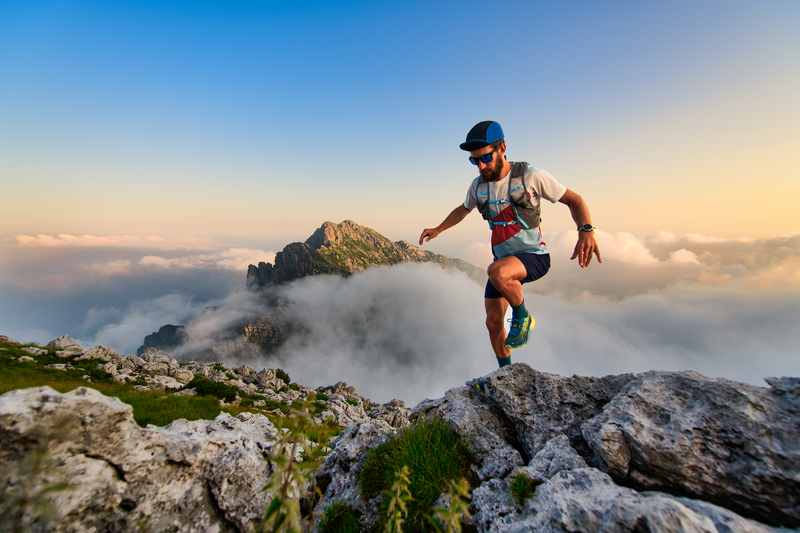
94% of researchers rate our articles as excellent or good
Learn more about the work of our research integrity team to safeguard the quality of each article we publish.
Find out more
ORIGINAL RESEARCH article
Front. Plant Sci. , 26 October 2020
Sec. Plant Biotechnology
Volume 11 - 2020 | https://doi.org/10.3389/fpls.2020.581100
Dengue fever is endemic in more than 120 countries, which account for 3.9 billion people at risk of infection worldwide. The absence of a vaccine with effective protection against the four serotypes of this virus makes differential molecular diagnosis the key step for the correct treatment of the disease. Rapid and efficient diagnosis prevents progression to a more severe stage of this disease. Currently, the limiting factor in the manufacture of dengue (DENV) diagnostic kits is the lack of large-scale production of the non-structural 1 (NS1) protein (antigen) to be used in the capture of antibodies from the blood serum of infected patients. In this work, we use plant biotechnology and genetic engineering as tools for the study of protein production for research and commercial purposes. Gene transfer, integration and expression in plants is a valid strategy for obtaining large-scale and low-cost heterologous protein production. The authors produced NS1 protein of the dengue virus serotype 2 (NS1DENV2) in the Arabidopsis thaliana plant. Transgenic plants obtained by genetic transformation expressed the recombinant protein that was purified and characterized for diagnostic use. The yield was 203 μg of the recombinant protein per gram of fresh leaf. By in situ immunolocalization, transgenic protein was observed within the plant tissue, located in aggregates bodies. These antigens showed high sensitivity and specificity to both IgM (84.29% and 91.43%, respectively) and IgG (83.08% and 87.69%, respectively). The study goes a step further to validate the use of plants as a strategy for obtaining large-scale and efficient protein production to be used in dengue virus diagnostic tests.
This study goes a step further to validate the use of plants as a strategy for obtaining large-scale and efficient protein production to be used in dengue virus diagnostic tests.
Dengue virus (DENV) infection is one of the most important human diseases transmitted by arthropods. Virus transmission is performed by mosquito vector, with predominance of the genus Aedes (Bhatt et al., 2013). Like other emerging infectious diseases, outbreaks of the DENV are unpredictable and potentially widespread, and can result in epidemics that threaten global public health (Fauci and Morens, 2012; Morens and Fauci, 2013; McArthur, 2019). The disease is endemic in over 120 countries, which account for 3.9 billion people at risk of infection worldwide (World Health Organization, 2020). From 1998 to 2018, more than 20 million cases were recorded in the Americas, 65% of which were reported in Brazil (Pan American Health Organization, 2019).
The DENV belongs to the Flaviviridae family and has four antigenically distinct serotypes, DENV1, DENV2, DENV3, and DENV4 (Lindenbach and Rice, 2003; Best, 2016). The DENV viruses are enveloped, with a single-stranded RNA genome with positive sense. Genome translation results in three structural proteins (envelope protein – E, membrane protein – M, and capsid protein – C) and seven non-structural proteins (NS1, NS2a, NS2b, NS3, NS4a, NS4b, and NS5). Non-structural (NS) proteins are related to viral replication, protein expression and virulence of serotypes (Heinz and Stiasny, 2012; Simmonds et al., 2017).
Non-structural 1 is a highly conserved glycoprotein among all Flaviviruses, with molecular weights ranging from 46 to 55 kDa, depending on the glycan pattern (Winkler et al., 1988; Pryor and Wright, 1994). Correct glycosylation is related to its efficient secretion, virulence, and virus replication. Protein can be found in three forms: monomer, dimer (membrane anchored), and hexamer (secreted) (Noisakran et al., 2008; Chuang et al., 2013; Muller and Young, 2013). The different actions attributed to NS1 include the activation of toll-like receptors (TLRs), complement system inhibition, and immune response induction linked to the acute phase in severe cases (Libraty et al., 2002; Avirutnan et al., 2010; Rastogi et al., 2016; Modhiran et al., 2017): it is the first viral protein present in the bloodstream of infected patients, and is currently used as a biomarker for early diagnosis in the acute phase (Shu et al., 2009).
The first licensed tetravalent vaccine, Dengvaxia (Sanofi Pasteur), presented complications in protecting individuals who had never had the disease before, resulting in a WHO alert with recommendations for further testing (Guy et al., 2015; Iacobucci, 2018). A problem presented in recent years is the occurrence of dengue infections in regions where cases had not yet been reported. The spreading of the virus is cause for alarm, and intensifies the need to control infections through correct diagnosis, avoiding underreported cases. Since many infected patients are asymptomatic and present non-specific conditions, it is not possible to rely solely on clinical manifestations, which reinforces the need for differential molecular diagnosis (Mardekian and Roberts, 2015; Katzelnick et al., 2017; Muller et al., 2017; Wiemer et al., 2017). Therefore, a quick, accurate and low-cost diagnosis is essential to confirm suspicions of dengue cases, favoring the adequate treatment of infected patients, especially in countries with limited health care resources.
Due to the lack of a vaccine with effective protection against all the four serotypes of the virus (Shrivastava et al., 2017), differential molecular diagnosis is the best and safest alternative for the correct treatment of the disease (Pang et al., 2017). As recommended by the WHO Special Program for Research and Training in Tropical Diseases (TDR), the specifications of an ideal test for dengue should address the following points: (i) high sensitivity and specificity in detection, (ii) fast results, and (iii) low cost (Peeling et al., 2010).
Detection of anti-dengue antibodies in serum samples is widely used, especially in developing countries, due to its ease of use compared to other techniques such as viral RNA detection. In the primary infection with DENV, IgM response has higher titers and is more specific than during subsequent infections. In contrast, the IgG titer is higher in a second infection. Several IgM and IgG capture ELISA kits are commercially available, with sensitivity ranging from 21 to 99% and 8 to 89%, respectively, and specificities varying from 52 to 100% for IgM and 63 to 100% for IgG. The antigens used in the commercial kits are obtained from virus culture in mouse brain, Vero cells and C6/36 mosquito cells (Groen et al., 2000; Pal et al., 2014; Fatima and Wang, 2015; Guerrero and Bello, 2019), which require high levels of biosafety and make them expensive to prepare. In non-endemic regions, IgM-based tests can be used in clinical surveillance, with a high probability that positive results indicate recent infections (during the last 2–3 months) (Dutra et al., 2009; Tuan et al., 2015; Wang et al., 2015; Pang et al., 2017).
Plant biotechnology in recent years has evolved significantly with the use of genetic engineering as a tool for protein production, both for research and commercial purposes. Gene transfer, integration and expression in plants is a valid strategy for obtaining large-scale and low-cost heterologous proteins (Schillberg et al., 2019). In addition to transgenic systems, other plant production techniques are being explored, including transient expression systems, inducible expression systems, and protein targeting (Twyman et al., 2003; Schillberg et al., 2013; Spiegel et al., 2015). In transient expression, exogenous DNA is not integrated into the host plant genome. It generates results in a shorter time; however, in stable expression, the hosts can be used for several generations and for a longer time. Furthermore, new transfections are not necessary (Schillberg et al., 2019). Compared with other organisms, plants have advantages because they are eukaryotes with a mammalian-like post-translational processing system. Therefore, proteins that need modification, such as glycosylation, are more accurately represented in these organisms (Obembe et al., 2011; Kim et al., 2014; Ko, 2014).
The production of viral proteins in plants is commercially attractive because of the facility for increasing the scale of production and purification, with plants functioning as bioreactors. In addition to presenting advantages in terms of biosafety, plant production has a low cost (Commandeur et al., 2003). Since the first protein expressed in tobacco by Barta et al. (1986), research has evolved, and a large number of products with therapeutic importance, such as vaccines, antibodies and proteins with different purposes, have been expressed in plants, using different strategies and plant hosts (Mason et al., 1992; Mett et al., 2008; Demain and Vaishnav, 2009; Bock and Warzecha, 2010; Gomord et al., 2010; Vojta et al., 2015).
In this work, Arabidopsis thaliana was chosen because it is a small herbaceous plant, with short generation time and large seed production. The genome has a relatively small size and ease of transformation by Agrobacterium tumefaciens (Hwang et al., 2017). As a further advantage, the transformed plant functions as a bioreactor and can be cultivated in confined spaces, such as a controlled growth chamber or greenhouse (Lloyd et al., 1986; Feldmann and Marks, 1987; Chang et al., 1994; Lacroix and Citovsky, 2013; Pitzschke, 2013; Von Schaewen et al., 2018).
After genetic transformation, a clone with the DENV2 non-structural protein 1 (NS1) gene introduced into the genome was selected. From this, we purified and characterized the recombinant protein, which presented antigenic potential, with the ability to recognize anti-dengue antibodies in the serum of infected patients with high specificity and sensitivity in enzyme-linked immunosorbent assays (ELISA). The study takes a step forward by using the plant as the key part of a valid strategy for large-scale and efficient production of a protein that will be used in serological tests, since the production of this antigen is still a key point that generates high cost in commercial diagnosis kits.
Non-structural protein 1 (NS1) from DENV2 strain New Guinea C (GenBank access: MK506264) was codon-optimized for expression in A. thaliana and synthesized by GenScriptTM. The cassette inserted into the pUC57 vector was constructed with: (i) the restriction sites for Bgl II and BstE II enzymes flanking the gene (for cloning); (ii) Kozak sequence (ribosome recognition site in plant eukaryotic cells) (Kozak, 1978, 2002; Nakagawa et al., 2008); (iii) signal peptide Bip At5g44620 (targeting to the rough endoplasmic reticulum – RER) (Lee et al., 2011); (iv) polyhistidine tag (to purification step) (Hochuli et al., 1987; Terpe, 2003); and (v) HDEL sequence (RER membrane attachment) (Munro and Pelham, 1987; Napier et al., 1992; Koizumi, 1996), totaling 1292 bp. Restriction enzymes (Bgl II and BstE II) were used to cut and clone the insert into the expression vector pCAMBIA3301 (Figure 1).
The cloning vector obtained, pUC57_NS1DENV2, was maintained in Escherichia coli TOP10F. Binary vector pCAMBIA3301 has the herbicide resistance gene (bar) to phosphinothricin (PPT) and the kanamycin resistance gene as the selection markers. Subsequent to the cloning of the NS1DENV2 gene into the expression vector (pCAMBIA3301_NS1DENV2), the competent A. tumefaciens strain GV3101 was transformed by electroporation (McCormac et al., 1998), grown at 28°C on YEB medium (0.5% m/v peptone, 0.1% m/v yeast extract, 0.5% m/v meat extract, 0.5% m/v sucrose, and 0.024% m/v MgSO4, pH 6.8) with gentamycin (100 μg/ml) and kanamycin (50 μg/ml) antibiotics, and positive colonies were selected.
In the polymerase chain reaction (PCR), a pair of primers, pCambNS1s (5′-GGAGATCTATGGATAGTGGTT GCGTTGTGA-3′) and pCambNS1as (5′-GGGGTAACCTGAG GCTGTGACCAAGGAGT-3′), was used to confirm positive colonies, flanking the NS1DENV2 gene region, resulting in a 1056 bp fragment. The GoTaq® DNA Polymerase kit (PromegaTM, United States) was used, with an initial step of 94°C for 5 min, 35 cycles of 95°C for 1 min, 55°C for 2 min and 72°C for 2 min, and a final step of 72°C for 10 min.
The digestion reaction was performed using restriction enzymes Bgl II and BstE II (Thermo FisherTM, United States), which flank the NS1DENV2 gene and generate a fragment with 1292 bp. The double digestion reaction was incubated at 37°C for 12 h. For cloning, the linearized expression vector and the insert were ligated using the T4 DNA Ligase kit (PromegaTM), purified from 1% agarose gel using the QIAquick Gel Extraction kit (QIAGENTM, Germany).
The wild type (WT) A. thaliana Columbia (Col-0) seeds were incubated using a photoperiod with 16 h exposure to light (200 μmol/m2s) and 8 h dark at 25°C on a composite substrate (organic substrate and thick vermiculite, 2:1). After germination and vegetative growth until the emergence of the first flowers, the main shoot was removed to produce the emergence of a larger number of lateral shoots. Plants with lateral shoots with approximately 45 days in the early stage of flowering were used for transformation. The A. tumefaciens culture (grown at 28°C, 150 rpm, OD = 0.4/0.5, at 600 nm absorbance) with vector pCAMBIA3301_NS1DENV2 was used to perform floral-dip (Clough and Bent, 1998), a method that involves immersing the flower buds in the bacterial suspension three times in a 7-day cycle. Plants were kept in a dark environment for 24 h, after which they were subjected to the ideal conditions for vegetative growth until the reproductive cycle was complete for maturation and seed collection.
All generations were obtained for self-fertilization. T1 seeds (first generation, collected from plant T0, the initial transformed generation) were sterilized (sodium hypochlorite and autoclaved distilled water, 1:1) and seeded with MS medium (Murashige and Skoog, 1962) with phosphinothricin (7.5 μg/mL) for selection of transgenic plants. They were then incubated at 4°C in the dark for 72 h to break dormancy, and then placed in the growth chamber with a photoperiod of 8 h of light and 16 h of darkness at 24–26°C for germination. After 10 days, the seedlings that emerged were selected and transplanted into composite substrate until the next generation of seeds appeared. The T2 seeds were cultured as before until the T3 generation, and the dominant homozygous plants for the NS1DENV2 transgene were selected, cultured and stored at −80°C for further analysis.
At 40 days of age, the leaves of the T3 plants were macerated in nitrogen and the genomic DNA of the clones was extracted by the method described by Edwards et al. (1991) in triplicate. PCR was done to confirm the insertion of the transgene using the same primers previously employed for cloning confirmation. One of the clones was selected for the following steps.
For each 1 g of fresh leaf (both transformed and WT, which served as control) with 40 days of age, macerated in liquid nitrogen, 5 mL of extraction buffer (100 mM Tris–HCl, 1 mM EDTA, 2% SDS, pH 8.0) was added, containing 0.1 mM PMSF (protease inhibitor) and 0.2 g PVPP (Polyvinylpolypyrrolidone). Then, leaf extracts were sonicated on ice for 10 s at 30% amplitude, centrifuged at 12,000 × g, and the supernatant was stored at −80°C.
After total protein extraction, the supernatant was diluted in binding buffer (sodium phosphate 20 mM, NaCl 500 mM, imidazole 20 mM, pH 7.4) and purified by affinity chromatography. For each 1 ml of extraction supernatant, 1 ml of binding buffer was added. A 5 mL HisTrap® Fast Flow Crude column (GE HealthCareTM, Sweden), previously equilibrated with the binding buffer, was coupled to the AKTA® purification system (GE HealthcareTM, Sweden). The flow rate was 0.5 mL/min and the retention time in the column was 10 min/mL. After injection of total protein into the system and its flow through the column to bind it, it was washed with a 10X sample volume with binding buffer. The column-bound protein fraction was recovered using a pH 7.4 elution buffer (20 mM sodium phosphate, 500 mM NaCl, 400 mM imidazole). After purification the sample was filtered using Amicon® Ultra-15 Centrifugal Filter Units 10,000 NMWL to remove imidazole, using sodium phosphate buffer (20 mM). Then it was lyophilized and solubilized in 1 mL of Tris–HCl buffer (100 mM), pH 8.8. Total quantification was performed using the BCA kit (Pierce Chemical Co., United States). We estimated the specific concentration of NS1DENV2 by densitometry of bands in the gel with a standard curve of BSA. For this, we use the ImageJ software.
The protein extract (10 μL) was submitted to electrophoresis in acrylamide gel (SDS-PAGE 12%) and stained with Coomassie G-250. To perform the immunoassay the NS1-DENV2 protein was transferred to a nitrocellulose membrane. As a primary antibody, a polyclonal pool from three patients positive to the dengue disease was used. A goat-produced polyvalent anti-human IgM conjugated with peroxidase was used as a secondary antibody (Sigma-AldrichTM, Brazil).
The dot-blot was made from one drop (5 μL) of the crude extract of the transformed plant (P3) and the WT plant on a nitrocellulose membrane, using the same steps as western blot.
Confocal laser scanning microscopy (CLSM) with the LSM 510 (Zeiss®, Germany) at the Microscopy and Microanalysis Nucleus (UFV) was used for the immunolocalization assay. One leaf of each plant, at 30 days of age, was used for in situ NS1DENV2 protein detection (Sauer et al., 2006).
After being fixed in 4% paraformaldehyde, both transgenic and wild plant tissues were labeled with primary anti-His tag monoclonal antibodies (Sigma-AldrichTM, Brazil) (1:500, v/v) in 1X PBS (0.02% KCl, 0.8% NaCl, 0.18% Na2HPO4.2H2O, 0.024% KH2PO4, pH 7.4), and sequentially, tissues were incubated with FITC-conjugated secondary antibody (Sigma-AldrichTM, Brazil) (1:500: v/v) in 1X PBS solution, for 2 h at 37°C. Then, To-Pro3 nucleic acid dye (Thermo FisherTM) was added and the slides were assembled in Mowiol (Sigma-AldrichTM, Brazil), analyzed and photographed.
For immunoassays, the serum from 253 patients from the Laboratório Central de Saúde Pública do Estado de Rondônia (Rondônia State Laboratory for Attention to Public Health, LACEN/RO) and the Banco Central de Sangue do Estado de Rondônia, Brazil (Rondônia State Central Blood Bank, FHEMERON/RO) was used. All serum was tested and used according to protocols approved by the Ethical Committee and Institutional Committee of Human/Animal Care and Use.
Samples were previously confirmed as positive for DENV IgM or IgG by IgM MAC-ELISA (Pan-BioTM, Australia) and Capture Duo IgM and IgG ELISA Kit (SanofiTM, EUA).
Recombinant NS1-DENV2 protein was used as an antigen coating to sensitize 96-well high-binding ELISA plates (JETBiofilTM, Korea) at a 1 μg/well concentration in a carbonate-bicarbonate buffer, pH 9.6. The patient serum samples were diluted 1/100, added in duplicates in the plates and incubated at 37°C for 60 min. Peroxidase-conjugated IgM (Sigma-AldrichTM, Brazil) and anti-human IgG (Sigma-AldrichTM, Brazil) secondary antibodies were added to the respective plates at 1/2500 dilution and incubated at 37°C for 60 min. After this incubation time, an ABTS substrate (2,2′-Azinobis [3-ethylbenzothiazoline-6-sulfonic acid]-diammonium salt) (Sigma-AldrichTM, Brazil) was added and reaction was blocked with a 2M solution of H2SO4. The absorbances were read by spectrophotometer (Multiskan GO, Thermo FisherTM) at a wavelength of 450 nm.
Receiver operating characteristic (ROC) curves were analyzed to estimate diagnostic cutoff, sensitivity and specificity using GraphPad Prism version 7.00 for Windows (GraphPad Software, United States). The mean of ELISA duplicates was used to determine the number of points in the curve (270 points for IgM and 222 points for IgG).
In this study, all experiments were performed with three biological replicates and three technical replicates per biological replicate.
Cloning and expression vectors pUC57_NS1DENV2 and pCAMBIA3301_empty were extracted from stored E. coli TOP10F to be digested by restriction enzymes Bgl II and BstE II (Supplementary Figures 1A,B). A NS1DENV2 fragment from pUC57_NS1DENV2 was inserted into pCAMBIA3301, forming pCAMBIA3301_NS1DENV2, which was transformed in E. coli TOP10F again and stored. In order to confirm cloning, a double digestion of the two clones – which grew in the presence of antibiotics – was performed (Supplementary Figure 1C).
The binary vector pCAMBIA3301_NS1DENV2 extracted from E. coli TOP10F was used to transform A. tumefaciens. Five Agrobacterium transformants were selected and confirmed by double digestion of the extracted plasmid DNA (Supplementary Figure 1D).
After A. thaliana WT germination and the appearance of lateral flowering shoots, the first cycle of the “floral-dip” method for plant genetic transformation was carried out. The procedure was repeated two more times, and the T0 plants (initial transformants) were kept in the growth chamber until the reproductive cycle was completed for maturation, drying of the siliqua and collection of the T1 seeds.
The first generation (T1) of transformed A. thaliana seeds was grown on a solid MS medium containing phosphinothricin (PPTR) as a selective agent, and the transformation efficiency was 3.59% (306 seeds plated – 11 seeds germinated).
The T1 seeds that germinated into healthy seedlings presented superior vegetative development, visually noticeable from the tenth day, compared to the seeds that could not develop vigorously (Figures 2A–E). Healthy seedlings had a green coloration, and physiological characteristics of the normal vegetative state of the A. thaliana. These differed from the other seeds that did not germinate or that gave rise to seedlings which did not develop and presented a yellowish coloration, with altered growth in vegetative state as a consequence of the herbicidal action.
Figure 2. Selection of Arabidopsis thaliana. (A) WT plant in MS medium without PPT; (B) WT plant in MS medium with PPT; (C–E) Germination of the A. thaliana transformed T1 generation (white arrow indicate seedlings with normal vegetative growth); (F) Transformed plants of generation T2; (G) Germination of 100% of the seeds in dominant homozygosis; (H) Transformed plants of generation T3 seeds; (I,J) Cultivation of the transformed plant until the production of seeds (60 days of age).
From seeds of the second generation, T2, a total of 11 seedlings were generated, which were transplanted to pots with a composite substrate and maintained in a growth chamber (Figure 2F). Of these 11 seedlings, nine reached the complete stage of the reproductive cycle with inflorescence formation, maturation and development of the embryos producing siliqua with seeds, around 60 days of age. Seeds of the second generation (T2) (nine plants) were collected and cultured in a solid MS medium with the phosphinothricin selective agent (PPTR).
From the last generation (T3) of cultivated A. thaliana, three plants (P1–P3) presented genetic segregation characteristic of dominant homozygosity, with approximately 100% seed growth (Figure 2G). These were grown until the production of seeds (Figures 2H–J). At the end of this period, these seeds were collected and stored at 4°C.
A transgenic plant (P3) producing the recombinant protein NS1DENV2 was submitted to total proteins extraction and purification by chromatography. SDS-PAGE electrophoresis analysis (Figure 3A) followed by western blotting (Figure 3B) was done using serum samples previously confirmed as positive for DENV infection. The immunolabeled band was around 50 kDa and was differentially expressed compared to A. thaliana WT. Quantification of total protein in crude extracts and the purified fraction was performed by BCA method (Table 1). The quantification of the recombinant protein yield was performed using a bovine serum albumin (BSA) to obtain a standard curve. From the curve, the pixel density was obtained for each concentration, using the ImageJ software1, and NS1 protein pixel density was interpolated with the standard curve. For each 1 g of fresh leaf used in the protein extraction, 1 mL of total proteins was obtained at the end of the purification process, solubilized in Tris–HCl buffer. Therefore, the yield obtained after purification on a nickel column was estimated using the densitometry approach to provide the 203 mg of NS1DENVV per kilogram of fresh leaf. The purity obtained was 76.58%, indicating the percentage of recombinant protein present in the sample after purification in a chromatographic column (Table 2 and Supplementary Figure 2).
Figure 3. Confirmation of genetic transformation of Arabidopsis thaliana. (A) Protein gel, SDS-PAGE 12% – (MM) molecular marker protein, (ce) crude extract, (p) purified fraction (black arrow head indicating NS1DENV2 protein) around 50 kDa, (Trans.) transformed and (WT) wild-type; (B) Western blot – (MM) molecular marker protein, (p) purified protein fraction, (Trans.) transformed and (WT) wild-type. Protein labeled with anti-dengue positive antibody in the fraction of the transformed plant. All experiments were performed with three biological replicates and three technical replicates per biological replicate.
Table 1. Total protein quantification and purification yield of leaf extracts from transformed and WT plants, using BCA method.
After 30 days of growth, a lower vegetative development was observed in transgenic plant P3 in relation to the WT plant, with a smaller rosette diameter and leaf area observed and a comparative dot-blot was made with the crude extract of each plant (Figures 4A,B). NS1DENV2 protein in situ immunolocalization in the P3 plant was done by confocal microscopy and compared to the wild plant (WT) (Figure 4C). Green fluorescent points were observed throughout the leaf tissue of the P3 plant in the form of oval protein bodies, which are clear evidence of the presence, expression and localization of NS1DENV2 protein within the plant cells of the transgenic A. thaliana. On the right side, in the wild type plant, green fluorescence was not detected, indicating the absence of immunostaining. Red dots observed are produced by the autofluorescence of chlorophyll present in chloroplasts which remained in the tissue after sample preparation (Figure 5).
Figure 4. Transformed plant vs. WT plant (28 days of age). (A) Dot blot; (B) Photo of the rosette in vegetative growth; (C) Confocal microscopy – Transformed (left bottom) and WT (right bottom). All experiments were performed with three biological replicates and three technical replicates per biological replicate.
Figure 5. Confocal microscopy. NS1DENV2 recombinant protein (green) spread throughout the leaf, labeled with FITC in the transformed plant, and in red the chlorophyll autofluorescence. All experiments were performed with three biological replicates and three technical replicates per biological replicate. (A) Transformed plant; (B) WT plant.
To detect IgM and IgG anti-dengue antibodies, the recombinant NS1DENV2 protein extracted from transgenic A. thaliana was used as the antigen in the tests, in exactly the same way as for the test plant. For anti-dengue IgM, 84.29% sensitivity, and 91.43% specificity were obtained with a cut-off value of 0.4737. For anti-dengue IgG, 83.08% sensitivity and 87.69% specificity were obtained with a cut-off of 0.4847 (Table 3). The graphs were reproduced from the ROC curve with 95% CI (confidence interval) and set the best cut-off for each assay (Figure 6). From the 253 anti-dengue serum samples (132 positives and 121 negatives), we demonstrated the numbers of false positives, false negatives, true positives, and true negatives (Table 4) for comparative evaluation. This survey was performed for each assay (IgM and IgG) using the samples tested on the transformed plant and WT plant.
Figure 6. Anti-dengue indirect ELISA, using purified plant protein as antigen to capture. (A) Anti-IgM and (B) Anti-IgG.
Table 4. Numbers of the true and false positive and negative samples according to the protein extract used (transformed and WT plants).
The results presented in this work are promising as a valid strategy for further studies to obtain protein on a large-scale and in an efficient form that can be used in the dengue serological tests. Confirmation of the NS1 gene cloning of DENV2 (NS1DENV2) in A. thaliana generated a functional transgenic clone, indicating that the insertion did not occur in key genes, rendering them inactive. In the first step, the gene was optimized for expression in plants, and was added to specific sequences to obtain higher levels of NS1DENV2 protein expression. Sequences added (Kozak sequence) in the expression cassette of the present work are involved in translation mechanisms and specific localization of proteins within the plant cells. The addition of small signal sequences (signal peptide, Bip and HDEL) in the cassette has the function of ensuring the address and retention of the protein in the rough endoplasmic reticulum, avoiding the protein degradation which would occur if these were free circulating in cytoplasm or an unwanted export to the outside of the cell (Benchabane et al., 2008; Pillay et al., 2014).
Wild type (WT) A. thaliana seeds were grown and the plants with the highest number of floral shoots were used for A. tumefaciens-mediated gene transformation containing the plasmid pCAMBIA3301_NS1DENV2. As in the work of Chaudhury et al. (2007); Khan et al. (2015); Schneider et al. (2015), the floral-dip method was repeated twice to ensure the transformation efficiency and a higher rate of transformants. From seeds obtained in the first generation, 306 were seeded on a plate with a selective medium and 11 germinated. The transformation efficiency obtained in this work was 3.59%, and agrees with the estimation of the original protocol described by Clough and Bent (1998), who determined an efficiency of up to 3%.
The yield obtained in this work, 203 mg of NS1DENV2 per kilogram of fresh leaf, showed that the plant expression system used was efficient and consistent with other systems aimed at optimizing the production of recombinant protein, which obtained, respectively, 70 mg/kg and 24.5 mg/kg (Fedosov et al., 2003; Rigano et al., 2004; Yang et al., 2015; Habibi et al., 2017; Okay and Sezgin, 2018). In their work, Jeong et al. (2018) demonstrated that it is possible to achieve overexpression of the mCherry protein (0.4 mg) per g of Arabidopsis callus. Another work using a transient expression method reached a higher yield, however, although transient expression does allow a higher yield, it has the disadvantage of the need for a recurrent transfection step (Garabagi et al., 2012). Some works present higher yields and different molecular strategies to increase the level of expression in plant hosts (Massa et al., 2018). Some highlights are 3.6 g/kg of 2G12 (monoclonal antibody against HIV) in A. thaliana (Loos et al., 2011); 4.5 g/kg of GAD65/67 (glutamic acid decarboxylase) in A. thaliana seeds (Morandini et al., 2011); 3.7 g/kg GFP in the fresh leaf of Nicotiana benthamiana (Yamamoto et al., 2018); among others that were covered in the comparative study of Merlin et al. (2014). In recent work, Diamos et al. (2020) optimized vectors expressing fluorescent proteins and obtained a yield of 3–5 g/kg of fresh leaf in N. benthamiana, being ∼50% of the amount of soluble proteins in the leaf. These same vectors were used to the expression of monoclonal antibodies and reached a yield between 1.2 and 1.4 g/kg. Recently, Marques et al. (2020) demonstrated the transient expression of the dengue virus NS1 protein in N. benthamiana for diagnostic purposes. Using an elastin-like polypeptide (ELP) as a fusion tag, the authors obtained 445 mg/kg yield, more than double that obtained in this work, but less than other works that used the transient expression approach.
The ELISA results obtained in the present study showed 84.29% sensitivity and 91.43% specificity for anti-dengue IgM, and 83.08% sensitivity and 87.69% specificity for anti-dengue IgG, with high ability to capture anti-dengue antibodies using NS1DENV2 recombinant protein. This result is consistent with other studies already published. The work published by Hunsperger et al. (2014) evaluated commercial diagnostic kits, resulting in anti-dengue IgM in ELISA with specificity of 78–91% and sensitivity 96–98%, including proving that anti-dengue IgM tests are less sensitive in secondary infections. The work of Lima et al. (2012) made the correlation between IgM and IgG reagents with primary dengue infections and subsequent reinfection. They showed that 38% of the primary infections are IgM positive and IgG negative (IgM+ / IgG−).
It is possible to observe a greater dispersion of IgG points in the sample with WT antigens. This occurs because it is a polyclonal primary serum, rich in immunoglobulins with convalescence phase isotypes (IgG), which treat the result as cross reactions which interfere with the specificity of the technique (Fairlie-Clarke et al., 2009). Specificity is the ability to recognize the antibody with its specific antigen, without cross-reactions with non-target molecules. And the expected is that other Flaviviruses antigens will not be recognized (Rathore and St John, 2020). However, we still obtained a good result compared to the works cited. Confocal microscopy analysis of A. thaliana showed recombinant NS1DENV2 protein localization in foliar tissue labeled with a fluorescent antibody. A primary antibody was used to recognize the polyhistidine tail present in the C-terminus of the recombinant protein. The labeling is evident and positive in the transgenic plant and negative in the wild-type, both receiving the same processing before the microscopic analysis. A difference in development was observed between the transgenic plant and the WT plant (Figure 4B), which suggest that at this point there is a physiological change related to the plant transformation, but that the clarification of this influence would need further investigation (Bent, 2000; Sung and Guy, 2003; Hwang et al., 2017).
Leaves observed from the transgenic clone showed a green fluorescent signal at concentrated points, but spread over the whole leaf. This analysis enabled the authors to hypothesize that the NS1DENV2 protein formed protein storage bodies, which suggest that it looks like a protein factory in the lumen of the RER, and this is in accordance with the results found in works by Stacey et al. (1999); Zang et al. (2009), who also used A. thaliana for protein expression and found these protein bodies dispersed in discrete subcellular localizations. In a study by Ishikawa et al. (2015), the expression of transgenic protein in tobacco leaves was shown, which also possessed the retention signal in the RER. Conley et al. (2011) reviewed different strategies for increasing the production of recombinant proteins within cellular subcompartments. Such strategies demonstrate that protein expression within the RER is formed from protein body structures (Joensuu et al., 2010; Gutiérrez et al., 2013; Saberianfar et al., 2016). The results of confocal microscopy demonstrated the formation of fluorescence focal points, consistent with the markings found in this work, reinforcing the previous hypothesis.
In a comprehensive study, Holtz et al. (2015) raised the point that rapid and large-scale manufacture can only be achieved by the technology of the plant-made pharmaceutical (PMP) production platform. Buyel et al. (2017) presented a general approach to scalable process models, in which small-scale models can be used to assess quality indicators quickly and economically, as a step which precedes the full development of a bio-factory in very-large scale. A variety of plants have been evaluated for the ability to produce recombinant proteins on an industrial scale. An ideal expression system must display a high-level of heterologous protein expression, while providing large biomass with an easy and fast growth profile, either in the greenhouse or in the field (Matoba et al., 2011; Xu et al., 2018). Nicotiana species present great potential for this purpose. They are widely used in the pre-production stages, in bench studies, and in mass production, both in stable expression systems and in transient systems (Sparrow and Twyman, 2009; Vancanneyt et al., 2009). Sheludko et al. (2007) compared different species of Nicotiana (N. benthamiana, Nicotiana debneyi, Nicotiana excelsior, Nicotiana exigua, Nicotiana maritima, and Nicotiana simulans) on the production capacity and concluded that N. excelsior had the best features in biomass terms.
This study demonstrated that plant antigen production to detect anti-dengue antibodies is a promising alternative to mouse brain and cell culture production of the antigens for diagnostic kits. And it could contribute to the development of future investigations for a rapid test which captures early anti-NS1 antibodies circulating in patients suspected of having this disease. The high yield and low cost for production of the recombinant protein are attractive aspects for commercialization, and thus this technique is a start for the manufacture of diagnostic kits in scalable process models.
The raw data supporting the conclusions of this article will be made available by the authors, without undue reservation.
MX: formal analysis, investigation, methodology, validation, writing – original draft, and writing – review and editing. RD: supervision, visualization, writing – original draft, and writing – review and editing. EF-A and JP: investigation and methodology. CS: resources, supervision, visualization, writing – original draft, and writing – review and editing. SP: project administration, resources, supervision, visualization, writing – original draft, and writing – review and editing. All authors contributed to the article and approved the submitted version.
The authors declare that the research was conducted in the absence of any commercial or financial relationships that could be construed as a potential conflict of interest.
The authors are grateful to LACEN/RO (state of Rondônia, Brazil) for serum sample donation. And to FAPEMIG, CNPq, and CAPES for the financial support.
The Supplementary Material for this article can be found online at: https://www.frontiersin.org/articles/10.3389/fpls.2020.581100/full#supplementary-material
Avirutnan, P., Fuchs, A., Hauhart, R. E., Somnuke, P., Youn, S., Diamond, M. S., et al. (2010). Antagonism of the complement component C4 by flavivirus nonstructural protein NS1. J. Exp. Med. 207, 793–806. doi: 10.1084/jem.20092545
Barta, A., Sommergruber, K., Thompson, D., Hartmuth, K., Matzke, M. A., and Matzke, A. J. (1986). The expression of a nopaline synthase – human growth hormone chimaeric gene in transformed tobacco and sunflower callus tissue. Plant Mol. Biol. 6, 347–357. doi: 10.1007/bf00034942
Benchabane, M., Goulet, C., Rivard, D., Faye, L., Gomord, V., and Michaud, D. (2008). Preventing unintended proteolysis in plant protein biofactories. Plant Biotechnol. J. 6, 633–648. doi: 10.1111/j.1467-7652.2008.00344.x
Bent, A. F. (2000). Arabidopsis in planta transformation. Uses, mechanisms, and prospects for transformation of other species. Plant Physiol. 124, 1540–1547. doi: 10.1104/pp.124.4.1540
Bhatt, S., Gething, P. W., Brady, O. J., Messina, J. P., Farlow, A. W., Moyes, C. L., et al. (2013). The global distribution and burden of dengue. Nature 496, 504–507.
Bock, R., and Warzecha, H. (2010). Solar-powered factories for new vaccines and antibiotics. Trends Biotechnol. 28, 246–252. doi: 10.1016/j.tibtech.2010.01.006
Buyel, J. F., Twyman, R. M., and Fischer, R. (2017). Very-large-scale production of antibodies in plants: the biologization of manufacturing. Biotechnol. Adv. 35, 458–465. doi: 10.1016/j.biotechadv.2017.03.011
Chang, S. S., Park, S. K., Kim, B. C., Kang, B. J., Kim, D. U., and Nam, H. (1994). Stable genetic transformation of Arabidopsis thaliana by Agrobacterium inoculation in planta. Plant J. 5, 551–558. doi: 10.1046/j.1365-313x.1994.5040551.x
Chaudhury, D., Madanpotra, S., Jaiwal, R., Saini, R., Kumar, P. A., and Jaiwal, P. K. (2007). Agrobacterium tumefaciens-mediated high frequency genetic transformation of an Indian cowpea (Vigna unguiculata L. Walp.) cultivar and transmission of transgenes into progeny. Plant Sci. 172, 692–700. doi: 10.1016/j.plantsci.2006.11.009
Chuang, Y. C., Wang, S. Y., Lin, Y. S., Chen, H. R., and Yeh, T. M. (2013). Re-evaluation of the pathogenic roles of nonstructural protein 1 and its antibodies during dengue virus infection. J. Biomed. Sci. 20:42. doi: 10.1186/1423-0127-20-42
Clough, S. J., and Bent, A. F. (1998). Floral dip: a simplified method for Agrobacterium−mediated transformation of Arabidopsis thaliana. Plant J. 16, 735–743. doi: 10.1046/j.1365-313x.1998.00343.x
Commandeur, U., Twyman, R. M., and Fischer, R. (2003). The biosafety of molecular farming in plants. Biosaf. Mol. Farm. Plants 5, 1–9. doi: 10.1007/978-94-007-2217-0_1
Conley, A. J., Joensuu, J. J., Richman, A., and Menassa, R. (2011). Protein body−inducing fusions for high−level production and purification of recombinant proteins in plants. Plant Biotechnol. J. 9, 419–433. doi: 10.1111/j.1467-7652.2011.00596.x
Demain, A. L., and Vaishnav, P. (2009). Production of recombinant proteins by microbes and higher organisms. Biotechnol. Adv. 27, 297–306. doi: 10.1016/j.biotechadv.2009.01.008
Diamos, A. G., Hunter, J. G., Pardhe, M. D., Rosenthal, S. H., Sun, H., Foster, B. C., et al. (2020). High level production of monoclonal antibodies using an optimized plant expression system. Front. Bioeng. Biotechnol. 7:472. doi: 10.3389/fbioe.2019.00472
Dutra, N. R., De Paula, M. B., De Oliveira, M. D., De Oliveira, L. L., and De Paula, S. O. (2009). The laboratorial diagnosis of dengue: applications and implications. J. Glob. Infect. Dis. 1, 38–44. doi: 10.4103/0974-777x.52980
Edwards, K., Johnstone, C., and Thompson, C. (1991). A simple and rapid method for the preparation of plant genomic DNA for PCR analysis. Nucleic Acids Res. 19:1349. doi: 10.1093/nar/19.6.1349
Fairlie-Clarke, K. J., Shuker, D. M., and Graham, A. L. (2009). Why do adaptive immune responses cross-react? Evol. Appl. 2, 122–131. doi: 10.1111/j.1752-4571.2008.00052.x
Fatima, A., and Wang, J. (2015). Progress in the diagnosis of dengue virus infections and importance of point of care test: a review. Pak. J. Pharm. Sci. 28, 271–280.
Fauci, A. S., and Morens, D. M. (2012). The perpetual challenge of infectious diseases. N. Engl. J. Med. 366, 454–461. doi: 10.1056/nejmra1108296
Fedosov, S. N., Laursen, N. B., Nexø, E., Moestrup, S. K., Petersen, T. E., Jensen, E. Ø., et al. (2003). Human intrinsic factor expressed in the plant Arabidopsis thaliana. Eur. J. Biochem. 270, 3362–3367.
Feldmann, K. A., and Marks, M. D. (1987). Agrobacterium-mediated transformation of germinating seeds of Arabidopsis thaliana: a non-tissue culture approach. Mol. Gen. Genet. 208, 1–9. doi: 10.1007/bf00330414
Garabagi, F., McLean, M. D., and Hall, J. C. (2012). “Transient and stable expression of antibodies in Nicotiana species,” in Antibody Engineering (Totowa, NJ: Humana Press), 389–408.
Gomord, V., Fitchette, A. C., Menu-Bouaouiche, L., Saint-Jore-Dupas, C., Plasson, C., Michaud, D., et al. (2010). Plant-specific glycosylation patterns in the context of therapeutic protein production. Plant Biotechnol. J. 8, 564–587. doi: 10.1111/j.1467-7652.2009.00497.x
Groen, J., Koraka, P., Velzing, J., Copra, C., and Osterhaus, A. D. (2000). Evaluation of six immunoassays for detection of dengue virus-specific immunoglobulin M and G antibodies. Clin. Diagn. Lab. Immunol. 7, 867–871. doi: 10.1128/cdli.7.6.867-871.2000
Guerrero, N. A. S., and Bello, F. J. (2019). Comparative assessment of the replication efficiency of dengue, yellow fever, and chikungunya arboviruses in some insect and mammalian cell lines. Revist. Soc. Bras. Med. Trop. 52:e20180511.
Gutiérrez, S. P., Saberianfar, R., Kohalmi, S. E., and Menassa, R. (2013). Protein body formation in stable transgenic tobacco expressing elastin-like polypeptide and hydrophobin fusion proteins. BMC Biotechnol. 13:40. doi: 10.1186/1472-6750-13-40
Guy, B., Briand, O., Lang, J., Saville, M., and Jackson, N. (2015). Development of the Sanofi Pasteur tetravalent dengue vaccine: one more step forward. Vaccine 33, 7100–7111. doi: 10.1016/j.vaccine.2015.09.108
Habibi, P., Prado, G. S., Pelegrini, P. B., Hefferon, K. L., Soccol, C. R., and Grossi-De-Sa, M. F. (2017). Optimization of inside and outside factors to improve recombinant protein yield in plant. Plant Cell Tissue Organ Cult. 130, 449–467. doi: 10.1007/s11240-017-1240-5
Heinz, F. X., and Stiasny, K. (2012). Flaviviruses and their antigenic structure. J. Clin. Virol. 55, 289–295. doi: 10.1016/j.jcv.2012.08.024
Hochuli, E., Döbeli, H., and Schacher, A. (1987). New metal chelate adsorbent selective for proteins and peptides containing neighbouring histidine residues. J. Chromatogr. A 411, 177–184. doi: 10.1016/s0021-9673(00)93969-4
Holtz, B. R., Berquist, B. R., Bennett, L. D., Kommineni, V. J., Munigunti, R. K., White, E. L., et al. (2015). Commercial−scale biotherapeutics manufacturing facility for plant−made pharmaceuticals. Plant Biotechnol. J. 13, 1180–1190. doi: 10.1111/pbi.12469
Hunsperger, E. A., Yoksan, S., Buchy, P., Nguyen, V. C., Sekaran, S. D., Enria, D. A., et al. (2014). Evaluation of commercially available diagnostic tests for the detection of dengue virus NS1 antigen and anti-dengue virus IgM antibody. PLoS Negl. Trop. Dis. 8:e3171. doi: 10.1371/journal.pntd.0003171
Hwang, H. H., Yu, M., and Lai, E. M. (2017). Agrobacterium-mediated plant transformation: biology and applications. Arabidopsis Book 15:e0186. doi: 10.1199/tab.0186
Iacobucci, G. (2018). WHO recommends additional tests for Sanofi’s dengue vaccine after safety concerns. BMJ 361:k1765. doi: 10.1136/bmj.k1765
Ishikawa, K., Miura, C., Maejima, K., Komatsu, K., Hashimoto, M., Tomomitsu, T., et al. (2015). Nucleocapsid protein from fig mosaic virus forms cytoplasmic agglomerates that are hauled by endoplasmic reticulum streaming. J. Virol. 89, 480–491. doi: 10.1128/jvi.02527-14
Jeong, I. S., Lee, S., Bonkhofer, F., Tolley, J., Fukudome, A., Nagashima, Y., et al. (2018). Purification and characterization of Arabidopsis thaliana oligosaccharyltransferase complexes from the native host: a protein super−expression system for structural studies. Plant J. 94, 131–145. doi: 10.1111/tpj.13847
Joensuu, J. J., Conley, A. J., Lienemann, M., Brandle, J. E., Linder, M. B., and Menassa, R. (2010). Hydrophobin fusions for high-level transient protein expression and purification in Nicotiana benthamiana. Plant Physiol. 152, 622–633. doi: 10.1104/pp.109.149021
Katzelnick, L. C., Coloma, J., and Harris, E. (2017). Dengue: knowledge gaps, unmet needs, and research priorities. Lancet Infect. Dis. 17, e88–e100.
Khan, K., Agarwal, P., Shanware, A., and Sane, V. (2015). Heterologous expression of two Jatropha aquaporins imparts drought and salt tolerance and improves seed viability in transgenic Arabidopsis thaliana. PLoS One 10:e0128866. doi: 10.1371/journal.pone.0128866
Kim, H. S., Jeon, J. H., Lee, K. J., and Ko, K. (2014). N-glycosylation modification of plant-derived virus-like particles: an application in vaccines. Biomed Res. Int. 2014:249519.
Ko, K. (2014). Expression of recombinant vaccines and antibodies in plants. Monoclon. Antib. Immunodiagn. Immunother. 33, 192–198. doi: 10.1089/mab.2014.0049
Koizumi, N. (1996). Isolation and responses to stress of a gene that encodes a luminal binding protein in Arabidopsis thaliana. Plant Cell Physiol. 37, 862–865. doi: 10.1093/oxfordjournals.pcp.a029023
Kozak, M. (1978). How do eucaryotic ribosomes select initiation regions in messenger RNA? Cell 15, 1109–1123. doi: 10.1016/0092-8674(78)90039-9
Kozak, M. (2002). Pushing the limits of the scanning mechanism for initiation of translation. Gene 299, 1–34. doi: 10.1016/s0378-1119(02)01056-9
Lacroix, B., and Citovsky, V. (2013). The roles of bacterial and host plant factors in Agrobacterium-mediated genetic transformation. Int. J. Dev. Biol. 57, 467–481. doi: 10.1387/ijdb.130199bl
Lee, J., Lee, H., Kim, J., Lee, S., Kim, D. H., Kim, S., et al. (2011). Both the hydrophobicity and a positively charged region flanking the C-terminal region of the transmembrane domain of signal-anchored proteins play critical roles in determining their targeting specificity to the endoplasmic reticulum or endosymbiotic organelles in Arabidopsis cells. Plant Cell 23, 1588–1607. doi: 10.1105/tpc.110.082230
Libraty, D. H., Young, P. R., Pickering, D., Endy, T. P., Kalayanarooj, S., Green, S., et al. (2002). High circulating levels of the dengue virus nonstructural protein NS1 early in dengue illness correlate with the development of dengue hemorrhagic fever. J. Infect. Dis. 186, 1165–1168. doi: 10.1086/343813
Lima, J. R. C., Rouquayrol, M. Z., Callado, M. R. M., Guedes, M. I. F., and Pessoa, C. (2012). Interpretation of the presence of IgM and IgG antibodies in a rapid test for dengue: analysis of dengue antibody prevalence in Fortaleza City in the 20th year of the epidemic. Rev. Soc. Bras. Med. Trop. 45, 163–167. doi: 10.1590/s0037-86822012000200005
Lindenbach, B. D., and Rice, C. (2003). Molecular biology of flaviviruses. Adv. Virus Res. 59, 23–62. doi: 10.1016/s0065-3527(03)59002-9
Lloyd, A. M., Barnason, A. R., Rogers, S. G., Byrne, M. C., Fraley, R. T., and Horsch, R. B. (1986). Transformation of Arabidopsis thaliana with Agrobacterium tumefaciens. Science 234, 464–466.
Loos, A., Van Droogenbroeck, B., Hillmer, S., Grass, J., Kunert, R., Cao, J., et al. (2011). Production of monoclonal antibodies with a controlled N−glycosylation pattern in seeds of Arabidopsis thaliana. Plant Biotechnol. J. 9, 179–192. doi: 10.1111/j.1467-7652.2010.00540.x
Mardekian, S. K., and Roberts, A. L. (2015). Diagnostic options and challenges for Dengue and Chikungunya viruses. Biomed Res. Int. 2015:834371.
Marques, L. ÉC., Silva, B. B., Dutra, R. A. F., Florean, E. O. P. T., Menassa, R., and Guedes, M. I. F. (2020). Transient expression of dengue virus NS1 antigen in Nicotiana benthamiana for use as a diagnostic antigen. Front. Plant Sci. 10:1674. doi: 10.3389/fpls.2019.01674
Mason, H. S., Lam, D. M., and Arntzen, C. J. (1992). Expression of hepatitis B surface antigen in transgenic plants. Proc. Natl. Acad. Sci. U.S.A. 89, 11745–11749.
Massa, S., Presenti, O., and Benvenuto, E. (2018). “Engineering plants for the future: farming with value-added harvest,” in Progress in Botany, Vol. 80, (Cham: Springer), 65–108. doi: 10.1007/124_2018_20
Matoba, N., Davis, K. R., and Palmer, K. E. (2011). “Recombinant protein expression in Nicotiana,” in Plant Chromosome Engineering, ed. J. Birchler (Totowa, NJ: Humana Press), 199–219. doi: 10.1007/978-1-61737-957-4_11
McCormac, A. C., Elliott, M. C., and Chen, D. F. (1998). A simple method for the production of highly competent cells of Agrobacterium for transformation via electroporation. Mol. Biotechnol. 9, 155–159. doi: 10.1007/bf02760816
Merlin, M., Gecchele, E., Capaldi, S., Pezzotti, M., and Avesani, L. (2014). Comparative evaluation of recombinant protein production in different biofactories: the green perspective. BioMed Res. Int. 2014:136419.
Mett, V., Farrance, C. E., Green, B. J., and Yusibov, V. (2008). Plants as biofactories. Biologicals 36, 354–358. doi: 10.1016/j.biologicals.2008.09.001
Modhiran, N., Watterson, D., Blumenthal, A., Baxter, A. G., Young, P. R., and Stacey, K. J. (2017). Dengue virus NS1 protein activates immune cells via Tlr4 but not Tlr2 or Tlr6. Immunol. Cell Biol. 95, 491–495. doi: 10.1038/icb.2017.5
Morandini, F., Avesani, L., Bortesi, L., Van Droogenbroeck, B., De Wilde, K., Arcalis, E., et al. (2011). Non−food/feed seeds as biofactories for the high−yield production of recombinant pharmaceuticals. Plant Biotechnol. J. 9, 911–921. doi: 10.1111/j.1467-7652.2011.00605.x
Morens, D. M., and Fauci, A. S. (2013). Emerging infectious diseases: threats to human health and global stability. PLoS Pathog. 9:e1003467. doi: 10.1371/journal.ppat.1003467
Muller, D. A., Depelsenaire, A. C., and Young, P. R. (2017). Clinical and laboratory diagnosis of dengue virus infection. J. Infect. Dis. 215, S89–S95.
Muller, D. A., and Young, P. R. (2013). The flavivirus NS1 protein: molecular and structural biology, immunology, role in pathogenesis and application as a diagnostic biomarker. Antiviral Res. 98, 192–208. doi: 10.1016/j.antiviral.2013.03.008
Munro, S., and Pelham, H. R. (1987). A C-terminal signal prevents secretion of luminal ER proteins. Cell 48, 899–907. doi: 10.1016/0092-8674(87)90086-9
Murashige, T., and Skoog, F. (1962). A revised medium for rapid growth and bio assays with tobacco tissue cultures. Physiol. Plant. 15, 473–497. doi: 10.1111/j.1399-3054.1962.tb08052.x
Nakagawa, S., Niimura, Y., Gojobori, T., Tanaka, H., and Miura, K. (2008). Diversity of preferred nucleotide sequences around the translation initiation codon in eukaryote genomes. Nucleic Acids Res. 36, 861–871. doi: 10.1093/nar/gkm1102
Napier, R. M., Fowke, L. C., Hawes, C., Lewis, M., and Pelham, H. R. (1992). Immunological evidence that plants use both HDEL and KDEL for targeting proteins to the endoplasmic reticulum. J. Cell Sci. 102, 261–271.
Noisakran, S., Dechtawewat, T., Avirutnan, P., Kinoshita, T., Siripanyaphinyo, U., Puttikhunt, C., et al. (2008). Association of dengue virus NS1 protein with lipid rafts. J. Gen. Virol. 89, 2492–2500. doi: 10.1099/vir.0.83620-0
Obembe, O. O., Popoola, J. O., Leelavathi, S., and Reddy, S. V. (2011). Advances in plant molecular farming. Biotechnol. Adv. 29, 210–222. doi: 10.1016/j.biotechadv.2010.11.004
Okay, S., and Sezgin, M. (2018). Transgenic plants for the production of immunogenic proteins. AIMS Bioeng. 5:151. doi: 10.3934/bioeng.2018.3.151
Pal, S., Dauner, A. L., Mitra, I., Forshey, B. M., Garcia, P., Morrison, A. C., et al. (2014). Evaluation of dengue NS1 antigen rapid tests and ELISA kits using clinical samples. PLoS One 9:e113411. doi: 10.1371/journal.pone.0113411
Pan American Health Organization (2019). Dengue: Reported Cases. Washington, DC: Pan American Health Organization.
Pang, J., Chia, P. Y., Lye, D. C., and Leo, Y. S. (2017). Progress and challenges towards point-of-care diagnostic development for dengue. J. Clin. Microbiol. 55, 3339–3349. doi: 10.1128/jcm.00707-17
Peeling, R. W., Artsob, H., Pelegrino, J. L., Buchy, P., Cardosa, M. J., Devi, S., et al. (2010). Evaluation of diagnostic tests: dengue. Nat. Rev. Microbiol. 8, S30–S38.
Pillay, P., Schlüter, U., Van Wyk, S., Kunert, K. J., and Vorster, B. J. (2014). Proteolysis of recombinant proteins in bioengineered plant cells. Bioengineered 5, 15–20. doi: 10.4161/bioe.25158
Pitzschke, A. (2013). Agrobacterium infection and plant defense-transformation success hangs by a thread. Front. Plant Sci. 4:519. doi: 10.3389/fpls.2013.00519
Pryor, M. J., and Wright, P. J. (1994). Glycosylation mutants of dengue virus NS1 protein. J. Gen. Virol. 75(Pt 5), 1183–1187. doi: 10.1099/0022-1317-75-5-1183
Rastogi, M., Sharma, N., and Singh, S. K. (2016). Flavivirus NS1: a multifaceted enigmatic viral protein. Virol. J. 13:131.
Rathore, A. P., and St John, A. L. (2020). Cross-reactive immunity among flaviviruses. Front. Immunol. 11:334. doi: 10.3389/fimmu.2020.00334
Rigano, M., Alvarez, M., Pinkhasov, J., Jin, Y., Sala, F., Arntzen, C., et al. (2004). Production of a fusion protein consisting of the enterotoxigenic Escherichia coli heat-labile toxin B subunit and a tuberculosis antigen in Arabidopsis thaliana. Plant Cell Rep. 22, 502–508. doi: 10.1007/s00299-003-0718-2
Saberianfar, R., Sattarzadeh, A., Joensuu, J. J., Kohalmi, S. E., and Menassa, R. (2016). Protein bodies in leaves exchange contents through the endoplasmic reticulum. Front. Plant Sci. 7:693. doi: 10.3389/fpls.2016.00693
Sauer, M., Paciorek, T., Benkova, E., and Friml, J. (2006). Immunocytochemical techniques for whole-mount in situ protein localization in plants. Nat. Protoc. 1, 98–103. doi: 10.1038/nprot.2006.15
Schillberg, S., Raven, N., Fischer, R., Twyman, R. M., and And Schiermeyer, A. (2013). Molecular farming of pharmaceutical proteins using plant suspension cell and tissue cultures. Curr. Pharm. Des. 19, 5531–5542. doi: 10.2174/1381612811319310008
Schillberg, S., Raven, N., Spiegel, H., Rasche, S., and Buntru, M. (2019). Critical analysis of the commercial potential of plants for the production of recombinant proteins. Front. Plant Sci. 10:720. doi: 10.3389/fpls.2019.00720
Schneider, J., Castilho, A., Pabst, M., Altmann, F., Gruber, C., Strasser, R., et al. (2015). Characterization of plants expressing the human β1, 4-galactosyltrasferase gene. Plant Physiol. Biochem. 92, 39–47. doi: 10.1016/j.plaphy.2015.04.010
Sheludko, Y. V., Sindarovska, Y. R., Gerasymenko, I. M., Bannikova, M. A., and Kuchuk, N. V. (2007). Comparison of several Nicotiana species as hosts for high−scale Agrobacterium−mediated transient expression. Biotechnol. Bioeng. 96, 608–614. doi: 10.1002/bit.21075
Shrivastava, A., Tripathi, N. K., Dash, P. K., and Parida, M. (2017). Working towards dengue as a vaccine-preventable disease: challenges and opportunities. Expert Opin. Biol. Ther. 17, 1193–1199. doi: 10.1080/14712598.2017.1356284
Shu, P. Y., Yang, C. F., Kao, J. F., Su, C. L., Chang, S. F., Lin, C. C., et al. (2009). Application of the dengue virus NS1 antigen rapid test for on-site detection of imported dengue cases at airports. Clin. Vaccine Immunol. 16, 589–591. doi: 10.1128/cvi.00475-08
Simmonds, P., Becher, P., Bukh, J., Gould, E. A., Meyers, G., Monath, T., et al. (2017). Ictv virus taxonomy profile: flaviviridae. J. Gen. Virol. 98, 2–3. doi: 10.1099/jgv.0.000672
Sparrow, P. A., and Twyman, R. M. (2009). Biosafety, risk assessment and regulation of plant-made pharmaceuticals. Methods Mol. Biol. 483, 341–353. doi: 10.1007/978-1-59745-407-0_20
Spiegel, H., Boes, A., Voepel, N., Beiss, V., Edgue, G., Rademacher, T., et al. (2015). Application of a scalable plant transient gene expression platform for malaria vaccine development. Front. Plant Sci. 6:1169. doi: 10.3389/fpls.2015.01169
Stacey, M. G., Hicks, S. N., and Von Arnim, A. G. (1999). Discrete domains mediate the light-responsive nuclear and cytoplasmic localization of Arabidopsis COP1. Plant Cell 11, 349–363. doi: 10.1105/tpc.11.3.349
Sung, D. Y., and Guy, C. L. (2003). Physiological and molecular assessment of altered expression of Hsc70-1 in Arabidopsis. Evidence for pleiotropic consequences. Plant Physiol. 132, 979–987. doi: 10.1104/pp.102.019398
Terpe, K. (2003). Overview of tag protein fusions: from molecular and biochemical fundamentals to commercial systems. Appl. Microbiol. Biotechnol. 60, 523–533. doi: 10.1007/s00253-002-1158-6
Tuan, N. M., Nhan, H. T., Chau, N. V., Hung, N. T., Tuan, H. M., Tram, T. V., et al. (2015). Sensitivity and specificity of a novel classifier for the early diagnosis of dengue. PLoS Negl. Trop. Dis. 9:e0003638. doi: 10.1371/journal.pntd.0003638
Twyman, R. M., Stoger, E., Schillberg, S., Christou, P., and And Fischer, R. (2003). Molecular farming in plants: host systems and expression technology. Trends Biotechnol. 21, 570–578. doi: 10.1016/j.tibtech.2003.10.002
Vancanneyt, G., Dubald, M., Schröder, W., Peters, J., and Botterman, J. (2009). “A case study for plant-made pharmaceuticals comparing different plant expression and production systems,” in Recombinant Proteins From Plants, eds L. Faye and V. Gomord (Totowa, NJ: Humana Press), 209–221. doi: 10.1007/978-1-59745-407-0_12
Vojta, L., Ljuma-Skupnjak, L., Budimir, A., Vukicevic, S., and Fulgosi, H. (2015). Rapid transient expression of human granulocyte-macrophage colony-stimulating factor in two industrial cultivars of tobacco (Nicotiana tabacum L.) by agroinfiltration. Biotechnol. Rep. 7, 81–86. doi: 10.1016/j.btre.2015.05.006
Von Schaewen, A., Jeong, I. S., Rips, S., Fukudome, A., Tolley, J., Nagashima, Y., et al. (2018). Improved recombinant protein production in Arabidopsis thaliana. Plant Signal. Behav. 13:e1486149. doi: 10.1080/15592324.2018.1486149
Wang, D., Zheng, Y., Kang, X., Zhang, X., Hao, H., Chen, W., et al. (2015). A multiplex Elisa-based protein array for screening diagnostic antigens and diagnosis of Flaviviridae infection. Eur. J. Clin. Microbiol. Infect. Dis. 34, 1327–1336. doi: 10.1007/s10096-015-2353-6
Wiemer, D., Frickmann, H., and Kruger, A. (2017). Dengue fever: symptoms, epidemiology, entomology, pathogen diagnosis and prevention. Hautarzt 68, 1011–1020.
Winkler, G., Randolph, V. B., Cleaves, G. R., Ryan, T. E., and Stollar, V. (1988). Evidence that the mature form of the flavivirus nonstructural protein NS1 is a dimer. Virology 162, 187–196. doi: 10.1016/0042-6822(88)90408-4
Xu, J., Towler, M., and Weathers, P. J. (2018). “Platforms for plant-based protein production,” in Bioprocessing of Plant in Vitro Systems, eds A. Pavlov and T. Bley (Cham: Springer), 509. doi: 10.1007/978-3-319-54600-1_14
Yamamoto, T., Hoshikawa, K., Ezura, K., Okazawa, R., Fujita, S., Takaoka, M., et al. (2018). Improvement of the transient expression system for production of recombinant proteins in plants. Sci. Rep. 8, 1–10.
Yang, J., Guan, L., Guo, Y., Du, L., Wang, F., Wang, Y., et al. (2015). Expression of biologically recombinant human acidic fibroblast growth factor in Arabidopsis thaliana seeds via oleosin fusion technology. Gene 566, 89–94.
Keywords: dengue, NS1 protein, Arabidopsis thaliana, diagnosis 2, plant expression
Citation: Xisto MF, Dias RS, Feitosa-Araujo E, Prates JWO, da Silva CC and de Paula SO (2020) Efficient Plant Production of Recombinant NS1 Protein for Diagnosis of Dengue. Front. Plant Sci. 11:581100. doi: 10.3389/fpls.2020.581100
Received: 07 July 2020; Accepted: 02 October 2020;
Published: 26 October 2020.
Edited by:
Nobuyuki Matoba, University of Louisville, United StatesReviewed by:
Johannes Felix Buyel, Fraunhofer Society (FhG), GermanyCopyright © 2020 Xisto, Dias, Feitosa-Araujo, Prates, da Silva and de Paula. This is an open-access article distributed under the terms of the Creative Commons Attribution License (CC BY). The use, distribution or reproduction in other forums is permitted, provided the original author(s) and the copyright owner(s) are credited and that the original publication in this journal is cited, in accordance with accepted academic practice. No use, distribution or reproduction is permitted which does not comply with these terms.
*Correspondence: Sérgio Oliveira de Paula, ZGVwYXVsYUB1ZnYuYnI=
Disclaimer: All claims expressed in this article are solely those of the authors and do not necessarily represent those of their affiliated organizations, or those of the publisher, the editors and the reviewers. Any product that may be evaluated in this article or claim that may be made by its manufacturer is not guaranteed or endorsed by the publisher.
Research integrity at Frontiers
Learn more about the work of our research integrity team to safeguard the quality of each article we publish.