- 1Department of Biochemistry, University of Cambridge, Cambridge, United Kingdom
- 2School of Chemistry, University of St Andrews, St Andrews, United Kingdom
- 3Department of Natural Product Biosynthesis, Max Planck Institute for Chemical Ecology, Jena, Germany
Plant secondary metabolites have applications for the food, biofuel, and pharmaceutical industries. Recent advances in pathway elucidation and host expression systems now allow metabolic engineering of plant metabolic pathways to produce “new-to-nature” derivatives with novel biological activities, thereby amplifying the range of industrial uses for plant metabolites. Here we use a transient expression system in the model plant Nicotiana benthamiana to reconstitute the two-step plant-derived biosynthetic pathway for auxin (indole acetic acid) to achieve accumulation up to 500 ng/g fresh mass (FM). By expressing these plant-derived enzymes in combination with either bacterial halogenases and alternative substrates, we can produce both natural and new-to-nature halogenated auxin derivatives up to 990 ng/g FM. Proteins from the auxin synthesis pathway, tryptophan aminotransferases (TARs) and flavin-dependent monooxygenases (YUCs), could be transiently expressed in combination with four separate bacterial halogenases to generate halogenated auxin derivatives. Brominated auxin derivatives could also be observed after infiltration of the transfected N. benthamiana with potassium bromide and the halogenases. Finally, the production of additional auxin derivatives could also be achieved by co-infiltration of TAR and YUC genes with various tryptophan analogs. Given the emerging importance of transient expression in N. benthamiana for industrial scale protein and product expression, this work provides insight into the capacity of N. benthamiana to interface bacterial genes and synthetic substrates to produce novel halogenated metabolites.
Introduction
Many natural products that share a common scaffold have subtle modifications to the core structure that dramatically modulate the biological function of the molecule (Pickens et al., 2011). The chemical similarity between product families mirrors a homology in genes featuring in their biosynthetic pathways. Notably, the encoded enzymes among very similar pathways can have altered substrate and product specificities. Mixing and matching enzymes from analogous pathways from different organisms, could enhance our chances of success in creating new-to-nature products with novel biological functions.
Halogenation is a particularly important chemical modification. In fact, the presence of a halogen affects multiple physiochemical properties of molecules including lipophilicity, size, polarity, and capacity for hydrogen bonding (Jeschke, 2010), which in turn affects biological activity. Notably, many pharmaceuticals and agrochemicals contain halogens. For example, the potent anti-cancer agent salinosporamide A (marizomib) uses a chlorine atom as a stable leaving group, a crucial feature for irreversible inhibition of the proteasome in cancerous cells (Miller et al., 2011).
Halogenation in natural systems typically involves an enzymatic halogenation reaction (Neumann et al., 2008). For aromatic substrates, an FAD-dependent halogenase most commonly catalyzes the oxidation of a benign halide salt, readily available in vivo, to the corresponding hypohalous acid equivalent that is then installed in a regioselective manner on the aromatic substrate via an electrophilic aromatic substitution mechanism (Neumann et al., 2008; Figure 1). Among the most widely researched halogenases are the Trp-7 halogenases, PrnA (Keller et al., 2000) and RebH (Yeh et al., 2005), from Pseudomonas fluorescens and Saccharothrix aerocolonigenes, respectively, a Trp-6 halogenase, ThdH (Milbredt et al., 2014), from Streptomyces albogriseolus and a Trp-5 halogenase, PyrH (Zehner et al., 2005), from Streptomyces rugosporus. These enzymes have the unique ability to selectively halogenate the indole moiety of tryptophan under mild aqueous conditions. In contrast, synthetic halogenation of tryptophan and other aromatic compounds requires harsh reaction conditions and the reaction often lacks regioselectivity (Frese et al., 2014). Not surprisingly, there is substantial interest in using these flavin-dependent halogenases to replace or supplement current methods of chemically synthesizing halogenated compounds (Menon et al., 2016). Much effort has been made to expand the substrate scope and regioselectivity of these enzymes (Payne et al., 2013; Frese et al., 2014; Shepherd et al., 2016). Since these enzymes exhibit poor stability and low activity in vitro (Menon et al., 2016), in vivo systems are typically used for large-scale production of the halogenated metabolites. This approach has been used to produce antibiotics such as vancomycin (Jung et al., 2007) and griseofulvin (Saykhedkar and Singhal, 2004) in microbial host systems.
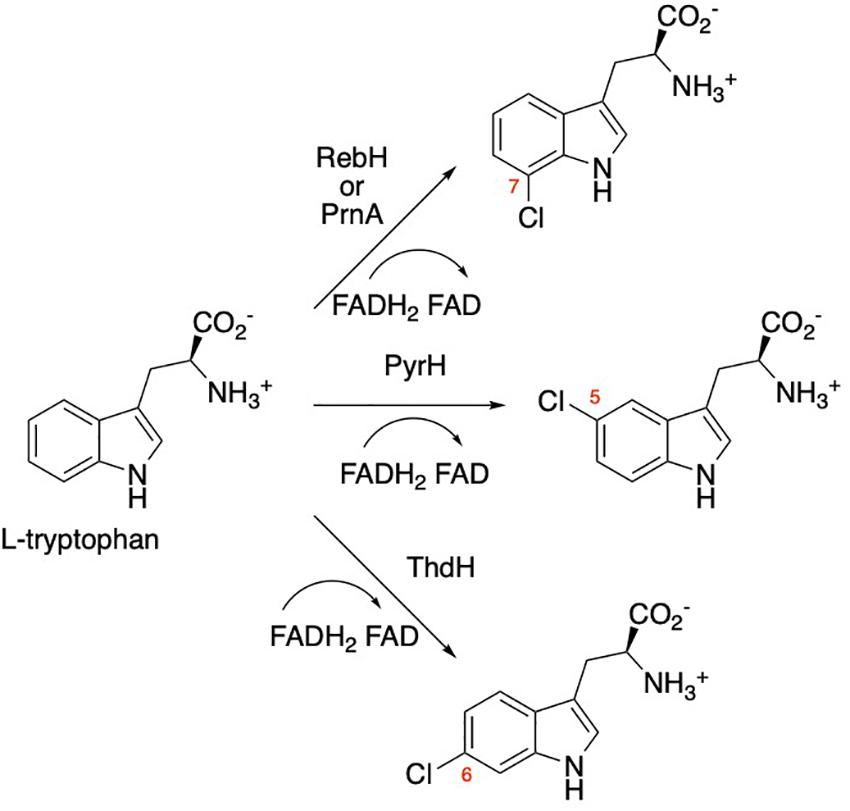
Figure 1. Regioselective halogenation of tryptophan by flavin-dependent bacterial halogenases RebH, PyrnA, PyrH, and ThdH. RebF is a flavin reductase (not shown) that recycles the flavin cofactor.
Although halogenation is widely observed among bacterial metabolites, this modification is relatively rare in terrestrial plants (Monde et al., 1998; Lam et al., 2015; Kim et al., 2020). However, there is an opportunity to use flavin-dependent halogenases from bacteria to create halogenated derivatives of plant natural products. For example, PyrH and RebH have been stably transformed into root cultures of the medicinal plant Catharanthus roseus to generate chlorinated tryptophan analogs that were subsequently incorporated into downstream alkaloid pathways (Runguphan et al., 2010). This approach relied on the native biosynthetic pathways of the host plant C. roseus. However, the model plant Nicotiana benthamiana is increasingly being used as factory in which secondary metabolite pathways are heterologously expressed and the resulting heterologous natural products can be produced in isolatable quantities (Reed and Osbourn, 2018). The method of agroinfiltration used to transiently transform the leaves of N. benthamiana allows the introduction of multiple enzymes by simply mixing together Agrobacterium tumefaciens strains harboring different biosynthetic genes (Sainsbury and Lomonossoff, 2014). This means that entire biosynthetic pathways, and combinations thereof, can be readily transformed into and expressed by this plant. Since recent work has demonstrated that bacterial halogenases can be functionally expressed in A. thaliana (Walter et al., 2020), Brassica rapa (Neumann et al., 2020), and N. benthamiana (Frabel et al., 2016), we set out to use N. benthamiana as a host for incorporation of bacterial halogenases into heterologous plant pathways to generate novel halogenated products.
Although previous work in our group established that halogenated monoterpene indole alkaloids can be produced in C. roseus root culture that is transformed with a bacterial halogenase (Runguphan et al., 2010), heterologous reconstitution of monoterpene indole alkaloid pathways (>10 enzymes) presents a formidable metabolic engineering challenge that is still being addressed. Instead, we chose to use a shorter plant-derived pathway as a test case for this study. Indole-3-Acetic Acid (IAA) is the most common auxin in higher plants, with a key role in plant growth and development (Cook and Ross, 2016). IAA can be formed from hydrolytic cleavage of IAA conjugates such as sugars, amino acids and peptides (Yang et al., 2008), but it is also produced directly via a de novo synthesis pathway (Mano and Nemoto, 2012). The synthesis of terpene indole alkaloids and the synthesis of IAA have a key similarity in that they are derived from tryptophan (O’Connor and Maresh, 2006). Here the similarity ends as the major IAA production pathway involves first a member of an aminotransferase family (TAR) that converts tryptophan to indole pyruvic acid (Tao et al., 2008; Zheng et al., 2013). This intermediate undergoes oxidative decarboxylation by a flavin-dependent monooxygenase (YUC) in the second step to produce IAA (Gao et al., 2016; Figure 2). Synthetic auxin analogs have been produced through chemical synthesis (Caboche et al., 1987), and a number of these derivatives display altered bioactivities. One halogenated auxin, 4-Cl-IAA, is produced naturally in certain members of the Fabaceae plant family, through the conversion of 4-Cl-Trp to Cl-IPA and finally to Cl-IAA (Tivendale et al., 2012), though the source of this halogenated tryptophan is still unknown (Karcz and Burdach, 2002). The accumulation of 4-Cl-IAA during the seed development in Pisum sativum, suggests that the enzymes involved in its biosynthesis are able to handle halogenated substrates. Therefore, we looked at this plant as a source of enzymes for our study.
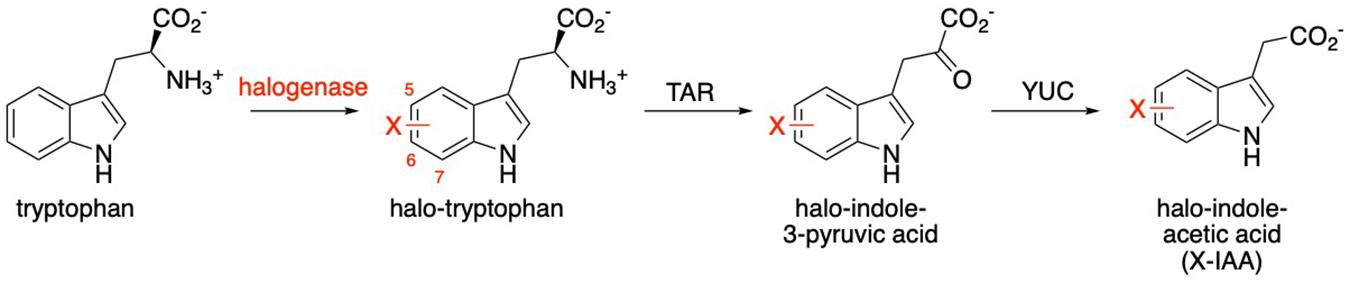
Figure 2. Production of indole-3-acetic acid (IAA) from tryptophan. The role of the bacterial halogenase (incorporating halogen X, typically Cl) is indicated in red.
The short, modular pathway of IAA seemed to be well-suited for combinatorial biosynthesis in N. benthamiana using a deconstructed plant virus, an approach in which genes from various biosynthetic pathways are mixed and matched to create new-to-nature products with potentially novel biological functions (Pickens et al., 2011). Here we express the 2-step IAA pathway using TAR genes from Arabidopsis thaliana and YUC genes from P. sativum. An agroinfiltration approach was applied to allow transient expression of multiple genes, including that of multiple bacterial halogenases, to produce novel halogenated IAA analogs in vivo. Additional auxin derivatives were produced by co-infiltration of the biosynthetic genes with various tryptophan analogs. These experiments demonstrate the capacity of N. benthamiana to produce a suite of halogenated products using combinatorial biosynthesis.
Results
Heterologous Expression of TAA and YUC to Produce IAA in N. benthamiana
We first assembled the suite of genes required for IAA biosynthesis. The members of the TAR aminotransferase family in A. thaliana are named TAA1 (AT1g70560) and TAR1-4 (AT1G23320, AT4G24670, AT1G34040, AT1G34060). These A. thaliana genes were selected for this study since their biochemical activity has been examined previously (Stepanova et al., 2008). The genes PsTAR1 (JQ002582.1) and PsTAR2 (JQ002584.1) have also been characterized (Caboche et al., 1987). Our preliminary experiments did not reveal any substantial difference in activity between the various aminotransferases when expressed in N. benthamiana but suggested that co-expression of two enzymes together slightly improved the conversion (data not shown). Therefore, all our experiments were performed using both AtTAA1 and AtTAR2. The second and last step of IAA biosynthesis is catalyzed by the flavin containing YUC enzymes. Whilst the YUC gene family in A. thaliana is known and some of the genes have been extensively characterized (Kim et al., 2007; Dai et al., 2013), information about the YUC genes in P. sativum is limited. In fact, only two genes, named PsYUC1 (HQ439907.2) and PsYUC2 (HQ439908.1) have been identified but not fully characterized at a functional level (Tivendale et al., 2010). Therefore, we performed RNA sequencing of developing pea seeds to identify all of the YUC genes expressed during the accumulation of 4-Cl-IAA. Eight genes were identified (PsYUC1-8, accession codes can be found in Supplementary Table S1) and were cloned from a cDNA library of the same tissues.
Following the expression of AtTAA1 and AtTAR2 and YUC genes (PsYUC1-8 or AtYUC6) in N. benthamiana leaves, free IAA accumulation was observed by UPLC/MS in transformed leaf tissue extracts (Figure 3). The production of free IAA from control plants infiltrated only with empty vector was below quantifiable levels. A total of 9 YUC genes from A. thaliana and P. sativum were tested with both AtTAA1 and AtTAR2 and although expression of each construct combination led to IAA production, the highest yields (approximately 500 ng/g FM) were observed with PsYUC7 and PsYUC4. The identity of the product was confirmed using UPLC-MS by comparison with an IAA standard (Supplementary Figure S1) and the quantification was based on a calibration curve. As there was no significant difference between the yield of IAA from expression of PsYUC4 and PsYUC7, we arbitrarily chose PsYUC7 to perform all further experiments requiring the YUC monooxygenase. Plants expressing the combination of TAR and YUC genes displayed a curly leaf phenotype, consistent with auxin over-production (Klee et al., 1987; Jackson et al., 2002; Supplementary Figure S2).
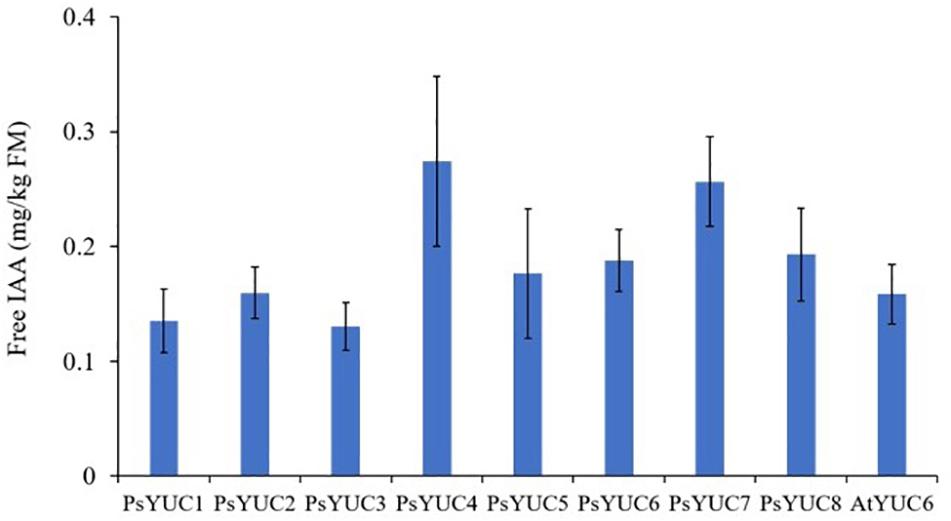
Figure 3. Free IAA production by N. benthamiana leaves above background levels following co-infiltration of AtTAA1, AtTAR2 and one of nine YUC genes. In each case, bars show the mean ± SEM, n = 4. The accumulation of free IAA in control plants infiltrated only with empty vector was below quantifiable levels so it is not shown in the figure.
Production of Chlorinated Tryptophan and Chlorinated IAA in N. benthamiana
Expression of each of the bacterial halogenases, RebH, PyrH, PrynA and ThdH, in N. benthamiana led to production of chlorinated tryptophan (Cl-Trp) (Figure 4). It has been reported previously that when expressed in cytosol in planta, RebH was capable of producing only trace amounts of halogenated tryptophan without the presence of the partner flavin reductase, RebF (Frabel et al., 2016). In the present study, each halogenase was expressed both with and without the FAD-reductase RebF, and in each case Cl-Trp could be observed by UPLC/MS (Supplementary Figure S3). The co-expression of RebF improved the yield of Cl-Trp only in the case of PyrH, whilst for the other halogenases no significant differences were observed. Identification of the chlorinated tryptophan products was performed by comparison using UPLC-MS with authentic 5-, 6-, and 7-chlorotryptophan standards (Supplementary Figure S4).
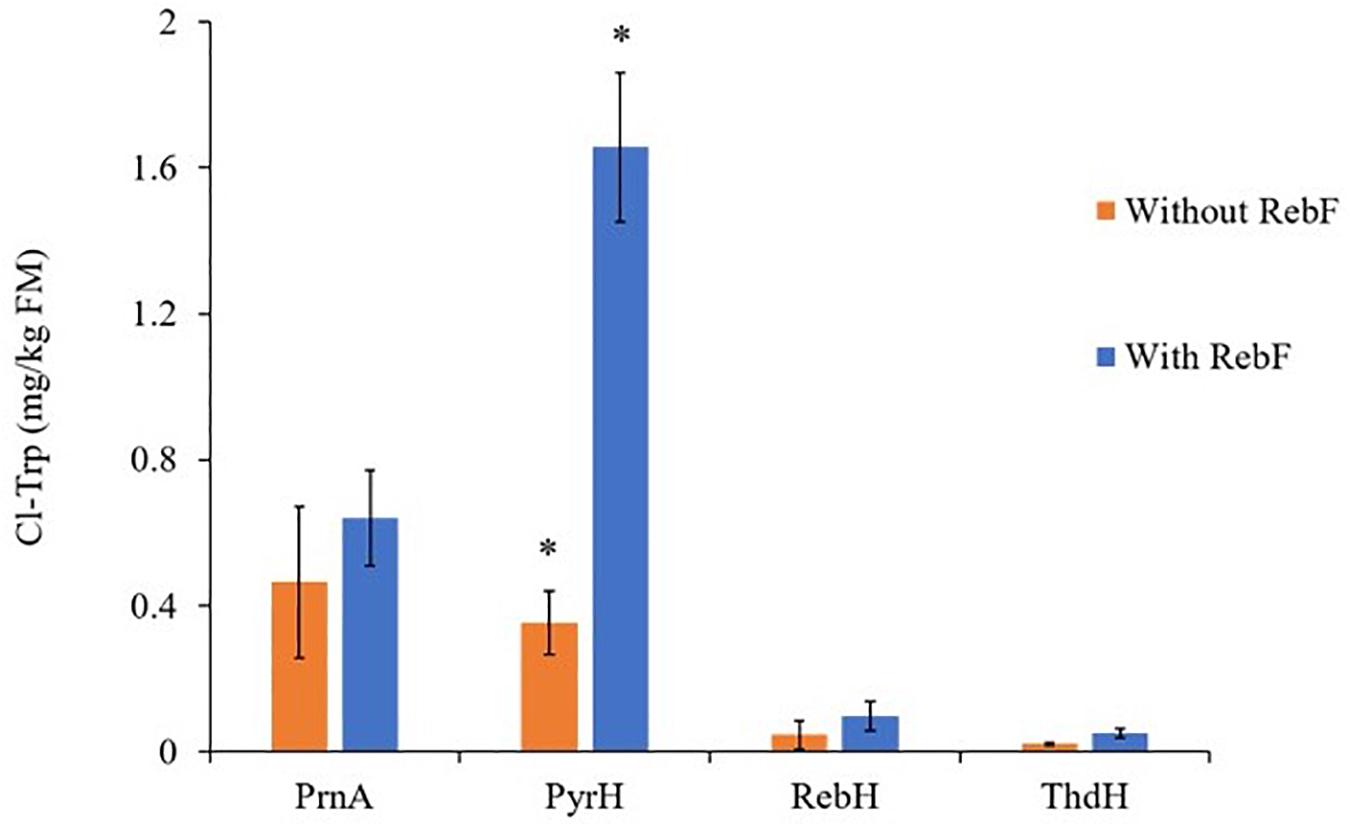
Figure 4. Cl-Trp production above background levels by N. benthamiana leaves following infiltration of a flavin-dependent halogenase with and without the partner reductase, RebF. In all cases bars show mean ± SEM, n = 4. * indicates significant difference following analysis by a two-sample, two-tailed Welch’s t-test in RStudio, p ≤ 0.005. A Shapiro–Wilks test was used to verify that the Cl-Trp production by each replicate leaf followed a normal distribution. The outcome of the t-test cannot be relied upon for the comparison between RebH +/– RebF, as the Cl-Trp replicate yields for RebH – RebF are not normally distributed.
Expression of each of the bacterial halogenases individually with AtTAA1, AtTAR2, and PsYUC7 led to production of Cl-IAA derivatives with the predicted regioselectivity (Figure 5). As above, the halogenases were active both in the presence and absence of RebF, and in both cases Cl-IAA was observed. The presence of RebF made a significant difference to the yield of Cl-IAA in the case of PyrH, leading to the accumulation of up to ∼990 ng/g FM of Cl-IAA. The identity of Cl-IAA was confirmed by comparison with peak retention times of relevant authentic 5-, 6-, and 7-Cl-IAA standards (Supplementary Figure S4). Beyond the curling leaf phenotype characteristic of auxin over-production, no additional phenotype was observed in plants producing halogenated versions of tryptophan or IAA.
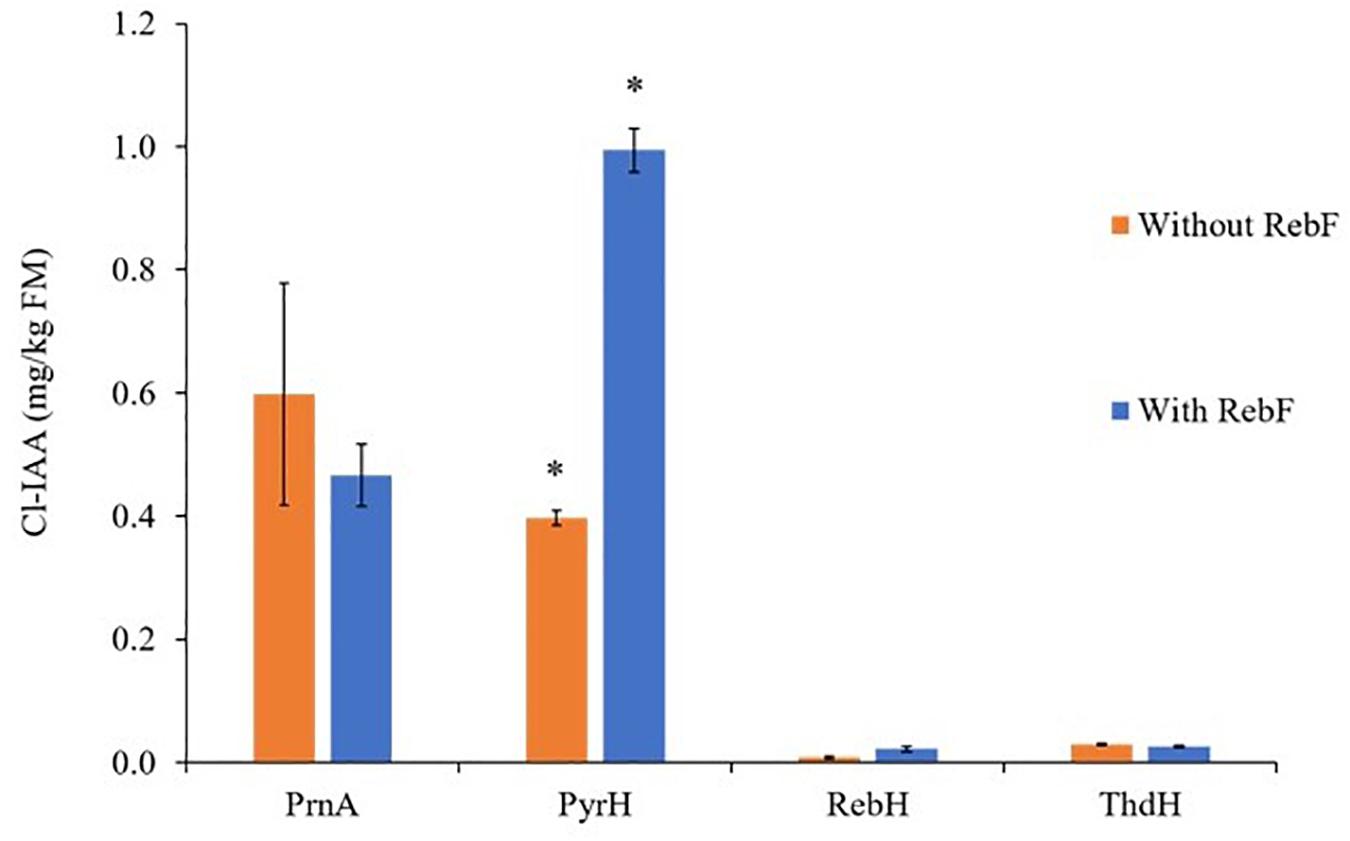
Figure 5. Free Cl-IAA production above background levels in N. benthamiana leaves following co-infiltration of AtTAA1, AtTAR2, PsYUC7 and one of four flavin-dependent halogenases with and without the partner reductase, RebF. Bars represent mean ± SEM, n = 4, except for RebH without RebF and PyrH with RebF for which n = 3. * indicates significant difference following a two-sample, two-tailed Welch’s t-test in RStudio, p ≤ 0.005. A Shapiro–Wilks test was used to verify that the Cl-Trp production by each replicate leaf followed a normal distribution. The outcome of the t-test cannot be relied upon for the comparison between ThdH+/– RebF, as the replicate Cl-IAA yields for ThdH + RebF are not normally distributed.
Production of Brominated IAA in N. benthamiana
RebH has been reported to install bromine onto the 7-position of tryptophan in planta following transformation in buffer containing KBr (Frabel et al., 2016). By infiltrating AtTAA1, AtTAR2, PsYUC7, RebF and each of the bacterial halogenases in buffer containing KBr, the production of Br-Trp and Br-IAA was achieved (Table 1). Although the production of Br-Trp was observed with all the halogenases tested, only transformations with PrnA, PyrH, and RebH genes led to successful production of Br-IAA (Supplementary Figure S5). In particular, of the total halogenated IAA PrnA produced 9.6% of Br-IAA, PyrH 3.5%, and RebH 17.6%. The identity of brominated tryptophan was confirmed using UPLC-MS, by comparison with authentic Br-Trp standards (Supplementary Figure S6).
Infiltration of Tryptophan Analogs for Additional IAA Analogs
Commercially available tryptophan analogs that cannot be readily accessed by known halogenases (Table 2) were infiltrated in N. benthamiana leaves, along with AtTAA1, AtTAR2, and PsYUC7. Formation of the corresponding IAA analogs was then assessed by LC-MS based metabolite analysis of the infiltrated leaves (Supplementary Figure S7). Although successful conversion to a product with a mass corresponding to the expected IAA derivative was observed in some cases, no IAA formation was observed for the fluoro-tryptophan series, for 7-Br-Trp and for 5-OH-Trp. We speculated that the electronic properties of the fluoro series and the 5-OH-Trp could interfere with enzyme recognition. We attributed the failure to produce 7-Br-Trp after infiltration to the poor solubility of this substrate in the infiltration buffer, which thus was not available to the enzymes. In fact, 7-Br-Trp also accumulated upon co-infiltration of KBr with the halogenases. Due to the lack of standards for the IAA analogs, the yields of these novel metabolites could not be quantified accurately.
Discussion
In this study, we explored the use of combinatorial biosynthesis to produce novel analogs of IAA in N. benthamiana, with a particular focus on generating halogenated analogs. Agroinfiltration was used to transiently express genes from the IAA pathway in N. benthamiana leaves. The background level of free IAA in the N. benthamiana leaf was below the limit of detection of our UPLC-MS analysis. While the specific pathway for auxin production in N. benthamiana is not currently known, the lack of free IAA was likely due to conversion of native IAA to conjugates to both spatially and temporally regulate the concentrations of this growth hormone (Walter et al., 2020). Over-production of auxin was also evident in transformed leaves by an apparent downward-curled phenotype. Previous literature has described this phenotype as being due to non-homeostatic levels of IAA affecting the regulation of adaxial (upper leaf layer) and abaxial (lower leaf layer) cell growth (Klee et al., 1987; Jackson et al., 2002).
To produce chlorinated IAA, chlorinated tryptophan substrate was first produced by expressing one of four bacterial halogenases individually in N. benthamiana. Relying on the cytosolic supply of tryptophan, the highest amount of Cl-Trp product (5-chlorotryptophan) was achieved using PyrH (∼1.66 μg/g FM). All four bacterial halogenases have similar Km and kcat values for chlorination of tryptophan (Pée et al., 2016), so the observed difference in product yield between the halogenases may be due to differences in transcription, translation or other factors. However, considering that all enzymes were produced in the plant using the same virus-derived vector that mediates episomal expression, we could hypothesize that the different product yields could results from different protein stabilities. PyrH was the only halogenase that showed a significantly higher production of Cl-Trp in presence of a partner reductase (RebF). In absence of RebF, the endogenous supply of FADH2 may have been sufficient for production of Cl-Trp by each halogenase (Figure 4). However, given the higher titers of chlorotryptophan that were obtained using PyrH, the endogenous supply of FADH2 may have been a rate-limiting factor for this halogenase. A previous study has shown that cytosolic expression of RebH in N. benthamiana leaves, in the absence of RebF, produces only trace amounts of Cl-Trp (Frabel et al., 2016). Only when localized to the chloroplast was a partner reductase unnecessary to produce quantifiable levels of Cl-Trp (Frabel et al., 2016). Although the data in our investigation are not entirely consistent with this, as quantifiable levels of Cl-Trp were achieved by cytosolic RebH in absence of RebF, it should be noted that the yield of Cl-Trp achieved is lower than that achieved by Frabel et al. (2016) in the chloroplast: up to ∼77.8 ng/mg, in comparison to ∼0.1 ± ng/mg achieved in this investigation. This difference could be due to higher levels of FADH2 and tryptophan in the chloroplast than in the cytosol.
Previous studies have confirmed the ability of bacterial halogenases to incorporate bromine as well as chlorine into a tryptophan scaffold in vitro. In planta, Frabel et al. (2016) achieved production of brominated tryptophan by infiltrating N. benthamiana with A. tumefaciens expressing RebH and Stth (coding for a tryptophan-6 halogenase) in buffer containing 40 mM KBr. Similarly, following infiltration of PyrH, RebH, and PrnA with AtTARs and PsYUC7 in KBr buffer, a compound with a mass corresponding to Br-IAA was observed in this investigation. Cl-IAA was also produced following infiltration with buffer containing KBr (Supplementary Figure S6), suggesting that the bacterial halogenases readily utilize the in-planta supply of chloride for tryptophan chlorination. By infiltrating N. benthamiana with tryptophan analogs, rather than bacterial halogenases, additional novel IAA analogs were produced, though the lack of appropriate standards prevented quantification and rigorous structural characterization of these novel products.
In this proof-of-concept study, we demonstrate that both directed biosynthesis and combinatorial biosynthesis can be used to generate halogenated metabolite analogs in a transient N. benthamiana expression system. Plants are increasingly being used as effective natural product factories due to their low-maintenance growth requirements (light, water, and nutrients) and also to the development of highly efficient transient expression systems. This study highlights how N. benthamiana can serve as a robust platform to rapidly access a library of halogenated derivatives through the incorporation of FAD-dependent halogenases into an established plant biosynthetic pathway. The new-to-nature auxin analogs generated within this investigation may therefore have applications in the study of plant developmental biology but more importantly, the possibility to produce tryptophan analogs in planta will provide access to precursors for further biosynthetic steps leading to compounds with diverse biological activities, such as molecules with anticancer, antiarrhythmic, and antidiabetic activities. This work provides a platform for tryptophan-based combinatorial biosynthesis, and is a starting point for optimization of gene expression and downstream processing to increase productivity and yields of the final products.
Materials and Methods
P. sativum Developing Seeds RNA Sequencing
Pisum sativum seeds (cultivar Cameor) were obtained from the Germplasm Resources Unit at the John Innes Centre United Kingdom and were grown in a glasshouse at 24/16°C day/night temperature with a 14-h photoperiod and at 70% relative humidity. The developing seeds were collected between 7 and 21 days after floral anthesis and the RNA was extracted using a RNeasy Plant mini kit (Qiagen). RNA sequencing was performed at the Earlham Institute (United Kingdom). Briefly, Illumina barcoded TruSeq RNA library were prepared and sequenced using Illumina HiSeq 2000/2500 in High-Output mode using 100 bp paired-end reads to generate at least 100 million pairs of reads per lane. FASTQC1 was used on the reads to assess the quality. Trinity software (Grabherr et al., 2011) was used to assembly a de novo transcriptome from the paired-end reads with the default parameters after removal of low-quality reads and trimming. PsYUC genes were identified by blasting the AtYUC6 sequence into the assembled transcriptome and retrieving the closest homologs.
Gene Cloning and Agrobacterium tumefaciens Transformation
Genes in Supplementary Table S1 were amplified by PCR from a cDNA library prepared using the SuperScript III kit (Invitrogen) using primers with vector specific over-hangs (Supplementary Table S2) and a high-fidelity polymerase (CloneAmp HiFi PCR Premix, Clontech-Takara). The PCR product was gel-purified and ligated using In-Fusion HD Cloning Kit (Clontech-Takara) into a modified TRBO (Lindbo, 2007) vector, in which the cloning cassette of the pOPINF (Berrow et al., 2007) vector was inserted in the NotI restriction site. Codon optimized bacterial halogenase genes RebH, PyrH, PrynA and ThdH and the flavin reductase RebF with the cloning overhangs were ligated into the linearized vector using In-Fusion HD Cloning Kit (Clontech-Takara). Competent Stellar E. coli cells were transformed with the constructs and selected on Luria-Bertani (LB) agar plates containing 50 μg/ml kanamycin. Colony PCR and Sanger sequencing were used to confirm the correct insertion of the genes. Electroporation was used to transform 50 μL aliquots of competent Agrobacterium tumefaciens (strain GV3101) cells with 2 μL of each plasmid (100–200 ng of DNA) containing a gene of interest. Following recovery in non-selective SOC medium for 2 h at 28°C, cells were plated on selective LB-agar containing 50 μg/mL kanamycin, 50 μg/mL rifampicin, and 25 μg/mL gentamicin and grown for 48 h at 28°C. Colonies formed were used to inoculate 10 mL aliquots of selective LB media in universal tubes and grown for 48 h at 28°C and 200 rpm. The cells were collected by centrifugation at 4000 × g for 10 min, resuspended in 10 mL infiltration buffer (10 mM NaCl, 1.75 mM CaCl2, 100 μM acetosyringone) to an OD600 of 1.0 and incubated at room temperature for 2 h.
N. benthamiana Transformation and Harvesting
Agrobacterium tumefaciens suspensions were diluted to an OD600 of 0.1 or 0.5 in infiltration buffer, as the OD600nm of the injected A. tumefaciens culture does not seem to impact significantly the amount of protein produced (Buyel and Fischer, 2012). Combinations of constructs were made to infiltrate each leaf with genes for completing multiple steps of the biosynthetic pathway. Control plants were infiltrated with cultures containing the modified TRBO vector, without an insert. Four leaves of 3-week-old N. benthamiana plants, grown in climate chambers at 24/21°C day/night temperature with a 16-h photoperiod and at 70% relative humidity, were infiltrated by injection with each bacterial suspension, forming four replicates for each construct combination. Syringes (1 mL, without needle) loaded with each bacterial culture were used to infiltrate the entire leaf from the abaxial side. Exogenous tryptophan substrates, if used, were infiltrated by the same method on the 4th day of growth as 100 μM solutions in infiltration buffer. Plants were harvested 5 days following infiltration with bacterial culture; harvested leaves were frozen in liquid nitrogen, individually ground to a powder using a pestle and mortar and stored at −80°C until extraction.
For Br incorporation the above protocol was used, replacing NaCl buffer with KBr buffer (40 mM KBr, 100 μM acetosyringone).
Metabolite Extraction
Metabolite extraction was performed using 200 mg of each powdered leaf sample, following a method described previously (Novák et al., 2012). Briefly, samples were vortexed in 1 mL phosphate buffer (100 mM K2HPO4, pH 7.0) and sonicated in a water bath for 5 min. Samples were centrifuged for 10 min at top speed in a table-top centrifuge, 7 μL 1 M HCl were added to the supernatants (pH to c.a. 2.7). Samples were centrifuged for a second time for 5 min. Supernatants were passed through an Oasis HLB solid phase extraction (SPE) column, washed with 1 mL of 5% MeOH and metabolites were eluted with 200 μL of 80% MeOH. This method was used for all harvested plant tissue except for the infiltrated 5-OH-Trp sample, for which the SPE column was washed with phosphate buffer prior to elution in 80% MeOH.
Metabolite Analysis by LC/MS
Ultra-performance liquid chromatography-Tandem mass spectrometry (UPLC-MS/MS) analysis of the metabolites was carried out on a Waters Acquity UPLC system connected to a Xevo TQS (Waters) spectrometer. Chromatography was performed using an Acquity BEH C18 1.7 μm 2.1 × 50 mm column kept at 35°C. Water containing 0.1% formic acid and acetonitrile containing 0.1% formic acid were used as mobile phases A and B, respectively with a flow rate of 0.35 mL min–1. The gradient was 1% B from 0 to 6 min followed by a linear gradient to 60% B for 6 to 6.5 min followed by isocratic 60% B. The injection volume of both the standard solutions and the samples was 2 μL. Samples were kept at 10°C during the analysis. MS detection was performed in positive ESI. Capillary voltage was 3.0 kV; the source was kept at 150°C; desolvation temperature was 500°C; cone gas flow, 100 L h–1; and desolvation gas flow, 800 L h–1. Unit resolution was applied to each quadrupole. Multiple reaction monitoring (MRM) signals, optimized using authentic standards, were used for detection and quantification of chlorinated tryptophan. MRM signals for IAA derivatives generated in this study from tryptophan analogs, for which we did not have standards, were predicted based on the fragmentation patterns and collision energies of halogenated IAA (Supplementary Table S3).
One-Pot Biotransformation of L-Halotryptophans With Tryptophan Synthase
The indole substrate (2 mmol) and serine (1.25 eq, 2.5 mmol) were suspended in 100 ml of reaction buffer (100 mM potassium phosphate, adjusted to pH 7.8). The crude lysate containing the overexpressed tryptophan synthase enzyme from Salmonella enterica [protocol for production detailed in Smith et al. (2014)] was thawed in a 37°C water bath, and 3 ml of lysate contained in cellulose tubing were used for each biotransformation. The biotransformations were incubated at 37°C with shaking at 180 rpm for 48 h. After completion of the reaction, the cellulose tubing was removed and washed with water. Unreacted indole was extracted with ethyl acetate (2 × 50 ml) and the aqueous phase was concentrated under reduced pressure to approximately 50 ml. The halogenated tryptophans were purified using manual reverse phase column chromatography. The pure samples were then treated twice with 20 ml 0.1 M hydrochloric acid to convert the tryptophan to the hydrochloride salt, removing the solvent under reduced pressure. The salt was then dissolved in 50 – 100 ml water and lyophilized to obtain the purified L-halotryptophan as a colorless, pink, yellow, or tan powder.
Data Availability Statement
The datasets generated for this study can be found in Data Dryad (Pisum sativum transcriptome) (https://datadryad.org/stash/sha re/xJfxCr7hevbW7EwC6B_tR5q_8nZapNZZ0vOK92x-wFc) and GenBank [PsYUC1-8 accession numbers, PsYUC1 (MW158556), PsYUC2 (MW158557), PsYUC3 (MW158558), PsYUC4 (MW158559), PsYUC5 (MW158560), PsYUC6 (MW158561), PsYUC7 (MW158562), and PsYUC8 (MW158563)].
Author Contributions
KD and LC performed and designed the experiments. DG, DS, and RG synthesized all tryptophan analogs. SO’C assisted with experimental design and managed the project. KD, LC, and SO’C all wrote the manuscript. All authors contributed to the article and approved the submitted version.
Funding
We gratefully acknowledge the Max Planck Society, ERA-IB project NBCPBH-EIB.13.008 (SO’C and RG) and ERC 788301 (SO’C).
Conflict of Interest
The authors declare that the research was conducted in the absence of any commercial or financial relationships that could be construed as a potential conflict of interest.
Acknowledgments
We thank Prof. Jutta Ludwig-Müller (Technische Universität Dresden, Germany) for providing us with PyrH, PrynA, and ThdH synthetic genes and Prof. Dr. Karl-Heinz van Pée (Technische Universität Dresden, Germany) for helpful discussions.
Supplementary Material
The Supplementary Material for this article can be found online at: https://www.frontiersin.org/articles/10.3389/fpls.2020.581675/full#supplementary-material
Footnotes
References
Berrow, N. S., Alderton, D., Sainsbury, S., Nettleship, J., Assenberg, R., Rahman, N., et al. (2007). A versatile ligation-independent cloning method suitable for high-throughput expression screening applications. Nucleic Acids Res. 35:748.
Buyel, J. F., and Fischer, R. (2012). Predictive models for transient protein expression in tobacco (Nicotiana tabacum L.) can optimize process time, yield, and downstream costs. Biotechnol. Bioeng. 109, 2575–2588. doi: 10.1002/bit.24523
Caboche, M., Muller, J. F., Chanut, F., Aranda, G., and Cirakoglu, S. (1987). Comparison of the growth promoting activities and toxicities of various auxin analogs on cells derived from wild type and a nonrooting mutant of tobacco. Plant Physiol. 83, 795–800. doi: 10.1104/pp.83.4.795
Cook, S. D., and Ross, J. J. (2016). The auxins, IAA and PAA, are synthesized by similar steps catalyzed by different enzymes. Plant Signal. Behav. 11:e1250993. doi: 10.1080/15592324.2016.1250993
Dai, X., Mashiguchi, K., Chen, Q., Kasahara, H., Kamiya, Y., Ojha, S., et al. (2013). The biochemical mechanism of auxin biosynthesis by an Arabidopsis YUCCA flavin-containing monooxygenase. J. Biol. Chem. 288, 1448–1457. doi: 10.1074/jbc.m112.424077
Frabel, S., Krischke, M., Staniek, A., and Warzecha, H. (2016). Recombinant flavin-dependent halogenases are functional in tobacco chloroplasts without co-expression of flavin reductase genes. Biotechnol. J. 11, 1586–1594. doi: 10.1002/biot.201600337
Frese, M., Guzowska, P. H., Voß, H., and Sewald, N. (2014). regioselective enzymatic halogenation of substituted tryptophan derivatives using the FAD-dependent halogenase RebH. ChemCatChem 6, 1270–1276.
Gao, Y., Dai, X., Zheng, Z., Kasahara, H., Kamiya, Y., Chory, J., et al. (2016). Overexpression of the bacterial tryptophan oxidase RebO affects auxin biosynthesis and Arabidopsis development. Sci. Bull. 61, 859–867. doi: 10.1007/s11434-016-1066-2
Grabherr, M. G., Haas, B. J., Yassour, M., Levin, J. Z., Thompson, D. A., Amit, I., et al. (2011). Full-length transcriptome assembly from RNA-Seq data without a reference genome. Nat. Biotechnol. 29, 644–652. doi: 10.1038/nbt.1883
Jackson, R., Kowalczyk, M., Li, Y., Higgins, G., Ross, J., Sandberg, G., et al. (2002). Over-expression of an Arabidopsis gene encoding a glucosyltransferase of indole-3-acetic acid: Phenotypic characterisation of transgenic lines. Plant J. 32, 573–583. doi: 10.1046/j.1365-313x.2002.01445.x
Jeschke, P. (2010). The unique role of halogen substituents in the design of modern agrochemicals †. Pest Manag. Sci. 66, 10–27. doi: 10.1002/ps.1829
Jung, H., Kim, S., and Moon, H. (2007). Optimization of culture conditions and scale-up to pilot and plant scales for vancomycin production by Amycolatopsis orientalis. Appl. Microbiol. Biotechnol. 77, 789–795. doi: 10.1007/s00253-007-1221-4
Karcz, W., and Burdach, Z. A. (2002). comparison of the effects of IAA and 4-Cl-IAA on growth, proton secretion and membrane potential in maize coleoptile segments. J. Exp. Bot. 53, 1089–1098. doi: 10.1093/jexbot/53.371.1089
Keller, S., Wage, T., Hohaus, K., Hölzer, M., Eichhorn, E., and van Pée, K. H. (2000). Purification and partial characterization of tryptophan 7-halogenase (PrnA) from Pseudomonas fluorescens. Angew. Chem. Int. Ed. 39, 2300–2302. doi: 10.1002/1521-3773(20000703)39:13<2300::aid-anie2300>3.0.co;2-i
Kim, C. Y., Mitchell, A. J., Glinkerman, C. M., Fu- Li, S., Pluskal, T., and Weng, J. (2020). The chloroalkaloid (-)-acutumine is biosynthesized via a Fe(II)- And 2-oxoglutarate-dependent halogenase in menispermaceae plants. Nat. Commun. 11:1867.
Kim, J. I., Sharkhuu, A., Jin, J. B., Li, P., Jeong, J. C., Baek, D., et al. (2007). yucca6, a dominant mutation in Arabidopsis, affects auxin accumulation and auxin-related phenotypes. Plant Physiol. 145, 722–735. doi: 10.1104/pp.107.104935
Klee, H. J., Horsh, R. B., Hinchee, M. A., Hein, M. B., and Hoffmann, N. L. (1987). The effects of overproduction of two Agrobacterium tumefaciens T-DNA auxin biosynthetic gene products in trangenic petunia plants. Genes Dev. 1, 86–96. doi: 10.1101/gad.1.1.86
Lam, H. K., Mcadam, S. A. M., Mcadam, E. L., and Ross, J. J. (2015). Evidence that chlorinated auxin is restricted to the fabaceae but not to the fabeae. Plant Physiol. 168, 798–803. doi: 10.1104/pp.15.00410
Lindbo, J. A. (2007). TRBO: a high efficiency tobacco mosaic virus RNA based overexpression vector. Plant Physiol. 145:89384.
Mano, Y., and Nemoto, K. (2012). The pathway of auxin biosynthesis in plants. J. Exp. Bot. 63, 2853–2872. doi: 10.1093/jxb/ers091
Menon, B. R., Latham, J., Dunstan, M. S., Brandenburger, E., Klemstein, U., Leys, D., et al. (2016). Structure and biocatalytic scope of thermophilic flavin-dependent halogenase and flavin reductase enzymes. Org. Biomol. Chem 14, 9354–9361. doi: 10.1039/c6ob01861k
Milbredt, D., Patallo, E. P., and Pøe, K. (2014). A tryptophan 6-halogenase and an aminotransferase are involved in thienodolin biosynthesis. ChemBioChem 15, 1011–1020. doi: 10.1002/cbic.201400016
Miller, C. P., Manton, C. A., Hale, R., Debose, L., Macherla, V. R., Potts, B. C., et al. (2011). Specific and prolonged proteasome inhibition dictates apoptosis induction by marizomib and its analogs. Chem. Biol. Interact. 194, 58–68. doi: 10.1016/j.cbi.2011.08.005
Monde, K., Satoh, H., Nakamura, M., Tamura, M., and Takasugi, M. (1998). Organochlorine compounds from a terrestrial higher plant?: structures and origin of chlorinated orcinol derivatives from diseased bulbs of Lilium maximowiczii. J. Nat. Prod. 61, 913–921. doi: 10.1021/np980062r
Neumann, C. S., Fujimori, D. G., and Walsh, C. T. (2008). Halogenation strategies in natural product biosynthesis. Chem. Biol. 15, 99–109. doi: 10.1016/j.chembiol.2008.01.006
Neumann, M., Prahl, S., Caputi, L., Hill, L., Kular, B., Walter, A., et al. (2020). Hairy root transformation of brassica rapa with bacterial halogenase genes and regeneration to adult plants to modify production of indolic compounds. Phytochemistry 175:112371. doi: 10.1016/j.phytochem.2020.112371
Novák, O., Hényková, E., Sairanen, I., Kowalczyk, M., Pospíšil, T., and Ljung, K. (2012). Tissue-specific profiling of the Arabidopsis thaliana auxin metabolome. Plant J. 72, 523–536. doi: 10.1111/j.1365-313x.2012.05085.x
O’Connor, S. E., and Maresh, J. J. (2006). Chemistry and biology of monoterpene indole alkaloid biosynthesis. Nat. Prod. Rep. 23, 532–547. doi: 10.1039/b512615k
Payne, J. T., Andorfer, M. C., and Lewis, J. C. (2013). Regioselective arene halogenation using the FAD-dependent halogenase RebH. Angew. Chem. Int. Edgew. Chem. Int. Ed. 52, 5271–5274. doi: 10.1002/anie.201300762
Pée, K., Milbredt, D., Patallo, E. P., Weichold, V., and Gajewi, M. (2016). Application and Modification of Flavin-Dependent Halogenases. Synthetic Biology and Metabolic Engineering in Plants and Microbes Part A: Metabolism in Microbes 575. Amsterdam: Elsevier Inc.
Pickens, L. B., Tang, Y., and Chooi, Y. (2011). Metabolic engineering for the production of natural products. Annu. Rev. Chem. Biomol. Eng. 2, 211–236. doi: 10.1146/annurev-chembioeng-061010-114209
Reed, J., and Osbourn, A. (2018). Engineering terpenoid production through transient expression in Nicotiana benthamiana. Plant Cell Rep. 37, 1431–1441. doi: 10.1007/s00299-018-2296-3
Runguphan, W., Qu, X., and O’Connor, S. E. (2010). Integrating carbon–halogen bond formation into medicinal plant metabolism. Nature 468, 461–464. doi: 10.1038/nature09524
Sainsbury, F., and Lomonossoff, G. P. (2014). Transient expressions of synthetic biology in plants. Curr. Opin. Plant Biol. 19, 1–7. doi: 10.1016/j.pbi.2014.02.003
Saykhedkar, S. S., and Singhal, R. S. (2004). Solid-state fermentation for production of griseofulvin on rice bran using Penicillium griseofulvum. Biotechnol. Prog. 20, 1280–1284.
Shepherd, S. A., Menon, B. R., Fisk, H., Struck, A. W., Levy, C., Leys, D., et al. (2016). Structure-guided switch in the regioselectivity of a tryptophan halogenase. ChemBioChem 17, 821–824. doi: 10.1002/cbic.201600051
Smith, D. R., Willemse, T., Gkotsi, D. S., Schepens, W., Maes, B. U., Ballet, S., et al. (2014). The first one-pot synthesis of L-7-iodotryptophan from 7-iodoindole and serine, and an improved synthesis of other L-7-halotryptophans. Org. Lett. 16, 2622–2625. doi: 10.1021/ol5007746
Stepanova, A. N., Robertson-Hoyt, J., Yun, J., Benavente, L. M., Xie, D. Y., Dolezal, K., et al. (2008). TAA1-mediated auxin biosynthesis is essential for hormone crosstalk and plant development. Cell 133, 177–191. doi: 10.1016/j.cell.2008.01.047
Tao, Y., Ferrer, J. L., Ljung, K., Pojer, F., Hong, F., Long, J. A., et al. (2008). Rapid synthesis of auxin via a new tryptophan-dependent pathway is required for shade avoidance in plants. Cell 133, 164–176. doi: 10.1016/j.cell.2008.01.049
Tivendale, N. D., Davidson, S. E., Davies, N. W., Smith, J. A., Dalmais, M., Bendahmane, A. I., et al. (2012). Biosynthesis of the halogenated auxin, 4-chloroindole-3-acetic acid. Plant Physiol. 159, 1055–1063. doi: 10.1104/pp.112.198457
Tivendale, N. D., Davies, N. W., Molesworth, P. P., Davidson, S. E., Smith, J. A., Lowe, E., et al. (2010). Reassessing the role of N-hydroxytryptamine in auxin biosynthesis. Plant Physiol. 154, 1957–1965. doi: 10.1104/pp.110.165803
Walter, A., Caputi, L., O’Connor, S., van Pée, K.-H., and Ludwig-Müller, J. (2020). Chlorinated auxins-how does arabidopsis thaliana deal with them? Int. J. Mol. Sci. 21:2567. doi: 10.3390/ijms21072567
Yang, Y., Xu, R., Ma, C. J., Vlot, A. C., Klessig, D. F., and Pichersky, E. (2008). Inactive methyl indole-3-acetic acid ester can be hydrolyzed and activated by several esterases belonging to the AtMES esterase family of Arabidopsis. Plant Physiol. 147, 1034–1045. doi: 10.1104/pp.108.118224
Yeh, E., Garneau, S., and Walsh, C. T. (2005). Robust in vitro activity of RebF and RebH, a two-component reductase/halogenase, generating 7-chlorotryptophan during rebeccamycin biosynthesis. Proc. Natl. Acad. Sci. U.S.A. 102, 3960–3965. doi: 10.1073/pnas.0500755102
Zehner, S., Kotzsch, A., Bister, B., Süssmuth, R. D., Méndez, C., Salas, J. A., et al. (2005). Regioselective tryptophan 5-halogenase is involved in pyrroindomycin biosynthesis in streptomyces rugosporus LL-42D005. Chem. Biol. 12, 445–452. doi: 10.1016/j.chembiol.2005.02.005
Keywords: indole-acetic acid, halogenase, combinatorial biosynthesis, auxin, unnatural natural product, new to nature products
Citation: Davis K, Gkotsi DS, Smith DRM, Goss RJM, Caputi L and O’Connor SE (2020) Nicotiana benthamiana as a Transient Expression Host to Produce Auxin Analogs. Front. Plant Sci. 11:581675. doi: 10.3389/fpls.2020.581675
Received: 09 July 2020; Accepted: 26 October 2020;
Published: 20 November 2020.
Edited by:
Agnieszka Ludwików, Adam Mickiewicz University, PolandReviewed by:
Linda Avesani, University of Verona, ItalyJohannes Felix Buyel, Fraunhofer Society (FHG), Germany
Copyright © 2020 Davis, Gkotsi, Smith, Goss, Caputi and O’Connor. This is an open-access article distributed under the terms of the Creative Commons Attribution License (CC BY). The use, distribution or reproduction in other forums is permitted, provided the original author(s) and the copyright owner(s) are credited and that the original publication in this journal is cited, in accordance with accepted academic practice. No use, distribution or reproduction is permitted which does not comply with these terms.
*Correspondence: Sarah E. O’Connor, oconnor@ice.mpg.de