- State Key laboratory of Tree Genetics and Breeding, Northeast Forestry University, Harbin, China
Larix olgensis or larch is an economically important coniferous tree species with rapid growth in the early stages, strong adaptability, and a short time to harvest. The genetic improvement of larch has garnered considerable attention in recent years for reclaiming timber forests. However, traditional breeding methods are largely ineffective for achieving rapid genetic improvement of L. olgensis. Studies show that the efficiency of plant regeneration can be improved by optimizing somatic embryogenesis. On this basis, we devised a stable, fast and efficient Agrobacterium-mediated genetic transformation method using suspended embryogenic calluses as explants and β-glucuronidase as the reporter. We evaluated the effects of the Agrobacterium load, co-culture period, and addition of acetosyringone and transformant screening antibiotic on the transformation efficiency. In addition, we tested the pCAMBIA 1300-PtHCA 2-1 promoter-GUS binary expression vector, which contains the GUS gene ORF under the control of Populus trichocarpa high cambial activity PtHCA 2-1 promoter, and observed the tissue-specific expression of the GUS gene in the somatic embryos of transgenic larch. This novel technique can not only accelerate the generation of superior transgenic strains of L. olgensis but also aid in future gene functional studies.
Introduction
Larix olgensis is a coniferous tree distributed across northeastern China, Russia, and Korea, and its timber is used in construction and other industries (Hu et al., 2015). It is an important species for reclaiming timber forests due to its rapid growth at the early stages, strong adaptability, and a short harvesting cycle (Keith and Chauret, 2011; Boruszewski et al., 2017). With a globally dwindling forest cover, there has been an increased focus in recent years to develop genetically improved strains of Larix in order to accelerate afforestation. However, as most woody plants, Larix has a long juvenile period, and the offspring produced by sexual reproduction show considerable genetic variation. Therefore, traditional breeding methods cannot achieve rapid genetic improvement of L. olgensis.
On the other hand, transgenesis is a promising approach for accelerating the genetic improvement of forest trees (Vain et al., 2004; Flachowsky et al., 2009; Ren et al., 2017). The regenerative ability of the recipient parts, such as zygotic embryos, hypocotyl, embryogenic callus, and somatic embryos (Lin et al., 2005; Prakash and Gurumurthi, 2009; Belide et al., 2017), is a major determinant of genetic transformation. The embryogenic callus is an ideal material for transgenesis on account of its stable proliferation, high regeneration rate, sensitivity to screening antibiotics, such as kanamycin and hygromycin, and tolerance to Agrobacterium tumefaciens (Belide et al., 2017; Ratjens et al., 2018). Several groups have successfully used embryonic tissues or cellular suspension for genetic transformation of conifers through A. tumefaciens (Levee et al., 1997, 1999; Ismail et al., 2004) or gene gun bombardment (Klimaszewska et al., 1997).
Agrobacterium-mediated transformation has the advantages of technical ease and stable expression of exogenous genes (Takata and Eriksson, 2012). Larix are the natural hosts of Agrobacterium, and Huang et al. (1991) first reported the effective genetic transformation of Larix decidua seedlings using Agrobacterium rhizogenes back in 1991. Subsequently, the transgenic lines of Larix kaempferi × decidua (Levee et al., 1997), L. decidua (Ismail et al., 2004), L. kaempferi × Larix principis (Wang, 2007), and Larix leptolepis (Zhu et al., 2011) were also established by the A. tumefaciens-mediated transformation method (Supplementary Table S1). In addition, gene gun bombardment (Duchesne et al., 1993; Klimaszewska et al., 1997; Qi et al., 2000), pollen tube (Han et al., 2006), and electroporation (Charest et al., 2011) have also been utilized for Larix transgenesis, although the transformation efficiency was unsatisfactory.
According to previous reports (Supplementary Table S1), the efficacy of Agrobacterium-mediated T-DNA transformation of larch depends on the donor and recipient strains, infection time, co-cultivation time, selection pressure, etc. Moreover, the suitable transformation conditions of different larch species (including interspecific hybrids) are distinct. Our preliminary results showed that the expression rate of exogenous gene in embryogenic callus of L. olgensis infected by A. tumefaciens using previously established protocols was either very low (less than 5%) or undetectable. Therefore, it is essential to develop a stable and efficient genetic transformation system for L. olgensis.
In this study, we have described a novel Agrobacterium-based transformation method for L. olgensis using embryogenic calluses as the explants. Furthermore, the somatic embryos of transgenic larch transformed with GUS gene ORF under the control of Populus trichocarpa HCA 2-1 promoter expressed the gene in a tissue-specific manner. This novel transformation system can greatly accelerate the development of genetically improved L. olgensis strains.
Materials and Methods
Callus Induction
The immature seeds of L. olgensis were collected in May 2014 from the Heilongjiang Province. The average size of the embryo proper obtained after peeling the endosperm was 318.04 ± 83.23 μm. The seeds were sterilized using 75% ethanol for 30 s and then 3% sodium hypochlorite for 10 min, cut longitudinally, and then inoculated onto the basic medium (BM; Supplementary Table S2) supplemented with 1.5 mg⋅L–1 2,4-dichlorophenoxyacetic acid (2,4-D), 0.5 mg⋅L–1 6-benzylaminopurine (6-BA), 0.5 mg⋅L–1 kinetin (KT), 1.0 g⋅L–1 glutamine (Gln), 0.5 g⋅L–1 acid hydrolysis casein (CH), 25.0 g⋅L–1 sucrose, and 6.0 g⋅L–1 agar at pH 6 ± 0.02 (Wang et al., 2009; Song et al., 2016). Callus formation was induced at 23 ± 2°C in the dark. After 7 weeks, the embryogenic calluses were inoculated into liquid proliferation medium (PM) consisting of the basic elements of BM (2,4-D 0.15 mg⋅L–1, 6-BA 0.05 mg⋅L–1, KT 0.05 mg⋅L–1, Gln 1.0 g⋅L–1, CH 0.5 g⋅L–1 and sucrose 25.0 g⋅L–1) at the calluses/medium ratio of 1:100 (w/v). The cultures were maintained with constant shaking at 120 rpm and passaged every 14 days.
Callus Synchronization and Maturation of Somatic Embryos
The embryogenic suspension calluses were isolated from liquid medium and transferred to maturation medium (MM) consisting of the basic salts of BM supplemented with 20.0 mg⋅L–1 abscisic acid (ABA), 80.0 g⋅L–1 PEG4,000, 5.0 g⋅L–1 silver nitrate (AgNO3), and 60.0 g⋅L–1 sucrose. Somatic embryo maturation was induced for 7 weeks. Normal cotyledon embryo germination was observed after 7 days of inoculation on L&M woody plant basal medium (WPM; PhytoTech Labs, United States) supplemented with 20.0 g⋅L–1 sucrose, 4.0 g⋅L–1 agar, 3.0 g⋅L–1 vitamin B1 and 2.0 g⋅L–1 activated carbon under a 16 h/day photoperiod (50 μmol⋅m–2⋅s–1). To increase the efficiency of somatic embryogenesis and optimize the synchronization, embryonic calluses were cultured in synchronized culture medium (SM) before the somatic embryo maturation. To determine the optimum culture period, 0.2 g calluses were cultured on medium containing 1/4 BM basic salts with the addition of 60.0 g⋅L–1 sucrose, 10.0 g⋅L–1 inositol, 1.0 g⋅L–1 Gln, 0.5 g⋅L–1 CH, and 6.0 g⋅L–1 agar for 1, 7, 14, 21, or 28 days in the dark. In addition, different concentrations of sucrose (30, 60, and 90 g⋅L–1), inositol (1, 10, and 15 g⋅L–1), Gln (0, 0.5, and 1 g⋅L–1), CH (0, 0.25, and 0.5 g⋅L–1) and total, 1/2, or 1/4 BM salts were also tested for 14 days. The process is outlined in Figure 1A. Each treatment was performed in triplicate, and at least 15 embryogenic calluses of L. olgensis were examined for each experiment.
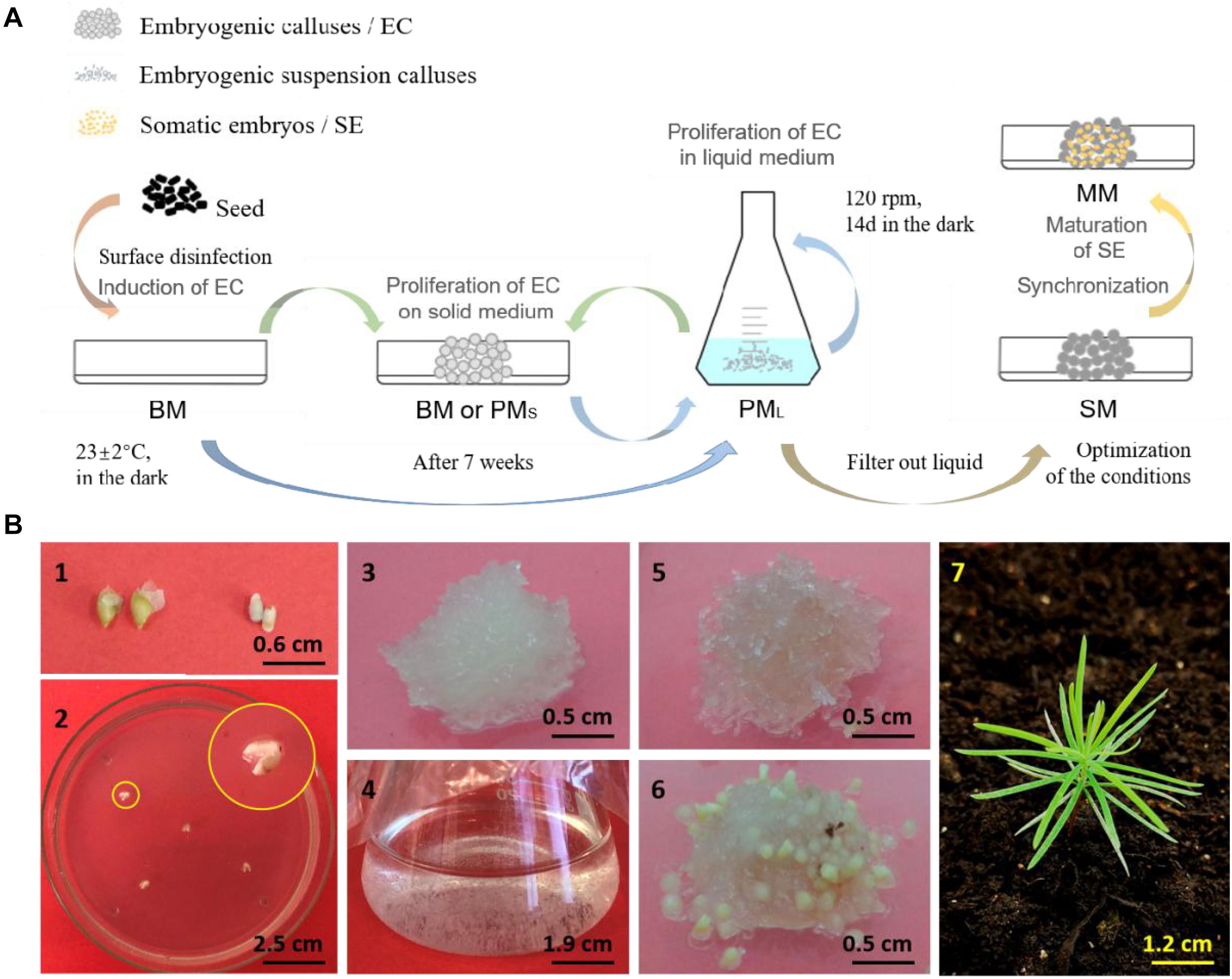
Figure 1. Somatic embryogenesis and regeneration of embryogenic callus of L. olgensis. The schematics (A) of somatic embryogenesis of L. olgensis. Representative images of the (B) immature seeds (1) exfoliated from megasporophyll and (2) inoculated into the basic medium (BM) to induce callus formation, (3) embryogenic callus (EC) cultured on proliferation medium (PMS) for 14 days, (4) EC cultured in liquid proliferation medium (PML) for 7 days, (5) EC cultured on synchronization medium (SM) for 14 days, (6) induction of pro-embryo maturation on maturation medium (MM) for 15 days, and (7) plantlet from somatic embryo transplants.
Antibiotic Sensitivity Test
The embryogenic calluses were filtered out using a 60-mesh cellular sieve (0.3 mm), and the excess amount of water was absorbed on a sterile filter paper. The calluses were weighed, and 0.25 g was inoculated into 25 ml PM supplemented with 0, 100, 200, 300, or 400 mg⋅L–1 cefotaxime (Cef). After culturing for 14 days at 120 rpm and 23 ± 2°C in the dark, the proliferative calluses were separated as above and weighed, and 0.5 g calluses were seeded on solid PM (6 g⋅L–1 agar) supplemented with various concentrations (0, 10, 20, 30, or 40 mg⋅L–1) of kanamycin (Kan) or (0, 2, 4, 6, 8, or 10 mg⋅L–1) hygromycin (Hyg). The plates were incubated at 23 ± 2°C in the dark for 15 days. Each callus was weighed, transferred to SM (with the same respective concentration of Kan or Hyg), and cultured for 14 days and thereafter to antibiotic-supplemented MM. The number of somatic embryos was counted after 8 weeks. The experiment was repeated thrice, and at least 15 embryogenic calluses were examined each time.
Agrobacterium Strain Cultivation and Transformation
Agrobacterium tumefaciens strain GV3101 was transformed with the pBI121, pCAMBIA 1300, and recombinant pCAMBIA-PTHCA 2-1 Promoter-GUS binary vectors (Supplementary Figure S1). The pBI121 contains the CaMV35S-activated β-glucuronidase (GUS) gene, the neomycin phosphotransferase gene (npt II) as selective markers, and Nos terminator. The plasmid pCAMBIA 1301 contains the hygromycin phosphotransferase gene (hpt) and the GUS gene under the control of CaMV35S. The modified p1300-PtHCA 2-1 Promoter-GUS also contains the GUS gene under the control of the P. trichocarpa PtHCA 2-1 promoter. The cryopreserved transformed bacteria were revived, and a single colony was spread on YEB solid medium containing 50 mg⋅L–1 hygromycin and 50 mg⋅L–1 rifampicin. After incubating the plates at 28°C for 2 days, the bacteria were inoculated into 25 ml YEP medium and cultured overnight at 28°C with constant shaking at 200 rpm till the logarithmic phase. The cells were harvested by centrifuging at 8,000 rpm for 15 min at 4°C and resuspended in liquid BM at the appropriate density.
Agrobacterium-Mediated Transformation of Embryogenic Calluses
Freshly proliferated embryogenic calluses were harvested, and ∼5 g callus was inoculated into 20 ml A. tumefaciens suspension of OD600 0.2, 0.4, 0.6, or 0.8 with/out 100 μM acetosyringone (AS). The calluses were infected for 15–20 min with periodic shaking to allow even dispersion, separated, dried on a sterile filter paper, and seeded into the PM. The callus bacteria were co-cultivated at 25°C in the dark for 1, 2, 3, or 4 days in the presence or absence of 100 μM AS.
The cultured calluses were washed sequentially with sterile water and 500 mg⋅L–1 Cef for 5 min each, dried on a sterilized filter paper, and cut into small pieces of diameter 1 ± 0.2 cm. The pieces were placed on PM supplemented with 200 mg⋅L–1 Cef and cultured at 25°C in the dark for 0, 3, 7, 14, or 21 days. The antibiotic-resistant calluses were selected thrice on PM containing 200 mg⋅L–1 Cef and 20 mg⋅L–1 Kan or 4 mg⋅L–1 Hyg at 25°C in the dark for 21 days, and the number of calluses was counted. All treatments were repeated at least three times, and at least 15 calluses were examined from each independent experiment.
Molecular Analysis
Genomic DNA was isolated from the wild-type and transgenic calluses and somatic embryos using a plant DNA extraction kit (Qiagen, China) according to the manufacturer’s instructions. GUS and npt II genes were amplified by PCR using specific primers (Supplementary Table S3) and the Premix Taq DNA Polymerase Kit (Clontech, China) according to the manufacturer’s instructions. The PCR conditions were as follows: initial denaturation at 95°C for 3 min, 30 cycles at 94°C for 30 s, 60°C for 30 s, and 72°C for 45 s and a final extension step at 72°C for 10 min. For gene expression analysis, total RNA was extracted from the calluses and somatic embryos using the RNeasy Plant Mini Kit (Qiagen, China), reverse transcribed using the ReverTra Ace kit (Clontech, China) according to the manufacturer’s instructions, and amplified by reverse transcription (RT)-PCR using the same primers as listed in Supplementary Table S3.
GUS Staining
Glucuronidase staining was performed as described previously (Jefferson et al., 1987). Briefly, hygromycin-resistant callus and somatic embryos were immersed in a solution consisting of 2 mM X-Gluc (5-bromo-4-chloro-3-indolyl-β-D-glucuronide), 5 mM ferro-ferricyanide buffer, 100 mM Na-phosphate buffer (pH 7.0), 10 mM EDTA, and 0.1% Triton X-100 and incubated at 37°C for 7 days. The resistant embryogenic calluses stained blue were counted under a microscope, and the staining intensity was evaluated by extracting the chlorophyll of somatic embryos using 75% (v/v) ethanol.
Statistical Analysis
SPSS 18.0 (Chicago, United States) was used for all data analyses. Data were compared by ANOVA, followed by Fisher’s LSD test, and p < 0.05 was considered statistically significant.
Results
Synchronization of L. olgensis Calluses Improved Embryonic Maturation
After 7 days of synchronized culture, the embryogenic calluses surface changed from translucent to opalescent, and the internal areas were browned 28 days later. Following synchronization, the calluses were exposed to maturation stimuli for approximately 7 days. The pro-embryo masses sprouted short, milky-yellow rod-like protuberances on the surface, which is indicative of close to maturation. After 15 days, some embryos differentiated and formed cotyledons (Figure 1B). In contrast, the unsynchronized calluses began to mature approximately 45 days after the initial ABA stimulus.
The number of somatic embryos increased gradually for a short period during synchronous culture (day 1–14), peaked on the 14th day, and decreased after the 21st day (Figure 2). Therefore, we limited the duration of synchronized culture to 14 days for the subsequent experiments. The yield of mature somatic embryos was also affected by the concentration of salts ion (p = 0.000) and inositol (p = 0.002) in the synchronization medium (Figures 3A,B). Somatic embryogenesis increased by 160.76% when the salts ion concentration decreased to 25%, whereas the addition of 15 g⋅L–1 inositol increased the average embryo yield to 88.77/g. However, the concentration of sucrose, Gln, and CH did not significantly affect the number of somatic embryos, although the combination of 60 g⋅L–1 sucrose, 0.5 g⋅L–1 Gln, and 0.25 g⋅L–1 CH promoted subsequent germination (data not shown). Taken together, these results indicate that synchronization of L. olgensis embryogenic calluses can accelerate somatic embryo maturation.
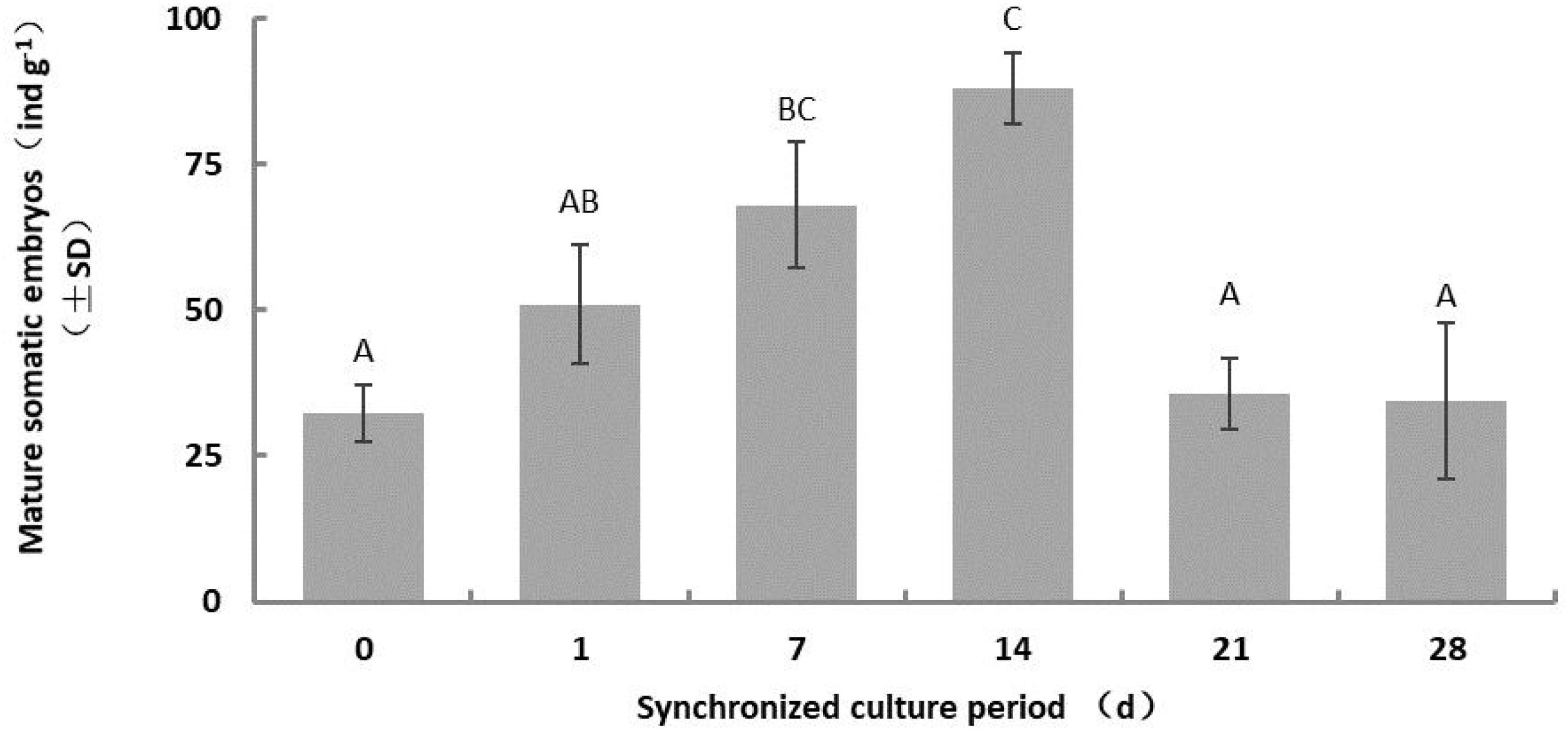
Figure 2. Effect of the synchronous culture period on somatic embryo maturation of L. olgensis. SM contained 1/2 BM salts, 60 g⋅L–1 sucrose, 10 g⋅L–1 inositol, 1 g⋅L–1 glutamine, and 0.5 g⋅L–1 hydrolyzed casein. Each value represents the mean of three independent experiments with standard deviation (SD). Different letters indicate p < 0.05 (Duncan’s multiple range test).
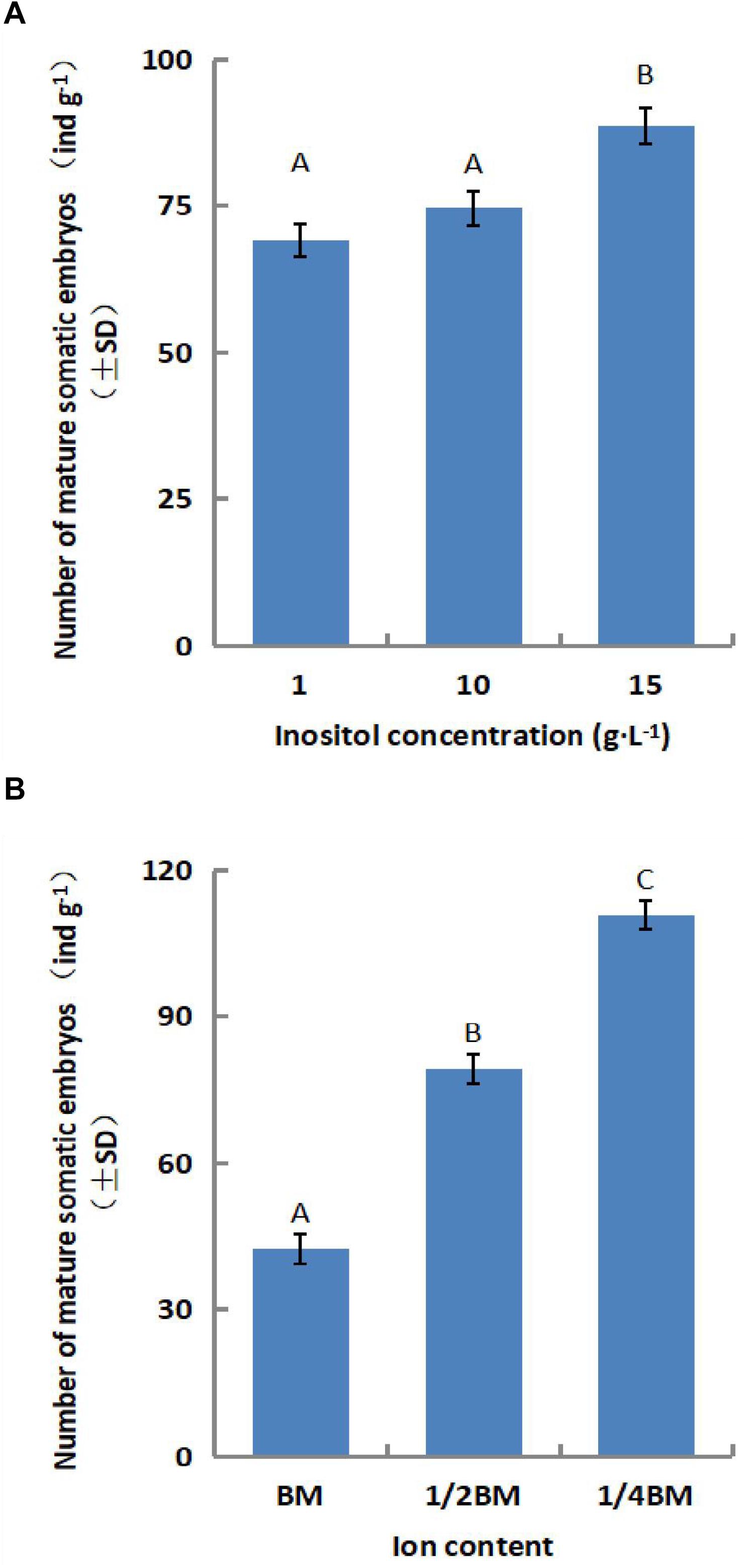
Figure 3. Effect of the SM composition on somatic embryo maturation of L. olgensis. The effects of (A) inositol concentration and (B) ion content on the number of somatic embryos. SM contained 1/4 BM salts, 10 g⋅L–1 inositol, 1 g⋅L–1 glutamine, 0.5 g⋅L–1 hydrolyzed casein, and 60 g⋅L–1 sucrose. Each value represents the mean of three independent experiments with standard deviation (SD). Different letters indicate p < 0.05 (Duncan’s multiple range test).
Effects of Antibiotics on Callus Proliferation and Somatic Embryogenesis
Cefotaxime inhibited the proliferation of L. olgensis embryogenic calluses at concentrations exceeding 200 mg⋅L–1 (Supplementary Figure S2A), whereas kanamycin exerted a significant inhibitory effect at 10 mg⋅L–1 and altogether stalled callus proliferation at 20 mg⋅L–1 (Supplementary Figure S2B). In addition, 10 mg⋅L–1 kanamycin significantly inhibited the maturation of somatic embryos. The L. olgensis calluses were highly sensitive to hygromycin. Compared with the control, 2 mg⋅L–1 Hyg decreased the multiplication of embryogenic calluses by 65.66% (Supplementary Figure S2C) and completely inhibited embryo maturation, whereas 4 mg⋅L–1 of the antibiotic almost stalled embryogenic callus proliferation. Variance analysis showed that Cef (p = 0.000), Kan (p = 0.000), and Hyg (p = 0.000) significantly influenced the growth of embryonic callus, and Kan (p = 0.000) and Hyg (p = 0.000) affected somatic embryogenesis.
Agrobacterium Load and Co-Culture Duration Affected Transformation Efficiency
The transformation efficiency of embryogenic calluses was the highest at 92.5 ± 22.13% when infected with Agrobacterium suspension of OD600 0.6 and decreased slightly with a lower bacterial load (OD600 0.4). In contrast, low (OD600 0.2) and high (OD600 0.8) density bacterial suspensions decreased the transformation efficiency. Previous reports show that T-DNA transfer and integration from A. tumefaciens to the recipient plant cells require at least 16 h. Consistent with this, the transformation efficiency of the embryogenic calluses was the highest after 3 days of bacterial co-culture, and Kan-resistant calluses were not formed when the bacteria were removed. Extensive bacterial overgrowth was observed with highly dense Agrobacterium suspension (OD600 > 0.8) and co-culture duration longer than 4 days. The multiple sterilization steps required to remove the bacteria led to considerable loss of the transformed embryogenic calluses. Therefore, to ensure maximum transformation efficiency and transformant recovery, we infected the calluses with Agrobacterium suspension of OD600 0.6 for 15–20 min and co-cultured them for 3 days (Figure 4A). Directional transfer of T-DNA from A. tumefaciens to target cells requires the expression of the Vir genes (Turk et al., 1993; Wang et al., 2002), which can be activated by some carbohydrates and phenols (Song et al., 1991). The average transformation efficiency of embryonic calluses reached 56.11 ± 16.95% when 100 μM AS was present in both the infection solution and co-culture medium, whereas the optimizing effect was not observed when only either of the two were supplemented with AS. In addition, the efficiency of transformation also increased when the bacterial suspension was chilled at 4°C for 1 h before infection (Figure 4B).
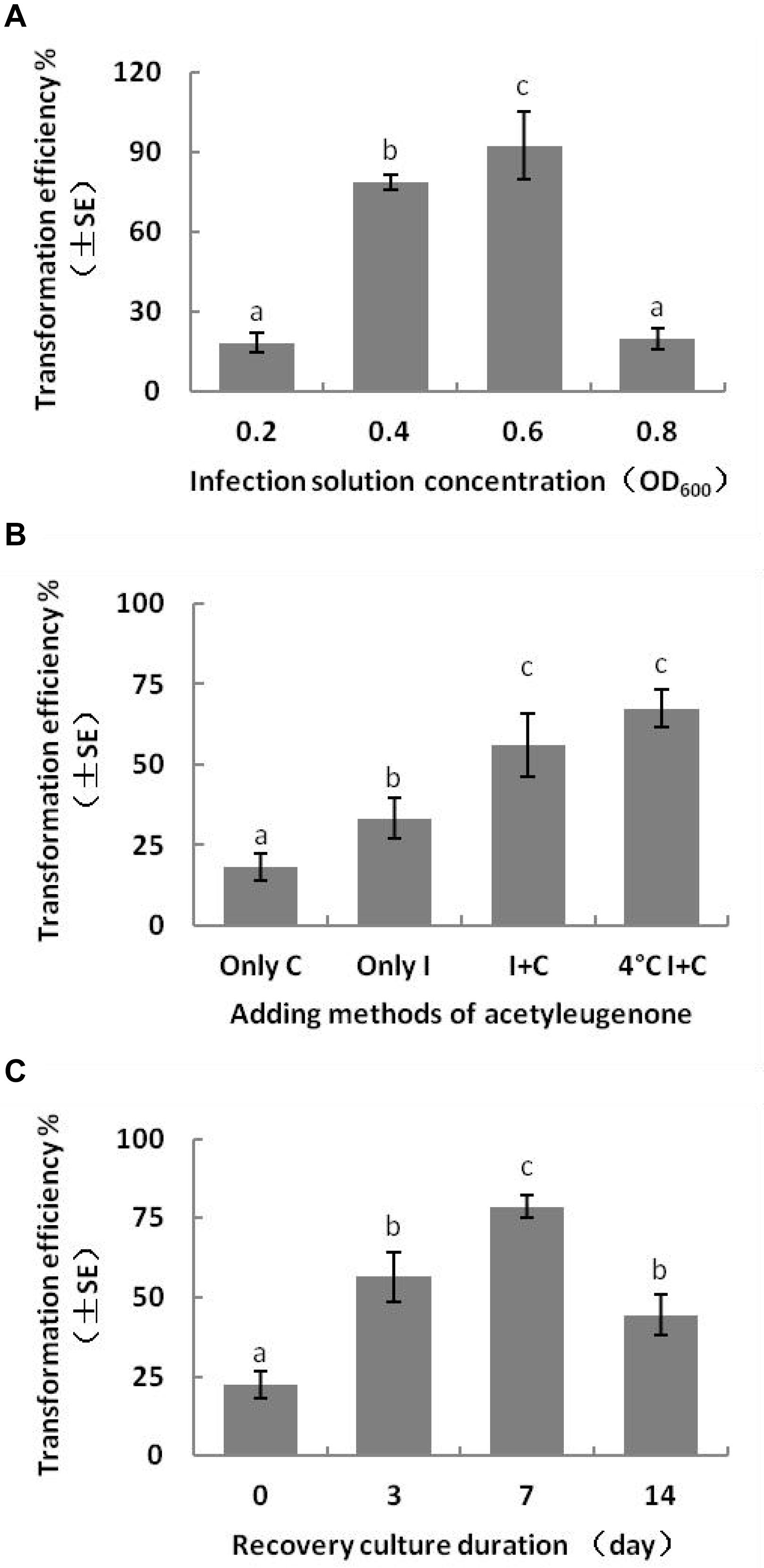
Figure 4. Effect of genetic transformation conditions on transformation efficiency of L. olgensis. Effects of (A) Agrobacterium load, (B) presence of acetosyringone in different stages, and (C) duration of the recovery period after disinfection of callus with cefotaxime. Acetosyringone (100 μM) was added in the bacterial suspension and/or co-culture medium or pre-chilled for 1 h. The resistant calluses were selected on the screening medium containing 4 mg⋅L–1 hygromycin. Each value represents the mean of three independent experiments with the standard error (SE). Different letters indicate p < 0.05 (Duncan’s multiple range test).
Effect of Degerming and Screening Methods on Transformation Efficiency
In order to ensure normal growth of the Agrobacterium-transformed plant tissues, the latter are treated with bacteriostatic antibiotics after co-culture to check further bacterial growth (Kumlehn et al., 2006). The bacterial growth on calluses was completely inhibited after washing the latter for 10 min with 100 mg⋅L–1 Cef (Supplementary Figure S3) or for 5 min with 200 mg⋅L–1 Cef. Furthermore, two successive washes with 200 mg⋅L–1 Cef prevented any secondary contamination of A. tumefaciens. However, the transformation efficiency was adversely affected when the tissues were inoculated in the screening medium right after bacteriostatic antibiotic treatment, and the average number of resistant tissues formed per slice (approximately 1.4 ± 0.1 cm2) was only 0.23 ± 0.08 (Figure 4C). A recovery period of 3 or 7 days post-disinfection in PM lacking the screening antibiotics increased the number of resistant calluses to 0.56 ± 0.13 and 0.79 ± 0.60 per slice, respectively. In contrast, a longer recovery period of 14 days decreased the transformation efficiency to 44.44 ± 11.11% (Table 1) due to excessive reproduction of the pseudo-positive tissues that competed for nutrients with the transformed tissues. Furthermore, the false positive rate of the resistant tissues was nearly 100% after 21 days. The embryogenic calluses of L. olgensis were more sensitive to Hyg than to Kan, indicating a possible effect of antibiotic screening on transformation efficiency. Indeed, the false positive rate of the resistant tissues was ∼86.71% when screened with 20 mg⋅L–1 Kan compared with only 11.66% with 4 mg⋅L–1 Hyg (Table 2). Therefore, Hyg was more beneficial for screening the genetically transformed calluses of L. olgensis. The transgenic calluses were further verified by analyzing the expression of GUS and npt II genes. The negative and positive controls were the uninfected tissues and suspension of Agrobacterium GV3101 transformed with binary vector, respectively. As shown in Figures 5A,B, 6A–F, GUS was amplified in the transgenic and not in the wild-type calluses. In addition, npt II was not detected in any of the transformed tissues, indicating the absence of Agrobacterium contamination.
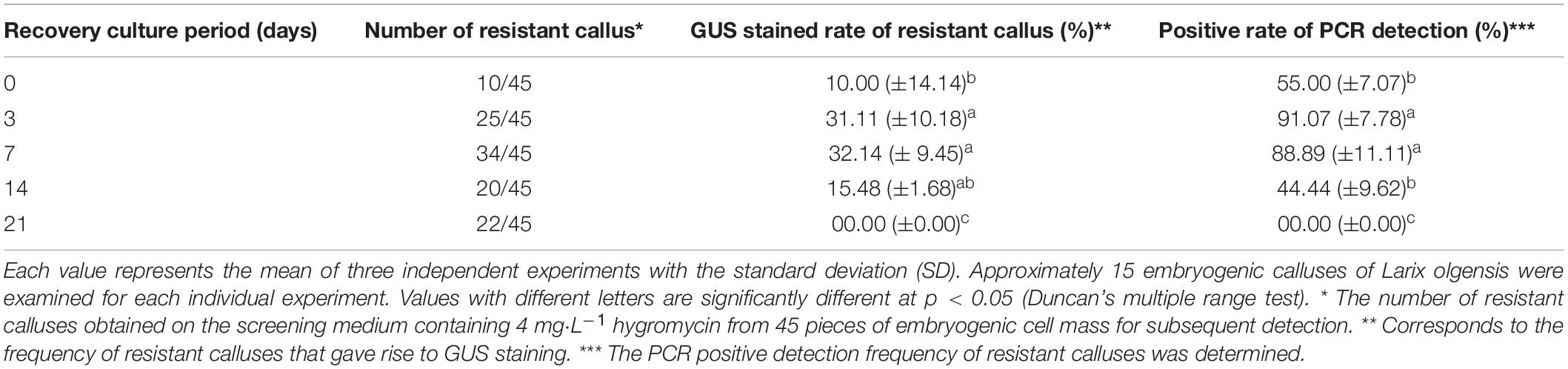
Table 1. Effects of the duration of recovery post-bacteriostasis on the transformation efficiency of L. olgensis.
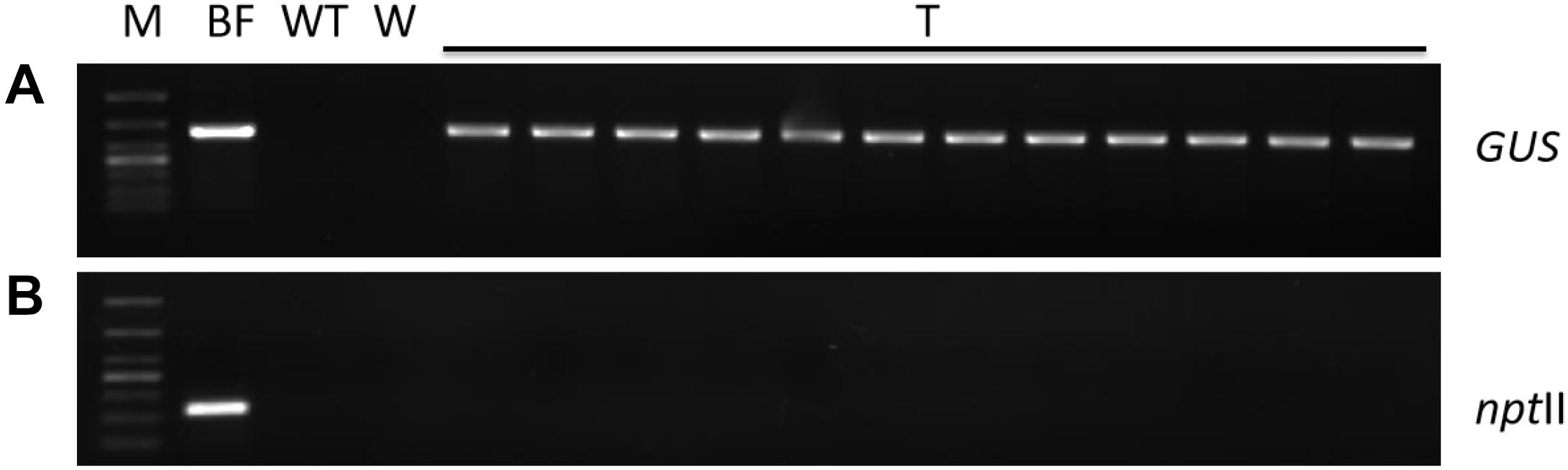
Figure 5. Molecular detection of transgenic embryogenic callus of L. olgensis. PCR amplification of (A) GUS and (B) npt II gene in A. tumefaciens GV3101 containing pCAMBIA1301, untransformed wild-type (WT), and hygromycin-resistant callus (T). Lanes from left to right: 1 kb plus DNA ladder, bacterial fluid, WT callus DNA, water control, and resistant callus DNA (T1–12).
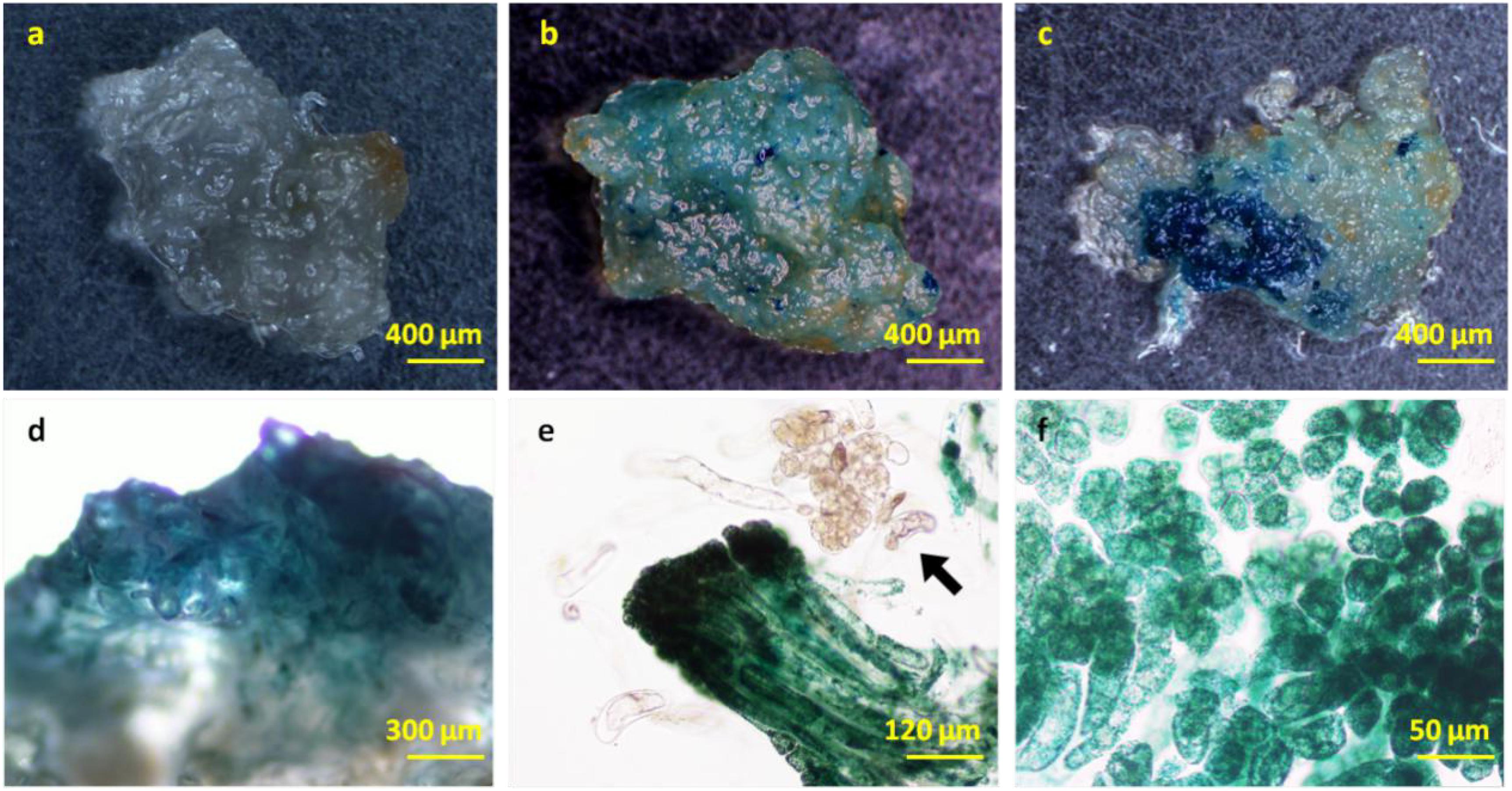
Figure 6. GUS staining in the transgenic L. olgensis embryogenic callus. GUS staining of (a) WT, (b) kanamycin-, and (c) hygromycin-resistant calluses infected with A. tumefaciens GV3101 containing plasmid pBI121 and pCAMBIA1301, respectively. Expression pattern of 35S promoter-driven GUS in embryogenic callus (d), whole embryogenic suspended mass (e), and embryogenic cells (f). The arrow indicates a chimeric cell mass lacking exogenous GUS gene activity.
Transformed Buds Expressed GUS in a Tissue-Specific Manner Under the Control of PtHCA 2-1 Promoter
To further validate the applicability of somatic embryogenesis, we expressed the GUS gene under the control of the P. trichocarpa PtHCA 2-1 promoter in embryogenic calluses and somatic embryo seedlings. The detailed flowchart of transformation is shown in Supplementary Figure S4. HCA2 (high cambial activity 2) regulates interfascicular cambium formation and vascular tissue development in Arabidopsis (Pineau et al., 2005). It is constitutively expressed in the vascular tissues from the seedling stage to the mature plant (Pineau et al., 2005; Guo et al., 2009). Premature and many cambial cell divisions in both the fascicular and interfascicular regions typically result in the loss of the alternate vascular bundle/fiber organization. Arabidopsis strains expressing mutant HCA show increased vascular tissue development, stunting, and a delay in flowering and senescence (Guo et al., 2009).
In our study, the transgenic calluses, somatic embryos, and seedling terminal buds stained an intense blue color with X-Gluc, indicating GUS expression (Figure 7A–E). Interestingly, the resistant callus expressed GUS in all cells, including the embryos and suspensor without any visible chimera. However, the seedling roots arising from the germination of these resistant tissues did not express GUS, indicating that the PtHCA 2-1 promoter ensures tissue-specific transcription of exogenous genes in L. olgensis. Furthermore, the transgenic and wild-type calluses yielded similar number of somatic embryos (Supplementary Table S4), indicating that genetic transformation and expression of exogenous genes do not affect the maturation and germination of somatic embryos. The transgenic seedlings were transplanted into soil mixed with vermiculite (3:1), and their survival rate after 4 weeks was higher than 95% (Supplementary Figure S5).
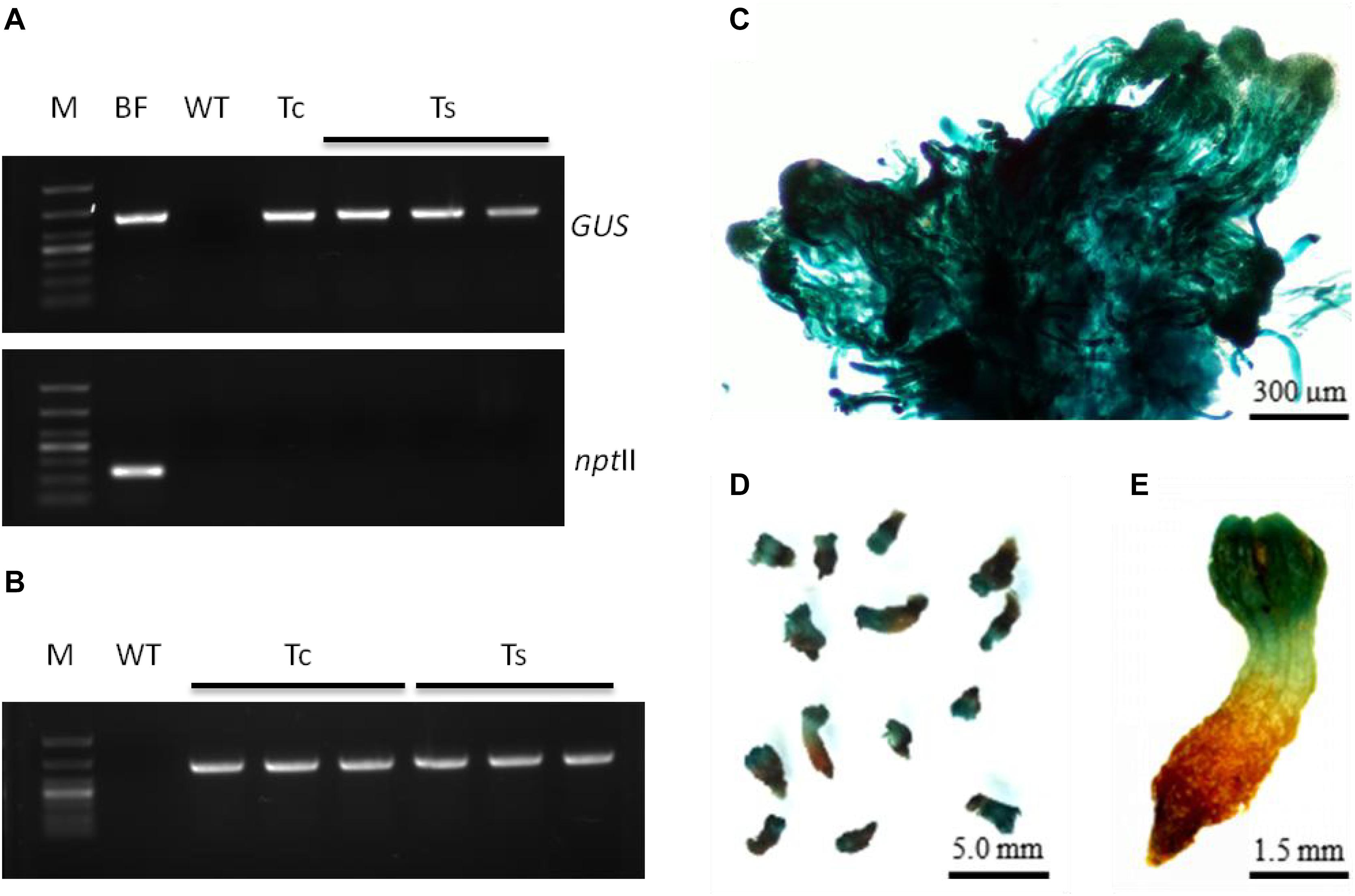
Figure 7. Molecular detection and expression analysis of transgenic embryogenic cultures of L. olgensis with GUS gene. (A) PCR amplification of GUS and npt II genes in Agrobacterium GV3101 containing p1300-PtHCA 2-1pro-GUS, untransformed wild-type (WT), and transgenic callus (Tc) or somatic embryos (Ts). Lane M is 1 kb ladder. (B) RT-PCR amplification of GUS gene in WT and transgenic embryogenic cultures (Tc or Ts). PtHCA 2-1pro-GUS transformed callus (C) or somatic embryos (D,E) after staining with X-Gluc.
Discussion
Plant somatic embryogenesis is a highly complex process (Sharifi et al., 2012; Smertenko and Bozhkov, 2014) and requires highly calibrated conditions for ex vivo culture. Embryonic development of pine cones can be divided into the pro-embryo, early embryogenesis, and late embryogenesis stages (Smertenko and Bozhkov, 2014). Morphologically, the pro-embryo mass period can be further divided into stages I, II, and III (Filonova et al., 2000b), of which only stage III can further develop into mature somatic embryos. Cytokinins, such as 6-BA and KT, and auxins, such as 2,4-D and naphthaleneacetic acid (NAA), added to promote plant tissue growth in vitro can skew cell division and polarity of the pro-embryos, resulting in their disintegration before stage III (Nishl et al., 1997; Filonova et al., 2000a). Therefore, we synchronized the embryogenic calluses of L. olgensis by removing growth regulators from the medium at an early stage, which significantly accelerated somatic embryo maturation. Interestingly, the number of somatic embryos was affected by the ion and inositol content in the synchronized culture medium, and this relatively novel molecular mechanism needs further study. In addition, although glutamine or hydrolyzed casein alone had no significant effect on the embryo yield, their combined effect on somatic embryogenesis was significant. The major factors affecting Agrobacterium-mediated transformation of conifers are the physiological status of the plant recipient, the bacterial strain and plasmid vectors, and the infection and screening conditions (Wu et al., 2015; Kim et al., 2016; Aggarwal et al., 2018; Li et al., 2018). Furthermore, Agrobacterium load and the infection time are also important determinants for successful transformation (Li et al., 2017, 2018). The optimum transformation efficiency in this study was obtained with a moderate bacterial load and co-culturing for 2–3 days, which is similar to previous findings (Wang, 2007; Zhu et al., 2011). A sufficient co-culture duration of 2–3 days is necessary for ensuring complete transfer of the T-DNA from A. tumefaciens to plant cells. Excessive Agrobacterium load and/or longer infection time can considerably lower plant viability and reduce the transformation efficiency. In addition, phenolic substances secreted by damaged plant cells attract A. tumefaciens via chemotaxis and promote the transfer and integration of T-DNA by inducing the Vir genes. Previous studies have shown that acetosyringone can improve the transformation efficiency of conifers (Song et al., 1991; Zhu et al., 2011). Although the addition of inducers does not necessarily increase the efficiency of Agrobacterium-mediated transformation, and some may even have a negative effect, we found that acetosyringone increased the transformation rate of L. olgensis.
Optimal selection pressure, usually an antibiotic, is another determinant of the efficacy of genetic transformation of conifers (Wu et al., 2015; Li et al., 2017); therefore, it is essential to determine the antibiotic sensitivity of the recipient in order to improve transformation efficiency, allow normal growth of the transformants, and reduce the false positive rate. NptII (neomycin phosphotransferase gene), hpt (hygromycin phosphotransferase gene), and bar (glyphosate acetate transferase gene) are commonly used as selection markers for conifer genetic transformation (Duchesne et al., 1993; Wenck et al., 1999; Tang et al., 2001). We found that the embryogenic callus of L. olgensis was highly sensitive to all antibiotics, and 4 mg⋅L–1 Hyg was selected for screening transformants due to the lower false positive rate.
Taken together, Agrobacterium-mediated transformation of synchronized embryonic calluses can rapidly produce a relatively large number of transgenic somatic embryos or plants and can also be scaled up. Compared with the previous studies on larches (Supplementary Table S1), L. olgensis was genetically transformed at significantly higher efficiency under appropriate conditions. This approach not only can accelerate the generation of genetically superior L. olgensis varieties but can also potentially aid protein subcellular localization studies and other applications requiring transgene expression. Our future efforts will focus on further enhancing the transformation efficiency of L. olgensis and conducting functional gene studies.
Conclusion
We improved the protocol of larch somatic embryogenesis and developed a rapid and simple transformation method for L. olgensis embryogenic calluses using A. tumefaciens strain GV3101 expressing pCAMBIA 1300, bacterial density OD600 of 0.6, 3 days of co-culture, 100 μM acetosyringone as inducer, and transformant screening with 4 mg⋅L–1 Hyg. Under these optimum conditions, the transformation efficiency was almost 90%, which is higher compared with that reported for other conifers and larch species. This novel method can potentially accelerate genetic improvement of L. olgensis.
Data Availability Statement
The original contributions presented in the study are included in the article/Supplementary Material, further inquiries can be directed to the corresponding author/s.
Author Contributions
SL and HZ conceived the study. YS and SL designed the study. YS, XB, and NW performed the experiments. YS, SD, YY, and HD contributed to data interpretation. YS and SL wrote the manuscript. All authors read and approved the final manuscript.
Conflict of Interest
The authors declare that the research was conducted in the absence of any commercial or financial relationships that could be construed as a potential conflict of interest.
Acknowledgments
This work was supported by the National Science and Technology Major Project (2018ZX08020003-001-001), and the Natural Science Foundation of Heilongjiang Province, China (C2017008).
Supplementary Material
The Supplementary Material for this article can be found online at: https://www.frontiersin.org/articles/10.3389/fpls.2020.584492/full#supplementary-material
Supplementary Figure 1 | Schematic representation of binary expression vectors used in this study.
Supplementary Figure 2 | Effect of antibiotics on the proliferation of embryogenic callus of L. olgensis.
Supplementary Figure 3 | Comparison of the bacteriostatic effects of different concentrations of cefotaxime.
Supplementary Figure 4 | Schematic representation of the timeline for A. tumefaciens-mediated transformation of L. olgensis embryogenic callus.
Supplementary Figure 5 | The seedling transplanting of transgenic L. olgensis.
Supplementary Table 1 | Efficiency and conditions of Agrobacterium-mediated genetic transformation of Larix.
Supplementary Table 2 | Composition of BM (basic medium).
Supplementary Table 3 | List of primers used for PCR.
Supplementary Table 4 | Comparison of the number of mature somatic embryos and somatic embryo germination rate of transgenic and non-transgenic embryogenic callus of L. olgensis.
References
Aggarwal, P. R., Nag, P., Choudhary, P., Chakraborty, N., and Chakraborty, S. (2018). Genotype-independent Agrobacterium rhizogenes-mediated root transformation of Chickpea: a rapid and efficient method for reverse genetics studies. Plant Methods 14:55. doi: 10.1186/s13007-018-0315-6
Belide, S., Vanhercke, T., Petrie, J. R., and Singh, S. P. (2017). Robust genetic transformation of Sorghum (Sorghum bicolor L.) using differentiating embryogenic callus induced from immature embryos. Plant Methods 13:109. doi: 10.1186/s13007-017-0260-9
Boruszewski, P., Jankowska, A., and Kurowska, A. (2017). Comparison of the structure of juvenile and mature wood of Larix decidua mill. from fast-growing plantations in Poland. Bioresources 12, 1813–1825. doi: 10.15376/biores.12.1.1813-1825
Charest, P. J., Yvonne, D., Christine, W., Catherine, J., Ulriche, S., and Klimaszewska, K. (2011). Transient expession of foreign chimeric genes in the gymnosperm hybrid larch following electroporation. Can. J. Bot. 69, 1731–1736. doi: 10.1139/b91-220
Duchesne, L., Lelu, M. A., Aderkas, V. P., and Charest, P. J. (1993). Microprojection-mediated DNA delivery in embryogenic cells of Larix spp. Can. J. Forest Res. 23, 312–316. doi: 10.1139/x93-042
Filonova, L. H., Bozhkov, P. V., and Arnold, S. V. (2000a). Developmental pathway of somatic embryogenesis in Picea abies as revealed by time-lapse tracking. J. Exp. Bot. 51, 249–264. doi: 10.1093/jexbot/51.343.249
Filonova, L. H., Bozhkov, P. V., Brukhin, V. B., Daniel, G., Zhivotovsky, B., and Von, A. S. (2000b). Two waves of programmed cell death occur during formation and development of somatic embryos in the gymnosperm, Norway spruce. J. Cell Sci. 113(Pt 24), 4399–4411. doi: 10.1007/BF02703793
Flachowsky, H., Haaanke, M. V., Peil, A., Strauss, S. H., and Fladung, M. (2009). A review on transgenic approaches to accelerate breeding of woody plants. Plant Breed. 128, 217–226. doi: 10.1111/j.1439-0523.2008.01591.x
Guo, Y., Qin, G., Gu, H., and Qua, L. J. (2009). Dof5.6/HCA2, a Dof transcription factor gene, regulates interfascicular cambium formation and vascular tissue development in Arabidopsis. Plant Cell 21, 3518–3534. doi: 10.1105/tpc.108.064139
Han, S., Wang, Q., Zhou, C., Liu, G., and Qi, L. (2006). Transformation of hybrid larch (Larix leptolepis × L. olgensis) with P5CS gene cloned from Populus simonii cDNA library. Biotechnol. Bull. 32, 88–92.
Hu, X., Yang, J., and Li, C. (2015). Transcriptomic response to nitric oxide treatment in Larix olgensis Henry. Int. J. Mol. Sci. 16, 28582–28597. doi: 10.3390/ijms1612 26117
Huang, Y., Diner, A. M., and Karnosky, D. F. (1991). Agrobacterium rhizogenes-mediated genetic transformation and regeneration of a conifer: Larix decidua. In Vitro Cell. Dev. Biol. Plant 27, 201–207. doi: 10.1007/BF02632217
Ismail, G., Schnabl, H., Zoglauer, K., and Boehm, R. (2004). Agrobacterium-mediated transformation of Larix decidua: an assessment of factors influencing the efficiency of gus gene transfer. J. Appl. Bot. Food Qual. 78, 83–90.
Jefferson, R. A., Bevan, M., and Kavanagh, T. (1987). The use of the Escherichia coli beta-glucuronidase as a gene fusion marker for studies of gene expression in higher plants. Biochem. Soc. Trans. 15, 17–18. doi: 10.1042/bst0150017
Keith, C. T., and Chauret, G. (2011). Basic wood properties of European larch from fast-growth plantations in Eastern Canada. Can. J. Forest Res. 18, 1325–1331. doi: 10.1139/x88-204
Kim, M. J., An, D. J., Moon, K. B., Cho, H. S., Min, S. R., Sohn, J. H., et al. (2016). Highly efficient plant regeneration and Agrobacterium-mediated transformation of Helianthus tuberosus L. Ind. Crops Prod. 83, 670–679. doi: 10.1016/j.indcrop.2015.12.054
Klimaszewska, K., Devantier, Y., Lachance, D., Lelu, M. A., and Charest, P. J. (1997). Larix laricina (tamarack): somatic embryogenesis and genetic transformation. Can. J. Forest Res. 27, 538–550. doi: 10.1139/x96-208
Kumlehn, J., Serazetdinova, L., Hensel, G., Becker, D., and Loerz, H. (2006). Genetic transformation of barley (Hordeum vulgare L.) via infection of androgenetic pollen cultures with Agrobacterium tumefaciens. Plant Biotechnol. J. 4, 251–261. doi: 10.1111/j.1467-7652.2005.00178.x
Levee, V., Garin, E., Klimaszewska, K., and Seguin, A. (1999). Stable genetic transformation of White Pine (Pinus strobus L.) after cocultivation of embryogenic tissues with Agrobacterium tumefaciens. Mol. Breed. 5, 429–440. doi: 10.1023/A:1009683605841
Levee, V., Lelu, M. A., Jouanin, L., Cornu, D., and Pilate, G. (1997). Agrobacterium tumefaciens-mediated transformation of Hybrid Larch (Larix kaempferi × L.decidua) and transgenic plant regeneration. Plant Cell Rep. 16, 680–685. doi: 10.1007/s002990050301
Li, H. P., Li, K., Guo, Y. T., Guo, J. G., Miao, K. T., Botella, J. R., et al. (2018). A transient transformation system for gene characterization in upland cotton (Gossypium hirsutum). Plant Methods 14:50. doi: 10.1186/s13007-018-0319-2
Li, S., Zhen, C., Xu, W., Wang, C., and Cheng, Y. (2017). Simple, rapid and efficient transformation of genotype Nisqually-1: a basic tool for the first sequenced model tree. Sci. Rep. 7:2638. doi: 10.1038/s41598-017-02651-x
Lin, X. F., Zhang, W. B., Takechi, K., Takio, S., Ono, K., and Takano, H. (2005). Stable genetic transformation of Larix gmelinii L. by particle bombardment of zygotic embryos. Plant Cell Rep. 24, 418–425. doi: 10.1007/s00299-005-0955-7
Nishl, A., Kato, K., Takahashi, M., and Yoshida, R. (1997). Partial synchronization of carrot cell culture by auxin deprivation. Physiol. Plant 39, 9–12. doi: 10.1111/j.1399-3054.1977.tb09277.x
Pineau, C., Freydier, A., Ranocha, P., Jauneau, A., Turner, S., Lemonnier, G., et al. (2005). Hca: an Arabidopsis mutant exhibiting unusual cambial activity and altered vascular patterning. Plant J. 44, 271–289. doi: 10.1111/j.1365-313X.2005.02526.x
Prakash, M. G., and Gurumurthi, K. (2009). Genetic transformation and regeneration of transgenic plants from precultured cotyledon and hypocotyl explants of Eucalyptus tereticornis Sm. Using Agrobacterium tumefaciens. In Vitro Cell. Dev. Biol. Plant 45, 429–434. doi: 10.1007/s11627-008-9179-1
Qi, L. W., Han, Y. F., Li, L., Ewald, D., and Han, S. Y. (2000). The somatic embryogenesis and establishment of transformation experiment system in Larix principis-Rupprechtii. Acta Biol. Exp. Sin. 33, 357–365.
Ratjens, S., Mortensen, S., Kumpf, A., Bartsch, M., and Winkelmann, T. (2018). Embryogenic callus as target for efficient transformation of cyclamen persicum enabling gene function studies. Front. Plant Sci. 9:1035. doi: 10.3389/fpls.2018.01035
Ren, Y. C., Zhang, J., Liang, H. Y., Wang, J. M., and Yang, M. S. (2017). Inheritance and expression stability of exogenous genes in insect-resistant transgenic poplar. Plant Cell Tissue Organ Cult. 130, 567–576. doi: 10.1007/s11240-017-1247-y
Sharifi, G., Ebrahimzadeh, H., Ghareyazie, B., Gharechah, J., and Vatankhah, E. (2012). Identification of differentially accumulated proteins associated with embryogenic and non-embryogenic calli in Saffron (Crocus sativus L.). Proteome Sci. 10:3. doi: 10.1186/1477-5956-10-3
Smertenko, A., and Bozhkov, P. V. (2014). Somatic embryogenesis: life and death processes during apical-basal patterning. J. Exp. Bot. 65, 1343–1360. doi: 10.1093/jxb/eru005
Song, Y., Zhang, H., Li, S., and Li, S. (2016). Relationship between the induction of embryogenic callus of larch and the morphology of immature embryos. J. North-East Forest. Univ. 44, 25–30.
Song, Y. N., Shibuya, M., Ebizuka, Y., and Sankawa, U. (1991). Synergistic action of phenolic signal compounds and carbohydrates in the induction of virulence gene expression of Agrobacterium tumefaciens. Chem. Pharmaceut. Bull. 39, 2613–2616. doi: 10.1248/cpb.39.2613
Takata, N., and Eriksson, M. E. (2012). A simple and efficient transient transformation for hybrid Aspen (Populus tremula × P. tremuloides). Plant Methods 8:30. doi: 10.1186/1746-4811-8-30
Tang, W., Sederoff, R., and Whetten, R. (2001). Regeneration of transgenic Loblolly pine (Pinus taeda L.) from Zygotic Embryos Transformed with Agrobacterium tumefaciens. Planta 213, 981–989. doi: 10.1007/s004250100566
Turk, S. C., Lange, R. P., Sonneveld, E., and Hooykaas, P. J. (1993). The chimeric VirA-tar receptor protein is locked into a highly responsive state. J. Bacteriol. 175, 5706–5709. doi: 10.1128/JB.175.17.5706-5709.1993
Vain, P., Harvey, A., Worland, B., Ross, S., Snape, J. W., and Lonsdale, D. (2004). The effect of additional virulence genes on transformation efficiency, transgene integration and expression in rice plants using the pGreen/pSoup dual binary vector system. Transgenic Res. 13, 593–603. doi: 10.1007/s11248-004-2808-5
Wang, J. (2007). Studies on the Transformation of Larix Based the System of Somatic Embryogenesis. Dissertation for the Degree. Beijing: Chinese Academy of Forestry.
Wang, W., Li, C., Yang, J., Zhang, H., and Zhang, S. (2009). Somatic embryogenesis and plantlet regeneration from immature zygotic embryos of Hybrid Larch. Sci. Silvae Sin. 45, 34–38.
Wang, Y., Gao, R., and Lynn, D. G. (2002). Ratcheting Up vir gene expression in Agrobacterium tumefaciens: coiled coils in histidine kinase signal transduction. Chembiochem 3, 311–317. doi: 10.1002/1439-7633
Wenck, A. R., Quinn, M., Whetten, R. W., Pullman, G., and Sederoff, R. (1999). High-efficiency Agrobacterium-mediated transformation of Norway spruce (Picea abies) and Loblolly Pine (Pinus taeda). Plant Mol. Biol. 39, 407–416. doi: 10.1023/A:1006126609534
Wu, J., Liu, C., Seng, S. S., Khan, M. A., Sui, J. J., Gong, B. H., et al. (2015). Somatic Embryogenesis and Agrobacterium-mediated transformation of Gladiolus hybridus cv. ‘Advance Red’. Plant Cell Tissue Organ Cult. 120, 717–728. doi: 10.1007/s11240-014-0639-5
Keywords: conifers, Larix, Larix olgensis, somatic embryogenesis, synchronized culture, transgenic, GUS
Citation: Song Y, Bai X, Dong S, Yang Y, Dong H, Wang N, Zhang H and Li S (2020) Stable and Efficient Agrobacterium-Mediated Genetic Transformation of Larch Using Embryogenic Callus. Front. Plant Sci. 11:584492. doi: 10.3389/fpls.2020.584492
Received: 17 July 2020; Accepted: 16 October 2020;
Published: 25 November 2020.
Edited by:
Joachim Hermann Schiemann, Julius Kühn-Institut, GermanyReviewed by:
Pandiyan Muthuramalingam, Alagappa University, IndiaFernando Pliego-Alfaro, University of Malaga, Spain
Copyright © 2020 Song, Bai, Dong, Yang, Dong, Wang, Zhang and Li. This is an open-access article distributed under the terms of the Creative Commons Attribution License (CC BY). The use, distribution or reproduction in other forums is permitted, provided the original author(s) and the copyright owner(s) are credited and that the original publication in this journal is cited, in accordance with accepted academic practice. No use, distribution or reproduction is permitted which does not comply with these terms.
*Correspondence: Hanguo Zhang, aGFuZ3VvemhhbmcxQHNpbmEuY29t; Shujuan Li, bGlzaHVqdWFuQG5lZnUuZWR1LmNu