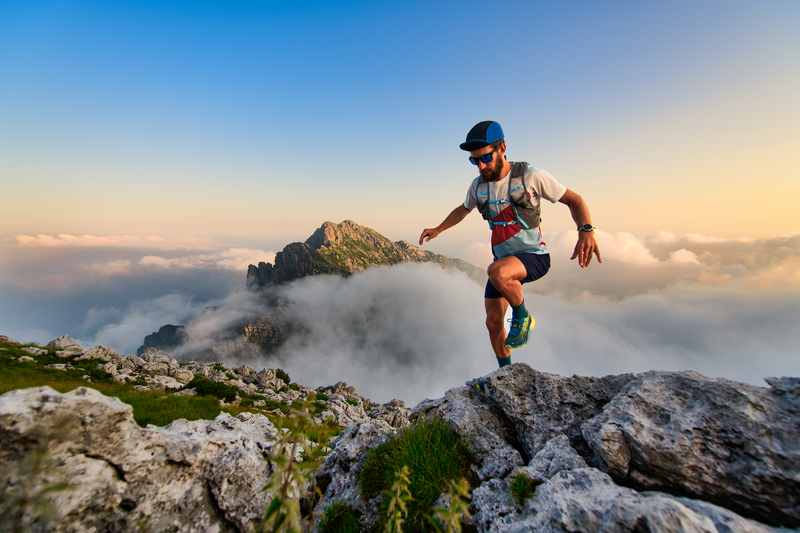
94% of researchers rate our articles as excellent or good
Learn more about the work of our research integrity team to safeguard the quality of each article we publish.
Find out more
REVIEW article
Front. Plant Sci. , 08 January 2021
Sec. Plant Physiology
Volume 11 - 2020 | https://doi.org/10.3389/fpls.2020.606918
Sink organs, the net receivers of resources from source tissues, provide food and energy for humans. Crops yield and quality are improved by increased sink strength and source activity, which are affected by many factors, including sugars and hormones. With the growing global population, it is necessary to increase photosynthesis into crop biomass and yield on a per plant basis by enhancing sink strength. Sugar translocation and accumulation are the major determinants of sink strength, so understanding molecular mechanisms and sugar allocation regulation are conducive to develop biotechnology to enhance sink strength. Grapevine (Vitis vinifera L.) is an excellent model to study the sink strength mechanism and regulation for perennial fruit crops, which export sucrose from leaves and accumulates high concentrations of hexoses in the vacuoles of fruit mesocarp cells. Here recent advances of this topic in grape are updated and discussed, including the molecular biology of sink strength, including sugar transportation and accumulation, the genes involved in sugar mobilization and their regulation of sugar and other regulators, and the effects of hormones on sink size and sink activity. Finally, a molecular basis model of the regulation of sugar accumulation in the grape is proposed.
With an increasing global population estimated to reach 9 billion people by 2050 (Nelson et al., 2012), a dramatic increase in agricultural productivity must occur. However, this increase has to be achieved without additional land for production and within the context of uncertain climatic shifts (Beddington, 2010). Thus, it is imperative to produce higher biomass and yield on a per plant basis to sustain the growing human population. In plants, the “source” refers to an organ with a net export of assimilates required for plant growth, such as carbon; whereas the “sink” is an organ that consumes or accumulates resources from the source (Doehlert, 1993). Photoassimilates are usually transported from sources to sinks as simple sugars, typically as sucrose (White et al., 2016). Sink strength determines photoassimilates importation and accumulation, thus controlling crop yield and affecting fruit quality. Clearly the phloem loading of sucrose at the source organ, transporting from source to sink, and unloading and metabolism in the sink organ are major processes affecting sink strength (Zhang et al., 2005). A complex signaling network encompassing sugars, hormones, and environmental factors determine sink strength by regulating sugar mobilization (Yu et al., 2015). Therefore, understanding sucrose delivery and accumulation regulation is vital to enabling new technological breakthroughs to improve crop yield and food quality.
Grape (Vitis vinifera L.) is a widely grown economic fruit species consumed as fresh fruit, dried raisins, and wine (Bondada et al., 2017; Imran et al., 2017; Khalil-Ur-Rehman et al., 2017; Wang W. et al., 2017). As in many crops, soluble sugar content is a primary component of yield and market value for grapes. Sucrose translocated from grape leaves is unloaded and metabolized in fruit (sink) and eventually accumulates as hexose (Lecourieux et al., 2014). Hexose accumulation not only provides carbon and energy for berry growth, but it also contributes to the sweetness and flavor of grapes. This review focuses on the mechanism related to the formation and regulation of sink strength in grape, including the molecular mechanism of sugar transport and accumulation, the genes involved in sugar allocation and its regulation, the genetic regulation of hormones and their role in regulating sugar transport, phloem unloading, and metabolism. This review also provides a premise for further research and development of new technologies to regulate photosynthetic assimilate partitioning in perennial fruit crops.
Sink strength has been defined as an organ’s competitive ability to import photoassimilates, which can be expressed as the product of sink size and sink activity (Ho, 1988). Sink size is the total biomass of the sink tissue (g), reflecting the cell number and size. Genetic factors and plant growth regulators regulate them during cell division (Bünger-Kibler and Bangerth, 1982; Geiger and Shieh, 1993). Sink activity refers to a particular resource’s specific uptake rate (mol g–1 s–1) (Geiger and Shieh, 1993). Three crucial physiological processes govern sink activity: phloem unloading and post-phloem transport of sugars in the sink cells; the absorption of the sink organ itself; and carbohydrate accumulation in sink organs (Roitsch, 1999). Also, external factors can modulate sink strength by increasing source strength (generally increasing source strength by influencing photosynthetic rate or increasing source capacity), regulating the loading and unloading of the assimilate in the phloem, and participating in the formation of sink size (cell number and size) and sink activity (sugar transport, phloem unloading, and accumulation in the sink organs). Studies on grapes have shown that the vital step controlling sugar accumulation is determined within the developing fruit rather than the source leaves’ ability to export photosynthetic products or the transport efficiency of the phloem pathway (Zhang et al., 2006; Xie et al., 2009). This key step occurs in the process of sugar entering berry cells after the sugar is unloaded from fruit phloem, which is called “post-sieve molecular transport” or “post-phloem transport” (Fisher and Oparka, 1996). It is the critical step that restricts the improvement of berry quality. Therefore, the external factors involved in regulating the sink activity, including sugar phloem unloading and sugar accumulation in the sink cells, could strongly affect sink strength in the grape.
Assimilate input and sucrose metabolism in sink organs directly affect the development and vitality of sink organs and determines sink strength (Chourey et al., 2012). Clarifying the molecular biology of sugar transport and accumulation is essential for the identification of the key enzyme/genes that control the distribution of assimilates from “source” to “sink” in perennials fruit crops.
The grape berry exhibits a double sigmoidal growth pattern (Coombe, 1992). Its development can be divided into stage I and stage III, characterized by rapid growth, whereas stage II is a lag period. The first rapid growth phase that occurs after the fruit set is due to increased cell number and expansion of existing cells. Cell division in the flesh is almost complete during the first few weeks of development (Harris et al., 1968). Stage II, 7 to 10 weeks after flowering, is when little or no growth occurs. The second rapid growth phase occurs at the end of the lag phase. The transition from stage II to stage III is completed within 24 h, and is known as véraison, associated with a change in skin color, and marks the beginning of maturity (Coombe, 1992). Before véraison, the fruit is hard, green, acidic, and lacks sugar (<150 mM sugar content) and glucose to fructose ratio is about 2:1. During véraison, organic acid content begins to decrease, and soluble sugar concentration increases. About 20 days after the onset of véraison, the hexose concentration increases to around 1 M and the ratio of glucose to fructose is 1:1 (Findlay et al., 1987). Sucrose is exported from the source organ (leaf) and imported into berry flesh cells via two pathways: the symplastic pathway and the apoplastic pathway. Firstly, the sucrose, produced in the photosynthetic mesophyll cells (MC) of leaves, is loaded into the phloem sieve tubes through a symplastic-loading pathway or apoplastic-loading pathway. In the symplastic-loading pathway, sucrose is loaded into the sieve tubes through plasmodesmata between the sieve elements (SE)/companion cell (CC) complex and the surrounding parenchymal cells without specific carries (Turgeon and Wolf, 2009). In the apoplastic-loading pathway, sucrose is loaded and accumulated in the phloem by passing through the apoplast between the phloem parenchyma cells (PP) and the CC with specific transporters, which can be of the PLANT SUGAR WILL EVENTUALLY BE EXPORTED TRANSPORTER (SWEETs) family (Chen et al., 2012) and SUCROSE TRANSPORTERS (SUT1/SUC2) proteins coupled with a proton pump (H + − ATPase) (Gottwald et al., 2000). Then sucrose is transported in the sieve tubes via hydrostatic pressure. Following long-distance delivery, sucrose is unloaded from the phloem SE to the storage parenchyma cells through the symplastic-unloading pathway or the apoplastic-unloading pathway. In the latter case, sucrose is taken up into the parenchyma apoplasm by the members of the SUT1/SUC2 transporter family and is then hydrolyzed by cell-wall invertase (CWINV) or sucrose synthase into hexoses (Chen et al., 2017; Wan et al., 2018; Duan et al., 2020). Hexoses enter the parenchyma cells via specific monosaccharide transporters at the plasma membrane or the tonoplast level (Corelli Grappadelli et al., 2019). Alternatively, sucrose is imported into the vacuoles, where it is converted into hexoses by vacuolar invertase (VIN). These processes maintain a sucrose concentration gradient at the unloading site to maintain a high rate of unloading and hexoses accumulation (Lecourieux et al., 2014). Studies with grape have shown that the phloem unloading routes are changeable with grape sink development. A shift of phloem unloading from symplastic to apoplastic pathways was verified at or just before the onset of véraison, indicating the phloem unloading pathway changes in response to grape sink development (Zhang et al., 2006). It appears that sugar transporters and sugar metabolism-related enzymes are linked to a complex regulatory network that determines the fruit’s sugar accumulation.
Four sucrose transporters (SUT/SUC) were identified in the grape genome (Table 1), three of them were cloned from Shiraz and Cabernet Sauvignon berries (VvSUC11; AF021808, also identified as VvSUT1 AF182445; VvSUC12 AF021809; and VvSUC27 AF021810) and the sucrose transport activity was characterized by heterologous expression in Saccharomyces cerevisiae (Afoufa-Bastien et al., 2010). VvSUC11 belongs to the SUT4 subfamily, including AtSUC4. VvSUC12 contains two structural characteristics specific to the SUT2/SUC3 subfamily, which includes AtSUC3. VvSUC27 is a SUT located at the plasma membrane and belongs to the dicot-specific SUT1 subfamily, which includes the remaining AtSUCs (Cai et al., 2017). Overexpression of VvSUCs (VvSUC11 or VvSUC12, or VvSUC27) in both tobacco and Arabidopsis conferred more rapid development, higher yield and enhanced abiotic stress resistance (Cai et al., 2017, 2020). Similarly, Cai et al. (2019) reported SUTs in ‘Zuoshan-1’ (Vitis vinifera) responded to various stress stimuli and subsequently promoted sucrose metabolism and hormone synthesis.
Monosaccharide transporter genes are present in 59 loci in grapevine, which can be grouped into seven subfamilies, including 20 hexose transporters (VvHT; subfamily I), three tonoplast monosaccharide transporters (VvTMT; subfamily II), five polyol/monosaccharide transporters (VvPMT; subfamily III), ERD6-like Transporters (subfamily IV), two vacuolar glucose transporters (VvVGT; subfamily V), 3 inositol transporters (VvINT; subfamily VI) and other four monosaccharide transporters (VvpGlcT/VvSGB1; subfamily VII) (Table 1; Afoufa-Bastien et al., 2010). Seven full-length cDNAs encoding monosaccharide transporter, named VvHT1–VvHT6/VvTMT2, VvTMT1 (VvHT1 AJ001061; VvHT2 AY663846; VvHT3 AY538259 and AY854146; VvHT4 AY538260; VvHT5 AY538261; VvHT6 AY861386, DQ017393) have been isolated from various cultivars such as Pinot noir, Ugni blanc, Chardonnay, Cabernet Sauvignon, Syrah and Riesling (Fillion et al., 1999; Vignault et al., 2005; Hayes et al., 2007; Zeng et al., 2011). Three of them (VvHT1, VvHT4, and VvHT5) belong to plasma membrane hexose transporters. Their plasma membrane localization has been verified by immunofluorescence, immunolabeling, and GFP fusion proteins. They all facilitated glucose uptake, VvHT1 has a higher affinity for glucose than VvHT4 and VvHT5 and displays broad substrate specificity, being able to recognize both d-glucose and d-fructose. On the contrary, VvHT3 is not able to transport any of the tested radiolabeled sugars in the deficient yeast model (Vignault et al., 2005; Conde et al., 2006; Hayes et al., 2007). VvHT2 and VvHT6/VvTMT2 seem to be localized in the tonoplast and VvHT6/VvTMT2 has high sequence similarity to the previously described tonoplast monosaccharide transporter of Arabidopsis thaliana AtTMT2 (Agasse et al., 2009; Afoufa-Bastien et al., 2010). Induction of VvTMT1-GFP fusion protein expression in transgenic yeast revealed its tonoplast localization. Glucose/other monosaccharide -uptake activities of VvTMT1 have been detected by heterologous expression in the hxt-null mutant yeast (Zeng et al., 2011). Recent function analysis indicated PbTMT4 regulates the accumulation of sugars in the vacuole and it is a strong contributor to fructose, glucose, and sucrose accumulation in frutescence of pears (Pyrus bretschneideri) (Cheng et al., 2018). Transient silencing of one Prunus persica tonoplast sugar transporter (PpTST1) significantly inhibited sugar accumulation in peach fruit (Peng et al., 2020). For ripe grapes, a very high concentration of glucose and fructose (∼1 M each) accumulates in the vacuole of flesh cells (Fontes et al., 2011). Clearly, monosaccharide transporters play important roles in vacuolar accumulation of hexose in grape berry. To date, the function of these transporters has not been studied in detail, though different locations and affinity for monosaccharide of these monosaccharide transporters showed diversified functions in sugar transport process.
SWEETs is a family of a plasma membrane-localized sugar uniporters that have been found in recent years. Arabidopsis thaliana AtSWEET1 was the first identified plant SWEET transporter and responsible for glucose unidirectional transport in different systems (Chen et al., 2010). AtSWEET11, AtSWEET12, and AtSWEET17 were subsequently characterized to export sucrose out of the phloem parenchyma cells or fructose efflux from vacuole (Chen et al., 2012; Chardon et al., 2013). Seventeen SWEET homologes were identified in the grape genome (Table 1), VvSWEET4 is characterized as a glucose transporter localized in the plasma membrane (Chong et al., 2014). Overexpression of the VvSWEET4 in grapevine hairy roots increases sugar transportation and content and enhances resistance to soilborne pathogens (Meteier et al., 2019). Similarly, VvSWEET7 is a mono- and disaccharide transporter and its expression is up-regulated in response to Botrytis cinerea infection in grapes (Breia et al., 2019). VvSWEET10 has a broad spectrum of sugar transport functions. Overexpression of VvSWEET10 in grapevine calli and tomatoes increased the glucose, fructose, and total sugar levels significantly (Zhang et al., 2019). VvSWEET15 was highly expressed in three grape cultivars’ berries and obviously positively correlated with berry hexose content during ripening (Ren et al., 2020). In summary, these findings demonstrated the role of VvSWEETs in sugar accumulation and biotic stress response.
After importation into sink parenchyma cells, sucrose is rapidly taken into the vacuole for storage or degraded into hexoses for a wide range of metabolism in order to maintain the gradients in the sink cells, thus maintaining phloem unloading (Lecourieux et al., 2014). Sucrose-cleaving enzymes such as sucrose synthase (SuSy) and invertase are mainly responsible for sucrose metabolism in sink cells (Chen et al., 2017; Wan et al., 2018). Stein and Granot (2019) reviewed SuSy is the only Suc-metabolizing enzyme that can catalyze both the synthesis of sucrose from fructose and uridine diphosphate glucose (UDP-G) and the cleavage of sucrose, in the presence of UDP or other nucleotide phosphates (especially ADP), to fructose and UDP-G or adenosine diphosphate glucose (ADP-G). Subcellular localization of SuSy is detected both in cytosolic fractions or/and plasma-membrane in several plants. SuSy contributes to sucrose hydrolysis mainly in the cytosol and it responds to sink strength in different plants (Braun et al., 2014). Five SuSy genes (VvSS1-5) have been identified in the grape genome (Zhu et al., 2017). VvSS3 was reported to co-express with VvSWEET15 to regulate sucrose hydrolysis and transport, leading to increase hexose accumulation in grapes (Ren et al., 2020). Invertase hydrolyzes sucrose irreversibly into glucose and fructose and has a crucial function in establishing and maintaining sink metabolism, and hence considered to be a central molecular sink strength determinant (Koch, 2004). Three types of invertase isoenzymes are distinguished based on their solubility, subcellular localization, pH optima, and isoelectric point: (1) Soluble acid invertase (VIN) is located primarily in vacuoles; (2) Cell wall-bound invertase (CWINV) binds to cell walls; (3) Soluble alkaline or Neutral invertase (A/N-INV) is present in the cytoplasm and has a low activity in plant tissues (Sturm, 1996). Invertases are also divided into Acid Invertase (AI) and Neutral Invertase (NI) according to the optimal pH required by the reaction (Avigad, 1982). To our knowledge, nine neutral/alkaline invertase (NI) genes have been identified in the 8.4X grape genome (Nonis et al., 2008). But genes encoding AI have not been identified so far. We conducted a BLASTP search against the 12X grape genome database (Grapevine Genome Browser. Available online1) using known protein sequences of invertase genes from Arabidopsis as queries (tair datebase. Available online2). After manually removing redundant sequences and verifying existence of the core domains with the Conserved Domains Database (CDD. Available online3), 19 putative invertase genes were identified in the grape genome (Supplementary File 1). The phylogenetic analysis revealed that 11 were grouped into the neutral/alkaline invertase sub-family, and eight were from the acid invertase sub-family, of which there were three vacuolar acidic invertases (VIN) and five cell wall acidic invertases (CWINV) (Figure 1A). Multiple alignments of acid invertase sub-family (Figure 1B) revealed that the only one CWINV (GSVIVT01033873001) and one VIN (GSVIVT01006154001) contain the DPNGD domains, which were well-conserved in this family and are essential for β-fructosidase catalytic activity (Sturm and Chrispeels, 1990). The other six genes contain the variable NDPNG motif. The conservative Cys catalytic site (MWEC-P/V-D) (Chen et al., 2015) was found at the C-terminal of all acid invertase members, the exception being one VIN gene (GSVIVT01024570001) with ‘MWECAN’ motif, a proline (P) residue was present in MWEC-P/V-D motif of CWINV sequences, whereas a valine (V) was at the same position of VIN sequences. This finding is consistent with the previous study (Goetz and Roitsch, 1999). The variation of NDPNG motif among acid invertase genes may indicate their specific function in grape. Several AI genes have been functionally validated in sink strength through their involvement in sucrose metabolism in some species (Liu et al., 2016; Xu et al., 2019; Deng X. et al., 2020). In grape, an increase in the expression and activity of CWINV was concomitant with a rise in apoplastic sugar concentration and osmotic pressure (Zhang et al., 2006; Xie et al., 2009). While some studies reported invertase and hexose transporters are unnecessary for sugar differential accumulation among cultivars (Ren et al., 2020), others found that it was mainly due to acid invertase activity (Guan, 2011). However, no functional identification is focused on the member of VvSSs or invertase family in grape so far. Therefore, a transgenic functional characterization experiment would help explore the specific role of each VvSSs or invertase genes in sugar accumulation in grape berry.
Figure 1. (A) Phylogenetic tree of invertase genes from different plants. Amino acid sequences from Arabidopsis (tair database), populus (Chen et al., 2015) and grapevine were used to construct the unrooted phylogenetic tree by MEGA v. 6.0 (Tamura et al., 2013) with the neighbor-joining (N-J) method, p-distance, pairwise deletion method, and a bootstrap test with 1,000 replicates. The red and green circles indicate vacuolar acidic invertases and cell wall acidic invertases of Arabidposis and populus, respectively. Blue and purple triangles indicate alkaline/neutral invertase of Arabidposis and populus, respectively. (B) Multiple alignment of the acid invertase sub-family in grape with two known populus vacuolar acidic invertase (PtrVINV1) and cell wall acidic invertase (PtrCWINV3) as references. The red box shows β-fructosidase motif (DPNGP), the yellow box shows the cys catalytic site (MWEC-P/V-D).
The current investigations opine that the plant sugar transporters and sugar-cleaving enzymes under the control of their substrate either at the transcriptional or post-transcriptional levels. Exogenous sucrose feeding controls the transcription level of SUC and SUC protein amount in sugar beet (Vaughn et al., 2002). Glucose could regulate the expression of monosaccharide transporters (VvHT3, VvHT4, VvHT5, and VvHT6) by inducing VvSK1 transcription, a Glycogen Synthase Kinase3 protein kinase that modulates sugar uptake and accumulation in grape (Lecourieux et al., 2010). Several studies reported that extracellular invertases are induced by sugars (Roitsch et al., 1995; Tymowska-Lalanne and Kreis, 1998). The expression of CWINV and SuSy was repressed by glucose, and the intensity of repression depended on glucose concentration and incubation time. Hexokinase (HXK, EC 2.7.1.1) involved in hexose phosphorylation, plays a crucial role in sugar sensing and signaling. It has been found HXK activity had an inverse relationship with the endogenous glucose or fructose levels during grape development. The phosphorylation of hexoses by HXK was an essential component in the glucose-dependent VvCWINV and VvSuSy expression (Wang et al., 2014). Additionally, glucose could repress VvHT1 transcription via an HXK-dependent pathway. While inducing the reduction of VvHT1 protein in the plasma membrane by a HXK-independent post-transcriptional regulation (Conde et al., 2006). Thus, it is clear that sugar could play a regulatory role in sink activity by regulating its transport carrier or metabolic enzymes.
Except for sugar’s regulatory role, little is known about protein/regulators that control sugar transporters and metabolic enzymes in the grapevine. In Arabidopsis, identification of SUC2-interaction partners indicated that SUC2 activity is controlled by its protein turnover rate and phosphorylation state. UBIQUITIN-CONJUGATING ENZYME 34 (UBC34) is responsible for triggering the turnover of SUC2 in a light-dependent manner. WALL-ASSOCIATED KINASE LIKE 8 (WAKL8) functions on the phosphorylation of SUC2 for increasing transport activity (Xu et al., 2020). In cotton (Gossypium hirsutum), CBL-interacting protein kinase GhCIPK6 is recruited to the tonoplast by Calcineurin B-like protein GhCBL2 and interacts with the tonoplast-localized sugar transporter GhTST2 to regulates plant sugar homeostasis, in particular glucose homeostasis (Deng J. et al., 2020). HpWRKY3, a WRKY transcription factor in pitaya (Hylocereus), was identified as the putative binding protein of the HpINV2 and HpSuSy1 promoters and could activate the expression of HpINV2 and HpSuSy1. It is proposed that HpWRKY3 is involved in sugar accumulation by inducing the sucrose metabolic genes in pitaya fruit (Wei et al., 2019). Similarly, Li et al. (2020) found that PuWRKY31 bound to the PuSWEET15 promoter and induced its transcription to promote sugar accumulation in Ussurian pear (Pyrus ussuriensis).
Grape production benefits from plant growth regulators as long as they are applied at the recommended dose and frequency, and pre-harvest intervals are strictly followed (Ugare et al., 2013). For instance, CPPU [forchlorfenuron,N-(2-Chloro-4pyridinyl-N-phenylurea)], a synthetic cytokinin-like plant regulator, promotes berry set and increases berry size (Reynolds et al., 1992). Application of gibberellic acid (GA) is a routine practice to increase berry size or induce seedlessness in table grapes (Weaver and MacCune, 1961); Other plant hormones such as Abscisic acid (ABA), auxin, and brassinosteroids (BR) also play important roles in the formation and maintenance of fruit sink strength.
A study that tested nine plant hormones found that only 6-benzylaminopurine (6-BA, artificial agent for cytokinin) and ABA stimulated unloading photosynthetic products in bean seeds, which occurred within 10–12 min after 6-BA or ABA treatment (Clifford et al., 1986). Several studies supported that cytokinin may regulate rate-limiting steps in nutrient utilization and distribution. For instance, radioactive nutrients were preferentially transferred and accumulated in cytokinin-treated areas (Mothes and Engelbrecht, 1962). Localized overexpression of the ISOPENTENYLTRANSFERASE (IPT, the enzyme that mediates the rate-limiting step in cytokinin synthesis) caused local sink enhancement (Guivarc’h et al., 2002). The formation of green areas on senescing leaves suggested an alteration of the sink-source relation by cytokinin (Engelbrecht et al., 1969; Body et al., 2013). A later study found photosynthetic capacity and soluble sugar content were not significantly affected in the source organs (leaves) of a cytokinin-deletion mutant, however, soluble sugar content, invertase activity and ATP content in sink organs (shoot tip) were obviously reduced (Werner et al., 2008). Recent research reported phytochromobilin deficiency impairs sugar metabolism through the regulation of cytokinin and auxin signaling in tomato fruit (Bianchetti et al., 2017). Additionally, light can weaken the sink activity and inhibit axillary bud germination by downregulating cytokinin signals in rose. Under dark conditions, 6-BA treatment rapidly activated the key components of sink strength, including vacuolar acid invertase, sucrose transporter and sucrose synthase, and promoted axillary bud growth (Roman et al., 2016). In summary, these findings support the role of cytokinin in regulating sink strength, and suggest that localized cytokinin production could create a localized sink and result in a new source-sink relationship, thereby modulating nutrient mobilization.
Several studies have related the availability of cytokinin to plant sink strength under abiotic stress in recent years. For instance, the exogenous application of cytokinin (KIN) to developing tomato fruit or the increase in endogenous cytokinins (tZ) under salinity partially restored sink activity and fruit growth, and also activated most sugar metabolic enzymes (Albacete et al., 2014). Delay of senescence in IPT transgenic tobacco (Nicotiana tabacum) plants correlated with elevated CWINV activity. Localized induction of a CWINV under control of a chemically inducible promoter resulted in an ectopic delay of senescence, resembling the naturally occurring green areas on senescing leaves, these results established a causal relationship between cytokinin and extracellular invertase for delaying senescence (Balibrea Lara et al., 2004). P(SARK): IPT transgenic rice plants, expressing IPT driven by P(SARK), a maturation- and stress-induced promoter, had enhanced drought tolerance and higher grain yield with improved quality (nutrients and starch content). This phenomenon indicated that stress-induced cytokinin synthesis in the P(SARK):IPT plants modified source/sink relationships and promoted sink strength through a cytokinin-dependent coordinated regulation of carbon and nitrogen metabolism that allowed plant adaptation and survival under water stress (Reguera et al., 2013). Results in many crops that overexpress IPT under the control of P(SARK) have demonstrated a delay in leaf senescence and improved crop growth and yield under different stress conditions (Décima Oneto et al., 2016; Joshi et al., 2019). Therefore, it appears that cytokinin plays a role in making up the deficiencies caused by stress in plant. Cytokinin may function in two ways when plants are under abiotic stress. Cytokinin could delay the stress response or senescence of leaves (thus maintaining source activity), resulting in the production and/or export of more assimilates for sink organs’ growth. On the other hand, cytokinin could expand the sink capacity by increasing cell proliferation or maintaining the sink activity by regulating sucrolytic enzymes to acquire more photo-assimilates.
Forchlorfenuron,N-(2-Chloro-4pyridinyl-N-phenylurea) is widely used for fruit size enhancement in table grape production (Reynolds et al., 1992; Wang W. et al., 2017). Hence, it may be suggested that CPPU treatment is conducive to forming the sink capacity at early berry development. Indeed, endogenous cytokinin levels are critically involved in the regulation of early fruit growth through the regulation of cell division by D-type cyclin expression (Baldet et al., 2006). Similar results were also observed with barley (Hordeum vulgare L.), wherein the effects of foliar application of 6-BA resulted in increased sink size soon after anthesis and increased sink demand was met by current photosynthesis of organs (Hosseini et al., 2008). However, the application of CPPU had some adverse effects on grapes, including inhibiting anthocyanin and sugar accumulation (Khalil-Ur-Rehman et al., 2017). Moreover, the endogenous cytokinin zeatin levels are high early in the flesh of immature berries but decrease rapidly at the time of véraison when sugar and anthocyanin start to accumulate (Zhang et al., 2003). On the other hand, some studies found a rapid increase in isopentenyl adenine (ip) at véraison and remained at elevated levels throughout grape ripening (Böttcher et al., 2013, 2015). To date, studies have not given any clear indications for possible functions of endogenous cytokinin on the sugar accumulation in grapes. Therefore, why CPPU caused decreased sugar accumulation in grapes is still unknown. This adverse effect may be related to two main reasons. One is the ability of cytokinin to establish local metabolic sink capacity. Under normal growth conditions, exogenous cytokinin application could reconstruct other competitive sink organs and break the original balance between the source (leaf) and sink (berry), resulting in reduced accumulation of sugar in fruit. The second reason is that cytokinin prefers to enhance sink size rather than to sink activity. Application of CPPU could trigger fruit growth and delay fruit maturity, therefore not conducive to initiating fruit ripening and sugar accumulation. Future studies should focus on fine-tuning the regulation of cytokinin concentrations and the tissue-specific responses during grape development.
A two-step GA application has usually been employed before anthesis and again after anthesis; the former for inducing seedlessness and the later for berry enlargement (Dass and Randhawa, 1968; Wang X. et al., 2017). GA3 is thought to increase fruit size by triggering cell division and expansion in many fruit crops (Zhang and Whiting, 2011). Recently, transcriptome analysis revealed that cell-wall relaxation could be the main process in exogenous GA3-triggered berry enlargement at the early stage of grape fruit development (Chai et al., 2014). GA3 application at the 3–4 mm berry stage increased grape size, and the top five genes upregulated by GA3 are related to cell wall formation in berries (Upadhyay et al., 2018). Similarly, proteome analysis of the berry-sizing effect of GA3 on seedless grape shows cytoskeleton and cell-wall modification proteins are up-regulated in the stages II and III of berry development (Wang et al., 2012). Hence, post-bloom GA3 application induced berry expansion through modifying cell wall components. Recent studies reported GA could regulate genes of the lignin biosynthesis (Garcia-Rojas et al., 2018), cell wall metabolism, xylem development, phenylpropanoids, and the cell cycle (Meneses et al., 2020), generating changes in cell wall composition that caused an increase in berry drop. These studies provide some clues to explore further the mechanism underlying the effects of GA on the fruit cell cycle and expansion affected by GA.
Sucrose, glucose, and fructose in grapes’ suspension cell cultures significantly increased after GA3 treatment (Zhang et al., 2014). These findings, coupled with previous studies, corroborate that GA3 can hasten the accumulation of hexoses within the expanding cells (Kordel and Kutschera, 2000). GA3 could relieve the repression of glucose on CWINV and SuSy1 expression by regulating the HXK gene expression, thereby further regulating intracellular glucose metabolism to maintain normal cell growth in grape (Zhang et al., 2014). Similarly, a significant increase in sink demand and larger fruit size caused by applied GA was shown in Japanese pear. This enhanced sink strength was closely correlated with increased activities of sugar metabolizing enzymes induced by GA application during rapid fruit growth (Zhang et al., 2007). The expression of the Rosa hybrida vacuolar invertase 1 gene (RhVI1) was controlled by sugar/light and gibberellin/light synergistically. Further study demonstrated that the 127 bp RhVI1 promoter fragment located between −595 and −468 bp was critical for this synergism (Rabot et al., 2014). Therefore, GA3 functions as a regulator of sugar accumulation in the plant; however, the precise mechanisms involved remain elusive in many plants, including grape.
Extensive studies showed that ABA enhanced sugar accumulation in crop sink organs (Kobashi et al., 2001; Kuhn et al., 2013). In Malbec grapevines treated with ABA, increased accumulation of glucose and fructose in berries was correlated with enhanced VvHT2 and VvHT6 gene expression and increased phloem area and sucrose content in leaves (Murcia et al., 2016). When Hayes et al. (2010) studied the pathogen-induced regulators of carbohydrate sink strength, they found powdery and downy mildew infections induced VvHT5 activity in coordination with VvCWINV in grape leaves and this was controlled through ABA. These findings indicated ABA regulated VvCWINV and VvHT5 expression during the transition from source to sink in response to infection by biotrophic pathogens (Hayes et al., 2010). Some cross-mediation among ABA signaling and sugar metabolism and accumulation have been identified in different species. MdAREB2, an ABA-responsive transcription factor in apple (Malus domestica), directly activated the amylase and the sugar transporter gene MdSUT2 to promote soluble sugar accumulation (Ma et al., 2017). The grape ASR (ABA, stress, ripening) protein VvMSA, a protein induced by sucrose, stress and ABA during fruit development, combines to a 160 bp interval of VvHT1 promoter to regulate sugar movement and accumulation via regulating the expression of VvHT1 (Carrari et al., 2004). A VvMSA transcriptional regulation model at the convergence of ABA, glucose, hexokinase1, and SnRK1 was proposed. In short, VvMSA expression is inhibited by hexokinase1 and stimulated by ABA at high glucose levels, whereas the inhibition by hexokinase1 is released at low glucose levels in grape protoplasts (Saumonneau et al., 2012; Dominguez and Carrari, 2015). ABSCISIC ACID-INSENSITIVE4 (ABI4) is a member of the AP2/ERF family. Its expression is induced in the presence of low glucose concentrations (Cho et al., 2010) and high glucose concentrations and ABA (Arroyo et al., 2003; Zheng et al., 2019). An ATX5-HY1-ABI4 regulatory module governing the glucose response was identified recently. More specifically, trithorax-group Protein ARABIDOPSIS TRITHORAX5 (ATX5) directly regulates the transcription of HY1 by trimethylating H3 lysine 4 of the Arabidopsis Heme Oxygenase1 (HY1) locus. Glucose signaling suppresses ATX5 activity and subsequently reduces the H3K4me3 levels at the HY1 locus, thereby leading to the increased expression of ABI4 (Liu et al., 2018). ABA not only could activate both the VIN and CWINV during grape berry development through enhancing their activities and amounts (Pan et al., 2010), but also could block the inhibitory effect of glucose on the expression of SuSy and CWINV in grapes. This inhibition was linked with the glucose sensor HKX1 (Wang X. Q. et al., 2017). Therefore, HXK may be another potential cross-mediator between ABA and glucose signaling to control sugar accumulation.
Fruit growth usually begins with cell division, continues with cell division and expansion, and only ends with cell expansion. The SAUR19 subfamily, one of the auxin signaling components, has been shown to function as a positive effector of cell expansion in Arabidposis (Spartz et al., 2012). The cell elongation bHLH protein (VvCEB1) was identified and characterized to control cell expansion in grape. VvCEB1opt-overexpressing lines significantly elevated auxin content and increased the number of lateral leaf primordia within meristems relative to control, demonstrating that cell expansion and organ number proliferation were likely an auxin-mediated process (Lim et al., 2018). Overexpression of VvCEB1 in grape embryos resulted in either activation or repression of the VvIAA family members’ expression while inducing the expression of VvSAUR and VvGH3 genes. These disparities in the expression levels of auxin-regulated genes may reflect their complex regulation of cell expansion (Nicolas et al., 2013). Combined physiological, transcriptome and cis-regulatory element analyses in grapes suggest that fruit size is associated with changes in the berry’s ripening physiology, where large berries approach ripening faster. Compared with large berries, auxin levels are high in small berries, accompanied with upregulation of transcripts encoding TAR4 and YUC (Wong et al., 2016). It may be related to inhibition of cell expansion and fruit ripening by auxin. A recent study reported that auxin slowed down the onset of grape berry ripening by delaying cell expansion (Dal Santo et al., 2020). Taken together, these data provide insight into the link between auxin signaling and cell expansion in grapes, which may affect the sink capacity and sink demand in subsequent berry development.
Additionally, Böttcher et al. (2012) reported that the initiation of sugar accumulation was delayed. The sugar accumulation rate was lower in NAA-treated grape berries, resulting in a 15-day delay in harvest. Antisense suppression of Sl4RF4, an auxin response factor (ARF) gene in tomato (Solanum lycopersicum), resulted in higher starch content in developing fruits correlating with the up-regulation of genes and enzyme activities involved in starch biosynthesis. This phenomenon suggested the involvement of ARFs in the control of sugar content (Sagar et al., 2013). Altogether, the findings indicated that auxin delayed the accumulation of sugar content during fruit development.
Application of exogenous BR (24-epibrassinolide; EBR) increased soluble sugar content in Cabernet Sauvignon berries accompanied by increased activities of invertases and sucrose synthase. These increases coincided with the upregulation of transcription levels of VvCWIN, VvHT3, 4, 5, 6, and VvSUC12, 27 during véraison (Xu et al., 2015). Vergara et al. (2018) also found that BR analogs increased soluble solids and anthocyanins in ‘Redglobe’ grapes. Together, these data suggested that BR positively controls sugar accumulation in grapes during véraison. In the review of Kuhn (2016), it was concluded that sugar transporter SUT2 and BR signaling cross-talk regulated plant immunity. Recent studies found that the phenotypic effects of SlSUT2 silencing in tomato could partially be rescued by EBR treatment, demonstrating that SlSUT2 interconnects sucrose partitioning with brassinosteroid signaling (Hansch et al., 2020). These studies could provide guidance for further research into the involvement of BR in the regulation of sugar transport and accumulation in grapes.
To summarize, the regulation of hormones on sink strength reported in grape berry was constructed to a regulatory network in the present review (Figure 2). Cytokinin could facilitate sink size formation by activating D-type cyclin gene expression to promote cell division. However, exogenous cytokinin (CPPU) is not conducive to sugar accumulation in grape berry. The level of endogenous ip increased rapidly at véraison when sugar and anthocyanin accumulate. It remained at elevated levels throughout grape ripening, whereas zeatin decreased rapidly, reaching low levels at around véraison. However, no studies confirm the repression of zeatin or the promotion of ip on sugar accumulation. Auxin was found to inhibit cell expansion in grape berry. However, the differential response of auxin-related genes to the cell elongation bHLH protein (VvCEB1) suggests their complex cell expansion regulation. Recent reports suggest auxin could restrain the sink activity via delaying the initiation of sugar accumulation. GA is widely used to expand berry size at an early stage of grape development; this expansion could be involved in the regulation of cell wall formation genes, cytoskeleton and cell-wall modification proteins, and cell-wall relaxation process by GA. GA is also reported to contribute to sugar accumulation by alleviating glucose inhibition on the expression of VvCWINV/VvSuSy. The same regulation was found in ABA; both alleviate glucose’s repression on the VvCWINV/VvSuSy expression by an HXK dependent pathway. Additionally, VvMSA could respond to ABA to directly promote hexose transporter genes and promote sugar accumulation. BR could upregulate expression of VvCWIN, VvHT3, 4, 5, 6, and VvSUC12, 27, and facilitate to sugar accumulation during the véraison stage of grape development. More work is needed to fill the gaps in this network.
Figure 2. The regulation model of hormones on sink strength reported in grape. Symbol ‘?’ means no clear relationship between hormones and sink activity (sugar accumulation), → stands for promotion, —∙ stands for inhibition. Solid line means direct regulation relationship, dotted line means indirect regulation relationship or no further study for direct regulation.
The present status of our knowledge on the regulation of sink strength in grapevine is summarized in Figure 3. The pattern of sugar accumulation during berry development is clearly obtained from previous studies. Grapevine genome sequencing has made a valuable contribution to identify additional genes encoding sugar transporters and metabolic enzymes. These genes are involved in the complex molecular biology of sugar transport and accumulation. However, most research showed only the average levels of the gene expression and enzyme activity for whole berries or grape heterotrophic suspension-cultured cells system. In contrast, sugar concentration is different in the flesh tissue (low hexose concentration near the brush, high at the stylar end). Active uptake of d-glucose in the flesh is only detected at a specific stage (véraison), while it can be detected at both pre- and post-véraison stage in isolated skin pieces (Coombe, 1992). So advanced studies are needed to determine the precise function of these genes in specific phloem locations of the grapevine so that the precise tracking of sugar allocation is revealed. Moreover, the proteins or transcription factors that modulate sugar transporters and metabolic enzymes could be further explored at the transcription or post-transcription levels. The potential regulation of the sugar transporters and metabolic enzymes would provide practical benefits, maximizing crop yield, and improving food quality.
Figure 3. Model of the molecular basis for the progress and regulation of sugar accumulation in grape berry. Symbol ‘?’ means no clear relationship between hormones and sink size (berry expansion) and sink activity (sugar accumulation), → in blue stands for promotion, —∙ in blue stands for inhibition.
Additionally, the role of various hormones in the regulation of sink size and activity is currently in progress in fruits (Figure 3). Although it is well established that ABA and BR promote sugar accumulation in grape, the mechanisms of these promotions remain to be elucidated. Cytokinin and GA are well known to be involved in berry expansion, promoting sink capacity, but no clear relationship has been established between sugar accumulation and endognous/exogenous cytokinin in grape. Several studies have contributed to the regulation of auxin regarding sink size and activity; however, the disparities in the expression levels of auxin-related genes reflect their complex regulation in cell expansion and sugar accumulation. To use hormones more effectively in grape production, we need a finely tuned regulation of hormone concentration and type that controls cell expansion/sugar allocation at given tissue locations during grape berry development.
Y-ML and Z-SX jointly wrote and revised the manuscript. CF and BB revised and rewrote sections of the manuscript. FL involved in revising the manuscript. All authors contributed to the article and approved the submitted version.
This work was supported by the grants 31872050 from the National Natural Science Foundation of China.
The authors declare that the research was conducted in the absence of any commercial or financial relationships that could be construed as a potential conflict of interest.
The Supplementary Material for this article can be found online at: https://www.frontiersin.org/articles/10.3389/fpls.2020.606918/full#supplementary-material
At, Arabidopsis thaliana; Vv, Vitis vinifera L; Ptr, Populus trichocarpa; SE, sieve elements; CC, companion cell; UDP-G, diphosphate glucose; ADP-G, adenosine diphosphate glucose; CPPU, forchlorfenuron, N-(2-Chloro-4pyridinyl-N-phenylurea); GA, Gibberellin acid; ip, isopentenyladenine; ABA, Abscisic acid; 6-BA, 6-benzylaminopurine; BR, brassinosteroids; EBR, 24-epibrassinolide.
Afoufa-Bastien, D., Medici, A., Jeauffre, J., Coutos-Thevenot, P., Lemoine, R., Atanassova, R., et al. (2010). The Vitis vinifera sugar transporter gene family: phylogenetic overview and macroarray expression profiling. BMC Plant Biol. 10:245. doi: 10.1186/1471-2229-10-245
Agasse, A., Vignault, C., Kappel, C., Conde, C., Gerós, H., and Delrot, S. (2009). “Sugar transport and sugar sensing in grape,” in Grapevine Molecular Physiology and Biotechnology, 2nd Edn, ed. K. A. Roubelakis-Angelakis (New York, NY: Springer), 105–139. doi: 10.1007/978-90-481-2305-6_5
Albacete, A. A., Martinez-Andujar, C., and Perez-Alfocea, F. (2014). Hormonal and metabolic regulation of source-sink relations under salinity and drought: from plant survival to crop yield stability. Biotechnol. Adv. 32, 12–30. doi: 10.1016/j.biotechadv.2013.10.005
Arroyo, A., Bossi, F., Finkelstein, R. R., and Leon, P. (2003). Three genes that affect sugar sensing (abscisic acid insensitive 4, abscisic acid insensitive 5, and constitutive triple response 1) are differentially regulated by glucose in Arabidopsis. Plant Physiol. 133, 231–242. doi: 10.1104/pp.103.021089
Avigad, G. (1982). “Sucrose and other disaccharides,” in Encyclopedia of Plant Physiology, New Series, eds F. A. Loewus and W. Tanner (New York, NY: Springer-Verlag), 216–347.
Baldet, P., Hernould, M., Laporte, F., Mounet, F., Just, D., Mouras, A., et al. (2006). The expression of cell proliferation-related genes in early developing flowers is affected by a fruit load reduction in tomato plants. J. Exp. Bot. 57, 961–970. doi: 10.1093/jxb/erj082
Balibrea Lara, M. E., Gonzalez Garcia, M. C., Fatima, T., Ehness, R., Lee, T. K., Proels, R., et al. (2004). Extracellular invertase is an essential component of cytokinin-mediated delay of senescence. Plant Cell 16, 1276–1287. doi: 10.1105/tpc.018929
Beddington, J. (2010). Food security: contributions from science to a new and greener revolution. Philos. Trans. R. Soc. Lond. B Biol. Sci. 365, 61–71. doi: 10.1098/rstb.2009.0201
Bianchetti, R. E., Cruz, A. B., Oliveira, B. S., Demarco, D., Purgatto, E., Peres, L. E. P., et al. (2017). Phytochromobilin deficiency impairs sugar metabolism through the regulation of cytokinin and auxin signaling in tomato fruits. Sci. Rep. 7:7822.
Body, M., Kaiser, W., Dubreuil, G., Casas, J., and Giron, D. (2013). Leaf-miners co-opt microorganisms to enhance their nutritional environment. J. Chem. Ecol. 39, 969–977. doi: 10.1007/s10886-013-0307-y
Bondada, B., Harbertson, E., Shrestha, P. M., and Keller, M. (2017). Temporal extension of ripening beyond its physiological limits imposes physical and osmotic challenges perturbing metabolism in grape (Vitis vinifera L.) berries. Sci. Hortic. 219, 135–143. doi: 10.1016/j.scienta.2017.03.002
Böttcher, C., Boss, P. K., and Davies, C. (2012). Delaying Riesling grape berry ripening with a synthetic auxin affects malic acid metabolism and sugar accumulation, and alters wine sensory characters. Funct. Plant Biol. 39, 745–753. doi: 10.1071/fp12132
Böttcher, C., Boss, P. K., and Davies, C. (2013). Increase in cytokinin levels during ripening in developing Vitis vinifera cv. Shiraz berries. Am. J. Enol. Vitic. 64, 527–531. doi: 10.5344/ajev.2013.13053
Böttcher, C., Burbidge, C. A., Boss, P. K., and Davies, C. (2015). Changes in transcription of cytokinin metabolism and signalling genes in grape (Vitis vinifera L.) berries are associated with the ripening-related increase in isopentenyladenine. BMC Plant Biol. 15:223. doi: 10.1186/s12870-015-0611-5
Braun, D. M., Wang, L., and Ruan, Y. L. (2014). Understanding and manipulating sucrose phloem loading, unloading, metabolism, and signalling to enhance crop yield and food security. J. Exp. Bot. 65, 1713–1735. doi: 10.1093/jxb/ert416
Breia, R., Conde, A., Pimentel, D., Conde, C., Fortes, A. M., Granell, A., et al. (2019). VvSWEET7 Is a Mono- and disaccharide transporter up-regulated in response to Botrytis cinerea infection in grape berries. Front. Plant Sci. 10:1753. doi: 10.3389/fpls.2019.01753
Bünger-Kibler, S., and Bangerth, F. (1982). Relationship between cell number, cell size and fruit size of seeded fruits of tomato (Lycopersicon esculentum Mill.), and those induced parthenocarpically by the application of plant growth regulators. Plant Growth Regul. 1, 143–154.
Cai, Y., Tu, W., Zu, Y., Jing, Y., Xu, Z., Lu, J., et al. (2017). Overexpression of a grapevine sucrose transporter (VvSUC27) in tobacco improves plant growth rate in the presence of sucrose in vitro. Front. Plant Sci. 8:1069. doi: 10.3389/fpls.2017.01069
Cai, Y., Yan, J., Li, Q., Deng, Z., Liu, S., Lu, J., et al. (2019). Sucrose transporters of resistant grapevine are involved in stress resistance. Plant Mol. Biol. 100, 111–132. doi: 10.1007/s11103-019-00847-5
Cai, Y., Yan, J., Tu, W., Deng, Z., Dong, W., Gao, H., et al. (2020). Expression of sucrose transporters from Vitis vinifera confer high yield and enhances drought resistance in Arabidopsis. Int. J. Mol. Sci. 21, 2624. doi: 10.3390/ijms21072624
Carrari, F., Fernie, A. R., and Iusem, N. D. (2004). Heard it through the grapevine? ABA and sugar cross-talk: the ASR story. Trends Plant Sci. 9, 57–59. doi: 10.1016/j.tplants.2003.12.004
Chai, L., Li, Y., Chen, S., Perl, A., Zhao, F., and Ma, H. (2014). RNA sequencing reveals high resolution expression change of major plant hormone pathway genes after young seedless grape berries treated with gibberellin. Plant Sci. 229, 215–224. doi: 10.1016/j.plantsci.2014.09.010
Chardon, F., Bedu, M., Calenge, F., Klemens, P. A., Spinner, L., Clement, G., et al. (2013). Leaf fructose content is controlled by the vacuolar transporter SWEET17 in Arabidopsis. Curr. Biol. 23, 697–702. doi: 10.1016/j.cub.2013.03.021
Chen, C., Yuan, Y., Zhang, C., Li, H., Ma, F., and Li, M. (2017). Sucrose phloem unloading follows an apoplastic pathway with high sucrose synthase in Actinidia fruit. Plant Sci. 255, 40–50. doi: 10.1016/j.plantsci.2016.11.011
Chen, L. Q., Hou, B. H., Lalonde, S., Takanaga, H., Hartung, M. L., Qu, X. Q., et al. (2010). Sugar transporters for intercellular exchange and nutrition of pathogens. Nature 468, 527–532.
Chen, L. Q., Qu, X. Q., Hou, B. H., Sosso, D., Osorio, S., Fernie, A. R., et al. (2012). Sucrose efflux mediated by SWEET proteins as a key step for phloem transport. Science 335, 207–211. doi: 10.1126/science.1213351
Chen, Z., Gao, K., Su, X., Rao, P., and An, X. (2015). Genome-Wide Identification of the Invertase Gene Family in Populus. PLoS One 10:e0138540. doi: 10.1371/journal.pone.0138540
Cheng, R., Cheng, Y., Lu, J., Chen, J., Wang, Y., Zhang, S., et al. (2018). The gene PbTMT4 from pear (Pyrus bretschneideri) mediates vacuolar sugar transport and strongly affects sugar accumulation in fruit. Physiol. Plant. 164, 307–319. doi: 10.1111/ppl.12742
Cho, Y. H., Sheen, J., and Yoo, S. D. (2010). Low glucose uncouples hexokinase1-dependent sugar signaling from stress and defense hormone abscisic acid and C2H4 responses in Arabidopsis. Plant Physiol. 152, 1180–1182. doi: 10.1104/pp.109.148957
Chong, J., Piron, M. C., Meyer, S., Merdinoglu, D., Bertsch, C., and Mestre, P. (2014). The SWEET family of sugar transporters in grapevine: VvSWEET4 is involved in the interaction with Botrytis cinerea. J. Exp. Bot. 65, 6589–6601. doi: 10.1093/jxb/eru375
Chourey, P. S., Li, Q. B., and Cevallos-Cevallos, J. (2012). Pleiotropy and its dissection through a metabolic gene Miniature1 (Mn1) that encodes a cell wall invertase in developing seeds of maize. Plant Sci. 184, 45–53. doi: 10.1016/j.plantsci.2011.12.011
Clifford, P. E., Offler, C. E., and Patrick, J. W. (1986). Growth regulators have rapid effects on photosynthetic unloading from seed coats of Phaseolus vulgaris L. Plant Physiol. Biochem. 80, 635–637. doi: 10.1104/pp.80.3.635
Conde, C., Agasse, A., Glissant, D., Tavares, R., Geros, H., and Delrot, S. (2006). Pathways of glucose regulation of monosaccharide transport in grape cells. Plant Physiol. 141, 1563–1577. doi: 10.1104/pp.106.080804
Coombe, B. C. (1992). Research on development and ripening of the grape berry. Am. J. Enol. Vitic. 43, 101–110.
Corelli Grappadelli, L., Morandi, B., Manfrini, L., and O’connell, M. (2019). Apoplasmic and simplasmic phloem unloading mechanisms: Do they co-exist in Angeleno plums under demanding environmental conditions? J. Plant Physiol. 237, 104–110. doi: 10.1016/j.jplph.2019.04.005
Dal Santo, S., Tucker, M. R., Tan, H. T., Burbidge, C. A., Fasoli, M., Bottcher, C., et al. (2020). Auxin treatment of grapevine (Vitis vinifera L.) berries delays ripening onset by inhibiting cell expansion. Plant Mol. Biol. 103, 91–111. doi: 10.1007/s11103-020-00977-1
Dass, H. C., and Randhawa, G. S. (1968). Effect of gibberellin on seeded ‘Vitis vinifera’ with special reference to induction of seedlessness. Vitis 7, 10–21.
Décima Oneto, C., Otegui, M. E., Baroli, I., Beznec, A., Faccio, P., Bossio, E., et al. (2016). Water deficit stress tolerance in maize conferred by expression of an isopentenyltransferase (IPT) gene driven by a stress- and maturation-induced promoter. J. Biotechnol. 220, 66–77. doi: 10.1016/j.jbiotec.2016.01.014
Deng, J., Yang, X., Sun, W., Miao, Y., He, L., and Zhang, X. (2020). The calcium sensor CBL2 and its interacting kinase CIPK6 are involved in plant sugar homeostasis via interacting with Tonoplast sugar transporter TST2. Plant Physiol. 183, 236–249. doi: 10.1104/pp.19.01368
Deng, X., Han, X., Yu, S., Liu, Z., Guo, D., He, Y., et al. (2020). OsINV3 and its homolog, OsINV2, control grain size in rice. Int. J. Mol. Sci. 21:2199. doi: 10.3390/ijms21062199
Doehlert, D. C. (1993). Sink strength: dynamic with source strength. Plant Cell Environ. 16, 1027–1028. doi: 10.1111/j.1365-3040.1996.tb02053.x
Dominguez, P. G., and Carrari, F. (2015). ASR1 transcription factor and its role in metabolism. Plant Signal. Behav. 10:e992751. doi: 10.4161/15592324.2014.992751
Duan, S., Wu, Y., Zhang, C., Wang, L., Song, S., Ma, C., et al. (2020). Differential regulation of metabolic enzyme activities and physio-anatomical aspects in Ca2+ sufficient and starved grapevine (Vitis vinifera L.). Sci. Hortic. 272:109423. doi: 10.1016/j.scienta.2020.109423
Engelbrecht, L., Orban, U., and Heese, W. (1969). Leaf-miner caterpillars and cytokinins in the ‘green islands’ of autumn leaves. Nature 223, 319–321. doi: 10.1038/223319a0
Fillion, L., Ageorges, A., Picaud, S., Coutos-Thevenot, P., Lemoine, R., Romieu, C., et al. (1999). Cloning and expression of a hexose transporter gene expressed during the ripening of grape berry. Plant Physiol. 120, 1083–1094. doi: 10.1104/pp.120.4.1083
Findlay, N., Oliver, K. J., Nil, N., and Coombe, B. G. (1987). Solute accumulation by grape pericarp cells. IV. Perfusion of pericarp apoplast via the pedicel and evidence for xylem malfunction in ripening berries. J. Exp. Bot. 38, 668–679. doi: 10.1093/jxb/38.4.668
Fisher, D. B., and Oparka, K. J. (1996). Post-phloem transport: principles and problems. J. Exp. Bot. 47, 1141–1154. doi: 10.1093/jxb/47.special_issue.1141
Fontes, N., Geros, H., and Delrot, S. (2011). Grape berry vacuole: a complex and heterogeneous membrane system specialized in the accumulation of solutes. Am. J. Enol. Vitic. 62, 270–278. doi: 10.5344/ajev.2011.10125
Garcia-Rojas, M., Meneses, M., Oviedo, K., Carrasco, C., Defilippi, B., Gonzalez-Aguero, M., et al. (2018). Exogenous gibberellic acid application induces the overexpression of key genes for pedicel lignification and an increase in berry drop in table grape. Plant Physiol. Biochem. 126, 32–38. doi: 10.1016/j.plaphy.2018.02.009
Geiger, D. R., and Shieh, W. (1993). Sink strength: learning to measure, measuring to learn. Plant Cell Environ. 16, 1017–1018. doi: 10.1111/j.1365-3040.1996.tb02048.x
Goetz, M., and Roitsch, T. (1999). The different pH optima and substrate specificities of extracellular and vacuolar invertases from plants are determined by a single amino-acid substitution. Plant J. 20, 707–711. doi: 10.1046/j.1365-313x.1999.00628.x
Gottwald, J. R., Krysan, P. J., Young, J. C., Evert, R. F., and Sussman, M. R. (2000). Genetic evidence for the in planta role of phloem-specific plasma membrane sucrose transporters. Proc. Natl. Acad. Sci. U.S.A. 97, 13979–13984. doi: 10.1073/pnas.250473797
Guan, L. (2011). Carbohydrate metabolism in grape cultivars that differ in sucrose accumulation. Vitis 50, 51–57.
Guivarc’h, A., Rembur, J., Goetz, M., Roitsch, T., Noin, M., Schmulling, T., et al. (2002). Local expression of the ipt gene in transgenic tobacco (Nicotiana tabacum L. cv. SR1) axillary buds establishes a role for cytokinins in tuberization and sink formation. J. Exp. Bot. 53, 621–629. doi: 10.1093/jexbot/53.369.621
Hansch, F., Jaspar, H., Von Sivers, L., Bitterlich, M., Franken, P., and Kuhn, C. (2020). Brassinosteroids and sucrose transport in mycorrhizal tomato plants. Plant Signal. Behav. 15, 1714292. doi: 10.1080/15592324.2020.1714292
Harris, J. M., Kriedemann, P. E., and Possingham, J. V. (1968). Anatomical aspects of grape berry development. Vitis 7, 106–119.
Hayes, M. A., Davies, C., and Dry, I. B. (2007). Isolation, functional characterization, and expression analysis of grapevine (Vitis vinifera L.) hexose transporters: differential roles in sink and source tissues. J. Exp. Bot. 58, 1985–1997. doi: 10.1093/jxb/erm061
Hayes, M. A., Feechan, A., and Dry, I. B. (2010). Involvement of abscisic acid in the coordinated regulation of a stress-inducible hexose transporter (VvHT5) and a cell wall invertase in grapevine in response to biotrophic fungal infection. Plant Physiol. 153, 211–221. doi: 10.1104/pp.110.154765
Ho, L. C. (1988). Metabolism and compartmentation of imported sugars in sink organs in relation to sink strength. Annu. Rev. Plant Physiol. Plant Mol. Biol. 39, 355–378. doi: 10.1146/annurev.pp.39.060188.002035
Hosseini, S. M., Poustini, K., and Ahmadi, A. (2008). Effects of foliar application of bap on source and sink strength in four six-rowed barley (Hordeum vulgare L.) cultivars. Plant Growth Regul. 54, 231–239. doi: 10.1007/s10725-007-9245-4
Imran, M., Rauf, A., Imran, A., Nadeem, M., Ahmad, Z., Atif, M., et al. (2017). Health benefits of grapes polyphenols. J. Environ. Agric. Sci. 10, 40–51.
Joshi, S., Choukimath, A., Isenegger, D., Panozzo, J., Spangenberg, G., and Kant, S. (2019). Improved wheat growth and yield by delayed leaf senescence using developmentally regulated expression of a cytokinin biosynthesis gene. Front. Plant Sci. 10:1285. doi: 10.3389/fpls.2019.01285
Khalil-Ur-Rehman, M., Sun, L., Li, C. X., Faheem, M., Wang, W., and Tao, J. M. (2017). Comparative RNA-seq based transcriptomic analysis of bud dormancy in grape. BMC Plant Biol. 17:18. doi: 10.1186/s12870-016-0960-8
Kobashi, K., Sugaya, S., Gemma, H., and Iwahori, S. (2001). Effect of abscisic acid (aba) on sugar accumulation in the flesh tissue of peach fruit at the start of the maturation stage. Plant Growth Regul. 35, 215–223.
Koch, K. (2004). Sucrose metabolism: regulatory mechanisms and pivotal roles in sugar sensing and plant development. Curr. Opin. Plant Biol. 7, 235–246. doi: 10.1016/j.pbi.2004.03.014
Kordel, B., and Kutschera, U. (2000). Effects of gibberellin on cellulose biosynthesis and membrane-associated sucrose synthase activity in pea internodes. J. Plant Physiol. 156, 570–573. doi: 10.1016/s0176-1617(00)80176-5
Kuhn, C. (2016). Review: post-translational cross-talk between brassinosteroid and sucrose signaling. Plant Sci. 248, 75–81. doi: 10.1016/j.plantsci.2016.04.012
Kuhn, N., Guan, L., Dai, Z. W., Wu, B. H., Lauvergeat, V., Gomès, E., et al. (2013). Berry ripening: recently heard through the grapevine. J. Exp. Bot. 65, 4543–4559. doi: 10.1093/jxb/ert395
Lecourieux, F., Kappel, C., Lecourieux, D., Serrano, A., Torres, E., Arce-Johnson, P., et al. (2014). An update on sugar transport and signalling in grapevine. J. Exp. Bot. 65, 821–832. doi: 10.1093/jxb/ert394
Lecourieux, F., Lecourieux, D., Vignault, C., and Delrot, S. (2010). A sugar-inducible protein kinase, VvSK1, regulates hexose transport and sugar accumulation in grapevine cells. Plant Physiol. 152, 1096–1106. doi: 10.1104/pp.109.149138
Li, X., Guo, W., Li, J., Yue, P., Bu, H., Jiang, J., et al. (2020). Histone acetylation at the promoter for the transcription factor PuWRKY31 affects sucrose accumulation in pear fruit. Plant Physiol. 182, 2035–2046. doi: 10.1104/pp.20.00002
Lim, S. D., Yim, W. C., Liu, D., Hu, R., Yang, X., and Cushman, J. C. (2018). A Vitis vinifera basic helix-loop-helix transcription factor enhances plant cell size, vegetative biomass and reproductive yield. Plant Biotechnol. J. 16, 1595–1615. doi: 10.1111/pbi.12898
Liu, Y., Wang, J., Yin, H., Zhang, A., Huang, S., Wang, T. J., et al. (2018). Trithorax-group protein ATX5 mediates the glucose response via impacting the HY1-ABI4 signaling module. Plant Mol. Biol. 98, 495–506. doi: 10.1007/s11103-018-0791-0
Liu, Y. H., Offler, C. E., and Ruan, Y. L. (2016). Cell wall invertase promotes fruit set under heat stress by suppressing ROS-independent cell death. Plant Physiol. 172, 163–180. doi: 10.1104/pp.16.00959
Ma, Q. J., Sun, M. H., Lu, J., Liu, Y. J., Hu, D. G., and Hao, Y. J. (2017). Transcription factor AREB2 is involved in soluble sugar accumulation by activating sugar transporter and amylase genes. Plant Physiol. 174, 2348–2362. doi: 10.1104/pp.17.00502
Meneses, M., Garcia-Rojas, M., Munoz-Espinoza, C., Carrasco-Valenzuela, T., Defilippi, B., Gonzalez-Aguero, M., et al. (2020). Transcriptomic study of pedicels from GA3-treated table grape genotypes with different susceptibility to berry drop reveals responses elicited in cell wall yield, primary growth and phenylpropanoids synthesis. BMC Plant Biol. 20:66. doi: 10.1186/s12870-020-2260-6
Meteier, E., La Camera, S., Goddard, M. L., Laloue, H., Mestre, P., and Chong, J. (2019). Overexpression of the VvSWEET4 transporter in grapevine hairy roots increases sugar transport and contents and enhances resistance to Pythium irregulare, a Soilborne Pathogen. Front. Plant Sci. 10:884. doi: 10.3389/fpls.2019.00884
Mothes, K., and Engelbrecht, L. (1962). On the activity of a kinetin-like root factor. Life Sci. 11, 852–857. doi: 10.1016/0024-3205(63)90098-5
Murcia, G., Pontin, M., Reinoso, H., Baraldi, R., Bertazza, G., Gomez-Talquenca, S., et al. (2016). ABA and GA3 increase carbon allocation in different organs of grapevine plants by inducing accumulation of non-structural carbohydrates in leaves, enhancement of phloem area and expression of sugar transporters. Physiol. Plant. 156, 323–337. doi: 10.1111/ppl.12390
Nelson, W. J., Lee, B. C., Gasperini, F. A., and Hair, D. M. (2012). Meeting the challenge of feeding 9 billion people safely and securely. J. Agromedicine 17, 347–350. doi: 10.1080/1059924x.2012.726161
Nicolas, P., Lecourieux, D., Gomes, E., Delrot, S., and Lecourieux, F. (2013). The grape berry-specific basic helix-loop-helix transcription factor VvCEB1 affects cell size. J. Exp. Bot. 64, 991–1003. doi: 10.1093/jxb/ers374
Nonis, A., Ruperti, B., Pierasco, A., Canaguier, A., Adam-Blondon, A. F., Di Gaspero, G., et al. (2008). Neutral invertases in grapevine and comparative analysis with Arabidopsis, poplar and rice. Planta 229, 129–142. doi: 10.1007/s00425-008-0815-0
Pan, Q. H., Li, M. J., Peng, C. C., Zhang, N., Zou, X., Zou, K. Q., et al. (2010). Abscisic acid activates acid invertases in developing grape berry. Physiol. Plant. 125, 157–170. doi: 10.1111/j.1399-3054.2005.00552.x
Peng, Q., Wang, L., Ogutu, C., Liu, J., Liu, L., Mollah, M. D. A., et al. (2020). Functional analysis reveals the regulatory role of PpTST1 encoding tonoplast sugar transporter in sugar accumulation of peach fruit. Int. J. Mol. Sci. 21:1112. doi: 10.3390/ijms21031112
Rabot, A., Portemer, V., Peron, T., Mortreau, E., Leduc, N., Hamama, L., et al. (2014). Interplay of sugar, light and gibberellins in expression of Rosa hybrida vacuolar invertase 1 regulation. Plant Cell Physiol. 55, 1734–1748. doi: 10.1093/pcp/pcu106
Reguera, M., Peleg, Z., Abdel-Tawab, Y. M., Tumimbang, E. B., Delatorre, C. A., and Blumwald, E. (2013). Stress-induced cytokinin synthesis increases drought tolerance through the coordinated regulation of carbon and nitrogen assimilation in rice. Plant Physiol. 163, 1609–1622. doi: 10.1104/pp.113.227702
Ren, R., Yue, X., Li, J., Xie, S., Guo, S., and Zhang, Z. (2020). Coexpression of sucrose synthase and the SWEET transporter, which are associated with sugar hydrolysis and transport, respectively, increases the hexose content in Vitis vinifera L. Grape Berries. Front. Plant Sci. 11:321. doi: 10.3389/fpls.2020.00321
Reynolds, A. G., Wardle, D. A., Zurowski, C., and Looney, N. E. (1992). Phenylureas CPPU and thidiazuron affect yield components, fruit composition, and storage potential of four seedless grape selections. J. Am. Soc. Hortic. Sci. 117, 85–89. doi: 10.21273/jashs.117.1.85
Roitsch, T. (1999). Source-sink regulation by sugar and stress. Curr. Opin. Plant Biol. 2, 198–206. doi: 10.1016/s1369-5266(99)80036-3
Roitsch, T., Bittner, M., and Godt, D. E. (1995). Induction of apoplastic invertase of Chenopodium rubrum by D-glucose and a glucose analog and tissue-specific expression suggest a role in sink-source regulation. Plant Physiol. 108, 285–294.
Roman, H., Girault, T., Barbier, F., Peron, T., Brouard, N., Pencik, A., et al. (2016). Cytokinins are initial targets of light in the control of bud outgrowth. Plant Physiol. 172, 489–509. doi: 10.1104/pp.16.00530
Sagar, M., Chervin, C., Mila, I., Hao, Y., Roustan, J. P., Benichou, M., et al. (2013). SlARF4, an auxin response factor involved in the control of sugar metabolism during tomato fruit development. Plant Physiol. 161, 1362–1374. doi: 10.1104/pp.113.213843
Saumonneau, A., Laloi, M., Lallemand, M., Rabot, A., and Atanassova, R. (2012). Dissection of the transcriptional regulation of grape ASR and response to glucose and abscisic acid. J. Exp. Bot. 63, 1495–1510. doi: 10.1093/jxb/err391
Spartz, A. K., Lee, S. H., Wenger, J. P., Gonzalez, N., Itoh, H., Inze, D., et al. (2012). The SAUR19 subfamily of SMALL AUXIN UP RNA genes promote cell expansion. Plant J. 70, 978–990. doi: 10.1111/j.1365-313x.2012.04946.x
Stein, O., and Granot, D. (2019). An overview of sucrose synthases in plants. Front. Plant Sci. 10:95. doi: 10.3389/fpls.2019.00095
Sturm, A. (1996). Moleculor characterization and functional analysis of sucrose-cleaving enzymes in carrot (Daucus carota L.). J. Exp. Bot. 47, 1187–1192. doi: 10.1093/jxb/47.special_issue.1187
Sturm, A., and Chrispeels, M. J. (1990). cDNA cloning of carrot extracellular beta-fructosidase and its expression in response to wounding and bacterial infection. Plant Cell 2, 1107–1119. doi: 10.1105/tpc.2.11.1107
Tamura, K., Stecher, G., Peterson, D., Filipski, A., and Kumar, S. (2013). MEGA6: molecular evolutionary genetics analysis version 6.0. Mol. Biol. Evol. 30, 2725–2729. doi: 10.1093/molbev/mst197
Turgeon, R., and Wolf, S. (2009). Phloem transport: cellular pathways and molecular trafficking. Annu. Rev. Plant Biol. 60, 207–221. doi: 10.1146/annurev.arplant.043008.092045
Tymowska-Lalanne, Z., and Kreis, M. (1998). Expression of the Arabidopsis thaliana invertase gene family. Planta 207, 259–265. doi: 10.1007/s004250050481
Ugare, B., Banerjee, K., Ramteke, S. D., Pradhan, S., Oulkar, D. P., Utture, S. C., et al. (2013). Dissipation kinetics of forchlorfenuron, 6-benzyl aminopurine, gibberellic acid and ethephon residues in table grapes (Vitis vinifera). Food Chem. 141, 4208–4214. doi: 10.1016/j.foodchem.2013.06.111
Upadhyay, A., Maske, S., Jogaiah, S., Kadoo, N. Y., and Gupta, V. S. (2018). GA3 application in grapes (Vitis vinifera L.) modulates different sets of genes at cluster emergence, full bloom, and berry stage as revealed by RNA sequence-based transcriptome analysis. Funct. Integr. Genomics 18, 439–455. doi: 10.1007/s10142-018-0605-0
Vaughn, M. W., Harrington, G. N., and Bush, D. R. (2002). Sucrose-mediated transcriptional regulation of sucrose symporter activity in the phloem. Proc. Natl. Acad. Sci. U.S.A. 99, 10876–10880. doi: 10.1073/pnas.172198599
Vergara, A. E., Diaz, K., Carvajal, R., Espinoza, L., Alcalde, J. A., and Perez-Donoso, A. G. (2018). Exogenous applications of brassinosteroids improve color of red table grape (Vitis vinifera L. Cv. “Redglobe”) Berries. Front. Plant Sci. 9:363. doi: 10.3389/fpls.2018.00363
Vignault, C., Vachaud, M., Cakir, B., Glissant, D., Dedaldechamp, F., Buttner, M., et al. (2005). VvHT1 encodes a monosaccharide transporter expressed in the conducting complex of the grape berry phloem. J. Exp. Bot. 56, 1409–1418. doi: 10.1093/jxb/eri142
Wan, H., Wu, L., Yang, Y., Zhou, G., and Ruan, Y. L. (2018). Evolution of sucrose metabolism: the dichotomy of invertases and beyond. Trends Plant Sci. 23, 163–177. doi: 10.1016/j.tplants.2017.11.001
Wang, W., Khalil-Ur-Rehman, M., Feng, J., and Tao, J. (2017). RNA-seq based transcriptomic analysis of CPPU treated grape berries and emission of volatile compounds. J. Plant Physiol. 218, 155–166. doi: 10.1016/j.jplph.2017.08.004
Wang, X., Zhao, M., Wu, W., Korir, N. K., Qian, Y., and Wang, Z. (2017). Comparative transcriptome analysis of berry-sizing effects of gibberellin (GA3) on seedless Vitis vinifera L. Genes Genomics 39, 493–507. doi: 10.1007/s13258-016-0500-9
Wang, X. Q., Zheng, L. L., Lin, H., Yu, F., Sun, L. H., and Li, L. M. (2017). Grape hexokinases are involved in the expression regulation of sucrose synthase- and cell wall invertase-encoding genes by glucose and ABA. Plant Mol. Biol. 94, 61–78. doi: 10.1007/s11103-017-0593-9
Wang, X. Q., Li, L. M., Yang, P. P., and Gong, C. L. (2014). The role of hexokinases from grape berries (Vitis vinifera L.) in regulating the expression of cell wall invertase and sucrose synthase genes. Plant Cell Rep. 33, 337–347. doi: 10.1007/s00299-013-1533-z
Wang, Z., Zhao, F., Zhao, X., Ge, H., Chai, L., Chen, S., et al. (2012). Proteomic analysis of berry-sizing effect of GA3 on seedless Vitis vinifera L. Proteomics 12, 86–94. doi: 10.1002/pmic.201000668
Weaver, R. J., and MacCune, S. B. (1961). Effect of gibberellin on vine behavior and crop production in seeded and seedless Vitis vinifera. Hilgardia 30, 425–444. doi: 10.3733/hilg.v30n15p425
Wei, W., Cheng, M. N., Ba, L. J., Zeng, R. X., Luo, D. L., Qin, Y. H., et al. (2019). Pitaya HpWRKY3 is associated with fruit sugar accumulation by transcriptionally modulating sucrose metabolic genes HpINV2 and HpSuSy1. Int. J. Mol. Sci. 20:1890. doi: 10.3390/ijms20081890
Werner, T., Holst, K., Pors, Y., Guivarc’h, A., Mustroph, A., Chriqui, D., et al. (2008). Cytokinin deficiency causes distinct changes of sink and source parameters in tobacco shoots and roots. J. Exp. Bot. 59, 2659–2672. doi: 10.1093/jxb/ern134
White, A. C., Rogers, A., Rees, M., and Osborne, C. P. (2016). How can we make plants grow faster? A source-sink perspective on growth rate. J. Exp. Bot. 67, 31–45. doi: 10.1093/jxb/erv447
Wong, D. C., Lopez Gutierrez, R., Dimopoulos, N., Gambetta, G. A., and Castellarin, S. D. (2016). Combined physiological, transcriptome, and cis-regulatory element analyses indicate that key aspects of ripening, metabolism, and transcriptional program in grapes (Vitis vinifera L.) are differentially modulated accordingly to fruit size. BMC Genomics 17:416. doi: 10.1186/s12864-016-2660-z
Xie, Z. S., Li, B., Forney, C. F., Xu, W. P., and Wang, S. P. (2009). Changes in sugar content and relative enzyme activity in grape berry in response to root restriction. Sci. Hortic. 123, 39–45. doi: 10.1016/j.scienta.2009.07.017
Xu, F., Xi, Z. M., Zhang, H., Zhang, C. J., and Zhang, Z. W. (2015). Brassinosteroids are involved in controlling sugar unloading in Vitis vinifera ‘Cabernet Sauvignon’ berries during véraison. Plant Physiol. Biochem. 94, 197–208. doi: 10.1016/j.plaphy.2015.06.005
Xu, Q., Yin, S., Ma, Y., Song, M., Song, Y., Mu, S., et al. (2020). Carbon export from leaves is controlled via ubiquitination and phosphorylation of sucrose transporter SUC2. Proc. Natl. Acad. Sci. U.S.A. 117, 6223–6230. doi: 10.1073/pnas.1912754117
Xu, X., Ren, Y., Wang, C., Zhang, H., Wang, F., Chen, J., et al. (2019). OsVIN2 encodes a vacuolar acid invertase that affects grain size by altering sugar metabolism in rice. Plant Cell Rep. 38, 1273–1290. doi: 10.1007/s00299-019-02443-9
Yu, S. M., Lo, S. F., and Ho, T. D. (2015). Source-sink communication: regulated by hormone, nutrient, and stress cross-signaling. Trends Plant Sci. 20, 844–857. doi: 10.1016/j.tplants.2015.10.009
Zeng, L., Wang, Z., and Vainstein, A. E. A. (2011). Cloning, localization, and expression analysis of a new tonoplast monosaccharide transporter from Vitis vinifera L. J. Plant Growth Regul. 30, 199–212. doi: 10.1007/s00344-010-9185-5
Zhang, C., Tanabe, K., Tamura, F., Itai, A., and Yoshida, M. (2007). Roles of gibberellins in increasing sink demand in japanese pear fruit during rapid fruit growth. Plant Growth Regul. 52, 161–172. doi: 10.1007/s10725-007-9187-x
Zhang, C., Tanabe, K., Tamura, F., Matsumoto, K., and Yoshida, A. (2005). 13C-photosynthate accumulation in Japanese pear fruit during the period of rapid fruit growth is limited by the sink strength of fruit rather than by the transport capacity of the pedicel. J. Exp. Bot. 56, 2713–2719. doi: 10.1093/jxb/eri264
Zhang, C., and Whiting, M. D. (2011). Pre-harvest foliar application of Prohexadione-Ca and gibberellins modify canopy source-sink relations and improve quality and shelf-life of ‘Bing’ sweet cherry. Plant Growth Regul. 65, 145–156. doi: 10.1007/s10725-011-9584-z
Zhang, X., Luo, G., Wang, R., Wang, J., and Himelrick, D. (2003). Growth and developmental responses of seeded and seedless grape berries to shoot girdling. J. Am. Soc. Hortic. Sci. 128, 316–323. doi: 10.21273/jashs.128.3.0316
Zhang, X. Y., Wang, X. L., Wang, X. F., Xia, G. H., Pan, Q. H., Fan, R. C., et al. (2006). A shift of Phloem unloading from symplasmic to apoplasmic pathway is involved in developmental onset of ripening in grape berry. Plant Physiol. 142, 220–232. doi: 10.1104/pp.106.081430
Zhang, Y., Zhen, L., Tan, X., Li, L., and Wang, X. (2014). The involvement of hexokinase in the coordinated regulation of glucose and gibberellin on cell wall invertase and sucrose synthesis in grape berry. Mol. Biol. Rep. 41, 7899–7910. doi: 10.1007/s11033-014-3683-7
Zhang, Z., Zou, L., Ren, C., Ren, F., Wang, Y., Fan, P., et al. (2019). VvSWEET10 mediates sugar accumulation in grapes. Genes 10:255. doi: 10.3390/genes10040255
Zheng, M., Yang, T., Tao, P., Zhu, C., Fu, Y., and Hsu, Y. F. (2019). Arabidopsis GSM1 is involved in ABI4-regulated ABA signaling under high-glucose condition in early seedling growth. Plant Sci. 287:110183. doi: 10.1016/j.plantsci.2019.110183
Keywords: grape, sink strength, sugar accumulation, sugar transporters, sugar-cleaving enzymes, hormones
Citation: Li Y-M, Forney C, Bondada B, Leng F and Xie Z-S (2021) The Molecular Regulation of Carbon Sink Strength in Grapevine (Vitis vinifera L.). Front. Plant Sci. 11:606918. doi: 10.3389/fpls.2020.606918
Received: 16 September 2020; Accepted: 08 December 2020;
Published: 08 January 2021.
Edited by:
Sakiko Okumoto, Texas A&M University, United StatesReviewed by:
Zhanwu Dai, Institute of Botany (CAS), ChinaCopyright © 2021 Li, Forney, Bondada, Leng and Xie. This is an open-access article distributed under the terms of the Creative Commons Attribution License (CC BY). The use, distribution or reproduction in other forums is permitted, provided the original author(s) and the copyright owner(s) are credited and that the original publication in this journal is cited, in accordance with accepted academic practice. No use, distribution or reproduction is permitted which does not comply with these terms.
*Correspondence: Zhao-Sen Xie, eGllemhhb3NlbkBzaW5hLmNvbQ==
Disclaimer: All claims expressed in this article are solely those of the authors and do not necessarily represent those of their affiliated organizations, or those of the publisher, the editors and the reviewers. Any product that may be evaluated in this article or claim that may be made by its manufacturer is not guaranteed or endorsed by the publisher.
Research integrity at Frontiers
Learn more about the work of our research integrity team to safeguard the quality of each article we publish.