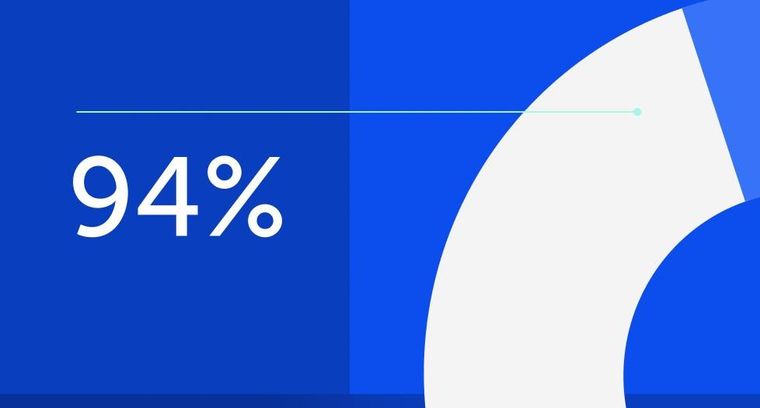
94% of researchers rate our articles as excellent or good
Learn more about the work of our research integrity team to safeguard the quality of each article we publish.
Find out more
ORIGINAL RESEARCH article
Front. Plant Sci., 11 December 2020
Sec. Plant Biotechnology
Volume 11 - 2020 | https://doi.org/10.3389/fpls.2020.602680
Precise expression of a transgene in the desired manner is important for plant genetic engineering and gene function deciphering, but it is a challenge to obtain specific transgene expression free from the interference of the constitutive promoters used to express the selectable marker gene, such as the Cauliflower mosaic virus (CaMV) 35S promoter. So, the solutions to avoid these inappropriate regulations are largely demanded. In this study, we report the characterization of a callus strong promoter (CSP1) in rice and its application for accurate transgene expression. Our results indicate that the high expression of the CSP1 promoter in the callus enables efficient selection of hygromycin equivalent to that provided by the CaMV 35S promoter, whereas its expression in other tissues is low. To evaluate possible leaky effects, the expression of a β-glucuronidase reporter driven by six specific promoters involving hormone signaling, pathogen response, cell fate determination, and proliferation was observed in transgenic rice plants generated by CSP1-mediated selection. Distinct β-glucuronidase expression was found consistently in most of the transgenic lines obtained for each promoter. In addition, we applied these specific marker lines to investigate the root cellular responses to exogenous cytokinin and auxin treatment. The results reveal that the root growth inhibition by cytokinin was differently regulated at high and low concentrations. In summary, we have established the feasibility of using callus-specific promoter-dependent selection to mitigate the transgene misexpression in rice. By enabling efficient transformation, rice plants with reliable transgene expression will be easily acquired for broad applications.
Genetic transformation is a valuable method to regulate agronomically important traits both for molecular breeding and for deciphering gene function (Roy et al., 2000). The expression level and specificity of the introduced transgene are largely dependent on the promoters used in the expression cassettes. Tissue-specific and condition-dependent promoters are highly preferred over constitutive promoters because they provide better gene expression and product accumulation while notably alleviating negative effects on plant growth. Numerous tissue-specific promoters have, therefore, been characterized and widely utilized (Potenza et al., 2004; Biłas et al., 2016). Striking examples include placing bioactive genes under the control of endosperm-specific promoters (Gt13a or Gt1) to produce plant-made pharmaceuticals (Ning et al., 2008; He et al., 2011) or to accumulate β-carotene in Golden Rice seeds (Paine et al., 2005). The cytokinin dehydrogenase gene (OsCKX4) controlled by the root-specific promoter (RCc3) can promote root development without shoot growth defects (Gao et al., 2014). Recently, the rice embryogenic initiation gene (BBM1) was found to trigger parthenogenesis when it was ectopically expressed under the control of the Arabidopsis egg-cell-specific promoter (pDD45), which enabled clonal seeds to be formed through asexual propagation (Khanday et al., 2019).
On the other hand, to enable efficient selection of transgenic plants, strong promoters are frequently used to ensure abundant transcription of the selectable marker genes. The 35S promoter from Cauliflower mosaic virus (CaMV) is one of the commonly used constitutive promoters to achieve this purpose (Franck et al., 1980). However, increasing evidence has shown that the 35S promoter used for selection affects the expression pattern of the transgene, possibly because of interactions with the enhancer sequence in the 35S promoter (Yoo et al., 2005). For example, a tapetum-specific promoter (TA29) was used to conditionally express two ribonuclease genes and induce male sterility in transgenic Brassica napus and tobacco plants (Mariani et al., 1990). However, it was observed later that the CaMV 35S or its double-enhancer variant used to express the marker gene caused the leaky expression of the cytotoxic gene, hindering the efficient production of stable male sterile plants (Jagannath et al., 2001). Using a spacer DNA fragment between the tapetum-specific promoter and the CaMV 35S promoter significantly improved the recovery of viable male sterile lines (Jagannath et al., 2001). However, this approach is not widely used because the spacers needed to block the interaction differ for each enhancer/promoter combination and so need to be determined case by case (Gudynaite-Savitch et al., 2009).
Several other strategies have been proposed to prevent unintended interactions between the promoters used for selection and transgene expression (Gudynaite-Savitch et al., 2009; Zhou et al., 2014). Clearly, the simple and practical strategy would be to use an alternative promoter that has no/low misexpression effect. The promoters derived from Agrobacterium tumefaciens, such as nopaline synthase (nos) (Kim et al., 1993), can reduce the ectopic expression of transgenes in some cases (Jagannath et al., 2001; Yoo et al., 2005; Gudynaite-Savitch et al., 2009), but the interference between the nos promoter and the target gene promoter often remains considerable especially when they are placed in head-to-head orientation (Denis et al., 1993; Ponstein et al., 2002; Gudynaite-Savitch et al., 2009; Zhou et al., 2014). Among the plant-derived promoters, the tobacco cryptic promoter (tCUP) has proved to be a promising substitute for selectable marker gene expression. It can maintain the appropriate expression of some seed-specific promoters in Arabidopsis regardless of the T-DNA configuration and the distance between the two promoters (Gudynaite-Savitch et al., 2009). The tCUP-derived promoter (tCUP1) also has a conserved property in rice for distinctive expression of DR5:GUS in the root apical meristem (Zhou et al., 2014). Although the tCUP promoter and its enhanced versions are strongly expressed in many plant species and tissues, with activities exceeding that of the 35S promoter (Malik et al., 2002; Tian et al., 2003), its activity proved too weak to drive vigorous growth of resistant calli in rice (Zhou et al., 2013). This prompted us to look for other cis-regulatory sequences to confer a strong expression of the selectable marker gene but with a low leaky effect on the specificity of the transgene.
Because the expression of the selectable marker gene is unnecessary once the transformed plants are acquired, the replacement of a strong constitutive promoter by one that is active only during the selection stage is a better choice to eliminate the interference from selectable markers on the expression of the target transgenes. Embryo differentiated callus is the most preferred explant for efficient and convenient rice transformation, and therefore, callus-specific promoters are largely required for marker gene selection, and some of these have been characterized. The β-glucanase 9 (Gns9) gene promoter was found to be active only in rice calli and has been used to express a selectable marker gene and obtain transformed plants without the accumulation of antibiotic-resistant protein in other tissues, especially in rice seeds (Huang et al., 2001). A callus-specific promoter from rice β-cysteine protease (CP) gene was also used to drive codon-optimized hygromycin phosphotransferase (HPT) gene expression for efficient rice transformation (Wang et al., 2012), but it was less effective to drive the HPT gene without optimization. These experiments proved that callus-specific promoters could lead to successful transformation and avoid the dispersal of the antibiotic-resistant protein, but their possible interference with transgene expression was not studied.
In the work described here, we identified a callus strong promoter (CSP1) by searching a gene expression microarray database. The CSP1 promoter activity was investigated in rice by fusion to both the β-glucuronidase (GUS) reporter and the HPT selectable marker. This resulted in strong GUS expression preferentially in callus and provided equivalent levels of a selection of hygromycin as compared with the 35S promoter. Used as an effective selectable marker promoter in rice, the influence of CSP1 on the tissue-specific promoters was evaluated using GUS as the reporter gene. GUS expression of six synthetic or native promoters was carefully observed in shoots and roots of transgenic plants, including the quiescent-center-specific promoter QHB, the cell cycle protein cyclin B1 promoter CYCB1, the auxin-inducible promoter DR5, the cytokinin two-component signaling sensor TCSn, the promoters of cytokinin responsive A-type response regulator OsRR6, and the pathogenesis-related gene PR1b. All these promoters were specifically expressed in most of the lines as expected, including the responsiveness to cytokinin and auxin applications, which confirmed the root development change controlled by these hormones. Thus, CSP1-mediated selection provides a robust and reliable tool to target transgene expression in a tissue-specific and condition-dependent manner in rice.
Use the Anatomy tool from the GENE SEARCH toolset of GENEVESTIGATOR1 and chose callus as a target. From the selected Affymetrix Rice Genome Array dataset, 10 distinct genes meeting the criteria were identified (Supplementary Figure 1). Then, using the Anatomy tool from the CONDITION SEARCH toolset to compare the target gene expression level in different tissues, the gene LOC_Os10g14020 was identified to be better than the other nine genes (Supplementary Figure 2). Use the Perturbation tool from the CONDITION SEARCH toolset to analyze the response to various stimuli.
To construct the CSP1:GUS expression vector, the CSP1 promoter was amplified with polymerase chain reaction (PCR) primers CSP1-Pr-F1 and CSP1-Pr-R1 from the 5′ upstream regulatory sequence of the gene LOC_Os10g14020. A 1,996-bp PCR fragment before the translation start codon (Supplementary Sequence 1) was cloned into the pMD19-T vector (Takara, Dalian, China) and then digested by SacI and KpnI for insertion into the GUS reporter vector tCUP1-HPT-T35S-GUS-Tnos (a3) at the same sites (Zhou et al., 2014). The position of cis-elements in the CSP1 promoter sequence was predicted by the PlantCARE program (Lescot et al., 2002).
The generation of new GUS reporter vectors relied on CSP1-activated HPT expression. The CSP1 promoter was amplified with primers CSP1-Pr-F2 and CSP1-Pr-R2 (attached to AscI) to replace the CaMV 35S promoter in the a1 vector (Zhou et al., 2014). The resulting vector was named CSP1-HPT-GUS (Figure 2A and Supplementary Excel 1) and rCSP1-HPT-GUS (Supplementary Figure 4A) with forward and reverse CSP1 insertion, respectively. Meanwhile, the CSP1 promoter was amplified again by primers CSP1-Pr-FW and CSP1-Pr-RV to replace tCUP1 by In-Fusion HD Cloning (Clontech Laboratories, Inc., CA, United States) into the b3 vector digested with AscI (Zhou et al., 2014). The resulting vector was named HPT-CSP1-GUS.
Six specific promoters were selected for the GUS expression assay in the CSP1-HPT-GUS vector. The DR5:GUS vector was obtained by replacing the tCUP1 promoter in the a3-DR5 vector (Zhou et al., 2014) with the AscI-digested CSP1 promoter.
For the QHB:GUS vector, a fragment containing the promoter (−1,495 +821) of the QHB gene (LOC_Os01g63510) was amplified from the QHB-GUS-101.3 vector (Jun et al., 2011) by using primers QHB-Pr-F and QHB-Pr-R. After cloning into the pMD19-T vector, it was released by SalI (from pMD19-T) and KpnI (added to the primer) and inserted to the same sites of the CSP1-HPT-GUS vector without in-frame fusion with the GUS reporter gene.
The CYCB1;1:GUS vector was constructed as described previously (Chen et al., 2013). A translational fusion of the 2,317-bp fragment upstream of the OsCYCB1;1 (LOC_Os01g59120) start codon plus a 912-bp fragment of the ORF starting at the ATG start site was amplified with the primers CYCB1;1-Pr-F and CYCB1;1-Pr-R. The 3,229-bp PCR product was re-amplified using primers CYCB1;1-Pr-FW and CYCB1;1-Pr-RV for In-Fusion HD Cloning into the CSP1-HPT-GUS vector digested with XbaI and SalI.
For the TCSn:GUS vector, the DNA sequence of TCSn1 (Supplementary Sequence 2) was synthesized commercially (Genscript, Nanjing, China) according to the vector sequence of TCSn1:GFP-ER (Zurcher et al., 2013). A PstI-digested fragment with mini 35S promoter and TMVΩ translation enhancer (Supplementary Sequence 3) was inserted to the end of TCSn1 to form a functional TCSn promoter. This was then digested by SalI and KpnI for insertion into the CSP1-HPT-GUS vector at the same sites.
For the RR6:GUS vector, a 2,811-bp PCR fragment upstream of the translation start codon of the cytokinin type A response regulator OsRR6 (LOC_Os04g57720) was amplified using the primers RR6-Pr-F and RR6-Pr-R. After being cloned into the pMD19-T vector, it was digested by XbaI and SalI and inserted into the CSP1-HPT-GUS vector at the same sites.
For the PR1b:GUS vector, a 2,579-bp PCR fragment upstream of the translation start codon of the pathogenesis-related gene PR1b (LOC_Os01g28450) was amplified using the primers PR1b-Pr-FW and PR1b-Pr-RV for In-Fusion Cloning into the CSP1-HPT-GUS vector digested with XbaI and SalI.
All PCR primer sequences are listed in Supplementary Table 1. Vectors used for experiments are listed in Supplementary Table 2.
The binary vectors described earlier were introduced separately into the A. tumefaciens strain EHA105 by electroporation and transformed to embryogenic calli developed from mature seeds of rice (Oryza sativa L. cv. Nipponbare) by the method previously reported (Hiei and Komari, 2008) with modifications; no hygromycin is included in the regeneration medium. For comparison of the two CSP1-based HPT-GUS vectors with their corresponding 35S versions a1 and c1 (Zhou et al., 2014), transformed calli were used to measure the callus growth activities and leaky GUS expression capacities as previously described (Zhou et al., 2013, 2014). Stable transgenic plants were obtained for tissue-specific GUS expression analysis of each promoter tested.
Histochemical analysis of GUS activity was performed as described by Jefferson et al. (1987). Transformed calli after the first round of selection (approximately 20 days) were incubated in X-gluc staining solution at 37°C for 16 h. For the CSP1:GUS transgenic plants, leaf and root samples were taken from 7-day-old (after germination) seedlings of the T1 generation of three independent lines in solution culture. Spikelets before flowering were dissected from T1 plants at the boot stage in the field. Seeds from the T2 generation were de-husked. All these prepared samples were stained with X-gluc solution at 37°C for 16 h. For the six specific promoters constructed in the CSP1-HPT-GUS vector, leaf and root samples from T0 transgenic plants and the 7-day-old seedlings of descendent generations were incubated with X-gluc solution at 37°C for 16 h, except that the root samples from DR5:GUS, TCS:GUS, and CYCB1;1:GUS transgenic plants were incubated for 30 min. The staining solution contained 1.0-mM 5-bromo-4-chloro-3-indolyl-β-D-glucuronide in 0.1-M phosphate buffer (pH 7.0) with 10-mM ethylene diamine tetraacetic acid disodium salt, 1-mM potassium ferricyanide, 1-mM potassium ferrocyanide, 20% (v/v) methyl hydrate, and 0.5% (v/v) Triton X-100.
After GUS staining, photos of callus, leaf segment, floret, and seed were taken through a Nikon SMZ1000 stereomicroscope equipped with a Nikon digital camera DS-Fi1. Samples containing chlorophyll were cleared in 100% ethanol before photography. For root samples, the staining solution was removed with water and infiltrated under vacuum for four to five periods of 30 s (Eppendorf, Concentrator Plus, Mode: D-AQ, 30°C). The water was replaced with enough chloral hydrate/glycerol solution (1.6-g chloral hydrate to 1-ml 20% glycerol) to cover the tissue and cleared for several hours. Cleared samples were mounted in chloral hydrate/glycerol solution under a coverslip and directly viewed with a Nikon Eclipse Ti inverted DIC microscope imaging system. The localization of GUS expression in rice roots was described according to the anatomy model illustrated by Coudert et al. (2010).
Germinated transgenic rice seeds were grown on a plastic net floating in International Rice Research Institute rice culture solution in a growth chamber with 30/25°C (day/night) temperature and 60–70% humidity under a 12-h photoperiod. The rice culture solution was supplemented with 0.5-mM 2-(N-morpholino)ethanesulfonic acid to stabilize the pH at 5.2. For cytokinin treatment, 6-day-old QHB:GUS, CYCB1;1:GUS, and TCS:GUS seedlings (after germination) were moved to fresh culture solution with or without 100-μM kinetin (KT) and treated for 24 h (TCS:GUS) or time series of 6, 10, and 24 h (QHB:GUS and CYCB1;1:GUS). QHB:GUS and CYCB1;1:GUS seedlings were also grown in culture solution with or without 0.2-μM KT for 1 week. For auxin treatment, 6-day-old DR5:GUS seedlings were moved to fresh culture solution with or without 1-μM naphthalene acetic acid (NAA) and treated for 24 h. Leaves or primary roots were sampled from treated and mock seedlings at each time point and stained in 1-mM X-gluc for the times indicated in the figure legend.
For expression analysis of the CSP1 gene, total RNAs were exacted from 14-day-old young leaves and roots, flag leaves, young and mature spikelets of the wild-type plants, and resistant calli transformed by 35S-HPT-GUS (a1). Total RNAs were extracted using Trizol reagent (Invitrogen, Carlsbad, CA, United States). First-strand complementary DNA was synthesized by using the iScript cDNA Synthesis Kit (Bio-Rad, Mississauga, ON, Canada) with 0.5-μg total RNA of each sample according to the manufacturer’s protocol. Quantitative real-time (qRT)-PCR was performed on the LightCycler 480 real-time PCR system (Roche Diagnostics, Basel, Switzerland) with UltraSYBR mixture (CWBIO, Beijing, China). The relative expression levels of messenger RNA (mRNA) were normalized using the rice Actin 1 gene (LOC_Os11g06390), and fold difference in expression was analyzed by the 2–Δ Δ CT method (Livak and Schmittgen, 2001). Error bars are standard deviations of three technical replicates for each sample. Three biological replicate samples were analyzed. Significant differences were accepted at P < 0.05 by the Student t-test.
To compare the activities of the CSP1 and 35S promoters, independent resistant calli were randomly collected from 25 primary calli in two independent transformations with the vectors of CSP1-HPT-GUS and 35S-HPT-GUS (a1) after 14 days of the second round of selection. Relative expressions of HPT and GUS were analyzed, as described earlier. All the primers used for qRT-PCR are listed in Supplementary Table 3.
Sixteen transgenic T0 plants from each transformation of HPT-35S-GUS (c1) or HPT-CSP1-GUS vector were randomly selected for Southern blot analysis. Rice genomic DNA was extracted from 1-g leaves of transgenic T0 plants using the sodium dodecyl sulfate method, according to Doyle and Doyle (1990), with a few modifications. Approximately 3-μg genomic DNA per sample was digested by NdeI (NEB, Ipswich, United Kingdom) and hybridized with an HPT probe, which was prepared using a random primed digoxigenin-DNA labeling kit (DIG High Prime DNA Labeling and Detection Starter Kit II, Roche Diagnostics, Basel, Switzerland) according to the manufacturer’s protocol. Primers for probe amplification are listed in Supplementary Table 1. The processes of DNA digestion, transfer, and hybridization were as previously reported (Zhou et al., 2013). Southern blot hybridization signals were detected with the chemiluminescence substrate CSPD and visualized by the Amersham Imager 600 (GE Healthcare Life Sciences, Marlborough, MA, United States). Copy number was estimated for each line by Image J software.
To search for genes more specifically expressed in callus cells as compared with other tissues, we analyzed rice Affymetrix expression microarray database by GENEVESTIGATOR (Hruz et al., 2008). Better than the other targeted genes (Supplementary Figure 2), the gene LOC_Os10g14020 was strictly expressed in the callus at a high level and only slightly expressed in the inflorescence and embryo (Figure 1A). We thus chose this gene as a candidate for further analysis and named its promoter as CSP1.
Figure 1. Tissue expression pattern of CSP1 in rice. (A) Tissue expression atlas of CSP1 gene. Gene expression profile among 39 specific tissues was retrieved from transcriptomes by the Genevestigator program (www.genevestigator.com). (B) Real-time RT-PCR analysis of CSP1 gene expression in different rice tissues. Roots and leaves were collected from 14-day-old solution cultured seedlings. Flag leaf, young spikelets were collected from soil-grown plants at maximum tillering stage. Embryogenic calli were collected from mature seeds after 30 days of inoculation on callus induction medium. ACT1 was used as the internal reference gene, and relative expression level in each sample is shown as a fold difference compared with roots. Data are means ± SD of three technical replicates as representative of three independent experiments. (C–E) Expression pattern of CSP1:GUS in roots of 9-day-old seedlings. Three parts of the primary root are shown from distal to proximal end: primary root tip (C), elongation zone (D), differentiation zone with developed lateral roots (E). Scale bars, 100 mm. (F–J) Expression of CSP1:GUS in leaf tip of 9-day-old seedling (F), tip of lemma and palea (G), anther and ovary (H) of mature flower, geminated embryo (I), and resistant calli (J). Scale bars, 1 mm. Images are representatives of three independent GUS-positive transgenic lines.
To confirm the transcriptome data, we first analyzed the gene expression level in different rice tissues by qRT-PCR. Consistent with the gene expression atlas provided by the Anatomy tool of GENEVESTIGATOR, the transcripts were abundant in the embryogenic calli and extremely low in other tissues (Figure 1B), although relatively higher expression was observed in young spikelets than in other tissues except for calli. We also found that the CSP1 expression level in resistant calli was similar or slightly lower than that in embryogenic calli (Supplementary Figure 3), suggesting that hygromycin treatment did not greatly affect the high CSP1 activity in the selection stage. To demonstrate the distinct expression pattern of the CSP1 promoter in rice, the 5′ upstream regulatory sequence before the translation start codon was subject to cis-element analysis. Within a 1,996-bp fragment (−1,896 to +100) (Supplementary Sequence 1), cis-elements related to the responsiveness of light, drought, cold, anaerobic induction, and seed or meristem-specific regulation were predicted (Supplementary Sequence 1 and Supplementary Excel 2). Then, this promoter fragment was cloned into the GUS reporter vector for transformation. After selection, 22 resistant calli were picked randomly for GUS staining, and all of them showed strong GUS expression on the surface of the calli (Figure 1J). After regeneration, 23 PCR-positive, fertile transgenic lines were recovered. Weak or undetectable GUS expressions were found in the T1 seedlings of the transgenic lines obtained. Most of the GUS-positive lines had a similar expression pattern to that shown in the representative line (Figures 1C–I), with specific expression in the meristematic zone of primary and developed lateral root (LR) tips (Figures 1C,E) and also in the tips of leaves, lemmas, and paleas (Figures 1F,G). As expected, there was also an expression in the reproductive organs (Figure 1H) and germinated embryo (Figure 1I). These results indicate that the CSP1 promoter is expressed in a tissue-specific manner in rice and is highly activated in callus.
As the CSP1 promoter is predominantly expressed in the callus, we compared its strength with the constitutive promoter CaMV 35S, which is used for selective marker expression in most binary vectors. We first compared the strength of the CSP1 and CaMV 35S promoters by their effects on the tolerance of the resistant calli to the selective agent. As shown in Figure 2B, the resistant calli with HPT controlled by CSP1 grew as vigorously as those controlled by CaMV 35S during both the first (S1) and second (S2) rounds of selection with hygromycin. There were no statistical differences in fresh weights at both the start of S1 and S2 between the CaMV 35S and CSP1-controlled vectors in two replicated experiments (Figure 2C). At the end of S1, there were approximately 2.3- to 2.4-fold and 2.2- to 2.3-fold increases of weight in calli selected with the CaMV 35S and CSP1 vectors, respectively (Supplementary Table 4). After the second round of selection, proliferated hygromycin-resistant calli were collected for qRT-PCR analysis. Consistent with the growth results, similar or slightly higher HPT mRNA levels were transcribed in resistant calli selected by the CSP1 promoter compared with those selected by CaMV 35S (Figure 2D). These results demonstrate that the CSP1 promoter could be used as effectively as the CaMV 35S promoter for embryogenic callus-based transformation in rice.
Figure 2. CSP1 promoter has high transcriptional activity in callus comparable with that of the CaMV 35S promoter. (A) Schematic diagram showing the structure of the HPT-GUS vectors with CaMV 35S and CSP1 promoters near the left border (LB) and controlling the expression of HPT. A promoterless GUS cassette with multiple cloning sites (MCS) was designed for histochemical analysis. (B) Growth of transformed calli 21 days after the onset of first-round selection on 50 mg/L Hyg (S1) and 14 days after the second-round selection on fresh medium with the same Hyg concentration (S2). Left panel, transformed with 35S controlled HPT-GUS vector; right panel, transformed with CSP1 controlled HPT-GUS vector. A representative dish of calli from two independent transformations with each vector is shown. (C) Hyg-tolerant growth of the calli transformed by the HPT-GUS vectors with HPT controlled by 35S and CSP1, respectively. Weight of fresh calli per dish was measured at the beginning of the first (S1) and second (S2) rounds of selection. Data are mean ± SD (n ≥ 5). Experiments were performed twice in two independent transformations (indicated as –1 and –2) with each vector. No significant difference was found at P < 0.1 in a Student’s t-test. (D,E) Relative HPT and GUS expression levels in secondary resistant calli transformed by 35S and CSP1-controlled HPT-GUS vectors. Data are mean ± SD of three technical replicates in each of two independent experiments (indicated as Rep 1 and Rep 2). * and *** indicate a significant difference at P < 0.05 and 0.001, respectively, in a Student’s t-test.
Previous work suggested that the activity of the promoter used for selective marker gene expression would affect the inserted T-DNA numbers in the transgenic plants (Zhou et al., 2013). We, therefore, investigated the T-DNA copy numbers integrated into the plants transformed via vectors with HPT controlled by CaMV 35S or CSP1 promoters near the GUS reporter (Figure 3A). Sixteen independent lines randomly selected from each transgenic population were used for Southern blot analysis. As expected, lines transformed with the CaMV 35S vector were found to have low numbers of hybridizing bands varying from 1 to 5, and most of them had only a single band (Figure 3B). Most lines transformed with the CSP1 vector had multiple bands; numbers varied from 1 to 12, with most having 2 or 3 (Figure 3C). On average, there were approximately 1.7 T-DNA copies integrated into lines transformed with the CaMV 35S vector, but 3.6 copies in those lines transformed with the CSP1 vector. Because the T-DNA integration pattern in the regenerated plant population represented that in the resistant calli, we previously found that inadequate expression of the selectable marker driven by a weak promoter can lead to preferential survival of cells with multiple copy number to provide sufficient HPT expression (Zhou et al., 2013). This, therefore, suggests that the actual activity of a single copy CSP1 promoter may likely be lower than CaMV 35S in callus, although their overall HPT levels were similar (Figure 2D), and that the CSP1 promoter enables the vigorous, resistant calli growth and high expression of HPT is probably achieved by increased copy number.
Figure 3. Comparison of copy numbers among transgenic T0 plants transformed with HPT-GUS vectors controlled by the CaMV 35S and CSP1 promoters. (A) Schematic diagram showing the structure of the HPT-GUS vectors with CaMV 35S and CSP1 promoters in reverse and upstream of the promoterless GUS gene and controlling the expression of HPT. Probe for Southern blot hybridization was designed from the 3′ end of HPT gene after NdeI. (B,C) Southern blot analysis of integrated T-DNA copy number in 16 independent T0 transgenic plants transformed with HPT-GUS vectors controlled by 35S (B) or CSP1 (C) promoters using the HPT probe shown in (A).
Before using the CSP1 promoter to select vectors with the GUS gene under specific promoters, we compared the CSP1 and CaMV 35S promoters for their effects on GUS leaking in transformed calli. Four promoterless GUS vectors in two stacking configurations were transformed for GUS staining (Figures 2A, 3A). GUS leaky expression was determined by histochemical staining of calli after 2 weeks of selection, and numbers of staining spots on the surface of each callus were counted. As reported previously, the inverted 35S promoter upstream of the GUS reporter (HPT-35S-GUS) activated the strongest GUS expression in massive cell clusters (Figure 4C), whereas the GUS expression spots were dramatically reduced to a low level when the inverted CSP1 promoter was at the same position (Figures 4D,G). The low reverse activity of the CSP1 promoter was further confirmed by its failure to drive effective HPT gene expression and allow the transformed calli to survive (Supplementary Figure 4B). When the selectable marker cassette was stacked upstream of the GUS reporter in head to tail orientation, the GUS leaky expression by the separated CSP1 promoter (CSP1-HPT-GUS) was slightly higher than that of the 35S promoter (35S-HPT-GUS) in terms of the mRNA level determined by RT-PCR (Figure 2E), but the distribution pattern and average spot number were similar between the two vectors (Figures 4A,B,E,F). Interestingly, among these vectors, the inverted CSP1 promoter seemed to have the least effect on the leaky expression of GUS, as the resultant staining spots were tiny and weak (Figures 4D,G).
Figure 4. GUS leaky expression in calli transformed by HPT-GUS vectors with HPT controlled by the CaMV 35S and CSP1 promoters in two stacking configurations. (A) GUS leaky expression in calli transformed by the 35S-HPT-GUS vector with the CaMV 35S promoter near the LB. (B) GUS leaky expression in calli transformed by the CSP1-HPT-GUS vector with the CSP1 promoter near the LB. (C) GUS leaky expression in calli transformed by the HPT-35S-GUS vector with the 35S promoter in reverse and near the GUS gene. (D) GUS leaky expression in calli transformed by the HPT-CSP1-GUS vector with the CSP1 promoter in reverse and near the GUS gene. Figures are representative calli from two independent transformations by each vector. Bars: 1 mm. (E–G), Histograms showing the numbers of spots of GUS leaky expression in calli transformed by 35S-HPT-GUS (E), CSP1-HPT-GUS (F), and HPT-CSP1-GUS (G) vectors in two independent experiments, and one of them was shown. A total of 30 transformed calli per vector were selected for GUS staining overnight at 37°C after 2 weeks of selection. X-axis indicates the range of numbers of GUS spots per callus from 0 to 50 and divided into 11 intervals: 0, 1–5, 6–10, 11–15, 16–20, 21–25, 26–30, 31–35, 36–40, 41–45, and 46–50. Y-axis shows the number of calli that corresponds to each range. Average number of GUS spots per callus derived from each vector is shown on each histogram.
Because the CSP1 promoter had similar or even higher potential than the 35S promoter to cause the mis-expression of non-adjacent downstream genes in callus, we investigated the interaction ability of the CSP1 promoter in other plant tissues. We selected six specific promoters for GUS expression using the vector with HPT driven by the CSP1 promoter (Figure 2A). The GUS expression patterns were first analyzed by histochemical staining of the newly developed adventitious root (AR) tips or leaf segments of the transgenic T0 plants. All the vectors except QHB:GUS had high GUS-positive staining percentages ranging from 84.6 to 92.0% (Table 1), which indicated that the transformants could be recovered efficiently by using the CSP1 promoter to drive HPT gene expression. Among the 57 GUS-positive lines transformed with QHB:GUS, 31 of them had clear and strong GUS staining in the quiescent center (QC) of both AR and LR, similar to the pattern in the T1 generation (Figure 5). The remaining 23 lines had weak or invisible GUS expression in the QC of AR but still had distinct expression in the QC of LR. Only three lines had non-specific GUS expression in the region outside the QC, such as the elongation zone. In transgenic plants containing CYCB1;1:GUS, all 89 GUS-positive lines had similar expression profiles in the meristematic zone of AR, the whole LR primordia, and emerged LR as shown in the primary root of their T1 seedlings (Figure 5). In the 77 GUS-positive transgenic plants containing DR5:GUS, four major staining patterns were observed and classified in the T0 ARs (Supplementary Table 5). Three of them (types c, d, and h) also occurred when the tCUP1 vector (a3-DR5) was used in previous experiments (Zhou et al., 2014), and 66 lines showed at least one of these three patterns. Typical DR5:GUS expression occurred in the root cap, QC, and protoxylem cells in the AR of T0 plants (Supplementary Figure 5). In addition to the same region of the primary root tip, most of the T1 seedlings also expressed the DR5:GUS in the meristematic zone (Figure 5). For the 45 GUS-positive TCSn:GUS transgenic lines, 43 of them had GUS expression confined to the outer layer of the root cap and the stele of AR (Supplementary Figure 6), whereas the other two lines had no expression in these tissues. In the primary roots of T1 seedlings, TCSn:GUS expression was strong in the outer layers of the root cap and gradually attenuated in the columella initials, QC, and stele initials in the meristematic zone (Figure 5). Strong expression was also observed in the vasculature of LR (Figure 5). As for the 75 GUS-positive RR6:GUS transgenic lines, 60 of them had GUS staining in the AR root cap and the stele of AR and LR as in the roots of the T1 generation (Figure 5). The other 15 lines had expression in the AR root cap or LR only. For the promoter of the pathogenesis-related gene OsPR1b, staining patterns in the leaf segments were characterized in the 46 GUS-positive T0 lines. Forty-four lines had the same distribution of random GUS spots on the leaf surface, as shown in Supplementary Figure 7. No GUS staining was found in the roots of T1 generation seedlings, which served as negative root staining controls (Figure 5). The other two lines had constitutive GUS staining on the leaf surface.
Figure 5. Specific GUS staining patterns in the roots of QHB/CYCB1;1/DR5/TCSn/RR6/PR1b:GUS transgenic lines selected by vectors based on CSP1-HPT-MCS-GUS. Primary roots of 8-day-old seedlings were subjected to GUS staining at 37°C for 22 h (QHB, TCSn, and PR1b) or 30 min (CYCB1;1, DR5, and RR6). GUS staining images of the root tips (lower panels), root segments with initiated lateral root primordia (middle panels), and root segments with elongated lateral roots (upper panels) were shown. Bars, 100 μm. Images are representative GUS expression patterns in T1 generation of at least three lines.
Except for the specific GUS expression in roots, we also observed different leaf expression patterns among these promoter lines. As shown in Supplementary Figure 7, the QHB:GUS and CYCB1;1:GUS lines had no GUS staining in leaves. The DR5:GUS line had weak GUS expression in the leaf tip. The TCSn:GUS line had GUS staining mainly in the leaf tip and edges of the upper leaf blade, whereas the RR6:GUS line had weak GUS staining of the whole leaf.
After characterizing the specific expression patterns of the promoters constructed in the CSP1-based expression vector, we then examined the hormone responsiveness of the GUS reporter lines of the QHB, CYCB1;1, TCSn, RR6, and DR5 promoters. As shown in Figure 6A, QHB:GUS expression in the root stem cells progressively decreased after continued application of 100-μM cytokinin KT for 24 h. Under the same conditions, the staining area of CYCB1;1:GUS in the primary root meristem was significantly reduced after treatment for 8 h and was almost completely lost after 24 h (Figure 6B). These results suggested that the high level of external cytokinin treatment may inhibit the root growth by dampening the QC identity and root meristem cell activity.
Figure 6. Cytokinin and auxin responsiveness in QHB/CYCB1;1/TCSn/DR5:GUS transgenic lines selected by vectors based on CSP1-HPT-MCS-GUS. (A) Six-day-old QHB:GUS transgenic seedlings (T2) were subjected to 100-μM KT treatment for 0, 6, 10, and 24 h. Primary root tips were collected at each time point and subjected to GUS staining for 6 h to show the progressively reduced GUS expression in response to prolonged KT treatment. (B) Six-day-old CYCB1;1:GUS transgenic seedlings (T2) were treated with 100-μM KT for the same periods as (A). Primary root tips were collected at each time point and subjected to GUS staining for 1 h. (C) Six-day-old TCSn:GUS transgenic seedlings (T2) were treated with 100-μM KT in fresh culture solution for 24 h or without KT as mock treatment. Primary root tips (left two panels) and a mature root zone with lateral roots (right two panels) were dissected for GUS staining. Strongly induced GUS expressions in the entire root are clearly shown after 7-h staining. (D) Six-day-old DR5:GUS transgenic seedlings (T2) were treated with 1-μM NAA in fresh culture solution for 24 h or without NAA as mock treatment. Primary root tips (left two panels) and a mature root zone with lateral roots (right two panels) were dissected for GUS staining. Increased GUS expression is clearly shown after 30-min staining. Bars, 100 μm. Images are representative GUS expression patterns of at least three lines.
To assess the sensitivity of TCSn:GUS to cytokinin, hydroponically cultured transgenic seedlings were treated with 100-μM KT for 24 h. Compared with the mock treatment, strong GUS expression was found in the entire primary root tip and the mature root region with the most induction in the vascular bundle and LRs (Figure 6C). A similar induction pattern was obtained with RR6:GUS under the same condition (Supplementary Figure 8). Meanwhile, the ability of auxin-induced DR5:GUS expression was also confirmed by the exogenous application of 1-μM NAA for 24 h. Compared with the restricted expression in the mock treatment, strong and constitutive expression was induced in the whole primary root tip and LRs (Figure 6D), similar to the auxin-responsive pattern of a3-DR5 reported previously. These experiments clearly demonstrated that the CSP1 promoter used for selectable marker expression did not affect the ability of the TCSn/RR6/DR5 promoters to respond to hormones.
The GENEVESTIGATOR analysis showed that the gene LOC_Os10g14020 was strongly expressed in the callus and relatively weakly in the inflorescence and embryo (Figure 1A), and it was higher and more specifically expressed in the callus than the reported Gns9 and CP genes. This expression pattern was consistent with the expression profile from the RiceXpro database2 (Sato et al., 2011), which showed specific expression of the target gene in ovule and embryo among the tissue/organs encompassing the entire growth of the rice plant except for callus (Supplementary Figure 9A). In line with the transcriptome analysis, qRT-PCR confirmed the overwhelming expression of the target gene in the embryogenic calli, far beyond the levels in other tissues (Figure 1B), and we, therefore, designated its promoter as CSP1. In silico promoter analysis on PlantCARE shows cis-acting regulatory elements related to the responsiveness of light, drought, and cold and also elements involved in seed or meristem-specific regulation (Supplementary Figure 1 and Supplementary Excel 2), which is supported by GENEVESTIGATOR perturbation analysis, which reveals the induction of CSP1 by light, high temperature, and dehydration (Supplementary PDF 1). Expression analysis on the RiceXpro database shows that the CSP1 is stable in response to plant hormone treatment (Supplementary Figure 9B). Matched with the in silico analysis, GUS histochemical analysis indicated that the CSP1 promoter was also expressed in the root meristem, leaf tips, lemma and palea, ovary, anther, and embryo of rice (Figures 1C,I). Although the function of the target gene in callus is not known, promoter deletion analysis would be helpful to isolate and characterize the cis-regulatory elements specific to callus and their role on CSP1 promoter regulated expression. In addition, CSP1 is annotated in the National Center for Biotechnology Information as a homolog of Arabidopsis TPD1 (tapetum determinant 1) protein, and so it is very likely to have similar involvement in reproductive organ development (Yang et al., 2003; Huang et al., 2016; Chen et al., 2019).
Cauliflower mosaic virus 35S is the most frequently used promoter in plant biotechnology. It can affect the expression of transgenes located either downstream or upstream (Yoo et al., 2005; Gudynaite-Savitch et al., 2009), possibly via its enhancer regions (Benfey et al., 1990a,b). As shown in this study as well as our previous report (Zhou et al., 2014), substantial GUS leaking occurred when the 35S promoter was placed either in reverse orientation (Figure 4C) or separated by the HPT gene (Figure 4A). In contrast to the strong bidirectional activity of the CaMV 35S promoter, the reverse CSP1 promoter had only the slightest capacity to activate adjacent GUS or HPT gene expression (Figures 4D,G and Supplementary Figure 4), indicating that the CSP1 promoter is under tight regulation. With its strict unidirectional expression, CSP1 could be used as an insulator to preventing inappropriate cross-regulation of its flanking neighborhood. Because only a few plant insulators have so far been identified and shown to be functional (Hily et al., 2009; Singer et al., 2011; Yang et al., 2011), it would be interesting to assess the feasibility of using CSP1 to block enhancer–promoter communication and to study its impact on upstream gene expression.
In this study, we examined the effect of CSP1 promoter on its downstream GUS gene expression. First, we compared the leaky expression of the promoterless GUS gene in callus caused by the upstream CSP1 or 35S promoters (Figure 2A). No big differences were found in terms of the spot size and average spot number per callus (Figures 4A,B,E,F), but two to sevenfold higher GUS gene transcription was caused by the CSP1 promoter compared with the 35S (Figure 2E). This suggests that the strong activity of CSP1 also leads to a high mis-expression of non-adjacent downstream genes in the callus. However, when we looked at the GUS expression in leaf or root after the introduction of particular promoters with distinct cellular expression, most of the transgenic plants had the GUS expression expected from the respective promoter (Table 1). Thus, it appears that the potential multi-promoter interactions may be mitigated if the promoter binding factors are not expressed at the same time and space. In the case of the CSP1 promoter, the brisk transcriptional activity was decreased and confined to specific regions when plants were regenerated from differentiated calli (Figure 1), which greatly reduces the contact opportunity between the factors binding to the CSP1 and other promoters in the same vector. Interestingly, even in the region where CSP1 was expressed, e.g., in the root meristematic region (Figures 1C,E), no override of expression was shown on the target promoters (Figure 5). The lines transformed by the same promoter vector differed at most in the varied intensity of GUS expression (Supplementary Figure 6). This could be the result of multiple T-DNA integrations in plants generated by CSP1-based selection (Figure 3B), and the number of T-DNA copies may positively affect transgene expression, as we reported previously (Zhou et al., 2013).
Based on this reporter vector, we characterized the GUS expression profiles of six synthetic or native promoters involving hormone signaling, pathogen response, cell fate determination, and proliferation in both roots and leaves (Figure 5 and Supplementary Figure 7). The synthetic auxin-inducible promoter DR5 is the most widely used sensor to monitor auxin response and distribution (Ulmasov et al., 1997). Previously, we have made a detailed observation of DR5:GUS expression in rice roots using vectors where a selectable marker gene was controlled by different promoters (Zhou et al., 2014). Typical patterns were obtained by the a3-DR5 vector in the root cap, QC, and protoxylem cells in the meristem, quite similar to that observed by DR5rev-controlled 3xVenus fluorescent reporters in rice roots (Heisler et al., 2005; Li et al., 2014). For comparison, we examined the expression of DR5 by the vector similar to a3-DR5, where the tCUP1 was replaced by CSP1. Although different patterns were observed in the T0 ARs (Supplementary Table 5), many of them (types c, d, and h) also occurred when the a3-DR5 vector was used. The classical DR5:GUS expression was also found in the AR tip of CSP1-DR5 T0 plants (Supplementary Figure 4), except that GUS expression was confined to the initial xylem cells and was weaker than a3-DR5. Overall, these data indicated that the specificity of DR5 could be retained by the use of the CSP1 promoter to drive selectable marker gene expression.
Although DR5 has been regularly used to study auxin, the synthetic two-component signaling sensor (TCS) has only been developed relatively recently to study cytokinin signaling output (Muller and Sheen, 2008). Because of some limitations, an improved new version TCSn was optimized with superior strength and sensitivity (Zurcher et al., 2013). The function of TCSn in correlation with cytokinin has mainly been studied in Arabidopsis involving multiple developmental contexts from embryogenesis to shoot and root formation. It was also conservatively expressed in rice by fusing to a GUS reporter (Tao et al., 2017). Here, we expressed TCSn:GUS in rice by the CSP1-based vector and analyzed the GUS expression in roots and leaves. In our experiments, TCSn-driven GUS was intensively expressed in the outer layers of the primary root cap and less so in the cells at the boundary between the root and root cap, including QC, root cap initials, and immature stele (Figure 5). This result was consistent with the TCSn expression in Arabidopsis root apex as well as that reported in rice (Zurcher et al., 2013; Tao et al., 2017) and in close agreement with the high-resolution cytokinin distribution measured in specific types of root cells (Antoniadi et al., 2015). Strong expression was also found in the steles of AR and LR (Figure 5 and Supplementary Figure 6) but not in the LR cap, as shown in the two previous reports (Zurcher et al., 2013; Tao et al., 2017). Besides the synthetic cytokinin sensor TCSn, we also expressed the endogenous cytokinin responsive promoter of RR6, an A-type response regulator in the rice two-component signaling system (Hirose et al., 2007). Maximum expression of RR6:GUS was also found in the root cap and stele tissues but not in the LR cap (Figure 5). Both TCSn and RR6-mediated GUS expression can be remarkably induced in roots by exogenous application of cytokinin (Figure 6B and Supplementary Figure 8), suggesting that their GUS activities are consistent with the function of cytokinin.
The root apical meristem is the important place where root radial organization begins by ordered cell division and differentiation from initial cells around the QC (Dolan et al., 1993). The QC in Arabidopsis comprises four mitotically inactive cells where the WUCHEL-RELATED HOMEOBOX 5 (WOX5) is specifically expressed (Sarkar et al., 2007). In rice, similar small numbers of QC cells are characterized, but the ortholog of WOX5, QUIESCENT-CENTER-SPECIFIC HOMEOBOX (QHB) is expressed in a broad area including stem cells near the QC (Ni et al., 2014). Here, we showed that QHB:GUS constructed in the CSP1-based vector had a similar expression level in the center of the root meristem containing the QC cells and its surrounding stem cells (Figure 5 and Supplementary Figure 10). The stability of the QC has been studied under various conditions (Ni et al., 2014). Although root architecture was altered, the cell patterns of the QC were not affected under nutrient deficiency or treatment with auxin and cytokinin, suggesting that QC activity was largely maintained to keep continuous root growth and function in the unfavorable environment. In agreement with that report, we found that expression of QHB:GUS in the stem cell niche was not affected by treatment with 0.2-μM KT for 7 days (Supplementary Figure 11B), but the number of cells with high mitotic activity indicated by CYCB1;1:GUS (Doerner et al., 1996) was reduced after the same treatment (Supplementary Figure 11C). These results indicated that a low cytokinin level greatly inhibited root growth (Supplementary Figure 11A) by damaging root meristem activity.
After becoming aware of the strong artificial effect of the CaMV 35S promoter on specific promoters constructed in the same vector, most of the subsequent promoter analysis in Arabidopsis and rice have been done in vectors where the Nos promoter was used for selectable marker gene expression. The reported promoter patterns we used for comparison were mainly obtained in these vectors. The consistent-specific expression could be obtained in some cases, such as the expression of QHB (Kamiya et al., 2003; Ni et al., 2014) and CYCB1;1 (Chen et al., 2013) was similar to that obtained in this study. The expression of PR1b previously analyzed in a Nos vector (Yu et al., 2014) was also similar to that expressed in the CSP1 vector. With TCSn, strong expression in the whole LR and root hairs were shown in two previous reports (Zurcher et al., 2013; Tao et al., 2017), but we found no expression in the LR cap and only weak expression in part of the root hairs. Both of the vectors in those earlier reports, pCB302 and pBI101, used the Nos promoter to express either Basta or kanamycin-resistant genes for selection (Jefferson et al., 1987; Xiang et al., 1999). The possible interference between the Nos promoter and TCSn in these tissues needs to be analyzed by further experiments.
The original contributions presented in the study are included in the article/Supplementary Material, further inquiries can be directed to the corresponding author/s.
XW, JZ, and JC conceived the project, designed the experiments, and wrote the manuscript. JZ, DL, and CZ performed the experiments with assistance from EZ, YY, CYu, and YC. JZ, XW, and CYa analyzed the results. All authors contributed to the article and approved the submitted version.
This work was supported by grants from the National Key Research and Development Program of China (2016YFD0100601-15), Zhejiang Fundamental Public Welfare Research Program (LGN19C140008), Zhejiang Provincial Key Research and Development Plan (2019C02006), Major “Scientific and Technological Innovation 2025” Project of Ningbo (2019B10004), and Key Scientific Technological Project of Ningbo (2016C11017).
The authors declare that the research was conducted in the absence of any commercial or financial relationships that could be construed as a potential conflict of interest.
We are grateful to Professor M. J. Adams from Rothamsted Research, United Kingdom, for critically reading the manuscript.
The Supplementary Material for this article can be found online at: https://www.frontiersin.org/articles/10.3389/fpls.2020.602680/full#supplementary-material
Supplementary Figure 1 | Screen of the callus-specific expressed genes in rice. Ten distinct genes were searched out using the Anatomy tool of GENEVESTIGATOR with callus as target.
Supplementary Figure 2 | Tissue expression patterns of the 10 callus-specific candidate genes. Relative expression levels are shown in different tissues and compared among the selected 10 genes using the Anatomy tool of GENEVESTIGATOR.
Supplementary Figure 3 | Relative CSP1 expression levels in untransformed calli and secondary resistant calli transformed by 35S controlled HPT-GUS vector. Data are mean ± SD of three technical replicates in each of two independent experiments (indicated as Rep 1 and Rep 2). ∗ indicate a significant difference at P < 0.05 in a Student’s t-test.
Supplementary Figure 4 | The reverse CSP1 promoter did not drive the effective expression of HPT gene in callus for transformant selection. Growth of transformed calli 14 days after the second-round selection on fresh medium with 50 mg/L Hyg. Left panel, transformed with 35S controlled HPT-GUS vector; right panel, transformed with reverse CSP1 controlled HPT-GUS vector. A representative dish of calli in each transformation is shown.
Supplementary Figure 5 | Typical GUS expression patterns in the adventitious roots of DR5:GUS T0 transgenic lines selected by the CSP1-HPT-MCS-GUS vector. Adventitious root tips of three independent lines T0-36 (left), T0-41 (middle), and T0-62 (right) are shown. GUS was mainly expressed in the root cap, quiescent center and protoxylem cells in the root meristem. Bars: 100 μm.
Supplementary Figure 6 | GUS expression patterns in the adventitious roots of TCSn:GUS T0 transgenic lines selected by the CSP1-HPT-MCS-GUS vector. Adventitious root tips of two independent lines are shown as representative of weak (T0-6) or strong (T0-42) GUS expression patterns of the TCSn promoter. Similar GUS staining was detected predominantly in the outer layers of root cap and stele. Bars: 100 μm.
Supplementary Figure 7 | Specific GUS staining patterns in the leaves of QHB/CYCB1;1/DR5/TCSn/RR6/PR1b:GUS transgenic lines selected by vectors based on CSP1-HPT-MCS-GUS. GUS expressions of the first fully expended leaf of 8-day-old seedlings were analyzed. Leaf tips (upper panels) and adjacent part near the leaf tips (lower panels) are shown. Bars, 2 μm.
Supplementary Figure 8 | Cytokinin responsiveness of RR6:GUS transgenic plant transformed by the CSP1 controlled vector. 8-day-old RR6:GUS transgenic seedlings (T1) were treated with 100 μM KT in fresh culture solution for 24 h or without KT as mock treatment. Primary root tips (left two panels) and mature root zone with lateral roots (right two panels) were dissected for GUS staining. Strongly induced GUS expressions in the entire root is clearly shown after 7 h staining. Bars, 100 μm.
Supplementary Figure 9 | Expression profile of CSP1 analyzed on the RiceXpro platform (http://ricexpro.dna.affrc.go.jp/). (A) The spatial temporal expression profile. (B) Expression profile in response to plant hormones in root and shoot.
Supplementary Figure 10 | The expression pattern of QHB in the root tip of rice. Primary root tip of 7-day-old QHB:GUS transgenic seedling (T1) was subjected to GUS staining for 6 h and observed under microscope with 20× objective lens after clearing. GUS expression was found in the center of the root meristem containing the QC and its surrounding stem cells. The red arrows indicate the single layer of epidermal cells originating from the stem cells near the QC. Bars: 50 μm.
Supplementary Figure 11 | GUS expression activity of QHB and CYCB1;1 in root tip after cytokinin treatment at low level. (A) Root growth inhibition of QHB:GUS and CYCB1;1:GUS transgenic seedlings (T3) grown on solution cultures with or without 0.2 μM KT for 7 days. Bars: 2.5 cm. (B) QHB:GUS expression in the quiescent center of the mock and KT treated roots after GUS staining for 16 h. Bars: 100 μm. (C) CYCB1;1:GUS expression in the meristematic region of the mock and KT treated roots after GUS staining for 1 h. Bars: 100 μm.
Supplementary Table 1 | Primers used for plasmid construction.
Supplementary Table 2 | Vectors used in experiments.
Supplementary Table 3 | Primers used for quantitative real-time PCR analysis and Southern blot.
Supplementary Table 4 | Callus weight at the start of the first (S1) and second (S2) rounds of selection.
Supplementary Table 5 | GUS expression patterns observed in roots of DR5:GUS T0 transgenic plants.
Supplementary Sequence 1 | Sequence of the CSP1 promoter amplified (1996 bp).
Supplementary Sequence 2 | Synthesized sequence of TCSn1 (282 bp).
Supplementary Sequence 3 | PstI digested mini35S-TMVΩ fragment for ligation of TCSn1 (147 bp).
Supplementary Excel 1 | Vector sequence of CSP1-HPT-T35S-MCS-GUS.
Supplementary Excel 2 | Cis-elements analysis of the CSP1 promoter by PlantCARE.
Supplementary PDF 1 | Perturbation analysis of the CSP1 gene by GENEVESTIGATOR.
Antoniadi, I., Plaèková, L., Simonovik, B., Doležal, K., Turnbull, C., Ljung, K., et al. (2015). Cell-Type-Specific Cytokinin Distribution within the Arabidopsis Primary Root Apex. Plant Cell 27:1955.
Benfey, P. N., Ren, L., and Chua, N. H. (1990a). Combinatorial and synergistic properties of CaMV 35S enhancer subdomains. EMBO J. 9, 1685–1696.
Benfey, P. N., Ren, L., and Chua, N. H. (1990b). Tissue-specific expression from CaMV 35S enhancer subdomains in early stages of plant development. EMBO J. 9, 1677–1684.
Biłas, R., Szafran, K., Hnatuszko-Konka, K., and Kononowicz, A. K. (2016). Cis-regulatory elements used to control gene expression in plants. PCTOC 127, 269–287.
Chen, W., Lv, M., Wang, Y., Wang, P. A., Cui, Y., Li, M., et al. (2019). BES1 is activated by EMS1-TPD1-SERK1/2-mediated signaling to control tapetum development in Arabidopsis thaliana. Nat. Commun. 10:4164.
Chen, X., Shi, J., Hao, X., Liu, H., Wu, Y., Wu, Z., et al. (2013). OsORC3 is required for lateral root development in rice. Plant J. 74, 339–350.
Coudert, Y., Perin, C., Courtois, B., Khong, N. G., and Gantet, P. (2010). Genetic control of root development in rice, the model cereal. Trends Plant Sci. 15, 219–226.
Denis, M., Delourme, R., Gourret, J. P., Mariani, C., and Renard, M. (1993). Expression of Engineered Nuclear Male Sterility in Brassica napus (Genetics, Morphology, Cytology, and Sensitivity to Temperature). Plant Physiol. 101, 1295–1304.
Doerner, P., Jorgensen, J. E., You, R., Steppuhn, J., and Lamb, C. (1996). Control of root growth and development by cyclin expression. Nature 380, 520–523.
Dolan, L., Janmaat, K., Willemsen, V., Linstead, P., Poethig, S., Roberts, K., et al. (1993). Cellular organisation of the Arabidopsis thaliana root. Development 119:71.
Franck, A., Guilley, H., Jonard, G., Richards, K., and Hirth, L. (1980). Nucleotide sequence of cauliflower mosaic virus DNA. Cell 21, 285–294.
Gao, S., Fang, J., Xu, F., Wang, W., Sun, X., Chu, J., et al. (2014). CYTOKININ OXIDASE/DEHYDROGENASE4 Integrates Cytokinin and Auxin Signaling to Control Rice Crown Root Formation. Plant Physiol. 165, 1035–1046.
Gudynaite-Savitch, L., Johnson, D. A., and Miki, B. L. (2009). Strategies to mitigate transgene-promoter interactions. Plant Biotechnol. J. 7, 472–485.
He, Y., Ning, T., Xie, T., Qiu, Q., Zhang, L., Sun, Y., et al. (2011). Large-scale production of functional human serum albumin from transgenic rice seeds. Proc. Natl. Acad. Sci. 108:19078.
Heisler, M. G., Ohno, C., Das, P., Sieber, P., Reddy, G. V., Long, J. A., et al. (2005). Patterns of auxin transport and gene expression during primordium development revealed by live imaging of the Arabidopsis inflorescence meristem. Curr. Biol. 15, 1899–1911.
Hiei, Y., and Komari, T. (2008). Agrobacterium-mediated transformation of rice using immature embryos or calli induced from mature seed. Nat. Protoc. 3, 824–834.
Hily, J. M., Singer, S. D., Yang, Y., and Liu, Z. (2009). A transformation booster sequence (TBS) from Petunia hybrida functions as an enhancer-blocking insulator in Arabidopsis thaliana. Plant Cell Rep. 28, 1095–1104.
Hirose, N., Makita, N., Kojima, M., Kamada-Nobusada, T., and Sakakibara, H. (2007). Overexpression of a type-A response regulator alters rice morphology and cytokinin metabolism. Plant Cell Physiol. 48, 523–539.
Hruz, T., Laule, O., Szabo, G., Wessendorp, F., Bleuler, S., Oertle, L., et al. (2008). Genevestigator V3: A Reference Expression Database for the Meta-Analysis of Transcriptomes. Adv. Bioinform. 2008:5.
Huang, J., Wijeratne, A. J., Tang, C., Zhang, T., Fenelon, R. E., Owen, H. A., et al. (2016). Ectopic expression of TAPETUM DETERMINANT1 affects ovule development in Arabidopsis. J. Exp. Bot. 67, 1311–1326.
Huang, N., Wu, L., Nandi, S., Bowman, E., Huang, J., Sutliff, T., et al. (2001). The tissue-specific activity of a rice beta-glucanase promoter (Gns9) is used to select rice transformants. Plant Sci. 161, 589–595.
Jagannath, A., Bandyopadhyay, P., Arumugam, N., Gupta, V., Burma, P., and Pental, D. (2001). The use of a Spacer DNA fragment insulates the tissue-specific expression of a cytotoxic gene (barnase) and allows high-frequency generation of transgenic male sterile lines in Brassica juncea L. Mole. Breed. 8, 11–23.
Jefferson, R. A., Kavanagh, T. A., and Bevan, M. W. (1987). GUS fusions: beta-glucuronidase as a sensitive and versatile gene fusion marker in higher plants. EMBO J. 6, 3901–3907.
Jun, N., Gaohang, W., Zhenxing, Z., Huanhuan, Z., Yunrong, W., and Ping, W. (2011). OsIAA23-mediated auxin signaling defines postembryonic maintenance of QC in rice. Plant J. 68, 433–442.
Kamiya, N., Nagasaki, H., Morikami, A., Sato, Y., and Matsuoka, M. (2003). Isolation and characterization of a rice WUSCHEL-type homeobox gene that is specifically expressed in the central cells of a quiescent center in the root apical meristem. Plant J. 35, 429–441.
Khanday, I., Skinner, D., Yang, B., Mercier, R., and Sundaresan, V. (2019). A male-expressed rice embryogenic trigger redirected for asexual propagation through seeds. Nature 565, 91–95.
Kim, S. R., Kim, Y., and An, G. (1993). Identification of methyl jasmonate and salicylic acid response elements from the nopaline synthase (nos) promoter. Plant Physiol. 103, 97–103.
Lescot, M., Dehais, P., Thijs, G., Marchal, K., Moreau, Y., Van De Peer, Y., et al. (2002). PlantCARE, a database of plant cis-acting regulatory elements and a portal to tools for in silico analysis of promoter sequences. Nucleic Acids Res. 30, 325–327.
Li, G., Liang, W., Zhang, X., Ren, H., Hu, J., Bennett, M. J., et al. (2014). Rice actin-binding protein RMD is a key link in the auxin-actin regulatory loop that controls cell growth. Proc. Natl. Acad. Sci. U S A. 111, 10377–10382.
Livak, K. J., and Schmittgen, T. D. (2001). Analysis of relative gene expression data using real-time quantitative PCR and the 2(-Delta Delta C(T)) Method. Methods 25, 402–408.
Malik, K., Wu, K., Li, X. Q., Martin-Heller, T., Hu, M., Foster, E., et al. (2002). A constitutive gene expression system derived from the tCUP cryptic promoter elements. Theor. Appl. Genet. 105, 505–514.
Mariani, C., Beuckeleer, M. D., Truettner, J., Leemans, J., and Goldberg, R. B. (1990). Induction of male sterility in plants by a chimaeric ribonuclease gene. Nature 347, 737–741.
Muller, B., and Sheen, J. (2008). Cytokinin and auxin interaction in root stem-cell specification during early embryogenesis. Nature 453, 1094–1097.
Ni, J., Shen, Y., Zhang, Y., and Wu, P. (2014). Definition and stabilisation of the quiescent centre in rice roots. Plant Biol. 16, 1014–1019.
Ning, T., Xie, T., Qiu, Q., Yang, W., Zhou, S., Zhou, L., et al. (2008). Oral administration of recombinant human granulocyte-macrophage colony stimulating factor expressed in rice endosperm can increase leukocytes in mice. Biotechnol. Lett. 30, 1679–1686.
Paine, J. A., Shipton, C. A., Chaggar, S., Howells, R. M., Kennedy, M. J., Vernon, G., et al. (2005). Improving the nutritional value of Golden Rice through increased pro-vitamin A content. Nat. Biotechnol. 23, 482–487.
Ponstein, A. S., Bade, J. B., Verwoerd, T. C., Molendijk, L., Storms, J., Beudeker, R. F., et al. (2002). Stable expression of Phytase (phyA) in canola (Brassica napus) seeds: towards a commercial product. Mole. Breed. 10, 31–44.
Potenza, C., Aleman, L., and Sengupta-Gopalan, C. (2004). Targeting transgene expression in research, agricultural, and environmental applications: Promoters used in plant transformation. In Vitro Cell. Devel. Biol. Plant 40, 1–22.
Roy, M., Jain, R. K., Rohila, J. S., and Wu, R. (2000). Production of agronomically superior transgenic rice plants using Agrobacterium transformation methods: Present status and future perspectives. Curr. Sci. 79, 954–960.
Sarkar, A. K., Luijten, M., Miyashima, S., Lenhard, M., Hashimoto, T., Nakajima, K., et al. (2007). Conserved factors regulate signalling in Arabidopsis thaliana shoot and root stem cell organizers. Nature 446, 811–814.
Sato, Y., Antonio, B. A., Namiki, N., Takehisa, H., Minami, H., Kamatsuki, K., et al. (2011). RiceXPro: a platform for monitoring gene expression in japonica rice grown under natural field conditions. Nucleic Acids Res. 39, D1141–D1148.
Singer, S. D., Hily, J. M., and Cox, K. D. (2011). Analysis of the enhancer-blocking function of the TBS element from Petunia hybrida in transgenic Arabidopsis thaliana and Nicotiana tabacum. Plant Cell Rep. 30, 2013–2025.
Tao, J., Sun, H., Gu, P., Liang, Z., Chen, X., Lou, J., et al. (2017). A sensitive synthetic reporter for visualizing cytokinin signaling output in rice. Plant Methods 13:89.
Tian, L., Wu, K., Levasseur, C., Ouellet, T., Foster, E., Latoszek-Green, M., et al. (2003). Activity of elements from the tobacco cryptic promoter, tCUP, in conifer tissues. In Vitro Cell. Devel. Biol. Plant 39, 193–202.
Ulmasov, T., Murfett, J., Hagen, G., and Guilfoyle, T. J. (1997). Aux/IAA proteins repress expression of reporter genes containing natural and highly active synthetic auxin response elements. Plant Cell 9, 1963–1971.
Wang, X., Ning, T., and Yang, D. (2012). Cloning and Application of a Callus-Specific Promoter of Rice. J. Wuhan Univ. 58, 327–331.
Xiang, C., Han, P., Lutziger, I., Wang, K., and Oliver, D. J. (1999). A mini binary vector series for plant transformation. Plant Mol. Biol. 40, 711–717.
Yang, S.-L., Xie, L.-F., Mao, H.-Z., Puah, C. S., Yang, W.-C., Jiang, L., et al. (2003). TAPETUM DETERMINANT1 Is Required for Cell Specialization in the Arabidopsis Anther. Plant Cell 15:2792.
Yang, Y., Singer, S., and Liu, Z. (2011). Evaluation and comparison of the insulation efficiency of three enhancer-blocking insulators in plants. Plant Cell, Tissue Organ. Cult. 105, 405–414.
Yoo, S. Y., Bomblies, K., Yoo, S. K., Yang, J. W., Choi, M. S., Lee, J. S., et al. (2005). The 35S promoter used in a selectable marker gene of a plant transformation vector affects the expression of the transgene. Planta 221, 523–530.
Yu, F., Zhou, J., Wang, X., Yang, Y., Yu, C., Cheng, Y., et al. (2014). Expression Characteristics of Rice OsPR1b Gene Promoter. Lett. Biotechnol. 25, 24–30.
Zhou, J., Yang, Y., Wang, X., Yu, F., Yu, C., Chen, J., et al. (2013). Enhanced transgene expression in rice following selection controlled by weak promoters. BMC Biotechnol. 13:29. doi: 10.1186/1472-6750-13-29
Zhou, J., Yu, F. B., Wang, X. M., Yang, Y., Yu, C. L., Liu, H. J., et al. (2014). Specific Expression of DR5 Promoter in Rice Roots Using a tCUP Derived Promoter-Reporter System. PLoS One 9:e87008. doi: 10.1371/journal.pone.0087008
Keywords: transgenic rice, selectable marker, callus strong promoter, transgene expression, β-glucuronidase
Citation: Zhou J, Li D, Zheng C, Xu R, Zheng E, Yang Y, Chen Y, Yu C, Yan C, Chen J and Wang X (2020) Targeted Transgene Expression in Rice Using a Callus Strong Promoter for Selectable Marker Gene Control. Front. Plant Sci. 11:602680. doi: 10.3389/fpls.2020.602680
Received: 04 September 2020; Accepted: 06 November 2020;
Published: 11 December 2020.
Edited by:
Goetz Hensel, Heinrich Heine University Düsseldorf, GermanyReviewed by:
Allah Bakhsh, Ni ð de Ömer Halisdemir University, TurkeyCopyright © 2020 Zhou, Li, Zheng, Xu, Zheng, Yang, Chen, Yu, Yan, Chen and Wang. This is an open-access article distributed under the terms of the Creative Commons Attribution License (CC BY). The use, distribution or reproduction in other forums is permitted, provided the original author(s) and the copyright owner(s) are credited and that the original publication in this journal is cited, in accordance with accepted academic practice. No use, distribution or reproduction is permitted which does not comply with these terms.
*Correspondence: Jianping Chen, anBjaGVuMjAwMUAxMjYuY29t; Xuming Wang, eG13YW5nQHphYXMuYWMuY24=
Disclaimer: All claims expressed in this article are solely those of the authors and do not necessarily represent those of their affiliated organizations, or those of the publisher, the editors and the reviewers. Any product that may be evaluated in this article or claim that may be made by its manufacturer is not guaranteed or endorsed by the publisher.
Research integrity at Frontiers
Learn more about the work of our research integrity team to safeguard the quality of each article we publish.