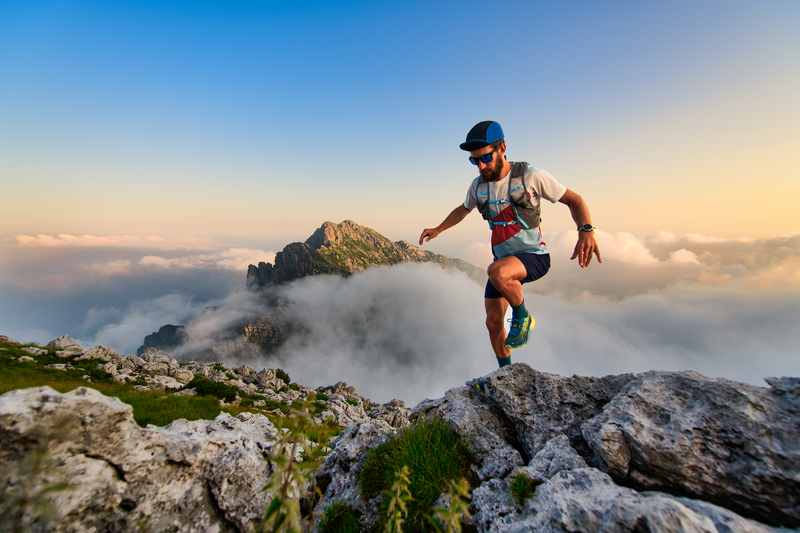
95% of researchers rate our articles as excellent or good
Learn more about the work of our research integrity team to safeguard the quality of each article we publish.
Find out more
ORIGINAL RESEARCH article
Front. Plant Sci. , 28 October 2020
Sec. Plant Physiology
Volume 11 - 2020 | https://doi.org/10.3389/fpls.2020.590337
This article is part of the Research Topic Cytokinins as Central Regulators of Plant Growth and Development View all 14 articles
Cytokinin is a phytohormone involved in the regulation of diverse developmental and physiological processes in plants. Its potential in biotechnology and for development of higher-yield and more resilient plants has been recognized, yet the molecular mechanisms behind its action are far from understood. In this report, the roots of barley seedlings were explored as a new source to reveal as yet unknown cytokinin-responsive proteins for crop improvement. Here we found significant differences reproducibly observed for 178 proteins, for which some of the revealed cytokinin-responsive pathways were confirmed in metabolome analysis, including alterations phenylpropanoid pathway, amino acid biosynthesis and ROS metabolism. Bioinformatics analysis indicated a significant overlap between cytokinin response and response to abiotic stress. This was confirmed by comparing proteome and metabolome profiles in response to drought, salinity or a period of temperature stress. The results illustrate complex abiotic stress response in the early development of model crop plant and confirm an extensive crosstalk between plant hormone cytokinin and response to temperature stimuli, water availability or salinity stress.
Cytokinin is a multifaceted plant hormone that plays major roles in plant growth and development as well as interacting with both biotic and abiotic factors, including light, temperature, drought, and salinity signals (as reviewed in Argueso et al., 2009; Kieber and Schaller, 2014; Zwack and Rashotte, 2015; Pavlů et al., 2018; Cortleven et al., 2019). The canonical cytokinin signal transduction pathway is a multistep phosphorelay with sensor hybrid histidine kinase that phosphorylates histidine-containing phosphotransfer proteins which are then translocated into the nucleus, where they transfer the phosphate to type-B response regulators. The previous proteome-wide analyses have also revealed a transcription-independent rapid response to cytokinin targeting predominantly plastidial proteins (Chen et al., 2010; Černý et al., 2011, 2014; Žd’árská et al., 2013; Hallmark et al., 2020).
Seed germination and early seedling establishment is a complex biological process that includes drastic morphological, physiological, and biochemical changes, and represents a period of life when a plant is highly susceptible to both biotic and abiotic stressors (Bewley et al., 2013; Kaur et al., 2017). The early germination phase is thought of as predominantly governed by the phytohormones abscisic acid and gibberellin, yet other growth regulators are also known to participate in the complex process. The role of cytokinin in seedling establishment is indisputable, including regulation of shoot and root apical meristems, development of lateral roots or vasculature system, light signaling, chloroplast development and de-etiolation and leaf development (Chory et al., 1994; Kieber and Schaller, 2014; Skalák et al., 2019). Early reports of Arabidopsis cytokinin mutants showed that a receptor mutation or an enhanced cytokinin degradation resulted in more rapid germination (Riefler et al., 2006). In contrast, later studies revealed that cytokinin antagonizes abscisic acid by downregulating ABI5 and promoting its degradation (Wang et al., 2011; Guan et al., 2014) and found that plants overexpressing cytokinin response regulators type-A were insensitive to abscisic acid (Huang et al., 2017).
Barley refers to the cereal Hordeum vulgare subsp. vulgare and belongs to the Triticeae tribe of grasses. The tribe comprises about 350 species among them the important cereals are wheat (Triticum spp.), rye (Secale cereale) and triticale (xTriticosecale), many forage grasses and ecologically important taxa of temperate grasslands (Blattner, 2018). Barley is the fourth most important cereal grain with a worldwide production exceeding 141 million tonnes (FAOSTAT, year 2018; http://faostat.fao.org) and an excellent crop model species with a sequenced genome (Mayer et al., 2012). Barley has a diploid genome (2n) with only 14 chromosomes making it more suitable for analyses than its close relative from Triticeae tribe, wheat. There are over 400,000 barley accessions conserved in collections world-wide (Dawson et al., 2015) and biotechnology tools for transformation have been developed (e.g., Harwood, 2019). Previous research on cytokinin signaling has successfully developed transgenic barley lines with an enlarged root system, higher yield and improved drought resilience (Zalewski et al., 2010; Mrízová et al., 2013; Pospíšilová et al., 2016; Vojta et al., 2016; Holubová et al., 2018; Ramireddy et al., 2018), but analyses targeting molecular mechanisms underlying cytokinin response in barley are limited. The Arabidopsis Information Resource lists 142 genes associated with cytokinin. In contrast, only 20 and 30 genes are associated with the cytokinin signaling or metabolism in the reference barley genomes IBSC and Morex, respectively (plants.ensembl.org; barlex.barleysequence.org).
Cytokinin is one of the principal players in the process of root organogenesis (e.g., Laplaze et al., 2007; Müller and Sheen, 2008; Bielach et al., 2012; Kurepa et al., 2019) and roots are thus an excellent target for the cytokinin response analysis. Further, the detection of plant proteins is often hindered by the presence of Ribulose bisphosphate carboxylase/oxygenase (RubisCO) which may represent more than 50% of the plant protein extracts (e.g., Righetti and Boschetti, 2016). Techniques have been developed to eliminate or deplete this highly abundant enzyme (Gupta et al., 2015) but the root tissue, being devoid of RubisCO, does not require this additional step. We hypothesized that barley seedling root could be an excellent model for analysis of cytokinin-responsive proteins and that comprehensive, integrated and high-resolution analyses could extend current understanding of cytokinin role in early plant development. To elicit the cytokinin response, we selected the treatment with trans-zeatin, which is together with cis-zeatin the dominant active cytokinin in barley (Powell et al., 2013).
Barley root proteome was extracted from roots of four-day-old seedlings as described in Materials and Method. Root proteome profiling identified over 10,000 peptides corresponding to more than 2,600 proteins represented by 1,735 protein families. The gene ontology (GO) analysis revealed that most proteins are identified as being involved in biosynthetic processes, including amino acid biosynthesis, proteosynthesis and the production of secondary metabolites (Figures 1A,B). Proportionally, the most abundant proteins representing 80% of the analyzed root proteome comprised of only 460 proteins and the majority of these was formed by carbohydrate metabolism enzymes (15.2%), ribosomal proteins and proteins involved in proteosynthesis (11.3%), energy metabolism (10.3%), protein folding (9.1%), histones (6.1%) and reactive oxygen species (ROS) metabolism (5.4%). There was also a noticeable amount of jasmonic acid (JA)-responsive proteins and JA biosynthetic enzymes (12-oxophytodienoate reductase, multiple lipoxygenases), representing more than 2.3% of all detected proteins.
Figure 1. Barley root proteome composition. (A) Enrichment analysis based on hypergeometric distribution followed by FDR correction. Two pathways are connected if they share 20% or more proteins. Darker and bigger nodes are more significantly enriched and larger sets, respectively. Analyzed by ShinyGO 0.61 (Ge et al., 2020); (B) The function of the most abundant proteins representing 80% of the barley root proteome. For the sake of clarity, only ten most abundant categories are labeled.
To elicit the cytokinin response, barley seedlings were treated with 1 μM of the cytokinin, trans-zeatin for 24 h. This is a similar concentration to that used in previous cytokinin transcriptomics and proteomics analyses but far above the natural endogenous levels (Černý et al., 2016). The addition of higher cytokinin concentrations may induce a hypersensitive-like response and promote cell death (Vescovi et al., 2012; Novák et al., 2013), and thus it cannot be excluded that some of the observed cytokinin-responsive proteins found here could represent a response to toxicity. However, as illustrated in Figure 2, the barley germination rate in the presence of 1 μM trans-zeatin was similar to that of mock-treated grains, and the percentage of germinated grains after 48 h was unaffected. This standard assay evaluates only the emergence of the radicle, but it is likely that barley seedlings can counter any negative effect of cytokinin at this concentration and duration by triggering its degradation, which is supported by the fact that cytokinin-treated roots accumulated a sufficient amount of cytokinin dehydrogenase (CKX6) to facilitate its detection in an untargeted proteome-wide analysis (Supplementary Table S2).
Figure 2. Barley germination in response to different growth regulators. Percentage of germinated seeds 24 and 48 h after imbibition in 4 ml water supplemented with 5 × 10–4% (v/v) dimethyl sulfoxide (mock buffer) or 1 μM phytohormone (tZ, trans-Zeatin; IAA, Indole-3-acetic acid; GA3, Gibberellic acid; ABA, abscisic acid) in dimethyl sulfoxide (final concentration, as for the mock). Different letters indicate significant differences (Kruskal-Wallis test, p < 0.05).
A comparison of proteome profiles between cytokinin and mock-treated roots showed significant separation (Figure 4A) and revealed 350 candidate cytokinin-responsive proteins (p < 0.05), including the above-mentioned enzyme CKX6 (found only in cytokinin-treated roots). Next, candidates failing stringent identification and quantitation criteria were removed and only differentially abundant proteins with at least 10 spectral matches in a biological replicate, more than one unique peptide and absolute fold-change of at least 1.4 were selected, limiting the set to 178 differentially abundant cytokinin-responsive proteins (Tables 1, 2). In total, 63 and 115 cytokinin-responsive proteins representing more than 12% of the total root proteome were found as significantly increased and decreased, respectively. The most numerous categories also matched the total proteome composition (carbohydrate metabolism, ribosomal proteins and proteosynthesis, energy metabolism; Figure 3D). Predicted cellular localization showed that the cytokinin-responsive proteins were found predominantly in the cytosol (35%), extracellular space (16%), plastids (14%), nucleus (10%), and mitochondrion (9%). Comparisons of cytokinin-responsive localization to the whole proteome dataset showed that mitochondrial and nuclear proteins were underrepresented (Figures 3B,C). The set of cytokinin-induced proteins included enzymes of phenylpropanoid biosynthesis, components of cytoskeleton and enzymes of lipid metabolism. The metabolic pathway enrichment revealed that proteins with a significant depletion were enriched in ribosomal proteins (11), enzymes of biosynthesis of secondary metabolites (17) or protein processing (8). About one-third of the cytokinin-repressed proteins was associated with a stress response, indicating a putative connection between cytokinin-induced alleviation or attenuation of the stress. Not all identified differentially abundant proteins are novel cytokinin-responsive proteins per se. The comparison to previously known cytokinin-responsive proteins identified at least 26 barley root proteins orthologs that were found with a similar response to cytokinin (Lochmanová et al., 2008; Liu et al., 2011; Černý et al., 2011, 2014; Zhang et al., 2012; Žd’árská et al., 2013; Hallmark et al., 2020) and 36 cytokinin-responsive genes from Arabidopsis thaliana (Hoth et al., 2003; Brenner et al., 2005; Kiba et al., 2005; Nemhauser et al., 2006; Argyros et al., 2008; Li et al., 2010; Brenner and Schmulling, 2012; Nishiyama et al., 2012; Bhargava et al., 2013).
Figure 3. Cytokinin impact on the barley root proteome and metabolome. (A) The proteome profile separation of cytokinin- and mock-treated samples. Independent component analysis based on quantitative data of 972 proteins. Results of three biological replicates represent proteins with at least 10 matched spectra in a biological replicate and more than > 95% of the detected root proteome. (B) Expected localization of quantified barley root proteins and (C) visualization of organellar enrichment in cytokinin-responsive proteins. Localization based on data prediction by CropPal and SUBA4.0 (Hooper et al., 2016, 2017); (D) Interactions and functional clusters of cytokinin-responsive proteins highlighted by String (Szklarczyk et al., 2019). The selected highlighted categories are represented by at least five and ten proteins for ‘Biological Processes’ and ‘KEGG Pathways,’ respectively; (E) Cytokinin impact on barley root metabolism. Highlighted pathways were identified by an integrative analysis of identified cytokinin-responsive proteins and metabolites (MetaboAnalyst; Pang et al., 2020). Results (D-E) are based on data available for putative Arabidopsis orthologs.
Figure 4. Comparison of cytokinin- and abiotic stress-induced changes in roots of barley. (A) Heat map visualization of detected root metabolites. Asterisks indicate statistically significant changes (p < 0.05); (B) Similarities in the average metabolome composition visualized by PCA. Presented data are means and standard error (n = 6); (C) Clusters of treatments obtained from independent component analysis of protein profiles; Dashed circles represent statistically significant separation (Kruskal-Wallis test, p < 0.05).
A GC-MS metabolome analysis for barley seedling roots provided quantitative data for 80 different root metabolites, giving a snapshot of the key primary metabolism pathways as well as phenylpropanoid biosynthesis or polyamine production (Figure 4A, Supplementary Table S3). The cytokinin treatment induced a significant accumulation of N-acetyl serine, mannitol, pyroglutamic acid, coumaric acid, polyamine spermidine, and tryptamine. The accumulation of an osmoprotective compound (mannitol), the product of glutathione degradation (pyroglutamic acid), phenolic compound (coumaric acid), and an important precursor for many secondary metabolites (tryptamine) was matching the observed accumulation of stress-responsive proteins. In parallel, the set of 22 metabolites that were significantly depleted in response to cytokinin fully supported observed changes on the proteome level. Namely, the depletion of oxaloacetate and aspartate correlated with a decrease in aspartate aminotransferase and asparagine synthase. The depletion of aminoacylase and alanine aminotransferase coincided with a decrease in arginine and proline. And, finally, a decrease in lysine was likely the result of diaminopimelate aminotransferase repression. The observed accumulation of coumaric acid, depletion of aromatic amino acids phenylalanine and tyrosine matched the accumulation of enzymes of phenylpropanoid pathway (Figures 3E, 4A).
The list of cytokinin-responsive proteins and metabolites indicated a similarity between cytokinin and an abiotic stimuli. Next, a set of experiments was designed to explore this putative crosstalk, including the response to salinity, temperature and drought. The same stringent protein quantitation criteria were applied, resulting in the identification of 308 stress-responsive proteins (Supplementary Table S1). Notably, the overlap between abiotic stress and cytokinin response was high, and only 76 cytokinin-responsive proteins were not considered to be differentially abundant in response to any of the abiotic stimuli (Supplementary Figure S1).
The functional analysis (Figure 3D) indicated that 17 barley cytokinin-responsive proteins could be induced by salinity stress. In order to validate this potential crosstalk, seedlings were put for 24h into medium supplemented with 80 mM NaCl to elicit a salinity stress. The consecutive proteomics analyses revealed a statistical significant separation of salinity- and cytokinin-treated roots (Figure 4C), and a depletion and accumulation of 55 and 59 root proteins, respectively (Supplementary Table S1). The accumulated proteins included multiple glutathione S-transferases, glutathione reductase and superoxide dismutase, indicating an increase in the ROS metabolism. Roots under salinity stress also accumulated enzymes of phenylpropanoid biosynthetic pathway (phenylalanine ammonia-lyase, HORVU0Hr1G016330; alcohol dehydrogenase, HORVU6Hr1G035420), enzymes of energy metabolism, sulfur metabolism, and an ortholog of lipocalin that confers resistance to high salt in poplar (Abo-Ogiala et al., 2014). A decrease was found for proteins associated with cell wall organization or biogenesis, including several peroxidases or chitinase. In total, only four out of 17 putative salinity-responsive proteins were significantly changed under salinity stress, but the comparison with the response to cytokinin showed 26 similar changes, including an accumulation of a jasmonic acid biosynthetic enzyme (HORVU2Hr1G077220), phenylpropanoid biosynthetic enzymes, potassium channel subunit and a putative regulator of cell elongation (HORVU1Hr1G045630). A contrasting response was found for 15 proteins, including nuclease S1 and acid phosphatase (HORVU2Hr1G112830, HORVU4Hr1G085050; positive response to cytokinin).
The cytokinin treatment resulted in a decrease of TCTP protein (HORVU4Hr1G033200) with a putative role in drought tolerance (Kim et al., 2012), and an increase in an osmoprotective compound mannitol and at least five proteins associated with the drought stress response. Next, in a parallel experiment, the three-day-old seedlings were deprived of water for 24 h, reaching the relative water content 83.2 ± 0.4% of control roots, and the identified cytokinin-responsive proteins were quantified (Tables 1, 2, Supplementary Table S1). The results confirmed that cytokinin-responsive proteins significantly overlapped with the drought-responsive subset which is well in line with the previous reports suggesting that cytokinin may induce osmotic stress hypersensitivity (Karunadasa et al., 2020). In total, out of 70 identified drought-responsive proteins, 39 were cytokinin-responsive and the response to cytokinin and drought was similar for 35 of these, including an accumulation of the subunit of a voltage-gated potassium channel (HORVU6Hr1G091250), annexin (HORVU6Hr1G074440) and depletion of ferredoxin–nitrite reductase (HORVU6Hr1G080750) and multiple enzymes of carbohydrate and amino acid metabolism.
Cytokinin signaling is an integral part of temperature-stress response, including heat stress, cold stress and temperature acclimation (Jeon et al., 2010; Macková et al., 2013; Černý et al., 2014; Danilova et al., 2016; Skalák et al., 2016). Here, 16 cytokinin-responsive proteins were associated with a temperature-stress. To provide evidence for this putative link, three-day old seedlings in magenta boxes were put onto an ice-bath or heated block, and temperature in the growth medium was kept for 120 min at 4 ± 2 and 30 ± 1°C to emulate cold- and heat-stress, respectively. The root tissue was harvested after 22 h of recovery at 20°C. Proteomics analysis revealed 152 and 108 cold- and heat-stress responsive proteins, respectively. Surprisingly, only 27 proteins were found to be differentially abundant in the both datasets and only seven of these manifested a temperature-dependent pattern, namely ribosomal protein S28 (HORVU3Hr1G115820), glutathione S-transferase (HORVU5Hr1G103420), peptidyl-prolyl cis-trans isomerase (HORVU6Hr1G077340), small heat shock protein (HORVU4Hr1G063350), aldehyde dehydrogenase 12A1 (HORVU1Hr1G080320), annexin 7 (HORVU6Hr1G074440) and asparagine–tRNA ligase (HORVU1Hr1G021130). The comparison with cytokinin-responsive proteins revealed a modest overlap, with 54 and 42 differentially abundant proteins for plants after a period of cold- and heat-stress, respectively. In total, out of 178 cytokinin-responsive proteins, 81 were found in the set of temperature-stress-responsive proteins, and most shared a similar response between temperature-stress and cytokinin treatment (Tables 1, 2, Supplementary Table S1, Supplementary Figure S1).
Metabolome profiling showed a surprising similarity between cytokinin treatment and abiotic stress (Figures 4A,C). Notable similarity was found for the depletion of oxaloacetic acid and amino acids, including serine and proline. Proline is a well-known osmoprotectant, and its content should gradually increase upon prolonged stress. However, it has been shown that the initial free proline content in barley roots is very low and at least 48 h of salinity stress are required for any positive changes to be manifested (Ueda et al., 2007). The most similar metabolome response to cytokinin of the abiotic stresses examined was found in samples that experienced a period of cold stress, sharing 23 of 29 differentially abundant metabolites. Not all of the observed changes in roots under abiotic stress were deemed statistically significant (compared to the mock-treated controls; p < 0.05), but analysis of principal components confirmed that all treatments were distinct from the control, but not indistinguishable from each other (Figure 4B). In contrast, the comparison of proteome profiles by independent component analysis (Figure 4C) showed statistically significant separation of cytokinin treatment, salinity stress (IC1), and cold stress (IC2) from each other and both drought and heat stress that were similar to the mock control treatment. In total, 46 proteins were found with a similar and statistically significant response in at least three different treatments. These proteins included an enzyme of ascorbate biosynthesis (HORVU5Hr1G079230; positive response to temperature stress and drought), Hsp70-Hsp90 organizing protein (HORVU2Hr1G092190; positive response to cytokinin, cold- and drought-stress), annexin (HORVU6Hr1G074440; positive response to cytokinin, heat, drought and salinity), phenyl ammonium lyase (HORVU0Hr1G016330; positive response to cytokinin, heat and salinity) and a subunit of potassium channel (HORVU6Hr1G091250; positive response to cytokinin, drought, salinity). Only three proteins were found with a similar and statistically significant response in all experiments indicating that they have a role in the general response to external stimuli, namely thioredoxin-like protein (HORVU7Hr1G046280; depleted), NAD(P)H dehydrogenase (HORVU3Hr1G077250; depleted) and unknown protein HORVU2Hr1G098860 (depleted).
The measurement of aqueous hydrogen peroxide showed that the cytokinin treatment significantly reduced hydrogen peroxide content in barley roots by more than 25% (Figure 5A). The histochemical staining indicated that the reduction in the root tips could be even higher and a similar response was found for superoxide radicals (Figures 5B–E).
Figure 5. Cytokinin impact on the ROS production. The estimated mean content of hydrogen peroxide in barley roots determined by (A) PeroxiDetect Kit and (B) 3,3′-diaminobenzidine (DAB) staining; (C) Cytokinin impact on superoxide radical production as estimated by histochemical staining with nitroblue tetrazolium (NBT); Representative images of cytokinin- and mock-treated roots stained with DAB (D) and NBT (E). Presented data are means and standard deviation of at least three biological replicates; Statistically significant differences (Student’s t-test) are indicated.
Changes in ROS metabolism enzymes have been previously reported in response to all major phytohormones (Černý et al., 2016), and it has been demonstrated that the ROS metabolism does not only represent a general stress response, but that it is required for mediating growth. For instance, phytohormone auxin promotes ROS production to facilitate cell wall loosening and cell elongation (Huan et al., 2016; Voxeur and Höfte, 2016). Here, out of more than 90 proteins involved in ROS metabolism, 11 were significantly differentially abundant in response to cytokinin, indicating an increase in the ROS production. Cytokinin application induced accumulation of all detected catalase isoforms (HORVU6Hr1G008640, HORVU7Hr1G121700), peroxidases (HORVU2Hr1G124970, HORVU1Hr1G016660), an enzyme that predicted to act on lipid peroxide-derived reactive aldehydes (HORVU3Hr1G015640), and a minor isoform of glutathione S-transferase (HORVU4Hr1G081100; less than 2% of the amount of all detected isoforms). Peroxiredoxin (HORVU3Hr1G037720) together with glutaredoxin (HORVU3Hr1G023970) and thioredoxin (HORVU7Hr1G046280) were depleted, as well as two peroxidases (HORVU2Hr1G125200, HORVU3Hr1G112350). The induction of ROS catabolism resulted in a decrease of hydrogen peroxide and superoxide radicals in the roots (Figure 5). This induction was likely triggered by a transient increase in the ROS production, which could correlate with an increase in spermidine and a depletion of its precursor putrescine (Figure 4A). Spermidine is a precursor of spermine (no difference in response to cytokinin detected) and both these polyamines reportedly mediate protection against oxidative damage caused by hydrogen peroxide (Rider et al., 2007). Polyamine production is also reportedly linked with an increase activity of catalase and glutathione S-transferase (Seifi and Shelp, 2019) which could coincide with the observed changes at the proteome level. Metabolomic data also indicate a higher turnover rate for glutathione, with a decrease of its precursor O-acetyl-serine and an increase in pyroglutamic acid (glutathione degradation product). However, a decrease in O-acetyl-serine could also be the result of cytokinin inactivation by zeatin 9-aminocarboxyethyltransferase which converts O-acetyl-serine and trans-zeatin into lupinic acid (Entsch et al., 1983). The existence of this enzyme found in Fabaceae has not yet been confirmed in barley, but the available data cannot exclude this possibility.
Drought and salinity are globally the most frequent abiotic stresses, and both significantly impair crop yields. Barley is more resilient to salinity than other cereals with some cultivars tolerating up to 250 mM NaCl (Hanin et al., 2016). Here, we employed 80 mM NaCl that showed only a mild effect on the leaf area (less than 10% reduction at a prolonged treatment; results of preliminary experiments, data not shown) but still elicited a more potent response on the seedling root proteome than the water deprivation (Supplementary Table S1 and Supplementary Figure S1). Modulations of cytokinin metabolism or signaling can improve drought and salt tolerance, and it has been demonstrated that both the depletion of cytokinin and an increase in the cytokinin pool can promote plant growth under abiotic stress (e.g., Nishiyama et al., 2012; Prerostova et al., 2018). The exact mechanisms are not yet fully understood, but at least a part of the cytokinin promoted stress alleviation could reflect the priming of antioxidant systems (Nakabayashi et al., 2014). The similar priming was found here (as discussed above). Further, abundances of 48 cytokinin-responsive root proteins were found with a similar response under salinity stress or water deprivation (Supplementary Figure S1). It is thus tempting to speculate that this cytokinin-induced priming could be responsible for an enhanced resilience found in plants with modulated cytokinin pool.
The proteome profiling revealed that ca. 5% of the root proteome is formed by heat shock proteins. Heat shock proteins are ubiquitous and widely spread proteins across all taxonomic kingdoms. These proteins were first discovered in the response to an increase in temperature, but accumulated evidence indicates that they are involved in diverse processes. Besides their chaperon functions, HSPs participate in proteasomal degradation, protein-protein interactions and may also play a role in signaling cascades (e.g., McLoughlin et al., 2019; ul Haq et al., 2019; Tichá et al., 2020). Here, seven heat shock proteins (representing ca. 10% of the total identified HSP protein amount) were significantly depleted in response to cytokinin, including three and four representatives of the HSP70 and sHSP family, respectively. Proteins HSP70-6 (an ortholog of HORVU4Hr1G089090 and HORVU4Hr1G012460) and HSP21 (HORVU4Hr1G063350) were found to be essential for chloroplast development and thermotolerance in germinating seeds of Arabidopsis (Latijnhouwers et al., 2010; Zhong et al., 2013). In general, the exogenous cytokinin treatment elicited (at least partially) a low-temperature response in barley roots, which was manifested in the observed significant changes at the metabolome level and 37 proteins with a cytokinin-like response under cold stress. However, all four cytokinin-responsive sHSP in barley roots were accumulated after a period of cold-stress and not affected or depleted in response to a period of heat. This indicates a variable role of cytokinin in temperature stress response and is well in line with the previous contrasting reports of cytokinin role in cold- and heat-shock response and temperature acclimation.
Cytokinin suppressed membrane steroid binding protein 1 (HORVU1Hr1G045630), an ortholog of Arabidopsis MSBP1 which regulates lignin biosynthesis, negatively regulates brassinosteroid signaling and cell elongation (Yang et al., 2005; Gou et al., 2018). A downregulation of MSBP in Arabidopsis resulted in a lower lignin deposition, and the accumulation of soluble phenolics in the monolignol branch (Gou et al., 2018). This would explain the observed significant accumulation of 4-coumaric acid which was specific for cytokinin treatment (Figure 4A). It has been previously reported that cytokinin signaling could result in a reduced lignification (reviewed in Didi et al., 2015), and results reported here indicate that MSBP could be its direct target. There are at least two plausible explanations for this cytokinin effect supported in the barley root dataset. First, lowering lignification could coincide with the cytokinin-induced accumulation of expansin B2 (HORVU1Hr1G054240) which is expected (by similarity) to cause loosening and extension of plant cell walls. Alternatively (or in parallel), cytokinin-induced reduction of lignin biosynthesis could be a switch in the phenylpropanoid pathway, promoting anthocyanin production, which is a well-known cytokinin response (Deikman and Hammer, 1995). This alternative is supported by the accumulation of phenylpropanoid biosynthetic enzymes, including two isoforms of phenylalanine ammonia-lyase 1 (HORVU0Hr1G016330, HORVU6Hr1G058820) or flavone 3′-O-methyltransferase 1 (HORVU7Hr1G082280).
The cytokinin response has been extensively characterized in the model plant Arabidopsis thaliana, but an equivalent study of a crop plant has been largely missing. This work provided the first insight into the cytokinin-responsive proteins and metabolites in developing roots of barley seedling. The observed overlap with known cytokinin-responsive genes and proteins showed that this could be an excellent model for identifying hormone-responsive proteins and for the analysis of intensive crosstalk between plant hormones and abiotic signaling pathways.
Grain samples of Hordeum vulgare L. sensu lato variety (Sebastian) were obtained from field grown plants from the breeding station Stupice (Czechia) in 2016 and were stored in a sealed container at 4°C. For germination assay, grains were imbibed in 4 ml water supplemented with 5 × 10–4% (v/v) dimethyl sulfoxide (mock buffer) or 1 μM phytohormone (tZ - trans-zeatin; IAA - indole-3-acetic acid; GA3 - gibberellic acid; ABA – abscisic acid; Duchefa) in dimethyl sulfoxide (final concentration, as for the mock) and incubated at 20°C for 48 h in dark. For root proteome and metabolome analysis, grains were surface-sterilized (2% hypochlorite), imbibed and stratified at 4°C for 48 h. Stratified seeds were transferred onto half-strength Murashige & Skoog medium and placed in a growth chamber providing 20°C and 16/8 h light/dark cycles with 100 μmol m–2 s–1 photon flux density during light periods. After 72 h, sets of 10 germinated seedlings were exposed to 1 μM trans-zeatin or an abiotic stress by exposure to: 30°C or 4°C temperature for 2 h followed by a 22 recovery period at 20°C; medium supplemented with 80 mM NaCl (final concentration); or drought (by transfer to a dry Magenta box). 24 h after each treatment, the root tissue was dissected from the shoot, frozen and stored at −80°C. All experiments were carried out in at least three biological replicates, each consisting of 10 seedlings per sample.
Total protein extracts were prepared as previously described (Hloušková et al., 2019) employing a combination of phenol/acetone/TCA extraction. Portions of samples corresponding to 5 μg of peptide were analyzed by nanoflow reverse-phase liquid chromatography-mass spectrometry using a 15 cm C18 Zorbax column (Agilent), a Dionex Ultimate 3000 RSLC nano-UPLC system (Thermo) and a qTOF maXis Impact mass spectrometer (Bruker) as previously described (Dufková et al., 2019). Peptides were eluted with up to a 120-min, 4% to 40% acetonitrile gradient. MS spectra were acquired at 2 Hz, while MS/MS spectra were acquired between 10–20 Hz using an intensity-dependent mode with a total cycle time of 7 s. The acquired spectra were recalibrated and searched against the reference barley (Mascher et al., 2017) by Proteome Discoverer 2.0, employing Sequest HT with the following parameters: Enzyme - trypsin, max two missed cleavage sites; Modifications - up to three dynamic modifications including Met oxidation, Asn/Gln deamidation, N-terminal acetylation, Met-loss (protein N-terminus), Met-loss + Acetylation (protein N-terminus); MS1 tolerance −35 ppm, MS2 tolerance −0.1 Da (Sequest). The quantitative differences were determined by the spectral counting method, followed by normalization and t-test (compared to the mock-treated roots; p-value < 0.05). For selected candidate proteins, the corresponding peptide peak areas were evaluated in Skyline (Pino et al., 2020). The proteomic data acquired have been deposited to the ProteomeXchange Consortium1 via the PRIDE partner repository (Vizcaíno et al., 2016) with the dataset identifier PXD020627.
Polar metabolites were extracted as previously described with few modifications (Cerna et al., 2017) and measured using a Q Exactive GC Orbitrap GC-tandem mass spectrometer and Trace 1300 Gas chromatograph (Thermo Fisher). Samples were injected using the split mode (inlet temperature 250°C, splitless time 0.8 min, purge flow 5.0 ml/min, split flow 6.0 ml/min) onto TG-5SILMS GC Column (Thermo Fisher, 30 m × 0.25 mm × 0.25 μm) with helium as a carrier gas at a constant flow of 1.2 ml/min. Metabolites were separated with a 28 min gradient (70°C for 5 min followed by 9°C per min gradient to 320°C and finally 10 min hold time) and ionized using the electron ionization mode (electron energy 70 eV, emission current 50 μA, transfer line and ion source temperature 250°C). The MS operated in the full scan mode, 60000 resolution, scan range 50–750 m/z, automatic maximum allowed injection time with automatic gain control set to 1e6, and lock mass [m/z]: 207.0323. Data were analyzed by TraceFinder 4.1 with Deconvolution Plugin 1.4 (Thermo) and searched against NIST2014, GC-Orbitrap Metabolomics library and inhouse library. Only metabolites fulfilling identification criteria (score ≥ 75 and ΔRI < 2%) were included in the final list.
The lyophilized root tissue was homogenized and aliquots corresponding to 30–40 mg were analyzed using PeroxiDetect Kit (Sigma-Aldrich) according to the manufacturer’s instructions. The distribution of hydrogen peroxide and superoxide radical was visualized by endogenous peroxidase-dependent histochemical staining using 3,3′-diaminobenzidine (e.g., Novák et al., 2013) and nitroblue tetrazolium, respectively (Zhang et al., 2014). The staining intensity was quantified using ImageJ 1.53e (Schneider et al., 2012).
The reported statistical tests were generated and implemented using Instant Clue (Nolte et al., 2018), Rapid Miner (www.rapidminer.com; Mierswa et al., 2006) and Proteome Discoverer. Significant differences refer to p < 0.05.
The datasets presented in this study can be found in online repositories. The names of the repository/repositories and accession number(s) can be found below: https://www.ebi.ac.uk/pride/archive/, PXD020627.
MC and MB designed research. MC, MB, and HD performed research. MC, MB, ML, JN, IS-F, AR, and BB analyzed data. MC prepared figures and wrote the manuscript. All authors contributed to the article and approved the submitted version.
Funding support for this work was provided by: the Ministry of Education, Youth and Sports of the Czech Republic (CR) to MC, MB, and BB (grant no. CZ.02.1.01/0.0/0.0/16_019/0000738) with support from the European Regional Development Fund “Centre for Experimental Plant Biology”; Mendel University in Brno grants (AF-IGA2019-IP081 and AF-IGA2020-IP048) to ML and MB; and the LQ1601 (CEITEC 2020) project, with financial contribution by the Ministry of Education, Youths and Sports of CR from National Programme for Sustainability II funds to BB.
The authors declare that the research was conducted in the absence of any commercial or financial relationships that could be construed as a potential conflict of interest.
We thank Vladěna Liberdová and Simona Menšíková for their technical assistance throughout the project, Dr. Vratislav Psota for providing barley grains and Brno City Municipality for the Brno Ph.D. Talent Scholarship.
The Supplementary Material for this article can be found online at: https://www.frontiersin.org/articles/10.3389/fpls.2020.590337/full#supplementary-material
Supplementary Figure 1 | Differentially abundant proteins in response to cytokinin or abiotic stimuli. Venn diagram summarizing (A) all identified differentially abundant proteins; (B) significantly accumulated proteins; and (C) significantly depleted proteins.
Supplementary Table 1 | Differentially abundant proteins in response to cytokinin or abiotic stimuli. Supplementary table to Figures 1, 3, 4 and Tables 1, 2.
Supplementary Table 2 | Results of proteome profiling. All identified proteins and peptides. Supplementary table to Figure 1 and Figure 3.
Supplementary Table 3 | Results of metabolome profiling. Supplementary table to Figure 4.
Abo-Ogiala, A., Carsjens, C., Diekmann, H., Fayyaz, P., Herrfurth, C., Feussner, I., et al. (2014). Temperature-induced lipocalin (TIL) is translocated under salt stress and protects chloroplasts from ion toxicity. J. Plant Physiol. 171, 250–259. doi: 10.1016/j.jplph.2013.08.003
Argueso, C. T., Ferreira, F. J., and Kieber, J. J. (2009). Environmental perception avenues: the interaction of cytokinin and environmental response pathways. Plant. Cell Environ. 32, 1147–1160. doi: 10.1111/j.1365-3040.2009.01940.x
Argyros, R. D., Mathews, D. E., Chiang, Y.-H., Palmer, C. M., Thibault, D. M., Etheridge, N., et al. (2008). Type B response regulators of Arabidopsis play key roles in cytokinin signaling and plant development. Plant Cell 20, 2102–2116.
Bewley, J. D., Bradford, K. J., Hilhorst, H. W. M., and Nonogaki, H. (2013). Seeds, 3rd Edn. New York, NY: Springer, doi: 10.1007/978-1-4614-4693-4
Bhargava, A., Clabaugh, I., To, J. P., Maxwell, B. B., Chiang, Y.-H., Schaller, E. G., et al. (2013). Identification of cytokinin responsive genes using microarray meta-analysis and RNA-seq in Arabidopsis thaliana. Plant Physiol 162, 272–294.
Bielach, A., Podlešáková, K., Marhavý, P., Duclercq, J., Cuesta, C., Müller, B., et al. (2012). Spatiotemporal regulation of lateral root organogenesis in arabidopsis by cytokinin. Plant Cell 24, 3967–3981. doi: 10.1105/tpc.112.103044
Blattner, F. R. (2018). “Taxonomy of the Genus Hordeum and Barley (Hordeum vulgare),” in The Barley Genome, eds N. Stein and G. J. Muehlbauer (New York, NY: Springer), 11–23. doi: 10.1007/978-3-319-92528-8_2
Brenner, W. G., Romanov, G. A., Köllmer, I., Bürkle, L., and Schmülling, T. (2005). Immediate-early and delayed cytokinin response genes of Arabidopsis thaliana identified by genome-wide expression profiling reveal novel cytokinin-sensitive processes and suggest cytokinin action through transcriptional cascades. Plant J. 44, 314–333. doi: 10.1111/j.1365-313X.2005.02530.x
Brenner, W. G., and Schmulling, T. (2012). Transcript profiling of cytokinin action in Arabidopsis roots and shoots discovers largely similar but also organ-specific responses. BMC Plant Biol. 12:112. doi: 10.1186/1471-2229-12-112
Cerna, H., Černý, M., Habánová, H., Šafářová, D., Abushamsiya, K., Navrátil, M., et al. (2017). Proteomics offers insight to the mechanism behind Pisum sativum L. response to pea seed-borne mosaic virus (PSbMV). J. Proteomics 153, 78–88. doi: 10.1016/j.jprot.2016.05.018
Černý, M., Dyčka, F., Bobál’ová, J., and Brzobohaty, B. (2011). Early cytokinin response proteins and phosphoproteins of Arabidopsis thaliana identified by proteome and phosphoproteome profiling. J. Exp. Bot. 62, 921–937. doi: 10.1093/jxb/erq322
Černý, M., Jedelský, P. L., Novák, J., Schlosser, A., and Brzobohatý, B. (2014). Cytokinin modulates proteomic, transcriptomic and growth responses to temperature shocks in Arabidopsis. Plant Cell Environ. 37, 1641–1655. doi: 10.1111/pce.12270
Černý, M., Novák, J., Habánová, H., Cerna, H., and Brzobohatý, B. (2016). Role of the proteome in phytohormonal signaling. Biochim. Biophys. Acta Prot. Proteom. 1864, 1003–1015. doi: 10.1016/j.bbapap.2015.12.008
Chen, Y., Hoehenwarter, W., and Weckwerth, W. (2010). Comparative analysis of phytohormone-responsive phosphoproteins in Arabidopsis thaliana using TiO2-phosphopeptide enrichment and mass accuracy precursor alignment. Plant J. 63, 1–17.
Chory, J., Reinecke, D., Sim, S., Washburn, T., and Brenner, M. (1994). A role for cytokinins in De-etiolation in Arabidopsis (det mutants have an altered response to cytokinins). Plant Physiol. 104, 339–347. doi: 10.1104/pp.104.2.339
Cortleven, A., Leuendorf, J. E., Frank, M., Pezzetta, D., Bolt, S., and Schmülling, T. (2019). Cytokinin action in response to abiotic and biotic stresses in plants. Plant. Cell Environ. 42, 998–1018. doi: 10.1111/pce.13494
Danilova, M. N., Kudryakova, N. V., Doroshenko, A. S., Zabrodin, D. A., Vinogradov, N. S., and Kuznetsov, V. V. (2016). Molecular and physiological responses of Arabidopsis thaliana plants deficient in the genes responsible for ABA and cytokinin reception and metabolism to heat shock. Russ. J. Plant Physiol. 63, 308–318. doi: 10.1134/S1021443716030043
Dawson, I. K., Russell, J., Powell, W., Steffenson, B., Thomas, W. T. B., and Waugh, R. (2015). Barley: a translational model for adaptation to climate change. New Phytol. 206, 913–931. doi: 10.1111/nph.13266
Deikman, J., and Hammer, P. E. (1995). Induction of anthocyanin accumulation by cytokinins in Arabidopsis thaliana. Plant Physiol. 108, 47–57. doi: 10.1104/pp.108.1.47
Didi, V., Jackson, P., and Hejátko, J. (2015). Hormonal regulation of secondary cell wall formation. J. Exp. Bot. 66, 5015–5027. doi: 10.1093/jxb/erv222
Dufková, H., Berka, M., Luklová, M., Rashotte, A. M., Brzobohatý, B., and Černý, M. (2019). Eggplant germination is promoted by hydrogen peroxide and temperature in an independent but overlapping manner. Molecules 24:4270. doi: 10.3390/molecules24234270
Entsch, B., Parker, C. W., and Letham, D. S. (1983). An enzyme from lupin seeds forming alanine derivatives of cytokinins. Phytochemistry 22, 375–381. doi: 10.1016/0031-9422(83)83008-8
Ge, S. X., Jung, D., and Yao, R. (2020). ShinyGO: a graphical gene-set enrichment tool for animals and plants. Bioinformatics 36, 2628–2629. doi: 10.1093/bioinformatics/btz931
Gou, M., Ran, X., Martin, D. W., and Liu, C. J. (2018). The scaffold proteins of lignin biosynthetic cytochrome P450 enzymes. Nat. Plants 4, 299–310. doi: 10.1038/s41477-018-0142-9
Guan, C., Wang, X., Feng, J., Hong, S., Liang, Y., Ren, B., et al. (2014). Cytokinin antagonizes abscisic acid-mediated inhibition of cotyledon greening by promoting the degradation of ABSCISIC ACID INSENSITIVE5 protein in Arabidopsis. PLANT Physiol. 164, 1515–1526. doi: 10.1104/pp.113.234740
Gupta, R., Wang, Y., Agrawal, G. K., Rakwal, R., Jo, I. H., Bang, K. H., et al. (2015). Time to dig deep into the plant proteome: a hunt for low-abundance proteins. Front. Plant Sci 6:22. doi: 10.3389/fpls.2015.00022
Hallmark, H. T., Černý, M., Brzobohatý, B., and Rashotte, A. M. (2020). trans-Zeatin-N-glucosides have biological activity in Arabidopsis thaliana. PLoS One 15:e0232762. doi: 10.1371/journal.pone.0232762
Hanin, M., Ebel, C., Ngom, M., Laplaze, L., and Masmoudi, K. (2016). New insights on plant salt tolerance mechanisms and their potential use for breeding. Front. Plant Sci 7:1787. doi: 10.3389/fpls.2016.01787
Harwood, W. A. (2019). An introduction to barley: the crop and the model. Methods Mol. Biol. 1900, 1–5. doi: 10.1007/978-1-4939-8944-7_1
Hloušková, P., Černý, M., Kořínková, N., Luklová, M., Minguet, E. G., Brzobohatý, B., et al. (2019). Affinity chromatography revealed 14-3-3 interactome of tomato (Solanum lycopersicum L.) during blue light-induced de-etiolation. J. Proteomics 193, 44–61. doi: 10.1016/j.jprot.2018.12.017
Holubová, K., Hensel, G., Vojta, P., Tarkowski, P., Bergougnoux, V., and Galuszka, P. (2018). Modification of barley plant productivity through regulation of cytokinin content by reverse-genetics approaches. Front. Plant Sci. 9:1676. doi: 10.3389/fpls.2018.01676
Hooper, C. M., Castleden, I. R., Aryamanesh, N., Jacoby, R. P., and Millar, A. H. (2016). Finding the subcellular location of barley, wheat, rice and maize proteins: the compendium of crop proteins with Annotated Locations (cropPAL). Plant Cell Physiol. 57:e9. doi: 10.1093/pcp/pcv170
Hooper, C. M., Castleden, I. R., Tanz, S. K., Aryamanesh, N., and Millar, A. H. (2017). SUBA4: the interactive data analysis centre for Arabidopsis subcellular protein locations. Nucleic Acids Res. 45, D1064–D1074. doi: 10.1093/nar/gkw1041
Hoth, S., Ikeda, Y., Morgante, M., Wang, X., Zuo, J., Hanafey, M. K., et al. (2003). Monitoring genome-wide changes in gene expression in response to endogenous cytokinin reveals targets in Arabidopsis thaliana. FEBS Lett. 554, 373–380. doi: 10.1016/S0014-5793(03)01194-3
Huan, C., Jiang, L., An, X., Yu, M., Xu, Y., Ma, R., et al. (2016). Potential role of reactive oxygen species and antioxidant genes in the regulation of peach fruit development and ripening. Plant Physiol. Biochem. 104, 294–303. doi: 10.1016/j.plaphy.2016.05.013
Huang, Y., Sun, M.-M., Ye, Q., Wu, X.-Q., Wu, W.-H., and Chen, Y.-F. (2017). Abscisic Acid modulates seed germination via ABA INSENSITIVE5-mediated PHOSPHATE1. Plant Physiol. 175, 1661–1668. doi: 10.1104/pp.17.00164
Jeon, J., Kim, N. Y., Kim, S., Kang, N. Y., Novák, O., Ku, S. J., et al. (2010). A subset of cytokinin two-component signaling system plays a role in cold temperature stress response in Arabidopsis. J. Biol. Chem. 285, 23371–23386. doi: 10.1074/jbc.M109.096644
Karunadasa, S. S., Kurepa, J., Shull, T. E., and Smalle, J. A. (2020). Cytokinin-induced protein synthesis suppresses growth and osmotic stress tolerance. New Phytol. 227, 50–64. doi: 10.1111/nph.16519
Kaur, N., Erickson, T. E., Ball, A. S., and Ryan, M. H. (2017). A review of germination and early growth as a proxy for plant fitness under petrogenic contamination — knowledge gaps and recommendations. Sci. Total Environ. 603, 728–744. doi: 10.1016/j.scitotenv.2017.02.179
Kiba, T., Yamashino, T., Naito, T., Koizumi, N., Sakakibara, H., and Mizuno, T. (2005). Combinatorial microarray analysis revealing araidopsis genes implicated in cytokinin responses through the His-to-Asp phosphorelay circuitry. Plant Cell Physiol. 46:S48.
Kim, Y. M., Han, Y. J., Hwang, O. J., Lee, S. S., Shin, A. Y., Kim, S. Y., et al. (2012). Overexpression of arabidopsis translationally controlled tumor protein gene AtTCTP enhances drought tolerance with rapid ABA-induced stomatal closure. Mol. Cells 33, 617–626. doi: 10.1007/s10059-012-0080-8
Kurepa, J., Shull, T. E., and Smalle, J. A. (2019). Antagonistic activity of auxin and cytokinin in shoot and root organs. Plant Dir. 3:e00121. doi: 10.1002/pld3.121
Laplaze, L., Benkova, E., Casimiro, I., Maes, L., Vanneste, S., Swarup, R., et al. (2007). Cytokinins act directly on lateral root founder cells to inhibit root initiation. Plant Cell 19, 3889–3900. doi: 10.1105/tpc.107.055863
Latijnhouwers, M., Xu, X. M., and Møller, S. G. (2010). Arabidopsis stromal 70-kDa heat shock proteins are essential for chloroplast development. Planta 232, 567–578. doi: 10.1007/s00425-010-1192-z
Li, X. G., Su, Y. H., Zhao, X. Y., Li, W., Gao, X. Q., and Zhang, X. S. (2010). Cytokinin overproduction-caused alteration of flower development is partially mediated by CUC2 and CUC3 in Arabidopsis. Gene 450, 109–120.
Liu, M.-S., Li, H.-C., Chang, Y.-M., Wu, M.-T., and Chen, L.-F. O. (2011). Proteomic analysis of stress-related proteins in transgenic broccoli harboring a gene for cytokinin production during postharvest senescence. Plant Sci. 181, 288–299. doi: 10.1016/j.plantsci.2011.06.005
Lochmanová, G., Zdráhal, Z., Konečná, H., Koukalová, Š, Malbeck, J., Souček, P., et al. (2008). Cytokinin-induced photomorphogenesis in dark-grown Arabidopsis: a proteomic analysis. J. Exp. Bot 59, 3705–3719. doi: 10.1093/jxb/ern220
Macková, H., Hronková, M., Dobrá, J., Turečková, V., Novák, O., Lubovská, Z., et al. (2013). Enhanced drought and heat stress tolerance of tobacco plants with ectopically enhanced cytokinin oxidase/dehydrogenase gene expression. J. Exp. Bot. 64, 2805–2815. doi: 10.1093/jxb/ert131
Mascher, M., Gundlach, H., Himmelbach, A., Beier, S., Twardziok, S. O., Wicker, T., et al. (2017). A chromosome conformation capture ordered sequence of the barley genome. Nature 544, 427–433. doi: 10.1038/nature22043
Mayer, K. F. X., Waugh, R., Langridge, P., Close, T. J., Wise, R. P., Graner, A., et al. (2012). A physical, genetic and functional sequence assembly of the barley genome. Nature 491, 711–716. doi: 10.1038/nature11543
McLoughlin, F., Kim, M., Marshall, R. S., Vierstra, R. D., and Vierling, E. (2019). HSP101 interacts with the proteasome and promotes the clearance of ubiquitylated protein aggregates. Plant Physiol. 180, 1829–1847. doi: 10.1104/pp.19.00263
Mierswa, I., Wurst, M., Klinkenberg, R., Scholz, M., and Euler, T. (2006). “YALE,” in Proceedings of the 12th ACM SIGKDD International Conference On Knowledge Discovery and Data Mining - KDD ’06. New York, NY: Association for Computing Machinery, 935. doi: 10.1145/1150402.1150531
Mrízová, K., Jiskrová, E., Vyroubalová, Š, Novák, O., Ohnoutková, L., Pospíšilová, H., et al. (2013). Overexpression of Cytokinin Dehydrogenase Genes in Barley (Hordeum vulgare cv. Golden Promise) fundamentally affects morphology and fertility. PLoS One 8:e79029. doi: 10.1371/journal.pone.0079029
Müller, B., and Sheen, J. (2008). Cytokinin and auxin interaction in root stem-cell specification during early embryogenesis. Nature 453, 1094–1097. doi: 10.1038/nature06943
Nakabayashi, R., Yonekura-Sakakibara, K., Urano, K., Suzuki, M., Yamada, Y., Nishizawa, T., et al. (2014). Enhancement of oxidative and drought tolerance in Arabidopsis by overaccumulation of antioxidant flavonoids. Plant J. 77, 367–379. doi: 10.1111/tpj.12388
Nemhauser, J. L., Hong, F., and Chory, J. (2006). Different plant hormones regulate similar processes through largely nonoverlapping transcriptional responses. Cell 126, 467–475.
Nishiyama, R., Le, D. T., Watanabe, Y., Matsui, A., Tanaka, M., Seki, M., et al. (2012). Transcriptome analyses of a salt-tolerant cytokinin-deficient mutant reveal differential regulation of salt stress response by cytokinin deficiency. PLoS One 7:e32124. doi: 10.1371/journal.pone.0032124
Nolte, H., MacVicar, T. D., Tellkamp, F., and Krüger, M. (2018). Instant clue: a software suite for interactive data visualization and analysis. Sci. Rep. 8:12648. doi: 10.1038/s41598-018-31154-6
Novák, J., Pavlù, J., Novák, O., Nožková-Hlaváčková, V., Špundová, M., Hlavinka, J., et al. (2013). High cytokinin levels induce a hypersensitive-like response in tobacco. Ann. Bot. 112, 41–55. doi: 10.1093/aob/mct092
Pang, Z., Chong, J., Li, S., and Xia, J. (2020). MetaboAnalystR 3.0: toward an optimized workflow for global metabolomics. Metabolites 10:186. doi: 10.3390/metabo10050186
Pavlů, J., Novák, J., Koukalová, V., Luklová, M., Brzobohatý, B., and Černý, M. (2018). Cytokinin at the crossroad of abiotic stress signalling pathways. Int. J. Mol. Sci. 19:2450.
Pino, L. K., Searle, B. C., Bollinger, J. G., Nunn, B., MacLean, B., and MacCoss, M. J. (2020). The Skyline ecosystem: informatics for quantitative mass spectrometry proteomics. Mass Spectrom. Rev. 39, 229–244. doi: 10.1002/mas.21540
Pospíšilová, H., Jiskrová, E., Vojta, P., Mrízová, K., Kokáš, F., Čudejková, M. M., et al. (2016). Transgenic barley overexpressing a cytokinin dehydrogenase gene shows greater tolerance to drought stress. N. Biotechnol. 33, 692–705. doi: 10.1016/J.NBT.2015.12.005
Powell, A. F., Paleczny, A. R., Olechowski, H., and Emery, R. J. N. (2013). Changes in cytokinin form and concentration in developing kernels correspond with variation in yield among field-grown barley cultivars. Plant Physiol. Biochem 64, 33–40. doi: 10.1016/j.plaphy.2012.12.010
Prerostova, S., Dobrev, P. I., Gaudinova, A., Knirsch, V., Körber, N., Pieruschka, R., et al. (2018). Cytokinins: their impact on molecular and growth responses to drought stress and recovery in Arabidopsis. Front. Plant Sci. 9:655. doi: 10.3389/fpls.2018.00655
Ramireddy, E., Hosseini, S. A., Eggert, K., Gillandt, S., Gnad, H., von Wirén, N., et al. (2018). Root engineering in barley: increasing cytokinin degradation produces a larger root system, mineral enrichment in the shoot and improved drought tolerance. Plant Physiol. 177, 1078–1095. doi: 10.1104/pp.18.00199
Rider, J. E., Hacker, A., Mackintosh, C. A., Pegg, A. E., Woster, P. M., and Casero, R. A. (2007). Spermine and spermidine mediate protection against oxidative damage caused by hydrogen peroxide. Amino Acids 33, 231–240. doi: 10.1007/s00726-007-0513-4
Riefler, M., Novak, O., Strnad, M., and Schmülling, T. (2006). Arabidopsis cytokinin receptor mutants reveal functions in shoot growth, leaf senescence, seed size, germination, root development, and cytokinin metabolism. Plant Cell 18, 40–54. doi: 10.1105/tpc.105.037796
Righetti, P. G., and Boschetti, E. (2016). Global proteome analysis in plants by means of peptide libraries and applications. J. Proteomics 143, 3–14. doi: 10.1016/j.jprot.2016.02.033
Schneider, C. A., Rasband, W. S., and Eliceiri, K. W. (2012). NIH Image to ImageJ: 25 years of image analysis. Nat. Methods 9, 671–675. doi: 10.1038/nmeth.2089
Seifi, H. S., and Shelp, B. J. (2019). Spermine differentially refines plant defense responses against biotic and abiotic stresses. Front. Plant Sci 10:117. doi: 10.3389/fpls.2019.00117
Skalák, J., Černý, M., Jedelský, P., Dobrá, J., Ge, E., Novák, J., et al. (2016). Stimulation of ipt overexpression as a tool to elucidate the role of cytokinins in high temperature responses of Arabidopsis thaliana. J. Exp. Bot. 67, 2861–2873. doi: 10.1093/jxb/erw129
Skalák, J., Vercruyssen, L., Claeys, H., Hradilová, J., Černý, M., Novák, O., et al. (2019). Multifaceted activity of cytokinin in leaf development shapes its size and structure in Arabidopsis. Plant J 97, 805–824. doi: 10.1111/tpj.14285
Szklarczyk, D., Gable, A. L., Lyon, D., Junge, A., Wyder, S., Huerta-Cepas, J., et al. (2019). STRING v11: protein-protein association networks with increased coverage, supporting functional discovery in genome-wide experimental datasets. Nucleic Acids Res. 47, D607–D613. doi: 10.1093/nar/gky1131
Tichá, T., Samakovli, D., Kuchařová, A., Vavrdová, T., and Šamaj, J. (2020). Multifaceted roles of heat shock protein 90 molecular chaperones in plant development. J. Exp. Bot. 71, 3966–3985. doi: 10.1093/jxb/eraa177
Ueda, A., Yamamoto-Yamane, Y., and Takabe, T. (2007). Salt stress enhances proline utilization in the apical region of barley roots. Biochem. Biophys. Res. Commun. 355, 61–66. doi: 10.1016/j.bbrc.2007.01.098
ul Haq, S., Khan, A., Ali, M., Khattak, A. M., Gai, W.-X., Zhang, H.-X., et al. (2019). Heat shock proteins: dynamic biomolecules to counter plant biotic and abiotic stresses. Int. J. Mol. Sci. 20:5321. doi: 10.3390/ijms20215321
Vescovi, M., Riefler, M., Gessuti, M., Novak, O., Schmulling, T., and Lo Schiavo, F. (2012). Programmed cell death induced by high levels of cytokinin in Arabidopsis cultured cells is mediated by the cytokinin receptor CRE1/AHK4. J. Exp. Bot. 63, 2825–2832. doi: 10.1093/jxb/ers008
Vizcaíno, J. A., Csordas, A., Del-Toro, N., Dianes, J. A., Griss, J., Lavidas, I., et al. (2016). 2016 update of the PRIDE database and its related tools. Nucleic Acids Res. 44, D447–D456.
Vojta, P., Kokáš, F., Husičková, A., Grúz, J., Bergougnoux, V., Marchetti, C. F., et al. (2016). Whole transcriptome analysis of transgenic barley with altered cytokinin homeostasis and increased tolerance to drought stress. N. Biotechnol. 33, 676–691. doi: 10.1016/j.nbt.2016.01.010
Voxeur, A., and Höfte, H. (2016). Cell wall integrity signaling in plants: “To grow or not to grow that’s the question”. Glycobiology 26, 950–960. doi: 10.1093/glycob/cww029
Wang, Y., Li, L., Ye, T., Zhao, S., Liu, Z., Feng, Y. Q., et al. (2011). Cytokinin antagonizes ABA suppression to seed germination of Arabidopsis by downregulating ABI5 expression. Plant J. 68, 249–261. doi: 10.1111/j.1365-313X.2011.04683.x
Yang, X. H., Xu, Z. H., and Xue, H. W. (2005). Arabidopsis membrane steroid binding protein 1 is involved in inhibition of cell elongation. Plant Cell 17, 116–131. doi: 10.1105/tpc.104.028381
Zalewski, W., Galuszka, P., Gasparis, S., Orczyk, W., and Nadolska-Orczyk, A. (2010). Silencing of the HvCKX1 gene decreases the cytokinin oxidase/dehydrogenase level in barley and leads to higher plant productivity. J. Exp. Bot. 61, 1839–1851. doi: 10.1093/jxb/erq052
Žd’árská, M., Zatloukalová, P., Benítez, M., Šedo, O., Potěšil, D., Novák, O., et al. (2013). Proteome analysis in Arabidopsis reveals shoot- and root-specific targets of cytokinin action and differential regulation of hormonal homeostasis. Plant Physiol. 161, 918–930.
Zhang, Y., Chen, B., Xu, Z., Shi, Z., Chen, S., Huang, X., et al. (2014). Involvement of reactive oxygen species in endosperm cap weakening and embryo elongation growth during lettuce seed germination. J. Exp. Bot. 65, 3189–3200. doi: 10.1093/jxb/eru167
Zhang, Y., Liu, S., Dai, S. Y., and Yuan, J. S. (2012). Integration of shot-gun proteomics and bioinformatics analysis to explore plant hormone responses. BMC Bioinformatics 13(Suppl. 1):S8. doi: 10.1186/1471-2105-13-S15-S8
Zhong, L., Zhou, W., Wang, H., Ding, S., Lu, Q., Wen, X., et al. (2013). Chloroplast small heat shock protein HSP21 interacts with plastid nucleoid protein pTAC5 and is essential for chloroplast development in arabidopsis under heat stress. Plant Cell 25, 2925–2943. doi: 10.1105/tpc.113.111229
Keywords: Hordeum vulgare, zeatin, proteome, metabolome, abiotic stress, phenylpropanoid biosynthesis, root, ROS
Citation: Berka M, Luklová M, Dufková H, Berková V, Novák J, Saiz-Fernández I, Rashotte AM, Brzobohatý B and Černý M (2020) Barley Root Proteome and Metabolome in Response to Cytokinin and Abiotic Stimuli. Front. Plant Sci. 11:590337. doi: 10.3389/fpls.2020.590337
Received: 01 August 2020; Accepted: 05 October 2020;
Published: 28 October 2020.
Edited by:
Wolfram G. Brenner, Universität Leipzig, GermanyReviewed by:
Branka Salopek Sondi, Rudjer Boskovic Institute, CroatiaCopyright © 2020 Berka, Luklová, Dufková, Berková, Novák, Saiz-Fernández, Rashotte, Brzobohatý and Černý. This is an open-access article distributed under the terms of the Creative Commons Attribution License (CC BY). The use, distribution or reproduction in other forums is permitted, provided the original author(s) and the copyright owner(s) are credited and that the original publication in this journal is cited, in accordance with accepted academic practice. No use, distribution or reproduction is permitted which does not comply with these terms.
*Correspondence: Martin Černý, bWFydGluY2Vybnk4M0BnbWFpbC5jb20=
Disclaimer: All claims expressed in this article are solely those of the authors and do not necessarily represent those of their affiliated organizations, or those of the publisher, the editors and the reviewers. Any product that may be evaluated in this article or claim that may be made by its manufacturer is not guaranteed or endorsed by the publisher.
Research integrity at Frontiers
Learn more about the work of our research integrity team to safeguard the quality of each article we publish.