- 1Laboratory Center of Life Sciences, College of Life Sciences, Nanjing Agricultural University, Nanjing, China
- 2Center of Hydrogen Science, Shanghai Jiao Tong University, Shanghai, China
Magnesium hydride (MgH2) is a promising solid-state hydrogen source with high storage capacity (7.6 wt%). Although it is recently established that MgH2 has potential applications in medicine because it sustainably supplies hydrogen gas (H2), the biological functions of MgH2 in plants have not been observed yet. Also, the slow reaction kinetics restricts its practical applications. In this report, MgH2 (98% purity; 0.5–25 μm size) was firstly used as a hydrogen generation source for postharvest preservation of flowers. Compared with the direct hydrolysis of MgH2 in water, the efficiency of hydrogen production from MgH2 hydrolysis could be greatly improved when the citrate buffer solution is introduced. These results were further confirmed in the flower vase experiment by showing higher efficiency in increasing the production and the residence time of H2 in solution, compared with hydrogen-rich water. Mimicking the response of hydrogen-rich water and sodium hydrosulfide (a hydrogen sulfide donor), subsequent experiments discovered that MgH2-citrate buffer solution not only stimulated hydrogen sulfide (H2S) synthesis but also significantly prolonged the vase life of cut carnation flowers. Meanwhile, redox homeostasis was reestablished, and the increased transcripts of representative senescence-associated genes, including DcbGal and DcGST1, were partly abolished. By contrast, the discussed responses were obviously blocked by the inhibition of endogenous H2S with hypotaurine, an H2S scavenger. These results clearly revealed that MgH2-supplying H2 could prolong the vase life of cut carnation flowers via H2S signaling, and our results, therefore, open a new window for the possible application of hydrogen-releasing materials in agriculture.
Introduction
Hydrogen is an ideal energy carrier that is being increasingly used in both power generation applications and transportation. Besides, hydrogen gas (H2) has been documented having a range of biological effects and gradually utilized in medicine and agriculture (Ohsawa et al., 2007; Xie et al., 2012; Zeng et al., 2013; Wu et al., 2019). Clearly, the storage of hydrogen is one of the key challenges in developing a hydrogen economy. The storage methods include pressurized gas, a cryogenic liquid, and solid fuel as chemically or physically combination with materials, such as metal hydrides (Sakintuna et al., 2007). At present, the supplementation of H2 for biological research includes a gas cylinder and water electrolysis, and H2 is normally dissolved in water and saline (Ohta, 2011; Xie et al., 2014; Li et al., 2018; Su et al., 2018). However, the extensive application of the hydrogen-rich liquid solution is limited due to the low solubility and short residence time of H2 in water. Fortunately, the growing development of solid hydrogen-storage materials may provide ways to improve the issues about production and storage of H2, considering portable, safety, large hydrogen contents, and sustainable hydrogen supply of solid-state storage (Hirscher et al., 2020).
Magnesium hydride (MgH2) stands as a promising hydrogen source because of its high hydrogen-storage capacity (7.6 wt%), abundant resources, and low cost (Grochala and Edwards, 2004). The research on applications of MgH2 and its related compounds has focused on thermal storage for solar power stations and hydrogen supply for vehicles (Bogdanović et al., 1995; Schlapbach and Zuttel, 2001; Baricco et al., 2017; Lototskyy et al., 2018; Hirscher et al., 2020). It is well documented that MgH2 can produce a desired quantity of H2 by the following hydrolysis reaction at room temperature: MgH2 + 2H2O → Mg(OH)2 + 2H2, the by-product of which is environmentally friendly. This property of MgH2 makes a possible for biological application. Amazingly, Kamimura et al. (2016) discovered that orally given MgH2 could increase the content of blood H2 and decrease the level of plasma triglyceride in rats, thus extending their average lifespan. These results indicated that MgH2 with biosafety might also have potential roles in medical applications.
In fact, there are two disadvantages of MgH2 restricting its further practical application: (1) the reaction kinetics of MgH2 hydrolysis is extremely slow in pure water; (2) the insoluble layer of magnesium hydroxide [Mg(OH)2] rapidly coated on the outer surface of the unreacted MgH2 to further hide reaction as the pH increases (Hiraki et al., 2012). Subsequently, some organic acids (including citric acid, ethylenediamine-tetraacetic acid, and tartaric acid) were found as good buffer agents to effectively accelerate the reaction, finally improving H2 generation by decreasing the pH and suppressing Mg(OH)2 formation (Hiraki et al., 2012; Chao, 2018). On the other hand, it is well-known that organic acid-induced decrease in pH of vase solutions inhibits bacterial growth and increases the water conduction in the xylem of cut flowers, thus prolonging the vase life (van Doorn, 2010).
The postharvest senescence of cut flowers results in significant commercial losses, which is closely associated with a series of signaling molecules, including ethylene (Kumar et al., 2008), reactive oxygen species (ROS; van Doorn and Woltering, 2008), nitric oxide (NO; Naing et al., 2017), and hydrogen sulfide (H2S; Zhang et al., 2011). Highly coordinated changes in gene expression are also involved (Shahri and Tahir, 2011). Many senescence-associated genes (SAGs) have been cloned from carnation petals, and their expression patterns were examined as well. For example, transcripts of representative genes encoding β-galactosidase (DcbGal) and glutathione-S-transferase (DcGST1), previously described as SR12 and SR8, are increased during flower senescence (Lawton et al., 1989; Meyer et al., 1991).
Recently, the usage of H2 in the form of hydrogen-rich water (HRW) was observed to delay postharvest senescence and improve the quality of cut flowers (Ren et al., 2017; Su et al., 2019; Wang et al., 2020). Subsequent biochemical analysis showed that H2 prolonged the vase life of cut rose and lily was mediated by maintaining water balance, increasing antioxidant defense, and prolonging cell membranes stability (Ren et al., 2017). Meanwhile, H2 can inhibit ethylene synthesis and corresponding signal transduction via regulating the expressions of related genes (such as ethylene synthesis genes Rh-ACS3 and Rh-ACO1 and ethylene receptor genes Rh-ETR1), thus delaying rose senescence during the vase period (Wang et al., 2020). In addition, H2-stimulated NO, another gaseous molecule, can act as a downstream signal molecule involving keeping postharvest freshness in cut lily (Huo et al., 2018). However, the effects of sustained hydrogen supply on prolonging the vase life of cut flowers and related mechanisms are still elusive.
In this study, we firstly aim to find an optimized condition for using MgH2 in the flower vase experiment. It was confirmed that the application of citrate buffer solution (CBS) could greatly accelerate the reaction rate of MgH2 hydrolysis, confirmed by the rapid and sustainable increased H2 generation, thus showing more efficiency in the residence time of H2 in solution, compared with HRW. By using pharmacological and molecular approaches, we discovered that the combined treatment of MgH2 and CBS could remarkably prolong the vase life of a cut carnation flower, compared with either treatment with MgH2 or HRW, or CBS alone. It is a new finding. Further results suggested that the discussed MgH2-CBS response is mediated by influencing H2S signaling. Together, this work will not only extend the application of MgH2 to agricultural practices but also provide a new idea for the development of new plant growth regulators.
Materials and Methods
Chemicals
All chemicals used in our experiments were purchased from Sigma-Aldrich (St. Louis, MO, United States) unless stated otherwise. MgH2 was obtained from the Center of Hydrogen Science, Shanghai Jiao Tong University (Ma et al., 2019). MgH2 was further characterized by using scanning electron microscopy (SU-8010, Hitachi, Tokyo, Japan), X-ray diffraction (D/MAX-Ultima III, Rigaku, Tokyo, Japan) with Cu K radiation source, differential scanning calorimetry (STA449F3, Netzsch, Selb, Germany), and thermogravimetry (TG209F3, Netzsch, Selb, Germany). In addition, sodium hydrosulfide (NaHS) and hypotaurine (HT) were used as an H2S releasing compound and a specific H2S-scavenger, respectively (Ortega et al., 2008). H2S fluorescent probe 3-oxo-3H-spiro[isobenzofuran-1,9’-xanthene]-3’,6’-diyl bis(2-(pyridin-2-yldisulfanyl)benzoate) (WSP-5; MKBio, Shanghai, China) was used to monitored endogenous H2S in cut flowers (Peng et al., 2014). The concentrations of these chemicals were selected based on the results of pilot experiments.
Plant Material and Treatments
Cut carnation “Pink Diamond” flowers at the typical commercial stage (the petals form a right angle with the stem axis) were purchased from a flower market in Nanjing City, Jiangsu Province, China, from July to September of 2019. They were transported within 1 h to the laboratory. Subsequently, the cut flower stems were placed in distilled water and re-cut underwater to a length of 25 cm. The top two leaves were kept as well.
The cut flower stems were incubated in glass bottles with 150-ml distilled water (control) and 0.1-M CBS (pH 3.4) containing 0.01, 0.1, and 1 g L–1 MgH2. Because the treatment with 0.1-M CBS (pH 3.4) plus 0.1 g L–1 MgH2 showed the most obvious effects on prolonging the vase life of a cut flower in a pilot experiment (Supplementary Figures 1A–C), this combined treatment was applied subsequently. Meanwhile, 0.1 g L–1 MgH2, 0.1 M CBS (pH 3.4), or 10% HRW (obtained by water electrolysis) alone was, respectively, regarded as controls, and HRW was prepared according to the previous method (Su et al., 2019).
To confirm the possibility that the effect of MgH2 was only due to molecular hydrogen and not associated with magnesium ion, MgH2-CBS solution was boiled for three times, 5 min each to remove the generated H2, followed by keeping under the normal temperature condition for 1 day until no H2 was detected.
Because 600-μM NaHS and 10-mM HT showed the obviously promoting and repressing effects on prolonging the vase life of a cut flower in pilot experiments, respectively (Supplementary Figures 1D,E), these treatments were also chosen. For further tests, the cut flower stems were incubated in treatment solutions (150 ml) containing distilled water (control), 0.1 g L–1 MgH2-CBS, 600-μM NaHS, or 10-mM HT, alone and in combination. For the entire tests, all stems were continuously kept in the treatment solutions throughout the vase period at 25 ± 2°C, 60–70% relative humidity, and 12 h per day of light (20 μmol m–2 s–1). All treatment solutions were renewed daily as well.
Determination of Hydrogen Gas Concentration
The concentration of H2 in solutions was measured by a portable dissolved hydrogen meter (ENH-1000, TRUSTLEX, Osaka, Japan) that was calibrated by gas chromatography (Su et al., 2019).
Vase Life, Relative Fresh Weight, and Flower Diameter
The vase life of each flower was calculated as the number of days from the day that the stems were placed in the vase solutions (recorded as day 0) until the day that 50% of petals had wilted or the stems had bent (bent-neck angle greater than 45°). During the vase period, the fresh weight of each sample was measured daily using an analytical balance. The relative fresh weight (RFW) was calculated as following: RFW% = (FWt/Fw0) × 100, where Wt is the fresh weight of the sample (g) at day t (t = 0, 1, 2, 3, etc.), and W0 is the fresh weight of the same sample (g) at day 0. Additionally, flower diameter was defined as the maximum width of each flower and measured daily using a digital caliper. In each experiment, 10 flowers were placed per treatment with three replications, and the means of the vase life, RFW, and flower diameter were determined.
Measurement of Endogenous Hydrogen Sulfide
With the aid of laser scanning confocal microscopy, H2S level in vivo was determined as described previously with minor modification (Kou et al., 2018). The petals were incubated with 20-μM WSP5 (an H2S fluorescent probe) in 20-mM 4-(2-hydroxyethyl)-1-piperazineethanesulfonic acid–sodium hydroxide buffer (pH 7.5) for 30 min in the dark (25°C). After three washes (10 min per time) with fresh 4-(2-hydroxyethyl)-1-piperazineethanesulfonic acid–sodium hydroxide buffer, the samples were observed using an LSM 710 microscope (Carl Zeiss, Oberkochen, Germany) with excitation at 495 nm and emission at 525 nm. The bright-field images were shown at the lower right corners of their corresponding fluorescent images. The relative fluorescence was presented as relative units of pixel intensities calculated by the ZEN software to the control samples. At least five sections per sample were determined, and three samples in each treatment were used.
Histochemical Staining and Corresponding Measurement of Hydrogen Peroxide Content
The hydrogen peroxide (H2O2) in petal was visually detected according to the method of Thordal-Christensen et al. (1997). The petals were stained with 0.1% 3,3-diaminobenzidine for 12 h at room temperature in the dark. Afterward, the petals were detected under a light microscope (Stemi 2000-C; Carl Zeiss, Germany).
The H2O2 content was measured by the spectrophotography (Mei et al., 2017). The samples were incubated with assay reagent (containing 50-mM H2SO4, 200-μM xylenol orange, and 200-mM sorbitol) for 45 min in the dark at 25°C. Then, the absorbance values were determined at 560 nm. A standard curve was obtained by adding a variable amount of H2O2.
Analysis of Senescence-Associated Genes Transcription
Quantitative real-time RT-PCR (qPCR) was used to analyze the expression of SAGs. Total RNA was extracted from petals using the SparkZol Reagent (SparkJade, Shandong, China). The concentration and quality of RNA were determined using a NanoDrop 2000 (Thermo Fisher Scientific, Wilmington, DE, United States), and RNA was treated with RNase-free DNase (TaKaRa Bio Inc., Dalian, China) to eliminate traces of DNA. Afterward, complementary DNAs were synthesized using HiScript III RT SuperMix (Vazyme, Nanjing, China). By using specific primers (Supplementary Table 1), qPCR was performed using a Mastercycler ep® realplex real-time PCR system (Eppendorf, Hamburg, Germany) with 2 × SYBR Green qPCR Mix (SparkJade, Shandong, China). Relative expression levels were calculated using the 2–ΔΔCT method (Livak and Schmittgen, 2001) and presented as values relative to the control samples (0 days) after the normalization with the transcript levels of an internal control gene DcActin.
Statistical Analysis
All values are means ± standard error (SE) of three independent experiments with three biological replicates for each. Data were analyzed by SPSS 22.0 software (IBM Corporation, Armonk, NY, United States). Differences among treatments were analyzed by one-way analysis of variance (ANOVA) followed by Duncan’s multiple range test or t-test, and P < 0.05 or 0.01 were considered as statistically significant.
Results
Characterization of Magnesium Hydride
As shown in the scanning electron microscopy images (Figure 1A), the as-received MgH2 particles are spherical with a diameter of 0.5–25 μm (mean diameter = 15 μm; Ma et al., 2019). The X-ray diffraction patterns (Figure 1B) confirmed that MgH2 is the majority phase with a small amount of unhydrided magnesium. The dehydriding properties of MgH2 were investigated by using differential scanning calorimetry and thermogravimetry. It was observed that the peak temperature of decomposition is 405°C at a heating rate of 10°C min–1 with a mass loss of about 7.2 wt% (Figure 1C).
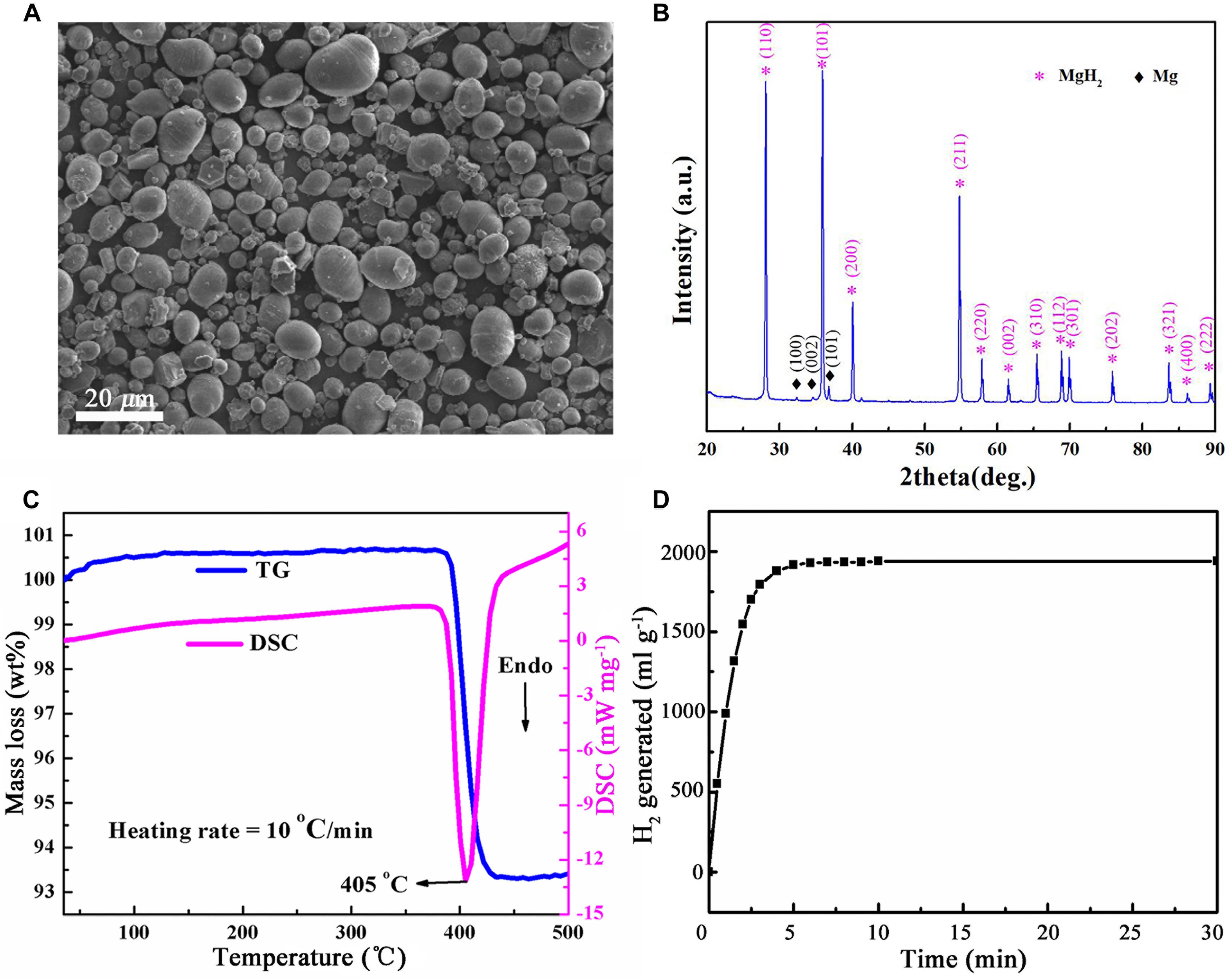
Figure 1. Characterization of MgH2 used in this work. (A) Scanning electron microscopy (SEM) micrographs of MgH2 (Scale bar = 20 μm). (B) X-ray diffraction (XRD) pattern of MgH2 powers. (C) Thermogravimetric (TG) and differential scanning calorimetry (DSC) curves of MgH2. (D) H2 generated from hydrolysis of MgH2.
The amount of H2 generated from complete hydrolysis of MgH2 was about 1,800 ml g–1 (Figure 1D); namely, the concentration of H2 in unit volume (1 m3) was 0.18% (v v–1). It is not flammable and explosive when the H2 concentration is less than 4% by volume (lower flammability limit of H2). Thus, it is generally safe by using MgH2 as a vase regent.
Magnesium Hydride–Citrate Buffer Solution Prolongs the Vase Life of Cut Carnation Flowers
In our experimental conditions, when 0.1 g L–1 MgH2 was dissolved in 0.1-M CBS (pH 3.4), this combined treatment (also abbreviated as MgH2-CBS in the following experiments) was observed as the most obvious effect on prolonging the vase life of carnation cut flowers, compared with different doses of MgH2, various CBS, or 10% HRW alone (Supplementary Figures 1A–C and Figures 2A,B). In the presence of 0.1 g L–1 MgH2-CBS (0.1 M, pH 3.4), for example, the vase life of the fresh cut flowers was the longest among all the treatment and was 11.4 days, which prolonged 3.9 days compared with the control, which was also significantly different from the treatments of 0.1 g L–1 MgH2 (prolonged about 2.0 days), 0.1-M CBS (pH 3.4; about 1.6 days), or 10% HRW (about 1.5 days) alone. This conclusion correlates with the data from other phenotypic parameters, including RFW and flower diameter in carnation (Figures 2C,D). By contrast, the removal of H2 by heating solution impaired the positive effects of MgH2-CBS. It was also confirmed that the boiling used in our experiment was sufficient to remove H2 from solutions (Figure 2E), thus suggesting the function of MgH2-CBS is H2-dependent.
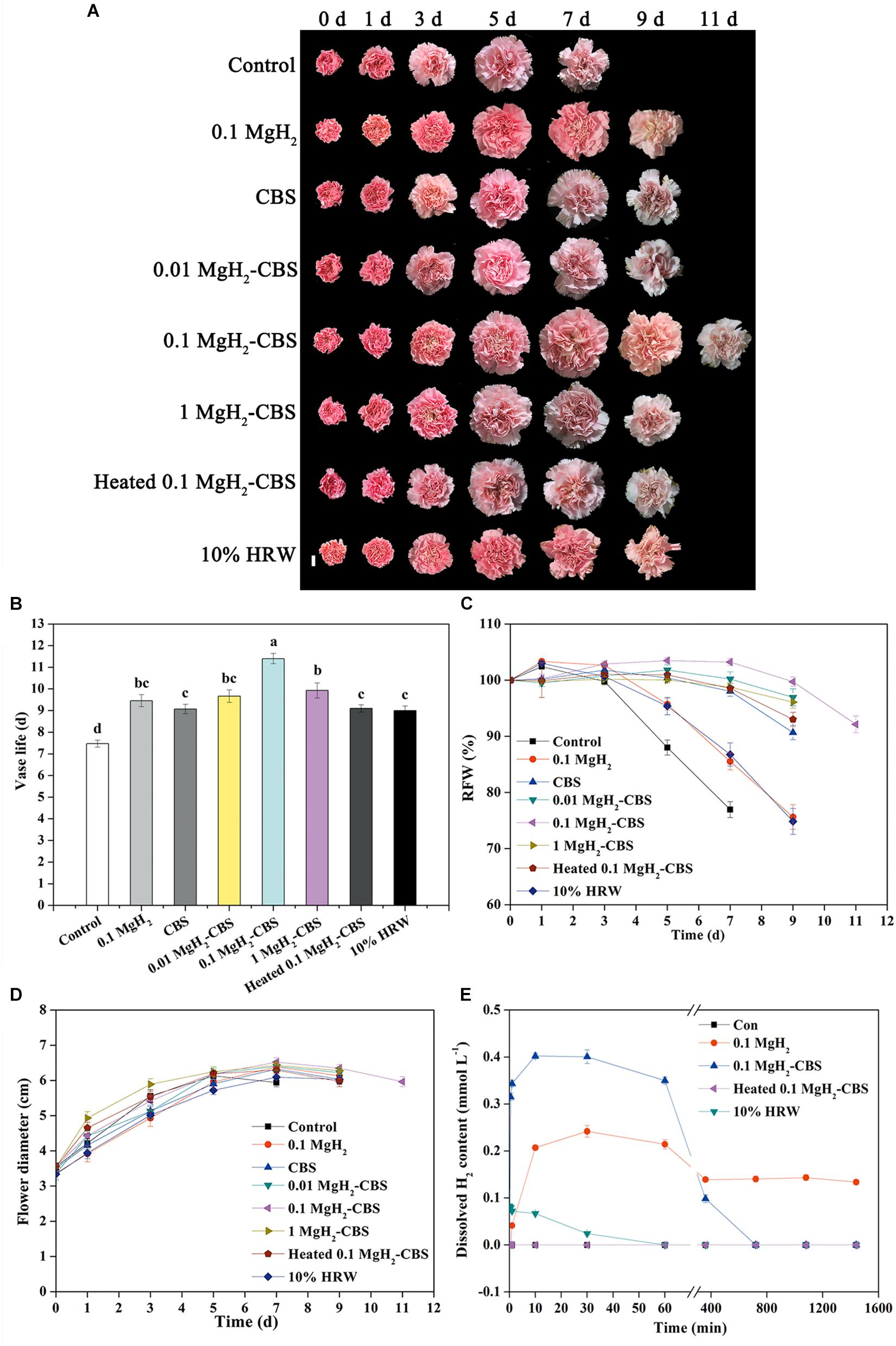
Figure 2. Changes in vase life, relative fresh weight (RFW), and flower diameter of cut carnations and dissolved H2 in solution subjected to MgH2, citrate buffer solution (CBS), MgH2-CBS, heated MgH2-CBS, and hydrogen-rich water (HRW). (A) Representative photographs of cut flowers (scale bar = 2 cm). Cut flower stems were incubated in untreated (control) and treatment solutions containing 0.1 g L–1 MgH2, 0.1-M CBS (pH 3.4) with or without 0.01, 0.1, and 1 g L–1 MgH2, 10% electrolytic HRW during vase period. Afterward, vase life (B), RFW (C), maximum flower diameter (D), and H2 content in solutions (E) were expressed as mean ± standard error (SE). There were three replicates and 10 flowers per each for (A–D), and three replicates per each for (E). Experiments were conducted for three times. Bars with different letters are significantly different (P < 0.05), as determined by Duncan’s multiple range test.
Consistently, the contents of dissolved H2 in MgH2-CBS and 0.1 g L–1 MgH2 solutions ranked the first and second (rapidly peaking at 0.80 and 0.48 ppm) and remained in higher levels until 6 and 12 h, respectively. Meanwhile, H2 existing in 10% HRW progressively decreased, just from an initial 0.16 ppm to the basal level after 6 h (Figure 2E).
Hydrogen Sulfide Is Involved in Magnesium Hydride–Citrate Buffer Solution-Prolonged Vase Life of Cut Carnation Flowers
To investigate whether H2S is involved in MgH2-CBS-prolonged vase life of carnation cut flowers, both MgH2-CBS and HT (a specific H2S scavenger; Ortega et al., 2008) were applied alone and in combination. Meanwhile, NaHS (an H2S releasing compound) was used as a positive control. The response of the endogenous H2S level in the petal was monitored by labeling H2S using an H2S-specific fluorescent probe (WSP-5; Peng et al., 2014) and imaging by laser scanning confocal microscopy (Kou et al., 2018). As shown in Figure 3, the WSP-5-dependent fluorescent intensity was increased by NaHS but was greatly impaired by HT. In addition, HT alone decreased fluorescent intensity in comparison with the chemical-free control. It was confirmed that some, if not most, of the WSP-5-related fluorescence is caused by H2S. Further results demonstrated that MgH2-CBS significantly increased endogenous H2S production. Consistently, the inducing effect achieved by MgH2-CBS could be prevented by HT. Moreover, there was no additive response in fluorescence when MgH2-CBS was added together with NaHS.
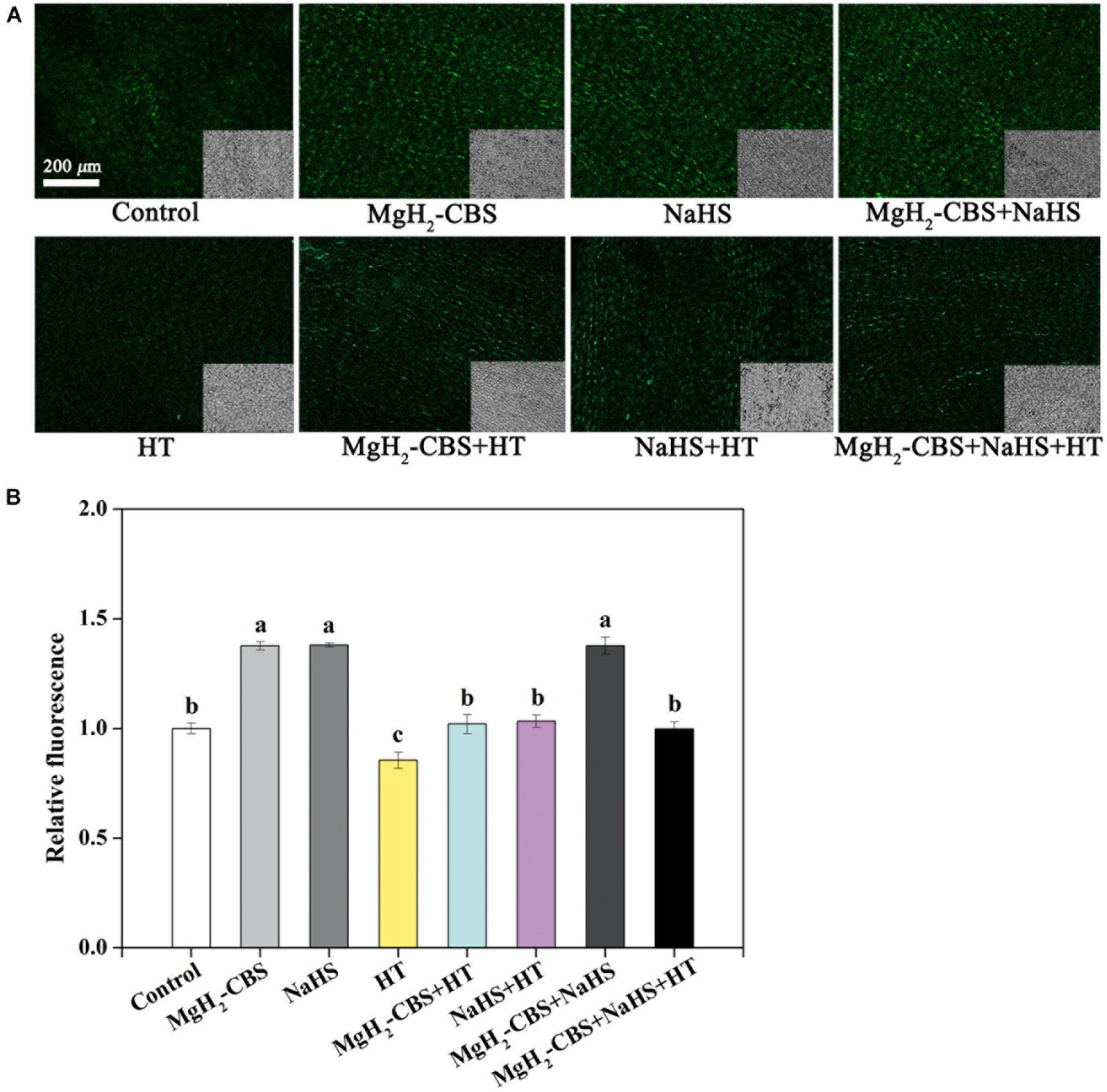
Figure 3. MgH2-CBS triggers H2S accumulation. (A) Cut flower stems were incubated in untreated (control) and treatment solutions containing 0.1 g L–1 MgH2-CBS, 600-μM NaHS, 10-mM HT (a scavenger of H2S), alone or their combinations for 3 days. Afterward, epidermis of petals was loaded with 20-μM WSP5 (an H2S fluorescent probe) and detected by laser scanning confocal microscopy (scale bar = 200 μm). Bright-field images corresponding to the fluorescent images were at the bottom right corner. (B) Relative fluorescence was also presented as values relative to control. Mean and SE values were calculated. At least five sections per sample were determined, and three samples in each treatment were used. Bars with different letters denoted significant differences in comparison with control at P < 0.05, according to Duncan’s multiple range test.
The subsequent experiment was to assess the contribution of H2S in prolonging carnation vase-life achieved by MgH2-CBS. Consistently, three parameters, in terms of vase life, RFW, and flower diameter, were used. As expected, compared with the responses of NaHS, the prolonged vase life of cut carnation flowers was intensified in the presence of MgH2-CBS, which was abolished when HT was added (Figure 4). In contrast, compared with control, HT alone shortened the vase life. However, MgH2-CBS co-treated with NaHS cannot result in an additive extension of carnation vase-life. Correlating with the changes in endogenous H2S production (Figure 3), the results indicated that endogenous H2S might participate in MgH2-CBS-prolonged the vase life of cut carnation flowers.
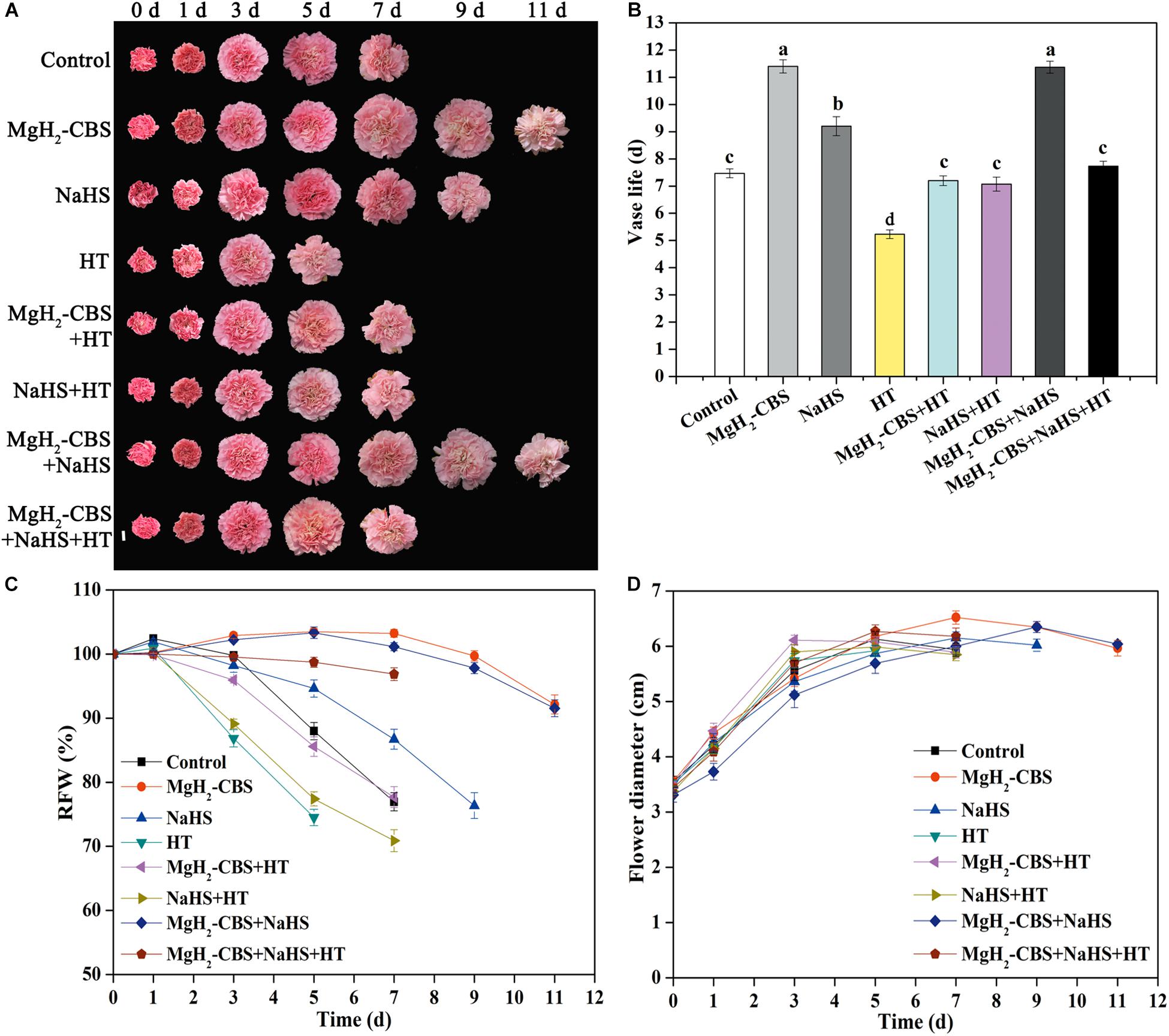
Figure 4. MgH2-CBS-prolonged vase life of cut carnation flowers is sensitive to the scavenger of H2S. (A) Cut flower stems were incubated in untreated (control) and treatment solutions containing 0.1 g L–1 MgH2-CBS, 600-μM NaHS, 10-mM HT (a scavenger of H2S), alone or their combinations throughout the vase period. Representative photographs of cut flowers were taken (scale bar = 2 cm). Vase life (B), relative fresh weight (RFW; C), and maximum flower diameter (D) were expressed as mean and SE values. There were three replicates and 10 flowers per each. Experiments were conducted for three times. Bars with different letters are significantly different (P < 0.05), as determined by Duncan’s multiple range test.
Magnesium Hydride–Citrate Buffer Solution Maintains Redox Homeostasis via Hydrogen Sulfide
Histochemical staining of ROS (H2O2) accumulation was then adopted to reveal the detailed mechanism underlying MgH2-CBS-prolonged carnation vase-life. As expected, it was observed that a gradual increase of 3,3-diaminobenzidine-dependent staining in the control during the vase period (Figure 5A). The change of endogenous H2O2 level determined with spectrophotography displayed a similar tendency (Figure 5B), indicating that redox homeostasis was disrupted during senescence.
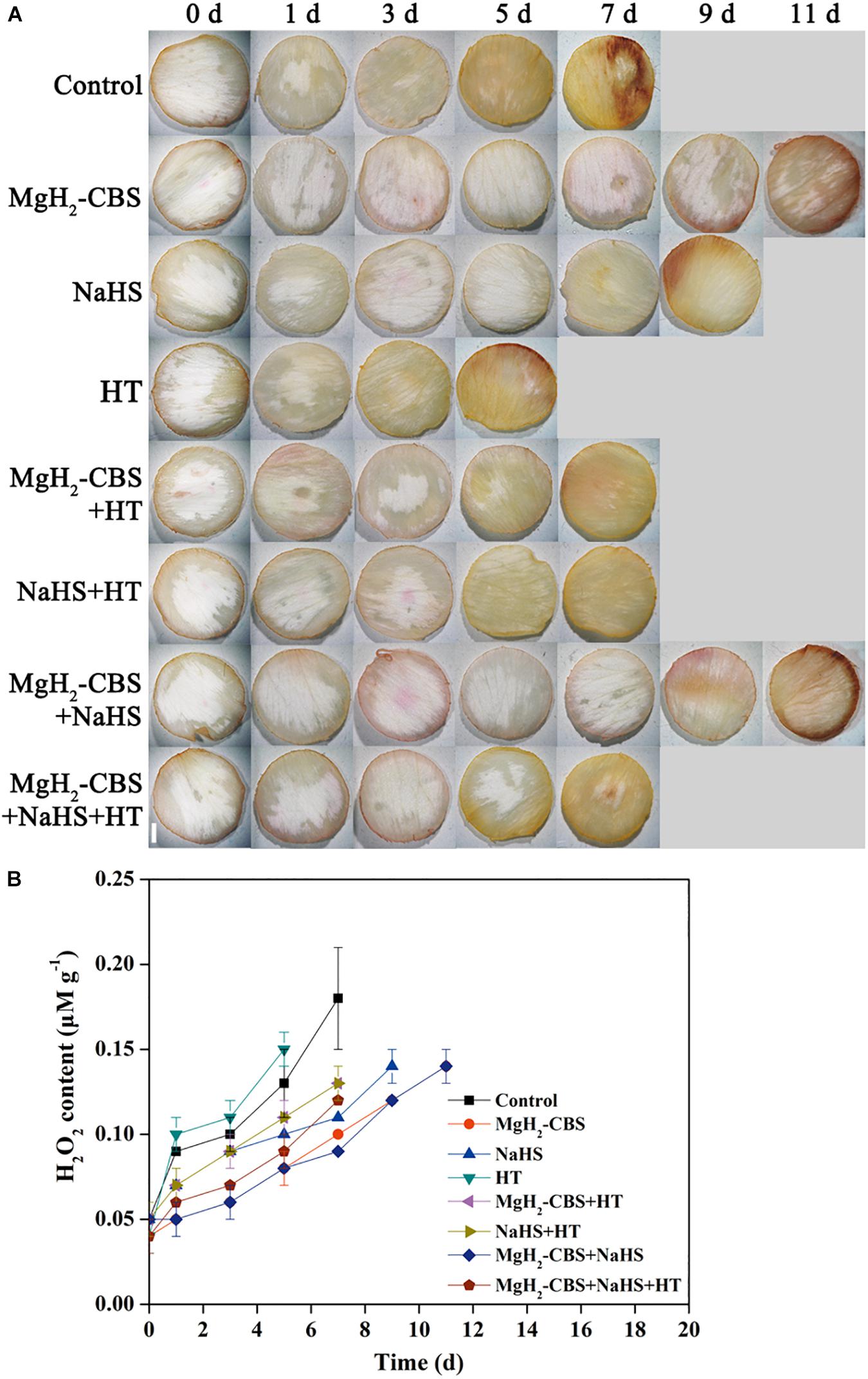
Figure 5. MgH2-CBS maintains redox homeostasis via H2S. (A) Cut flower stems were incubated in solutions containing 0.1 g L–1 MgH2-CBS, 600-μM NaHS, 10-mM HT, alone or their combinations throughout the vase period. The petals were stained with 3,3-diaminobenzidine (DAB), then photographed under a light microscope (scale bar = 1 mm). (B) Spectrophotography also determined H2O2 contents. Values are mean ± SE of three independent experiments with three replicated for each.
Compared with the control, the treatments with MgH2-CBS and NaHS individually resulted in slight staining patterns (Figure 5A). By contrast, the mentioned responses elicited by MgH2-CBS, and NaHS was reversed by the removal of endogenous H2S when HT was applied. Alone, HT brought out extensive straining compared with the control (5 days). No additive responses were observed in MgH2-CBS plus NaHS. Meanwhile, changes in endogenous H2O2 contents showed similar patterns (Figure 5B). These results suggested that MgH2-CBS could reestablish redox homeostasis in carnation flowers, which might be mediated by H2S.
Role of Hydrogen Sulfide in Magnesium Hydride–Citrate Buffer Solution-Modulated Senescence-Associated Genes During Postharvest Senescence
To further elucidate the molecular mechanism of how H2S is involved in MgH2-CBS-prolonged carnation vase-life, several molecular probes responsible for senescence, including DcbGal and DcGST1, were analyzed by qPCR. The time-course experiment showed that the expression levels of DcbGal and DcGST1 were increased during postharvest senescence, and those in petals of control were much higher than those in the presence of MgH2-CBS (Figures 6A,B). Similar to the responses of H2S, MgH2-CBS could also downregulate the transcripts of DcbGal and DcGST1 (5 days; Figures 6C,D). In contrast, the inhibition mentioned earlier was attenuated by the depletion of H2S with HT. Additionally, HT alone could greatly increase the expression levels of these two genes. No additive inhibition responses occurred in co-treatment of MgH2-CBS and H2S as well. Therefore, H2S was involved in MgH2-CBS-induced reduction of DcbGal and DcGST1 expression in carnation during the vase period.
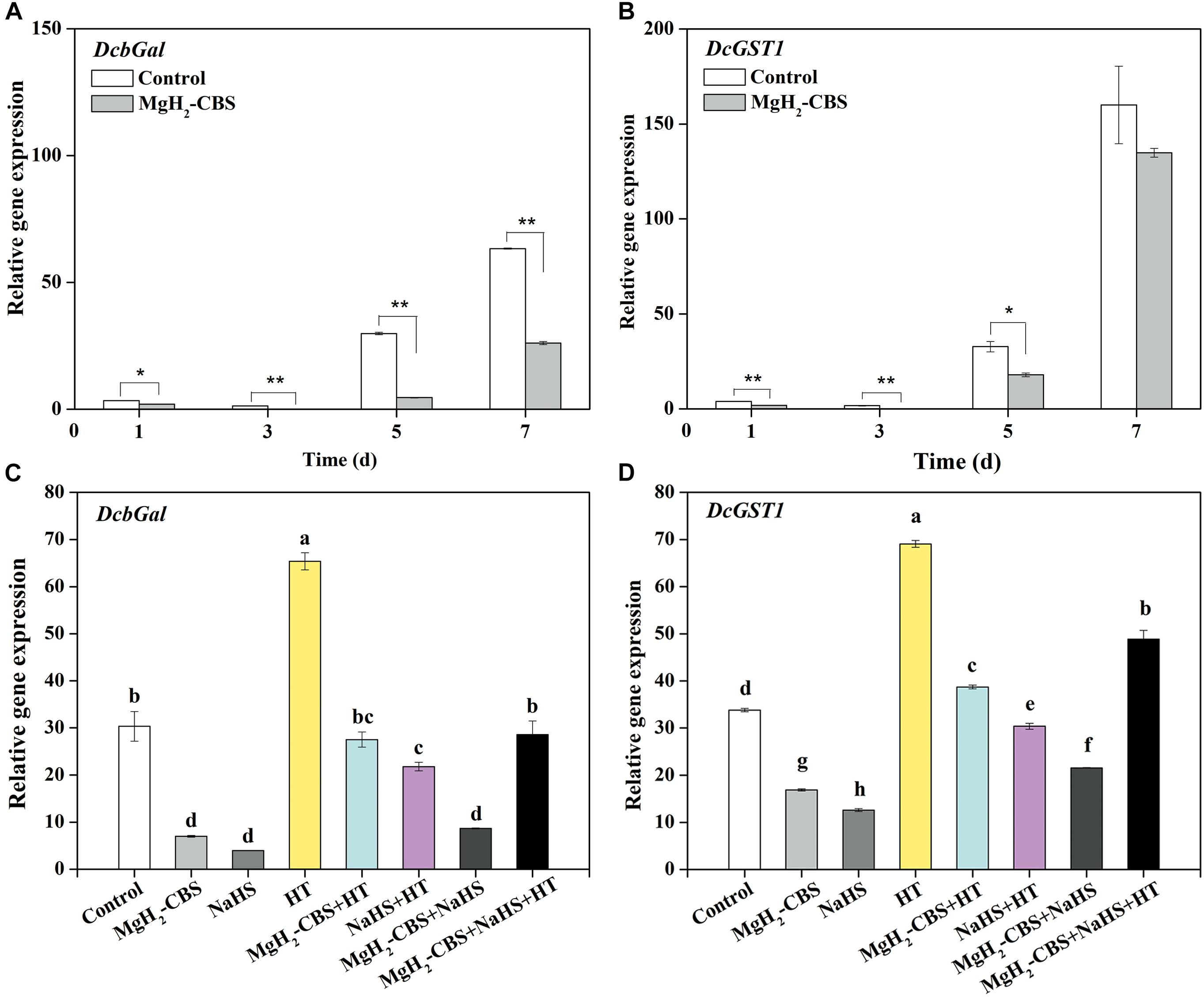
Figure 6. Changes in the transcripts of senescence-associated genes. Cut flower stems were incubated in solutions containing 0.1 g L–1 MgH2-CBS, 600-μM NaHS, 10-mM HT, alone or their combinations throughout the vase period. After treatments for the indicated time points or 5 days, the transcript levels of DcbGal (A,C) and DcGST1 (B,D) in petals were analyzed by qPCR and presented as values relative to the control samples (0 days) after the normalization with the transcript levels of an internal control gene DcActin. Values are mean ± SE of three independent experiments with three replicated for each. Bars with asterisks were significantly different in comparison with control at *P < 0.05 and **P < 0.01 according to t-test. Bars with different letters are significantly different (P < 0.05), as determined by Duncan’s multiple range test.
Discussion
At present, HRW is a major route of H2 administration (Shen and Sun, 2019). Ample evidence showed that HRW has positive effects on postharvest physiology. For example, HRW can prolong the shelf life (Hu et al., 2014) and decrease nitrite accumulation of fruits during storage (Zhang et al., 2019), as well as prolong the vase life of cut flowers (Ren et al., 2017; Su et al., 2019; Wang et al., 2020). Importantly, the HRW is presently mainly obtained by water electrolysis, which requires a hydrogen gas generator. Moreover, the solubility of H2 in water is very low (approximately 1.84 ml in 100-g H2O at 20°C, 1 atm; Safonov and Khitrin, 2013), and especially, the residence time of H2 in HRW is shorter, as the half-time of dissolved H2 in HRW is less than 1 h (Figure 2E), at least under our experimental conditions. The discussed disadvantages may restrict the practical applications of the electrolytic produced HRW.
In this study, H2 was generated by MgH2 hydrolysis, which was intensified when dissolved in CBS. Additionally, it can remain in higher amounts of dissolved H2 over a relatively longer period than the electrolytic HRW (Figure 2E). It has been reported that hydrolysis of magnesium particles can produce hydrogen nanobubbles that can exist in the water solution of a dietary supplement for a sufficiently long time (Bunkin et al., 2009; Safonov and Khitrin, 2013). A balance between surface tension and repulsive forces between surface electric charges is responsible for the stabilization of nanobubbles (Bunkin et al., 2009). We also found that the dissolution of MgH2 in water and CBS (in particular) was accompanied by a large number of small bubbles in the first 1–2 min. Thus, MgH2 may also produce hydrogen nanobubbles that increase the solubility and the residence time of H2. However, the dissolution of MgH2 in water led to a strongly alkaline environment (approximately pH 10; Supplementary Figure 1F). By contrast, the administration with CBS significantly accelerated the reaction of MgH2 hydrolysis and increased H2 generation (Figure 2E) by decreasing the pH, which is consistent with the previous studies (Hiraki et al., 2012; Chao, 2018).
It is worth noting the safety of MgH2 use. In fact, the concentration of H2 generated from MgH2 hydrolysis is far less than the lower flammability limit of H2 (4% in air). Therefore, it is safe by using MgH2 as a vase regent. It has been reported that the citric acid buffered around pH 3 can effectively prolong the vase life of cut flowers by reducing bacterial growth and maintaining the water balance (van Doorn, 2010). A similar result was observed in this study (Supplementary Figures 1B,C and Figures 2A,B). Although the combination of MgH2 and acid solutions is impractical for industry application because it causes equipment corrosion, it precisely favors postharvest preservation. We also observed that combining MgH2 with CBS may produce additive or synergistic effects in prolonging the vase life of cut carnation flowers. Together, MgH2 might be used as a promising chemical for producing a hydrogen-rich solution in horticulture.
H2S is a well-known important gaseous signaling molecule involved in plant developmental and environmental responses, such as root organogenesis, response to abiotic stresses, and delayed senescence of vegetables, fruits, and flowers (Zhang et al., 2011; Li et al., 2012, 2013; Wang et al., 2012; Ali et al., 2019; Corpas, 2019; Mei et al., 2019). It has been confirmed that L-cysteine desulfhydrase-dependent H2S acts as the downstream signal molecule involved in NO-induced heat tolerance of maize seedlings (Li et al., 2013) and methane-induced tomato and Arabidopsis lateral root formation (Mei et al., 2019). Interestingly, a similar requirement of H2S for MgH2-prolonged vase life of cut carnation flowers was discovered in this work. The conclusion is supported by the following pharmacologic and molecular evidence.
HT, a scavenger of H2S (Ortega et al., 2008; Fang et al., 2014; Mei et al., 2019), was used in our experiments, and its inhibitory role was confirmed. The increase in endogenous H2S accumulation triggered by MgH2-CBS was observed to be sensitive by HT (Figure 3). Correlating with the changes in the phenotypes of vase life, relative fresh weight, and flower diameter (Figure 4), the results presented here further revealed a requirement for endogenous H2S in MgH2-CBS-prolonged carnation vase-life.
Furthermore, ROS (especially H2O2) has been observed to increasingly produce during the senescence process in cut flower (Hossain et al., 2006; Kumar et al., 2007; Su et al., 2019). It has been demonstrated that H2S could inhibit ROS overproduction by increasing activities of antioxidant enzymes (Zhang et al., 2011; Hu et al., 2012, 2015). In this study, the contents of H2O2 gradually increased during the normal senescence of cut carnation flowers, which indicated the disruption of redox homeostasis (Figure 5B). The lower H2O2 levels maintained by MgH2-CBS might be, at least partially, responsible for delaying senescence. By contrast, the discussed responses of MgH2-CBS were reversed by the removal of endogenous H2S with HT (Figure 5B). Changes in histochemical staining showed a similar pattern (Figure 5A). The discussed results, therefore, confirmed that MgH2-CBS-reestablished redox homeostasis was closely associated with the alteration in endogenous H2S.
Recent evidence proved that H2S decreased the expression levels of SAGs, resulting in delaying the postharvest senescence of broccoli (Li et al., 2014). Furthermore, sucrose and silver thiosulphate (an inhibitor of ethylene receptor) could repress the upregulation of SAGs (including DcbGal and DcGST) in petals of carnation (Hoeberichts et al., 2007). Similarly, our further molecular data revealed that MgH2-CBS could downregulate the expression of DcbGal and DcGST (Figure 6). By contrast, such inhibition effects of MgH2-CBS were alleviated by HT. Combined with the changes in phenotypes and endogenous H2S level (Figures 3, 4), we also speculated that SAGs might be the target genes responsible for MgH2-CBS-triggered H2S-prolonged vase life of cut flowers.
Accordingly, a schematic model shown in Figure 7 summarizes the role of H2S in the MgH2-CBS-prolonged the vase life of cut carnation flowers.
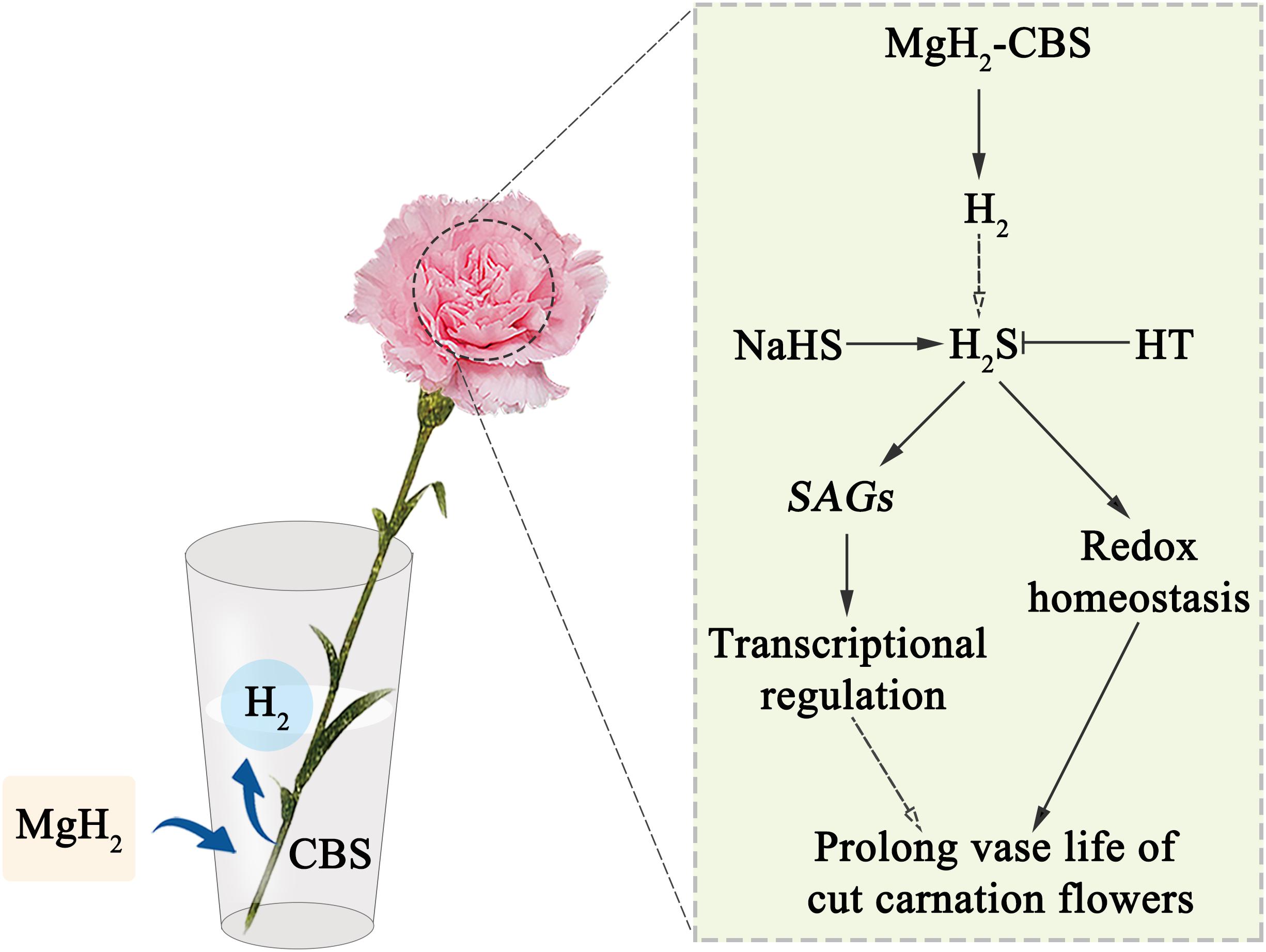
Figure 7. Schematic model summarizing the MgH2-CBS-prolonged the vase life of cut carnation flowers. CBS, citrate buffer solution; HT, hypotaurine; H2, hydrogen gas; H2S, hydrogen sulfide; MgH2, magnesium hydride; MgH2-CBS, magnesium hydride dissolved in citrate buffer solution; NaHS, sodium hydrosulfide; SAGs, senescence-associated genes.
Conclusion
This study revealed the effectiveness of MgH2-mediated H2 sustainable supply in postharvest preservation of cut flowers. Compared with hydrogen-rich water, the utilization efficiency of MgH2 was improved by buffering with CBS. Thus, MgH2 may have great potential for application in horticulture. In addition, it also demonstrated a vital role of H2S in MgH2-CBS-prolonged the vase life of cut flowers by modulating the expression of SAGs.
Data Availability Statement
All datasets generated for this study are included in the article/Supplementary Material, further inquiries can be directed to the corresponding author.
Author Contributions
WS and LL conceived and designed the research. LL, YL, and SW performed the experiments and analyzed the data. JZ and WD provided advice and materials for these experiments. LL, YL, SW, and WS wrote and revised the manuscript. All authors contributed to the article and approved the submitted version.
Funding
The work was financially supported by the funding from the Center of Hydrogen Science, Shanghai Jiao Tong University, China, and the Foshan Agriculture Science and Technology Project (Foshan City budget no. 140, 2019).
Conflict of Interest
The authors declare that the research was conducted in the absence of any commercial or financial relationships that could be construed as a potential conflict of interest.
Acknowledgments
We would like to thank Evan Evans (University of Tasmania; dGFzc2llYmVlcmRyQGdtYWlsLmNvbQ==) for the English editing of this manuscript.
Supplementary Material
The Supplementary Material for this article can be found online at: https://www.frontiersin.org/articles/10.3389/fpls.2020.595376/full#supplementary-material
References
Ali, S., Nawaz, A., Ejaz, S., Haider, S. T., Alam, M. W., and Javed, H. U. (2019). Effects of hydrogen sulfide on postharvest physiology of fruits and vegetables: an overview. Sci. Hortic. 243, 290–299. doi: 10.1016/j.scienta.2018.08.037
Baricco, M., Bang, M., Fichtner, M., Hauback, B., Linder, M., Luetto, C., et al. (2017). SSH2S: hydrogen storage in complex hydrides for an auxiliary power unit based on high temperature proton exchange membrane fuel cells. J. Power Sourc. 342, 853–860. doi: 10.1016/j.jpowsour.2016.12.107
Bogdanović, B., Ritter, A., Spliethoff, B., and Straβburger, K. (1995). A process steam generator based on the high temperature magnesium hydride/magnesium heat storage system. Int. J. Hydrogen Energ. 20, 811–822. doi: 10.1016/0360-3199(95)00012-3
Bunkin, N. F., Suyazov, N. V., Shkirin, A. V., Ignatiev, P. S., and Indukaev, K. V. (2009). Nanoscale structure of dissolved air bubbles in water as studied by measuring the elements of the scattering matrix. J. Chem. Phys. 130:476. doi: 10.1063/1.3095476
Chao, C. (2018). “Clinical applications of magnesium hydride,” in Magnesium Alloys - Selected Issue, eds T. Tański, W. Borek, and M. Król (London: IntechOpen), 115–128. doi: 10.5772/intechopen.79507
Corpas, F. J. (2019). Hydrogen sulfide: a new warrior against abiotic stress. Trends Plant Sci. 24, 983–988. doi: 10.1016/j.tplants.2019.08.003
Fang, T., Cao, Z., Li, J., Shen, W., and Huang, L. (2014). Auxin-induced hydrogen sulfide generation is involved in lateral root formation in tomato. Plant Physiol. Biochem. 76, 44–51. doi: 10.1016/j.plaphy.2013.12.024
Grochala, W., and Edwards, P. P. (2004). Thermal decomposition of the non-interstitial hydrides for the storage and production of hydrogen. Chem. Rev. 104, 1283–1315. doi: 10.1021/cr030691s
Hiraki, T., Hiroi, S., Akashi, T., Okinaka, N., and Akiyama, T. (2012). Chemical equilibrium analysis for hydrolysis of magnesium hydride to generate hydrogen. Int. J. Hydrogen Energ. 37, 12114–12119. doi: 10.1016/j.ijhydene.2012.06.012
Hirscher, M., Yartys, V. A., Baricco, M., Bellosta Von Colbe, J., Blanchard, D., Bowman, R. C., et al. (2020). Materials for hydrogen-based energy storage-past, recent progress and future outlook. J. Alloy Compd. 827:153548. doi: 10.1016/j.jallcom.2019.153548
Hoeberichts, F. A., van Doorn, W. G., Vorst, O., Hall, R. D., and van Wordragen, M. F. (2007). Sucrose prevents up-regulation of senescence-associated genes in carnation petals. J. Exp. Bot. 58, 2873–2885. doi: 10.1093/jxb/erm076
Hossain, Z., Mandal, A., Datta, S. K., and Biswas, A. K. (2006). Decline in ascorbate peroxidase activity-a prerequisite factor for tepal senescence in gladiolus. J. Plant Physiol. 163, 186–194. doi: 10.1016/j.jplph.2005.03.004
Hu, H., Li, P., Wang, Y., and Gu, R. (2014). Hydrogen-rich water delays postharvest ripening and senescence of kiwifruit. Food Chem. 156, 100–109. doi: 10.1016/j.foodchem.2014.01.067
Hu, H., Liu, D., Li, P., and Shen, W. (2015). Hydrogen sulfide delays leaf yellowing of stored water spinach (Ipomoea aquatica) during dark-induced senescence by delaying chlorophyll breakdown, maintaining energy status and increasing antioxidative capacity. Postharvest Biol. Technol. 108, 8–20. doi: 10.1016/j.postharvbio.2015.05.003
Hu, L., Hu, S., Wu, J., Li, Y., Zheng, J., Wei, Z., et al. (2012). Hydrogen sulfide prolongs postharvest shelf life of strawberry and plays an antioxidative role in fruits. J. Agric. Food Chem. 60, 8684–8693. doi: 10.1021/jf300728h
Huo, J., Huang, D., Zhang, J., Fang, H., Wang, B., Wang, C., et al. (2018). Comparative proteomic analysis during the involvement of nitric oxide in hydrogen gas-improved postharvest freshness in cut lilies. Int. J. Mol. Sci. 19:3955. doi: 10.3390/ijms19123955
Kamimura, N., Ichimiya, H., Iuchi, K., and Ohta, S. (2016). Molecular hydrogen stimulates the gene expression of transcriptional coactivator PGC-1α to enhance fatty acid metabolism. NPJ Aging Mech. Dis. 2:16008. doi: 10.1038/npjamd.2016.8
Kou, N., Xiang, Z., Cui, W., Li, L., and Shen, W. (2018). Hydrogen sulfide acts downstream of methane to induce cucumber adventitious root development. J. Plant Physiol. 228, 113–120. doi: 10.1016/j.jplph.2018.05.010
Kumar, N., Srivastava, G. C., and Dixit, K. (2007). Role of superoxide dismutases during petal senescence in rose (Rosa hybrida L.). J. Hortic. Sci. Biotechnol. 82, 673–678. doi: 10.1080/14620316.2007.11512290
Kumar, N., Srivastava, G. C., and Dixit, K. (2008). Hormonal regulation of flower senescence in roses (Rosa hybrida L.). Plant Growth Regul. 55, 65–71. doi: 10.1007/s10725-008-9259-6
Lawton, K. A., Huang, B., Goldsbrough, P. B., and Woodson, W. R. (1989). Molecular cloning and characterization of senescence-related genes from carnation flower petals. Plant Physiol. 90, 690–696. doi: 10.1104/pp.90.2.690
Li, H., Bai, G., Ge, Y., Zhang, Q., Kong, X., Meng, W., et al. (2018). Hydrogen-rich saline protects against small-scale liver ischemia-reperfusion injury by inhibiting endoplasmic reticulum stress. Life Sci. 194, 7–14. doi: 10.1016/j.lfs.2017.12.022
Li, L., Wang, Y., and Shen, W. (2012). Roles of hydrogen sulfide and nitric oxide in the alleviation of cadmium-induced oxidative damage in alfalfa seedling roots. Biometals 25, 617–631. doi: 10.1007/s10534-012-9551-9
Li, S., Hu, K., Hu, L., Li, Y., Jiang, A., Xiao, F., et al. (2014). Hydrogen sulfide alleviates postharvest senescence of broccoli by modulating antioxidant defense and senescence-related gene expression. J. Agric. Food Chem. 62, 1119–1129. doi: 10.1021/jf4047122
Li, Z., Yang, S., Long, W., Yang, G., and Shen, Z. (2013). Hydrogen sulphide may be a novel downstream signal molecule in nitric oxide-induced heat tolerance of maize (Zea mays L.) seedlings. Plant Cell Environ. 36, 1564–1572. doi: 10.1111/pce.12092
Livak, K. J., and Schmittgen, T. D. (2001). Analysis of relative gene expression data using real-time quantitative PCR and the 2−ΔΔCT method. Methods 25, 402–408. doi: 10.1006/meth.2001.1262
Lototskyy, M., Nyallang, N. S., Pasupathi, S., Wærnhus, I., Vik, A., Ilea, C., et al. (2018). A concept of combined cooling, heating and power system utilising solar power and based on reversible solid oxide fuel cell and metal hydrides. Int. J. Hydrogen Energ. 43, 1865–1866. doi: 10.1016/j.ijhydene.2018.05.075
Ma, Z., Zou, J., Hu, C., Zhu, W., Khan, D., Zeng, X., et al. (2019). Effects of trimesic acid-Ni based metal organic framework on the hydrogen sorption performances of MgH2. Int. J. Hydrogen Energ. 44, 29235–29248. doi: 10.1016/j.ijhydene.2019.01.288
Mei, Y., Chen, H., Shen, W., Shen, W., and Huang, L. (2017). Hydrogen peroxide is involved in hydrogen sulfide-induced lateral root formation in tomato seedlings. BMC Plant Biol. 17:162. doi: 10.1186/s12870-017-1110-7
Mei, Y., Zhao, Y., Jin, X., Wang, R., Xu, N., Hu, J., et al. (2019). L-Cysteine desulfhydrase-dependent hydrogen sulfide is required for methane-induced lateral root formation. Plant Mol. Biol. 99, 283–298. doi: 10.1007/s11103-018-00817-3
Meyer, J. R. C., Goldsbrough, P. B., and Woodson, W. R. (1991). An ethylene-responsive flower senescence-related gene from carnation encodes a protein homologous to glutathione S-transferases. Plant Mol. Biol. 17, 277–281. doi: 10.1007/BF00039505
Naing, A. H., Lee, K., Arun, M., Lim, K. B., and Kim, C. K. (2017). Characterization of the role of sodium nitroprusside (SNP) involved in long vase life of different carnation cultivars. BMC Plant Biol. 17:149. doi: 10.1186/s12870-017-1097-0
Ohsawa, I., Ishikawa, M., Takahashi, K., Watanabe, M., Nishimaki, K., Yamagata, K., et al. (2007). Hydrogen acts as a therapeutic antioxidant by selectively reducing cytotoxic oxygen radicals. Nat. Med. 13, 688–694. doi: 10.1038/nm1577
Ohta, S. (2011). Recent progress toward hydrogen medicine: potential of molecular hydrogen for preventive and therapeutic applications. Curr. Pharm. Design 17:2241. doi: 10.2174/138161211797052664
Ortega, J. A., Ortega, J. M., and Julian, D. (2008). Hypotaurine and sulfhydryl-containing antioxidants reduce H2S toxicity in erythrocytes from a marine invertebrate. J. Exp. Biol. 211, 3816–3825. doi: 10.1242/jeb.021303
Peng, B., Chen, W., Liu, C., Rosser, E. W., Pacheco, A., Zhao, Y., et al. (2014). Fluorescent probes based on nucleophilic substitution-cyclization for hydrogen sulfide detection and bioimaging. Chem. Eur. 20, 1010–1016. doi: 10.1002/chem.201303757
Ren, P., Jin, X., Liao, W., Wang, M., Niu, L., Li, X., et al. (2017). Effect of hydrogen-rich water on vase life and quality in cut lily and rose flowers. Hortic. Environ. Biotechnol. 58, 576–584. doi: 10.1007/s13580-017-0043-2
Safonov, V. L., and Khitrin, A. K. (2013). Hydrogen nanobubbles in a water solution of dietary supplement. Colloid Surf. A 436, 333–336. doi: 10.1016/j.colsurfa.2013.06.043
Sakintuna, B., Lamaridarkrim, F., and Hirscher, M. (2007). Metal hydride materials for solid hydrogen storage: a review. Int. J. Hydrogen Energ. 32, 1121–1140. doi: 10.1016/j.ijhydene.2006.11.022
Schlapbach, L., and Zuttel, A. (2001). Hydrogen-storage materials for mobile applications. Nature 414, 353–358. doi: 10.1038/35104634
Shahri, W., and Tahir, I. (2011). Flower senescence-strategies and some associated events. Bot. Rev. 77, 152–184. doi: 10.1007/s12229-011-9063-2
Shen, W., and Sun, X. (2019). Hydrogen biology: it is just beginning. Chin. J. Biochem. Mol. Biol. 35, 1037–1050. doi: 10.13865/j.cnki.cjbmb.2019.10.01
Su, J., Nie, Y., Zhao, G., Cheng, D., Wang, R., Chen, J., et al. (2019). Endogenous hydrogen gas delays petal senescence and extends the vase life of lisianthus cut flowers. Postharvest Biol. Technol. 147, 148–155. doi: 10.1016/j.postharvbio.2018.09.018
Su, J., Zhang, Y., Nie, Y., Cheng, D., Wang, R., Hu, H., et al. (2018). Hydrogen-induced osmotic tolerance is associated with nitric oxide-mediated proline accumulation and reestablishment of redox balance in alfalfa seedlings. Environ. Exp. Bot. 147, 249–260. doi: 10.1016/j.envexpbot.2017.12.022
Thordal-Christensen, H., Zhang, Z., Wei, Y., and Collinge, D. B. (1997). Subcellular localization of H2O2 in plants. H2O2 accumulation in papillae and hypersensitive response during the barley—powdery mildew interaction. Plant J. 11, 1187–1194. doi: 10.1046/j.1365-313X.1997.11061187.x
van Doorn, W. G. (2010). “Water relations of cut flowers,” in Horticultural Reviews, ed. J. J. Janick (New York, NY: John Wiley & Sons), 1–85. doi: 10.1002/9780470650608.ch1
van Doorn, W. G., and Woltering, E. J. (2008). Physiology and molecular biology of petal senescence. J. Exp. Bot. 59, 453–480. doi: 10.1093/jxb/erm356
Wang, C., Fang, H., Gong, T., Zhang, J., Niu, L., Huang, D., et al. (2020). Hydrogen gas alleviates postharvest senescence of cut rose ‘Movie star’ by antagonizing ethylene. Plant Mol. Biol. 102, 271–285. doi: 10.1007/s11103-019-00946-3
Wang, Y., Li, L., Cui, W., Xu, S., Shen, W., and Wang, R. (2012). Hydrogen sulfide enhances alfalfa (Medicago sativa) tolerance against salinity during seed germination by nitric oxide pathway. Plant Soil 351, 107–119. doi: 10.1007/s11104-011-0936-2
Wu, Y., Yuan, M., Song, J., Chen, X., and Yang, H. (2019). Hydrogen gas from inflammation treatment to cancer therapy. ACS Nano 13, 8505–8511. doi: 10.1021/acsnano.9b05124
Xie, Y., Mao, Y., Lai, D., Zhang, W., and Shen, W. (2012). H2 enhances Arabidopsis salt tolerance by manipulating ZAT10/12-mediated antioxidant defence and controlling sodium exclusion. PLoS One 7:e49800. doi: 10.1371/journal.pone.0049800
Xie, Y., Mao, Y., Zhang, W., Lai, D., Wang, Q., and Shen, W. (2014). Reactive oxygen species-dependent nitric oxide production contributes to hydrogen-promoted stomatal closure in Arabidopsis. Plant Physiol. 165, 759–773. doi: 10.1104/pp.114.237925
Zeng, J., Zhang, M., and Sun, X. (2013). Molecular hydrogen is involved in phytohormone signaling and stress responses in plants. PLoS One 8:e71038. doi: 10.1371/journal.pone.0071038
Zhang, H., Hu, S., Zhang, Z., Hu, L., Jiang, C., Wei, Z., et al. (2011). Hydrogen sulfide acts as a regulator of flower senescence in plants. Postharvest Biol. Technol. 60, 251–257. doi: 10.1016/j.postharvbio.2011.01.006
Keywords: magnesium hydride, hydrogen gas, hydrogen sulfide, vase life, cut carnation flowers
Citation: Li L, Liu Y, Wang S, Zou J, Ding W and Shen W (2020) Magnesium Hydride-Mediated Sustainable Hydrogen Supply Prolongs the Vase Life of Cut Carnation Flowers via Hydrogen Sulfide. Front. Plant Sci. 11:595376. doi: 10.3389/fpls.2020.595376
Received: 17 August 2020; Accepted: 13 October 2020;
Published: 09 December 2020.
Edited by:
Antonio Ferrante, University of Milan, ItalyReviewed by:
Hua Zhang, Hefei University of Technology, ChinaChao Ma, China Agricultural University, China
Copyright © 2020 Li, Liu, Wang, Zou, Ding and Shen. This is an open-access article distributed under the terms of the Creative Commons Attribution License (CC BY). The use, distribution or reproduction in other forums is permitted, provided the original author(s) and the copyright owner(s) are credited and that the original publication in this journal is cited, in accordance with accepted academic practice. No use, distribution or reproduction is permitted which does not comply with these terms.
*Correspondence: Wenbiao Shen, d2JzaGVuaEBuamF1LmVkdS5jbg==
†ORCID: Longna Li, orcid.org/0000-0003-0007-3165; Yuhao Liu, orcid.org/0000-0002-1666-208X; Shu Wang, orcid.org/0000-0002-4417-9386; Jianxin Zou, orcid.org/0000-0003-1041-121X; Wenjiang Ding, orcid.org/0000-0003-0948-8025; Wenbiao Shen, orcid.org/0000-0003-1525-9472