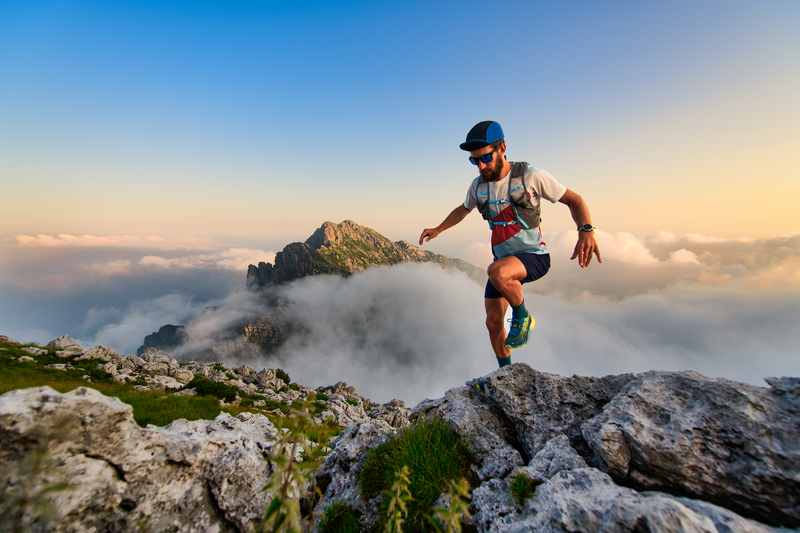
95% of researchers rate our articles as excellent or good
Learn more about the work of our research integrity team to safeguard the quality of each article we publish.
Find out more
REVIEW article
Front. Plant Sci. , 21 December 2020
Sec. Technical Advances in Plant Science
Volume 11 - 2020 | https://doi.org/10.3389/fpls.2020.583590
This article is part of the Research Topic Chlorophyll Fluorescence Imaging Analysis in Biotic and Abiotic Stress View all 9 articles
Imaging of chlorophyll a fluorescence (CFI) represents an easy, precise, fast and non-invasive technique that can be successfully used for discriminating plant response to phytotoxic stress with reproducible results and without damaging the plants. The spatio-temporal analyses of the fluorescence images can give information about damage evolution, secondary effects and plant defense response. In the last years, some studies about plant natural compounds-induced phytotoxicity have introduced imaging techniques to measure fluorescence, although the analysis of the image as a whole is often missed. In this paper we, therefore, evaluated the advantages of monitoring fluorescence images, presenting the physiological interpretation of different possible combinations of the most relevant parameters linked to fluorescence emission and the images obtained.
Considered as a non-invasive, fast and cheap technique that allows to rapidly detect and localize stressors effects on plants, chlorophyll a fluorescence (CF), has been largely used in plant science in the last decades, both clamping- and image-based Chlorophyll a fluorescence analysis (CFA) (Dayan and Zaccaro, 2012; de Carvalho et al., 2016). CFA gives the possibility to estimate, almost instantly, the photosynthetic efficiency under stress conditions and is especially useful in the detection of early stress responses (Chaerle and van der Straeten, 2000; Chaerle and Van Der Straeten, 2001; Chaerle et al., 2009). That is the reason why fluorescence measurement has been increasingly used to measure phytotoxicity in the last years.
Considering that herbicides will continue to be the worldwide method of weed control, easily and reproducibly exploring the wide range of molecules that nature offers seems the most appropriate way to search for new eco-herbicides. Secondary plant metabolites with relevant herbicide potential are essential in this context (Duke et al., 2000b), as the use of natural products allows protecting a priori the quality of the environment and, at the same time, the health of mammals during the control of weeds (Liebman, 2001). Therefore, in recent years, the study of the mode of action of natural compounds with herbicide potential is occupying a preferential place in integrated agriculture, thanks to the new physiological and molecular techniques that can integrate its multidisciplinary study (Sánchez-Moreiras et al., 2018). Among the several reasons for a long period without new herbicide target sites is also the difficulty to find new effective modes of actions for killing plants (Duke, 2011).
Looking at natural compounds, which have been undergoing strong evolutionary pressure for hundreds of years, would help to give light on this aspect. In fact, precisely the mode of action of these molecules is one of the potential advantages of secondary metabolites over synthetic herbicides. Natural compounds frequently present a strong structural diversity, which could result in novel molecular sites of action different from those already known for commercial herbicides. This would allow facing the weed resistance problem with a broader arsenal of modes of action (Duke et al., 2000a). The use of natural products as agrochemical compounds must necessarily go through the characterization of their mechanisms of action to know where, when and how they act in plant metabolism. That is the ultimate objective. However, a truly low number of modes of action is known at the current stage of research in plant natural compounds, which complicates their study and classification. Therefore, most studies on phytotoxicity induced by plant secondary metabolites focus on monitoring of their effects from a physiological perspective to detect the early effects of the compounds on roots and shoots. In this sense, CFA analysis is one of the techniques that could easily show the effects of bioherbicides on leaves. As stated by Dayan and Zaccaro (2012), the crucial step in the discovery of natural products that could be acting as photosystem II (PSII) inhibitors is simply evaluating the photosynthetic electron transport activity in leaves. Precise and reproducible CFA provides the demanded detailed analysis of the photosynthesis inhibition.
During the last 20 years, some excellent papers reviewed the use of CFA to measure stress response in plants (Maxwell and Johnson, 2000; Nedbal and Whitmarsh, 2004; Papageorgiou and Govindjee, 2004; Roháček et al., 2008; Kalaji et al., 2014, 2017; Guidi et al., 2019), while others focused on the use of imaging techniques to establish stress-related changes in chlorophyll fluorescence parameters (Chaerle et al., 2009; Martínez-Peñalver et al., 2011; Gorbe and Calatayud, 2012; Guidi et al., 2016). In this paper, we will provide a review of studies that applied chlorophyll fluorescence imaging (CFI) to monitoring the phytotoxic effects induced by plant natural compounds. Furthermore, we will present the physiological interpretation of possible results obtained by CF recording and will highlight the importance of considering both the PSII efficiency and its distribution over the plant.
The principles of chlorophyll fluorescence analysis are relatively simple. Basically, absorption of a photon promotes an electron of a chlorophyll a molecule to an excited state while a fluorescence photon is immediately emitted again during the return of the molecule to the ground state.
The first observation of fluorescence induced by solar radiation was recorded almost two centuries ago by David Brewster, which observed that illuminating a laurel leaves alcoholic extract with a sunbeam elicited a brilliant red light (Brewster, 1834). Moreover, he also observed that passing through the extract, the emission changed its color varying from red to orange and then to yellow, suggesting for the first time that this transition was probably due to chlorophyll re-absorption (Govindjee, 1995). The term “chlorophyll fluorescence” was coined by Stokes (1852) to describe this emission. Successively, Müller (1874) suggested a link between fluorescence emission and photosynthetic assimilation, which was successively confirmed by Kautsky and Hirsch (1931). These authors described, for the first time, the kinetics of chlorophyll a fluorescence emission of leaves previously adapted to dark, and then suddenly irradiated with light, correlating the signature of the initial fluorescence peak and its prompt decay to a lower steady-state to the time course of CO2 assimilation (for details see Govindjee, 2004).
The covariation between photosynthesis and chlorophyll fluorescence was successively described by McAlister and Myers (1940). They reported and described two different processes: (i) one characterized by an inverse relation between CF intensity and the rate of CO2 uptake, and the other (ii) considering a direct relationship between these two parameters.
A detailed description of this dual response was successively described by Duysens and Sweers (1963), which used a modulated excitation light for the first time and described, also for the first time, that the fluorescence yield was actively regulated by a process known today as “non-photochemical quenching” (Weis and Berry, 1987; Krause and Weis, 1991). This information was successively used to prove a quantitative relationship between the electron transport rate and the fluorescence yield (Weis and Berry, 1987; Genty et al., 1989).
After that, it became clear that CF is one of the mechanisms for energy dissipation, together with photochemistry and non-photochemical quenching (Schreiber, 1983; Krause and Weis, 1984; Demmig-Adams et al., 1990; Havaux et al., 1991; Björkman and Demmig-Adams, 1995). Therefore, being able to relate CF with changes in the photosynthetic apparatus it allows the quantification of the extent to which CF decreases by photochemistry (namely photochemical quenching) or non-radiative decay (non-photochemical quenching).
From the 1990s on, CFA has been widely used with the attempt to detect modifications occurring in the photosynthetic process, especially in plants under stress conditions (Schreiber and Neubauer, 1987; Endo et al., 1995; Maxwell and Johnson, 2000; Papageorgiou and Govindjee, 2004). CF parameters are very sensitive and detect the emergence of stress, even before visible symptoms appear over the leaf lamina, or a decline of photosynthesis can be determined by gas exchange measurements (Gorbe and Calatayud, 2012). However, in some cases, i.e., in plants subjected to abiotic stressors leading to rapid stomata closure, CF parameters might have a delayed response when compared to gas exchange analyses (Schreiber et al., 1995).
CF can be excited by illuminating green plant tissues with pulsed (Schreiber et al., 1986; Schreiber, 2004) or continuous (Strasser and Govindjee., 1991) visible or ultra violet (UV) light by either single or grouped LEDs (light emitting diode) or halogen lamps. In addition, deuterium lamps were also used in old systems (Schreiber et al., 1993). Using visible light, light-induction kinetics, as well as the measurement in the true steady state, is one of the most widely used approach in CF measurement (Lazár, 2003; Gorbe and Calatayud, 2012). This technique, which uses actinic light illumination, allows monitoring and measuring both the photochemical and non-photochemical processes involved in fluorescence quenching. The pulse amplitude modulated (PAM) fluorometer is the most common and largely used fluorometer in CF monitoring (Bolhar-Nordenkampf et al., 1989; Häder et al., 1998). In addition, also OIJP test is a very informative and routinely used method to evaluate fast kinetics of CF (Hermans et al., 2003; Digrado et al., 2017).
Depending on the state of electron transport chain components and dark/light adaptation of the photosynthetic tissues, five different CF emission signals can be detected: F0, F′0, Fm, F′m, and Fs (Maxwell and Johnson, 2000) (Table 1). F0 and Fm, determined on dark-adapted samples, correspond to both minimum and maximum fluorescence yield before and after a saturating light pulse takes place, respectively. Briefly, a period of dark adaptation (20–30 min) allows all reaction centers to be open and the exposure to modulated weak light (<1.0 μmol photons m–2 s–1 which is not sufficient to induce an electron transport chain) induces an increase in the CF yield, namely F0 (Table 1). Then, a strong saturating light pulse (from 8,000–15,000 μmol photons m–2 s–1 which induces the closure of all reaction centers) is applied and induces a rapid increase in the CF yield, namely Fm (Table 1). After that, CF yield decreases and, in the presence of actinic light, it reaches steady-state values (Fs) that correspond to a balance between the reduced and oxidized state of primary electron acceptor QA. Conversely, F′0 and F′m are determined after sample receives actinic illumination, and represent the minimal and maximal fluorescence yield in light conditions, respectively (Table 1). The determination of F0′ under light conditions requires the use of far-red light to transiently and selectively excite PSI, and thus to enhance the oxidation of the electron transport chain (Bilger and Schreiber, 1987).
Using these five fluorescence emission signals is possible to calculate other CF parameters. Among them, it is worth to mention the maximal PSII quantum yield (Fv/Fm), the effective PSII quantum yield (ΦII), both photochemical (qP) and non-photochemical quenching (NPQ), as well as the quantum yield of regulated (ΦNPQ) and non-regulated energy dissipation (ΦNO), which compete with ΦII for energy partitioning (i.e., ΦII + ΦNO + ΦNPQ = 1; Kramer et al., 2004) (Table 1). Of note, the qNP coefficient consists of three different components: energy-dependent quenching related to the build-up of the trans-thylakoidal pH-gradient, qE; quenching due to the state II-I transition of the phosphorylated light harvesting complex of PSII, qT; and photo-inhibitory quenching, qI (Horton and Hague, 1988).
Nowadays, other new parameters have been introduced with the attempt to make CF a very detailed diagnostic tool. In some cases some stress-related parameters, allow to anticipate the occurrence of visible symptoms over the leaf lamina. As an example qPd (photochemical quenching measuring in the dark) enables the detection of earliest signs of photoinhibition (when qPd value is 1 corresponds to 100% of open RCIIs) (Ruban and Murchie, 2012). In addition, the use of imaging-based instruments provides information on the distribution of CF over a selected area of the sample (Gorbe and Calatayud, 2012).
However, in view of the high number of excellent reviews on CF, both applicative and theoretical, the in-depth description of CF parameters is out of the scope of the present review (for example, for detailed reviews on CF parameters refer to Schreiber and Bilger, 1993; Schreiber et al., 1998; Maxwell and Johnson, 2000; Baker and Rosenqvist, 2004; Gorbe and Calatayud, 2012; Kalaji et al., 2014, 2017; Guidi et al., 2016).
The invention of video imaging systems was an important breakthrough in the field of chlorophyll fluorescence analysis (Omasa et al., 1987; Fenton and Crofts, 1990). In fact, this technique not only allowed to spatially examine the heterogeneity within a sample, but it also made possible to evaluate those changes simultaneously on a wide number of samples, making the CFI more popular as diagnostic (Baker, 2008), screening (Barbagallo et al., 2003) and phenotyping tool (Rühle et al., 2018; Pérez-Bueno et al., 2019).
The use of chlorophyll photometer capable to image CFA is known from decades. At the beginning, the CFI instruments were able to image chlorophyll fluorescence at Fm and Fm′ and at F or Fs by using a high incident irradiance, but those instruments were unable to image at F0, F0′, and Fs under moderate to high incident irradiance (Genty and Meyer, 1995; Siebke and Weis, 1995a,b; Scholes and Rolfe, 1996). Oxborough and Baker (1997) described the first instrument that was able to image those parameters. This instrument, mainly based on an epifluorescence microscopy connected to a CCD camera, was characterized by a large dynamic range, and by the capacity of generating images of CF using low incidence irradiance (0.1 μmol m–2 s–1). In addition, it gave the possibility to work on part of the leaf tissue as well as on single cells.
Lichtenthaler et al. (2005) developed a compact flash-lamp fluorescence imaging system, which was based on the Karlsruhe/Strasbourg laser-induced fluorescence imaging system (Lang et al., 1994; Lichtenthaler, 1996; Lichtenthaler and Miehé, 1997). The instrument was produced to replace, as excitation source, the expensive ND:YAG laser by a cheaper xenon flash lamp, which allows measurements with high pulse frequency and short gating times (Buschmann et al., 2000; Lichtenthaler and Babani, 2000; Lichtenthaler et al., 2005).
The technique, as well as the tools to monitor chlorophyll fluorescence emission, was further implemented and hyphenated with phenotyping tools for both diagnostic and breeding purposes.
CFI, associated to phenotyping tools, gives the possibility to early detect plants stress responses to both biotic and abiotic stressors, before the development of visible symptoms. At agricultural level, especially for sustainable/precision agriculture, the use of CFI as research tool could have an extensive field of application. CFI could facilitate the researchers in identifying and evaluating crop stress thresholds allowing, with the help of sensors (e.g., temperature, humidity and conductivity sensors among others), not only to make targeted and/or localized interventions but also to plan them only when necessary. This could permit to anticipate irreversible damages to the photosynthetic apparatus. Anyway, at the moment, CFI technique is too slow and expensive to be used as a commercial diagnostic tool in open field and/or greenhouses.
Depending on the crop and on the pedological and pedoclimatic conditions, it can be used as a research tool to extrapolate the stress-thresholds of a given species, which can be integrated in more complexes algorithms and used for crop management.
At both laboratory and field levels, Photon Systems Instruments (Brno, Czech Republic), Walz (Effeltrich, Germany), and Technologica Ltd., (Essex, United Kingdom) commercialized several instruments (for more details have a look at the website of the companies) that allow the application of this technology at macroscopic and microscopic levels, and on terrestrial and aquatic organisms (Levin et al., 2017; Ayalon et al., 2019).
Imaging fluorometers have been also integrated with a gas-exchange chamber, which allows to obtain information about CF, the imaging of its parameters, and also about the plant gaseous exchanges, giving wider information concerning the photosynthetic machinery status in response to stress (Daley et al., 1989; Rolfe and Scholes, 1995; Kurepin et al., 2018).
The combination of CFI with the technique of the infra-red gas exchange (IRGA) allows to directly correlating the efficiency of PSII with the CO2 assimilation rate. This could be achieved avoiding the photorespiration through the increase of CO2 concentration or reducing the concentration of O2. This technique has been used to visualize patterns of CO2 diffusion in leaves, enabling the determination of gas fluxes within leaves of different species (Morison et al., 2005, 2007; Lawson and Morison, 2006).
Also, the use of CFI tools in combination with other imaging techniques could give important information in plant phenotyping. For example, CFI coupled to hyperspectral imaging was used for early detecting fungal disease in wheat, whereas coupled to thermography it was used to provide information concerning the correlation between photosynthetic rate and stomatal behavior, as well as in imaging intrinsic water use efficiency (Chaerle et al., 2007; Lawson, 2009; Bauriegel et al., 2011).
Moreover, imaging fluorometers integrated with phenotyping platforms, which in the past were exclusively used for research purposes, are now commercially available (e.g., HyperAIxpert from Lemnatech; PlantScreen System from Photon Systems Instruments, etc.). These platforms, in addition to the phenotypic traits, allow imaging of chlorophyll fluorescence in order to get high-throughput analysis of plant phenotype (Awlia et al., 2016). To study plant-pathogen interactions as well as to investigate changes in plant physiology and biochemistry, in response to stress, imaging fluorometers have been integrated with a GFP filter for imaging (not simultaneously) green fluorescent proteins in transformed plants, and/or in plants inoculated with GFP-transformed strains of pathogens, which is extremely useful for molecular and cellular biology studies (Lee et al., 2019; Pérez-Bueno et al., 2019). Other imaging fluorometers have also been adapted to study the photosynthesis in plants growing under water, which allows to evaluate the response of plants to several stressors such as water pollution or CO2 variations (Papathanasiou et al., 2020).
Other tools, applicable to microscopy, have been also developed in order to allow the monitoring of CFI heterogeneities at the level of single cell (e.g., on algae or stomata guard-cells) and they could allow to taxonomically differentiate algae types, like diatoms, chlorophytes, and cyanobacteria (Oxborough and Baker, 1997; Levin et al., 2017).
For example, through the combination of a microscope CFI system equipped with an IRGA chamber, Lawson et al. (2002) were able to demonstrate that the major sink for guard cell photosynthetic electron transport was the Calvin cycle activity and that the photosynthetic efficiency of the guard cell is not linked to the opening and closing of the stomata.
The majority of CFI tools, to ensure a coverage of actinic illumination and fully saturating pulses on a huge portion of plant area, requires large panels on which light-emitting diode arrays are mounted making these tools extremely large in size and barely usable in open field. Nevertheless, some fluorescence monitoring tools, equipped with a cabinet (for dark-adaptation purposes) and mounted on wheels (to allow movement in the field collecting images over the crops), were built (Murchie and Lawson, 2013; Tan et al., 2018).
Finally, new generations of low-weight dfov (dual field of view) spectrometer systems have been developed to monitor and image solar-induced CF variations in both open fields and green houses. Those instruments can be managed remotely and located on both fixed and mobile workstations (i.e., drones) (Atherton et al., 2018).
The use of CF as a phenotyping and/or diagnostic tool was quickly growing in the last decade. Several reviews were written about it (Humplík et al., 2015; Pérez-Bueno et al., 2019) and new researchers are trying to extend its applications (Weber et al., 2017; McAusland et al., 2019).
Especially in herbicidal studies, CF has been extensively used as a marker for the study of herbicide mode of action or for the identification of weed resistance (Dayan and Zaccaro, 2012). However, although several manuscripts focused on both monitoring CF during several stress, including herbicides, and the pattern of fluorescence changes through imaging, relatively few information is available concerning the use of imaging signatures during the evaluation of the herbicidal potential of natural compounds and extracts.
Because of that, the best way to evaluate natural compounds target and mode of action should be to compare the fluorescence signature induced by natural compounds with that of the most known and used herbicides. Unluckily, the number of studies doing that is little, and the use of CFI tools in this field of the research is extremely low. Moreover, this technique is often used just to get PSII parameters without giving the right importance to the imaging output. As a consequence, the information concerning the pattern of action of natural phytotoxic compounds is fragmented and superficial.
One of the first studies, focusing on the application of CFI analysis on phytotoxic natural compounds, was reported by Beninger et al. (2004). In particular, they evaluated the phytotoxic potential of three phenolic compounds isolated from Chrysanthemum morifolium on the model species Lemna gibba. They observed that chlorogenic acid and the flavanone eriodyctiol strongly affected the photosynthetic activity with deep reductions in Fv/Fm. Both compounds acted as bleaching herbicides causing a loss of photosynthetic pigments and the inhibition of photosynthetic activity. However, interestingly, while overall Fv/Fm values were reduced just in the plants treated with 1000 ppm, the area of the plant experiencing photosynthesis was reduced also in the leaves treated with the lowest concentration (100 ppm), probably due to the loss of photosynthetic pigments.
Kriegs et al. (2010) demonstrated that the quantum yield efficiency (Fv/Fm) of Arabidopsis plants, fumigated with the monoterpene camphor, was directly affected by the treatment. In particular, they reported that during short-time fumigation the parameter Fv/Fm was strongly lowered compared to control, but it promptly recovered at the end of the treatment, and the plant fitness was significantly strengthened. On the other hand, repeated exposure to the monoterpene led to irreversible damages and alteration in plant phenotype. Probably, the negative effects induced by long-term exposure could be connected to the high cuticular dewaxing ability of camphor as previously demonstrated by Schulz et al. (2007). However, although authors used the imaging apparatus Growscreen-Fluoro, an automated imaging pipeline designed to analyze the chlorophyll fluorescence of rosette plants, no images about the effects of this monoterpene on plants were shown in the manuscript. This is a pity since images could be extremely helpful in understanding the dynamic of the effects of the terpenoid and the overall plant responses to the treatment.
An example of how imaging techniques can broaden our knowledge on natural compound effects was published by Sánchez-Moreiras et al. (2011) with the allelochemical 2-3H-benzoxazolinone (BOA). Previous single-point measurements of photochemical and non-photochemical parameters in BOA-treated plants suggested that increase in fluorescence emission was due to BOA-induced oxidative stress (Sánchez-Moreiras and Reigosa, 2005). However, CFI together with imaging hydrogen peroxide and superoxide anion, revealed that the primary phytotoxic effect of BOA was the induction of premature senescence, and oxidative stress was just a secondary effect of this treatment (Sánchez-Moreiras et al., 2011).
The advantage of obtaining an image of CF and its distribution all over the plant in intact and alive individuals is one of the aspects that can most enrich the study of phytotoxic compounds, as it can gives information about the area of the leaf where the effect starts first. Studies carried out on the trans-cinnamic derivative o-coumaric acid, one of the major constituents of the invasive species Eupatorium adenophorum, demonstrated that this molecule significantly affected the photosynthetic machinery of the model species Arabidopsis thaliana, pointing out a differential effect on photosynthesis depending on the age of the leaves (Zheng et al., 2012). In particular, they observed that after 7 d of treatment, Fv/Fm dropped dramatically in older leaves, whereas younger central leaves were hardly affected, suggesting that o-coumaric acid could promote leaf senescence. In addition, Graña et al. (2013b) demonstrated that adult Arabidopsis plants sprayed with the monoterpenoid citral had a heterogeneous spatial distribution in fluorescence emission, mainly concentrated at the edges of the older leaves, whereas the youngest leaves were not affected. This could suggest the initiation of early senescence processes, where the photosynthetic apparatus is systematically dismantled (Wingler et al., 2004).
Several studies evaluating effects of natural products by CFI indicated that the majority of these products and/or their extracts generally reduced Fv/Fm. This may suggest a direct damage to PSII, whereas the yield of non-photochemical energy dissipation, ΦNO and ΦNPQ, was extremely variable, probably depending on type and/or dose of the extract or compound assayed. In fact, natural compounds that are extremely phytotoxic at high doses can be stimulant at low doses, a phenomenon known as hormesis (Belz and Duke, 2017). For example, the aqueous extract of Mentha X piperita may be inhibitory or stimulatory on chlorophyll content and on PSII (analyzed by CFI) of sunflower leaves depending on the concentrations assayed (Skrzypek et al., 2015). The authors observed that low concentrations of peppermint extract significantly increased Fv/Fm ratio and the ΦNPQ on the entire leaf surface. In contrast, higher concentrations significantly reduced the regulated non-photochemical energy dissipation suggesting a negative effect on the photosynthetic machinery. Skrzypek et al. (2015) observed also that the highest changes in CF were specifically around the petioles and in the distal margins of leaf blade. Those results highlight the importance of imaging parameters, which can give a lot of quick and precise information of the exact stimulatory and inhibitory doses of a given compound, as well as the induced damage.
In adult chalcone-treated Arabidopsis plants, Díaz-Tielas et al. (2014) found an early decline in ETR concomitant with a significant increase in ΦNO and a late reduction in maximum PSII efficiency (Fv/Fm). Supported by the imaging of damages localization, they suggested that the chalcone-induced reduction in photosynthetic rate could have resulted in an excessive demand on regulated antenna de-excitation processes inducing damages to the antenna complex and, consequently, Fv/Fm reduction. Based on these previous imaging studies, Díaz-Tielas et al. (2017) observed that chalcone treatment on young Arabidopsis seedlings induced progressive pigment degradation and bleaching. They demonstrated that this progressive de-greening, together with Fv/Fm, ΦII reduction and ΦNO increase, was directly linked to early plasma membrane depolarization and dramatic effects on chloroplasts structure and function, as part of chalcone mode of action.
Essential oils (EOs) are among the most studied and commercially used natural extracts with phytotoxic potential. Recent studies demonstrated that pure terpenoids, isolated from EOs, have a wide range of metabolic targets and modes of action (Graña et al., 2013a; Araniti et al., 2017a). It has been proven that several compounds can act synergistically changing completely the effects of these natural mixtures and/or pure molecules on the photosynthetic machinery. For example, Araniti et al. (2018a) demonstrated that EO isolated from Origanum vulgare inhibits glutamate and aspartate metabolism in A. thaliana. The inhibition of these metabolic pathways induced an accumulation of ammonia in leaf cells and, concomitantly, a cascade of reactions that limited the efficiency of PSII. Through the imaging of Fv/Fm and ΦII parameters authors were able to identify which areas of the leaves were mostly affected by EOs treatment. Moreover, they highlighted through the imaging output an overlap between reactive oxygen species (ROS) accumulation (monitored through in situ staining methods) and damages to PSII (reduction in Fv\Fm).
This was not the first work detecting overlap among PSII damage and ROS accumulation in response to terpenoids. Araniti et al. (2017b), studying plant-plant interaction, mimicking natural conditions, demonstrated that volatiles released by the widespread species Dittrichia viscosa were able to induce ROS accumulation in lettuce leaves causing Fv/Fm inhibition. Through CFI, they also evidenced an overlap between the leaf area where ROS were accumulating and leaf area characterized by Fv/Fm reduction. Those results suggested that probably the burning activity of the EOs could be mainly due to a side effect (ROS burst) instead of a direct contact of the chemical mixture with the leaves.
Recently, Synowiec et al. (2019) bioassayed the effects of caraway and peppermint EOs on Zea mays and its associated weeds. They demonstrated, through CFI, that the application of caraway EOs at a given concentration strongly affected the PSII of Echinochloa crus-galli. However, it was hardly effective on Z. mays, highlighting the species-specific effects of EOs, and suggesting that caraway EOs could be a good candidate for weed management in maize crop.
Graña et al. (2013b), bioassaying through sub-irrigation or spraying, the monoterpene citral on adult plants of A. thaliana observed that both treatments strongly affected either the photochemical or non-photochemical activity. Citral effects were earlier and more pronounced in sprayed plants than in watered. In fact, while sprayed leaves were severely damaged by the treatment, leaves of sub-irrigated plants were not apparently affected. ETR, ΦII, and Fv/Fm values revealed that photosynthesis was reduced in citral-watered plants as a result of a general slowing down of the metabolism, while citral-sprayed plants were photoinhibited and presented physical damage due to the treatment. These results were also supported by the spatio-temporal CFI. The same experiments carried out by Araniti et al. (2017c), bioassaying the phytotoxicity of trans-caryophyllene on Arabidopsis adult plants, didn’t show any effects on plants sprayed, whereas PSII of plant sub-irrigated was extremely altered. They concluded that the observed PSII damage would be the consequence of plant water status alterations accompanied by ROS burst and oxidative stress. These results suggest that CFI outputs could be used in screening programs to highlight, depending on the molecule bioassayed, the best method of application of a chemical. In addition, concerning the last two chemicals (citral and trans-caryophyllene), the data further highlight the high potential of the imaging techniques as phenotyping tool, which can be helpful in giving hints concerning the target and the potential mode of action of natural phytotoxins.
CFI has been used also to evaluate the phytotoxic effects of natural compounds produced by fungi and bacteria. Guo et al. (2019) demonstrated that gliotoxin, a fungal secondary metabolite, affects both the electron transport through PSII at the acceptor side and the reduction rate of PSI end electron acceptors’ pool. Through CFI, authors identified the concentration inducing physical damage to PSII. In addition, Xiao et al. (2020) reported that alamethicin, an antimicrobial peptide isolated from fungus Trichoderma viride, acts on PSII with a mechanism similar to the commercial herbicide diuron (Laasch et al., 1983). In particular, this secondary metabolite would interrupt PSII electron transfer beyond the primary plastoquinone at the acceptor side, leading to PSII reaction centers inactivation. In addition, alamethicin destroys the PSII pigment architecture but does not affect the oxygen-evolving complex at the donor side. The damages in the leaves (necrotic areas on leaf surface) were visible only in plants treated with the highest concentrations of alamethicin, whereas the imaging output of the CFI system allowed to understand that even the lower concentrations altered the photosynthetic machinery (Fv/Fm decrease) although damages were not evident, confirming again the power of this technique.
Regarding the study of phytotoxicity induced by plant natural products, the majority of the works reported in literature, based on CF monitoring, were carried out with clamping tools that record single-point measurements on restricted leaf parts. The advantages of monitoring CF through imaging instead of single-point fluorescence have already been discussed (Oxborough, 2004; Lichtenthaler et al., 2005). Martínez-Peñalver et al. (2011) demonstrated that measurements obtained through single-point fluorescence do not always reflect the heterogeneity of the stress-related effects in plants. In their research, authors compared the temporal and spatial distribution of fluorescence emission in plants treated with allelochemicals, abiotic factors, and heavy metals. They observed that some stress factors, as the assayed heavy metals, induced a homogenous inhibition of the maximum PSII efficiency all over the whole plant, while in plants treated with allelochemicals the inhibition was mainly located on the margins of old leaves whereas younger leaves were unaffected. They suggested that these irregularities in CF distribution along plant make difficult any correlation with single-point measurements, typically done through clamping fluorometers.
Gao et al. (2018) bioassayed also the effects of usnic (UA), benzoic (BA), cinnamic (CA), and salicylic acid (SA) on the photosynthetic apparatus of the algae Chlamydomonas reinhardtii. They found that UA and SA have probably stronger photosynthetic inhibitory activity than CA and BA acids. The four phytotoxins showed multiple targets in chloroplasts. In particular, UA acid decreased photosynthesis inducing a reduction of PSII O2 evolution rate, interrupting PSII electron transport (strong ETR reductions), and inactivating the PSII reaction centers (strong Fv/Fm decrease). Based on the JIP-curve, UA-treated cells showed a fast increase of J-step, which is due to the accumulation of QA– when the electron flow beyond QA is blocked at the PSII acceptor side. The other site of action of UA would be the oxidizing site of PSII, as indicated by the low PSII O2 evolution rate. Moreover, UA reduced pigment content, damaging the conformation of antenna pigment assemblies, as suggested by the decreased values of ABS/CS (average antenna size per excited leaf cross-section) and TR0/CS (trapped energy flux per excited leaf cross-section), resulting in destroyed structure and function of PSII. Gao et al. (2018) suggested also that damages induced by SA on the photosynthetic machinery would be mainly attributed to inhibition of PSII electron transport beyond primary plastoquinone acceptor at the acceptor side, and the inactivation of the PSII reaction centers by decreasing ΨEO (the probability that an electron moves further than QA–) and φEO (the quantum yield for electron transport). SA induced as well thylakoid membranes destabilization by changes at D1/D2 dimer and the polypeptides of the water-oxidizing complex. Finally, both CA acid and BA acid would act by reducing PSII electron transport efficiency at the acceptor side and the amount of active PSII reaction centers, but with some differences. In particular, BA disrupted the flow between antenna pigments and PSII reaction center, with decreases of Ft, FM, Fv/Fm, and TR0/C, while CA did not affect these parameters, but decreased PSII O2 evolution rate.
Imaging techniques can be also decisive to study recovering processes. Plants treated with the allelochemical protocatechualdehyde (Martínez-Peñalver et al., 2012) highlighted tolerance to this molecule, as whole-plant images of Fv/Fm showed the greatest number of damage spots among 9 and 24 h after treatment, but images of 96, 144, and 192 h showed the recovering of PSII parameters within a week of treatment.
Recent studies carried on the phenolic acid trans-cinnamic acid, assayed at sub-lethal concentration, pointed out that, besides the reduction in growth, trans-cinnamic significantly stimulated the photosynthetic machinery of Zea mays seedlings (Araniti et al., 2018b). In fact, in plants treated with this known phytotoxic molecule, the dark adapted PSII parameter (Fv/Fm) was not affected, whereas a significant increment in pigment content, and the stimulation of the light adapted PSII efficiency (ΦII), the regulated dissipation of the energy in form of heat (ΦNPQ) and the apparent electron transport rate was observed. At the same time the CFA (ΦNO) was significantly stimulated by the treatment. Those data are supportive for the capability of the plant to cope with trans-cinnamic acid effects in different ways. From one hand, Z. mays plants increased the level of photochemistry and were able to increase the level of energy channeled toward the electron transport chain (see ETR and ΦII increments). On the other hand, treated plants showed also a higher capacity to dissipate the energy, which exceeds photochemistry through both regulated (see ΦNPQ) as well as unregulated mechanisms (see ΦNO), suggesting that plants were facing phytotoxicity but were able to cope with it (Araniti et al., 2018b).
Similarly, recent studies assayed rutin, a glycoside combining the flavonol quercetin and the disaccharide rutinose, on the model species A. thaliana. This metabolite, particularly present in the invasive species Acacia melanoxylon, affected the photosynthesis and excitation energy flux responses (Hussain and Reigosa, 2016). Hussain and Reigosa observed that the dark-adapted PSII (Fv/Fm) and the non-photochemical quenching ΦNPQ were significantly inhibited after 7 d of treatment. Moreover, plant response to rutin was characterized by a marked decrease in excitation energy fluxes (ΦII, non-photochemical quenching coefficient (qN) and ΦNPQ], suggesting plant damage.
The aromatic organic compound coumarin has been found to indirectly affect the photosynthetic machinery through induction of ROS burst and inhibition of several enzymes involved in ROS scavenging activity (Araniti et al., 2017d). Authors demonstrated, through an integrated physiological and -omic approach, that coumarin severely inhibited the effective quantum yield of the PSII, the maximum PSII efficiency, the energy dissipation in the form of heat, the estimated electron transport rate and the coefficient of the photochemical quenching. On the other hand, it significantly stimulated both the fluorescence emission and the coefficient of the non-photochemical quenching.
The identification of the mode of action of a molecule, both synthetic and natural, is a delicate process that needs to exclude from the experimental conditions all the sources of variability (e.g., lack of nutrients, temperature changes, light variation, etc.). CF could be affected by a wide number of both biotic and abiotic factors (e.g., drought, heat, flooding, temperature variation, parasitization, etc.) (Dong et al., 2020; Duarte et al., 2020; Zhou et al., 2020; Zhuang et al., 2020), which makes the evaluation of the mode of action of natural molecules quite impossible because of the overlapping of stressing conditions, which makes impossible to attribute the observed effects to the molecule assayed. Therefore, it is strongly suggested to perform experiments in completely controlled conditions using phytotrons and cropping plants hydroponically or on inert substrates enriched with nutrients and carbon sources (Graña et al., 2013b, 2016; Araniti et al., 2017c). Once identified the target and the potential mode of action of the molecule, the experiments could move to the next step, which consists in bioassays in microcosms trying to mimic natural conditions in greenhouses and/or open fields. This will allow the identification of the effectiveness of the molecules and their impact on the environment (e.g., on soil microorganisms) (Jouini et al., 2020). Barbagallo et al. (2003) used the CFI technique to rapidly screen the leaves metabolic perturbations induced by six different herbicides. However, although the same parameters are used to detect synthetic herbicides effects, those effects are not necessarily similar to natural bioherbicides, especially when secondary metabolites are known to commonly show more than one mode of action.
Multiple combinations of CFI parameters can be found in a leaf when exposed to a stressing factor (Table 2). Therefore, a correct physiological interpretation of the decreases and increases of the different parameters will give information about the ability of the plant to face stress with efficient protection mechanisms or with more toxic and inefficient dissipation mechanisms, which will lead to plant damage and stress. Increases of heat or fluorescence emission can occur in the plant without significantly reduce the PSII photochemical efficiency, (ΦII), as some types of stress do not instantly affect PSII quantum yield (Lopes et al., 2012). Heat increases are sometimes compensated by decreases in fluorescence emission and the other way around (scenarios 1–2, Table 2), because the stress is not strong enough, and effective compensation occurs in the electron transport chain, or because the measurements were recorded in an early phase of the stress response, before damage can be detected.
Table 2. Relevant parameters to be considered in the physiological interpretation of the measurement of chlorophyll a fluorescence.
Decreases of ΦII usually result in increases of non-photochemical quenching (heat) when the plant is able to orchestrate regulated energy dissipation through xanthophylls cycle, pH regulation, or reversible phosphorylation of light harvesting chlorophyll a/b binding proteins (LHCII) (scenarios 3–5, Table 2). By contrary, ΦII decreases will result in increases of non-regulated energy emission when photochemical energy conversion fails, and protective regulatory mechanisms are inefficient (scenarios 4–9). However, increases of ΦNO and decreases of ΦII does not necessarily indicate that the plant is already damaged, as might be being protected, albeit weakly, until further radiation occurs or stressing factor remains (scenarios 4–5). The latter was shown by Martínez-Peñalver et al. (2012) with the secondary metabolite protocatechualdehyde, where strong increases in ΦNO didn’t result in ETR or Fv/Fm decreases, or by Araniti et al. (2018b) in Zea mays seedlings treated with trans-cinnamic. Ferredoxin or Mehler reaction can be accepting the electrons diverted from photochemical reactions when no decreases in ETR are observed (scenario 4). The same values of ΦNPQ, ΦNO, ΦII, and Fv/Fm are observed in scenario 5, although decreases in ETR also occurred related to decreases in the efficiency of PSII.
Scenarios 4–7 of Table 2 show dynamic photoinhibition, where decreases of photochemical conversion occur and plant has serious problems to cope with the incident radiation, but no physical damage is detected at the photosynthetic apparatus. The latter is suggested by unchanged or very slight decreased Fv/Fm values, which reveal flexible adjustment to environmental conditions (Werner et al., 2002). In contrast, plants showing statistically significant decreases in PSII efficiency (ΦII) and strong increases in fluorescence emission (ΦNO) usually show weak to strong decreases in the maximum PSII efficiency (Fv/Fm), which suggests different degrees of physical damage at the protein-pigment complexes of the light harvesting antennae of PSII (scenarios 8–9, Table 2). Consequently, chronic photoinhibition due to acute or long-term environmental stress occurs, as previously found for some natural compounds as citral or trans-caryophyllene (Graña et al., 2013b; Araniti et al., 2017c). Late Fv/Fm decreases can suggest a secondary effect of the stressing factor; i.e., Sánchez-Moreiras et al. (2011) demonstrated that Fv/Fm and ΦII reduced values appeared to be correlated to necrotic processes after secondary oxidative stress, consequence of early senescence processes.
On the other hand (Table 2, scenario 9), plants could be unable to develop strategies of protection (as heat emission) when a stress factor has a direct effect on the photosynthetic machinery, increasing non-regulated emission of energy as fluorescence and inducing photoinhibition. Then, biochemical and photochemical phases of photosynthesis can be affected, altering electron transport and damaging PSII reaction centers, as found by Sánchez-Moreiras et al. (2011) in leaves of Arabidopsis plants treated with the secondary metabolite BOA.
Sometimes, similar values of ΦII, ΦNPQ, and ΦNO, and ETR result in totally different impact on the maximum PSII efficiency (Fv/Fm), as previously found by Díaz-Tielas et al. (2014). Authors demonstrated in this study that, although similar values of PSII quantum yield, heat dissipation, fluorescence emission and electron transport rate were found for Arabidopsis plants sprayed or watered with the flavonoid trans-chalcone, watered plants were able to protect the antennae complex and the reactions centers along the whole experiment, while sprayed plants showed decreased values of Fv/Fm at the end of the experiment, suggesting underprotection and possible physical damage at the antennae complex.
All of these scenarios (1 – 9) are usually found when the natural product affects photochemical phase first, by altering antennae complex, reaction centers, pH gradient through the thylakoid membranes, or competing with other molecules to accept the electrons coming from PSII and PSI. However, in some cases, i.e., senescence processes, stomatal closure or other situations restricting CO2 assimilation, is the biochemical phase the first part affected of the photosynthetic machinery (Ping et al., 2015), resulting in decreases of electron transport rate (ETR values) without or with slight alterations of the other parameters (scenario 10). When accumulated ROS cannot be detoxified (Paul and Foyer, 2001), and antioxidant systems or other photo-protective mechanisms, as xanthophyll cycle or increased photorespiration, are not enough, physical damages at the PSII reaction centers or antennae complexes can occur, resulting in a scenario similar to scenario 9 of Table 2. This has been recently found for plants treated with the nitrogen-containing natural compound norharmane (López-González et al., 2020).
On the other hand, the necessity of synthesizing new molecules in response to adverse conditions (i.e., proline, antioxidants, and others; Gonzáles et al., 2008) can lead to increased photosynthetic efficiency in plants (Desotgiu et al., 2012; scenario 11, Table 2). This increase in operating efficiency (ΦII) can be usually obtained by reducing regulated dissipation of light energy (ΦNPQ) as shown by Martínez-Peñalver et al. (2012) in plants treated with 3,4-dihydroxybenzaldehyde, which found a compensatory increase of ΦII at the beginning of the experiment that was correlated with a decrease of ΦNPQ.
Coefficients qN and qP (scenarios 12 and 13, Table 2), can give more information about non-photochemical quenching and status of the PSII reaction centers, making data interpretation easier. The increase of qN without ΦNPQ increase (scenario 12) suggests that plants are experiencing photoinhibition or about to undergo it. The same for the combination shown in scenario 13, where stressing situation reduced the coefficient qP, suggesting a physical damage at the light-harvesting complex II (LHCII), which could firstly occur at the site of PSII reaction center that is associated with PSII electron transport (inhibiting PSII repair), at the water splitting site in the oxygen evolving complex (OEC), or at both sites at the same time (Oguchi et al., 2009, 2011; Zavafer et al., 2015). Similar results were found by Araniti et al. (2017c) in plants treated with an intermediate concentration of trans-caryophyllene or by Gao et al. (2018), when testing different secondary metabolites, such as usnic, BA, CA, and SAs.
Finally, ΦNPQ/ΦNO ratio; i.e., the ratio of quantum yield of regulated to non-regulated energy dissipation in PSII, which is connected to the ability of photosynthetic apparatus to activate photoprotective mechanisms (Klughammer and Schreiber, 2008), can tell in a rapid and easy way if the plant is photo-protected (high values) or is undergoing photoinhibition or photodamage processes (low values).
However, all these data cannot provide direct information about where the damage is occurring first, or how the acclimation responses are being implemented into the leaf. Moreover, the so-called phenomenon of “patchy photosynthesis” due to ununiform stomatal closure or to heterogeneities in the leaves (Meyer and Genty, 1999; Beyschlag and Eckstein, 2001; Lopes et al., 2012) can be a source of errors when recording improperly single-point CF measurements. Imaging techniques avoid misunderstandings due to this phenomenon, as leaf fluorescence is obtained at once (Martínez-Peñalver et al., 2012).
Unfortunately, very few studies have analyzed the whole plant fluorescence emission after natural compound phytotoxicity, even though CFI allows this measurement in an easy and fast way. Therefore, with this paper, we want to encourage researchers in phytotoxicity to record the whole response of the plant and monitoring this response all over the time to obtain a more accurate information about the effect of the compound. Careful spatial analysis of the images of fluorescence emission is appropriate when studying phytotoxic effects in plants, as far as we do not know, before measuring fluorescence, which type of effect will be induced in the plant. This is applicable to sprayed but also to watered application of the compounds, since effects related to some types of stress induced by the compound (i.e., oxidative or water stress) can result in spots of fluorescence emission in the leaves of the treated plants. Figure 1A shows an Arabidopsis seedling grown under optimal conditions of light, temperature, humidity and CO2 concentration. As seen, Fv/Fm values are optimal and homogeneous all over the plant. However, some stressing factors, as plant natural compound-induced phytotoxicity, can decrease the overall photosynthetic capacity of the plant decreasing maximum PSII efficiency in young and old leaves, as shown in Figure 1B. In this case, the homogeneous distribution of the effect makes single-point measurements of fluorescence precise and accurate, anyway if measurements are done in young or old leaves, or in the center or the border of the leaves. By contrary, some natural compounds induce asymmetric effects on the photosynthetic capacity of the plant (Sánchez-Moreiras et al., 2011; Graña et al., 2013b), as shown in the rest of the images included in Figures 1C–F.
Figure 1. Imaging pictures of maximum PSII efficiency (Fv/Fm) of Arabidopsis seedlings at different physiological status. (A) Control plant with optimal PSII photosynthetic efficiency; (B) Whole decreased of photosynthetic efficiency that is homogenous all over the plant; (C) Decreased photosynthetic efficiency in the cells close to the vascular bundles. The rest of the plant remains intact; (D) Strong decreased of photosynthetic efficiency in the border of the older leaves; (E) Decreased photosynthetic efficiency mainly in the younger leaves; and (F) Strong decrease of photosynthetic efficiency in the whole plant, with punctual inhibition spots in the leaves.
An asymmetric effect starting in the cells closed to the vascular bundles is shown in Figure 1C, as indicated by orange and red colors related to decreased Fv/Fm values. This effect could be related to early water stress phenomenon, as previously shown in thermal and salt stress by Martínez-Peñalver et al. (2011), which found a characteristic pattern of reduction that affected the central areas of the young leaves close to the vascular bundles. This localized increase of fluorescence could be due to altered water stress status after treatment with a natural product, or to an abscisic acid mimic action of the natural product applied, as suggested by Graña et al. (2013b) when studying the effects of the monoterpenoid citral.
A different asymmetric effect is shown in Figure 1D. In this case, greater fluorescence emission is localized at the edges of the older leaves, while the vascular bundles and the youngest leaves in the rosette remain intact. Different studies have indicated that this pattern could be reminiscent of early senescence as suggested by the images of fluorescence distribution in Arabidopsis plants treated with citral (Graña et al., 2013b) or with the secondary metabolite o-coumaric acid (Zheng et al., 2012).
Figure 1E shows an unusual distribution of reduced maximum PSII efficiency, with lower values in younger than in older leaves. This scenario could be the case of a natural product inducing a strong effect on target sites directly related to the photosynthetic apparatus. This effect could result in a stronger damage on the fully synthesizing leaves, as that seen by Díaz-Tielas et al. (2017) in chalcone-treated seedlings, which induced early plasma membrane depolarization and dramatic effects on chloroplasts structure and function.
Finally, Figure 1F shows an image where heterogeneous damage appears indistinctly for young or old leaves. Arrows point out damaged spots in the different leaves. As indicated by orange and red color, some parts of the leaves remain intact while others are extremely damaged. This effect has been previously related to oxidative stress and necrotic process in Arabidopsis plants treated with different natural compounds (Martínez-Peñalver et al., 2011; Sánchez-Moreiras et al., 2011). In this way, Araniti et al. (2017b) demonstrated overlapping of ROS accumulation in the leaf and the area characterized by Fv/Fm reduction when lettuce was treated with volatiles released by Dittrichia viscosa. Moreover, this isolated inhibition spots (increased fluorescence emission) could be also found when the studied natural compound is sprayed to the leaves and behaves as a PSII inhibitor directly binding to the D protein and interrupting the electron transfer chain of PSII re-emitting the excitation energy as fluorescence, which will result in an easily discernable decrease of Fv/Fm values, as has been previously found for synthetic herbicides (Muller et al., 2008; Wang et al., 2018). This decrease will happen first in the areas of contact of the sprayed compound with the leaf, resulting in isolated spots of increased fluorescence emission.
As reviewed at the beginning of this paper, and analyzed in the physiological interpretations of the images obtained, imaging fluorescence analyses of the whole affected plant provides accurate and fundamental information for the interpretation of plant response to stress not provided by single-point techniques or analyses of photosynthetic parameters independently analyzed of their spatial distribution in the plant. Monitoring imaging fluorescence can help to understand how damage evolves and how response is orchestrated into the plants.
Because of the lack of information and the restricted use of this technique in the field of natural-herbicide discovery, research efforts should be focused on creating a common procedure to study the mode of action of natural compounds, without avoiding protocol innovations. The use of a model sensitive species, such as A. thaliana, could allow the standardization of the method, which could be used worldwide allowing the reproducibility of the data. This could allow the creation of a database of the signatures of the CF and the trend of the various parameters in response to the treatments. In addition, this technique could be further used as a sensitive tool to identify the effective doses (stimulatory and inhibitory) of the natural compounds as alternative or support to the classical dose-responses curve.
AS-M: idea and writing and editing. FA: writing and reviewing. EG: image acquisition and editing. MR: idea and reviewing. All authors contributed to the article and approved the submitted version.
This work was supported by the Spanish Ministry of Science, Innovation and Universities (project number: RTI2018-094716-B-I00).
The authors declare that the research was conducted in the absence of any commercial or financial relationships that could be construed as a potential conflict of interest.
Araniti, F., Bruno, L., Sunseri, F., Pacenza, M., Forgione, I., Bitonti, M. B., et al. (2017a). The allelochemical farnesene affects Arabidopsis thaliana root meristem altering auxin distribution. Plant Physiol. Biochem. 121, 14–20. doi: 10.1016/j.plaphy.2017.10.005
Araniti, F., Landi, M., Lupini, A., Sunseri, F., Guidi, L., and Abenavoli, M. R. (2018a). Origanum vulgare essential oils inhibit glutamate and aspartate metabolism altering the photorespiratory pathway in Arabidopsis thaliana seedlings. J. Plant Physiol. 231, 297–309. doi: 10.1016/j.jplph.2018.10.006
Araniti, F., Lupini, A., Mauceri, A., Zumbo, A., Sunseri, F., and Abenavoli, M. R. (2018b). The allelochemical trans-cinnamic acid stimulates salicylic acid production and galactose pathway in maize leaves: A potential mechanism of stress tolerance. Plant Physiol. Biochem. 128, 32–40. doi: 10.1016/j.plaphy.2018.05.006
Araniti, F., Lupini, A., Sunseri, F., and Abenavoli, M. R. (2017b). Allelopathic potential of Dittrichia viscosa (L.) W. Greuter mediated by VOCs: a physiological and metabolomic approach. PloS One 12:e0170161. doi: 10.1371/journal.pone.0170161
Araniti, F., Sánchez-Moreiras, A. M., Graña, E., Reigosa, M. J., and Abenavoli, M. R. (2017c). Terpenoid trans-caryophyllene inhibits weed germination and induces plant water status alteration and oxidative damage in adult Arabidopsis. Plant Biol. 19, 79–89. doi: 10.1111/plb.12471
Araniti, F., Scognamiglio, M., Chambery, A., Russo, R., Esposito, A., D’abrosca, B., et al. (2017d). Highlighting the effects of coumarin on adult plants of Arabidopsis thaliana (L.) Heynh. by an integrated-omic approach. J. Plant Physiol. 213, 30–41. doi: 10.1016/j.jplph.2017.02.013
Atherton, J., MacArthur, A., Hakala, T., Maseyk, K., Robinson, I., Liu, W., et al. (2018). “Drone measurements of solar-induced chlorophyll fluorescence acquired with a low-weight DFOV spectrometer system,” in IGARSS 2018-2018 IEEE International Geoscience and Remote Sensing Symposium, (Piscataway: IEEE), 8834–8836.
Awlia, M., Nigro, A., Fajkus, J., Schmoeckel, S. M., Negrão, S., Santelia, D., et al. (2016). High-throughput non-destructive phenotyping of traits that contribute to salinity tolerance in Arabidopsis thaliana. Front. Plant Sci. 7:1414. doi: 10.3389/fpls.2016.01414
Ayalon, I., de Barros Marangoni, L. F., Benichou, J. I., Avisar, D., and Levy, O. (2019). Red Sea corals under Artificial Light Pollution at Night (ALAN) undergo oxidative stress and photosynthetic impairment. Global Change Biol. 25, 4194–4207. doi: 10.1111/gcb.14795
Baker, N. R. (2008). Chlorophyll fluorescence: a probe of photosynthesis in vivo. Ann. Rev. Plant Biol. 59, 89–113. doi: 10.1146/annurev.arplant.59.032607.092759
Baker, N. R., and Rosenqvist, E. (2004). Applications of chlorophyll fluorescence can improve crop production strategies: an examination of future possibilities. J. Exp. Bot. 55, 1607–1621. doi: 10.1093/jxb/erh196
Barbagallo, R. P., Oxborough, K., Pallett, K. E., and Baker, N. R. (2003). Rapid noninvasive screening for perturbations of metabolism and plant growth using chlorophyll fluorescence imaging. Plant Physiol. 132, 485–493. doi: 10.1104/pp.102.018093
Bauriegel, E., Giebel, A., Geyer, M., Schmidt, U., and Herppich, W. B. (2011). Early detection of Fusarium infection in wheat using hyper-spectral imaging. Comput. Electron. Agr. 75, 304–312. doi: 10.1016/j.compag.2010.12.006
Belz, R. G., and Duke, S. O. (2017). “Herbicide-mediated hormesis,” in Pesticide Dose: Effects on the Environment and Target and Non-target Organisms, eds S. O. Duke, P. Kudsk, and K. Solomon (Washington, DC: American Chemical Society), 135–148. doi: 10.1021/bk-2017-1249.ch010
Beninger, C. W., Abou-Zaid, M. M., Kistner, A. L., Hallett, R. H., Iqbal, M. J., Grodzinski, B., et al. (2004). A flavanone and two phenolic acids from Chrysanthemum morifolium with phytotoxic and insect growth regulating activity. J. Chem. Ecol. 30, 589–606. doi: 10.1023/b:joec.0000018631.67394.e5
Beyschlag, W., and Eckstein, J. (2001). Towards a causal analysis of stomatal patchiness: the role of stomatal size variability and hydrological heterogeneity. Acta Oecol. 22, 161–173. doi: 10.1016/s1146-609x(01)01110-9
Bilger, W., and Schreiber, U. (1987). ““Energy-dependent quenching of dark-level chlorophyll fluorescence in intact leaves”,” in Excitation Energy and Electron Transfer in Photosynthesis, eds J. Govindjee, W. A. Barber, J. H. C. Cramer, J. Goedheer, R. Lavorel, and B. A. Zilinskas (Netherlands: Springer), 157–162. doi: 10.1007/978-94-009-3527-3_19
Björkman, O., and Demmig-Adams, B. (1995). “Regulation of photosynthetic light energy capture, conversion, and dissipation in leaves of higher plants,” in Ecophysiology of Photosynthesis, eds E. D. Schulze and M. M. Caldwell (Heidelberg: Springer), 17–47. doi: 10.1007/978-3-642-79354-7_2
Bolhar-Nordenkampf, H. R., Long, S. P., Baker, N. R., Oquist, G., Schreiber, U., and Lechner, E. G. (1989). Chlorophyll fluorescence as a probe of the photosynthetic competence of leaves in the field: a review of current instrumentation. Funct. Ecol. 1989, 497–514. doi: 10.2307/2389624
Brewster, D. (1834). On the colours of natural bodies. Roy. Soc. Edinb. Trans. 12, 538–545. doi: 10.1017/s0080456800031203
Buschmann, C., Langsdorf, G., and Lichtenthaler, H. K. (2000). Imaging of the blue, green, and red fluorescence emission of plants: an overview. Photosynthetica 38, 483–491. doi: 10.1023/a:1012440903014
Chaerle, L., and van der Straeten, D. (2000). Imaging techniques and the early detection of plant stress. Trends Plant Sci. 5, 495–501. doi: 10.1016/s1360-1385(00)01781-7
Chaerle, L., and Van Der Straeten, D. (2001). Seeing is believing: imaging techniques to monitor plant health. Biochim. Biophys. Acta 1519, 153–166. doi: 10.1016/s0167-4781(01)00238-x
Chaerle, L., Leinonen, I., Jones, H. G., and Van Der Straeten, D. (2007). Monitoring and screening plant populations with combined thermal and chlorophyll fluorescence imaging. J. Exp. Bot. 58, 773–784. doi: 10.1093/jxb/erl257
Chaerle, L., Lenk, S., Leinonen, I., Jones, H. G., Van Der Straeten, D., and Buschmann, C. (2009). Multi-sensor plant imaging: towards the development of a stress catalogue. Biotechnol. J. 4, 1152–1167. doi: 10.1002/biot.200800242
Daley, P. F., Raschke, K., Ball, J. T., and Berry, J. A. (1989). Topography of photosynthetic activity of leaves obtained from video images of chlorophyll fluorescence. Plant Physiol. 90, 1233–1238. doi: 10.1104/pp.90.4.1233
Dayan, F. E., and Zaccaro, M. L. M. (2012). Chlorophyll fluorescence as a marker for herbicide mechanisms of action. Pest. Biochem. Physiol. 102, 189–197. doi: 10.1016/j.pestbp.2012.01.005
de Carvalho, A. C., Salvador, J. P., Pereira, T., de, M., Ferreira, P. H. A., Lira, J. C. S., et al. (2016). Fluorescence of chlorophyll a for discovering inhibitors of photosynthesis in plant extracts. Am. J. Plant Sci. 7, 1545–1554. doi: 10.4236/ajps.2016.711146
Demmig-Adams, B., Adams, W. W., Heber, U., Neimanis, S., Winter, K., Krüger, A., et al. (1990). Inhibition of zeaxanthin formation and of rapid changes in radiationless energy dissipation by dithiothreitol in spinach leaves and chloroplasts. Plant Physiol. 92, 293–301. doi: 10.1104/pp.92.2.293
Desotgiu, R., Pollastrini, M., Cascio, C., Gerosa, G., Marzuoli, R., and Bussotti, F. (2012). Chlorophyll a fluorescence analysis along a vertical gradient of the crown in a poplar (Oxford clone) subjected to ozone and water stress. Tree Physiol. 32, 976–986. doi: 10.1093/treephys/tps062
Díaz-Tielas, C., Graña, E., Maffei, M., Reigosa, M. J., and Sánchez-Moreiras, A. M. (2017). Plasma membrane depolarization precedes photosynthesis damage and long-term leaf bleaching in (E)-chalcone-treated Arabidopsis shoots. J. Plant Physiol. 218, 56–65. doi: 10.1016/j.jplph.2017.07.014
Díaz-Tielas, C., Sotelo, T., Graña, E., Reigosa, M. J., and Sánchez-Moreiras, A. M. (2014). Phytotoxic potential of trans-chalcone on crop plants and model species. J. Plant Growth Regul. 33, 181–194. doi: 10.1007/s00344-013-9360-6
Digrado, A., Bachy, A., Mozaffar, A., Schoon, N., Bussotti, F., Amelynck, C., et al. (2017). Long-term measurements of chlorophyll a fluorescence using the JIP-test show that combined abiotic stresses influence the photosynthetic performance of the perennial ryegrass (Lolium perenne) in a managed temperate grassland. Physiol. Plant 161, 355–371. doi: 10.1111/ppl.12594
Dong, Z., Men, Y., Liu, Z., Li, J., and Ji, J. (2020). Application of chlorophyll fluorescence imaging technique in analysis and detection of chilling injury of tomato seedlings. Comput. Electron. Agr. 168:105109. doi: 10.1016/j.compag.2019.105109
Duarte, C. I., Martinazzo, E. G., Bacarin, M. A., and Colares, I. G. (2020). Seed germination, growth and chlorophyll a fluorescence in young plants of Allophylus edulis in different periods of flooding. Acta Physiol. Plant 42, 1–11. doi: 10.9734/ajea/2016/20467
Duke, S. O. (2011). Why have no new herbicide modes of action appeared in recent years? Pest. Manag. Sci. 68, 505–512. doi: 10.1002/ps.2333
Duke, S. O., Dayan, F. E., and Rimando, A. M. (2000a). “Natural products and herbicide discovery,” in Herbicides and their Mechanisms of Action, eds A. H. Cobb and R. C. Kirkwood (Sheffield: Academic Press), 105–133.
Duke, S. O., Dayan, F. E., Romagni, J. G., and Rimando, A. M. (2000b). Natural products as sources of herbicides: current status and future trends. Weed Res. 40, 99–111. doi: 10.1046/j.1365-3180.2000.00161.x
Duysens, L. N. M., and Sweers, H. E. (1963). “Mechanism of two photochemical reactions in algae as studied by means of fluorescence,” in Studies on Microalgae and Photosynthetic Bacteria. A Collection of Papers, ed. Japanese Society of Plant Physiologists (Tokyo: University of Tokyo Press), 353–372.
Endo, T., Schreiber, U., and Asada, K. (1995). Suppression of quantum yield of photosystem II by hyperosmotic stress in Chlamydomonas reinhardtii. Plant Cell Physiol. 36, 1253–1258.
Fenton, J. M., and Crofts, A. R. (1990). Computer aided fluorescence imaging of photosynthetic systems - Application of video imaging to the study of fluorescence induction in green plants and photosynthetic bacteria. Photosyn. Res. 26, 59–66. doi: 10.1007/BF00048977
Gao, Y., Liu, W., Wang, X., Yang, L., Han, S., Chen, S., et al. (2018). Comparative phytotoxicity of usnic acid, salicylic acid, cinnamic acid and benzoic acid on photosynthetic apparatus of Chlamydomonas reinhardtii. Plant Physiol. Biochem. 128, 1–12. doi: 10.1016/j.plaphy.2018.04.037
Genty, B., and Meyer, S. (1995). Quantitative mapping of leaf photosynthesis using chlorophyll fluorescence imaging. Funct. Plant Biol. 22, 277–284. doi: 10.1071/pp9950277
Genty, B., Briantais, J. M., and Baker, N. R. (1989). The relationship between quantum yield of photosynthetic electron transport and quenching of chlorophyll fluorescence. Biochim. Biophys. Acta 990, 87–92. doi: 10.1016/s0304-4165(89)80016-9
Gonzáles, W. L., Suárez, L. H., Molina-Montenegro, M. A., and Gianoli, E. (2008). Water availability limits tolerance of apical damage in the Chilean tarweed Madia sativa. Acta Oecol. 34, 104–110. doi: 10.1016/j.actao.2008.04.004
Gorbe, E., and Calatayud, A. (2012). Applications of chlorophyll fluorescence imaging technique in horticultural research: a review. Sci. Hort. 138, 24–35. doi: 10.1016/j.scienta.2012.02.002
Govindjee, E. (1995). 63 Years since Kautsky-chlorophyll-a fluorescence. Aust. J. Plant Physiol. 22, 131–160. doi: 10.1071/pp9950131
Govindjee, G. (2004). ““Chlorophyll a fluorescence: a bit of basics and history”,” in Chlorophyll a Fluorescence: a Signature of Photosynthesis, eds G. C. Papageorgiou, and Govindjee (Dordrecht, NL: Springer), 1–41. doi: 10.1007/978-1-4020-3218-9_1
Graña, E., Díaz-Tielas, C., López-González, D., Martínez-Peñalver, A., Reigosa, M. J., and Sánchez-Moreiras, A. M. (2016). The plant secondary metabolite citral alters water status and avoids seed formation in Arabidopsis thaliana plants. Plant Biol. 18, 423–432. doi: 10.1111/plb.12418
Graña, E., Sotelo, T., Díaz-Tielas, C., Araniti, F., Krasuska, U., Bogatek, R., et al. (2013a). Citral induces auxin and ethylene-mediated malformations and arrests cell division in Arabidopsis thaliana roots. J. Chem. Ecol. 39, 271–282. doi: 10.1007/s10886-013-0250-y
Graña, E., Sotelo, T., Díaz-Tielas, C., Reigosa, M. J., and Sánchez-Moreiras, A. M. (2013b). The phytotoxic potential of the terpenoid citral on seedlings and adult plants. Weed Sci. 61, 469–481. doi: 10.1614/ws-d-12-00159.1
Guidi, L., Landi, M., Penella, C., and Calatayud, A. (2016). Application of modulated chlorophyll fluorescence and modulated chlorophyll fluorescence imaging in studying environmental stresses effect. Ann. Bot. 6, 5–22.
Guidi, L., Lo Piccolo, E., and Landi, M. (2019). Chlorophyll fluorescence, photoinhibition and abiotic stress: does it make any difference the fact to be a C3 or C4 species? Front. Plant Sci. 10:174. doi: 10.3389/fpls.2019.00174
Guo, Y., Cheng, J., Shi, J., Gao, Y., Wang, H., Yin, C., et al. (2019). Novel action targets of natural product gliotoxin in photosynthetic apparatus. Front. Plant Sci. 10:1688. doi: 10.3389/fpls.2019.01688
Häder, D. P., Lebert, M., Figueroa, F. L., Jiménez, C., Viñegla, B., and Perez-Rodriguez, E. (1998). Photoinhibition in Mediterranean macroalgae by solar radiation measured on site by PAM fluorescence. Aquat. Bot. 61, 225–236. doi: 10.1016/s0304-3770(98)00068-0
Havaux, M., Strasser, R. J., and Greppin, H. (1991). A theoretical and experimental analysis of the qP and qN coefficients of chlorophyll fluorescence quenching and their relation to photochemical and nonphotochemical events. Photosyn. Res. 27, 41–55. doi: 10.1007/bf00029975
Hermans, C., Smeyers, M., Rodriguez, R. M., Eyletters, M., Strasser, R. J., and Delhaye, J. P. (2003). Quality assessment of urban trees: a comparative study of physiological characterization, airborne imaging and on site fluorescence monitoring by the OJIP-test. J. Plant Physiol. 160, 81–90. doi: 10.1078/0176-1617-00917
Horton, P., and Hague, A. (1988). Studies on the induction of chlorophyll fluorescence in isolated barley protoplasts. IV. Resolution of non-photochemical quenching. Biochim. Biophys. Acta Bioenerg. 932, 107–115. doi: 10.1016/0005-2728(88)90144-2
Humplík, J. F., Lazár, D., Husičková, A., and Spíchal, L. (2015). Automated phenotyping of plant shoots using imaging methods for analysis of plant stress responses–a review. Plant Meth. 11:29.
Hussain, M. I., and Reigosa, M. J. (2016). Plant secondary metabolite rutin affects the photosynthesis and excitation energy flux responses in Arabidopsis thaliana. Allelopath. J. 38, 215–228.
Jouini, A., Verdeguer, M., Pinton, S., Araniti, F., Palazzolo, E., Badalucco, L., et al. (2020). Potential effects of essential oils extracted from Mediterranean aromatic plants on target weeds and soil microorganisms. Plants 9:1289. doi: 10.3390/plants9101289
Kalaji, H. M., Schansker, G., Brestic, M., Bussotti, F., Calatayud, A., Ferroni, L., et al. (2017). Frequently asked questions about chlorophyll fluorescence, the sequel. Photosyn. Res. 132, 13–66.
Kalaji, H. M., Schansker, G., Ladle, R. J., Goltsev, V., Bosa, K., Allakhverdiev, S. I., et al. (2014). Frequently asked questions about in vivo chlorophyll fluorescence: practical issues. Photosyn. Res. 122, 121–158.
Kalaji, H. M., Schansker, G., Ladle, R. J., Goltsev, V., Bosa, K., Allakhverdiev, S. I., et al. (2014). Frequently asked questions about in vivo chlorophyll fluorescence: practical issues. Photosyn. Res. 122, 121–158.
Kautsky, H., and Hirsch, A. (1931). Neue versuche zur kohlensaureassimilation. Naturwissenschaften 48:964.
Klughammer, C., and Schreiber, U. (2008). Complementary PS II quantum yields calculated from simple fluorescence parameters measured by PAM fluorometry and the Saturation Pulse method. PAM Appl. Notes 1, 27–35.
Kramer, D. M., Johnson, G., Kiirats, O., and Edwards, G. E. (2004). New fluorescence parameters for the determination of QA redox state and excitation energy fluxes. Photosyn. Res. 79, 209–218. doi: 10.1023/b:pres.0000015391.99477.0d
Krause, G. H., and Weis, E. (1984). Chlorophyll fluorescence as a tool in plant physiology. Photosyn. Res. 5, 139–157. doi: 10.1007/bf00028527
Krause, G. H., and Weis, E. (1991). Chlorophyll fluorescence and photosynthesis: the basics. Annu. Rev. Plant Biol. 42, 313–349.
Kriegs, B., Jansen, M., Hahn, K., Peisker, H., Šamajová, O., Beck, M., et al. (2010). Cyclic monoterpene mediated modulations of Arabidopsis thaliana phenotype: effects on the cytoskeleton and on the expression of selected genes. Plant Signal. Behav. 5, 832–838. doi: 10.4161/psb.5.7.12032
Kurepin, L. V., Stangl, Z. R., Ivanov, A. G., Bui, V., Mema, M., Hüner, N. P., et al. (2018). Contrasting acclimation abilities of two dominant boreal conifers to elevated CO2 and temperature. Plant Cell Environ. 41, 1331–1345. doi: 10.1111/pce.13158
Laasch, H., Urbach, W., and Schreiber, U. (1983). Binary flash-induced oscillations of [14C]DCMU binding to the Photosystem II acceptor complex. FEBS Lett. 159, 275–279. doi: 10.1016/0014-5793(83)80463-3
Lang, M., Lichtenthaler, H. K., Sowinska, M., Summ, P., and Heisel, F. (1994). Blue, green and red fluorescence signatures and images of tobacco leaves. Bot. Acta 107, 230–236. doi: 10.1111/j.1438-8677.1994.tb00790.x
Lawson, T. (2009). Guard cell photosynthesis and stomatal function. New Phytol. 181, 13–34. doi: 10.1111/j.1469-8137.2008.02685.x
Lawson, T., and Morison, J. (2006). Visualizing patterns of CO2 diffusion in leaves. New Phytol. 169, 641–643. doi: 10.1111/j.1469-8137.2006.01655.x
Lawson, T., Oxborough, K., Morison, J. I., and Baker, N. R. (2002). Responses of photosynthetic electron transport in stomatal guard cells and mesophyll cells in intact leaves to light, CO2, and humidity. Plant Physiol. 128, 52–62. doi: 10.1104/pp.010317
Lazár, D. (2003). Chlorophyll a fluorescence rise induced by high light illumination of dark-adapted plant tissue studied by means of a model of photosystem II and considering photosystem II heterogeneity. J. Theor. Biol. 220, 469–503. doi: 10.1006/jtbi.2003.3140
Lee, H. Y., Mang, H., Seo, Y. E., Oh, S., Kim, S. B., Park, E., et al. (2019). Genome-wide expression analysis of immune receptors in hot pepper reveals an autonomous NLR cluster in higher plants. BioRxiv 2019:878959.
Levin, R. A., Suggett, D. J., Nitschke, M. R., van Oppen, M. J., and Steinberg, P. D. (2017). Expanding the Symbiodinium (Dinophyceae, Suessiales) toolkit through protoplast technology. J. Eukaryotic Microbiol. 64, 588–597. doi: 10.1111/jeu.12393
Lichtenthaler, H. K. (1996). Vegetation stress: an introduction to the stress concept in plants. J. Plant Physiol. 148, 4–14. doi: 10.1016/s0176-1617(96)80287-2
Lichtenthaler, H. K., and Babani, F. (2000). Detection of photosynthetic activity and water stress by imaging the red chlorophyll fluorescence. Plant Physiol. Biochem. 38, 889–895. doi: 10.1016/s0981-9428(00)01199-2
Lichtenthaler, H. K., and Miehé, J. A. (1997). Fluorescence imaging as a diagnostic tool for plant stress. Trends Plant Sci. 2, 316–320. doi: 10.1016/s1360-1385(97)89954-2
Lichtenthaler, H. K., Langsdorf, G., Lenk, S., and Buschmann, C. (2005). Chlorophyll fluorescence imaging of photosynthetic activity with the flash-lamp fluorescence imaging system. Photosynthetica 43, 355–369. doi: 10.1007/s11099-005-0060-8
Liebman, M. (2001). “Weed management: a need for ecological approaches,” in Ecological Management of Agricultural Weeds, eds M. Liebman, ChL Mohler, and ChP Staver (Cambridge: Cambridge University Press), 544.
Lopes, M., Nogués, S., and Molero, G. (2012). “Gas exchange and chlorophyll fluorescence-Principles and applications,” in Physiological Breeding I: Interdisciplinary Approaches to improve Crop Adaptation, eds M. P. Reynolds, A. J. D. Pask, and D. M. Mullan (Mexico: CIMMYT), 188.
López-González, D., Ledo, D., Cabeiras-Freijanes, L., Verdeguer, M., Reigosa, M. J., and Sánchez-Moreiras, A. M. (2020). Phytotoxic activity of the natural compound norharmane on crops, weeds and model plants. Plants 9:1328. doi: 10.3390/plants9101328
Martínez-Peñalver, A., Pedrol, N., Reigosa, M. J., and Sánchez-Moreiras, A. M. (2012). Tolerance of Arabidopsis thaliana to the allelochemical protocatechualdehyde. J. Plant Growth Regul. 31, 406–415. doi: 10.1007/s00344-011-9250-8
Martínez-Peñalver, A., Reigosa, M. J., and Sánchez-Moreiras, A. M. (2011). Imaging chlorophyll a fluorescence reveals specific spatial distributions under different stress conditions. Flora 206, 836–844. doi: 10.1016/j.flora.2011.02.004
Maxwell, K., and Johnson, G. N. (2000). Chlorophyll fluorescence—a practical guide. J. Exp. Bot. 51, 659–668. doi: 10.1093/jxb/51.345.659
McAlister, E. D., and Myers, J. (1940). Time course of photosynthesis and fluorescence. Science 92, 241–243. doi: 10.1126/science.92.2385.241
McAusland, L., Atkinson, J. A., Lawson, T., and Murchie, E. H. (2019). High throughput procedure utilising chlorophyll fluorescence imaging to phenotype dynamic photosynthesis and photoprotection in leaves under controlled gaseous conditions. Plant Meth. 15:109.
Meyer, S., and Genty, B. (1999). Heterogenous inhibition of photosynthesis over the leaf surface of Rosa rubiginosa L. during water stress and abscisic acid treatment: induction of a metabolic component by limitation of CO2 diffusion. Planta 210, 126–131. doi: 10.1007/s004250050661
Morison, J. I., Gallouët, E., Lawson, T., Cornic, G., Herbin, R., and Baker, N. R. (2005). Lateral diffusion of CO2 in leaves is not sufficient to support photosynthesis. Plant Physiol. 139, 254–266. doi: 10.1104/pp.105.062950
Morison, J. I., Lawson, T., and Cornic, G. (2007). Lateral CO2 diffusion inside dicotyledonous leaves can be substantial: quantification in different light intensities. Plant Physiol. 145, 680–690. doi: 10.1104/pp.107.107318
Müller, N. J. C. (1874). Beziehungen zwischen assimilation, absorption und fluoreszenz im chlorophyll des lebenden blattes. Jahrb. Wiss. Bot. 9, 42–49.
Muller, R., Schreiber, U., Escher, B. I., Quayle, P., Nash, S. M. B., and Mueller, J. F. (2008). Rapid exposure assessment of PSII herbicides in surface water using a novel chlorophyll a fluorescence imaging assay. Sci. Total Environ. 401, 51–59. doi: 10.1016/j.scitotenv.2008.02.062
Murchie, E. H., and Lawson, T. (2013). Chlorophyll fluorescence analysis: a guide to good practice and understanding some new applications. J Exp. Bot. 64, 3983–3998. doi: 10.1093/jxb/ert208
Nedbal, L., and Whitmarsh, J. (2004). “Chlorophyll fluorescence imaging of leaves and fruits,” in Chlorophyll a Fluorescence: A Signature of Photosynthesis, eds G. C. Papageorgiou, and Govindjee (Dordrecht, NL: Springer), 389. doi: 10.1007/978-1-4020-3218-9_14
Oguchi, R., Terashima, I., and Chow, W. S. (2009). The involvement of dual mechanisms of photoinactivation of Photosystem II in Capsicum annuum L. plants. Plant Cell Physiol. 50, 1815–1825. doi: 10.1093/pcp/pcp123
Oguchi, R., Terashima, I., Kou, J., and Chow, W. S. (2011). Operation of dual mechanisms that both lead to photoinactivation of Photosystem II in leaves by visible light. Physiol. Plant. 142, 47–55. doi: 10.1111/j.1399-3054.2011.01452.x
Omasa, K., Shimazaki, K., Aiga, I., Larcher, W., and Onoe, M. (1987). Image analysis of chlorophyll fluorescence transients for diagnosing the photosynthetic system of attached leaves. Plant Physiol. 84, 748–752. doi: 10.1104/pp.84.3.748
Oxborough, K. (2004). “Using chlorophyll a fluorescence imaging tomonitor photosynthetic performance,” in Chlorophyll a Fluorescence: A Signature of Photosynthesis, eds G. C. Papageorgiou, and Govindjee (Dordrecht, NL: Springer), 409. doi: 10.1007/978-1-4020-3218-9_15
Oxborough, K., and Baker, N. R. (1997). An instrument capable of imaging chlorophyll a fluorescence from intact leaves at very low irradiance and at cellular and subcellular levels of organization. Plant Cell Environ. 20, 1473–1483. doi: 10.1046/j.1365-3040.1997.d01-42.x
Papageorgiou, G. C., and Govindjee (eds) (2004). Chlorophyll a Fluorescence: A Signature of Photosynthesis. Dordrecht, NL: Springer.
Papathanasiou, V., Kariofillidou, G., Malea, P., and Orfanidis, S. (2020). Effects of air exposure on desiccation and photosynthetic performance of Cymodocea nodosa with and without epiphytes and Ulva rigida in comparison, under laboratory conditions. Mar. Environ. Res 158:104948. doi: 10.1016/j.marenvres.2020.104948
Paul, M. J., and Foyer, C. H. (2001). Sink regulation of photosynthesis. J. Exp. Bot. 52, 1383–1400. doi: 10.1093/jexbot/52.360.1383
Pérez-Bueno, M. L., Pineda, M., and Barón Ayala, M. (2019). Phenotyping plant responses to biotic stress by chlorophyll fluorescence imaging. Front. Plant Sci. 10:1135. doi: 10.3389/fpls.2019.01135
Ping, M. A., Tuan-Hui, B. A. I., and Feng-Wang, M. A. (2015). Effects of progressive drought on photosynthesis and partitioning of absorbed light in apple trees. J. Integr. Agric. 14, 681–690. doi: 10.1016/s2095-3119(14)60871-6
Roháček, K., Soukupová, J., and Barták, M. (2008). “Chlorophyll fluorescence: a wonderful tool to study plant physiology and plant stress,” in Plant Cell Compartments-Selected Topics, ed. B. Schoefs (Kerala: Research Signpost), 41–104.
Rolfe, S. A., and Scholes, J. D. (1995). Quantitative imaging of chlorophyll fluorescence. New Phytol. 131, 69–79. doi: 10.1111/j.1469-8137.1995.tb03056.x
Ruban, A. V., and Murchie, E. H. (2012). Assessing the photoprotective effectiveness of non-photochemical chlorophyll fluorescence quenching: a new approach. Biochim. Biophys. Acta Bioenerg. 1817, 977–982. doi: 10.1016/j.bbabio.2012.03.026
Rühle, T., Reiter, B., and Leister, D. (2018). Chlorophyll fluorescence video imaging: A versatile tool for identifying factors related to photosynthesis. Front. Plant Sci. 9:55. doi: 10.3389/fpls.2018.00055
Sánchez-Moreiras, A. M., and Reigosa, M. J. (2005). Whole plant response of lettuce after root exposure to BOA (2(3H)-benzoxazolinone). J. Chem. Ecol. 31, 2689–2703. doi: 10.1007/s10886-005-7620-z
Sánchez-Moreiras, A. M., Graña, E., Díaz-Tielas, C., López-González, D., Araniti, F., Celeiro, M., et al. (2018). “Elucidating the phytotoxic potential of natural compounds,” in Advances in Plant Ecophysiology Techniques, eds A. M. Sánchez-Moreiras and M. J. Reigosa (New York, NY: Springer), 419–438.
Sánchez-Moreiras, A. M., Martínez-Peñalver, A., and Reigosa, M. J. (2011). Early senescence induced by 2-3H-benzoxazolinone (BOA) in Arabidopsis thaliana. J. Plant Physiol. 168, 863–870. doi: 10.1016/j.jplph.2010.11.011
Scholes, J. D., and Rolfe, S. A. (1996). Photosynthesis in localised regions of oat leaves infected with crown rust (Puccinia coronata): quantitative imaging of chlorophyll fluorescence. Planta 199, 573–582.
Schreiber, U. (1983). Chlorophyll fluorescence yield changes as a tool in plant physiology I. The measuring system. Photosyn. Res. 4, 361–373. doi: 10.1007/bf00054144
Schreiber, U. (2004). “Pulse-amplitude-modulation (PAM) fluorometry and saturation pulse method: an overview,” in Chlorophyll a Fluorescence. Advances in Photosynthesis and Respiration, eds G. C. Papageorgiou, and Govindjee (Dordrecht, NL: Springer), 279–319. doi: 10.1007/978-1-4020-3218-9_11
Schreiber, U., and Bilger, W. (1993). “Progress in chlorophyll fluorescence research: major developments during the past years in retrospect,” in Progress in Botany / Fortschritte der Botanik, eds H. D. Behnke, U. Lüttge, K. Esser, J. W. Kadereit, and M. Runge (Heidelberg: Springer), 151–173. doi: 10.1007/978-3-642-78020-2_8
Schreiber, U., and Neubauer, C. (1987). The polyphasic rise of chlorophyll fluorescence upon onset of strong continuous illumination: II. Partial control by the photosystem II donor side and possible ways of interpretation. Z. Naturforsch. C 42, 1255–1264. doi: 10.1515/znc-1987-11-1218
Schreiber, U., Bilger, W., and Neubauer, C. (1995). “Chlorophyll fluorescence as a nonintrusive indicator for rapid assessment of in vivo photosynthesis,” in Ecophysiology of Photosynthesis, eds E. D. Schulze and M. M. Caldwell (Heidelberg: Springer), 49–70. doi: 10.1007/978-3-642-79354-7_3
Schreiber, U., Bilger, W., Hormann, H., and Neubauer, C. (1998). “Chlorophyll fluorescence as a diagnostic tool: basics and some aspects of practical relevance,” in Photosynthesis: A Comprehensive Treatise, ed. A. S. Raghavendra (Cambridge: Cambridge University Press), 320–336.
Schreiber, U., Neubauer, C., and Schliwa, U. (1993). PAM fluorometer based on medium-frequency pulsed Xe-flash measuring light: a highly sensitive new tool in basic and applied photosynthesis research. Photosyn. Res. 36, 65–72. doi: 10.1007/bf00018076
Schreiber, U., Schliwa, U., and Bilger, W. (1986). Continuous recording of photochemical and non-photochemical chlorophyll fluorescence quenching with a new type of modulation fluorometer. Photosyn. Res. 10, 51–62. doi: 10.1007/bf00024185
Schulz, M., Kussmann, P., Knop, M., Kriegs, B., Gresens, F., Eichert, T., et al. (2007). Allelopathic monoterpenes interfere with Arabidopsis thaliana cuticular waxes and enhance transpiration. Plant Signal. Behav. 2, 231–239. doi: 10.4161/psb.2.4.4469
Siebke, K., and Weis, E. (1995a). Imaging of chlorophyll-a-fluorescence in leaves: topography of photosynthetic oscillations in leaves of Glechoma hederacea. Photosyn. Res. 45, 225–237. doi: 10.1007/bf00015563
Siebke, K., and Weis, E. (1995b). Assimilation images of leaves of Glechoma hederacea: analysis of non-synchronous stomata related oscillations. Planta 196, 155–165.
Skrzypek, E., Repka, P., Stachurska-Swakon, A., Barabasz-Krasny, B., and Mozdzen, K. (2015). Allelopathic effect of aqueous extracts from the leaves of peppermint (Mentha piperita L.) on selected physiological processes of common sunflower (Helianthus annuus L.). Notul. Bot. Hort. Agr. Cluj-Napoca 43, 335–342. doi: 10.15835/nbha43210034
Stokes, G. G. (1852). On the change of refrangibility of light. Philos. Trans. R. Soc. Lond. 142, 463–562. doi: 10.1098/rstl.1852.0022
Strasser, R. J., and Govindjee (1991). “The Fo and the O-J-I-P Fluorescence Rise in Higher Plants and Algae,” in Regulation of Chloroplast Biogenesis. Nato ASI Series (Series A: Life Sciences), ed. J. H. Argyroudi-Akoyunoglou (Boston, MA: Springer).
Synowiec, A., Możdżeń, K., Krajewska, A., Landi, M., and Araniti, F. (2019). Carum carvi L. essential oil: A promising candidate for botanical herbicide against Echinochloa crus-galli (L.) P. Beauv. in maize cultivation. Ind. Crops Prod. 140:111652. doi: 10.1016/j.indcrop.2019.111652
Tan, S., Smith, N. C., Padilla, K., and Ashley, E. (2018). Autonomous Ground Photographer. Washington: Washington University Open Scholarship.
Wang, P., Li, H., Jia, W., Chen, Y., and Gerhards, R. (2018). A fluorescence sensor capable of real-time herbicide effect monitoring in greenhouses and the field. Sensors 18:3771. doi: 10.3390/s18113771
Weber, J. F., Kunz, C., Peteinatos, G. G., Santel, H. J., and Gerhards, R. (2017). Utilization of chlorophyll fluorescence imaging technology to detect plant injury by herbicides in sugar beet and soybean. Weed Technol. 31, 523–535. doi: 10.1017/wet.2017.22
Weis, E., and Berry, J. A. (1987). Quantum efficiency of photosystem II in relation to ‘energy’-dependent quenching of chlorophyll fluorescence. Biochim. Biophys. Acta Bioenerg. 894, 198–208. doi: 10.1016/0005-2728(87)90190-3
Werner, C., Correia, O., and Beyschlag, W. (2002). Characteristic patterns of chronic and dynamic photoinhibition of different functional groups in a Mediterranean ecosystem. Funct. Plant Biol. 29, 999–1011. doi: 10.1071/pp01143
Wingler, A., Marčs, M., and Pourtau, N. (2004). Spatial patterns and metabolic regulation of photosynthetic parameters during leaf senescence. New Phytol. 161, 781–789. doi: 10.1111/j.1469-8137.2004.00996.x
Xiao, W., Wang, H., Liu, W., Wang, X., Guo, Y., Strasser, R., et al. (2020). Action of alamethicin in photosystem II probed by the fast chlorophyll fluorescence rise kinetics and the JIP-test. Photosynthetica 58, 358–368. doi: 10.32615/ps.2019.172
Zavafer, A., Cheah, M., Hillier, W., Chow, W. S., and Takahashi, S. (2015). Photodamage to the oxygen evolving complex of photosystem II by visible light. Sci. Rep. 5:16363. doi: 10.1038/srep16363
Zheng, G., Zhao, X., Zhang, F., Luo, S., Li, S., and Li, W. (2012). o-Coumaric acid from invasive Eupatorium adenophorum is a potent phytotoxin. Chemoecology 22, 131–138. doi: 10.1007/s00049-012-0105-y
Zhou, C., Mao, J., Zhao, H., Rao, Z., and Zhang, B. (2020). Monitoring and predicting Fusarium wilt disease in cucumbers based on quantitative analysis of kinetic imaging of chlorophyll fluorescence. Appl. Optics 59, 9118–9125. doi: 10.1364/ao.399320
Keywords: plant natural compounds, phytotoxicity, imaging fluorescence, photosynthesis, plant stress
Citation: Sánchez-Moreiras AM, Graña E, Reigosa MJ and Araniti F (2020) Imaging of Chlorophyll a Fluorescence in Natural Compound-Induced Stress Detection. Front. Plant Sci. 11:583590. doi: 10.3389/fpls.2020.583590
Received: 15 July 2020; Accepted: 19 November 2020;
Published: 21 December 2020.
Edited by:
Angeles Calatayud, Instituto Valenciano de Investigaciones Agrarias, SpainReviewed by:
Werner B. Herppich, Leibniz Institute for Agricultural Engineering and Bioeconomy (ATB), GermanyCopyright © 2020 Sánchez-Moreiras, Graña, Reigosa and Araniti. This is an open-access article distributed under the terms of the Creative Commons Attribution License (CC BY). The use, distribution or reproduction in other forums is permitted, provided the original author(s) and the copyright owner(s) are credited and that the original publication in this journal is cited, in accordance with accepted academic practice. No use, distribution or reproduction is permitted which does not comply with these terms.
*Correspondence: Adela M. Sánchez-Moreiras, YWRlbGFAdXZpZ28uZXM=
Disclaimer: All claims expressed in this article are solely those of the authors and do not necessarily represent those of their affiliated organizations, or those of the publisher, the editors and the reviewers. Any product that may be evaluated in this article or claim that may be made by its manufacturer is not guaranteed or endorsed by the publisher.
Research integrity at Frontiers
Learn more about the work of our research integrity team to safeguard the quality of each article we publish.