Agreement 774078 (Pharma-Factory). The funding bodies were not involved in the design of the study, collection and interpretation of data nor in the writing of the manuscript.
Conflict of Interest
The authors declare that the research was conducted in the absence of any commercial or financial relationships that could be construed as a potential conflict of interest.
The reviewer FA declared a past co-authorship with one of the authors MB to the handling editor.
Acknowledgments
The authors thank both the University of Rouen Normandie and Normandie University for their support.
References
Adams, B. M., Oster, M. E., and Hebert, D. N. (2019). Protein Quality Control in the Endoplasmic Reticulum. Protein J. 38, 317–329. doi: 10.1007/s10930-019-09831-w
Baek, K., Kim, D. H., Jeong, J., Sim, S. J., Melis, A., Kim, J. S., et al. (2016). DNA-free two-gene knockout in Chlamydomonas reinhardtii via CRISPR-Cas9 ribonucleoproteins. Sci. Rep. 6:30620. doi: 10.1038/srep30620Nymark
Baïet, B., Burel, C., Saint-Jean, B., Louvet, R., Menu-Bouaouiche, L., Kiefer-Meyer, M.-C., et al. (2011). N-glycans of Phaeodactylum tricornutum diatom and functional characterization of its N-acetylglucosaminyltransferase I enzyme. J. Biol. Chem. 286, 6152–6164. doi: 10.1074/jbc.M110.175711
Bakker, H., Bardor, M., Molthoff, J. W., Gomord, V., Elbers, I., Stevens, L. H., et al. (2001). Galactose-extended glycans of antibodies produced by transgenic plants. Proc. Natl. Acad. Sci. U S A. 98, 2899–2904. doi: 10.1073/pnas.031419998
Balshüsemann, D., and Jaenicke, L. (1990). The oligosaccharides of the glycoprotein pheromone of Volvox carteri f. nagariensis Iyengar (Chlorophyceae). Eur. J. Biochem. 192, 231–237. doi: 10.1111/j.1432-1033.1990.tb19220.x
Barolo, L., Abbriano, R. M., Commault, A. S., George, J., Kahlke, T., et al. (2020). Perspectives for glyco-engineering of recombinant biopharmaceuticals from microalgae. Cells 9:633. doi: 10.3390/cells9030633
Bennett, E. P., Mandel, U., Clausen, H., Gerken, T. A., Fritz, T. A., and Tabak, L. A. (2012). Control of mucin-type O-glycosylation: a classification of the polypeptide GalNAc-transferase gene family. Glycobiology 22, 736–756. doi: 10.1093/glycob/cwr182
Benyair, R., Ogen-Shtern, N., and Lederkremer, G. Z. (2015). Glycan regulation of ER-associated degradation through compartmentalization. Semin. Cell Dev. Biol. 41, 99–109. doi: 10.1016/j.semcdb.2014.11.006
Beverley, S. M., Showalter, M., Griffith, C. L., Doering, T. L., et al. (2005). Eukaryotic UDP-galactopyranose mutase (GLF gene) in microbial and metazoal pathogens. Eukaryot. Cell 4, 1147–1154. doi: 10.1128/EC.4.6.1147-1154.2005
Bickel, T., Lehle, L., Schwarz, M., Aebi, M., and Jakob, C. A. (2005). Biosynthesis of lipid-linked oligosaccharides in Saccharomyces cerevisiae: Alg13p and Alg14p form a complex required for the formation of GlcNAc(2)-PP-dolichol. J. Biol. Chem. 280, 34500–34506. doi: 10.1074/jbc.m506358200
Binzer, S. B., Svenssen, D. K., Daugbjerg, N., Alves-de-Souza, C., Pinto, E., Hansen, P. J., et al. (2019). A-, B- and C-type prymnesins are clade specific compounds ans chemotaxonomic markers in Prymnesium parvum. Harmful Algae 81, 10–17. doi: 10.1016/j.hla.2018.11.010
Bloch, J. S., Pesciullesi, G., Boilevin, J., Nosol, K., Irobalieva, R. N., Darbre, T., et al. (2020). Structure and mechanism of the ER-based glucosyltransferase ALG6. Nature 579, 443–447. doi: 10.1038/s41586-020-2044-z
Bollig, K., Lamshöft, M., Schweimer, K., Marner, F.-J., Budzikiewicz, H., and Waffenschmidt, S. (2007). Structural analysis of linear hydroxyproline-bound O-glycans of Chlamydomonas reinhardtii-conservation of the inner core in Chlamydomonas and land plants. Carbohydr. Res. 342, 2557–2566. doi: 10.1016/j.carres.2007.08.008
Bragonzi, A., Distefano, G., Buckberry, L. D., Acerbis, G., Foglieni, C., Lamotte, D., et al. (2000). A new Chinese hamster ovary cell line expressing alpha2,6-sialyltransferase used as universal host for the production of human-like sialylated recombinant glycoproteins. Biochim. Biophys. Acta 1474, 273–282. doi: 10.1016/S0304-4165(00)00023-4
Bretthauer, R. K. (2009). Structure, expression, and regulation of UDP-GlcNAc: dolichol phosphate GlcNAc-1-phosphate transferase (DPAGT1). Curr. Drug Targets 10, 477–482. doi: 10.2174/138945009788488369
Brodie, J., Chan, C. X., De Clerck, O., Cock, J. M., Coelho, S. M., Gachon, C., et al. (2017). The Algal Revolution. Trends Plant Sci. 22, 726–738. doi: 10.1016/j.tplants.2017.05.005
Burda, P., and Aebi, M. (1998). The ALG10 locus of Saccharomyces cerevisiae encodes the alpha-1,2 glucosyltransferase of the endoplasmic reticulum: the terminal glucose of the lipid-linked oligosaccharide is required for efficient N-linked glycosylation. Glycobiology 8, 455–462. doi: 10.1093/glycob/8.5.455
Burki, F., Roger, A. J., Brown, M. W., and Simpson, A. G. B. (2020). The New Tree of Eukaryotes. Trends Ecol. Evol. 35, 43–55. doi: 10.1016/j.tree.2019.08.008
Castilho, A., and Steinkellner, H. (2012). Glyco-engineering in plants to produce human-like N-glycan structures. Biotechnol. J. 7, 1088–1098. doi: 10.1002/biot.201200032
Castilho, A., Strasser, R., Stadlmann, J., Grass, J., Jez, J., et al. (2010). In planta protein sialylation through overexpression of the respective mammalian pathway. J. Biol. Chem. 285, 15923–15930. doi: 10.1074/jbc.M109.088401
Cavalier-Smith, T. (1999). Principles of protein and lipid targeting in secondary symbiogenesis: euglenoid, dinoflagellate, and sporozoan plastid origins and the eukaryote family tree. J. Eukaryot. Microbiol. 46, 347–366. doi: 10.1111/j.1550-7408.1999.tb04614.x
Daboussi, F., Leduc, S., Maréchal, A., Dubois, G., Guyot, V., Perez-Michaut, C., et al. (2014). Genome engineering empowers the diatom Phaeodactylum tricornutum for biotechnology. Nat. Commun. 5:3831. doi: 10.1038/ncomms4831
De La Canal, L., and Parodi, A. J. (1985). Glycosylation of proteins in the protozoan Euglena gracilis. Compar. Biochem. Physiol. Part B 81, 803–805. doi: 10.1016/0305-0491(85)90409-2
De La Canal, L., and Parodi, A. J. (1987). Synthesis of dolichol derivatives in trypanosomatids. Characterization of enzymatic patterns. J. Biol. Chem. 262, 11128–11133.
Dehghani, J., Adibkia, K., Movafeghi, A., Maleki-Kakelar, H., Saeedi, N., and Omidi, Y. (2020). Towards a new avenue for producing therapeutic proteins: Microalgae as a tempting green biofactory. Biotechnol. Adv. 40:107499. doi: 10.1016/j.biotechadv.2019.107499
Dicker, M., Tschofen, M., Maresch, D., König, J., Juarez, P., et al. (2016). Transient glyco-engineering to produce recombinant IgA1 with defined N- and O-glycans in plants. Front. Plant Sci. 29:18. doi: 10.3389/fpls.2016.00018
Dumontier, R., Mareck, A., Mati-Baouche, N., Lerouge, P., and Bardor, M. (2018). Toward Future engineering of the N-glycosylation pathways in microalgae for optimizing the Production of biopharmaceuticals. Microalgal Biotech. IntechOpen 2018, 177–193. doi: 10.5772/intechopen.73401
Eder, M., Tenhaken, R., Driouich, A., and Lütz-Meindl, U. (2008). Occurrence and characterization of arabinogalactan-like proteins and hemicelluloses in Micrasterias (Streptophyta). J. Phycol. 44, 1221–1234. doi: 10.1111/j.1529-8817.2008.00576.x
Egelund, J., Obel, N., Ulvskov, P., Geshi, N., Pauly, et al. (2007). Molecular characterization of two Arabidopsis thaliana glycosyltransferase mutants, rra1 and rra2, which have a reduced residual arabinose content in a polymer tightly associated with the cellulosic wall residue. Plant Mol. Biol. 64, 439–449. doi: 10.1007/s11103-007-9162-y
Fabris, M., Abbriano, R. M., Pernice, M., Sutherland, D. L., Commault, A. S., et al. (2020). Emerging technologies in algal biotechnology: Toward the establishment of a sustainable, algae-based bioeconomy. Front. Plant Sci. 11:279. doi: 10.3389/fpls.2020.00279
Farid, A., Pabst, M., Schoberer, J., Altmann, F., Glössl, J., and Strasser, R. (2011). Arabidopsis thaliana alpha1,2-glucosyltransferase (ALG10) is required for efficient N-glycosylation and leaf growth. Plant J. 68, 314–325. doi: 10.1111/j.1365-313X.2011.04688.x
Frank, C. G., Sanyal, S., Rush, J. S., Waechter, C. J., and Menon, A. K. (2008). Does Rft1 flip an N-glycan lipid precursor? Nature 31:454. doi: 10.1038/nature07165
Fu, W., Nelson, D. R., Mystikou, A., Daakour, S., and Salehi-Ashtiani, K. (2019). Advances in microalgal research and engineering development. Curr. Opin. Biotechnol. 59, 157–164. doi: 10.1016/j.copbio.2019.05.013
Gao, X. D., Tachikawa, H., Sato, T., Jigami, Y., and Dean, N. (2005). Alg14 recruits Alg13 to the cytoplasmic face of the endoplasmic reticulum to form a novel bipartite UDP-N-acetylglucosamine transferase required for the second step of N-linked glycosylation. J. Biol. Chem. 280, 36254–36262. doi: 10.1074/jbc.M507569200
Garénaux, E., Shams-Eldin, H., Chirat, F., Bieker, U., Schmidt, J., Michalski, J.-C., et al. (2008). The dual origin of Toxoplasma gondii N-glycans. Biochemistry 47, 12270–12276. doi: 10.1021/bi801090a
Gilmour, D. J. (2019). Microalgae for biofuel production. Adv. Appl. Microbiol. 109, 1–30. doi: 10.1016/bs.aambs.2019.10.001
Gödel, S., Becker, B., and Melkonian, M. (2000). Flagellar membrane proteins of Tetraselmis striata butcher (Chlorophyta). Protist 151, 147–159. doi: 10.1078/1434-4610-00015
Goodenough, U. W., Gebhart, B., Mecham, R. P., and Heuser, J. (1986). Crystals of the Chlamydomonas reinhardtii cell wall: polymerization, depolymerization, and purification of the glycoprotein mono-mers. J. Cell Biol. 103, 405–417. doi: 10.1083/jcb.103.2.405
Gould, S. B., Waller, R. F., and McFadden, G. I. (2008). Plastid evolution. Annu. Rev. Plant Biol. 59, 491–517. doi: 10.1146/annurev.arplant.59.032607.092915
Grunow, A., Becker, B., and Melkonian, M. (1993). Isolation and characterization of the Golgi apparatus of a flagellate scaly green alga. Eur. J. Cell Biol. 61, 10–20.
Guiry, M. D. (2012). How many species of algae are there ? J. Phycol. 48, 1057–1063. doi: 10.1111/j.1529-8817.2012.01222.x
Guzmán-Zapata, D., Sandoval-Vargas, J. M., Macedo-Osorio, K. S., Salgado-Manjarrez, E., Castrejón-Flores, J. L., et al. (2019). Efficient editing of the nuclear APT reporter gene in Chlamydomonas reinhardtii via expression of a CRISPR-Cas9 module. Int. J. Mol. Sci. 20:1247. doi: 10.3390/ijms20051247
Haserick, J. R., Leon, D. R., Samuelson, J., and Costello, C. E. (2017). Asparagine-Linked Glycans of Cryptosporidium parvum Contain a Single Long Arm, Are Barely Processed in the Endoplasmic Reticulum (ER) or Golgi, and Show a Strong Bias for Sites with Threonine. Mol. Cell. Proteomics. 16(Suppl. 1), S42–S53. doi: 10.1371/journal.pone.0182395
Hempel, F., and Maier, U. G. (2016). Microalgae as Solar-Powered Protein Factories. Adv. Exp. Med. Biol. 896, 241–262. doi: 10.1007/978-3-319-27216-0_16
Huang, W., and Daboussi, F. (2017). Genetic and metabolic engineering in diatoms. Philos. Trans. R. Soc. Lond. B Biol. Sci. 372:20160411. doi: 10.1098/rstb.2016.0411
Hung, C. H., Kanehara, K., and Nakamura, Y. (2016). Isolation and characterization of a mutant defective in triacylglycerol accumulation in nitrogen-starved Chlamydomonas reinhardtii. Biochim. Biophys. Acta Mol. Cell. Biol. Lipids 1861, 1282–1293. doi: 10.1016/j.bbalip.2016.04.001
Jackson, C., Knoll, A. H., Chan, C. X., and Verbruggen, H. (2018). Plastid phylogenomics with broad taxon sampling further elucidates the distinct evolutionary origins and timing of secondary green plastids. Sci. Rep. 8:1523. doi: 10.1038/s41598-017-18805-w
Kallolimath, S., Castilho, A., Strasser, R., Grünwald-Gruber, C., Altmann, F., Strubl, S., et al. (2016). Engineering of complex protein sialylation in plants. Proc. Natl. Acad. Sci. 113, 9498–9503. doi: 10.1073/pnas.1604371113
Keeling, P.-J. (2009). Chromalveolates and the evolution of plastids by secondary endosymbiosis. J. Eukaryot Microbiol. 56, 1–8. doi: 10.1111/j.1550-7408.2008.00371.x
Keskiaho, K., Hieta, R., Sormunen, R., and Myllyharju, J. (2007). Chlamydomonas reinhardtii has multiple prolyl 4-hydroxylases, one of which is essential for proper cell wall assembly. Plant Cell 19, 256–269. doi: 10.1105/tpc.106.042739
Kotani, A., Tsuji, M., Azama, Y., Ishi, T., Takeda, T., et al. (2013). Purification and characterization of UDP-arabinopyranose mutase from Chlamydomonas reinhardtii. Biosci. Biotechnol. Biochem. 77, 1874–1878. doi: 10.1271/bbb.130302
Lamport, D. T. A., and Miller, D. H. (1971). Hydroxyproline Arabinosides in the Plant Kingdom. Plant Physiol. 48, 454–456. doi: 10.1104/pp.48.4.454
Levy-Ontman, O., Arad, S. M., Harvey, D. J., Parsons, T. B., Fairbanks, A., and Tekoah, Y. (2011). Unique N-glycan moieties of the 66-kDa cell wall glycoprotein from the red microalga Porphyridium sp. J. Biol. Chem. 286, 21340–21352. doi: 10.1074/jbc.M110.175042
Levy-Ontman, O., Fisher, M., Shotland, Y., Tekoah, Y., and Malis Arad, S. (2015). Insight into glucosidase II from the red marine microalga Porphyridium sp. (Rhodophyta). J. Phycol. 51, 1075–1087. doi: 10.1111/jpy.12341
Levy-Ontman, O., Fisher, M., Shotland, Y., Weinstein, Y., Tekoah, Y., and Arad, S. M. (2014). Genes involved in the endoplasmic reticulum N-glycosylation path-way of the red microalga Porphyridium sp.: a bioinformatic study. Int. J. Mol. Sci. 15, 2305–2326. doi: 10.3390/ijms15022305
Li, K., Liu, Q., Fang, F., Luo, R., Lu, Q., Zhou, W., et al. (2019). Microalgae-based wastewater treatment for nutrients recovery: A review. Bioresour. Technol. 291:121934. doi: 10.1016/j.biortech.2019.121934
Lingg, N., Zhang, P., Song, Z., and Bardor, M. (2012). The sweet tooth of biopharmaceuticals: importance of recombinant protein glycosylation analysis. Biotechnol. J. 7, 1462–1472. doi: 10.1002/biot.201200078
Lucas, P.-L., Dumontier, R., Loutelier-Bourhis, C., Mareck, A., Afonso, C., Lerouge, P., et al. (2018). User-friendly extraction and multistage tandem mass spectrometry based analysis of lipid-linked oligosaccharides in microalgae. Plant Methods. 14:107. doi: 10.1186/s13007-018-0374-8
Lucas, P.-L., Mathieu-Rivet, E., Song, P. C. T., Oltmanns, A., Loutelier-Bourhis, C., Plasson, C., et al. (2020). Multiple xylosyltransferases heterogeneously xylosylate protein N-linked glycans in Chlamydomonas reinhardtii. Plant J. 102, 230–245. doi: 10.1111/tpj.14620
Mathieu-Rivet, E., Kiefer-Meyer, M.-C., Vanier, G., Ovide, C., Burel, C., Lerouge, P., et al. (2014). Protein N-glycosylation in eukaryotic microalgae and its impact on the production of nuclear expressed biopharmaceuticals. Front. Plant Sci. 5:359. doi: 10.3389/fpls.2014.00359
Mathieu-Rivet, E., Lerouge, P., and Bardor, M. (2017). “Chlamydomonas reinhardtii: Protein glycosylation and production of biopharmaceuticals,” in Chlamydomonas: Biotechnology and Biomedicine. Microbiology Monographs, Vol. 31, ed. M. Hippler (Berlin: Springer).
Mathieu-Rivet, E., Scholz, M., Arias, C., Dardelle, F., Schulze, S., Le Mauff, F., et al. (2013). Exploring the N-glycosylation pathway in Chlamydomonas reinhardtii unravels novel complex structures. Mol. Cell Proteomics 12, 3160–3183. doi: 10.1074/mcp.M113.028191
Matsui, T., Takita, E., Sato, T., Kinjo, S., Aizawa, M., Sugiura, Y., et al. (2011). N-Glycosylation at non canonical Asn-X-Cys sequences in plant cells. Glycobiology 21, 994–999. doi: 10.1093/glycob/cwq198
Mewono, L., Nguema-Ona, E., Gotté, M., Koroney, A. S., Follet Gueye, M. L., et al. (2015). O-glycosylation in plant and mammal cells: the use of chemical inhibitors to understand the biosynthesis and function of O-glycosylated proteins. Plant Sci. Today 2, 43–51. doi: 10.14719/pst.2015.2.2.67
Miller, D. H., Lamport, D. T., and Miller, M. (1972). Hydroxyproline heterooligosaccharides in Chlamydomonas. Science 176, 918–920. doi: 10.1126/science.176.4037.918
Mócsai, R., Blaukopf, M., Svehla, E., Kosma, P., and Altmann, F. (2020a). The N-glycans of Chlorella sorokiniana and a related strain contain arabinose but have strikingly different structures. Glycobiology 30, 663–676. doi: 10.1093/glycob/cwaa012
Mócsai, R., Figl, R., Sützl, L., Fluch, S., and Altmann, F. (2020b). A first view on the unsuspected intragenus diversity of N-glycans in Chlorella microalgae. Plant J. 103, 184–196. doi: 10.1111/tpj.14718
Mócsai, R., Figl, R., Troschl, C., Strasser, R., Svehla, E., Windwarder, M., et al. (2019). N-glycans of the microalga Chlorella vulgaris are of the oligomannosidic type but highly methylated. Sci. Rep. 9:331. doi: 10.1038/s41598-018-36884-1
Monk, C. B. (1988). The cell wall of Chlamydomonas reinhardtii gametes: Composition, structure and autolysin-mediated shedding and dissolution. Planta 176, 441–450. doi: 10.1007/BF00397650
Moosburner, M. A., Gholami, P., McCarthy, J. K., Tan, M., Bielinski, V. A., et al. (2020). Multiplexed knockouts in the model diatom Phaeodactylum by episomal delivery of a selectable Cas9. Front. Microbiol. 11:5. doi: 10.3389/fmicb.2020.00005
Moremen, K. W., Tiemeyer, M., and Nairn, A. V. (2012). Vertebrate protein glycosylation: diversity, synthesis and function. Nat. Rev. Mol. Cell. Biol. 13, 448–462. doi: 10.1038/nrm3383
Müller, T., Bause, E., and Jaenicke, L. (1984). Evidence for an incomplete dolichyl-phosphate pathway of lipoglycan formation in Volvox carteri f. nagariensis. Eur. J. Biochem. 138, 153–159. doi: 10.1111/j.1432-1033.1984.tb07894.x
Mussgnug, J. H. (2015). Genetic tools and techniques for Chlamydomonas reinhardtii. Appl. Microbiol. Biotechnol. 99, 5407–5418. doi: 10.1007/s00253-015-6698-7
Neelamegham, S., Aoki-Kinoshita, K., Bolton, E., Frank, M., Lisacek, F., Lütteke, T., et al. (2019). Updates to the symbol nomenclature for glycans (SNFG) guidelines. Glycobiology 29, 620–624. doi: 10.1093/glycob/cwz045
Nguema-Ona, E., Vicre-Gibouin, M., Gotte, M., Plancot, B., Lerouge, P., Bardor, M., et al. (2014). Cell wall O-glycoproteins and N-glycoproteins: aspects of biosynthesis and function. Front. Plant Sci. 5:499. doi: 10.3389/fpls.2014.00499
Novoveská, L., Ross, M. E., Stanley, M. S., Pradelles, R., Wasiolek, V., and Sassi, J. F. (2019). Microalgal Carotenoids: A Review of Production, Current Markets, Regulations, and Future Direction. Mar. Drugs 17:640. doi: 10.3390/md17110640
Nymark, M., Sharma, A. K., Sparstad, T., Bones, A. M., and Winge, P. (2016). A CRISPR/Cas9 system adapted for gene editing in marine algae. Sci. Rep. 6:24951. doi: 10.1038/srep24951
O’Flaherty, R., Trbojeviæ-Akmaèiæ, I., Greville, G., Rudd, P. M., and Lauc, G. (2017). The sweet spot for biologics: recent advances in characterization of biotherapeutic glycoproteins. Exp. Rev. Proteomics 22, 1–17. doi: 10.1080/14789450.2018.1404907
O’Neill, E., Kuhaudomlarp, S., Rejzek, M., Fangel, J., Alagesan, K., Kolarich, D., et al. (2017). Exploring the glycans of Euglena gracilis. Biology 6:45. doi: 10.3390/biology6040045
Oey, M., Sawyer, A. L., Ross, I. L., and Hankamer, B. (2016). Challenges and opportunities for hydrogen production from microalgae. Plant Biotechnol. J. 14, 1487–1499. doi: 10.1111/pbi.12516
Ogawa-Ohnishi, M., Matsushita, W., and Matsubayashi, Y. (2013). Identification of three hydroxyproline O-arabinosyltransferases in Arabidopsis thaliana. Nat. Chem. Biol. 9, 726–730. doi: 10.1038/nchembio.1351
Ohyama, K., Shinohara, H., and Ogawa-Ohnishi, M. (2009). A glycopeptide regulating stem cell fate in Arabidopsis thaliana. Nat. Chem. Biol. 5, 578–580. doi: 10.1038/nchembio.182
Oltmanns, A., Hoepfner, L., Scholz, M., Zinzius, K., Schulze, S., and Hippler, M. (2020). Novel insights into N-glycan fucosylation and core xylosylation in C. reinhardtii. Front Plant Sci. 10:1686. doi: 10.3389/fpls.2019.01686
Paccalet, T., Bardor, M., Rihouey, C., Delmas, F., Chevalier, C., D’Aoust, M. −A., et al. (2007). Engineering of a sialic acid synthesis pathway in transgenic plants by expression of bacterial Neu5Ac−synthesizing enzymes. Plant Biotech. J. 5, 16–25. doi: 10.1111/j.1467-7652.2006.00211.x
Park, R. V., Asbury, H., and Miller, S. M. (2020). Modification of a Chlamydomonas reinhardtii CRISPR/Cas9 transformation protocol for use with widely available electroporation equipment. MethodsX 7:100855. doi: 10.1016/j.mex.2020.100855
Parodi, A. J. (1993). N-glycosylation in trypanosomatid protozoa. Glycobiology 3, 193–199. doi: 10.1093/glycob/3.3.193
Rautengarten, C., Ebert, B., Herter, T., Petzold, C. J., Ishii, T., et al. (2011). The interconversion of UDP-arabinopyranose and UDP-arabinofuranose is indispensable for plant development in Arabidopsis. Plant Cell 23, 1373–1390. doi: 10.1105/tpc.111.083931
Rodríguez-Ezpeleta, N., Brinkmann, H., Burey, S. C., Roure, B., Burger, G., Löffelhardt, W., et al. (2005). Monophyly of primary photosynthetic eukaryotes: green plants, red algae, and glaucophytes. Curr. Biol. 15, 1325–1330. doi: 10.1016/j.cub.2005.06.040
Rosales-Mendoza, S., Solís-Andrade, K. I, Márquez-Escobar, V. A., González-Ortega, O., and Bañuelos-Hernandez, B. (2020). Current advances in the algae-made biopharmaceuticals field. Exp. Opin. Biol. Ther. 20, 751–766. doi: 10.1080/14712598.2020.1739643
Rose, D. R. (2012). Structure, mechanism and inhibition of Golgi α-mannosidase II. Curr. Opin. Struct. Biol. 22, 558–562. doi: 10.1016/j.sbi.2012.06.005
Rösti, J., Barton, C. J., Albrecht, S., Dupree, P., Pauly, M., et al. (2007). UDP-glucose 4-epimerase isoforms UGE2 and UGE4 cooperate in providing UDP-galactose for cell wall biosynthesis and growth of Arabidopsis thaliana. Plant cell 19, 1565–1579. doi: 10.1105/tpc.106.049619
Roycewicz, P. S., and Malamy, J. E. (2014). Cell wall properties play an important role in the emergence of lateral root primordia from the parent root. J. Exp. Bot. 65, 2057–2069. doi: 10.1093/jxb/eru056
Rumin, J., Nicolau, E., Junior, R. G. O., Fuentes-Grünewald, C., Flynn, K. J., and Picot, L. (2020). A Bibliometric Analysis of Microalgae Research in the World, Europe, and the European Atlantic Area. Mar. Drugs 18:79. doi: 10.3390/md18020079
Saito, F., Suyama, A., Oka, T., Yoko-O, T., Matsuoka, K., Jigami, Y., et al. (2014). Identification of Novel Peptidyl Serine α-Galactosyltransferase Gene Family in Plants. J. Biol. Chem. 30, 20405–20420. doi: 10.1074/jbc.M114.553933
Samuelson, J., Banerjee, S., Magnelli, P., Cui, J., Kelleher, D. J., Gilmore, R., et al. (2005). The diversity of dolichol-linked precursors to Asn-linked glycans likely results from secondary loss of sets of glycosyltransferases. Proc. Natl. Acad. Sci. U S A. 102, 1548–1553. doi: 10.1073/pnas.0409460102
Schjoldager, K. T., and Clausen, H. (2012). Site-specific protein O-glycosylation modulates proprotein processing - deciphering specific functions of the large polypeptide GalNAc-transferase gene family. Biochim. Biophys. Acta 1820, 2079–2094. doi: 10.1016/j.bbagen.2012.09.014
Schoberer, J., and Strasser, R. (2018). Plant glyco-biotechnology. Semin. Cell Dev. Biol. 80, 133–141. doi: 10.1016/j.semcdb.2017.07.005
Schulze, S., Oltmanns, A., Machnik, N., Liu, G., Xu, N., Jarmatz, N., et al. (2018). N-Glycoproteomic Characterization of Mannosidase and Xylosyltransferase Mutant Strains of Chlamydomonas reinhardtii. Plant Physiol. 176, 1952–1964. doi: 10.1104/pp.17.01450
Schulze, S., Urzica, E., Reijnders, M. J. M. F., van de Geest, H., Warris, S., Bakker, L. V., et al. (2017). Identification of methylated GnTI-dependent N-glycans in Botryococcus braunii. New Phytol. 215, 1361–1369. doi: 10.1111/nph.14713
Schwarz, F., and Aebi, M. (2011). Mechanisms and principles of N-linked protein glycosylation. Curr. Opin. Struct. Biol. 21, 576–582. doi: 10.1016/j.sbi.2011.08.005
Seifert, G. J. (2020). On the potential function of type II arabinogalactan O-glycosylation in regulating the fate of plant secretory proteins. Front. Plant Sci. 11:563735. doi: 10.3389/fpls.2020.563735
Shin, S. E., Lim, J. M., Koh, H. G., Kim, E. K., Kang, N. K., Jeon, S., et al. (2016). CRISPR/Cas9-induced knockout and knock-in mutations in Chlamydomonas reinhardtii. Sci. Rep. 6:27810. doi: 10.1038/srep27810
Shinohara, H., and Matsubayashi, Y. (2010). Arabinosylated glycopeptide hormones: new insights into CLAVATA3 structure. Curr. Opin. Plant Biol. 13, 515–519. doi: 10.1016/j.pbi.2010.05.008
Slattery, S. S., Diamond, A., Wang, H., Therrien, J. A., Lant, J. T., et al. (2018). An expanded plasmid-based genetic toolbox enables Cas9 genome editing and stable maintenance of synthetic pathways in Phaeodactylum tricornutum. ACS Synth. Biol. 7, 328–338. doi: 10.1021/acssynbio.7b00191
Stanley, P., Taniguchi, N., and Aebi, M. (2017). “N-Glycans,” in Essentials of Glycobiology, 3rd Edn, eds A. Varki, R. D. Cummings, and J. D. Esko (Cold Spring Harbor: Cold Spring Harbor Laboratory Press).
Staudacher, E. (2012). Methylation-an uncommon modification of glycans. Biol. Chem. 393, 675–685. doi: 10.1515/hsz-2012-0132
Strasser, R. (2016). Plant protein glycosylation. Glycobiology 26, 926–939. doi: 10.1093/glycob/cww023
Strasser, R. (2018). Protein Quality Control in the Endoplasmic Reticulum of Plants. Annu. Rev. Plant Biol. 69, 147–172. doi: 10.1146/annurev-arplant-042817-040331
Van Beers, M. M., and Bardor, M. (2012). Minimizing immunogenicity of biopharmaceuticals by controlling critical quality attributes of proteins. Biotechnol. J. 7, 1473–1484. doi: 10.1002/biot.201200065
Vanier, G., Hempel, F., Chan, P., Rodamer, M., Vaudry, D., Maier, U. G., et al. (2015). Biochemical characterization of human anti-hepatitis B monoclonal antibody produced in the microalgae Phaeodactylum tricornutum. PLoS One 10:e0139282. doi: 10.1371/journal.pone.0139282
Vanier, G., Lucas, P.-L., Loutelier-Bourhis, C., Vanier, J., Plasson, C., Walet-Balieu, M.-L., et al. (2017). Heterologous expression of the N-acetylglucosaminyltransferase I dictates a reinvestigation of the N-glycosylation pathway in Chlamydomonas reinhardtii. Sci. Rep. 7:10156. doi: 10.1038/s41598-017-10698-z
Velasquez, S. M., Marzol, E., Borassi, C., and Pol-Fachin, L. (2015). Low sugar is not always good: Impact of specific O-glycan defects on tip growth in Arabidopsis. Plant Physiol. 168, 808–813. doi: 10.1104/pp.114.255521
Velasquez, S. M., Ricardi, M. M., Dorosz, J. G., Fernandez, P. V., Nadra, A. D., et al. (2011). O-glycosylated cell wall extensins are essential in root hair growth. Science 33, 1401–1403. doi: 10.1126/science.1206657
Vesteg, M., Hadariová, L., Horváth, A., Estraño, C. E., Schwartzbach, S. D., and Krajèoviè, J. (2019). Comparative molecular cell biology of phototrophic euglenids and parasitic trypanosomatids sheds light on the ancestor of Euglenozoa. Biol. Rev. Camb. Philos. Soc. 94, 1701–1721. doi: 10.1111/brv.12523
Vézina, L. P., Faye, L., Lerouge, P., D’Aoust, M. A., Marquet-Blouin, E., et al. (2009). Transient co-expression for fast and high-yield production of antibodies with human-like N -glycans in plants. Plant. Biotech. J. 5, 442–455. doi: 10.1111/j.1467-7652.2009.00414.x
Voigt, J., Frank, R., and Wöstemeyer, J. (2009). The chaotrope-soluble glycoprotein GP1 is a constituent of the insoluble glycoprotein framework of the Chlamydomonas cell wall. FEMS Microbiol. Lett. 291, 209–215. doi: 10.1111/j.1574-6968.2008.01456.x
Voigt, J., Stolarczyk, A., Zych, M., Malec, P., and Burczyk, J. (2014). The cell-wall glycoproteins of the green alga Scenedesmus obliquus. The predominant cell-wall polypeptide of Scenedesmus obliquus is related to the cell-wall glycoprotein gp3 of Chlamydomonas reinhardtii. Plant Sci. 215-216, 39–47. doi: 10.1016/j.plantsci.2013.10.011
Walsh, G. (2014). Biopharmaceutical benchmarks. Nat. Biotechnol. 32, 992–1000. doi: 10.1038/nbt.3040
Walski, T., De Schutter, K., Van Damme, E. J. M., and Smagghe, G. (2017). Diversity and functions of protein glycosylation in insects. Insect. Biochem. Mol. Biol. 83, 21–34. doi: 10.1016/j.ibmb.2017.02.005
Wang, P., Wang, H., Gai, J., Tian, X., Zhang, X., Lv, Y., et al. (2017). Evolution of protein N-glycosylation process in Golgi apparatus which shapes diversity of protein N-glycan structures in plants, animals and fungi. Sci. Rep. 7:40301. doi: 10.1038/srep40301
Wang, Q., Lu, Y., Xin, Y., Wei, L., Huang, S., and Xu, J. (2016). Genome editing of model oleaginous microalgae Nannochloropsis spp. by CRISPR/Cas9. Plant J. 88, 1071–1081. doi: 10.1111/tpj.13307
Wohlschlager, T., Butschi, A., Grassi, P., Sutov, G., Gauss, R., et al. (2014). Methylated glycans as conserved targets of animal and fungal innate defense. PNAS 111:27. doi: 10.1073/pnas.1401176111
Yagodnik, C., De La Canal, L., and Parodi, A. J. (1987). Tetrahymena pyriformis cells are deficient in all mannose-P-dolichol-dependent mannosyltransferases but not in mannose-P-dolichol synthesis. Biochemistry 26, 5937–5943. doi: 10.1021/bi00392a054
Yang, Z., Drew, D. P., Jørgensen, B., Mandel, U., Bach, S. S., Ulvskov, P., et al. (2012). Engineering mammalian mucin-type O-glycosylation in plants. J. Biol. Chem. 287, 11911–11923. doi: 10.1074/jbc.M111.312918
Zhang, P., Burel, C., Plasson, C., Kiefer-Meyer, M.-C., Ovide, C., Gügi, B., et al. (2019). Characterization of a GDP-Fucose transporter and a fucosyltransferase involved in the fucosylation of glycoproteins in the diatom Phaeodactylum tricornutum. Front. Plant. Sci. 10:610. doi: 10.3389/fpls.2019.00610
Keywords: microalgae, post-translational modification, N-glycosylation, O-glycosylation, protein, biopharmaceuticals, endoplasmic reticulum, Golgi apparatus
Citation: Mathieu-Rivet E, Mati-Baouche N, Walet-Balieu M-L, Lerouge P and Bardor M (2020) N- and O-Glycosylation Pathways in the Microalgae Polyphyletic Group. Front. Plant Sci. 11:609993. doi: 10.3389/fpls.2020.609993
Received: 24 September 2020; Accepted: 23 November 2020;
Published: 17 December 2020.
Edited by:
Richard Strasser, University of Natural Resources and Life Sciences, Vienna, AustriaReviewed by:
Friedrich Altmann, University of Natural Resources and Life Sciences, Vienna, AustriaEva L. Decker, University of Freiburg, Germany
Copyright © 2020 Mathieu-Rivet, Mati-Baouche, Walet-Balieu, Lerouge and Bardor. This is an open-access article distributed under the terms of the Creative Commons Attribution License (CC BY). The use, distribution or reproduction in other forums is permitted, provided the original author(s) and the copyright owner(s) are credited and that the original publication in this journal is cited, in accordance with accepted academic practice. No use, distribution or reproduction is permitted which does not comply with these terms.
*Correspondence: Muriel Bardor, bXVyaWVsLmJhcmRvckB1bml2LXJvdWVuLmZy
†These authors have contributed equally to this work