- 1Department of Horticulture, Oregon Wine Research Institute, Oregon State University, Corvallis, OR, United States
- 2USDA-ARS Horticulture Crops Research Unit, Corvallis, OR, United States
- 3Oregon Wine Research Institute, Corvallis, OR, United States
In viticulture, rootstocks are essential to cope with edaphic constraints. They can also be used to modulate scion growth and development to help improve berry yield and quality. The rootstock contribution to scion growth is not fully understood. Since nitrogen (N) is a significant driver of grapevine growth, rootstock properties associated with N uptake and transport may play a key role in the growth potential of grafted grapevines. We evaluated N uptake and transport in a potted system using two grapevines rootstocks [Riparia Gloire (RG) and 1103 Paulsen (1103P)] grafted to Pinot noir (Pommard clone) scion. Combining results of nitrate induction and steady-state experiments at two N availability levels, we observed different responses in the uptake and utilization of N between the two rootstocks. The low vigor rootstock (RG) exhibited greater nitrate uptake capacity and nitrate assimilation in roots after nitrate resupply than the more vigorous 1103P rootstock. This behavior may be attributed to a greater root carbohydrate status observed in RG for both experiments. However, 1103P demonstrated a higher N translocation rate to shoots regardless of N availability. These distinct rootstock behaviors resulted in significant differences in biomass allocation between roots and shoots under N-limited conditions, although the overall vine biomass was not different. Under sufficient N supply, differences between rootstocks decreased but 1103P stored more N in roots, which may benefit growth in subsequent growing seasons. Overall, greater transpiration of vines grafted to 1103P rootstock causing higher N translocation to shoots could partially explain its known growth-promoting effect to scions under low and high N availability, whereas the low vigor typically conferred to scions by RG may result from the combination of lower N translocation to shoots and a greater allocation of biomass toward roots when N is low.
Introduction
In response to the outbreak of Grape phylloxera (Daktulosphaira vitifoliae) in European Vitis vinifera vineyards during the 19th century, grafting vines to North American Vitis species and their hybrids as rootstocks became commonplace worldwide. While the primary goal was to prevent vine decline due to phylloxera, there became apparent other benefits imparted to scions by rootstocks to thrive under biotic and abiotic constraints. Additionally, rootstocks were found to influence scion development and yield, which could be used to modulate cultivar traits and improve grape and wine quality (Jones et al., 2009; Ollat et al., 2016; Zhang et al., 2016).
Rootstocks influence whole plant development since they serve as the vines’ root system. Genetic variability among rootstocks can result in differences in water and nutrient uptake and transport, and the regulation of hormones and other long-distance signal molecules that impact scion growth and development (Albacete et al., 2015; Zhang et al., 2016; Gautier et al., 2019; Ibacache et al., 2020). Apart from water, nitrogen (N) is the most limiting abiotic factor that affects plant growth. In grapevine, N accumulation in shoots is positively correlated to scion growth, and is influenced by the rootstock genotype (Nikolaou et al., 2000; Zerihun and Treeby, 2002; Ibacache et al., 2020). Moreover, the impact of rootstocks on scion growth is stronger under N limitation (Lecourt et al., 2015), indicating that N uptake and transport to the shoot may explain differences observed among rootstock genotypes.
Nitrate is the main source of N taken-up by plants from soil and acts as a signal molecule to regulate its own uptake, transport, and assimilation (Crawford and Glass, 1998). Plants actively take up nitrate through a proton/nitrate-coupled mechanism mainly mediated by Low and High Affinity Transport Systems (LATS and HATS, respectively) (Lejay and Gojon, 2018; Wang Y.-Y. et al., 2018). In grapevines and other plants, HATS and LATS are rapidly (<24 h) induced in response to increasing nitrate availability (Pii et al., 2014; Tomasi et al., 2015), and the genotype of the root system modulates (i) the kinetics of HATS (Kulmann et al., 2020) and (ii) the intensity of its induction response (Tomasi et al., 2015). However, no information is available on the variability of LATS among grapevine rootstocks. In addition, some inconsistencies in the properties of HATS (Yang et al., 2007; Tomasi et al., 2015; Kulmann et al., 2020) make it difficult to draw conclusions about the relationship between nitrate uptake capacity of a given rootstock and the biomass growth conferred to scions. While the specific location of nitrate uptake among different parts of the overall root system is unclear, the youngest roots that still have an intact cortex and account for most of the overall length of roots within a given root system are thought to contribute most to ion uptake (Kozlowski and Pallardy, 1997). A study in various trees found that younger primary roots had the highest net flux of NH4+ and NO3– even though corky and woody root zones showed some net N uptake (Hawkins et al., 2014). In grapevines, the rate of nitrate uptake was two to three times higher in 1 day-old root tips than root tips that were 10–20 days-old (Volder et al., 2005).
Gene expression analyses of Arabidopsis thaliana for the closest homologs to major nitrate transporters and N-signaling genes were performed in grapevine in response to nitrate resupply (Pii et al., 2014; Tomasi et al., 2015; Cochetel et al., 2017, 2019). Expression of VitviNRT2.4A and VitviNRT3 homologs in SO4 rootstock (Pii et al., 2014) or of VitviNAXT, a putative nitrate efflux transporter, in K5BB rootstocks (Tomasi et al., 2015), seem to align with the induction response of HATS. Using genome-wide expression analyses, Cochetel et al. (2017) reported on differential responses between two rootstocks 3 and 24 h after nitrate resupply. In that experiment, nitrate accumulation in roots was associated with variations between rootstocks in the expression of genes involved in N metabolism and transport. Surprisingly, the rootstock with the lowest vigor showed the greatest nitrate accumulation and the greatest induction of genes involved in nitrate transport and assimilation (Cochetel et al., 2017). These results suggest that net nitrate uptake may be uncoupled from scion growth promotional effects of the rootstock.
Root-to-shoot transport of nitrate participates to the regulation of N shoot status and its influence on scion growth. Rootstock contribution to nitrate xylem loading and transpiration flow may aid to the differences in N shoot status observed in scions (Zhang et al., 2016; Ibacache et al., 2020). Xylem loading flux is regulated by the balance between nitrate loading and unloading in root pericycle cells. The movement of nitrate from cell to cell could either be passive, through anion channels like X-QUAC proteins (Köhler et al., 2002) or active, through members of NRT1/NPFs proteins family (Lin et al., 2008; Li et al., 2010; Léran et al., 2013; Taochy et al., 2015). In grapevine, differences in gene expression of nitrate transporters including one associated with xylem loading (VitviNAXT1) was observed between rootstocks under normal growing conditions (Henderson et al., 2014). Varying N concentrations in xylem exudate observed in ten rootstocks grafted to Thomson Seedless were found also (Nikolaou et al., 2000). Altogether, this may suggest a different ability of rootstocks to regulate the net xylem loading of nitrate in grapevine.
Plant transpiration also contributes to nitrate root-to-shoot transport directly by regulating xylem sap flow and indirectly by increasing nitrate availability through the control of the mass flow movement in soil (Cramer et al., 2009). The tight relationship between water and nitrate transport in plants may depend on the regulation of aquaporin activity and root hydraulic conductivity by nitrate availability (Tyerman et al., 2017). In grapevine, the rootstock contribution to scion transpiration has been extensively studied in the context of water deficit and scion growth response (Ollat et al., 2016; Zhang et al., 2016). The strong correlation between scion growth, transpiration, and rootstock hydraulic conductance suggests that rootstocks can modulate the translocation of N toward shoots by controlling the plant water flow (Gambetta et al., 2012; Zhang et al., 2016). Likewise, the graft union could influence translocation of N to the shoot and the transport of N-reserves to roots. The extent of healing at the graft union can alter local xylem anatomy locally (Goncalves et al., 2007). As a consequence, hydraulic properties of the whole plant could be modified with an impact on the movement of water, hormones, and other nutrients like NO3– and NH4+ (Atkinson et al., 2003). Scion development is often altered in grafted plants with significant effects on transpiration and vigor (Edwards et al., 2014). Conversely, root growth can be impacted via poor phloem connections at the graft union that limits shoot-to-root transport of assimilates (Bieleski, 2000; Olmstead et al., 2006). Finally, as nitrate reduction mainly occurs in grapevine leaves (Zerihun and Treeby, 2002), the rootstock contribution to N translocation toward shoots may modulate the rate of N assimilation and thus the plant N status.
Nitrate reduction and assimilation are essential regulators of nitrate uptake and transport (Dechorgnat et al., 2011; Tegeder and Masclaux-Daubresse, 2018). The dependence of carbon (C) resource(s) for amino acids and proteins synthesis requires a fine regulation of the C/N balance in plants. C and N assimilates can stimulate or repress nitrate uptake and transport, respectively. Furthermore, C/N balance regulates C and N assimilation steps, which in turn affects the source-sink relationship between the root and shoot systems, and controls growth and biomass allocation (Nunes-Nesi et al., 2010; Wang and Ruan, 2016). In grapevine, biomass allocation responds to the internal C/N balance (Grechi et al., 2007) that could be modulated by rootstocks through their influence on C and N assimilation in scion leaves (Zerihun and Treeby, 2002; Zhang et al., 2016). Although scion genotype seems to play a major role on the N uptake regulation (Tomasi et al., 2015) and biomass partitioning (Tandonnet et al., 2010), the rootstock influence on C/N balance was never properly assessed in the context of shoot growth response to rootstocks under N-limited supply.
The main goal of the current study was to further understand the mechanisms of rootstocks on the N-driven scion growth response. Our objectives were to measure root nitrate uptake properties when nitrate was resupplied to plants after N-starvation in two rootstocks [Riparia Gloire (RG) and 1103 Paulsen (1103P)] known to differ in vigor conferred to the scion. We also wanted to determine if the rootstocks would influence the rate of nitrate translocation to the scion under two levels of N supply. Additionally, we evaluated the rootstock influence on C/N balance in roots and leaves, and their relationship with plant growth and biomass partitioning. Our results confirmed higher nitrate uptake capacity and N-signaling responses of RG root tips after N-resupply. However, under steady state nitrate supply, nitrate concentration in xylem sap and transpiration flow contributed to a higher delivery of nitrate to shoots when vines were grafted to 1103P. Rootstocks did not alter total plant biomass but changed partitioning under N-limited conditions, resulting in higher shoot growth performance in vines grafted to 1103P. The rootstock genotype seems to control C and N metabolites partitioning between leaves and roots that could triggers the observed variability in nitrate uptake, sensing and biomass partitioning. Overall, this study identified that nitrate translocation to shoots rather than nitrate uptake capacity of rootstocks could modulate N-driven vigor of the scion under N-limited conditions.
Materials and Methods
Grapevine Material
One-year-old Riparia Gloire (RG) and 1103 Paulsen (1103P) rootstocks grafted to Vitis vinifera “Pinot noir” clone FPS04 (91 Pommard) scion were re-potted in 6 L pots filled with sand and grown in a semi-controlled greenhouse with a dark/light regime of 8/16 h, a temperature (day/night) of 25/20°C and a minimum light intensity of 400 μmol m–2s–1 of photosynthetically active radiation, supplied by 1000 W phosphor coated metal-halide lamps (General Electric, United States). Two weeks after budbreak, plants were pruned to maintain a single shoot, and emerging lateral shoots were removed during experiments.
Nitrate Uptake Induction and Kinetic Experiments
Eight plants per scion/rootstock combination were watered daily for 8 weeks with 0.5 N solution (N-NO3; Table 1) and then subjected to nitrate depletion with 0 N solution for 10 days. One hour after daylight, plants were divided into two groups each receiving either 2 L of 0 N solution supplemented with 0.5 mM of Ca(NO3)2 (induced plants) or with 0.5 mM of CaSO4 (non-induced plants). Roots tips (≈2 cm) were collected at six time points (0, 2, 4, 8, 12, and 24 h) through precut windows (≈40 cm2) on the sides of the pots and immediately immersed in root harvest solution (Table 1). Within 5 min of sampling, young roots were divided into three sub-samples within 5 min: one sample (≈300 mg) was snap frozen in liquid nitrogen and stored at −80°C until mRNA extraction; the two other samples (≈200 mg) were immediately subjected to 15N uptake assays at 0.2 and at 1 mM K15NO3 availability. One extra young root sample (≈200 mg) was collected at 0 and 24 h for analysis of metabolites. Labeled 15N uptake kinetic parameters were determined in a separate experiment for the time point 8 h using identical plants and growing conditions as described above. The only difference was that 15N uptake assays were carried out at the following concentration: 0.05; 0.1; 0.2; 0.3; 0.4; 0.5; 0.75; 1; 2; 5; 10 mM of K15NO3 availability.
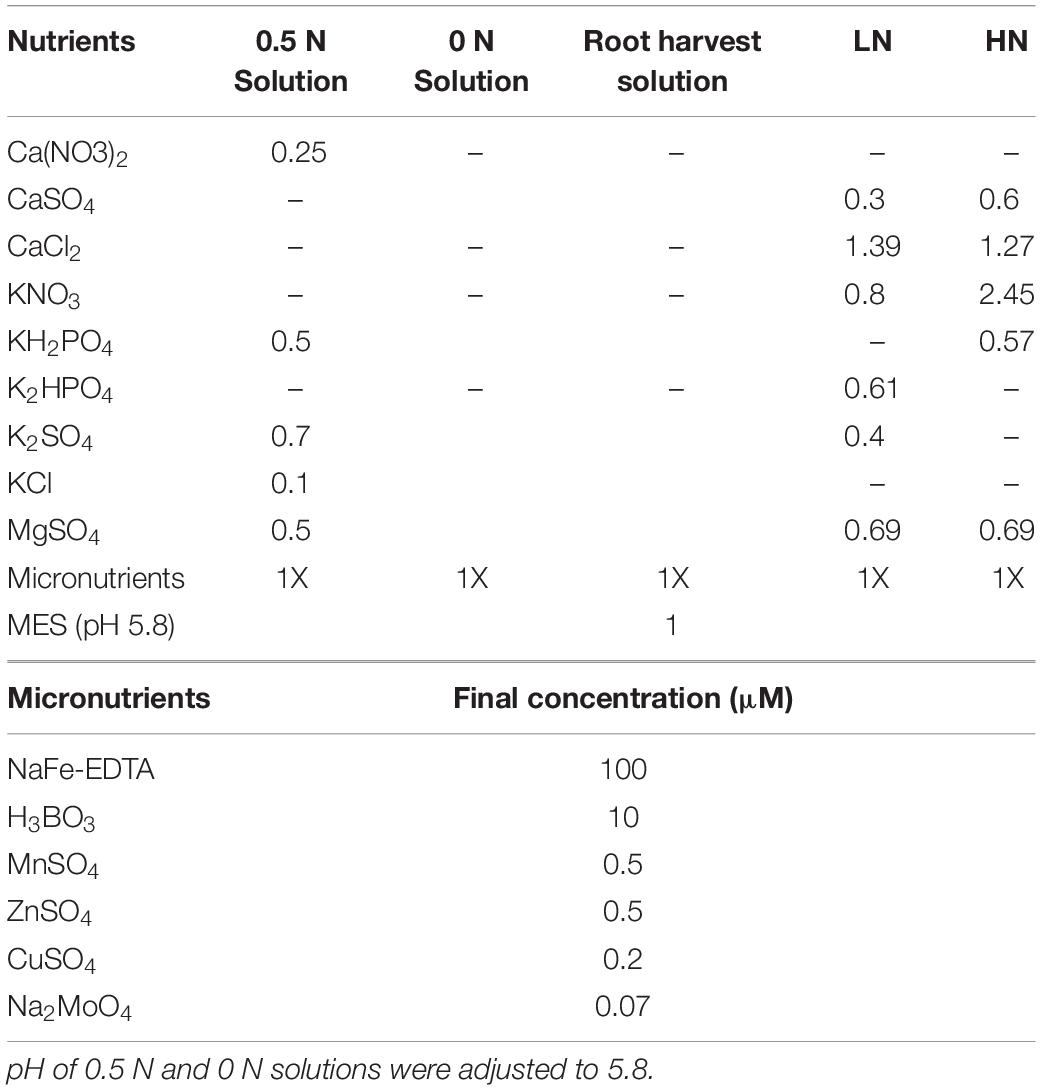
Table 1. Macro (mM) and micronutrient (μM) composition for the different experiments conducted in the study.
15N Uptake Assays and Analysis
Assays were performed by exposing the young roots (≈200 mg) for 5 min to K15NO3 (99% 15N) solutions in 1 mM MES buffer (pH = 5.8) and supplemented with 0.5 mM CaSO4. Roots were then rinsed 1 min with 0.5 mM CaSO4, before snap-freezing the samples and storage at −80°C. Following the grinding using a mixer mill (MM 400, Retsch GmbH, Haan, Germany), root samples were freeze dried (LABCONCO Lyph Lock 6). The amount of 15N in the freeze-dried roots was analyzed at the Oregon State University, Stable Isotope Research Unit, via an elemental analyzer interfaced with a mass spectrometer (Sercon GSL, PDZ Europa 20/20; Sercon, Cheshire, United Kingdom).
Steady-State Experiment
Eight plants per scion/rootstock combination were grown for 12 weeks under low (0.8 mM; LN, Table 1) and high (2.4 mM; HN, Table 1) nitrogen solutions (Lecourt et al., 2015). Stem length was measured weekly and the dry mass of roots, trunk, stem and leaves was recorded after drying the tissues at 60°C for 72 h at harvest. One day before harvest, two liters of LN or HN solution containing K15NO3 (50% 15N) were applied 1 h after daylight to evaluate the effect of nitrogen supply on nitrate root-to-shoot transport. At time 0, 4, 8, 12, and 24 h, one leaf per plant was selected and sequentially subjected to a measure of transpiration, xylem sap collection, surface area measurement, and finally collected for metabolite analyses. Selected leaves were fully expanded, fully exposed to light and located at similar internodes between plants, which is in the upper part of the plant (2/3 of plant height). Young root samples (≈400 mg each) were also collected at the same time points through precut windows (≈40 cm2) on the side of the pots and rinsed 1 min in 0.5 mM CaSO4 to remove surface contaminants. Leaves, roots, and xylem sap samples were snap frozen upon collection. Leaf transpiration was measured using a Li-6400XT photosynthesis system (Li-Cor, Inc., Lincoln, NE, United States) according to manufacturer’s instructions, and setup for ambient conditions in terms of temperature and relative humidity. The leaf xylem sap was collected using a Scholander pressure chamber (PMS Instruments, Alban, OR, United States). An overpressure of 0.5 MPa was applied after reaching the leaf water potential, the first drop was removed, and xylem sap was collected with a pipette within 5 min. Homogenized xylem sap sample (5 μL) and 50 μg of N (K14NO3) were applied onto an acid washed Whatman paper (10 mm2) and dried overnight at room temperature. 15N was determined on this subsample as previously described. Root and leaves samples collected at time 0 were used for metabolites quantification.
Gene Expression Analysis
Total RNA was extracted from 100 mg fresh powder of young root tissue according to Reid et al. (2006) with slight modifications. Genomic DNA contamination was removed using the Turbo DNA-free kit (Life technologies). Reverse transcription was performed using M-MuLV Reverse Transcriptase (New England Biolabs) using oligo dT primers with 2 μg of total RNAs. Transcript abundance was quantified on a Bio-Rad CFX96 machine using SsoAdvancedTM Universal SYBR® Green Supermix (Bio-Rad). PCR efficiency for each primer pair was calculated using LinRegPCR (Ruijter et al., 2009). Relative genes’ expression was calculated using the 2ΔΔCt method (Livak and Schmittgen, 2001) with the VitviACT7 ortholog as reference gene for normalization. Studied genes and primers used are presented in Table 2. Each PCR product were amplified first from cDNA roots libraries, cloned into a pGEM-T Easy vector (Promega, United States), and the sequence of the amplicons were confirmed by Sanger Sequencing (Center for Genome Research and Biocomputing, CGRB – OSU).
Metabolite Analyses
Approximately 30 mg of freeze-dried tissue (LABCONCO Lyph Lock 6) was extracted three times in a prechilled methanol/chloroform/water extraction solution (2.5/1/1 – v/v/v). The mixture was vortexed for 1 min and centrifuged for 5 min at 20,000 g at 4°C. Supernatants of the three extracts were pooled and mixed with equal volumes of chloroform and HPLC-grade water, vortexed and then centrifuged at 20,000 g for 5 min. The aqueous phase was collected for colorimetric assays of nitrate (Cataldo et al., 1975), Free Amino Acids (FAA) (Sun et al., 2006) and Total Soluble Carbohydrate (TSC) (Edwards et al., 2011). The remaining pellet was dried under vacuum overnight, and 5 mg was sampled for insoluble starch analysis (Edwards et al., 2011). TSC, starch and FAA concentrations were expressed as fructose, glucose and leucine equivalents.
Statistics and Multivariate Analyses
All statistical analyses were performed using the R software (R Core Team, 2017). In the induction experiment, paired t-tests and Welch paired-tests were performed for each rootstock, using t0 as control, to evaluate the induction of nitrate uptake rate and metabolite changes. Comparison between two means using student t-test, Welch t-test or Wilcoxon test depending on normality or homoscedasticity of the data were conducted to validate the nitrate kinetic induction at each nitrate concentration, and to evaluate rootstock effects on nitrate uptake rate. An Analysis of Covariance was performed to compare nitrate uptake rate between rootstocks, and in response to nitrate resupply. Rootstock, time, and treatment effects on gene expression were evaluated by one-way Analysis of Variance (ANOVA). In the steady-state experiment, a two-way ANOVA was performed to compare 15N flow differences between rootstock and treatment, and to compare biomass allocation and metabolite concentration changes between rootstock and time. For this dataset, the ANOVA was followed by a Tukey’s multiple test from log-transformed data when assumptions were not met from the raw data.
Results
Nitrate Uptake Induction Response
Nitrate uptake rate of fine roots of both rootstocks increased upon nitrate resupply (Figure 1). At the lowest concentration (0.2 mM), rootstocks increased their nitrate uptake capacity after 4 h of nitrate exposure and maintained a high rate for up to 24 h (Figure 1A). At 1 mM nitrate, RG experienced an abrupt increase 4 h after the resupply with a maximum of uptake at 8 h, followed by a decrease 12 h after the treatment (Figure 1B). A steady state uptake was then observed until the end of the experiment. For 1103P, the 1 mM resupply progressively increased nitrate uptake capacity up to 12 h before plateauing at 24 h (Figure 1B). The maximum difference in nitrate uptake capacity between rootstocks was observed at 8 h, and this time point was chosen to compare their nitrate uptake kinetics.
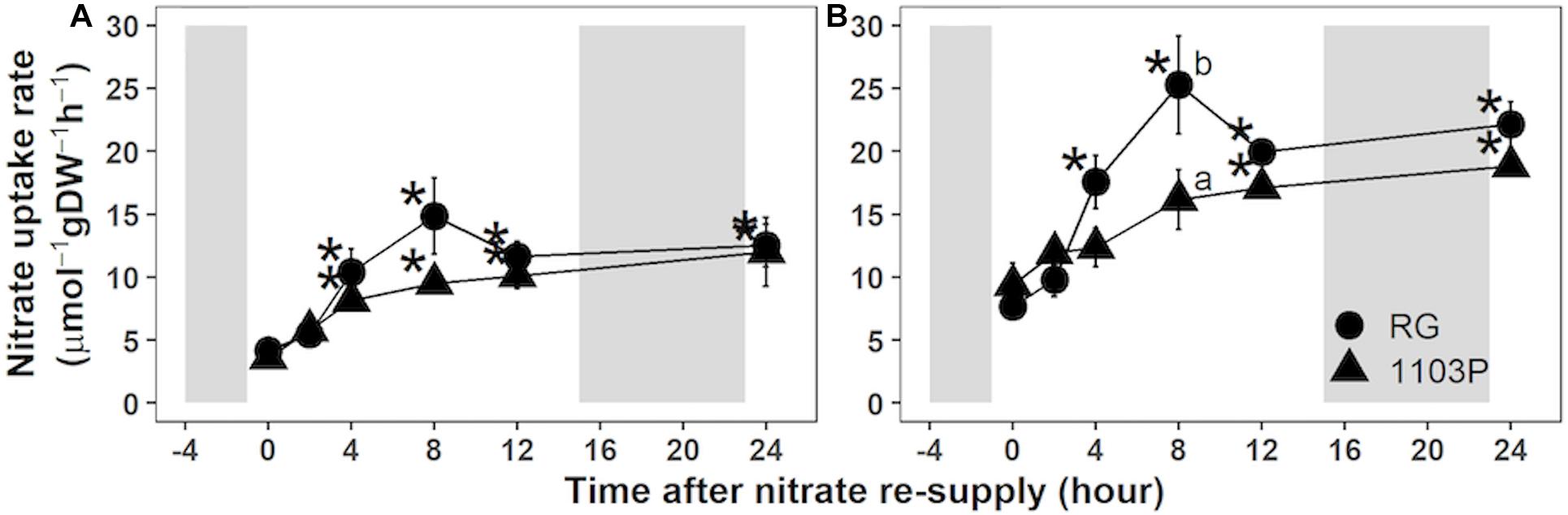
Figure 1. Nitrate uptake rate in roots of Riparia Gloire (circle) and 1103 Paulsen (triangle) rootstocks grafted to Pinot Noir scion (mean ± se; n = 4). Plants were grown 10 days without N, and then resupplied with 0.5 mM Ca(NO3)2. At the indicated times, young roots samples were transferred to 0.2 mM (A) or 1 mM (B) K15NO3 solution for 5 min (as detail in “Materials and Methods”). For each rootstock, asterisks show significant difference to the untreated sample (Time = 0 h; Paired t-test; P < 0.05) whereas letters refer to significant difference between rootstocks at each time point (Wilcoxon test; P < 0.05). Shaded areas represent dark period.
Nitrate Uptake Kinetic
For both rootstocks, nitrate uptake measurements with a gradient of nitrate availability ranging from 0.05 to 10 mM, revealed the presence of the two inducible systems for nitrate transport [Low Affinity Transport (LATS, Figures 2A,B) and High Affinity Transport Systems (HATS, Figures 2C,D)]. At high concentration (>1 mM), nitrate uptake rate increased in a nearly linear fashion to nitrate concentration with no sign of saturation up to 10 mM (Figures 2A,B). The 8-h exposure to 1 mM of nitrate significantly increased the capacity of LATS in both rootstocks (ANCOVA; RG, p < 0.001; 1103P, p = 0.003) although the magnitude of response was larger in RG compared to 1103P. The comparison between rootstocks revealed a greater LATS capacity for non-induced 1103P in comparison with non-induced RG (ANCOVA; p = 0.041). The opposite trend is observed in nitrate induced plants of each rootstock (ANCOVA; p = 0.025). At low nitrate availability (<1 mM), nitrate uptake rate followed a biphasic Michaelis-Menten kinetic pattern in both rootstocks (Figures 2C,D) with a separation of the kinetic phases occurring at 0.3–0.4 mM. For all the concentrations tested and for both rootstocks, exposure to nitrate induced an increase in nitrate uptake capacity. Kinetics parameters (Vmax and Km) could be calculated separately for each rootstock using either Michaelis-Menten or Lineweaver–Burk models, but both rootstocks could not satisfy the regression statistics on the same enzyme kinetics model. However, a general observation suggests that the two rootstocks share a common saturable component value (Vmax) for the first kinetic phase (0.05–0.4 mM), but the Vmax appeared to be higher for RG during the second kinetic phase (0.5–1 mM). In support of this observation, the nitrate uptake rate measured at 1 mM availability for the induced vines was significantly higher for RG than 1103P (t-test, p = 0.005).
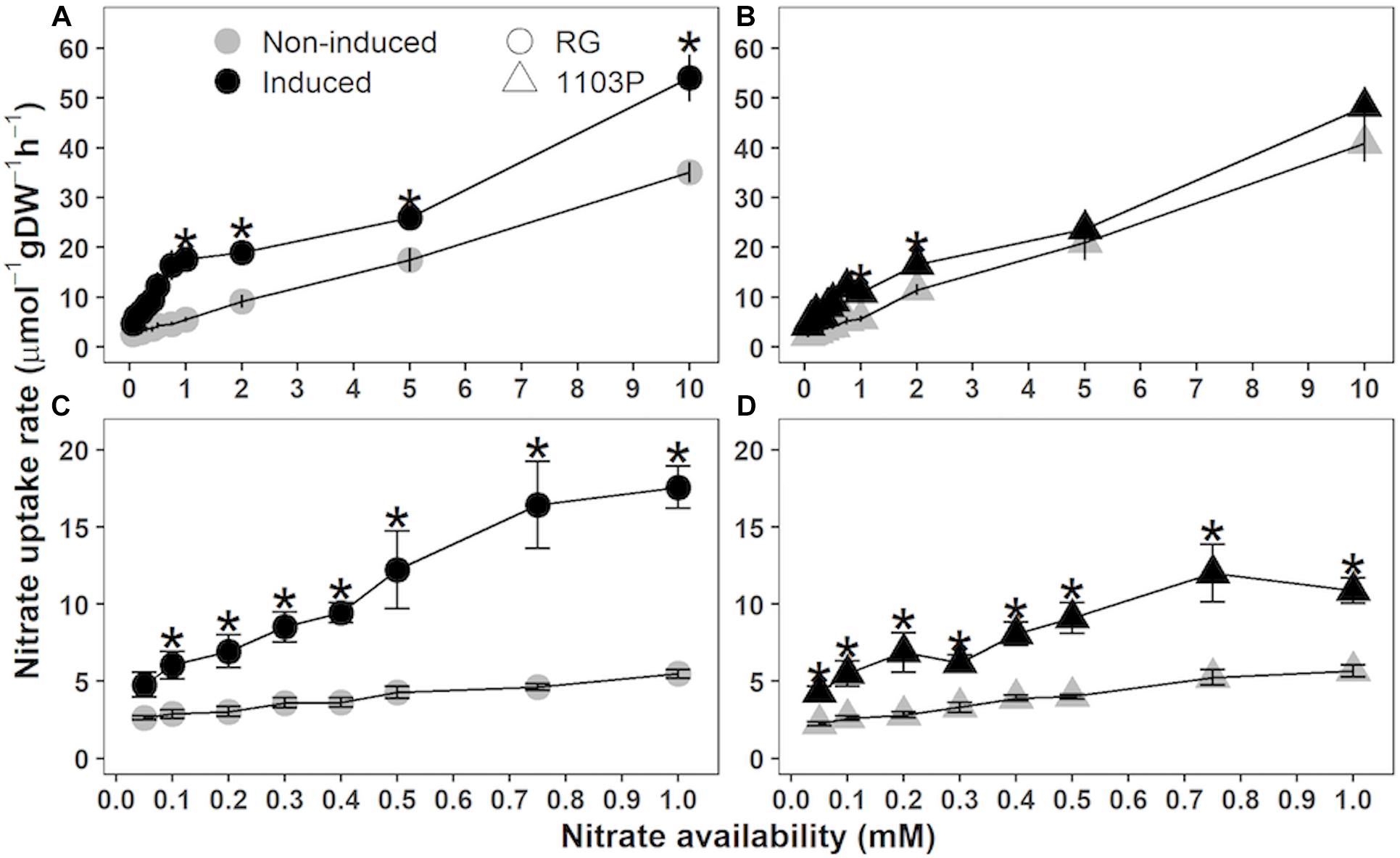
Figure 2. Nitrate uptake kinetics in roots of Riparia Gloire (A,C, circle) and 1103 Paulsen (B,D, triangle) rootstocks grafted to Pinot Noir scion (mean ± se; n = 4). Plants were grown 10 days without N, and then resupplied with 0.5 mM Ca(NO3)2 (Induced, black) or with 0.5 mM CaSO4 (non-induced, gray). After 8 h, young roots samples were transferred to a solution of K15NO3 in 0.05–10 mM concentration range. Asterisks show significant difference between induced and non-induced samples (t-test, P < 0.05).
Transcript Abundance of Nitrogen Transport, Assimilation, and Signaling Following Nitrate Resupply
Using Real-Time PCR, we monitored in both rootstocks the expression of the closest ortholog genes involved in nitrate uptake and assimilation (Figure 3 and Supplementary Figure 1). Expression profiles for VitviNRT1.1, VitviNRT2.4A, VitviNRT2.4B, VitviNRT3, VitviNR, and VitviGS2 showed a strong induction 2 h after nitrate resupply followed by a down-regulation upon 24 h. Significant differences between rootstocks for the expression of VitviNRT2.4A, VitviNRT3, and VitviGS2 appeared at 8 h with a higher expression for RG than for 1103P (Figure 3). Relative expressions of VitviNAXT and VitviBTB, involved in nitrate efflux (Segonzac et al., 2007) and nitrate signaling (Araus et al., 2016), respectively, were significantly affected depending on the rootstock genotype with a higher expression level for 1103P and RG, respectively. Rootstock differences for VitviBTB expression was observed mainly for the time 2 and 8 h while the level of expression was identical between rootstocks at 0 and 24 h. Expression of VitviHY5 and VitviCIPK23, whose orthologs in other plants are regarded as major regulators of the nitrogen signaling, were not affected by rootstocks or by nitrate resupply (Supplementary Figure 1).
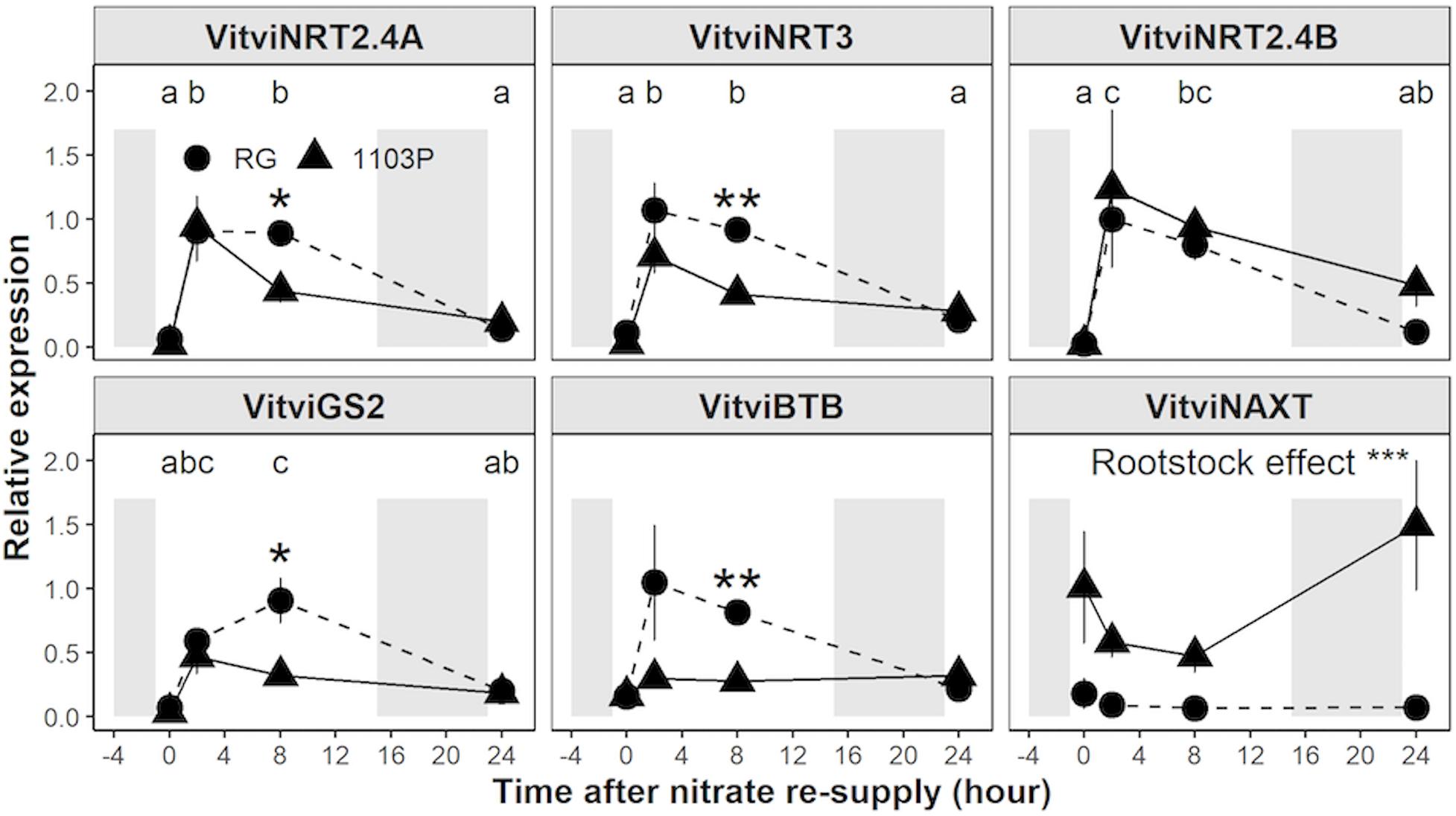
Figure 3. Relative gene expression of VitviNRT2.4A, VitviNRT3, VitviNRT2.4B, VitviGS2, VitviBTB, and VitviNAXT in roots tips (mean ± se; n = 3) of RG (circle) and 1103P (triangle) in response to nitrate resupply. Indicated time correspond to exposure duration to 0.5 mM Ca(NO3)2 after a 10 days period of N-starvation; shaded areas represent dark period. Letters refers to a significant difference between time point considering the two rootstocks (ANOVA, P < 0.05). Asterisks show significant difference between rootstocks at each time point (t-test, *P < 0.05, **P < 0.01). Overall rootstock differences are presented (ANOVA, ***P < 0.001).
Metabolite Dynamics in Root After Nitrate Resupply
Total Soluble Carbohydrate (TSC), starch, nitrate and Free Amino Acids (FAA) concentrations were compared in young roots before and after 24 h of nitrate resupply (Figure 4 and Supplementary Figure 2). The concentration of amino acids increased after nitrate induction only in RG but not in 1103P (Figure 4A). No other significant changes were observed between rootstocks or in response to nitrate resupply, but the TSC concentration was 35% higher in RG young roots. The ratio of soluble C/N, estimated by the formula TSC/(FAA + Nitrate), significantly decreased for RG after nitrate resupply whereas it stayed stable for 1103P (Figure 4B).
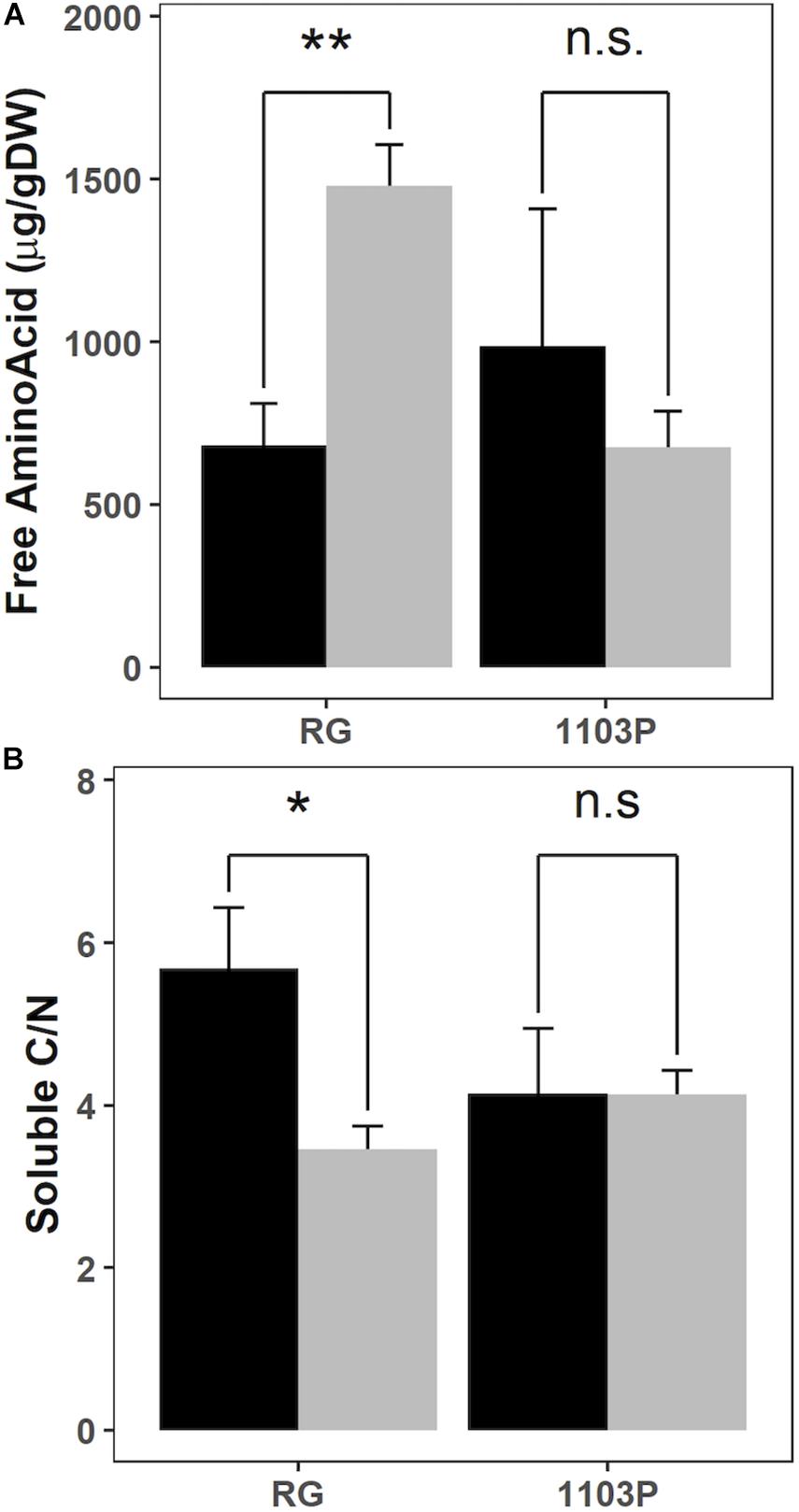
Figure 4. Evolution of free amino acids concentration (A) and soluble C/N calculated as TSC/(FAA + NO3) (B) in root tips of RG and 1103P before (black) and 24 h after (gray) 1 mM of nitrate resupply (mean ± se; n = 4). Asterisks show significant difference between rootstocks at each time point (Welch paired test, **P < 0.01*P < 0.05, n.s., non-significant).
15N Root-to-Shoot Transport
15N root-to-shoot transport was measured after 12 weeks of growth in grafted Pinot noir plants on RG and 1103P roots supplied with two levels of nitrate (Figure 5). Overall, the 15N flow rate followed the plant transpiration circadian rhythm and spiked after 4 h of labeling (5 h after sunrise) when the leaf transpiration was maximal (Supplementary Figure 3). At this time point, 1103P clearly showed a higher 15N transport than RG at both low and high nitrate availability (Figure 5). The leaf transpiration rate and 15N concentration in the xylem sap appeared to differ by rootstock at 4 h (Supplementary Figure 3). Rootstock affected carbon assimilation and stomatal behavior of the Pinot noir scion depending on nitrate availability (Supplementary Figure 3). In LN treatment, 1103P induced a higher carbon assimilation at 8 h and higher stomatal conductance at 0 and 8 h compared to RG. In HN treatment, stomatal conductance of 1103P was higher than that of RG at 0 and 4 h, while no differences were found for carbon assimilation (Supplementary Figure 3).
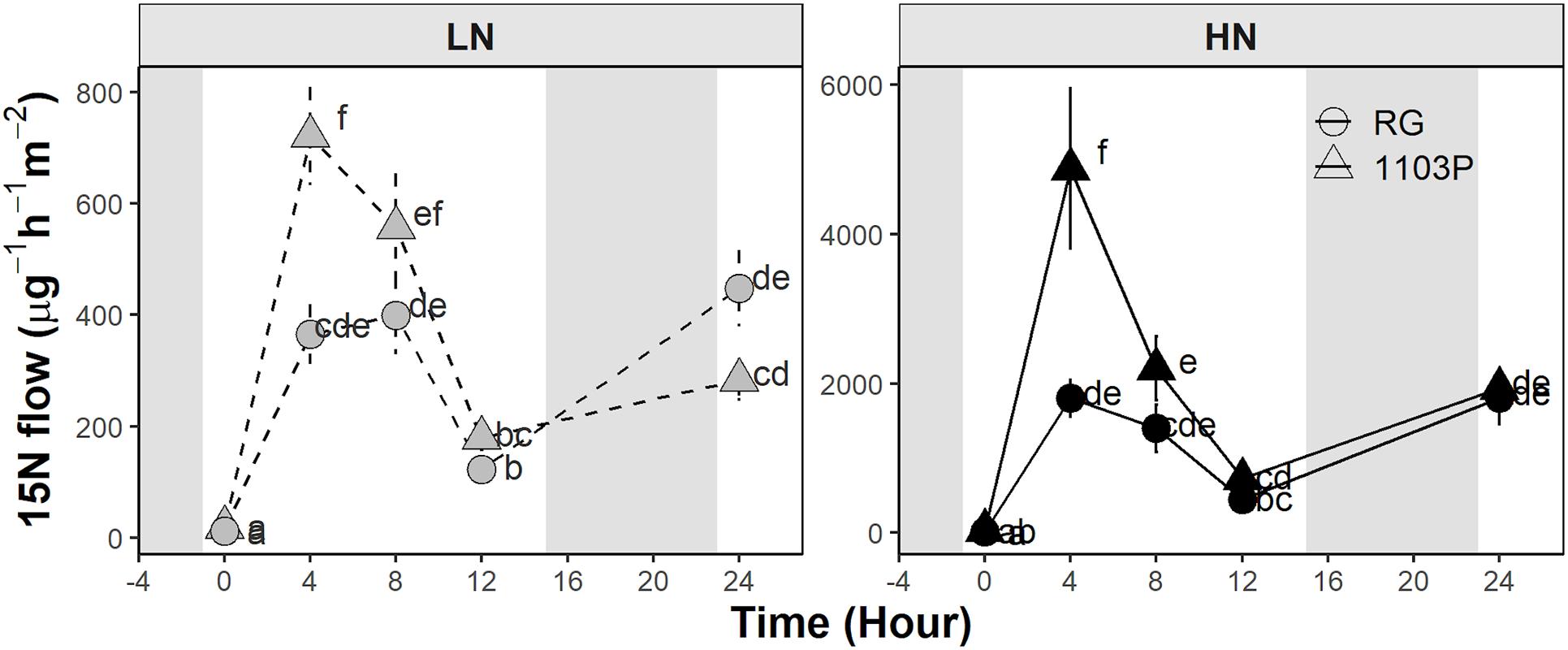
Figure 5. Flow of 15N measured in leaf xylem sap of Pinot Noir grafted to RG (circle) and 1103P (triangle) after 12 weeks of growth in LN (0.8 mM, gray) and HN (2.4 mM, black) solutions (mean ± se, n = 5–7). Letters refers to a significant difference between groups for each treatment separately (ANOVA, P < 0.05).
Gene Expression in Root Under Steady-State Nitrate Supply
Except for VitviNAXT, all other genes examined showed a diurnal variation in the first 8 h of light (Figure 6 and Supplementary Figure 4). When considering all data together, VitviCIPK23 and VitviHY5 expression decreased, VitviBTB and VitviNRT1.5 expression increased, whereas nitrate uptake and assimilation genes peaked at 4 h before decreasing at 8 h to a level similar to 0 h. VitviNTR1.1, VitviNRT2.4A, VitviNRT2.4B, VitviHY5 showed differences between rootstock depending on nitrate availability. VitviNRT1.1 expression was higher for RG only in LN treatment whereas VitviNRT2.4A and VitviNRT2.4B expressions were higher in RG only in HN treatment. A trend for more expression of VitviHY5 at 0 h for 1103P was observed but it did not reach statistical significance (Supplementary Figure 4). For VitviGS2, VitviNRT3, VitviNAXT, and VitviCIPK23, the rootstocks differences were not dependent on nitrate availability. VitviGS2, VitviNRT3, and VitviCIPK23 was higher for RG than 1103P whereas the opposite was observed for VitviNAXT expression. No differences between rootstocks were observed for VitviBTB, VitviNR, and VitviNRT1.5 (Supplementary Figure 4).
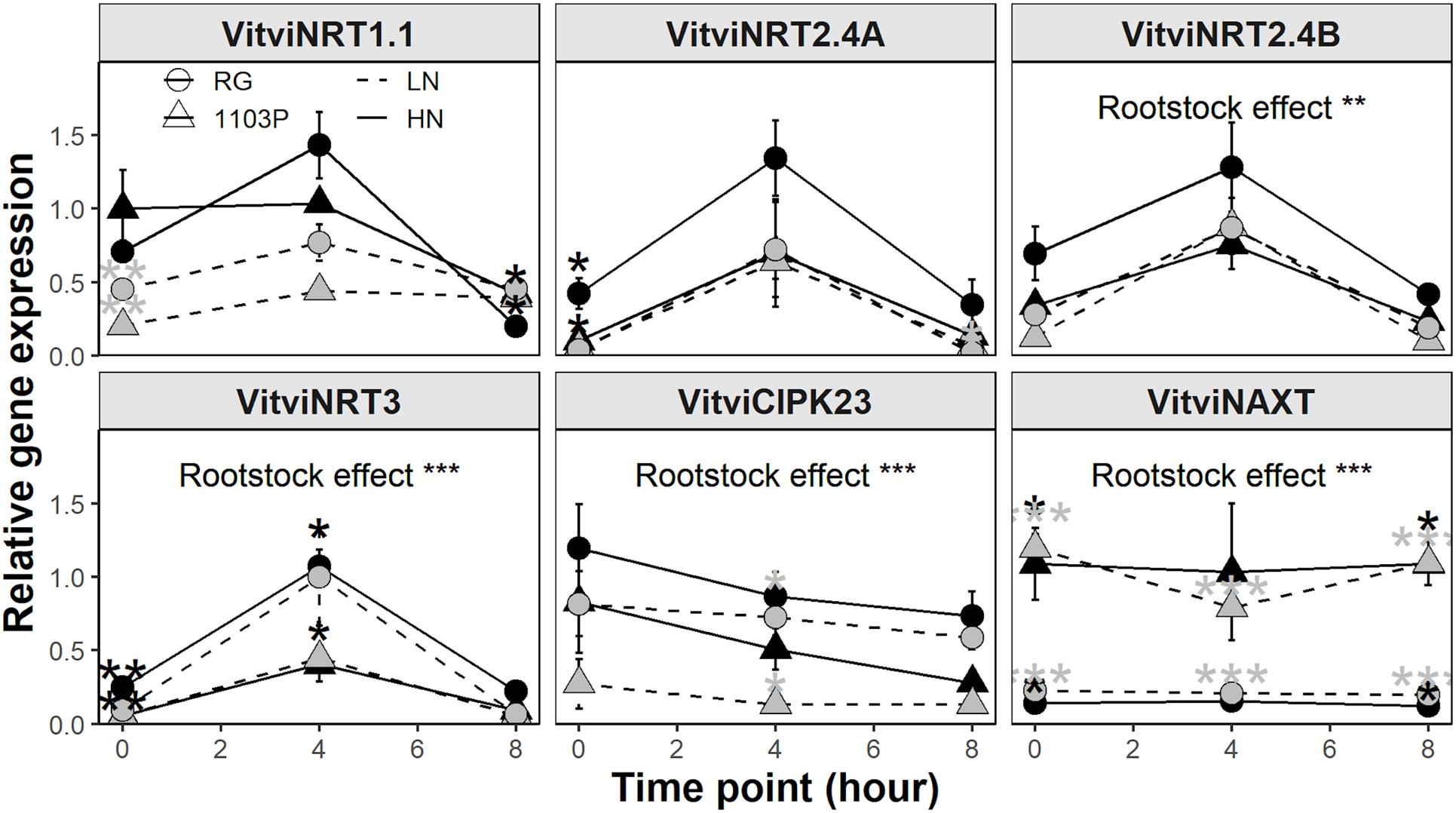
Figure 6. Relative gene expression of VitviNRT1.1, VitviNRT2.4A, VitviNRT2.4B, VitviNRT3, VitviCIPK23, and VitviNAXT in roots tips (mean ± se; n = 3) of RG (circle) and 1103P (triangle) after 12 weeks of growth in LN (0.8 mM, gray) and HN (2.4 mM, black) solutions. Time point correspond to collection time after exposure to labeled solutions (15N, 50%). Asterisks show significant difference between rootstocks at each time point for each nutrient solution (t-test, *P < 0.05, **P < 0.01; ***P < 0.001). Overall rootstock differences are presented (ANOVA, **P < 0.01; ***P < 0.001).
Biomass Allocation
Biomass allocation to roots, trunks, stems and leaves were measured for Pinot noir grafted to RG and 1103P after 12 weeks of growth under LN and HN treatments (Figure 7). Nitrate treatment affected biomass allocation in all tissues in a similar manner between rootstocks. Regardless of the rootstock, allocation of biomass was greater in roots and trunks and lesser in stems and leaves under N-limitation (LN) in comparison with HN treatment. We also observed a greater allocation of biomass to root tissues in RG at the expense of stem and trunk tissues. Lower allocation of biomass to the stem in RG under LN treatment is supported by the weekly measure of stem length during the growth period, where significant difference between rootstocks appeared after the fifth week of LN treatment (Supplementary Figure 5). Total plant biomass increased in response to increasing nitrate availability but did not differ between rootstocks (Supplementary Figure 6).
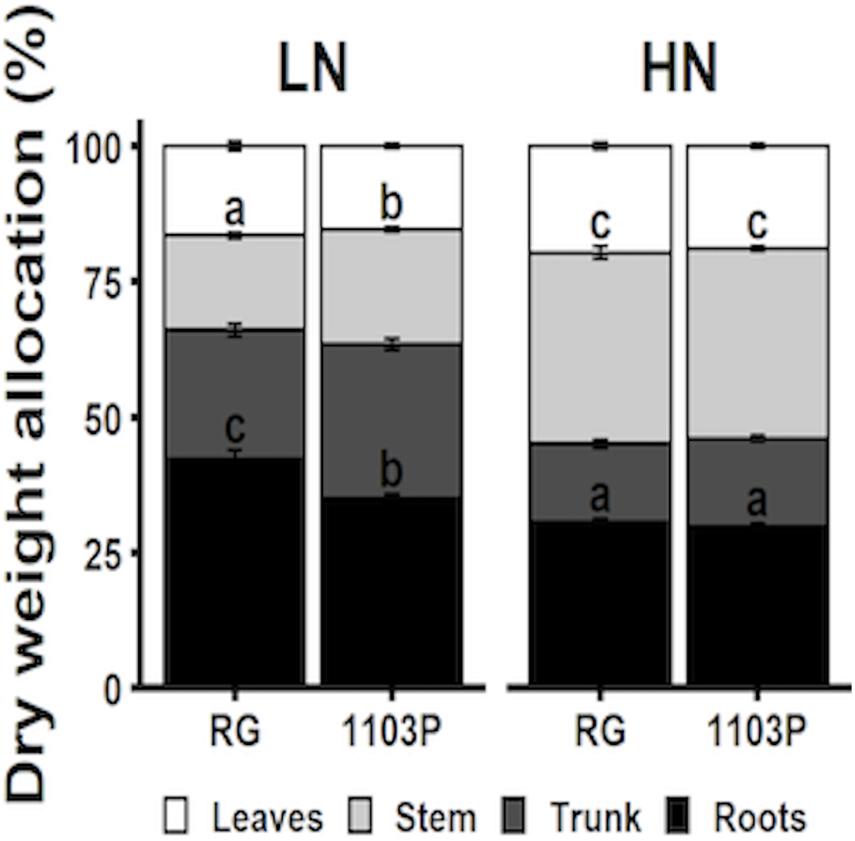
Figure 7. Allocation of total biomass (%) distributed in roots (dark), trunk (dark gray), stem (light gray), and leaves (white) of Pinot Noir grafted to RG and 1103P after 12 weeks of growth under LN (0.8 mM, gray) and HN (2.4 mM, black) solutions (n = 6–8, mean ± se). Letters when present shows significant interaction response between rootstocks and treatment per tissue (two-way ANOVA, P < 0.05). Absolute dry biomass allocation is provided in Supplementary Figure 6.
Metabolite Allocation
Nitrate, FAA, TSC, and starch concentrations were measured in leaves and roots after 12 weeks of growth under LN and HN treatment (Supplementary Figure 7). In leaves, N limitation (LN) enhanced nitrate and starch accumulation and decreased TSC and FAA concentration. In roots, only the concentration of FAA was affected by the N treatment. Increasing N availability, increased FAA concentration in roots, and the intensity of the response was higher for 1103P than RG (Table 3).
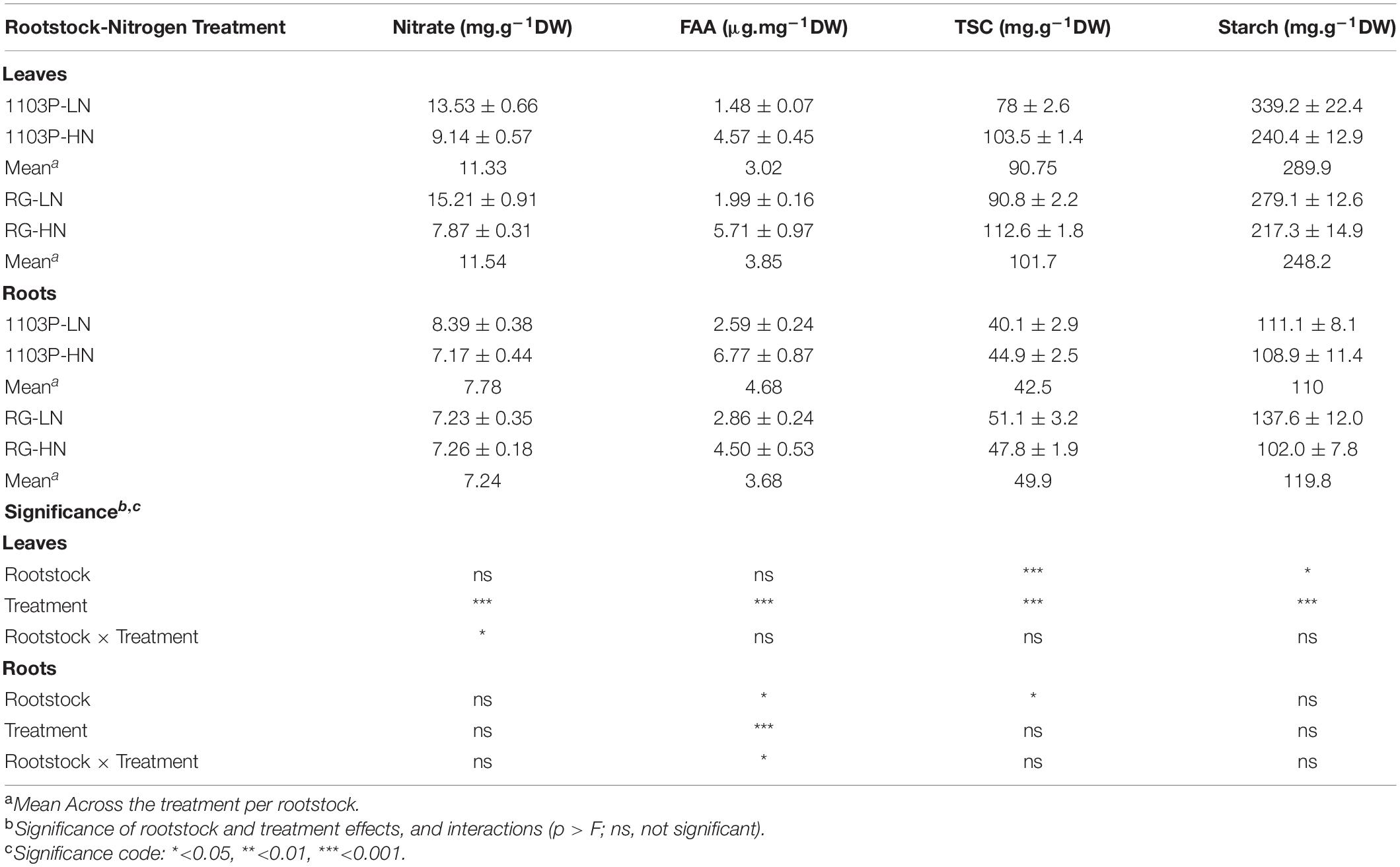
Table 3. Nitrate, FAA, TSC and starch as affected by Nitrogen treatment (HN and LN) and Rootstocks (1103P and RG) in leaves and root samples after 12 weeks of nitrogen supply.
Carbon allocation in leaves and roots was influenced by the rootstocks. For RG, TSC concentration in leaves and in roots was higher than 1103P whereas the opposite was observed for starch concentration in leaves. These differences in carbon metabolite preference and allocation between rootstocks seem accentuated in LN condition (Supplementary Figure 7). Total C/N ratio, estimated by the formula (TSC + Starch)/(FAA + Nitrate), illustrated the different rootstock strategy in allocation of C and N metabolites between leaves and roots (Figure 8). While total C/N ratio in leaves and roots were not dependent on the rootstock under HN, N limitation resulted in higher proportion of C metabolites in leaves for 1103P and in roots for RG.
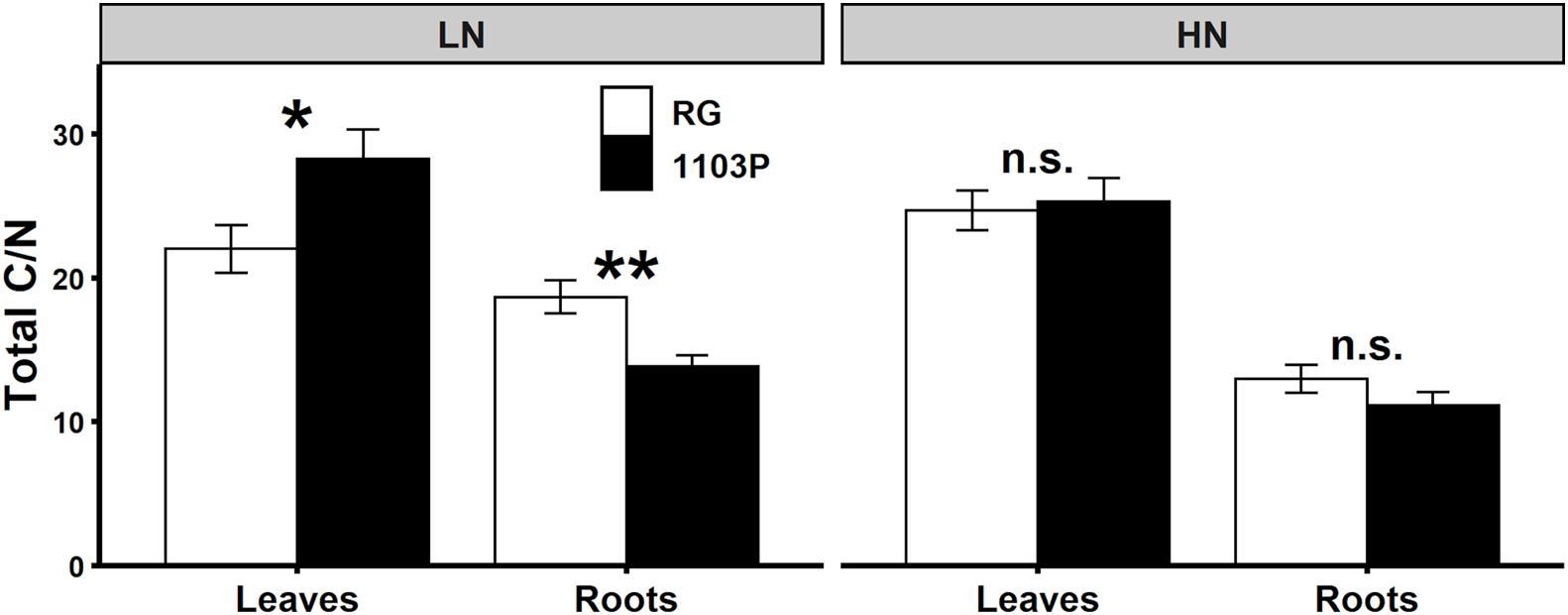
Figure 8. Ratio of total C/N in leaves and roots of Pinot Noir grafted to RG (white) and 1103P (black) after 12 weeks of growth under LN (0.8 mM) and HN (2.4 mM) solutions (n = 6–8, mean ± se). Total C is the addition of TSC and starch, and total N is the addition of FAA and nitrate. Asterisks show significant difference between rootstocks for each tissue and each treatment (t-test, *P < 0.05, **P < 0.01; n.s., non-significant).
Discussion
The overall objective of our study was to compare nitrate uptake and its root-to-shoot transport between two rootstocks known to differ in their capacity to stimulate scion growth in grafted grapevines. Our study revealed that nitrate uptake capacity and biomass adaptation to N-limiting conditions are enhanced in the less vigorous rootstock (RG). These differences can be explained by a greater partitioning of C metabolites to the roots in RG as compared to 1103P. By contrast, the transpiration rate and N loading into xylem sap contributed to higher root-to-shoot transport of N in the vigorous rootstock (1103P). Higher transport of N to leaves with 1103P rootstock will promote N assimilation that can maintain shoot growth under LN conditions and increase FAA storage in roots under HN conditions. Overall, rootstocks did not affect the total plant biomass but appeared to contribute to a modification of biomass allocation in N-limited conditions. This is likely caused by a different ability of the rootstocks to control C and N metabolism and/or to transport water and mineral nutrients to the scion.
Nitrate Uptake in Grapevine
Our experiments reinforce the established knowledge regarding induced nitrate uptake mechanisms in young grapevine roots. Previous studies demonstrated that grapevine HATS and LATS were induced after nitrate resupply (Yang et al., 2007; Pii et al., 2014; Tomasi et al., 2015; Kulmann et al., 2020), and their kinetic parameters were dependent on both rootstock and scion genotypes (Tomasi et al., 2015; Kulmann et al., 2020) or under spatial restricting conditions impeding the growth of the root system (Yang et al., 2007). Our results confirm the induction of both HATS and LATS and a faster response to the nitrate resupply compared to what is observed in other woody species (Tomasi et al., 2015).
Our work also revealed a dual Michaelis-Menten curve under low nitrate availability (<1 mM), which seems to validate that grapevine HATS consists of more than one high affinity nitrate transporter (Tomasi et al., 2015). In Arabidopsis thaliana, NRT2.1 is the major HATS controlling 75% of nitrate uptake under low nitrate availability (Cerezo et al., 2001; Filleur et al., 2001; Li et al., 2007). In response to heterogenous and homogenous N resupply, the expression of VitviNRT2.4A, of AtNRT2.1 putative ortholog, is higher in RG than in 1103P (Cochetel et al., 2017, 2019; Figure 3). Because the difference in nitrate uptake was observed only during the second phase of the HATS kinetic pattern (Figures 2C,D), it is possible that VitviNRT2.4A is responsible for HATS activity in this concentration range. Though, it is still unclear whether VitviNRT2.4A acts alone in the higher nitrate uptake of RG. VitviNRT3, the closest ortholog of AtNRT3, shows an identical induction pattern of expression to VitviNRT2.4A (Figure 3). AtNRT3 is essential to regulate the activity of HATS by formation of a tetrameric protein complex with AtNRT2.1 (Orsel et al., 2006; Yong et al., 2010; Kotur et al., 2012). Further experiments will be needed to determine the contribution of each transporter in the inducible HATS activity in young primary roots of grapevine.
Our results confirm the differential regulation of VitviBTB between rootstocks upon nitrate resupply (Figure 3) as proposed by Cochetel et al., 2017. However, neither nitrate availability nor rootstock genotype affected VitviBTB expression under steady state N supply (Supplementary Figure 4). Gene regulation of AtBT2, the closest homolog of VitviBTB in Arabidopsis thaliana, is dependent on the direct activity of NIN-LIKE PROTEIN (NLP) transcription factors (Sato et al., 2017), which modulates the majority of nitrate signaling and assimilation genes during the Primary Nitrate Response (PNR) (Marchive et al., 2013). AtBT2 was shown to be a negative regulator of Nitrogen Use Efficiency (NUE) decreasing the expression of both AtNRT2.1 and AtNRT2.4, and the nitrate uptake under low nitrate availability (Araus et al., 2016). In fact, AtBT2 protein mediates multiple responses to nutrients, stresses and hormones (Mandadi et al., 2009) and may function as ubiquitin ligase to target specific proteins for degradation in response to calcium signaling (Misra et al., 2018) or UV-B treatment (An et al., 2019). Upregulation of VitviBTB only in RG could be the consequence of the more pronounced transcriptomic reprogramming generally observed during PNR among grapevine rootstocks (Cochetel et al., 2017). Further studies focusing at the protein level should be considered to determine the contribution of post-translational modifications to the different behavior of rootstocks to nitrate.
Responsiveness of Riparia Gloire to N Availability Is Greater Than 1103P by Remobilizing C Metabolites Toward the Roots
The marked response of nitrate uptake and gene expression for nitrate transporters in RG roots (Figures 1–3) can be explained by differences in the management of C and N metabolites (Figure 4 and Supplementary Figure 2). We observed that FAA accumulated only in RG roots 24 h after nitrate induction (Figure 4A). The induced expression of VitviGS2 ortholog in RG roots strengthens the hypothesis for enhanced N assimilation. N assimilation genes are known to be upregulated in roots by the level of nitrate and soluble sugars (Lejay et al., 2008; Bussell et al., 2013), and downregulated by amino acids (Tegeder and Masclaux-Daubresse, 2018). Overall, the greater soluble C/N ratio observed at t0h in RG (Figure 4B) might suggest a better or more rapid ability to respond to nitrate resupply than 1103P. This greater ratio was more associated with the TSC concentration that is 35% higher in RG roots compared to 1103P at t0h. Higher level of sugars in roots could enhance the nitrate uptake rate and gene regulation of nitrate transporters (Lejay et al., 1999; Guo et al., 2017), which could contribute to the differences in gene expression observed between the two rootstocks for VitviNRT2.4A and VitviNRT3.
A strong connection between total C/N ratio in root and plant biomass allocation is observed under steady state N supply. Under LN, higher TSC concentration (Supplementary Figure 7) is associated to higher biomass allocation toward the roots in RG rather than in 1103P. These differences in total C/N ratio disappear in HN availability consistent with similar biomass allocation for both rootstocks (Figure 7). Although biomass partitioning is known to respond to C/N ratio in grapevine (Grechi et al., 2007), this is the first report describing rootstock specificity in the allocation of C metabolites in roots without affecting the overall plant biomass. Root development in RG will compete with shoot growth for C and N resources especially in N-limited conditions with a greater tendency of RG to reduce the nitrate in roots. This could explain the lower shoot vigor typically conferred to the scion by RG, as opposed to poor phloem compatibility reducing C allocation to roots and reducing the overall size of the root system (Bieleski, 2000; Olmstead et al., 2006). However, more work is needed to confirm this, and whether or not such a mechanism only operates in young vines. Interestingly, the rate of photoassimilation or C content in leaves cannot explain the better root allocation of C in RG (Supplementary Figures 3, 7). However, rootstocks can actively modulate C flux through hormonal regulation. In grapevine, abscisic acid enhances C allocation toward roots and berries (Moreno et al., 2011), possibly by the regulation of sugar-related SWEET transporters (Meteier et al., 2019). Although, we did not validate this hypothesis, the lower stomatal conductance and transpiration rates under HN for RG during the highest transpiration period of the day (Supplementary Figure 3) suggests that a higher level of abscisic acid was present in the scion with RG, which in turn could promote the transport of sugars toward the roots as proposed by Meteier et al. (2019). A stomatal closure induced by ABA could reduce the rate of photoassimilation in leaves, which in turn would reduce the flow of carbon moving toward sinks like roots. More research is needed to understand rootstock effect on hydraulic properties and C and N source/sink strength between shoot and root growth.
1103P Rootstock Favors N Flux Toward Scion
Regardless of N availability, N flow in leaf xylem sap (Figure 5) was higher in 1103P, especially at 4 h when leaf transpiration is at the maximum (Supplementary Figure 3). Despite differences in N flow rate between rootstocks, nitrate and FAA were almost identical in scion leaves. This result could suggest that 1103P has a greater rate of assimilation of the newly transported nitrate that may promote shoot growth under limited conditions (Figure 7). Under non-limiting conditions, both rootstocks fulfilled the N demand of the scion in terms of N assimilation, but higher N flow of plants grafted to 1103P may translate into a greater reallocation of FAA in 1103P roots. Further investigations are needed to determine if the ability of 1103P to reallocate more FAA in the roots under these conditions is consistent across years. This adaptive strategy could be important for sustaining shoot growth in N-fluctuating field environments. Further, it is unknown whether this strategy leads to more developed root systems in 1103P which could explain its influence on shoot vigor; this needs to be validated in field experiments.
In the N-steady state experiment, differences in N xylem flow between rootstocks were noted through variations in N xylem sap concentration and the transpiration stream (Supplementary Figure 3). While xylem loading and transpiration are independent processes, their cumulative effects mutually impact N xylem flow (Macduff and Bakken, 2003; Siebrecht et al., 2003). We did not measure N uptake in this experiment, so nitrate uptake and xylem loading cannot be estimated. The greater expression of VitviNRT3 orthologs, a possible activator of the HATS, suggests a greater N-uptake in RG in this steady-state experiment (Figure 6). However, the N concentration in the xylem sap was lower in RG, and the concentration of nitrate and FAA did not suggest a higher N accumulation in RG roots (Supplementary Figure 7). The greater nitrate uptake capacity measured from root tips (Figures 1, 2) may not translate into a greater overall nitrate uptake into the roots. Recent advances on NRT1.1 demonstrate that its phosphorylation status determines whether it will act as nitrate or auxin transporters. Phosphorylation of NRT1.1 caused by CIPK23 in Arabidopsis (Ho et al., 2009), promotes the basipetal transport of auxin in fine roots, thereby inhibiting the formation of lateral roots (Liu and Tsay, 2003; Bouguyon et al., 2015, 2016; Zhang et al., 2019). We observed that the expression of the AtCIPK23 ortholog, VitviCIPK23 (Griesser et al., 2017; Xi et al., 2017), was higher in RG root tips regardless of N availability (Figure 6). While the N uptake in RG seems higher at the root tip level, this rootstock was shown to limit the formation of lateral roots when compared with 1103P (Cochetel et al., 2019), which could limit nitrate uptake, and thereby could reduce the global N uptake. Moreover, the phosphorylation of NRT1.1 by CIPK23 limits its uptake capacity when N is available (Wang C. et al., 2018), which could explain the lack of FAA accumulation in RG root under HN.
Could VitviNAXT Be a Xylem Loading NO3 Transporter?
In our experiments, VitviNAXT transcript abundance was not affected by N resupply and N plant status whereas its expression was significantly higher in 1103P than in RG (Figures 3, 7). In Arabidopsis, NAXT1/NPF2.7 has been demonstrated to regulate nitrate efflux to the media (Segonzac et al., 2007). Function of VitviNAXT as a nitrate efflux transporter appears unlikely, as its expression is much higher in 1103P in which we observed higher N translocation rate. VitviNAXT has not been functionally characterized in grapevine, but it may participate in rootstock chloride exclusion of rootstocks under salt stress (Henderson et al., 2014). AtNAXT1 is a NRT1 transporter part of the NPF2a clade containing seven members (Segonzac et al., 2007). Three of them demonstrated ion transport activity. Two members, NPF2.4 (Li B. et al., 2016) and NPF2.5 (Li et al., 2017), have been characterized in Arabidopsis and participate to chloride loading into the xylem and chloride efflux into the media, respectively. The third member, AtNPF2.3, contributes more to the nitrate xylem loading under salt stress (Taochy et al., 2015). KO mutants of AtNRT1.5/NPF7.3 showed only partial limitation of translocation rate to the xylem (Lin et al., 2008); its loss of function under salt stress was found to be compensated by the functional redundancy of AtNPF2.3 (Taochy et al., 2015). Like AtNPF2.3, VitviNAXT is not responsive to salt stress (Henderson et al., 2014). However, functional characterization will be needed to determine whether VitviNAXT can transport nitrate.
Rootstock differences in N translocation to the shoot is partially explained by their impact on scion transpiration (Supplementary Figure 3). In the HN condition, 1103P displayed increased scion stomatal conductance and transpiration leading to higher xylem sap flow than RG, especially during high transpiration demand (at 4 and 8 h). In the LN condition, this observation was true only at 8 h and could be associated with partial stomatal closure for RG probably caused by lower leaf water potential at 4 h (Supplementary Figure 3). This transpiration behavior under LN conditions during a specific time of the day in 1103P were also confirmed when the daily transpiration rate of the plants was calculated 1 day before the 15N was supplied (Supplementary Figure 8). Altogether, these higher transpiration patterns observed with 1103P would facilitate greater root-to-shoot transport of nutrients and hormones but also increases the mass flow movement of soluble nutrients in the soil toward the root surface (Cramer et al., 2009). In addition to a deeper rooting phenotype for 1103P (Cochetel et al., 2019), the promotion of scion transpiration with 1103P might increase nutrient acquisition by facilitating bulk flow of soluble nutrients like nitrate in soil toward the root surface. In grapevine, scion transpiration and shoot vigor are tightly correlated to the root hydraulic conductance and the activity of root aquaporins (Vandeleur et al., 2009; Gambetta et al., 2012; Perrone et al., 2012; Zhang et al., 2016). Nitrate availability modulates transcription and/or activity of root aquaporins in many species to optimize nitrate uptake in homogenous or heterogenous availability (Gloser et al., 2007; Gorska et al., 2008a,b; Ishikawa-Sakurai et al., 2014; Li G. et al., 2016). Thus, the variation of root hydraulic conductivity under the control of aquaporin activity among rootstocks (Lovisolo et al., 2008; Gambetta et al., 2012) may also contribute to the rootstock performance in its capacity to facilitate the transport of nitrate toward the upper part of the plants in limited and heterogenous nitrate availability.
Conclusion
The physiological communication between scions and rootstocks is essential to regulate plant development and the acquisition of C and N. In our experiments, the contribution of the rootstock to scion vigor was observed only when N supply was limited and resulted in the alteration of biomass allocation rather than total biomass production. Biomass allocation to the roots and remobilization of C resources from shoot-to-root are part of the adaptive strategy under N-limiting conditions to stimulate N uptake in plants. Here, we demonstrated that two commonly used commercial rootstocks possess distinct strategies to transport, assimilate, and allocate N in the plant. Our findings also suggest that the greater capacity of 1103P to translocate N to shoots, possibly through an increase of transpiration, would allow greater shoot growth under limiting conditions. The capacity of different rootstocks to affect the regulation of transpiration to the scion may be considered a major contributing factor to explain the differences observed in grapevine C and N status. Further experiments including manipulation of grapevine water status should be conducted to validate the influence of transpiration on N uptake, its root-to-shoot transport, and its assimilation in grapevines. By contrast, the higher responsiveness of RG with its capacity to put more N resources in roots rather than in shoots appears to be a more conservative strategy to allocate N to perennial tissues. In both rootstocks, it is unclear whether these mechanisms are controlled directly by the rootstock itself or by interactions with the scion through a feedback loop mechanism. Further investigation is needed to clarify the roles played by both scions and rootstocks on the regulatory activity of the other partner in grafted grapevines with respect to C and N acquisition. Ultimately, whether these distinct behaviors between both rootstocks are maintained over seasons and in the field have yet to be addressed.
Data Availability Statement
The original contributions presented in the study are included in the article/Supplementary Material, further inquiries can be directed to the corresponding author.
Author Contributions
LR, RS, PS, and LD conceived and planned the study. LR, RS, and LD collected the samples and LR processed the samples for nitrate uptake, gene expression, biomass allocation, and metabolite and physiological measurements. LR performed all the statistical analyses and wrote the body of the manuscript with LD. All authors reviewed and approved the manuscript.
Funding
The authors thank the College of Agricultural Sciences and the Oregon Wine Research Institute, both of Oregon State University, for their financial support.
Conflict of Interest
The authors declare that the research was conducted in the absence of any commercial or financial relationships that could be construed as a potential conflict of interest.
Acknowledgments
The authors thank Duarte Nursery Inc., for providing plant material and Matthew Scott for assisting LR in the collection and the data measurements.
Supplementary Material
The Supplementary Material for this article can be found online at: https://www.frontiersin.org/articles/10.3389/fpls.2020.608813/full#supplementary-material
Abbreviations
1103P, 1103 Paulsen; RG, Riparia Gloire; LATS, Low Affinity Transporter System; HATS, High Affinity Transporter System; FAA, Free Amino Acids; TSC, Total Soluble Carbohydrates; ns, not significant; se, standard error.
References
Albacete, A., Martínez-Andújar, C., Martínez-Pérez, A., Thompson, A. J., Dodd, I. C., and Pérez-Alfocea, F. (2015). Unravelling rootstock×scion interactions to improve food security. J. Exp. Bot. 66, 2211–2226. doi: 10.1093/jxb/erv027
An, J., Wang, X., Zhang, X., Bi, S., You, C., and Hao, Y. (2019). Md BBX 22 regulates UV -B-induced anthocyanin biosynthesis through regulating the function of Md HY 5 and is targeted by Md BT 2 for 26S proteasome-mediated degradation. Plant Biotechnol. J. 17, 2231–2233. doi: 10.1111/pbi.13196
Araus, V., Vidal, E. A., Puelma, T., Alamos, S., Mieulet, D., Guiderdoni, E., et al. (2016). Members of BTB gene family of scaffold proteins suppress nitrate uptake and nitrogen use efficiency. Plant Physiol. 171, 1523–1532. doi: 10.1104/pp.15.01731
Atkinson, C. J., Else, M. A., Taylor, L., and Dover, C. J. (2003). Root and stem hydraulic conductivity as determinants of growth potential in grafted trees of apple (Malus pumila Mill.). J. Exp. Bot. 54, 1221–1229. doi: 10.1093/jxb/erg132
Bieleski, R. L. (2000). The bigger picture - phloem seen through horticultural eyes. Aust. J. Plant Physiol. 27, 615–624. doi: 10.1071/PP99148/0310-7841/00/060615
Bouguyon, E., Brun, F., Meynard, D., Kubeš, M., Pervent, M., Leran, S., et al. (2015). Multiple mechanisms of nitrate sensing by Arabidopsis nitrate transceptor NRT1.1. Nat. Plants 1:15015. doi: 10.1038/nplants.2015.15
Bouguyon, E., Perrine-Walker, F., Pervent, M., Rochette, J., Cuesta, C., Benkova, E., et al. (2016). Nitrate controls root development through post-transcriptional regulation of the NRT1.1/NPF6.3 transporter/sensor. Plant Physiol. 172, 1237–1248. doi: 10.1104/pp.16.01047
Bussell, J. D., Keech, O., Fenske, R., and Smith, S. M. (2013). Requirement for the plastidial oxidative pentose phosphate pathway for nitrate assimilation in Arabidopsis. Plant J. 75, 578–591. doi: 10.1111/tpj.12222
Cataldo, D. A., Maroon, M., Schrader, L. E., and Youngs, V. L. (1975). Rapid colorimetric determination of nitrate in plant tissue by nitration of salicylic acid. Commun. Soil Sci. Plant Anal. 6, 71–80. doi: 10.1080/00103627509366547
Cerezo, M., Tillard, P., Filleur, S., Muños, S., Daniel-Vedele, F., and Gojon, A. (2001). Major alterations of the regulation of root NO 3 - uptake are associated with the mutation of Nrt2.1 and Nrt2.2 genes in Arabidopsis. Plant Physiol. 127, 262–271. doi: 10.1104/pp.127.1.262
Cochetel, N., Escudié, F., Cookson, S. J., Dai, Z., Vivin, P., Bert, P.-F., et al. (2017). Root transcriptomic responses of grafted grapevines to heterogeneous nitrogen availability depend on rootstock genotype. J. Exp. Bot. 68, 4339–4355. doi: 10.1093/jxb/erx224
Cochetel, N., Hévin, C., Vivin, P., Ollat, N., and Lauvergeat, V. (2019). Grapevine rootstocks differentially regulate root growth and architecture in response to nitrogen availability. Acta Hortic. 1248, 521–530. doi: 10.17660/ActaHortic.2019.1248.70
Cramer, M. D., Hawkins, H.-J., and Verboom, G. A. (2009). The importance of nutritional regulation of plant water flux. Oecologia 161, 15–24. doi: 10.1007/s00442-009-1364-3
Crawford, N. M., and Glass, A. D. M. (1998). Molecular and physiological aspects of nitrate uptake in plants. Trends Plant Sci. 3, 389–395. doi: 10.1016/S1360-1385(98)01311-9
Dechorgnat, J., Nguyen, C. T., Armengaud, P., Jossier, M., Diatloff, E., Filleur, S., et al. (2011). From the soil to the seeds: the long journey of nitrate in plants. J. Exp. Bot. 62, 1349–1359. doi: 10.1093/jxb/erq409
Edwards, E. J., Downie, A. F., and Clingeleffer, P. R. (2011). A simple microplate assay to quantify nonstructural carbohydrates of grapevine tissues. Am. J. Enol. & Vit. 62, 133–137. doi: 10.5344/ajev.2010.10051
Edwards, E. J., Collins, M. J., Boettcher, A., Clingeleffer, P. C., and Walker, R. R. (2014). The role of rootstocks in grapevine water use efficiency: impacts on transpiration, stomatal control and yield efficiency. Acta Hortic. 1038, 121–128. doi: 10.17660/actahortic.2014.1038.13
Filleur, S., Dorbe, M.-F., Cerezo, M., Orsel, M., Granier, F., Gojon, A., et al. (2001). An Arabidopsis T-DNA mutant affected in Nrt2 genes is impaired in nitrate uptake. FEBS Lett. 489, 220–224. doi: 10.1016/S0014-5793(01)02096-8
Gambetta, G. A., Manuck, C. M., Drucker, S. T., Shaghasi, T., Fort, K., Matthews, M. A., et al. (2012). The relationship between root hydraulics and scion vigour across Vitis rootstocks: What role do root aquaporins play? J. Exp. Bot. 63, 6445–6455. doi: 10.1093/jxb/ers312
Gautier, A. T., Chambaud, C., Brocard, L., Ollat, N., Gambetta, G. A., Delrot, S., et al. (2019). Merging genotypes: graft union formation and scion–rootstock interactions. J. Exp. Bot. 70, 747–755. doi: 10.1093/jxb/ery422
Gloser, V., Zwieniecki, M. A., Orians, C. M., and Holbrook, N. M. (2007). Dynamic changes in root hydraulic properties in response to nitrate availability. J. Exp. Bot. 58, 2409–2415. doi: 10.1093/jxb/erm118
Goncalves, B., Correia, C. M., Silva, A. P., Bacelar, E. A., Santos, A., Ferreira, H., et al. (2007). Variation in xylem structure and function in roots and stems of scion-rootstock combinations of sweet cherry tree (Prunus avium L.). Trees 21, 121–130. doi: 10.1007/s00468-006-0102-2
Gorska, A., Ye, Q., Holbrook, N. M., and Zwieniecki, M. A. (2008a). Nitrate control of root hydraulic properties in plants: translating local information to whole plant response. Plant Physiol. 148, 1159–1167. doi: 10.1104/pp.108.122499
Gorska, A., Zwieniecka, A., Michele Holbrook, N., and Zwieniecki, M. A. (2008b). Nitrate induction of root hydraulic conductivity in maize is not correlated with aquaporin expression. Planta 228, 989–998. doi: 10.1007/s00425-008-0798-x
Grechi, I., Vivin, P. H., Hilbert, G., Milin, S., Robert, T., and Gaudillère, J.-P. (2007). Effect of light and nitrogen supply on internal C:N balance and control of root-to-shoot biomass allocation in grapevine. Environ. Exp. Bot. 59, 139–149. doi: 10.1016/j.envexpbot.2005.11.002
Griesser, M., Crespo Martinez, S., Weidinger, M. L., Kandler, W., and Forneck, A. (2017). Challenging the potassium deficiency hypothesis for induction of the ripening disorder berry shrivel in grapevine. Sci. Hortic. 216, 141–147. doi: 10.1016/j.scienta.2016.12.030
Guo, Q., Turnbull, M. H., Song, J., Roche, J., Novak, O., Späth, J., et al. (2017). Depletion of carbohydrate reserves limits nitrate uptake during early regrowth in Lolium perenne L. J. Exp. Bot. 68, 1569–1583. doi: 10.1093/jxb/erx056
Hawkins, B. J., Robbins, S., and Porter, R. B. (2014). Nitrogen uptake over entire root systems of tree seedlings. Tree Physiol. 34, 334–342. doi: 10.193/treephys/tpu005
Henderson, S. W., Baumann, U., Blackmore, D. H., Walker, A. R., Walker, R. R., and Gilliham, M. (2014). Shoot chloride exclusion and salt tolerance in grapevine is associated with differential ion transporter expression in roots. BMC Plant Biol. 14:273. doi: 10.1186/s12870-014-0273-8
Ho, C.-H., Lin, S.-H., Hu, H.-C., and Tsay, Y.-F. (2009). CHL1 functions as a nitrate sensor in plants. Cell 138, 1184–1194. doi: 10.1016/j.cell.2009.07.004
Ibacache, A., Verdugo-Vásquez, N., and Zurita-Silva, A. (2020). “Rootstock: scion combinations and nutrient uptake in grapevines,” in Fruit Crops, ed. A. K. Srivastava (Amsterdam: Elsevier), 297–316. doi: 10.1016/B978-0-12-818732-6.00021-6
Ishikawa-Sakurai, J., Hayashi, H., and Murai-Hatano, M. (2014). Nitrogen availability affects hydraulic conductivity of rice roots, possibly through changes in aquaporin gene expression. Plant Soil 379, 289–300. doi: 10.1007/s11104-014-2070-4
Jones, T. H., Cullis, B. R., Clingeleffer, P. R., and Rühl, E. H. (2009). Effects of novel hybrid and traditional rootstocks on vigour and yield components of Shiraz grapevines. Aust. J. Grape Wine Res. 15, 284–292. doi: 10.1111/j.1755-0238.2009.00061.x
Köhler, B., Wegner, L. H., Osipov, V., and Raschke, K. (2002). Loading of nitrate into the xylem: apoplastic nitrate controls the voltage dependence of X-QUAC, the main anion conductance in xylem-parenchyma cells of barley roots. Plant J. 30, 133–142. doi: 10.1046/j.1365-313X.2002.01269.x
Kotur, Z., Mackenzie, N., Ramesh, S., Tyerman, S. D., Kaiser, B. N., and Glass, A. D. M. (2012). Nitrate transport capacity of the Arabidopsis thaliana NRT2 family members and their interactions with AtNAR2.1. New Phytol. 194, 724–731. doi: 10.1111/j.1469-8137.2012.04094.x
Kozlowski, T. T., and Pallardy, S. G. (1997). Physiology of Woody Plants, 2nd Edn. (San Diego, CA: Academic Press), 411.
Kulmann, M. S., Sete, P. B., de Paula, B. V., Stefanello, L. O., Schwalbert, R., Schwalbert, R. A., et al. (2020). Kinetic parameters govern of the uptake of nitrogen forms in ‘Paulsen’ and ‘Magnolia’ grapevine rootstocks. Sci. Hortic. 264:109174. doi: 10.1016/j.scienta.2020.109174
Lecourt, J., Lauvergeat, V., Ollat, N., Vivin, P., and Cookson, S. J. (2015). Shoot and root ionome responses to nitrate supply in grafted grapevines are rootstock genotype dependent: rootstock and nitrogen supply affect grapevine ionome. Aust. J. Grape Wine Res. 21, 311–318. doi: 10.1111/ajgw.12136
Lejay, L., and Gojon, A. (2018). “Root nitrate uptake,” in Advances in Botanical Research, ed. C. Maurel (Amsterdam: Elsevier), 139–169. doi: 10.1016/bs.abr.2018.09.009
Lejay, L., Tillard, P., Lepetit, M., Olive, F. D., Filleur, S., Daniel-Vedele, F., et al. (1999). Molecular and functional regulation of two NO3- uptake systems by N- and C-status of Arabidopsis plants. Plant J. 18, 509–519. doi: 10.1046/j.1365-313X.1999.00480.x
Lejay, L., Wirth, J., Pervent, M., Cross, J. M.-F., Tillard, P., and Gojon, A. (2008). Oxidative pentose phosphate pathway-dependent sugar sensing as a mechanism for regulation of root ion transporters by photosynthesis. Plant Physiol. 146, 2036–2053. doi: 10.1104/pp.107.114710
Léran, S., Muños, S., Brachet, C., Tillard, P., Gojon, A., and Lacombe, B. (2013). Arabidopsis NRT1.1 is a bidirectional transporter involved in root-to-shoot nitrate translocation. Mol. Plant 6, 1984–1987. doi: 10.1093/mp/sst068
Li, B., Byrt, C., Qiu, J., Baumann, U., Hrmova, M., Evrard, A., et al. (2016). Identification of a Stelar-localized transport protein that facilitates root-to-shoot transfer of chloride in Arabidopsis. Plant Physiol. 170, 1014–1029. doi: 10.1104/pp.15.01163
Li, B., Qiu, J., Jayakannan, M., Xu, B., Li, Y., Mayo, G. M., et al. (2017). AtNPF2.5 modulates chloride (Cl-) efflux from roots of Arabidopsis thaliana. Front. Plant Sci. 7:2013. doi: 10.3389/fpls.2016.02013
Li, G., Tillard, P., Gojon, A., and Maurel, C. (2016). Dual regulation of root hydraulic conductivity and plasma membrane aquaporins by plant nitrate accumulation and high-affinity nitrate transporter NRT2.1. Plant Cell Physiol. 57, 733–742. doi: 10.1093/pcp/pcw022
Li, J.-Y., Fu, Y.-L., Pike, S. M., Bao, J., Tian, W., Zhang, Y., et al. (2010). The Arabidopsis nitrate transporter NRT1.8 functions in nitrate removal from the xylem sap and mediates cadmium tolerance. Plant Cell 22, 1633–1646. doi: 10.1105/tpc.110.075242
Li, W., Wang, Y., Okamoto, M., Crawford, N. M., Siddiqi, M. Y., and Glass, A. D. M. (2007). Dissection of the AtNRT2.1?: AtNRT2.2 inducible high-affinity nitrate transporter gene cluster. Plant Physiol. 143, 425–433. doi: 10.1104/pp.106.091223
Lin, S.-H., Kuo, H.-F., Canivenc, G., Lin, C.-S., Lepetit, M., Hsu, P.-K., et al. (2008). Mutation of the Arabidopsis NRT1.5 nitrate transporter causes defective root-to-shoot nitrate transport. Plant Cell 20, 2514–2528. doi: 10.1105/tpc.108.060244
Liu, K.-H., and Tsay, Y.-F. (2003). Switching between the two action modes of the dual-affinity nitrate transporter CHL1 by phosphorylation. EMBO J. 22, 1005–1013. doi: 10.1093/emboj/cdg118
Livak, K. J., and Schmittgen, T. D. (2001). Analysis of relative gene expression data using real-time quantitative PCR and the 2-ΔΔCT method. Methods 25, 402–408. doi: 10.1006/meth.2001.1262
Lovisolo, C., Tramontini, S., Flexas, J., and Schubert, A. (2008). Mercurial inhibition of root hydraulic conductance in Vitis spp. rootstocks under water stress. Environ. Exp. Bot. 63, 178–182. doi: 10.1016/j.envexpbot.2007.11.005
Macduff, J. H., and Bakken, A. K. (2003). Diurnal variation in uptake and xylem contents of inorganic and assimilated N under continuous and interrupted N supply to Phleum pratense and Festuca pratensis. J. Exp. Bot. 54, 431–444. doi: 10.1093/jxb/erg058
Mandadi, K. K., Misra, A., Ren, S., and McKnight, T. D. (2009). BT2, a BTB protein, mediates multiple responses to nutrients, stresses, and hormones in Arabidopsis. Plant Physiol. 150, 1930–1939. doi: 10.1104/pp.109.139220
Marchive, C., Roudier, F., Castaings, L., Bréhaut, V., Blondet, E., Colot, V., et al. (2013). Nuclear retention of the transcription factor NLP7 orchestrates the early response to nitrate in plants. Nat. Commun. 4:1713. doi: 10.1038/ncomms2650
Meteier, E., La Camera, S., Goddard, M.-L., Laloue, H., Mestre, P., and Chong, J. (2019). Overexpression of the VvSWEET4 transporter in grapevine hairy roots increases sugar transport and contents and enhances resistance to Pythium irregulare, a soilborne pathogen. Front. Plant Sci. 10:884. doi: 10.3389/fpls.2019.00884
Misra, A., McKnight, T. D., and Mandadi, K. K. (2018). Bromodomain proteins GTE9 and GTE11 are essential for specific BT2-mediated sugar and ABA responses in Arabidopsis thaliana. Plant Mol. Biol. 96, 393–402. doi: 10.1007/s11103-018-0704-2
Moreno, D., Berli, F. J., Piccoli, P. N., and Bottini, R. (2011). Gibberellins and abscisic acid promote carbon allocation in roots and berries of grapevines. J. Plant Growth Regul. 30, 220–228. doi: 10.1007/s00344-010-9186-4
Nikolaou, N., Koukourikou, M., and Karagiannidis, N. (2000). Effects of various rootstocks on xylem exudates cytokinin content, nutrient uptake and growth patterns of grapevine Vitis vinifera L. cv. Thompson seedless. Agronomie 20, 363–373. doi: 10.1051/agro:2000133
Nunes-Nesi, A., Fernie, A. R., and Stitt, M. (2010). Metabolic and signaling aspects underpinning the regulation of plant carbon nitrogen interactions. Mol. Plant 3, 973–996. doi: 10.1093/mp/ssq049
Ollat, N., Peccoux, A., Papura, D., Esmenjaud, D., Marguerit, E., Tandonnet, J.-P., et al. (2016). “Rootstocks as a component of adaptation to environment,” in Grapevine in a Changing Environment, eds H. Gerós, M. Manuela Chaves, H. Medrano Gil, and S. Delrot (Hoboken, NJ: John Wiley & Sons Ltd), 68–108. doi: 10.1002/9781118735985.ch4
Olmstead, M. A., Lang, N. S., and Lang, G. A. (2006). Examining the vascular pathway of sweet cherries grafted onto dwarfing rootstocks. Hortscience 41, 674–679. doi: 10.21273/hortsci.41.3.674
Orsel, M., Chopin, F., Leleu, O., Smith, S. J., Krapp, A., Daniel-Vedele, F., et al. (2006). Characterization of a two-component high-affinity nitrate uptake system in Arabidopsis. physiology and protein-protein interaction. Plant Physiol. 142, 1304–1317. doi: 10.1104/pp.106.085209
Perrone, I., Gambino, G., Chitarra, W., Vitali, M., Pagliarani, C., Riccomagno, N., et al. (2012). The grapevine root-specific aquaporin VvPIP2;4N controls root hydraulic conductance and leaf gas exchange under well-watered conditions but not under water stress. Plant Physiol. 160, 965–977. doi: 10.1104/pp.112.203455
Pii, Y., Alessandrini, M., Guardini, K., Zamboni, A., and Varanini, Z. (2014). Induction of high-affinity NO3– uptake in grapevine roots is an active process correlated to the expression of specific members of the NRT2 and plasma membrane H+-ATPase gene families. Funct. Plant Biol. 41, 353–365. doi: 10.1071/FP13227
R Core Team (2017). R: A Language and Environment for Statistical Computing. Vienna: R Foundation for Statistical Computing.
Reid, K. E., Olsson, N., Schlosser, J., Peng, F., and Lund, S. T. (2006). An optimized grapevine RNA isolation procedure and statistical determination of reference genes for real-time RT-PCR during berry development. BMC Plant Biol. 6:27. doi: 10.1186/1471-2229-6-27
Ruijter, J. M., Ramakers, C., Hoogaars, W. M. H., Karlen, Y., Bakker, O., Hoff, M. J. B., et al. (2009). Amplification efficiency: linking baseline and bias in the analysis of quantitative PCR data. Nucleic Acids Res. 37:e45. doi: 10.1093/nar/gkp045
Sato, T., Maekawa, S., Konishi, M., Yoshioka, N., Sasaki, Y., Maeda, H., et al. (2017). Direct transcriptional activation of BT genes by NLP transcription factors is a key component of the nitrate response in Arabidopsis. Biochem. Biophys. Res. Commun. 483, 380–386. doi: 10.1016/j.bbrc.2016.12.135
Segonzac, C., Boyer, J.-C., Ipotesi, E., Szponarski, W., Tillard, P., Touraine, B., et al. (2007). Nitrate efflux at the root plasma membrane: identification of an Arabidopsis excretion transporter. Plant Cell 19, 3760–3777. doi: 10.1105/tpc.106.048173
Siebrecht, S., Herdel, K., Schurr, U., and Tischner, R. (2003). Nutrient translocation in the xylem of poplar — diurnal variations and spatial distribution along the shoot axis. Planta 217, 783–793. doi: 10.1007/s00425-003-1041-4
Sun, S. W., Lin, Y. C., and Chen, M. J. (2006). Efficiency improvements on ninhydrin method for amino quantification. J. Food Composit. Anal. 19, 112–117. doi: 10.1016/j.jfca.2005.04.006
Tandonnet, J.-P., Cookson, S. J., Vivin, P., and Ollat, N. (2010). Scion genotype controls biomass allocation and root development in grafted grapevine. Aust. J. Grape Wine Res. 16, 290–300. doi: 10.1111/j.1755-0238.2009.00090.x
Taochy, C., Gaillard, I., Ipotesi, E., Oomen, R., Leonhardt, N., Zimmermann, S., et al. (2015). The Arabidopsis root stele transporter NPF2.3 contributes to nitrate translocation to shoots under salt stress. Plant J. 83, 466–479. doi: 10.1111/tpj.12901
Tegeder, M., and Masclaux-Daubresse, C. (2018). Source and sink mechanisms of nitrogen transport and use. New Phytol. 217, 35–53. doi: 10.1111/nph.14876
Tomasi, N., Monte, R., Varanini, Z., Cesco, S., and Pinton, R. (2015). Induction of nitrate uptake in Sauvignon Blanc and Chardonnay grapevines depends on the scion and is affected by the rootstock. Aust. J. Grape Wine Res. 21, 331–338. doi: 10.1111/ajgw.12137
Tyerman, S. D., Wignes, J. A., and Kaiser, B. N. (2017). “Root hydraulic and aquaporin responses to N availability,” in Plant Aquaporins: From Transport to Signaling, Signaling and Communication in Plants, eds F. Chaumont and S. D. Tyerman (Cham: Springer), 207–236. doi: 10.1007/978-3-319-49395-4_10
Vandeleur, R. K., Mayo, G., Shelden, M. C., Gilliham, M., Kaiser, B. N., and Tyerman, S. D. (2009). The role of plasma membrane intrinsic protein aquaporins in water transport through roots: diurnal and drought stress responses reveal different strategies between Isohydric and Anisohydric Cultivars of Grapevine. Plant Physiol. 149, 445–460. doi: 10.1104/pp.108.128645
Volder, A., Smart, D. R., Bloom, A. J., and Eissenstat, D. M. (2005). Rapid decline in nitrate uptake and respiration with age in fine lateral roots of grape: implications for root efficiency and competitive effectiveness. New Phytol. 165, 493–502. doi: 10.1111/j.1469-8137.2004.01222.x
Wang, C., Zhang, W., Li, Z., Li, Z., Bi, Y., Crawford, N. M., et al. (2018). FIP1 plays an important role in nitrate signaling and regulates CIPK8 and CIPK23 expression in Arabidopsis. Front. Plant Sci. 9:593. doi: 10.3389/fpls.2018.00593
Wang, L., and Ruan, Y.-L. (2016). Shoot–root carbon allocation, sugar signalling and their coupling with nitrogen uptake and assimilation. Funct. Plant Biol. 43, 105–113. doi: 10.1071/FP15249
Wang, Y.-Y., Cheng, Y.-H., Chen, K.-E., and Tsay, Y.-F. (2018). Nitrate transport, signaling, and use efficiency. Annu. Rev. Plant Biol. 69, 85–122. doi: 10.1146/annurev-arplant-042817-040056
Xi, Y., Liu, J., Dong, C., and Cheng, Z.-M. (2017). The CBL and CIPK Gene family in grapevine (Vitis vinifera): genome-wide analysis and expression profiles in response to various abiotic stresses. Front. Plant Sci. 8:978. doi: 10.3389/fpls.2017.00978
Yang, T., Zhu, L., Wang, S., Gu, W., Huang, D., Xu, W., et al. (2007). Nitrate uptake kinetics of grapevine under root restriction. Sci. Hortic. 111, 358–364. doi: 10.1016/j.scienta.2006.11.005
Yong, Z., Kotur, Z., and Glass, A. D. M. (2010). Characterization of an intact two-component high-affinity nitrate transporter from Arabidopsis roots: characterization of an Arabidopsis nitrate transporter. Plant J. 63, 739–748. doi: 10.1111/j.1365-313X.2010.04278.x
Zerihun, A., and Treeby, M. T. (2002). Biomass distribution and nitrate assimilation in response to N supply for Vitis vinifera L. cv. Cabernet Sauvignon on five Vitis rootstock genotypes. Aust. J. Grape Wine Res. 8, 157–162. doi: 10.1111/j.1755-0238.2002.tb00251.x
Zhang, L., Marguerit, E., Rossdeutsch, L., Ollat, N., and Gambetta, G. A. (2016). The influence of grapevine rootstocks on scion growth and drought resistance. Theor. Exp. Plant Physiol. 28, 143–157. doi: 10.1007/s40626-016-0070-x
Keywords: grapevine vigor, rootstock, nitrate uptake, N transport, carbohydrate status, transpiration
Citation: Rossdeutsch L, Schreiner RP, Skinkis PA and Deluc L (2021) Nitrate Uptake and Transport Properties of Two Grapevine Rootstocks With Varying Vigor. Front. Plant Sci. 11:608813. doi: 10.3389/fpls.2020.608813
Received: 21 September 2020; Accepted: 22 December 2020;
Published: 18 January 2021.
Edited by:
Nathalie Ollat, University of Bordeaux, FranceCopyright © 2021 Rossdeutsch, Schreiner, Skinkis and Deluc. This is an open-access article distributed under the terms of the Creative Commons Attribution License (CC BY). The use, distribution or reproduction in other forums is permitted, provided the original author(s) and the copyright owner(s) are credited and that the original publication in this journal is cited, in accordance with accepted academic practice. No use, distribution or reproduction is permitted which does not comply with these terms.
*Correspondence: Laurent Deluc, bGF1cmVudC5kZWx1Y0BvcmVnb25zdGF0ZS5lZHU=