- 1Department of Plant Breeding, IFZ Research Centre for Biosystems, Land Use and Nutrition, Justus Liebig University Giessen, Giessen, Germany
- 2Department of Botany and Molecular Evolution, Senckenberg Research Institute and Natural History Museum Frankfurt, Frankfurt, Germany
Flowering is a vulnerable, but crucial phase in building crop yield. Proper timing of this period is therefore decisive in obtaining optimal yields. However, genetic regulation of flowering integrates many different environmental signals and is therefore extremely complex. This complexity increases in polyploid crops which carry two or more chromosome sets, like wheat, potato or rapeseed. Here, I summarize the current state of knowledge about flowering time gene copies in rapeseed (Brassica napus), an important oil crop with a complex polyploid history and a close relationship to Arabidopsis thaliana. The current data show a high demand for more targeted studies on flowering time genes in crops rather than in models, allowing better breeding designs and a deeper understanding of evolutionary principles. Over evolutionary time, some copies of rapeseed flowering time genes changed or lost their original role, resulting in subfunctionalization of the respective homologs. For useful applications in breeding, such patterns of subfunctionalization need to be identified and better understood.
Introduction
Rapeseed for Future
In a future fossil-free mobility strategy, plant-based fuels cannot fully replace fossil fuels, as the production quantity of plant oils is by far too low (Carlsson et al., 2011), but they may be used in all cases where electricity-based machines cannot provide sufficient power, like tractors or harvesting machines (Bender, 1999). Plant-based fuels will therefore become an important building block in decarbonizing agriculture. The most popular plant-based fuel in temperate areas is biodiesel, which is mostly produced from rapeseed oil (Bušić et al., 2018). Although rapeseed oil is also a healthy edible oil, its use for fuel is dominating. Rapeseed is grown all across the globe in different climate zones from boreal to subtropical climates and constitutes the second most important oil crop of the world after soybean, comparable to oil palm (FAOSTAT, sourced July 2020). Breeding has formed three distinct oilseed rape growth types: spring rapeseed, which is grown as an annual, mostly in Australia and Canada, semi-winter rapeseed, which gives better yield but has an increased vegetation period, and winter rapeseed, which is biennial and yields best of all three types. Growth type is largely determined by flowering behavior and winter hardiness. Winter types are winter-hardy and depend on a period of prolonged cold to attain the ability to flower (vernalization), while semi-winter types are less vernalization dependent and lack winter hardiness, and spring types lack both traits (Schiessl et al., 2017b). Besides those oilseed forms, a further subspecies, ssp. napobrassica, is cultivated as beets and known as swedes or rutabagas. Swedes are generally strongly vernalization-dependent, but lack strong winter hardiness. Due to the importance of those traits, each growth type usually constitutes its own breeding pool. However, breeding for other traits, mainly oil and seed quality, has strongly reduced genetic diversity within the breeding pools, and cross-breeding between pools might be one solution to increase genetic diversity and increase breeding gains (Snowdon and Iniguez Luy, 2012). Shifts in climate zones due to climate change may also demand growth type adaptation in future (IPCC, 2019).
Why Timing of Flowering Does Matter
In most plants and also in oilseed rape, flowering is the most sensitive phase for yield building due to various reasons: the increased energy demand due to flower formation (Borghi et al., 2019), but also due to shadowing of the leaves by the flowers, reducing photosynthesis (Diepenbrock, 2000). Flowering time is also critical in terms of nitrogen use efficiency, as N uptake after flowering is strongly correlated to yield (Berry et al., 2010). Moreover, drought stress during flowering was found to be much more devastating than during vegetative development (Hohmann, 2017). Many rapeseed growing areas face increased likelihoods of droughts during rapeseed flowering times due to climate change (Lu et al., 2019). If the drought period is short, drought avoidance including later flowering can be a successful strategy (Schiessl et al., 2020), but this is highly dependent on synchronization of drought period and flowering time. In winter oilseed rape, flowering time is also a general obstacle to breeding progress. Winter oilseed rape requires long periods of 6 to 10 weeks of vernalization to achieve flowering competence, leading to long generation times. In other crops and in spring rapeseed, attempts to shorten generation times in growth chambers were successful using special light regimes (Ghosh et al., 2018; Watson et al., 2018); however, this remains to be achieved for winter rapeseed. Finally, oilseed rape is a facultative outcrossing species and therefore not strictly dependent on pollinators, however, the presence of pollinators in the field normally increase seed yield and quality (Bommarco et al., 2012; Zou et al., 2017; Adamidis et al., 2019). It is thus helpful to synchronize flowering time with pollinators’ activity. Flowering time has therefore an important influence on many agronomic traits and environment-specific flowering time adaptation needs to be carried out in individual breeding programs to maximize yield.
What Is Different to Arabidopsis thaliana?
Brassica napus is a close relative to the model crucifer Arabidopsis thaliana. Most A. thaliana flowering time genes are conserved in the species (Osborn et al., 1997), indicating that flowering time regulation in oilseed rape is regulated in a similar way as in the model system. There are, however, species-specific drawbacks in this conclusion which are mainly due to the polyploid nature of the B. napus genome. B. napus is a recent allotetraploid carrying a subgenome A from the donor species B. rapa as well as a subgenome C from the donor species B. oleracea (Morinaga, 1934; Nagaharu, 1935). B. rapa and B. oleracea are two closely related species separated by around 4.5 Mio years of evolution, going back to a common hexaploid ancestor (Schiessl and Mason, 2020). This evolutionary history raises the theoretical number of homologs to six, although gene loss has reduced the average copy number to 4.4 (Parkin et al., 2010). For the main flowering time regulators, the average copy number was found to be 4.8, ranging from one to up to twelve copies (Schiessl et al., 2014). Moreover, the individual copy number in flowering time was found to be highly variable in a representative diversity set, although this did not affect all copies equally (Schiessl et al., 2017b). At the same time, several transcriptomic studies have shown that expression patterns vary a lot between copies of the same Arabidopsis homolog, with different expression maxima, different tissue or age specificity or different reactivity to stress (Zhou et al., 2007; Guo et al., 2014; Shah et al., 2018; Kittipol et al., 2019; Schiessl et al., 2019a). Together, this indicates that flowering time genes in B. napus underwent considerable subfunctionalization. To identify useful breeding targets, it is therefore crucial to identify the conserved or acquired role of each flowering time gene copy individually. In the next paragraphs, I will summarize the progress of such attempts for the different regulatory modules identified in Arabidopsis. A list of B. napus homologs detected within QTL, GWAS peaks or located in selective sweeps is given in Table 1. For general reviews about flowering time regulation in models and crops, please refer to the excellent works of others in this area (Jung and Muller, 2009; Srikanth and Schmid, 2011; Blümel et al., 2015).
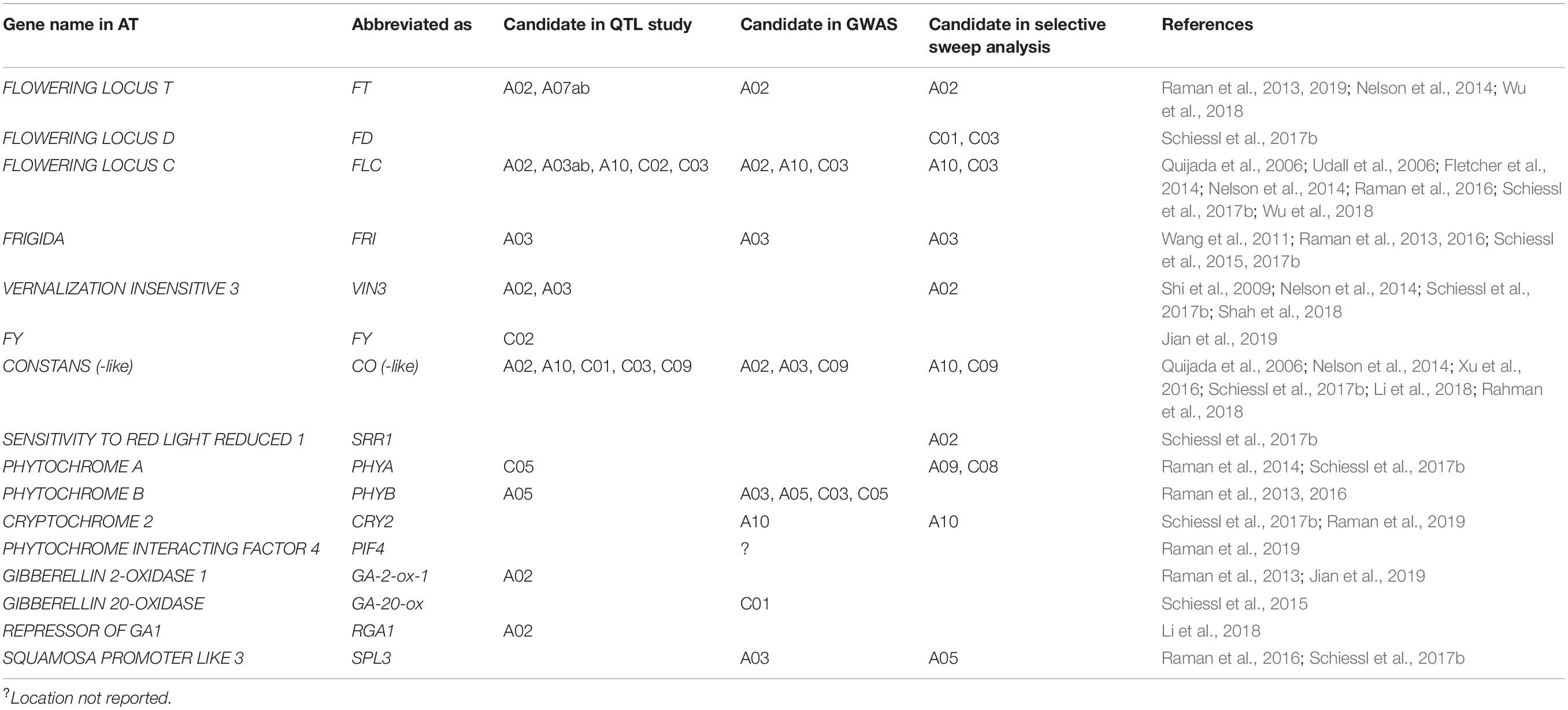
Table 1. List of most important flowering time genes with respective QTL, GWAS peaks or within selective sweeps in B. napus with chromosomal locations and references.
The Central Hub: FT, FD, and SOC1
The major flowering regulator FLOWERING LOCUS T (FT) has six copies in B. napus, located on chromosomes A02, A07 (two copies), C02 and C06 (two copies) (Wang J. et al., 2009). BLAST positions in the first published reference genome, however, were partially different and assigned one copy to C04, which could be misassembly (Schiessl et al., 2017b). Bna.FT.C02 was reported to be pseudogenized, obviously due to a transposon insertion into its promoter region (Wang et al., 2012). Non-expression of Bna.FT.C02 was later confirmed in a winter type (Guo et al., 2014), while others found flowering time QTL (Rahman et al., 2018) and reported expression in spring type (Raman et al., 2019). The same study found a correlation between the expression of all copies with flowering time, at least in spring type B. napus (Raman et al., 2019). Several copies of Bna.FT (on A02 or A07) were found in major QTL intervals in different studies, all of them in populations derived from spring type rapeseed (Raman et al., 2013, 2019; Nelson et al., 2014). EMS mutants of Bna.FT.C06b, but not of Bna.FT.C06a changed flowering time in a winter type (Guo et al., 2014). The copies on A07 and C06 are located within inverted duplicated regions, indicating they arose from a tandem duplication before the speciation separating B. rapa and B. oleracea (Wang J. et al., 2009). Regulatory regions of Bna.FT.A02 and Bna.FT.C02 were lacking a binding site known to be important for binding of the vernalization regulator FLC, the so-called CArG box, but were containing a binding motif for the photoperiod regulator CONSTANS (CO) (Wang J. et al., 2009; Raman et al., 2019). The copies on A07 and C06 showed the opposite pattern (Wang J. et al., 2009; Raman et al., 2019). This indicates that regulation via vernalization and regulation via photoperiod might have been split between the A02/C02 copies and the A07/C06 copies, although this has not been demonstrated yet. An extensive study on natural variation in almost 1,000 B. napus accessions found that the second strongest selection signature between winter and spring type B. napus was located in a region harboring Bna.FT.A02 (Wu et al., 2018). A region 3 kb upstream of this copy also showed the strongest GWAS peak for flowering time in the same study, indicating promoter variation accounts for the effect (Wu et al., 2018). Indeed Bna.FT.A02 expression was different between winter, semi-winter and spring material (Wu et al., 2018). Another study found that Bna.FT.A02 expression was not released directly after vernalization, but only later shortly before BBCH60, beginning of flowering (Guo et al., 2014). The A07/C06 copies, however, responded directly to vernalization (Guo et al., 2014). Together with the promoter motif analysis, this indicates that the A07/C06 copies may be majorly regulated by vernalization (Bna.FLC), while the A02 copy may be majorly regulated by day length (Bna.CO) (see Figure 1 for a model). Interestingly, no functional variation for the coding regions of Bna.FT.A02 and Bna.FT.C02 was found across 280 accessions of B. napus, indicating expression variation was exclusively due to promoter variation (Schiessl et al., 2017b). The same study also found that Bna.FT.A02 was never affected by a deletion event, supportive of this copy being essential (Schiessl et al., 2017b). Bna.FT.A02 was also found to react to drought stress in winter type rapeseed (Schiessl et al., 2020), possibly via the age pathway. A recent study comparing Bna.FT.A02 expression in early and late winter type rapeseed found that there may be genotypic variance in the responsiveness to vernalization, as the early flowering Cabriolet was able to upregulate Bna.FT.A02 in response to vernalization, while the late flowering genotype Darmor was not or only slightly (Tudor et al., 2020).
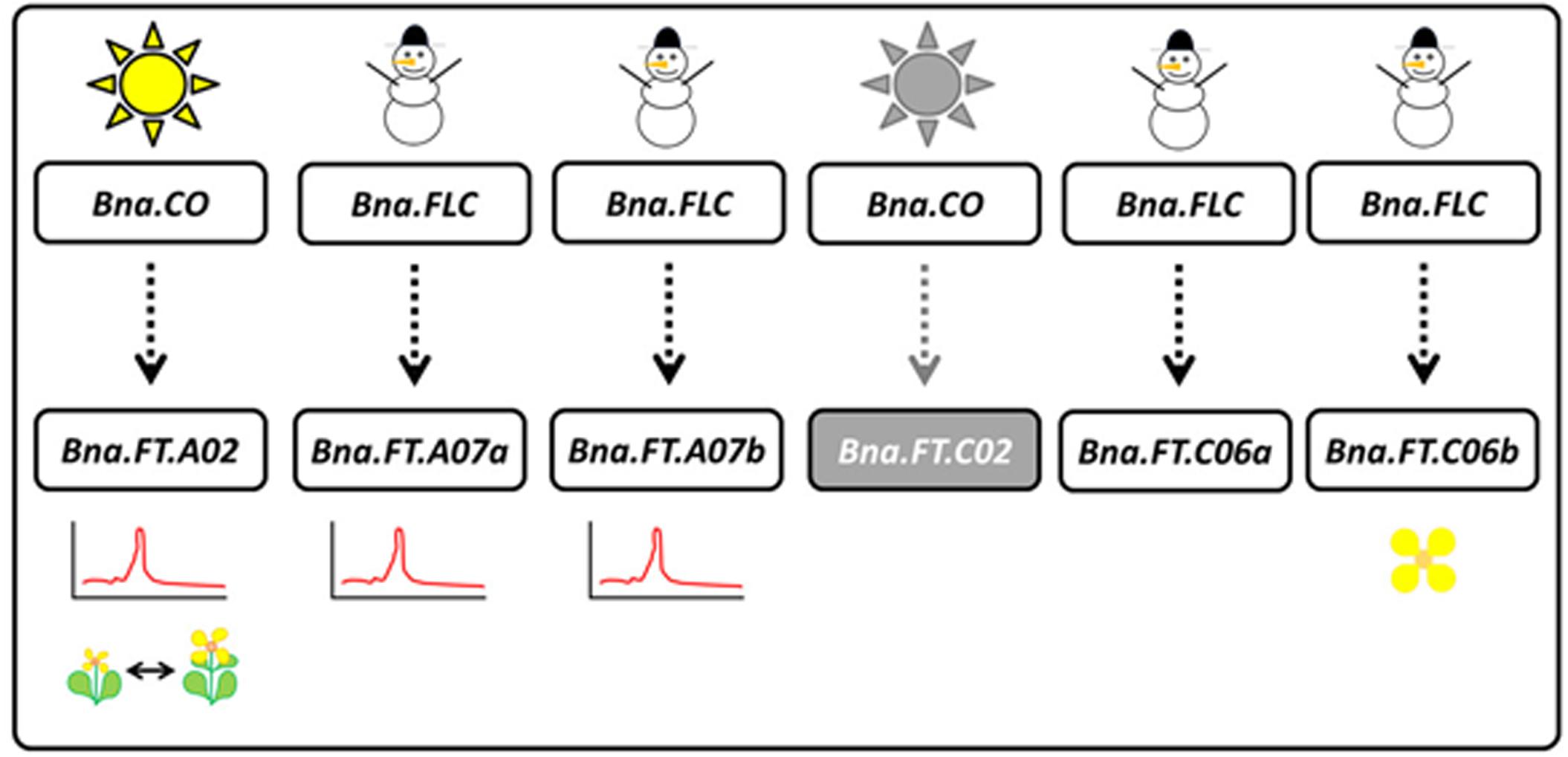
Figure 1. Model of Bna.FT regulation based on available literature data (references see main text). Bna.FT.C02 is most likely to be a pseudogene (gray). Bna.FT.C06b mutation was shown to influence flowering time (flower). Bna.FT copies on A02 and A07 were found in flowering time QTL (LOD plot). Promoter and gene expression analysis indicates that Bna.FT.A02 (and unlikely Bna.Ft.C02, if expressed) responds to Bna.CO and day length regulation (sun), while copies on A07 and C06 respond to Bna.FLC and vernalization (snowman). Bna.FT.A02 was also found to underlie a selective sweep between winter, semi-winter, and spring material (two contrasting rapeseed plants).
The protein binding partner of FT, FLOWERING LOCUS D (FD) (note: there’s also another gene named the same way, but abbreviated as FLD working in the autonomous pathway) has received much less attention than FT, and has also not be named as a candidate gene in any of the numerous QTL studies for flowering time up to date. Two copies, Bna.FD.C01 and Bna.FD.C03, showed distinct patterns of allelic variation in swedes, indicating they would contribute to the differential flowering behavior of this subspecies (Schiessl et al., 2017b).
The second most important floral integrator, SUPPRESSOR OF OVEREXPRESSION OF CONSTANS 1 (SOC1) is also not well studied in B. napus. No data is available on Bna.SOC1 expression patterns for its six copies. One of its copies, Bna.SOC1.A05, was found to be highly conserved across 280 diverse B. napus accessions, possibly indicating special functional importance (Schiessl et al., 2017b). Analysis of promoter sequences of different Brassica SOC1 homologs point into the same direction (Sri et al., 2020). The authors found that all six B. napus SOC1 promoter sequences show distinct patterns of transcription factor binding sites, similar to the diploid Brassica species and B. juncea (Sri et al., 2020). In B. rapa and B. juncea, the resulting expression patterns were markedly different, with differences in expression between tissues (for copies on A05 and A03) and in total expression level and response (for the A04 copy) (Sri et al., 2020). In B. juncea, the patterns of transcription factor binding sites were correlating with the respective expression patterns and with expression of putative regulators (Sri et al., 2020), showing elegantly how post-polyploidization diversification in promoter sequences can lead to subfunctionalization.
Vernalization: FLC, FRI, and VIN3
Most studies on B. napus flowering time genes have been performed on the main vernalization regulator Bna.FLC, mostly because it was recognized as a candidate gene for many flowering time QTL quite early on (Quijada et al., 2006; Udall et al., 2006; Fletcher et al., 2014; Nelson et al., 2014; Raman et al., 2016). Bna.FLC has nine annotated copies in the B. napus genome, located on chromosomes A02, A03 (two copies), A10, C02, and C03 (two copies), and C09 (two copies) (Zou et al., 2012). Moreover, an incomplete copy on A01 has also been reported, most likely a duplicate of the A02 copy (Schiessl et al., 2019a). Early transformation studies indicated not all of them have the same effect, with the copy on A10 having the strongest effect on vernalization (Tadege et al., 2001). Later on, it was found that this effect was due to a MITE transposon insertion into the promoter of Bna.FLC increasing Bna.FLC expression in winter type rapeseed (Hou et al., 2012). While semi-winter material originally showed a comparable Bna.FLC.A10 expression, downregulation in response to cold happened much quicker than in a winter type, presumably because the transposon sequence interfered with the mechanism of downregulation (Hou et al., 2012). In spring type accessions, Bna.FLC.A10 expression was found to be low even before vernalization (Wu et al., 2018; Schiessl et al., 2019a). Wu et al. (2018) subsequently also found that the strongest selection signal between winter and spring accessions was located close to the A10 copy, linked to differential expression between winter and spring material, a finding confirmed by others (Schiessl et al., 2017b, 2019a). At the same time, Bna.FLC.C03b was found to be a pseudogene (Zou et al., 2012; Schiessl et al., 2019a). Meanwhile, the role of the other seven copies remains unclear. Both copies on C09 and partly also Bna.FLC.C03a were found to have lost their cold responsiveness (Schiessl et al., 2019a). However, Bna.FLC.A02, Bna.FLC.A03ab, and Bna.FLC.C02 as well as Bna.FLC.A10 are downregulated by cold (Raman et al., 2016; Schiessl et al., 2019a). Raman et al. claim that FLC2 (Bna.FLC.A02/C02) is responsible for 22% of the flowering time variation in non-vernalized conditions in a diverse population of spring type rapeseed (Raman et al., 2016). Only Bna.FLC.A03a and Bna.FLC.C02 show some degree of differential expression between winter and spring, although this seems to be dependent on the age of the sampled leaf material (Schiessl et al., 2019a). Varying tissue-specific differences in Bna.FLC expression have been reported before (Zou et al., 2012). The data indicate that Bna.FLC.A10 is not only responsible for the high vernalization dependency of winter types, but also for the moderate vernalization dependency of semi-winter types. A recent study identified additional transposon insertions influencing expression in the promoters of Bna.FLC.A10 and Bna.FLC.A02 and concluded that Bna.FLC.A10 determines growth type in combination with Bna.FLC.A02 (Yin et al., 2020). Interestingly, swedes (ssp. napobrassica), showing an extremely strong vernalization dependency, were reported to carry two copies of Bna.FLC.A10, possibly as a result of selection against bolting (Schiessl et al., 2017b). In case of monomorphic Bna.FLC.A10, other Bna.FLC copies (A02, A03, and C02) may still show up as modulating flowering time factors in non-vernalized conditions. Likewise, Bna.FLC.A02, Bna.FLC.A03b, and Bna.FLC.C02 seem to be influential on flowering time in spring material, although they are only able to delay, but not to inhibit flowering. This was recently confirmed for Bna.FLC.A02 (Tudor et al., 2020) (Figure 2).
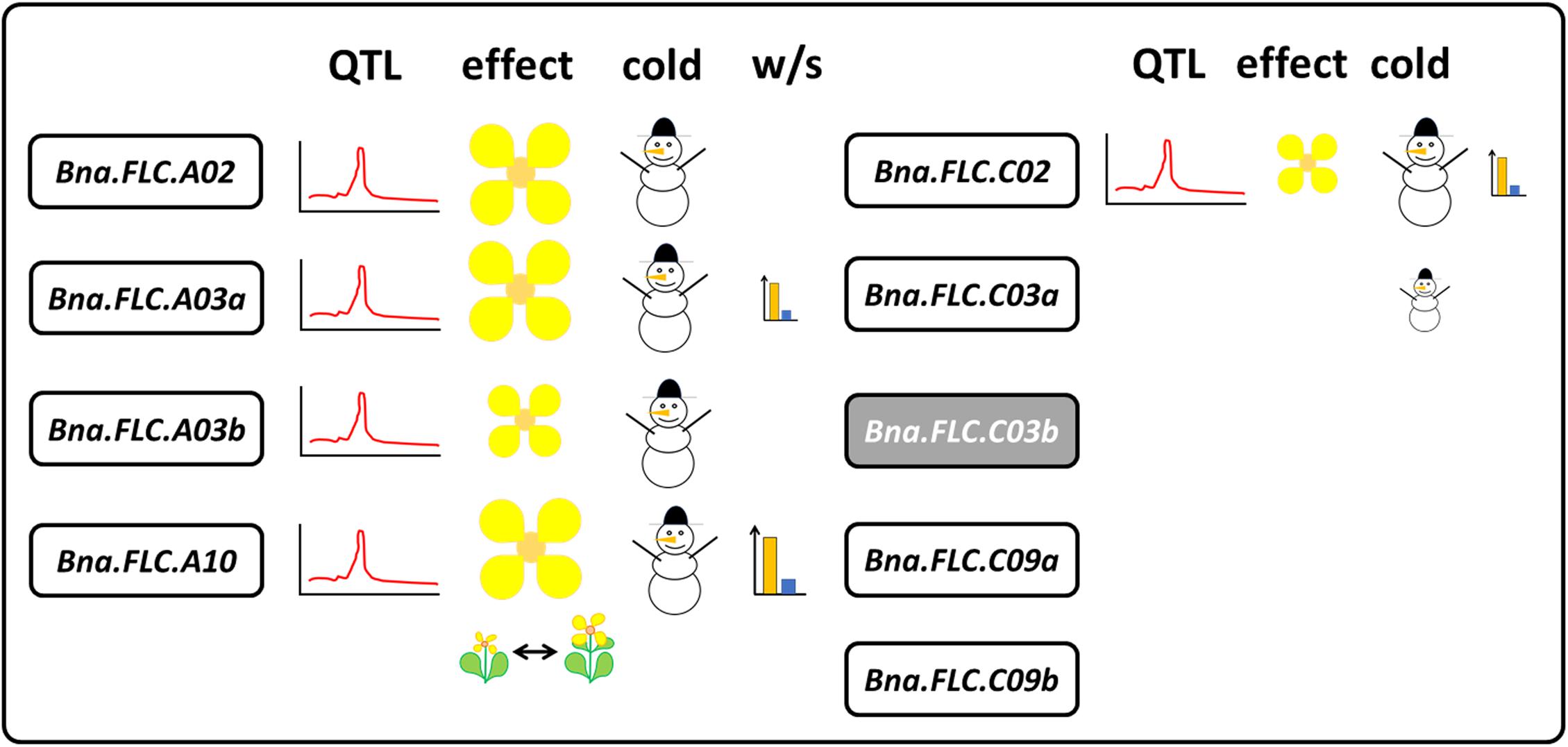
Figure 2. Summary of Bna.FLC copy functionality based on literature data (references see main text). Bna.FLC.C03b is most likely a pseudogene (gray). Copies on A02, A03, A10, and C02 have been found in QTL for flowering time (LOD plot). The same copies were able to complement the Arabidopsis flc mutation (flower, size indicates effect). The same copies were also shown to be downregulated under cold (snowman), while Bna.FLC.C03a still showed partial downregulation. Bna.FLC.A03b, Bna.FLC.A10, and Bna.FLC.C02 have been shown to be differentially expressed between winter and spring material (Barplot, size indicates degree), but only Bna.FLC.A10 was located in a selective sweep between winter and spring (gene expression co-segregating) and between swede and non-swede (two contrasting rapeseed plants).
In A. thaliana, vernalization requirement was attributed to variation in either FLC or FRIGIDA (FRI) (Jiang et al., 2009; Choi et al., 2011). FRI has four copies in the B. napus genome, of which one of them, Bna.FRI.A03, was associated to flowering variation quite early on (Wang et al., 2011). The same copy was later on found to be associated to the subspecies differentiation of swedes, but also for the winter-spring split (Schiessl et al., 2017b). All four copies are expressed (Wang et al., 2011; Schiessl et al., 2019a), and the expression level was found to be comparable between copies, growth types and seemed to be independent of vernalization, but the copies named BnaA.FRI.a (A03) and BnaX.FRI.d showed significant differences in tissue patterning, being expressed mostly in flowers, while showing very low expression in leaves (Wang et al., 2011). The copies on A03 and C03 were slightly upregulated upon cold, but returned to normal levels afterwards (Schiessl et al., 2019a). Four haplotypes which were associated to differential flowering behavior were detected, but not in spring types, loosely in semi-winter and strongly in winter type accessions (Wang et al., 2011). Those haplotypes contained several non-synonymous SNPs and InDels (Wang et al., 2011). A GWAS for flowering time in winter material in 12 different environments detected a respective peak in a Chinese environment with mild winters, indicating it might influence flowering under non-saturated vernalization conditions (Schiessl et al., 2015). A QTL study in spring material detected a QTL in the vicinity of Bna.FRI.A03, but no allelic effect of the copy itself (Raman et al., 2013) as well as a GWAS performed predominantly in semi-winter accessions (Raman et al., 2016). So while there is a clear consent that only Bna.FRI.A03 is influential for flowering time, the reason for this is unclear. As two of the other copies were predicted to have altered protein structures as compared to A. thaliana (Wang et al., 2011), these copies might already have attained a different role.
Another vernalization gene which has been brought up as a candidate gene to influence flowering time in oilseed rape is VERNALIZATION INSENSITIVE 3 (VIN3) (Shi et al., 2009; Nelson et al., 2014; Schiessl et al., 2017b; Shah et al., 2018). It has four copies in B. napus, of which mostly the A02 copy was attributed to phenotypic effects (Shi et al., 2009; Nelson et al., 2014; Schiessl et al., 2017b; Shah et al., 2018). However, gene expression analysis in a spring accession (Westar) over time showed that all four copies get upregulated during cold in both leaf and apex, as expected from A. thaliana, and get downregulated upon return to warmer temperatures (Schiessl et al., 2019a). Bna.VIN3.C03 had the lowest expression, while the others showed comparable expression levels. This would indicate a low level of subfunctionalization in terms of gene expression, and the reason why only Bna.VIN3.A02 has been found as candidate might be attributed to existing variation in coding regions. Analysis of SNP distribution in B. napus populations of winter, spring and swede growth types found Bna.VIN3.A02 as candidate for the winter-spring split, and Bna.VIN3.A03 as a candidate for the swede split (Schiessl et al., 2017b). However, no non-synonymous SNP was found to associate with this pattern (Schiessl et al., 2017b), contradicting this hypothesis. Works in B. oleracea (cauliflower) have shown that the dynamics of upregulation in Bol.VIN3 instead of absolute expression level are decisive for flowering time variation (Ridge et al., 2015). More precise time series of Bna.VIN3 expression in cold are therefore needed to judge the degree of subfunctionalization between the Bna.VIN3 copies.
Autonomous Pathway
The autonomous pathway in A. thaliana is regulating FLC mRNA concentration independently of cold (Cheng et al., 2017). The autonomous pathway gene Bna.FY was named particularly often in QTL studies before the publication of the B. napus reference genome, as many candidate regions showed a BLAST hit to “a region at the top of chromosome 5 containing the flowering time genes FLC, FY, and CO” (Raman et al., 2013; Fletcher et al., 2014; Luo et al., 2014); however, in most cases the major effects might rather have been due to FLC or CO than to FY. However, Jian et al. (2019) found seven members of the autonomous pathway were differentially expressed between parents of their RIL population and at the same time located in a respective flowering time QTL, also including Bna.FY, indicating it might still contribute to flowering time variation. Some other homologs of autonomous pathway genes have also been named as candidate genes for flowering time in B. napus, like FLD (Raman et al., 2016; Jian et al., 2019), AGL18 (Raman et al., 2016), or two copies of LD (Schiessl et al., 2015). However, data allowing a deeper insight into copy-specific regulation or involvement is not available up to now, and much is left to reveal for autonomous pathway genes in B. napus.
Photoperiod: CO, GI, SRR1, PHY, and CRY
In contrast to vernalization, the photoperiod behavior of rapeseed is not well studied. It is known that rapeseed does generally not flower at a day length of 8 h (most vernalization chambers run at this day length). However, in spring rapeseed, 24% of accessions were able to flower at 8 h day length (Raman et al., 2019), while in a different study, spring rapeseed was generally reported to flower at a day length of 10 h, although strongly delayed (Rahman et al., 2018). In the same study, flowering time was not different between 14 h day length and 18 h day length, so the critical day length at least for spring rapeseed lies between 10 and 14 h of light (Rahman et al., 2018). Others found that there is genotypic variation for critical day length between accessions, ranging between 10 and 12 h (Luo et al., 2018). However, in field trials, photoperiod is often confounded with temperature, so the exact influence of photoperiod remains elusive to date.
At the same time, not a lot is known about the main photoperiod pathway gene Bna.CO, although it was one of the first B. napus flowering time genes to be investigated (Robert et al., 1998). This early study identified four copies which were all expressed (Robert et al., 1998). One of them was able to complement the co-1 mutation in A. thaliana (Robert et al., 1998). When a reference genome for B. napus became available, six copies of Bna.CO were identified, along with four copies of CO-like genes, which complicate the analysis (Schiessl et al., 2014). Copies of Bna.CO or Bna.CO-like were named as candidate genes for flowering time QTL on A02 (Nelson et al., 2014) A10 (Quijada et al., 2006), C01 (Rahman et al., 2018), C03 (Li et al., 2018) and C09 (Xu et al., 2016). Copies of Bna.CO and Bna.CO-like on C09 with respective non-synonymous SNP variation were also found in regions separating swedes from non-swede material (Schiessl et al., 2017b). Although not every candidate gene might turn out as a true reason for phenotypic variance, the diversity of gene loci detected does not point to substantial subfunctionalization. This would in turn mean that gene dosage and therefore the number of loci able to produce functional protein could play a larger role here. Bna.CO copies on the C genome were found to be stable in copy number, while the other copies showed considerable variation (Schiessl et al., 2017b). When comparing gene expression between early and late flowering semi-winter accessions, no difference for any Bna.CO copy was found (Jian et al., 2019), however, the samples were taken at 10 am, where CO expression is normally still low. To our knowledge, more data on gene expression, subfunctionalization or protein stability have not been raised, and further conclusions on this important flowering time regulator are not possible to date.
In A. thaliana, CO transcription is repressed by CDF proteins, which in turn are negatively regulated by GIGANTEA (GI) and FKF1 (Johansson and Staiger, 2015). Bna.CDF1 and Bna.CDF2 were found to be down-regulated in early flowering semi-winter rapeseed relative to late flowering semi-winter in the morning, although single copies showed the opposite behavior (Jian et al., 2019). Bna.CDF1 has four copies in the B. napus genome, of which two were found to vary strongly in copy number (Schiessl et al., 2017b).
CDF1 seems to be controlled by a novel protein called SENSITIVITY TO RED LIGHT REDUCED 1 (SRR1) (Johansson and Staiger, 2014). In B. napus, Bna.SRR1 has five copies, of which two (on A03 and A10) were not expressed (Schiessl et al., 2019b). The remaining three showed differential expression patterns between spring and winter type rapeseed (Schiessl et al., 2019b), in line with the finding that Bna.SRR1.A02 was found to be a candidate for the winter-spring split earlier (Schiessl et al., 2017b). Bna.SRR1.A02 and Bna.SRR1.C02 were also able to complement the respective knockout mutant in A. thaliana, while Bna.SRR1.C09 was not (Schiessl et al., 2019b). Bna.SRR1.C09 carried a deletion of several amino acids in a putatively important region (Schiessl et al., 2019b). It is therefore highly likely that the gene copies underwent subfunctionalization, with Bna.SRR1.A02 being the most functionally conserved copy, influencing flowering time via CDF1 (Schiessl et al., 2019b).
In Arabidopsis, CO is further regulated post-translationally via the action of several ubiquitin-E3-ligases and photoreceptors like phytochromes and cryptochromes. Interestingly, Bna.PHYA, Bna.PHYB, and Bna.CRY2 all retained the same copy number (five copies) in B. napus (Schiessl et al., 2017a). Both Bna.PHYB, a negative regulator of CO protein stability (Raman et al., 2013, 2016) as well as the positive regulators Bna.PHYA (Raman et al., 2014) and Bna.CRY2 (Raman et al., 2019) were found to be candidates for flowering time in different QTL and GWAS studies. A structural rearrangement encompassing the A09 and C08 copies of Bna.PHYA was found to be associated to the swede morphotype, along with allelic variance in Bna.CRY2.A10 (Schiessl et al., 2017b), likely to represent adaptation of swede flowering to longer days. Data on transcription or protein levels of those genes in B. napus are not available, so no conclusion on subfunctionalization or mode of action can be drawn so far (Figure 3).
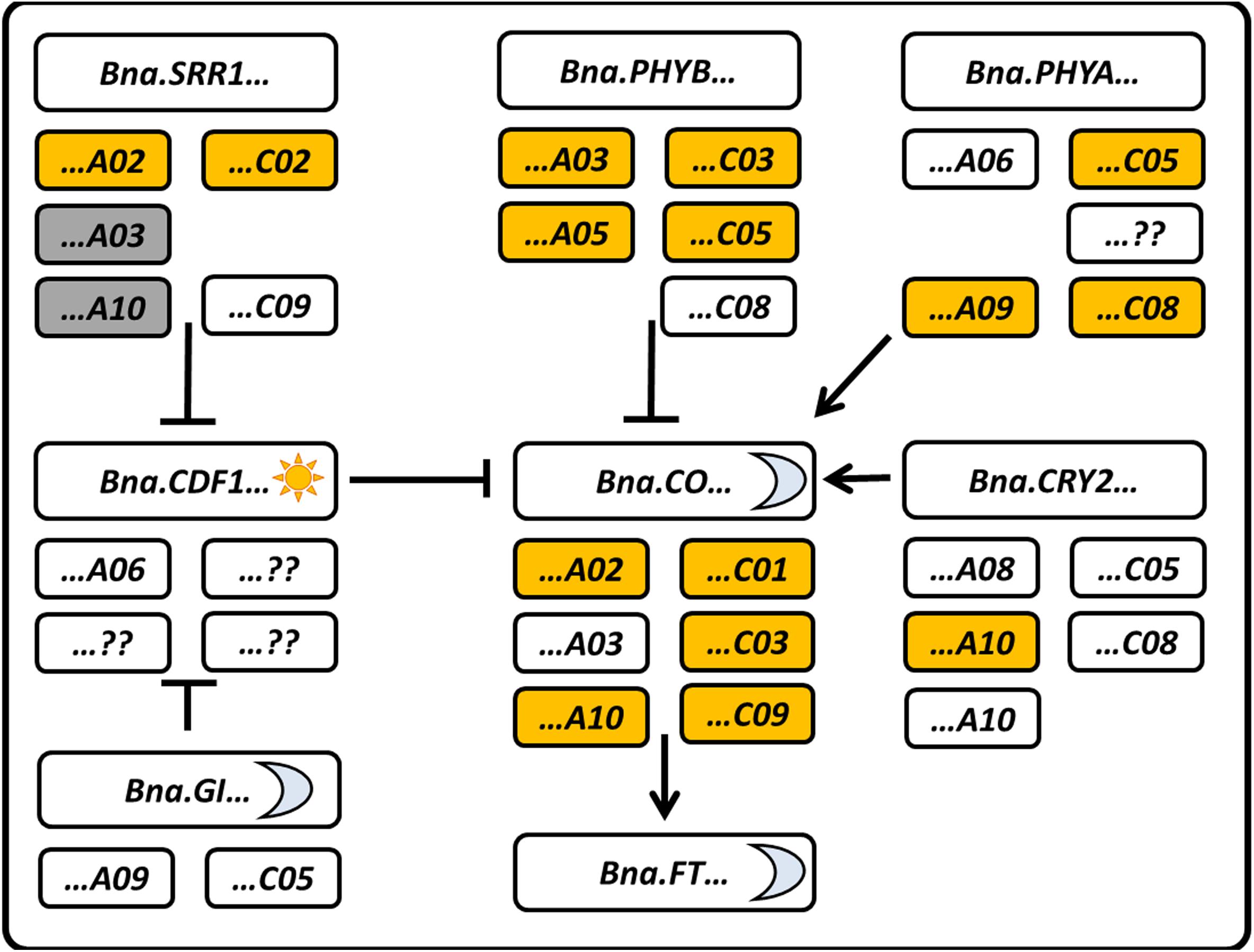
Figure 3. Schematic representation of selected genes from the photoperiodic pathway with their locations in B. napus. Gray boxes indicate pseudogenes, yellow boxes indicate this copy has been named as a candidate in a GWAS, QTL, or selective sweep analysis. Thy sun symbol marks a gene which peaks in the morning, while the moon indicates it peaks in the evening as inferred from A. thaliana. Arrows and blunt end indicate activation and inhibition, respectively.
Ambient Temperature: PIF4
In Arabidopsis, FT expression is further gated by binding of PHYTOCHROME INTERACTING FACTOR 4 (PIF4) to the FT promoter, which can only bind in warmer temperatures when the chromatin carries less H2A.Z histone (Wigge, 2013). PIF4 itself is also under transcriptional control of the circadian clock (Wigge, 2013). A copy of Bna.PIF4 was recently implicated in a photoperiod-sensitive flowering time QTL (Raman et al., 2019). In B. rapa, however, it was found that Bra.FT.A02 expression was decreased at warmer temperatures (28°C) compared to normal (21°C), obviously via an increase in H2A.Z in Bra.FT.A02 chromatin, resulting in later flowering (Del Olmo et al., 2019). Spring rapeseed growing at 18°C/8°C day/night cycles still flowered slightly later than plants at constant 20°C at the same photoperiod (16 h) (Rahman et al., 2018), so there seems to be an optimum temperature above 20°C and well below 28°C. Moreover, this indicates that temperature regulation in Brassica is different from what we observe in A. thaliana, and respective studies need to be carried out to dissect this trait in B. napus.
Gibberellins, Age, and Stress: SPL and DELLA
Two pathways regulate flowering time in absence of inductive conditions: the gibberellin (GA) pathway and the miR156/SPL module (Yu et al., 2012). GAs are negative regulators of DELLA proteins, which are interacting with SPLs, so there is interaction between both pathways (Yu et al., 2012). The miR156/SPL module is known as the age pathway, which is mediated via several highly conserved micro RNAs (miRNAs) like miR156 and miR172 (Wang J.-W. et al., 2009; Wang, 2014). miR156 is a negative post-transcriptional regulator of many different SQUAMOSA PROMOTER LIKE (SPL) genes (Wang J.-W. et al., 2009). In seedlings, miR156 levels are high, but steadily decrease with increasing plant age, and SPL repression is more and more released (Wang J.-W. et al., 2009; Wang, 2014). SPL9 induces expression of miR172, which in turn shuts off negative flowering regulators, while SPL3 directly activates downstream flowering time genes like LFY (Wang J.-W. et al., 2009; Yamaguchi et al., 2009).
In B. napus, two different GA synthesis enzymes have been found in QTL regions for flowering time: GA-2-ox-1 (Raman et al., 2013; Jian et al., 2019) and GA-20-ox (Schiessl et al., 2015), along with the DELLA protein RGA1 (Li et al., 2018; Jian et al., 2019). While miRNAs were not considered or reported in any QTL region in B. napus, majorly due to the lack of suitable miRNA gene annotation, one study reports Bna.SPL3.A03 to be located in a QTL region in spring material (Raman et al., 2016), while another claims the same copy to be a pseudogene (Schiessl et al., 2017b). Copies of DELLA proteins (A09, C09), Bna.GA-3-ox.A06 and Bna.SPL3.A05 were also found in selective sweeps between swede and non-swede material (Schiessl et al., 2017b). Bna.SPL has six copies in B. napus, of which one is a pseudogene, while all others carry some type of variation (Schiessl et al., 2017b). The relative lack of respective gene copies in QTLs might reflect the fact that most flowering time QTL studies take place under inductive conditions.
Interestingly, however, most flowering time genes affected under drought stress in B. napus were found to belong to the gibberellin and age pathway, which indicates that stress signaling might take this route to regulate flowering in response to abiotic stress (Schiessl et al., 2020). Candidates to mediate this response are, among others, miR156s, which were found to be differentially expressed under drought stress, possibly in reaction to altered sugar level under photosynthetic limitations (Schiessl et al., 2020). However, data from this study also confirmed the high complexity of those interactions in B. napus, as different copies of the same gene seemed to react differentially to the same miRNA level (Schiessl et al., 2020). This points to regulatory co-evolution of specific miRNA-gene pairs and stresses the strong need to perform studies on gene copy level in B. napus – inferring gene function from A. thaliana is a good start, but does not provide enough information on breeding targets.
Discussion
Although much progress has been achieved to shed light on flowering time regulation in rapeseed, we still lack answers to important questions in regards to the specific situation in this polyploid oil crop. Major genetic effects on traits like vernalization dependency have been characterized down to gene copy level and revealed considerable subfunctionalization. Other pathways, however, like photoperiod and temperature signaling, still remain largely obscure in this respect. Moreover, the few studies existing worked mainly with spring type rapeseed, and data on the same traits in semi-winter and winter rapeseed are scarce. More and more targeted studies will be necessary to provide reliable data for breeding programs, under consideration of cross-pathway effects, the influence of the circadian clock, the genetic background and epigenetic regulation. In the light of shifting climate zones, influences by day length, ambient temperature, and drought stress need more attention. While the close relationship to the model was and is very helpful for hypothesis development, the current review shows clearly that knowledge cannot be directly inferred from the model to the crop, making functional genetic studies in crops unreplaceable. On top of providing invaluable information for breeding programs, such data will also improve our understanding of post-polyploidization adaptation as an evolutionary principle.
Author Contributions
SS conceptualized and wrote the manuscript.
Funding
SS is currently funded by the Deutsche Forschungsgemeinschaft (DFG), grant number SCHI 1296/2-1.
Conflict of Interest
The authors declare that the research was conducted in the absence of any commercial or financial relationships that could be construed as a potential conflict of interest.
Acknowledgments
The author like to thank Annaliese Mason for English language revisions.
References
Adamidis, G. C., Cartar, R. V., Melathopoulos, A. P., Pernal, S. F., and Hoover, S. E. (2019). Pollinators enhance crop yield and shorten the growing season by modulating plant functional characteristics: a comparison of 23 canola varieties. Sci. Rep. 9:14208. doi: 10.1038/s41598-019-50811-y
Bender, M. (1999). Economic feasibility review for community-scale farmer cooperatives for biodiesel. Bioresour. Technol. 70, 81–87. doi: 10.1016/s0960-8524(99)00009-7
Berry, P. M., Spink, J., Foulkes, M. J., and White, P. J. (2010). The physiological basis of genotypic differences in nitrogen use efficiency in oilseed rape (Brassica napus L.). Field Crops Res. 119, 365–373. doi: 10.1016/j.fcr.2010.08.004
Blümel, M., Dally, N., and Jung, C. (2015). Flowering time regulation in crops-what did we learn from Arabidopsis? Curr. Opin. Biotechnol. 32, 121–129. doi: 10.1016/j.copbio.2014.11.023
Bommarco, R., Marini, L., and Vaissière, B. E. (2012). Insect pollination enhances seed yield, quality, and market value in oilseed rape. Oecologia 169, 1025–1032. doi: 10.1007/s00442-012-2271-6
Borghi, M., Perez de Souza, L., Yoshida, T., and Fernie, A. R. (2019). Flowers and climate change: a metabolic perspective. New Phytol. 224, 1425–1441. doi: 10.1111/nph.16031
Bušić, A., Kundas, S., Morzak, G., Belskaya, H., Marđetko, N., Ivančić Šantek, M., et al. (2018). Recent trends in biodiesel and biogas production. Food Technol. Biotechnol. 56, 152–173. doi: 10.17113/ftb.56.02.18.5547
Carlsson, A. S., Yilmaz, J. L., Green, A. G., Stymne, S., and Hofvander, P. (2011). Replacing fossil oil with fresh oil - with what and for what? Eur. J. Lipid Sci. Technol. 113, 812–831. doi: 10.1002/ejlt.201100032
Cheng, J.-Z., Zhou, Y.-P., Lv, T.-X., Xie, C.-P., and Tian, C.-E. (2017). Research progress on the autonomous flowering time pathway in Arabidopsis. Physiol. Mol. Biol. Plants 23, 477–485. doi: 10.1007/s12298-017-0458-3
Choi, K., Kim, J., Hwang, H.-J., Kim, S., Park, C., Kim, S. Y., et al. (2011). The FRIGIDA complex activates transcription of FLC, a strong flowering repressor in Arabidopsis, by recruiting chromatin modification factors. Plant Cell 23, 289–303. doi: 10.1105/tpc.110.075911
Del Olmo, I., Poza-Viejo, L., Piñeiro, M., Jarillo, J. A., and Crevillén, P. (2019). High ambient temperature leads to reduced FT expression and delayed flowering in Brassica rapa via a mechanism associated with H2A.Z dynamics. Plant J. 100, 343–356. doi: 10.1111/tpj.14446
Diepenbrock, W. (2000). Yield analysis of winter oilseed rape (Brassica napus L.): a review. Field Crops Res. 67, 35–49. doi: 10.1016/s0378-4290(00)00082-4
Fletcher, R. S., Mullen, J. L., Heiliger, A., and McKay, J. K. (2014). QTL analysis of root morphology, flowering time, and yield reveals trade-offs in response to drought in Brassica napus. J. Exp. Bot. 66, 245–256. doi: 10.1093/jxb/eru423
Ghosh, S., Watson, A., Gonzalez-Navarro, O. E., Ramirez-Gonzalez, R. H., Yanes, L., Mendoza-Suárez, M., et al. (2018). Speed breeding in growth chambers and glasshouses for crop breeding and model plant research. Nat. Protoc. 13, 2944–2963. doi: 10.1038/s41596-018-0072-z
Guo, Y., Hans, H., Christian, J., and Molina, C. (2014). Mutations in single FT- and TFL1-paralogs of rapeseed (Brassica napus L.) and their impact on flowering time and yield components. Front. Plant Sci. 5:282. doi: 10.3389/fpls.2014.00282
Hohmann, M. A. (2017). Untersuchung von Anzuchtsystemen und Selektionstechniken zur Züchtung von Trockenstressresistenz in Winterraps (Brassica napus L.): Zur Erlangung des akademischen Grades Doctor agriculturae. Ph.D. Dissertation, University of Giessen, Gießen.
Hou, J., Long, Y., Raman, H., Zou, X., Wang, J., Dai, S., et al. (2012). A tourist-like MITE insertion in the upstream region of the BnFLC.A10 gene is associated with vernalization requirement in rapeseed (Brassica napus L.). BMC Plant Biol. 12:238. doi: 10.1186/1471-2229-12-238
IPCC (2019). Climate Change and Land: An IPCC Special Report on Climate Change, Desertification, Land Degradation, Sustainable Land Management, Food Security, and Greenhouse gas Fluxes in Terrestrial Ecosystems. Technical Summary. Geneva: IPCC.
Jian, H., Zhang, A., Ma, J., Wang, T., Yang, B., Shuang, L. S., et al. (2019). Joint QTL mapping and transcriptome sequencing analysis reveal candidate flowering time genes in Brassica napus L. BMC Genomics 20:21. doi: 10.1186/s12864-018-5356-8
Jiang, D., Gu, X., and He, Y. (2009). Establishment of the winter-annual growth habit via FRIGIDA-mediated histone methylation at FLOWERING LOCUS C in Arabidopsis. Plant Cell Online 21, 1733–1746. doi: 10.1105/tpc.109.067967
Johansson, M., and Staiger, D. (2014). SRR1 is essential to repress flowering in non-inductive conditions in Arabidopsis thaliana. J. Exp. Bot. 65, 5811–5822. doi: 10.1093/jxb/eru317
Johansson, M., and Staiger, D. (2015). Time to flower: interplay between photoperiod and the circadian clock. J. Exp. Bot. 66, 719–730. doi: 10.1093/jxb/eru441
Jung, C., and Muller, A. E. (2009). Flowering time control and applications in plant breeding. Trends Plant Sci. 14, 563–573. doi: 10.1016/j.tplants.2009.07.005
Kittipol, V., He, Z., Wang, L., Doheny-Adams, T., Langer, S., and Bancroft, I. (2019). Genetic architecture of glucosinolate variation in Brassica napus. J. Plant Physiol. 240:152988. doi: 10.1016/j.jplph.2019.06.001
Li, B., Zhao, W., Li, D., Chao, H., Zhao, X., Ta, N., et al. (2018). Genetic dissection of the mechanism of flowering time based on an environmentally stable and specific QTL in Brassica napus. Plant Sci. 277, 296–310. doi: 10.1016/j.plantsci.2018.10.005
Lu, J., Carbone, G. J., and Grego, J. M. (2019). Uncertainty and hotspots in 21st century projections of agricultural drought from CMIP5 models. Sci. Rep. 9:157. doi: 10.1038/s41598-019-41196-z
Luo, T., Zhang, J., Khan, M. N., Liu, J., Xu, Z., and Hu, L. (2018). Temperature variation caused by sowing dates significantly affects floral initiation and floral bud differentiation processes in rapeseed (Brassica napus L.). Plant Sci. 271, 40–51. doi: 10.1016/j.plantsci.2018.03.004
Luo, Y. X., Luo, C. Y., Du, D. Z., Fu, Z., Yao, Y. M., Xu, C. C., et al. (2014). Quantitative trait analysis of flowering time in spring rapeseed (B. napus L.). Euphytica 200, 321–335. doi: 10.1007/s10681-014-1140-2
Morinaga, T. (1934). Interspecific hybridization in Brassica. Cytologia 6, 62–67. doi: 10.1508/cytologia.6.62
Nagaharu, U. (1935). Genome analysis in Brassica with special reference to the experimental formation of B. napus and peculiar mode of fertilisation. Jpn. J. Bot. 7, 389–452.
Nelson, M. N., Rajasekaran, R., Smith, A., Chen, S., Beeck, C. P., Siddique, K. H. M., et al. (2014). Quantitative trait loci for thermal time to flowering and photoperiod responsiveness discovered in summer annual-type Brassica napus L. PLoS One 9:e102611. doi: 10.1371/journal.pone.0102611
Osborn, T. C., Kole, C., Parkin, I. A. P., Sharpe, A. G., Kuiper, M., Lydiate, D. J., et al. (1997). Comparison of flowering time genes in Brassica rapa. B. napus and Arabidopsis thaliana. Genetics 146, 1123–1129.
Parkin, I. A. P., Clarke, W. E., Sidebottom, C., Zhang, W., Robinson, S. J., Links, M. G., et al. (2010). Towards unambiguous transcript mapping in the allotetraploid Brassica napus. Genome 25, 2013.
Quijada, P. A., Udall, J. A., Lambert, B., and Osborn, T. C. (2006). Quantitative trait analysis of seed yield and other complex traits in hybrid spring rapeseed (Brassica napus L.): 1. Identification of genomic regions from winter germplasm. Theor. Appl. Genet. 113, 549–561. doi: 10.1007/s00122-006-0323-1
Rahman, H., Bennett, R. A., and Kebede, B. (2018). Molecular mapping of QTL alleles of Brassica oleracea affecting days to flowering and photosensitivity in spring Brassica napus. PLoS One 13:e0189723. doi: 10.1371/journal.pone.0189723
Raman, H., Dalton-Morgan, J., Diffey, S., Raman, R., Alamery, S., Edwards, D., et al. (2014). SNP markers-based map construction and genome-wide linkage analysis in Brassica napus. Plant Biotechnol. J. 12, 851–860. doi: 10.1111/pbi.12186
Raman, H., Raman, R., Coombes, N., Song, J., Prangnell, R., Bandaranayake, C., et al. (2016). Genome-wide association analyses reveal complex genetic architecture underlying natural variation for flowering time in canola. Plant Cell Environ. 39, 1228–1239. doi: 10.1111/pce.12644
Raman, H., Raman, R., Eckermann, P., Coombes, N., Manoli, S., Zou, X., et al. (2013). Genetic and physical mapping of flowering time loci in canola (Brassica napus L.). Theor. Appl. Genet. 126, 119–132. doi: 10.1007/s00122-012-1966-8
Raman, H., Raman, R., Qiu, Y., Yadav, A. S., Sureshkumar, S., Borg, L., et al. (2019). GWAS hints at pleiotropic roles for FLOWERING LOCUS T in flowering time and yield-related traits in canola. BMC Genomics 20:636. doi: 10.1186/s12864-019-5964-y
Ridge, S., Brown, P. H., Hecht, V., Driessen, R. G., and Weller, J. L. (2015). The role of BoFLC2 in cauliflower (Brassica oleracea var. botrytis L.) reproductive development. Exp. Bot. J. 66, 125–135. doi: 10.1093/jxb/eru408
Robert, L. S., Robson, F., Sharpe, A., Lydiate, D., and Coupland, G. (1998). Conserved structure and function of the Arabidopsis flowering time gene CONSTANS in Brassica napus. Plant Mol. Biol. 37, 763–772.
Schiessl, S., Huettel, B., Kuehn, D., Reinhardt, R., and Snowdon, R. J. (2017a). Targeted deep sequencing of flowering regulators in Brassica napus reveals extensive copy number variation. Sci. Data 4:170013. doi: 10.1038/sdata.2017.13
Schiessl, S., Hüttel, B., Kuehn, D., Reinhardt, R., and Snowdon, R. J. (2017b). Post-polyploidisation morphotype diversification associates with gene copy number variation. Sci. Rep. 7:41845. doi: 10.1038/srep41845
Schiessl, S., Iniguez-Luy, F., Qian, W., and Snowdon, R. J. (2015). Diverse regulatory factors associate with flowering time and yield responses in winter-type Brassica napus. BMC Genomics 16:737. doi: 10.1186/s12864-015-1950-1
Schiessl, S., Quezada-Martinez, D., Tebartz, E., Snowdon, R. J., and Qian, L. (2019a). The vernalisation regulator FLOWERING LOCUS C is differentially expressed in biennial and annual Brassica napus. Sci. Rep. 9:14911. doi: 10.1038/s41598-019-51212-x
Schiessl, S., Samans, B., Hüttel, B., Reinhardt, R., and Snowdon, R. J. (2014). Capturing sequence variation among flowering-time regulatory gene homologs in the allopolyploid crop species Brassica napus. Front. Plant Sci. 5:404. doi: 10.3389/fpls.2014.00404
Schiessl, S., Williams, N., Specht, P., Staiger, D., and Johansson, M. (2019b). Different copies of sensitivity to red light reduced 1 show strong subfunctionalization in Brassica napus. BMC Plant Biol. 19:372. doi: 10.1186/s12870-019-1973-x
Schiessl, S. V., and Mason, A. S. (2020). “Ancient and recent polyploid evolution in Brassica,” in Brassica Improvement, Vol. 120, eds S. Wani, A. Thakur, and K. Y. Jeshima (Cham: Springer), 49–66. doi: 10.1007/978-3-030-34694-2_3
Schiessl, S. V., Quezada-Martinez, D., Orantes-Bonilla, M., and Snowdon, R. J. (2020). Transcriptomics reveal high regulatory diversity of drought tolerance strategies in a biennial oil crop. Plant Sci. 297:110515. doi: 10.1016/j.plantsci.2020.110515
Shah, S., Weinholdt, C., Jedrusik, N., Molina, C., Zou, J., Große, I., et al. (2018). Whole-transcriptome analysis reveals genetic factors underlying flowering time regulation in rapeseed (Brassica napus L.). Plant Cell Environ. 41, 1935–1947. doi: 10.1111/pce.13353
Shi, J., Li, R., Qiu, D., Jiang, C., Long, Y., Morgan, C., et al. (2009). Unraveling the complex trait of crop yield with quantitative trait loci mapping in Brassica napus. Genetics 182, 851–861. doi: 10.1534/genetics.109.101642
Snowdon, R. J., and Iniguez Luy, F. L. (2012). Potential to improve oilseed rape and canola breeding in the genomics era. Plant Breed. 131, 351–360. doi: 10.1111/j.1439-0523.2012.01976.x
Sri, T., Gupta, B., Tyagi, S., and Singh, A. (2020). Homeologs of Brassica SOC1, a central regulator of flowering time, are differentially regulated due to partitioning of evolutionarily conserved transcription factor binding sites in promoters. Mol. Phylogenet. Evol. 147, 106777. doi: 10.1016/j.ympev.2020.106777
Srikanth, A., and Schmid, M. (2011). Regulation of flowering time: all roads lead to Rome. Cell. Mol. Life Sci. 68, 2013–2037. doi: 10.1007/s00018-011-0673-y
Tadege, M., Sheldon, C. C., Helliwell, C. A., Stoutjesdijk, P., Dennis, E. S., and Peacock, W. J. (2001). Control of flowering time by FLC orthologues in Brassica napus. Plant J. 28, 545–553. doi: 10.1046/j.1365-313x.2001.01182.x
Tudor, E. H., Jones, D. M., He, Z., Bancroft, I., Trick, M., Wells, R., et al. (2020). QTL-seq identifies BnaFT.A02 and BnaFLC.A02 as candidates for variation in vernalisation requirement and response in winter oilseed rape (Brassica napus). Plant Biotechnol. J. 1–16. doi: 10.1111/pbi.13421
Udall, J. A., Quijada, P. A., Lambert, B., and Osborn, T. C. (2006). Quantitative trait analysis of seed yield and other complex traits in hybrid spring rapeseed (Brassica napus L.): 2. Identification of alleles from unadapted germplasm. Theor. Appl. Genet. 113, 597–609. doi: 10.1007/s00122-006-0324-0
Wang, J., Hopkins, C. J., Hou, J., Zou, X., Wang, C., Long, Y., et al. (2012). Promoter variation and transcript divergence in brassicaceae lineages of FLOWERING LOCUS T. PLoS One 7:e47127. doi: 10.1371/journal.pone.0047127
Wang, J., Long, Y., Wu, B., Liu, J., Jiang, C., Shi, L., et al. (2009). The evolution of Brassica napus FLOWERING LOCUST paralogues in the context of inverted chromosomal duplication blocks. BMC Evol. Biol. 9:271. doi: 10.1186/1471-2148-9-271
Wang, J.-W. (2014). Regulation of flowering time by the miR156-mediated age pathway. J. Exp. Bot. 65, 4723–4730. doi: 10.1093/jxb/eru246
Wang, J.-W., Czech, B., and Weigel, D. (2009). miR156-regulated SPL transcription factors define an endogenous flowering pathway in Arabidopsis thaliana. Cell 138, 738–749. doi: 10.1016/j.cell.2009.06.014
Wang, N., Qian, W., Suppanz, I., Wei, L., Mao, B., Long, Y., et al. (2011). Flowering time variation in oilseed rape (Brassica napus L.) is associated with allelic variation in the FRIGIDA homologue BnaA.FRI.a. J. Exp. Bot. 62, 5641–5658. doi: 10.1093/jxb/err249
Watson, A., Ghosh, S., Williams, M. J., Cuddy, W. S., Simmonds, J., Rey, M.-D., et al. (2018). Speed breeding is a powerful tool to accelerate crop research and breeding. Nat. Plants 4, 23–29. doi: 10.1038/s41477-017-0083-8
Wigge, P. A. (2013). Ambient temperature signalling in plants. Curr. Opin. Plant Biol. 16, 661–666. doi: 10.1016/j.pbi.2013.08.004
Wu, D., Liang, Z., Yan, T., Xu, Y., Xuan, L., Tang, J., et al. (2018). Whole-genome resequencing of a world-wide collection of rapeseed accessions reveals genetic basis of their ecotype divergence. Mol. Plant 12, 30–43. doi: 10.1016/j.molp.2018.11.007
Xu, L., Hu, K., Zhang, Z., Guan, C., Chen, S., Hua, W., et al. (2016). Genome-wide association study reveals the genetic architecture of flowering time in rapeseed (Brassica napus L.). DNA Res. 23, 43–52. doi: 10.1093/dnares/dsv035
Yamaguchi, A., Wu, M. F., Yang, L., Wu, G., Poethig, R. S., and Wagner, D. (2009). The MicroRNA-regulated SBP-Box transcription factor SPL3 is a direct upstream activator of leafy, fruitfull, and apetala1. Dev. Cell 17, 268–278. doi: 10.1016/j.devcel.2009.06.007
Yin, S., Wan, M., Guo, C., Wang, B., Li, H., Li, G., et al. (2020). Transposon Insertions within alleles of BnaFLC.A10 and BnaFLC.A2 are associated with rapeseed (Brassica napus L.) seasonal crop-type. J. Exp. Bot. 71, 4729–4741. doi: 10.1093/jxb/eraa237
Yu, S., Galvão, V. C., Zhang, Y.-C., Horrer, D., Zhang, T.-Q., Hao, Y.-H., et al. (2012). Gibberellin regulates the Arabidopsis floral transition through miR156-targeted SQUAMOSA promoter binding-like transcription factors. Plant Cell 24, 3320–3332. doi: 10.1105/tpc.112.101014
Zhou, Y. T., Wang, H. Y., Zhou, L., Wang, M. P., Li, H. P., Wang, M. L., et al. (2007). Analyses of the floral organ morphogenesis and the differentially expressed genes of an apetalous flower mutant in Brassica napus. Plant Cell Rep. 27, 9–20. doi: 10.1007/s00299-007-0426-4
Zou, X., Suppanz, I., Raman, H., Hou, J., Wang, J., Long, Y., et al. (2012). Comparative analysis of FLC homologues in brassicaceae provides insight into their role in the evolution of oilseed rape. PLoS One 7:e45751. doi: 10.1371/journal.pone.0045751
Keywords: polyploidy, gene evolution, subfunctionalization, flowering time, canola, oilseed rape
Citation: Schiessl S (2020) Regulation and Subfunctionalization of Flowering Time Genes in the Allotetraploid Oil Crop Brassica napus. Front. Plant Sci. 11:605155. doi: 10.3389/fpls.2020.605155
Received: 11 September 2020; Accepted: 29 October 2020;
Published: 20 November 2020.
Edited by:
Elizabeth Dennis, Commonwealth Scientific and Industrial Research Organisation (CSIRO), AustraliaReviewed by:
Ryo Fujimoto, Kobe University, JapanChris Helliwell, Commonwealth Scientific and Industrial Research Organisation (CSIRO), Australia
Copyright © 2020 Schiessl. This is an open-access article distributed under the terms of the Creative Commons Attribution License (CC BY). The use, distribution or reproduction in other forums is permitted, provided the original author(s) and the copyright owner(s) are credited and that the original publication in this journal is cited, in accordance with accepted academic practice. No use, distribution or reproduction is permitted which does not comply with these terms.
*Correspondence: Sarah Schiessl, sarah-veronica.schiessl@agrar.uni-giessen.de