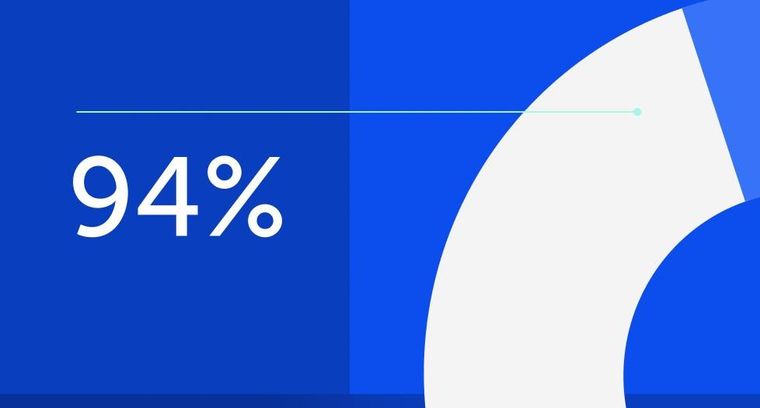
94% of researchers rate our articles as excellent or good
Learn more about the work of our research integrity team to safeguard the quality of each article we publish.
Find out more
MINI REVIEW article
Front. Plant Sci., 21 December 2020
Sec. Plant Nutrition
Volume 11 - 2020 | https://doi.org/10.3389/fpls.2020.591297
This article is part of the Research TopicRocks, Plants and MicrobesView all 7 articles
It is still an important aspect of global climate research to explore a low-cost method that can effectively reduce the increase of CO2 concentration in the global atmosphere. Oxalotrophic bacterial communities exist in agricultural or forest soil with ubiquitous oxalate as the only carbon and energy source. When soil oxalate is oxidized and degraded, carbonate is formed along with it. This process is called the oxalate carbonate pathway (OCP), which can increase soil inorganic carbon sink and soil organic matter content. This soil carbon sink is a natural CO2 trapping system and an important alternative if it is properly managed for artificial sequestration/storage. As the main driver of OCP, the oxalate degrading bacteria are affected by many factors during the oxalate conversion process. Understanding this process and the synergy of oxalogenic plants, saprophytic decomposers, and oxalotrophic bacteria in agricultural or forest soil is critical to exploiting this natural carbon capture process. This article aims to provide a broader perspective of OCP in CO2 sequestration, biomineralization, and elemental cycling.
Carbon dioxide (CO2) assimilation by photosynthesis is ubiquitous, whereas mineralization of CO2 into inorganic carbon compounds is majorly underrated, which usually involves the synergy of oxalogenic plants, saprophytic decomposers, and oxalotrophic bacteria. The metabolic pathway from oxalate to the mineralization of CO2 into carbonates, such as calcium carbonates is “The Oxalate Carbonate Pathway” (OCP) (Braissant et al., 2004; Cailleau et al., 2005; Rowley et al., 2017a; Durand et al., 2018). Mineralized carbon (carbonate) is substantially stable (102–106 years), unlike organic biomass and plays an important role in regulating CO2 content in the global C cycle (Cailleau et al., 2011, 2014). Hence, the OCP plays an important role in reducing atmospheric CO2 and increasing soil carbon content (Braissant et al., 2004; Cailleau et al., 2005; Rowley et al., 2017a; Durand et al., 2018), but requires numerous, autonomous, biotic, and abiotic components rendering OCP a unique and highly complex phenomenon with limited comprehensive studies (Martin et al., 2012; Cailleau et al., 2014; Rowley et al., 2017b). Despite such tremendous potential of OCP, current research is limited to the mechanism, participating and influencing agents with negligible quantitative characteristics (i.e., quantitative data regarding like net CO2 assimilated or carbonate formed is not available in most cases, only qualitative data are presented as OCP is extremely variable). Research on the management and enhancement of net assimilation/sequestration of CO2 via OCP in different soil environments can have major global implications by impacting net CO2 release in major ecosystems like the Amazon rainforest. Furthermore, management/regulation of CO2 mineralization via OCP influencing CO2 net release or assimilation has vast significance in remediating global climatic change. Research into OCP must focus on identifying the beneficial factors or habitat characteristics that result in a net increase in mineralized CO2 so that strategies can be used to make maximum use of this phenomenon in large terrestrial ecosystems. In this review, we intend to provide a perspective for the future use and deployment of CaOx generating plants and oxalotrophic bacteria across different scenarios, offering a realistic approach to impacting the natural environment with an outcome including, but not limited to, CO2 emissions mitigation, and soil organic carbon (OC) restoration.
Soil is the most complex ecosystem from a biological and geological point of view and is known to be the most vulnerable, prone to various impacts, ranging from erosion to pollution caused partly by nature but mostly due to human activities (Bellard et al., 2012). Furthermore, soil destabilization activities such as deforestation and intensive agricultural practices have reduced soil carbon both above and below ground, resulting in high CO2 emissions rather than reduction (Tanveer et al., 2019). The CO2 should be sequestrated as stable compounds for the simultaneous reduction of atmospheric CO2 concentration and OC depletion (Gross and Harrison, 2019). This can be achieved partly by the microflora involved in oxalate transformations, generating an increase in microcosm density and retaining the carbon sequestrated continuously over a long period, retarding environmental change (Lal, 2004; Lian et al., 2008; Sun et al., 2019a).
The biomineralization process involves oxalogenic plants (source of oxalate/oxalic acid) and oxalotrophic bacteria and is very important for certain barren soil ecosystems, particularly in desert soil and Karst topography, since they contain abundant precursors (Lal, 2002; Khaleghi and Rowshanzamir, 2019). Recent studies show many plants species (oxalogenic plants) contain oxalates (of calcium) as part of their metabolism and accumulate in various parts (commonly in roots, leaves, and barks) depending on the type of plant (Figure 1). Oxalogenic plants are imperative for the induction, maintenance, and strengthening of inorganic carbon assimilation (Borrelli et al., 2016; Pierantoni et al., 2018). Furthermore, the termites, saprophytic fungi, and rhizosphere microbial community carry out the degradation of oxalogenic biomass releasing and maintaining the oxalate pool (Cailleau et al., 2011).
Figure 1. Simplified representation of relationship between plants and fungi (oxalate producers) and oxalotrophic bacteria (oxalate degraders) in creating a soil-based carbon sink.
The oxalotrophic bacteria (having tolerance to different stresses—pH, moisture content, nutrient availability, contaminants) and oxalogenic crops (Amaranth, Rhubarb, Spinach, etc.) when grown together has the potential to precipitate atmospheric CO2 as carbonates (Gadd et al., 2014; Hervé et al., 2018). Oxalotrophic bacterial biomineralization is also beneficial in high calcite soils where OC is limited and rich in mineral calcium which serves as a substrate and is transformed into inorganic carbon (carbonates) (Zhu and Dittrich, 2016). Additionally, the carbonates formed influence the soil’s physico-chemical properties (particularly its pH), which in turn regulate the microbial community and its metabolic activities. Thus, the biomineralization enhances soil modifications that can help crop cultivation (Kalantary and Kahani, 2018; Kurganova et al., 2019; Liu et al., 2019).
Forests are the largest reserves of carbon both in the form of vegetation as well as soil OC. The diverse vegetation is crucial to absorb the CO2 released by natural and human activities (Zhang et al., 2015, 2018). Atmospheric CO2 is assimilated into plants as biomass, much later it accumulates in soil accounting for soil organic matter. Mismanagement of forests leads to deforestation, enhancing CO2 emissions during the post-industrial era. CO2 concentration in the atmosphere increased drastically to 400 from 270 ppm (pre-industrial era). Forest ecosystems aid in capturing 45% of terrestrial carbon and are responsible for 50% net ecosystem production (Ramachandra and Bharath, 2020). Terrestrial forests play a vital role in the carbon cycle, evident from the sequestration of about 30% of annual global anthropogenic CO2 emissions [2 petagrams (Pg) of carbon per year] from the atmosphere (Achat et al., 2015). Thus, soil carbon is the major pool of carbon in terrestrial ecosystem, with a vital role in nutritional security, water quality, biodiversity conservation, and elemental recycling (Chen et al., 2019) (Supplementary Figure S1). The afforestation efforts aimed to increase forest cover should consider the need to develop and sustain biodiversity to mitigate deforestation effects, as observed by some researchers (Qi et al., 2012; Brancalion et al., 2019).
CO2 retention in forest soil is dependent on land-use, anthropogenic stress, regimes of disturbance, and prevailing climatic conditions. The natural and intact forests increase the meantime of assimilated carbon’s residence with significantly limited re-emissions (Lal et al., 2015). The Iroko tree (Milicia excelsa), a prime example of an oxalogenic tree, produces and stores excess oxalates in its bark and roots, which enter soil over time to be metabolized into carbonates by the oxalotrophic microbiota. This results in the formation of indurated carbonate soils (calcrete forming) in semi-arid soils and affects the cycling of mineral nutrients like calcium, iron, and aluminum. The association and interdependent biomineralization of CO2 via oxalogenic plants, fungi and oxalotrophic bacteria can therefore be used in monoculture models to establish a healthy terrestrial carbon sink, as proposed by various researchers (Verrecchia et al., 2006; Whiffin et al., 2007; Peng et al., 2008). The oxalotrophic bacteria and oxalogenic trees can be used to cultivate barren landmasses and abandoned agroforestry strips to enhance soil organic content via sequestration of soil carbon through OCP. The increase in the forest cover would also mitigate the effect of deforestation and help to stabilize the local and global climate system (Bellassen and Luyssaert, 2014; Borchard et al., 2019).
Topsoil or the upper layer of the soil is crucial for many of the soil-based operations, such as plant growth, elemental cycling and also serves as a habitat for diverse soil microflora (Ducklow, 2008; Falkowski et al., 2008; Escalas et al., 2019). As previously mentioned, the presence of oxalotrophic bacteria around oxalate-producing plants can cause a pH shift (toward alkaline), promoting the formation of microbial assemblages that further improve carbon assimilation in the soil (Mazen, 2004). This induces OCP, which fixes atmospheric CO2 in the form of carbonates. The inorganic component is made of readily available metal in the soil either accumulated from mineral weathering or plant exudates/released from the decay of plant debris and ectomycorrhizal assemblages (mostly calcium) (Aragno and Verrecchia, 2012; Qin et al., 2013). The downside of this phenomenon is the need for the proximal coexistence of oxalogenic plants or oxalate minerals and oxalate-dependent heterotrophic microbiota that use the oxalate and acidic conditions in the habitat (oxalotrophy), leading to OCP (Dauer and Perakis, 2013; Lal et al., 2015). This is particularly effective in soils affected by mining, inorganic contaminants, and low OC content (Karst and sandy soils). With the increase in OC, the physical properties of the soil change dramatically due to the existence of a microbial population and thus affect crucial properties such as water-holding ability and pH (Ye et al., 2018).
These findings can lead to arid, dry, low fertile agroforestry strips being used to create green or agroforestry zones, creating soil-based carbon sinks specifically in areas without previous plant coverage (Supplementary Figure S2). These green zones would fix CO2 into plant biomass and create an opening for microbial community establishment. Besides, if the microbial community is controlled by human involvement, the organic biomass can be effectively mineralized, and added to the soil carbon sink.
The primary cause of soil instability is loose compaction of soil particles and the minerals with which it is made up of Pankova and Gerasimova (2012). The instability of soil leads to the loss of water holding capacity subsequently increasing soil aridity, the foremost reason for desertification. Moreover, desertification decreases biomass generation via plant photosynthesis, a major threat to grasslands and agricultural lands throughout the world. To fight desertification, many activities and methods are being utilized, yet the most effective approach, i.e., stabilizing soil particles, thus prevent desertification is left neglected (Cheng et al., 2014, 2017). The precipitation of CO2 through OCP by oxalogenic plants and oxalotrophic bacterial populations is efficient in binding the sand grains together, increasing the stability of the topsoil and also creates a carbon sink through carbonate accumulation (Mujah et al., 2016; Jiang et al., 2019; Seifan and Berenjian, 2019). In our laboratory experiments, when Streptomyces NJ10, an oxalotrophic bacteria with exceptional oxalate metabolizing potential isolated from bacterial assemblages in the ectomycorrhizosphere (Sun et al., 2019a), was grown in the presence of oxalate mineral in soils, cementing bridges were formed between the soil particles increasing the compaction of the soil (Supplementary Figure S3). Therefore, it is deduced that induction of CaCO3 precipitation binds sand grains together at the particle–particle contacts, increasing soil stability, particularly in loose soils with limited or low OC (DeJong et al., 2010; Mortensen et al., 2011; Chu et al., 2012). Many researchers have reported improvement of soil fertility and OC content when microbial communities capable of enhancing the mineral weathering are established, the CO32– ions precipitate with Ca2+ as calcite crystal, which generates cementing bridges between soil particles (Frankel, 2003; Dhami et al., 2013).
Heavy metals like lead (Pb), mercury (Hg), arsenic (As), cadmium (Cd), chromium (Cr, hexavalent), aluminum (Al), etc., are increasingly becoming a severe threat to the environment and the resident biota (Akoto et al., 2018; Al Osman et al., 2019; Obiora et al., 2019). The major sources of heavy metal pollution are inorganic fertilizers, metal-based pesticides and insecticides, industrial effluents, mine, and dumping yard leachate. Among many routes to stop and remove heavy metal contamination, microbial-based remediation techniques are preferential as they are effective, economical, and ecofriendly in their action (Jáuregui-Zúñiga et al., 2005; Pongrac et al., 2018; Tamayo-Figueroa et al., 2019). The oxalotrophic bacteria have great potential in this regard, as they can metabolize oxalic acid and produce carbonates (Aragno and Verrecchia, 2012; Xu et al., 2014; Pongrac et al., 2018), a stable end product, thus modifying the habitat pH and affecting the solubility and mobility of the heavy metals and prevent seeping into the subsoil (as the majority of the metals are insoluble in alkaline pH) (Zhang et al., 2020). Application of oxalic acid-producing heterotrophic fungi like Aspergillus spp. along with the oxalotrophic bacteria would possibly be a more effective way to precipitate the heavy metals as carbonates, neutralizing the heavy metal toxicity (Anbu et al., 2016). It is observed in our research when Aspergillus niger is grown in the presence of heavy metal Lead, it produced lead oxalate (Unpublished data). Additionally, Streptomyces NJ10 an oxalotrophic bacteria when grown in the presence of lead oxalate was able to utilize lead oxalate and grew well. Based on these findings, it can be concluded that heterotrophs capable of producing organic acid and oxalotrophic bacteria may be converted into a microbial preparation and applied to polluted sites to reduce the toxicity of heavy metals.
Soil carbon sink is suitable for many CO2 capture and storage routes but has much slower CO2 assimilation rates. The assimilation of CO2 in the soil depends on many factors including pH, microbial assemblies, water movement, soil geology, and seasonal weather variability. It is also well established that the microcosms created by ectomycorrhiza of many higher plants support diverse oxalotrophic bacteria and provide a stable oxalate pool along with a more suitable environment for the oxalate mineralization (Guggiari et al., 2011; Sun et al., 2019a). Besides, carbon capture, storage and stability in the form of inorganic compounds is typically dependent on microbial assemblies, plant diversity and geological characteristics in the soil and can be enhanced/influenced by human interventions such as the introduction of organic mineral fertilizers (Lian et al., 2008; Liu et al., 2012; Sun et al., 2019b) (Figure 2). The addition of nitrogen to the soil in the form of bio-organic fertilizer also increases CO2 assimilation in the form of OC by the growth of plant biomass as observed in several studies that can be adapted in the agricultural sector (Yang et al., 2020). However, this lacks stability and is promptly moved to the global carbon cycle as CO2 within a limited time (approximately 101–103 years). In comparison, mineralized carbon takes longer periods but is greatly influenced by soil pH, since acidic pH dissolves the mineralized carbon (HCO3-). Some researchers have demonstrated that silicate-rich clay mineral soils are significantly better choices for carbon sinks as they can provide the inorganic (metal) component for carbon mineralization through soil biological activity (Chen et al., 2017; Xiao et al., 2017). Furthermore, deeper studies are required on factors that have a significant impact on the soil carbon sink but have received little attention, including soil carbon management.
Figure 2. A typical oxalate carbonate pathway showing different biotic and abiotic influencing factors.
The carbon cycle has been extensively studied concerning climate change research and the sequestration of carbon is mostly attributed to OC storage alone in the global carbon cycle. Soil-mineral carbon sinks are of great interest for two reasons: (1) the stability of mineralized carbon (102–106 years) is up to 100,000 times longer than for soil organic matter carbon (101–103 years) and (2) thousands of plant species are known to mineralize atmospheric carbon but are usually ignored, whereas sequestration of carbon in soil may only be considered as OC sink. However, it represents a potentially more effective carbon sink if contained in large amounts, due to the resilience of mineralized carbon in soils. Oxalate and its transformations in various oxalogenic plants can affect the carbon sink of soil and global carbon beyond what was previously understood. Measures such as the conservation of carbon-mineralizing trees such as Iroko (M. excelsa) and other biomineralizing plant species in depleted or low-carbon soils are potentially important for significant mineral carbon sinks development. Research on the quantitative assimilation of CO2 into mineralized carbon is therefore required to create a commercially viable soil carbon sink, which is practically accessible via OCP. And hence, the cultivation of carbon-mineralizing trees in the form of agroforestry projects can serve multiple purposes of carbon assimilation and soil recovery. Finally, the major factor required to resolve sustainable mineralization of carbon is its potential disintegration by metabolic activities of soil bacteria and modifications of soil pH.
SS curated related data and prepared the original draft. BL conceptualized and reviewed the draft manuscript. VB reviewed and edited the draft manuscript. All authors contributed to the article and approved the submitted version.
This work was jointly supported by the National Natural Science Foundation of China (Grant Nos. 41772360 and 41373078) and the Introduction Plan for High-Level Foreign Experts (Grant No. G2019021434).
The authors declare that the research was conducted in the absence of any commercial or financial relationships that could be construed as a potential conflict of interest.
The Supplementary Material for this article can be found online at: https://www.frontiersin.org/articles/10.3389/fpls.2020.591297/full#supplementary-material
Supplementary Figure 1 | Carbon cycling in terrestrial forest ecosystems and its major services.
Supplementary Figure 2 | A possible model for utilizing oxalogenic crops/agroforestry and oxalotrophic bacteria to create soil-based carbon sinks in low fertile soils. Inset: typical carbonate mineralization.
Supplementary Figure 3 | Stereomicroscope images showing carbonate bridges formed by oxalotrophic bacteria Streptomyces NJ10, holding soil particles. (a) Carbonate bridge in between two sand particles indicated by arrow. (b) similar sand particles lacking such bridges are more prone to erosion (indicated by arrows).
Achat, D. L., Fortin, M., Landmann, G., Ringeval, B., and Augusto, L. (2015). Forest soil carbon is threatened by intensive biomass harvesting. Sci. Rep. 5:15991. doi: 10.1038/srep15991
Akoto, O., Nimako, C., Asante, J., Bailey, D., and Bortey-Sam, N. (2018). Spatial distribution, exposure, and health risk assessment of bioavailable forms of heavy metals in surface soils from abandoned landfill sites in Kumasi, Ghana. Human Ecol. Risk Assess. 25, 1–16. doi: 10.1080/10807039.2018.1476125
Al Osman, M., Yang, F., and Massey, I. Y. (2019). Exposure routes and health effects of heavy metals on children. Biometals 32, 563–573. doi: 10.1007/s10534-019-00193-5
Anbu, P., Kang, C. H., Shin, Y. J., and So, J. S. (2016). Formations of calcium carbonate minerals by bacteria and its multiple applications. Springerplus 5:250. doi: 10.1186/s40064-016-1869-2
Aragno, M., and Verrecchia, E. (2012). “The oxalate-carbonate pathway: a reliable sink for atmospheric CO2 through calcium carbonate biomineralization in ferralitic tropical soils,” in Microorganisms in Environmental Management, eds T. Satyanarayana, B. N. Johri, and A. Prakash (Dordrecht: Springer), 191–200.
Bellard, C., Bertelsmeier, C., Leadley, P., Thuiller, W., and Courchamp, F. (2012). Impacts of climate change on the future of biodiversity. Ecol. Lett. 15, 365–377.
Bellassen, V., and Luyssaert, S. (2014). Carbon sequestration: managing forests in uncertain times. Nature 506, 153–155. doi: 10.1038/506153a
Borchard, N., Bulusu, M., Meyer, N., Rodionov, A., Herawati, H., Blagodatsky, S., et al. (2019). Deep soil carbon storage in tree-dominated land use systems in tropical lowlands of Kalimantan. Geoderma 354:113864. doi: 10.1016/j.geoderma.2019.07.022
Borrelli, N., Benvenuto, M. L., and Osterrieth, M. (2016). Calcium oxalate crystal production and density at different phenological stages of soybean plants (Glycine max L.) from the southeast of the Pampean Plain, Argentina. Plant Biol. 18, 1016–1024.
Braissant, O., Cailleau, G., Aragno, M., and Verrecchia, E. P. (2004). Biologically induced mineralization in the tree Milicia excelsa (Moraceae): its causes and consequences to the environment. Geobiology 2, 59–66.
Brancalion, P. H. S., Niamir, A., Broadbent, E., Crouzeilles, R., Barros, F. S. M., Almeyda Zambrano, A. M., et al. (2019). Global restoration opportunities in tropical rainforest landscapes. Sci. Adv. 5:eaav3223. doi: 10.1126/sciadv.aav3223
Cailleau, G., Braissant, O., Dupraz, C., Aragno, M., and Verrecchia, E. P. (2005). Biologically induced accumulations of CaCO3 in orthox soils of Biga, Ivory Coast. Catena 59, 1–17.
Cailleau, G., Braissant, O., and Verrecchia, E. P. (2011). Turning sunlight into stone: the oxalate-carbonate pathway in a tropical tree ecosystem. Biogeosci. Discuss. 8, 1755–1767. doi: 10.5194/bg-8-1755-2011
Cailleau, G., Mota, M., Bindschedler, S., Junier, P., and Verrecchia, E. P. (2014). Detection of active-oxalate-carbonate pathway ecosystems in the Amazon Basin: global implications of a natural potential C sink. Catena 116, 132–141. doi: 10.1016/j.catena.2013.12.017
Chen, P., Ruan, Y. L., Wang, S. J., Liu, X. M., and Lian, B. (2017). Effects of organic mineral fertiliser on heavy metal migration and potential carbon sink in soils in a karst region. Acta Geochimica 36, 539–543. doi: 10.1007/s11631-017-0230-y
Chen, S., Zou, J., Hu, Z., and Lu, Y. (2019). Climate and vegetation drivers of terrestrial carbon fluxes: a global data synthesis. Adv. Atmos. Sci. 36, 679–696. doi: 10.1007/s00376-019-8194-y
Cheng, L., Shahin, M. A., and Cord-Ruwisch, R. (2014). Bio-cementation of sandy soil using microbially induced carbonate precipitation for marine environments. Géotechnique 64, 1010–1013. doi: 10.1680/geot.14.t.025
Cheng, L., Shahin, M. A., and Mujah, D. (2017). Influence of key environmental conditions on microbially induced cementation for soil stabilization. J. Geotech. Geoenviron. Eng. 143:04016083. doi: 10.1061/(asce)gt.1943-5606.0001586
Chu, J., Stabnikov, V., and Ivanov, V. (2012). Microbially induced calcium carbonate precipitation on surface or in the bulk of soil. Geomicrobiol. J. 29, 544–549. doi: 10.1080/01490451.2011.592929
Dauer, J. M., and Perakis, S. S. (2013). Contribution of calcium oxalate to soil-exchangeable calcium. Soil Sci. 178, 671–678.
DeJong, J. T., Mortensen, B. M., Martinez, B. C., and Nelson, D. C. (2010). Bio-mediated soil improvement. Ecol. Eng. 36, 197–210. doi: 10.1016/j.ecoleng.2008.12.029
Dhami, N. K., Reddy, M. S., and Mukherjee, M. S. (2013). Biomineralization of calcium carbonates and their engineered applications: a review. Front. Microbiol. 4:314. doi: 10.3389/fmicb.2013.00314
Ducklow, H. (2008). Microbial services: challenges for microbial ecologists in a changing world. Aquat. Microb. Ecol. 53, 13–19. doi: 10.3354/ame01220
Durand, N., Monger, H. C., Canti, M. G., and Verrecchia, E. P. (2018). “Calcium carbonate features,” in Interpretation of Micromorphological Features of Soils and Regoliths, eds G. Stoops, V. Marcelino, and F. Mees (Amsterdam: Elsevier), 149–194. doi: 10.1016/B978-0-444-53156-8.00009-X
Escalas, A., Hale, L., Voordeckers, J. W., Yang, Y., Firestone, M. K., Alvarez-Cohen, L., et al. (2019). Microbial functional diversity: from concepts to applications. Ecol. Evol. 9, 12000–12016. doi: 10.1002/ece3.5670
Falkowski, P. G., Fenchel, T., and Delong, E. F. (2008). The microbial engines that drive earth’s biogeochemical cycles. Science 320, 1034–1039. doi: 10.1126/science.1153213
Frankel, R. B. (2003). Biologically induced mineralization by bacteria. Rev. Mineral. Geochem. 54, 95–114. doi: 10.2113/0540095
Gadd, G. M., Bahri-Esfahani, J., Li, Q., Rhee, Y. J., Wei, Z., Fomina, M., et al. (2014). Oxalate production by fungi: significance in geomycology, biodeterioration and bioremediation. Fungal Biol. Rev. 28, 36–55.
Gross, C. D., and Harrison, R. B. (2019). The case for digging deeper: soil organic carbon storage, dynamics, and controls in our changing world. Soil Syst. 3:28.
Guggiari, M., Martin, G., Aragno, M., Verrecchia, E., and Job, D. (2011). “Assessment of the collaboration between fungi and bacteria during the oxalate-carbonate pathway in microcosms,” in Proceedings of the 3rd International Conference on Environmental, Industrial and Applied Microbiology: Microorganisms in Industry And Environment: From Scientific and Industrial Research to Consumer Products 2–4 December 2009, Lisbon, 148–152.
Hervé, V., Clerc, M., Cailleau, G., Bueche, M., Junier, T., Verrecchia, E., et al. (2018). Carbonate accumulation in the bark of Terminalia bellirica: a new habitat for the oxalate-carbonate pathway. Geomicrobiol. J. 35, 31–39.
Jáuregui-Zúñiga, D., Angeles-Ferrer, M., Calderón, A. A., Muñoz, R., and Moreno, A. (2005). Heavy metal stress reduces the deposition of calcium oxalate crystals in leaves of Phaseolus vulgaris. J. Plant Physiol. 162, 1183–1187. doi: 10.1016/j.jplph.2005.03.002
Jiang, N. J., Tang, C. S., Yin, L. Y., Xie, Y. H., and Shi, B. (2019). Applicability of microbial calcification method for sandy-slope surface erosion control. J. Mater. Civ. Eng. 31:04019250. doi: 10.1061/(asce)mt.1943-5533.0002897
Kalantary, F., and Kahani, M. (2018). Optimization of the biological soil improvement procedure. Int. J. Environ. Sci. Technol. 16, 4231–4240. doi: 10.1007/s13762-018-1821-9
Khaleghi, M., and Rowshanzamir, M. A. (2019). Biologic improvement of a sandy soil using single and mixed cultures: a comparison study. Soil Tillage Res. 186, 112–119. doi: 10.1016/j.still.2018.10.010
Kurganova, I., Merino, A., Lopes de Gerenyu, V., Barros, N., Kalinina, O., Giani, L., et al. (2019). Mechanisms of carbon sequestration and stabilization by restoration of arable soils after abandonment: a chronosequence study on Phaeozems and Chernozems. Geoderma 354:113882. doi: 10.1016/j.geoderma.2019.113882
Lal, R. (2002). Soil carbon sequestration in China through agricultural intensification, and restoration of degraded and desertified ecosystems. Land Degrad. Dev. 13, 469–478.
Lal, R. (2004). Soil carbon sequestration impacts on global climate change and food security. Science 304, 1623–1627. doi: 10.1126/science.1097396
Lal, R., Negassa, W., and Lorenz, K. (2015). Carbon sequestration in soil. Curr. Opin. Environ. Sustai. 15, 79–86.
Lian, B., Chen, Y., Lijun, Z., and Yang, R. (2008). Effect of microbial weathering on carbonate rocks. Earth Sci. Front. 15, 90–99. doi: 10.1016/S1872-5791(09)60009-9
Liu, D. F., Lian, B., and Dong, H. L. (2012). Isolation of mineral potassium-solubilizing bacteria Paenibacillus sp. and assessment of their potential for enhancing mineral weathering. Geomicrobiol. J. 29, 413–421.
Liu, S., Wen, K., Armwood, C., Bu, C., Li, C., Amini, F., et al. (2019). Enhancement of MICP-treated sandy soils against environmental deterioration. J. Mater. Civ. Eng. 31:04019294. doi: 10.1061/(asce)mt.1943-5533.0002959
Martin, G., Guggiari, M., Bravo, D., Zopfi, J., Cailleau, G., Aragno, M., et al. (2012). Fungi, bacteria and soil pH: the oxalate–carbonate pathway as a model for metabolic interaction. Environ. Microbiol. 14, 2960–2970.
Mazen, A. M. A. (2004). Calcium oxalate deposits in leaves of Corchorus olitotius as related to accumulation of toxic metals. Russ. J. Plant Physiol. 51, 281–285.
Mortensen, B. M., Haber, M. J., DeJong, J. T., Caslake, L. F., and Nelson, D. C. (2011). Effects of environmental factors on microbial induced calcium carbonate precipitation. J. Appl. Microbiol. 111, 338–349. doi: 10.1111/j.1365-2672.2011.05065.x
Mujah, D., Shahin, M. A., and Cheng, L. (2016). State-of-the-art review of biocementation by microbially induced calcite precipitation (MICP) for soil stabilization. Geomicrobiol. J. 34, 524–537. doi: 10.1080/01490451.2016.1225866
Obiora, S. C., Chukwu, A., Chibuike, G., and Nwegbu, A. N. (2019). Potentially harmful elements and their health implications in cultivable soils and food crops around lead-zinc mines in Ishiagu, Southeastern Nigeria. J. Geochem. Explor. 204, 289–296. doi: 10.1016/j.gexplo.2019.06.011
Pankova, E. I., and Gerasimova, M. I. (2012). Desert soils: properties, pedogenic processes, and classification. Arid Ecosyst. 2, 69–77. doi: 10.1134/s2079096112020084
Peng, Y., Thomas, S. C., and Tian, D. (2008). Forest management and soil respiration: implications for carbon sequestration. Environ. Rev. 16:93111. doi: 10.1139/a08-003
Pierantoni, M., Tenne, R., Rephael, B., Brumfeld, V., van Casteren, A., Kupczik, K., et al. (2018). Mineral deposits in Ficus leaves: morphologies and locations in relation to function. Plant Physiol. 176, 1751–1763.
Pongrac, P., Serra, T., Castillo-Michel, H., Vogel-Mikus, K., Arcon, I., Kelemen, M., et al. (2018). Cadmium associates with oxalate in calcium oxalate crystals and competes with calcium for translocation to stems in the cadmium bioindicator Gomphrena claussenii. Metallomics 10, 1576–1584. doi: 10.1039/C8MT00149A
Qi, Y., Chang, Q., Jia, K., Liu, M., Liu, J., and Chen, T. (2012). Temporal-spatial variability of desertification in an agro-pastoral transitional zone of northern Shaanxi province, China. Catena 88, 37–45. doi: 10.1016/j.catena.2011.08.003
Qin, Z., Huang, Y., and Zhuang, Q. (2013). Soil organic carbon sequestration potential of cropland in China. Global Biogeochem. Cycles 27, 711–722. doi: 10.1002/gbc.20068
Ramachandra, T. V., and Bharath, S. (2020). Carbon sequestration potential of the forest ecosystems in the Western Ghats, a global biodiversity hotspot. Nat. Resour. Res. 29, 2753–2771. doi: 10.1007/s11053-019-09588-0
Rowley, M. C., Estrada-Medina, H., Tzec-Gamboa, M., Rozin, A., Cailleau, G., Verrecchia, E. P., et al. (2017a). Moving carbon between spheres, the potential oxalate-carbonate pathway of Brosimum alicastrum Sw.; Moraceae. Plant Soil 412, 465–479.
Rowley, M. C., Grand, S., and Verrecchia, ÉP. (2017b). Calcium-mediated stabilisation of soil organic carbon. Biogeochemistry 137, 27–49. doi: 10.1007/s10533-017-0410-1
Seifan, M., and Berenjian, A. (2019). Microbially induced calcium carbonate precipitation: a widespread phenomenon in the biological world. Appl. Microbiol. Biotechnol. 103, 4693–4708. doi: 10.1007/s00253-019-09861-5
Sun, Q., Li, J., Finlay, R. D., and Lian, B. (2019a). Oxalotrophic bacterial assemblages in the ectomycorrhizosphere of forest trees and their effects on oxalate degradation and carbon fixation potential. Chem. Geol. 514, 54–64. doi: 10.1016/j.chemgeo.2019.03.023
Sun, Q., Ruan, Y. L., Chen, P., Wang, S. J., Liu, X. M., and Lian, B. (2019b). Effects of mineral-organic fertiliser on the biomass of green Chinese cabbage and potential carbon sequestration ability in karst areas of Southwest China. Acta Geochimica 38, 430–439. doi: 10.1007/s11631-019-00320-6
Tamayo-Figueroa, D. P., Castillo, E., and Brandão, P. F. B. (2019). Metal and metalloid immobilization by microbiologically induced carbonates precipitation. World J. Microbiol. Biotechnol. 35:58. doi: 10.1007/s11274-019-2626-9
Tanveer, S. K., Lu, X., Hussain, I., and Sohail, M. (2019). “Soil carbon sequestration through agronomic management practices,” in CO2 Sequestration, eds L. A. Frazão, A. M. Silva-Olaya and J. C. Silva (London: IntechOpen).
Verrecchia, E., Braissant, O., and Cailleau, G. (2006). “The oxalate–carbonate pathway in soil carbon storage: the role of fungi and oxalotrophic bacteria,” in Fungi in Biogeochemical Cycles, ed. G. Gadd (Cambridge: Cambridge University Press), 289–310. doi: 10.1017/CBO9780511550522.013
Whiffin, V. S., van Paassen, L. A., and Harkes, M. P. (2007). Microbial carbonate precipitation as a soil improvement technique. Geomicrobiol. J. 24, 417–423. doi: 10.1080/01490450701436505
Xiao, L. L., Sun, Q., Yuan, H. T., and Lian, B. (2017). A practical soil management to improve soil quality by applying mineral organic fertilizer. Acta Geochimica 36, 198–204. doi: 10.1007/s11631-017-0139-5
Xu, P., Leng, Y., Zeng, G., Huang, D., Lai, C., Zhao, M., et al. (2014). Cadmium induced oxalic acid secretion and its role in metal uptake and detoxification mechanisms in Phanerochaete chrysosporium. Appl. Microbiol. Biotechnol. 99, 435–443. doi: 10.1007/s00253-014-5986-y
Yang, Y., Shameer, S., Mao, S., Li, Q., Ge, F., Lian, B., et al. (2020). Bioorganic–mineral fertilizer can remediate chemical fertilizer-oversupplied soil: purslane planting as an example. J. Soil Sci. Plant Nutr. 20, 892–900. doi: 10.1007/s42729-020-00175-4
Ye, B., Luo, Y., He, J., Sun, L., Long, B., Liu, Q., et al. (2018). Investigation of lead bioimmobilization and transformation by Penicillium oxalicum SL2. Bioresour. Technol. 264, 206–210. doi: 10.1016/j.biortech.2018.05.066
Zhang, J., Yang, R., Li, Y. C., Peng, Y., Wen, X., and Ni, X. (2020). Distribution, accumulation, and potential risks of heavy metals in soil and tea leaves from geologically different plantations. Ecotoxicol. Environ. Saf. 195:110475.
Zhang, X., Guan, D., Li, W., Sun, D., Jin, C., Yuan, F., et al. (2018). The effects of forest thinning on soil carbon stocks and dynamics: a meta-analysis. For. Ecol. Manage. 429, 36–43. doi: 10.1016/j.foreco.2018.06.027
Zhang, X., Song, Z., McGrouther, K., Li, J., Li, Z., Ru, N., et al. (2015). The impact of different forest types on phytolith-occluded carbon accumulation in subtropical forest soils. J. Soils Sediments 16, 461–466. doi: 10.1007/s11368-015-1259-3
Keywords: oxalate/oxalic acid, soil carbon sink, CO2 sequestration, carbonates, oxalotrophic bacteria
Citation: Syed S, Buddolla V and Lian B (2020) Oxalate Carbonate Pathway—Conversion and Fixation of Soil Carbon—A Potential Scenario for Sustainability. Front. Plant Sci. 11:591297. doi: 10.3389/fpls.2020.591297
Received: 04 August 2020; Accepted: 30 November 2020;
Published: 21 December 2020.
Edited by:
Bruno Brito Lisboa, State Secretariat of Agriculture, Livestock and Irrigation, BrazilReviewed by:
Zhaoliang Song, Tianjin University, ChinaCopyright © 2020 Syed, Buddolla and Lian. This is an open-access article distributed under the terms of the Creative Commons Attribution License (CC BY). The use, distribution or reproduction in other forums is permitted, provided the original author(s) and the copyright owner(s) are credited and that the original publication in this journal is cited, in accordance with accepted academic practice. No use, distribution or reproduction is permitted which does not comply with these terms.
*Correspondence: Bin Lian, YmluMjM2OEB2aXAuMTYzLmNvbQ==
Disclaimer: All claims expressed in this article are solely those of the authors and do not necessarily represent those of their affiliated organizations, or those of the publisher, the editors and the reviewers. Any product that may be evaluated in this article or claim that may be made by its manufacturer is not guaranteed or endorsed by the publisher.
Research integrity at Frontiers
Learn more about the work of our research integrity team to safeguard the quality of each article we publish.