- Department of Viticulture and Enology, University of California, Davis, Davis, CA, United States
Rising temperatures in most agricultural regions of the world are associated with a higher incidence of extreme weather events such as heat waves. We performed an experiment to mitigate the impact of heat waves and exposure of berries in grapevine (Vitis vinifera cv. “Cabernet Sauvignon”) with untreated vines (Exposed) or with fruit-zone partial shading (Shaded) under 40 and 80% replacement of crop evapotranspiration (ETc) with sustained deficit irrigation in a factorially arranged experiment. The trial was performed in a vineyard with vertically shoot positioned trellis with a row orientation that concentrated solar radiation exposure on the southwest aspect of the fruit zone. Leaf stomatal conductance (gs) and net carbon assimilation (AN) were significantly lower in shaded leaves under partial fruit-zone shading that resulted in lower pruning mass for Shaded treatments. Stem water potential (Ψstem) responded to a large extent to increased irrigation. However, grapevines with partial fruit-zone shading had transiently better water status under 40% ETc. Cluster maximum temperatures were 3.9°C greater in Exposed grapevines. Exposed clusters had transiently lower acidity and higher pH. However, Exposed clusters on 40% ETc had higher total soluble solids (TSS). The experimental vineyard suffered a 4-day heat wave 21 days before harvest, resulting in 25% of the clusters being damaged in Exposed treatment, regardless of irrigation amount. Furthermore, berries in Exposed treatments suffered a great loss of anthocyanins and flavonols even if they were not damaged by direct solar exposure. The pre-planting decision of using a vertically shoot positioned trellis that concentrated solar radiation on the Southwest aspect offered mild protection in a hot climate region with a sunny growing season with extreme heat events during the execution of study. The extreme conditions under which this study was conducted are not unusual, and have become more expected. Our work provided evidence of the vulnerability of grape berry to heat waves and exposure during heat wave events and possible protection methods to mitigate these effects in situ in context of climate change.
Introduction
The commercial success of a grape growing region is based on a fine-tuned match between the climate and cultivar and rootstock selection (Ollat et al., 2016), and it has to be combined with an adequate market demand. By the middle of twenty-first century, climatic conditions are expected to change potentially affecting key physiological and production parameters (Hannah et al., 2013; Fraga et al., 2016). The increase in atmospheric CO2 and other greenhouse gasses most certainly will increase the temperature of the planet ranging from 1.5 to 4.5°C (IPCC, 2013). Furthermore, the incidence of extreme events, such as heat waves, is increasing with an associated risk for crops (Fischer and Schär, 2010; Smith, 2011; Deryng et al., 2014; Martínez-Lüscher et al., 2017b). Higher temperatures are associated with greater rates evaporation of water and therefore, higher global precipitation. However, these are unevenly distributed. In fact, most regions where grapevines are grown are forecasted to experience a reduction in cloud coverage and rainfall and an increase in solar radiation reaching the earth’s surface (Trenberth and Fasullo, 2009).
Grapevine is a rather resilient perennial crop, tolerating long periods of drought and extreme temperatures. However, as in many other fleshy fruits, grape berry is sensitive to exposure to solar radiation, causing damage on the surface of the fruit or even fruit abortion (Tinyane et al., 2018; Torres et al., 2020). Fruit exposure to solar radiation was highlighted for decades as a key factor to enhance ripening of fruits and their composition and is a very relevant concept for cultural practices in grapes (Jackson and Lombard, 1993; Cook et al., 2015). Fruit zone leaf removal in dense canopies can promote ripening and synthesis of flavonoids such as anthocyanins and flavonols (Pastore et al., 2013). Under controlled conditions, a combination of visible and UV radiation may upregulate structural and regulatory genes responsible for the synthesis of anthocyanins and flavonols (Azuma et al., 2012). These photomorphogenic effects are mediated by photoreceptors, phytochromes and cryptochromes and are responsive to changes in radiation spectra (González et al., 2015; Matus, 2016). However, solar radiation, especially infrared, transmits thermal energy to exposed objects with an intrinsic increase in their temperature. High temperature may play repressive role in the synthesis of anthocyanins, eventually inducing their degradation (Mori et al., 2007; Martínez-Lüscher et al., 2017b; Torres et al., 2020). In addition, high temperatures may be responsible for a desynchronization between the accumulation of sugars and anthocyanins leading to lower anthocyanin contents at harvest (Sadras and Moran, 2012).
In semiarid climates, where growing season rainfall is not enough to sustain production, grapevines are grown with supplemental irrigation. Mild water deficits may enhance grape ripening, through the re-concentration of the berry contents or improved grape composition through the synthesis of stress-related metabolites (Chaves et al., 2010; Kuhn et al., 2014). However, excessive water deficits in hot climates may lead to deleterious effect on fruit quality (Brillante et al., 2017; Martínez-Lüscher et al., 2017a; Yu and Kurtural, 2020; Yu et al., 2020). High temperatures may exacerbate water deficits by increasing vapor pressure deficit, thus increasing evapotranspiration. Furthermore, an optimal water status during heat events may increase stomatal conductance helping to reduce canopy temperature (Drake et al., 2018). As a consequence, water requirements for a plant under heat stress may be increased (Restaino et al., 2016). Failure to replace enough crop evapotranspiration demand under heat waves may lead to substantial reductions in yields and ultimately crop failure (Terry and Kurtural, 2011; Daryanto et al., 2016). In grapevine, in-season yield loss may be mediated through reductions in berry size, at first, and a severe shriveling and bunch stem necrosis if high water deficits persist (Krasnow et al., 2010; Hall et al., 2011; Cook et al., 2015; Yu and Kurtural, 2020). Furthermore, in hot climates, post-veraison water deficits led to a diminution of anthocyanin content (Martínez-Lüscher et al., 2017a), modulation of anthocyanin profile toward di-hydroxylated anthocyanidins (Yu et al., 2016) with rapid degradation of proanthocyanins of grape berry and wine (Yu et al., 2020).
In our previous research, qualitative and quantitative differences in fruit-zone microclimate were induced by means of color shade nets (Martínez-Lüscher et al., 2017b). We designed an experiment aiming to test the effect of partial solar radiation reduction and irrigation amounts and their interaction on gas exchange, stem water potential, berry temperature, must composition, and skin anthocyanins and flavonols. Specifically, the study aimed to evaluate the vulnerability of “Cabernet Sauvignon” grape berry to heat waves and exposure of clusters to solar radiation; and whether increased water application would palliate either high berry temperatures or deleterious effects on grape composition.
Materials and Methods
Experimental Site and Plant Material
The experiment was conducted at the University of California Davis, Oakville Experimental Vineyard (38.428, −122.409; Oakville, CA) during the 2017 growing season. Eight-year old Vitis vinifera “Cabernet Sauvignon” Clone FPS08 grapevines grafted on 110 Richter (Vitis berlandieri × Vitis rupestris) rootstock were used. Plants were trained to bilateral cordons 0.90 m above vineyard floor (cordon height) and shoots were vertically shoot-positioned on 30-single bud spurs. Vine and row spacing was 2.0 m × 2.4 m, respectively, and rows were oriented Northwest to Southeast. The plants were drip-irrigated with 2 pressure compensating emitters per plant delivering 1.9 L/h each. Air temperatures and reference crop evapotranspiration (ETo) from 1 March to 31 October for the year of study and the previous 10 years were obtained from the California Irrigation Management Information System network station installed on site. Clear sky days were calculated using the station radiometer and accounting for days with at least 75% to the total radiation ever recorded for that day of the year as reported elsewhere (Martínez-Lüscher et al., 2017b).
Experimental Design and Treatment Application
The treatments were arranged factorially in a randomized complete block design combining two sustained deficit irrigation amounts, which were 40 and 80% replacement of crop evapotranspiration (ETc), and the absence/presence of shade nets (Exposed and Shaded). Fractions of ETc were calculated weekly from the product of ETo and crop coefficient (Kc) as in Williams and Ayars (Williams and Ayars, 2005). The 80% ETc was achieved by adding two more emitters (1.9 L/h each) in the corresponding locations on a blind irrigation hose. All irrigations began on 20 May, on the day of 50% flowering and no special measures were taken prior to heat events as the intrinsic increase in ETo of high temperatures was calculated and applied at the end of each week. The amount of irrigation applied with the corresponding Kc is reported in Table 1. The Shade netting that allowed 40% of solar radiation to pass through (60% shading) (Black-40; Ginegar, Kibbutz, Israel) was applied on 15 June, 26 days after flowering (DAF), at 100% fruit set. The shade nets applied as follows: the nets were cut into 6 m (long) × 1 m (wide) strips (Supplementary Figure S1A) and hung onto the Southeast and Northwest sides of the canopy at 0.95–1.95 m above the vineyard floor, 0.25 m above the second catch wire as indicated in Supplementary Figure S1B.
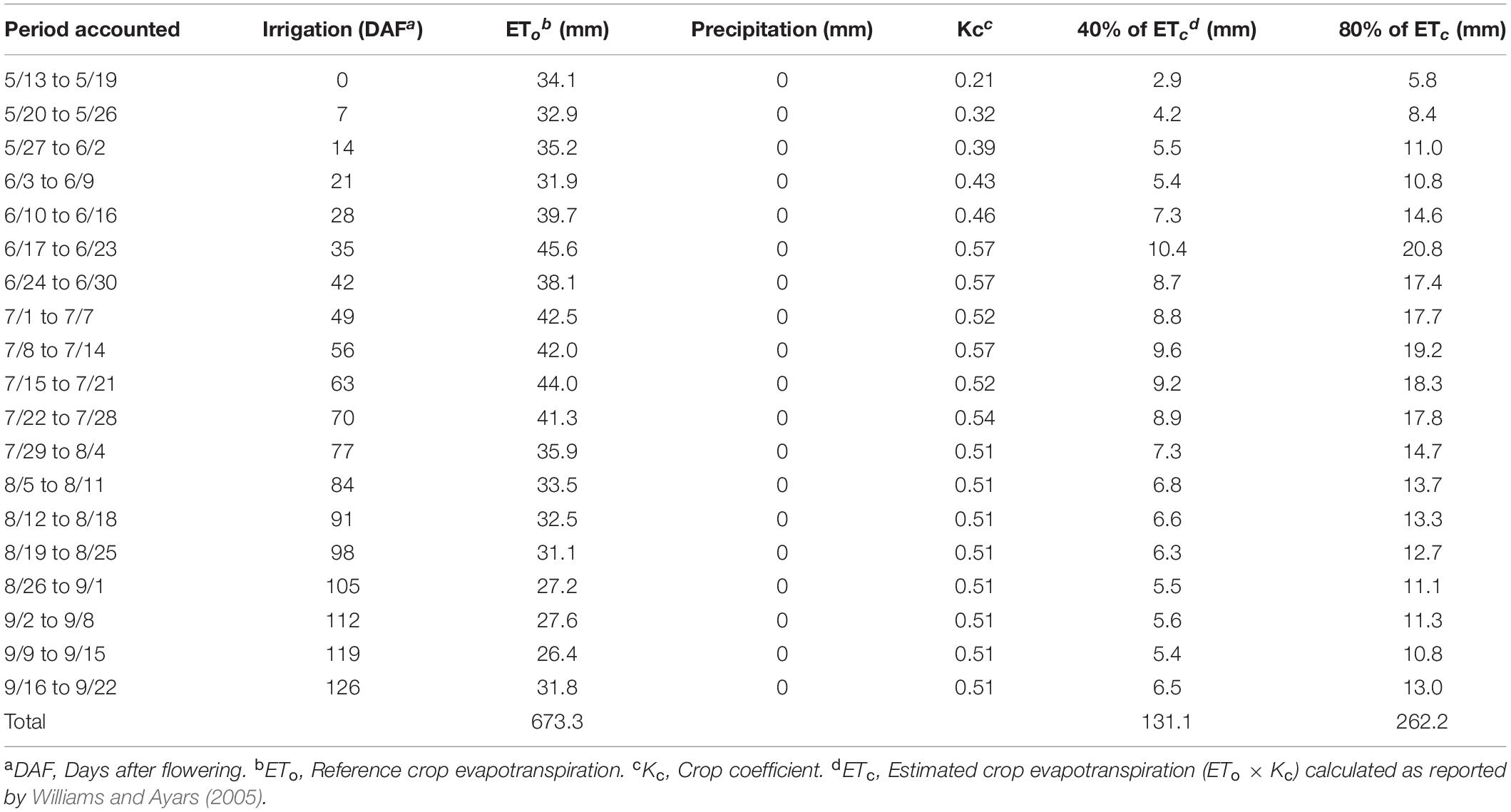
Table 1. Timing of irrigation amounts for the two treatments applied and parameters used to calculate crop evapotranspiration.
Fruit Zone Microclimate
Spectral radiation in the fruiting zone were quantified using a spectrometer with a cosine-corrected head (Black Comet-SR, StellarNet; Tampa, FL, United States) for each of the experimental units around 1500 h, which corresponded the hottest moment of the day (Figure 1). One measurement was taken pointing at the sun and 3 additional measurements were taken at 120° rotational intervals to estimate the light coming from every direction. Cluster temperatures were measured as follows. Portable infrared thermometers (Model 2956; Spectrum Technologies; Aurora, IL, United States) were used on berries following onset of anthocyanin accumulation (67 and 114 DAF, respectively) to measure diurnal shifts in cluster temperature. Two clusters with no leaves shading on either aspect of the canopy were chosen and marked in each experimental unit. Three measurements per cluster were averaged every 2 h from sunset until the moment in time when cluster temperature equilibrated with ambient temperature.
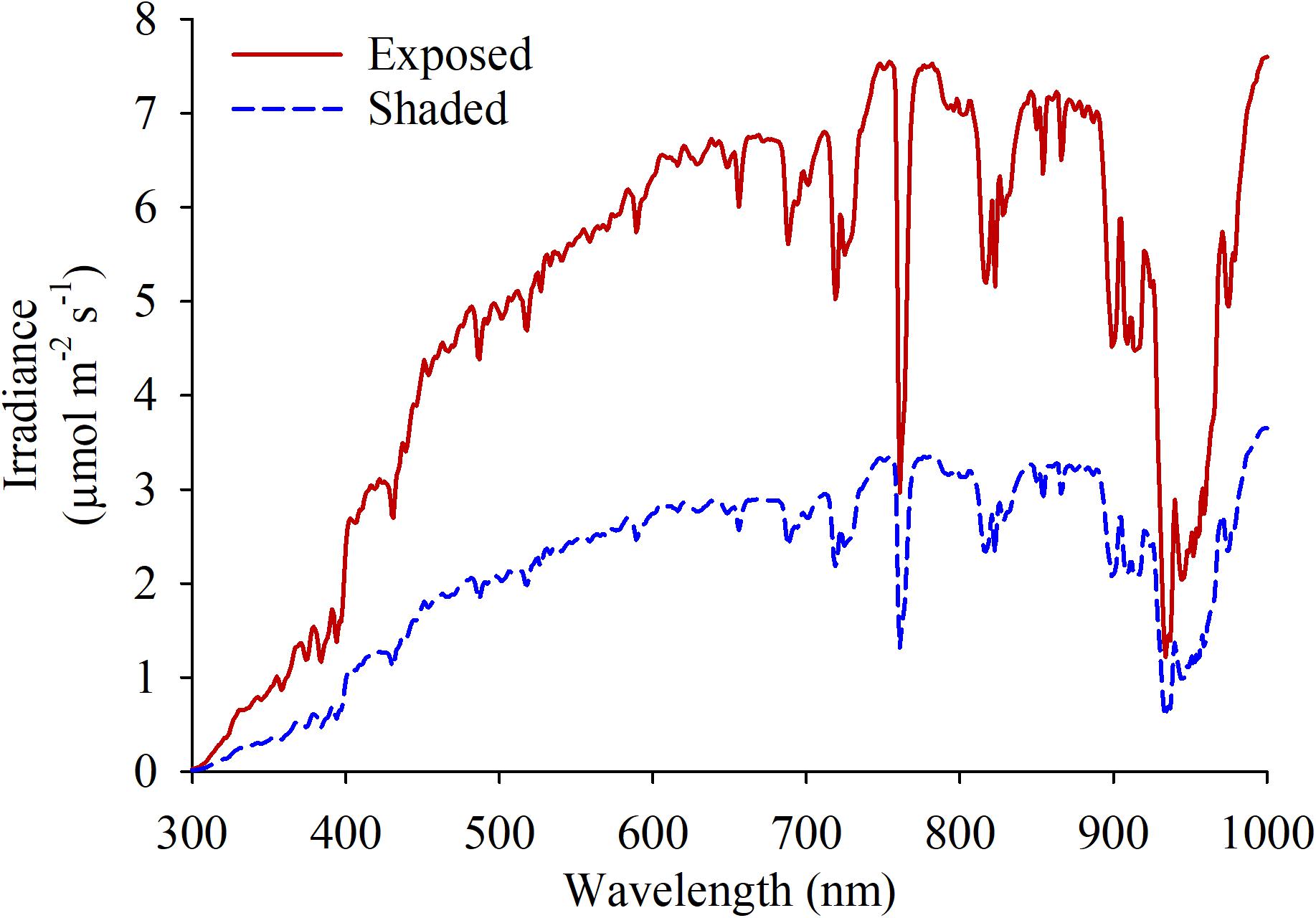
Figure 1. Spectral irradiance measured at the fruit-zone of grapevines fully exposed and shaded at the fruit-zone by Black-40 nets.
Mid-Day Stem Water Potential
Stem water potential (Ψstem) was measured from one fully expanded leaf per vine on the Northeast aspect of the canopy in each plant in the study, 9 times from bud break to harvest. One hour prior to measurement leaves were covered with a re-sealable zip-top foil bag and allowed to equilibrate with stem water potential. Between 1300 and 1400 h local time leaves were excised with a razor blade and measured for water potential with a portable pressure chamber (model 616, PMS Instrument Company, Albany, OR, United States).
Leaf Gas-Exchange
Leaf gas exchange was measured between 1130 and 1430 h local time 4 times (51, 82, 107, and 113 DAF) throughout the season, using a portable infrared gas analyzer CIRAS-3 (PP Systems, Amesbury, MA), featuring a broad-leaf chamber with 4.5 cm2 window. Chamber conditions were set up at 40% relative humidity, a CO2 concentration of 400 μmol⋅mol–1 and using a flow to the chamber of 300 mL⋅min–1. In each experimental unit, one sun-exposed leaf per plant were measured right above the level of nets. In each experimental unit with shade nets, one additional leaf per plant in the lower part of the canopy was measured keeping the shade nets over the cuvette.
Chemicals and Chromatography Standards
All solvents were of HPLC grade. Chemicals and procedures followed previously described work (Martínez-Lüscher et al., 2019a). Acetonitrile, formic acid, hydrochloric acid and methanol were purchased from Fisher Scientific (Santa Clara, CA). Standards for Malvidin 3-o-glucoside, Myricetin-3-o-glucoside, Quercetin 3-o-glucoside, Quercetin 3-o-glucunoride, Quercetin 3-o-galactoside, Kaempferol 3-o-glucoside, Isorhamnetin 3-o-glucoside, and Syringetin 3-o-glucoside, obtained from Sigma-Aldrich (St. Louis, MO, United States).
Sample Collection and Processing
The berries were collected from bunch-closure to harvest. A total of seventy-five berries were collected from each experimental unit on six dates, on both sides of the canopy in equivalent numbers, avoiding severely dehydrated or raisined berries as reported previously (Martínez-Lüscher et al., 2017b; Torres et al., 2020). Each set of seventy-five berries were weighed for individual berry mass calculations. Twenty randomly chosen berries, separate from the 75-berries chosen used in berry composition in section “Berry Composition” (10 from either side of canopy), were weighed and frozen in a −20°C freezer for a minimum of 1 day then individually peeled by hand using a scalpel. Skins were stored a in a −220°C freezer and freeze-dried (Centrivap, Labconco, Kansas City, MO, United States). Dried skins were pulverized in a ball mill (MM400, Retsch, Mammelzen, Germany). A solution of MeOH:H2O:7 M HCl (70:29:1) was added to 50 mg of freeze dried, pulverized skin to quantify flavonols and anthocyanins and allowed to extract overnight at 4°C. Following extraction, samples were centrifuged at 14,000 rpm for 10 min and supernatants filtered (0.45 μm; VWR, Seattle, WA, United States) into HPLC vials and analyzed.
Berry Composition
The 75 berries collected were crushed by hand and filtered to obtain must. A digital refractometer (Palette PR-32, Atago, Tokyo, Japan) was then used to measure total soluble solids (TSS) of filtered juice. Using an autotitrator (862 Compact Titrosampler, Herisau, Switzerland), pH and titratable acidity (TA) were measured. NaOH was used to titrate up to pH 8.2. The TA was expressed as g⋅L–1 equivalents of tartaric acid.
Reversed-Phase High Performance Liquid Chromatography
An HPLC-DAD (1260 series, Agilent, Santa Clara, CA) equipped with a degasser, quarternary pump, thermostatted column compartment and an auto-injector connected to a diode array detector was used to analyze the anthocyanins and flavonols. Mobile phase elution gradient, flavonol and anthocyanin quantification followed previously established procedures (Martínez-Lüscher et al., 2019a) with a reversed phase C18 column LiChrosphere® 100, 250 × 4 mm with a 5 μm particle size and a 4 mm guard column of the same material (Agilent Technologies, Santa Clara, CA, United States). The mobile phase flow rate was 0.5 mL min–1, and two mobile phases were used, which included solvent A = 5.5% aqueous formic acid; solvent B = 5.5% formic acid in acetonitrile. The HPLC flow gradient started with 91.5% A with 8.5% B, 87% A with 13% B at 25 min, 82% A with 18% B at 35 min, 62% A with 38% B at 70 min, 50% A with 50% B at 70.01 min, 30% A with 70% B at 75 min, 91.5% A with 8.5% B from 75.01 min to 91 min. The column temperature was maintained at 25°C. Detection of flavonols and anthocyanins was carried out by the diode array detector at 365 and 520 nm, respectively. A computer workstation with Agilent OpenLAB (Chemstation edition, version A.02.10) was used for chromatographic analysis.
Standards for identification of Malvidin 3-o-glucoside, Myricetin-3-o-glucoside, Quercetin 3-o-glucoside, Quercetin 3-o-glucunoride, Quercetin 3-o-galactoside, Kaempferol 3-o-glucoside, Isorhamnetin 3-o-glucoside, and Syringetin 3-o-glucoside, purchased from Sigma-Aldrich (St. Louis, MO, United States). Malvidin-3-o-glucoside and Quercetin-3-o-glucoside were used as qualitative standards for anthocyanins and flavonols at 520 and 365 nm, respectively. Other compounds were identified using past literature using mass spectrometry (Castillo-Muñoz et al., 2009; Martínez-Lüscher et al., 2014). Individual anthocyanins and flavonols were grouped by substituents in the 3’, 4’, and 5’ positions of the flavonoid B-ring.
Yield Components
At harvest, clusters were removed, counted, and weighed for each plant in the experiment. At harvest, visible damage to each cluster due to excess solar radiation was quantified on a scale of no damage, mild damage, moderate damage, and severe damage following the subjective criteria presented in Figure 2. In severely damaged clusters, all the grapes in the exposed side of the cluster where completely dehydrated. We measured dormant pruning weights on a top-loading scale, after pruning the grapevines to one bud spurs on 13 February 2018.
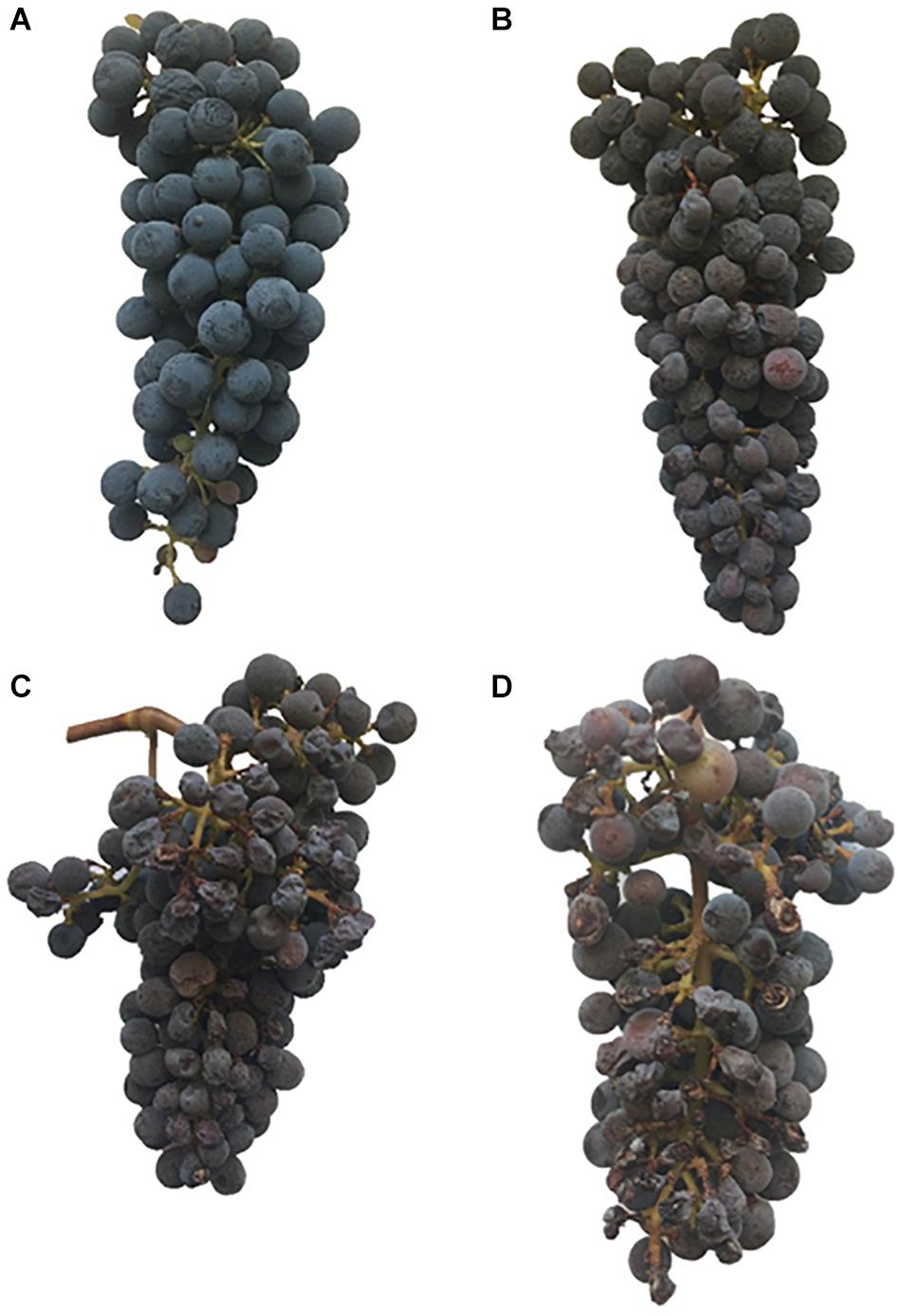
Figure 2. Cabernet Sauvignon (Vitis vinifera L.) cluster with no damage (A) used for the evaluation at harvest, with mild damage (B) some berries discolored and dehydrated, with moderate damage (C) many berries discolored, dehydrated, severe damage (D) most berries discolored or dehydrated and ripening impaired.
Statistical Analysis
Statistical analysis of data were conducted using SAS v.9.4 (SAS Institute Cary, NC). Data were tested for normality using Shapiro-Wilk’s test and were subjected Levene’s test (Levene, 1960) to ascertain the data met the assumptions of analysis of variance. Percentage of fruit damage data were log-transformed prior to statistical analysis according to results of the Shapiro-Wilk’s test, but non-transformed means are presented to aid in discussion of the corresponding figure. The data were then subjected to a two-way analysis of variance (ANOVA). When the results of ANOVA were significant at p < 0.05 data were then subjected to post hoc Tukey’s HSD test.
Results
Environmental Conditions and Heat Waves
During execution of the trial, 80% of the days had no cloud cover and were sunny. The conditions of no cloud cover and sunny days was similar to the conditions during the last 10 years at the study site. However, the growing season in which the experiment was conducted was slightly warmer. The average daily maximum temperature was 1.5°C warmer 2017 (28.2°C) when compared to the last 10 years’ average, at 26.7°C. Furthermore, the experimental year had several heat wave events where maximum air temperatures above 40°C that were recorded on 18 June (29 DAF), 7 July (48 DAF), 16 July (57 DAF), 26 August (98 DAF), 27 August (99 DAF), 1 September (104 DAF), and on 2 September (105 DAF) (Figure 3). In fact, on 2 September the maximum air temperature was 43.4°C which was the absolute maximum temperature recorded at the study site since 2006.
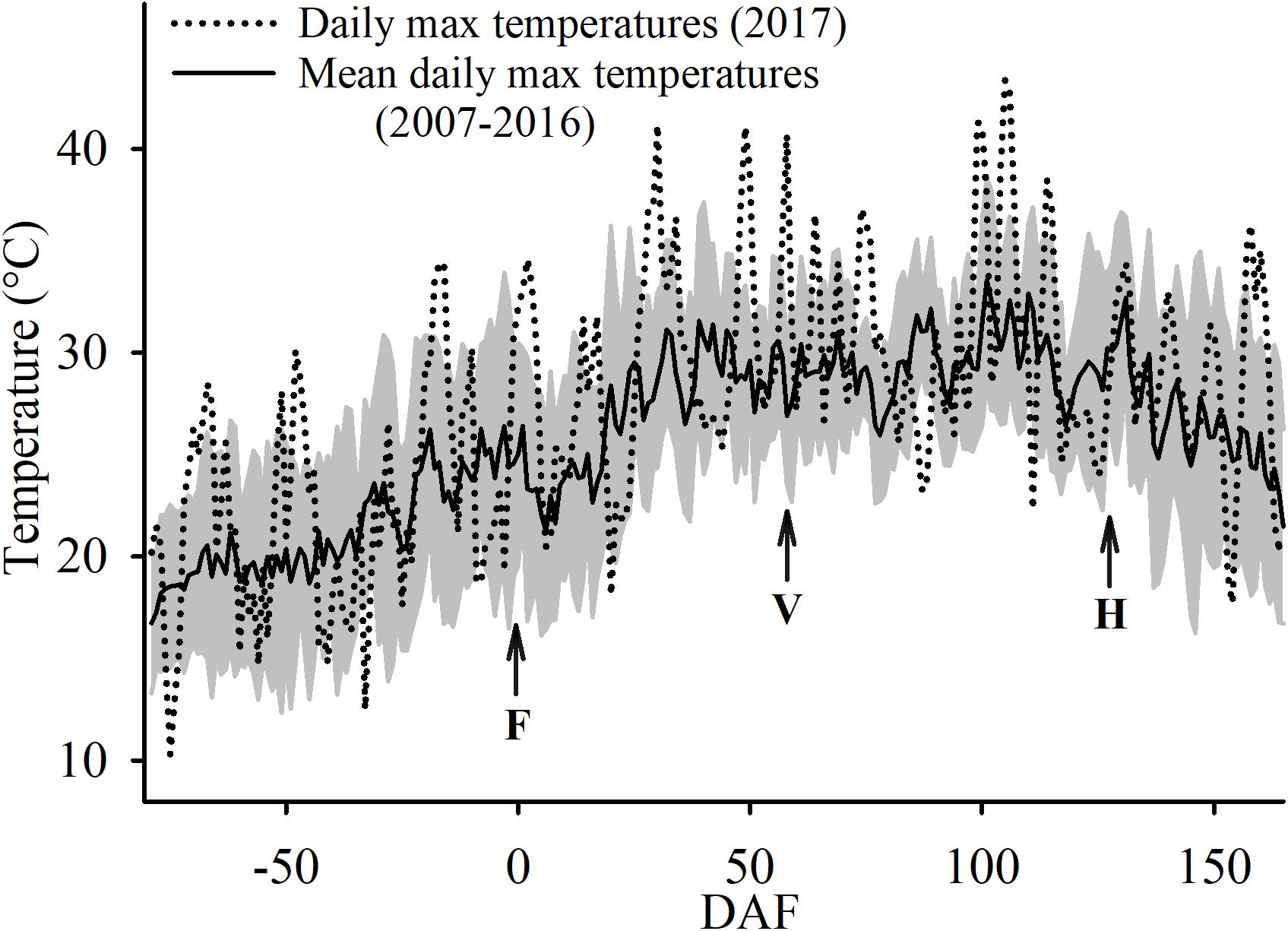
Figure 3. Ten-year average (± SD; shaded area) and 2017 maximum air temperatures at the Oakville Experimental Vineyard as recorded. F, for the date of 50% flowering; V, for 50% veraison and H, for commercial harvest.
Plant Water Status
Irrigation was not initiated until 20 May 2017 (Table 1 and Figure 4). There was no effect of partial shading on stem water potential throughout the monitoring period (Figure 4). The applied water amounts for the 40%ETc treatment were half of the 80%ETc treatment as planned (Table 1). The stem water potential of 80% ETc treatments started to be significantly different from 40% ETc on 65 DAF. The differences of stem water potentials between the 40 and 80% ETc treatments increased throughout the season, culminating at harvest, when 40% ETc had ca. −1.23 MPa and 80% ETc had −0.90 MPa.
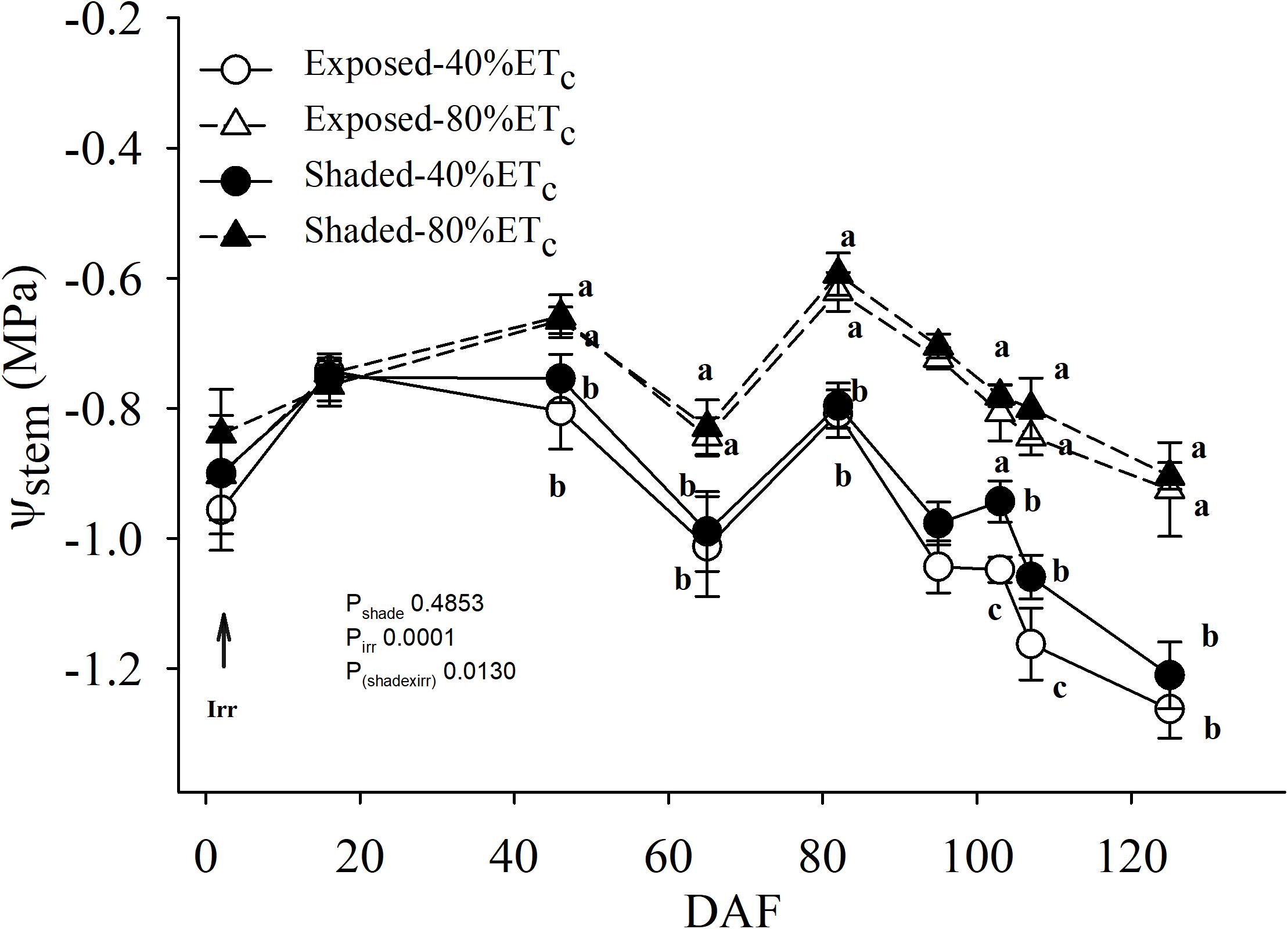
Figure 4. Mid-day stem water potential (Ψstem) of plants Exposed or 60% Shaded by nets at the fruit zone level combined with two applied water amounts, 40 and 80% replacement of crop evapotranspiration (ETc). Error bars represent standard errors (n = 4). Groups with no letters in common are statistically different (p < 0.05).
Leaf Gas Exchange and Microclimate
Net carbon assimilation (AN) was reduced in leaves under the partial shading (Figure 5A). This was associated with a strong decrease in incident PAR of nearly 60% (Figure 5D). Conversely, the leaves above the nets with 80% ETc had greater ANt than Exposed–40% ETc. The 80% ETc treatment did not display higher stomatal conductance (gs) or leaf evapotranspiration (E) (Figures 5B,C) under our conditions.
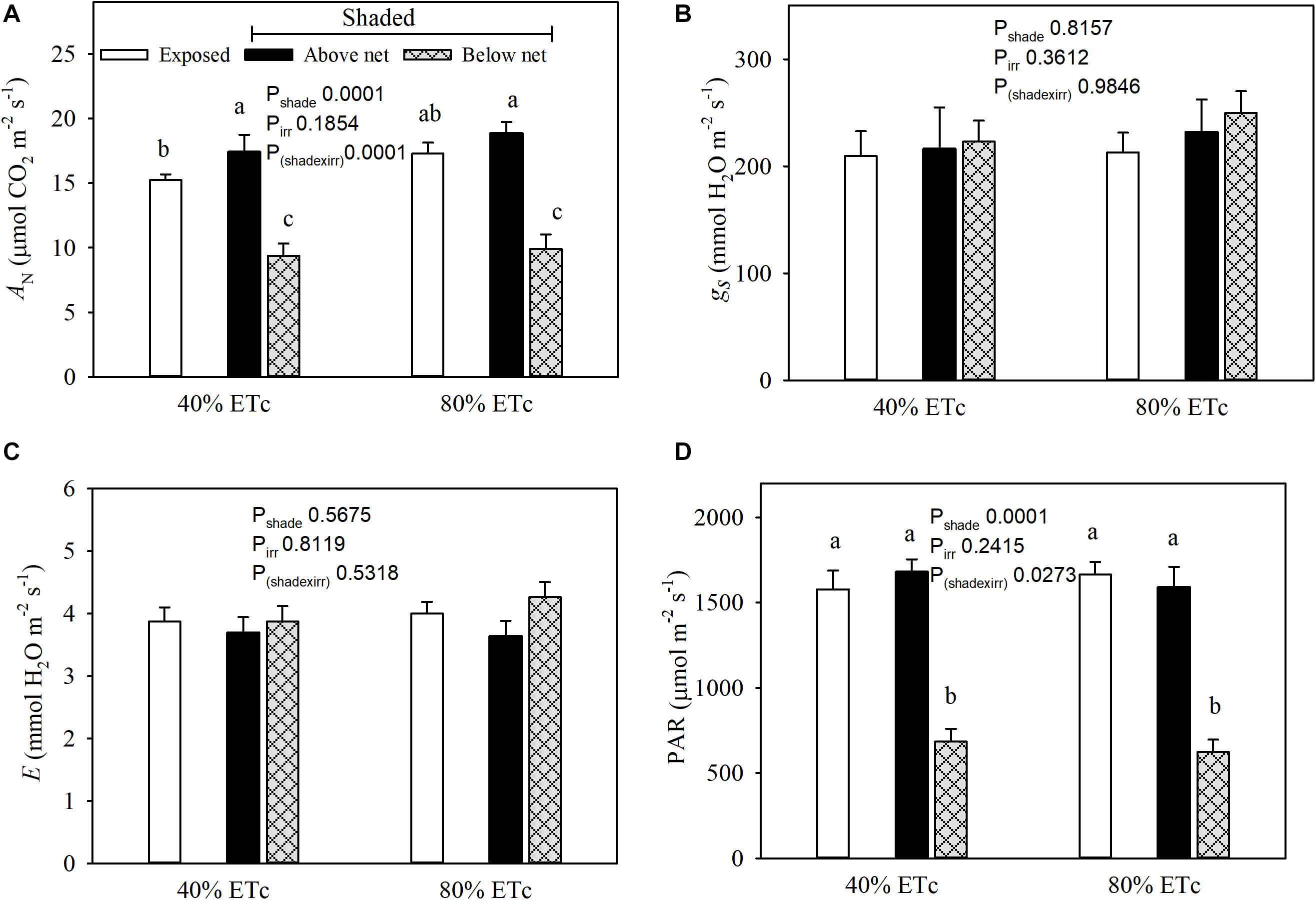
Figure 5. Seasonal averages of leaf gas exchange parameters (Net carbon assimilation, A; stomatal conductance, B; and evapotranspiration, C) and chamber PAR (D) measured in plants Exposed or 60% Shaded by nets at the fruit zone level combined with two applied water amounts, 40 and 80% replacement of crop evapotranspiration (ETc). For plants with fruit zone shade nets, leaves above and below the fruit-zone shade were measured. Error bars represent standard errors (n = 12). Groups with no letters in common are statistically different (p < 0.05).
Cluster Temperature
We initially determined the cluster temperatures at 65 DAF, during immediate pre-veraison (Figures 6A,B). The air temperature reached a maximum of 33.5°C. Under these conditions, Exposed-80% ETc Northeast-facing clusters started to warm up significantly above air temperature (up to 8°C with Exposed 80%-ETc at 0930 h) and reached 29.6°C, which was 3.5°C higher than the partially Shaded clusters (Figure 6A). However, after 1100 h solar radiation no longer reached Northeast-facing clusters and cluster temperatures tended to equilibrate with air temperature. Conversely, Southwest-facing clusters at 65 DAF started to be fully exposed to solar radiation after 1300 h and cluster temperatures started to separate among Exposed and Shaded clusters (Figure 6B). However, significant differences were not observed until 1730 h, when Exposed–40%ETc and Exposed–80% ETc reached 45.4°C, which was approximately 4.5°C greater than the Shaded clusters.
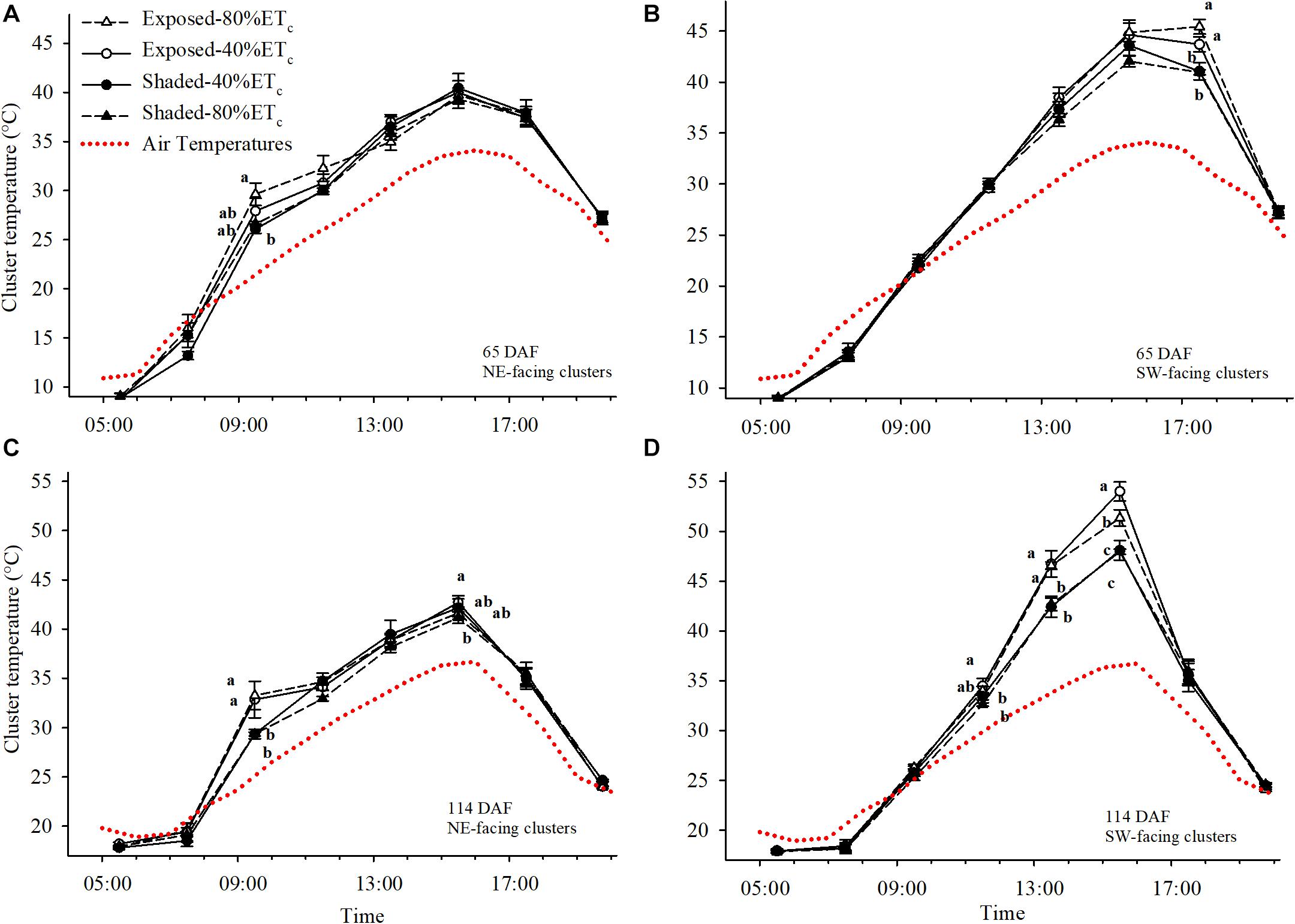
Figure 6. Cluster and air temperatures measured on 24 July (A,B) and 11 September (C,D) in plants Exposed or Shaded by nets at the fruit zone level combined with two applied water amounts, 40 and 80% replacement of crop evapotranspiration (ETc). Error bars represent standard errors (n = 4). Groups with no letters in common are statistically different (p < 0.05). The Northwest and Southwest aspects of the canopy were assessed.
At 114 DAF, when the second cluster temperature measurements were collected; clusters were more fully colored, and the air temperature reached 38°C. This resulted in cluster temperatures reaching 33.2°C at 1130 h in Northeast-facing Exposed clusters. These clusters were 3.85°C hotter than the Shaded (Figure 6C). Differences were more pronounced in the Southwest-facing Exposed clusters that were 4°C warmer than the Shaded clusters at 1330 h. Furthermore, cluster temperature of Exposed–40%ETc clusters were nearly 6°C higher than the Shaded clusters at 1530 h (Figure 6D). Increasing the irrigation amount to 80%ETc provided some relief to Exposed clusters, albeit the cluster temperatures of Exposed–80% ETc were 9°C greater than the ambient air temperature.
Berry Fresh Mass and Must Composition
Significant differences in berry fresh mass (BFM) were intermittent as 80% ETc tended to have greater BFM throughout the growing season (Figure 7A). For instance, the BFM of Exposed–80%ETc BFM was greater than the rest of the treatments at 65 DAF. At 115 DAF, BFM was higher in 80% ETc treatments regardless of the presence of Shading. However, at harvest (128 DAF), only the BFM of Shaded–80%ETc was higher than the rest of the treatments. TSS were lower with Shaded-80% ETc at the two last berry samplings (Figure 7B). The Exposed–40%ETc had the greatest TSS (27.2°Brix) and Shaded–80%ETc the lowest TSS (24.7°Brix) at harvest. Similar intermittent significant differences were observed for pH and titratable acidity (TA) as BFM. The Exposed–40%ETc had higher pH (Figure 7C) and lower TA (Figure 7D) than Shaded treatments at 86 DAF. We observed a similar trend at 102 and 115 DAF, where TA and pH were significantly different, respectively.
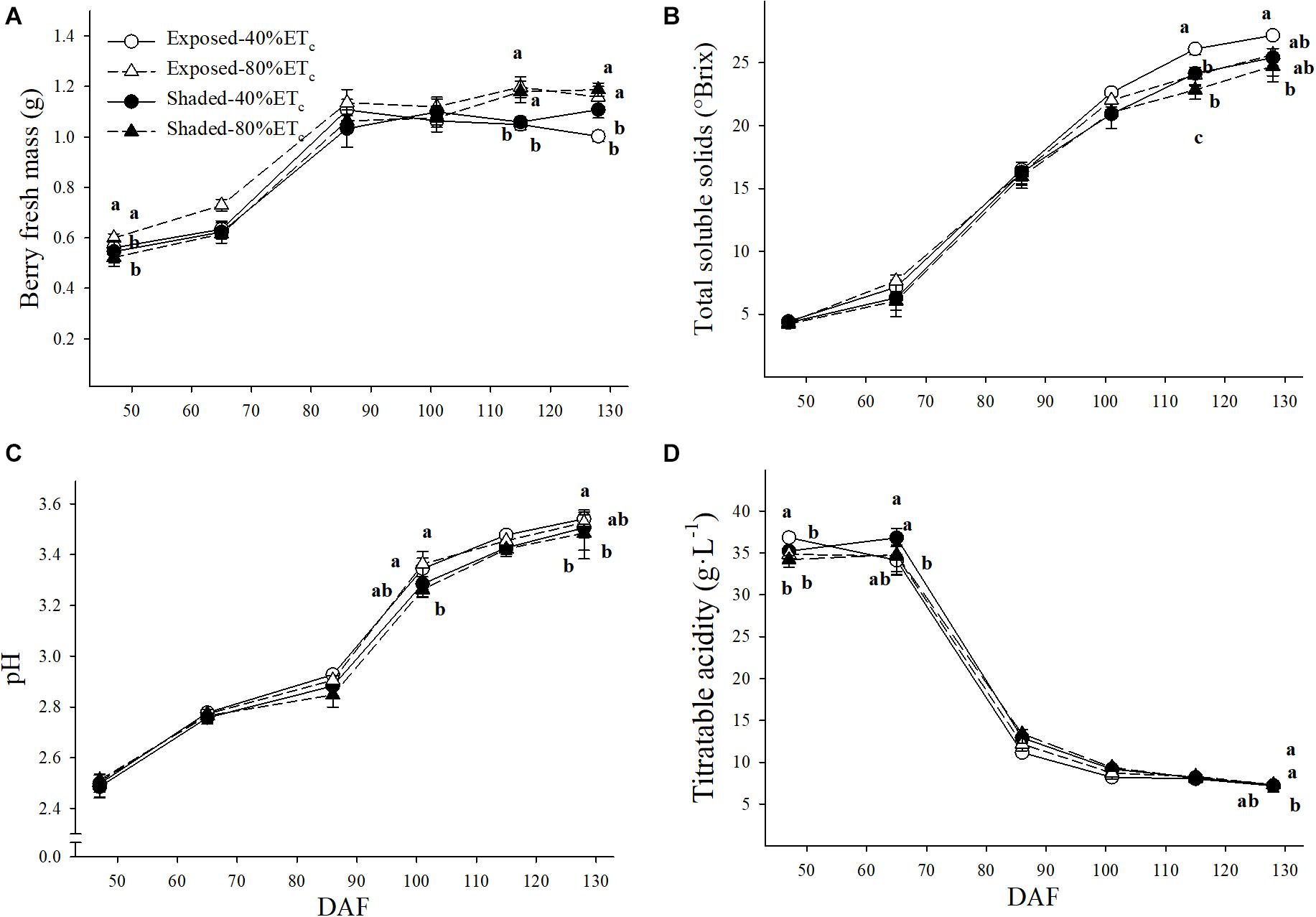
Figure 7. Berry fresh mass (A), must total soluble solids (B), pH (C) and titratable acidity (D) in plants Exposed or Shaded 60% by nets at the fruit zone level combined with two applied water amounts, 40 and 80% replacement of crop evapotranspiration (ETc). Error bars represent standard errors (n = 4). Groups with no letters in common are statistically different (p < 0.05).
Berry Skin Anthocyanins and Flavonols
Berry skin anthocyanins were initially greater in Exposed–40% ETc at 86 DAF (Figure 8A). However, as all treatments reached their maximum anthocyanin concentration, it started to decrease in the Exposed–40% ETc treatment. By harvest (128 DAF), Exposed treatments, regardless of irrigation treatment had lower anthocyanins than the Shaded treatments. Shading and irrigation appeared to have an additive effect on the anthocyanin profile. This effect revealed itself as a decreases in the proportion of petunidin (Figure 8B), and cyanidin (Figure 8E) in favor of malvidin (Figure 8F). This effect was more pronounced especially toward harvest. Furthermore, the proportion of peonidin was only lower in the Shaded–40%ETc for the last 3 sampling points (Figure 8D).
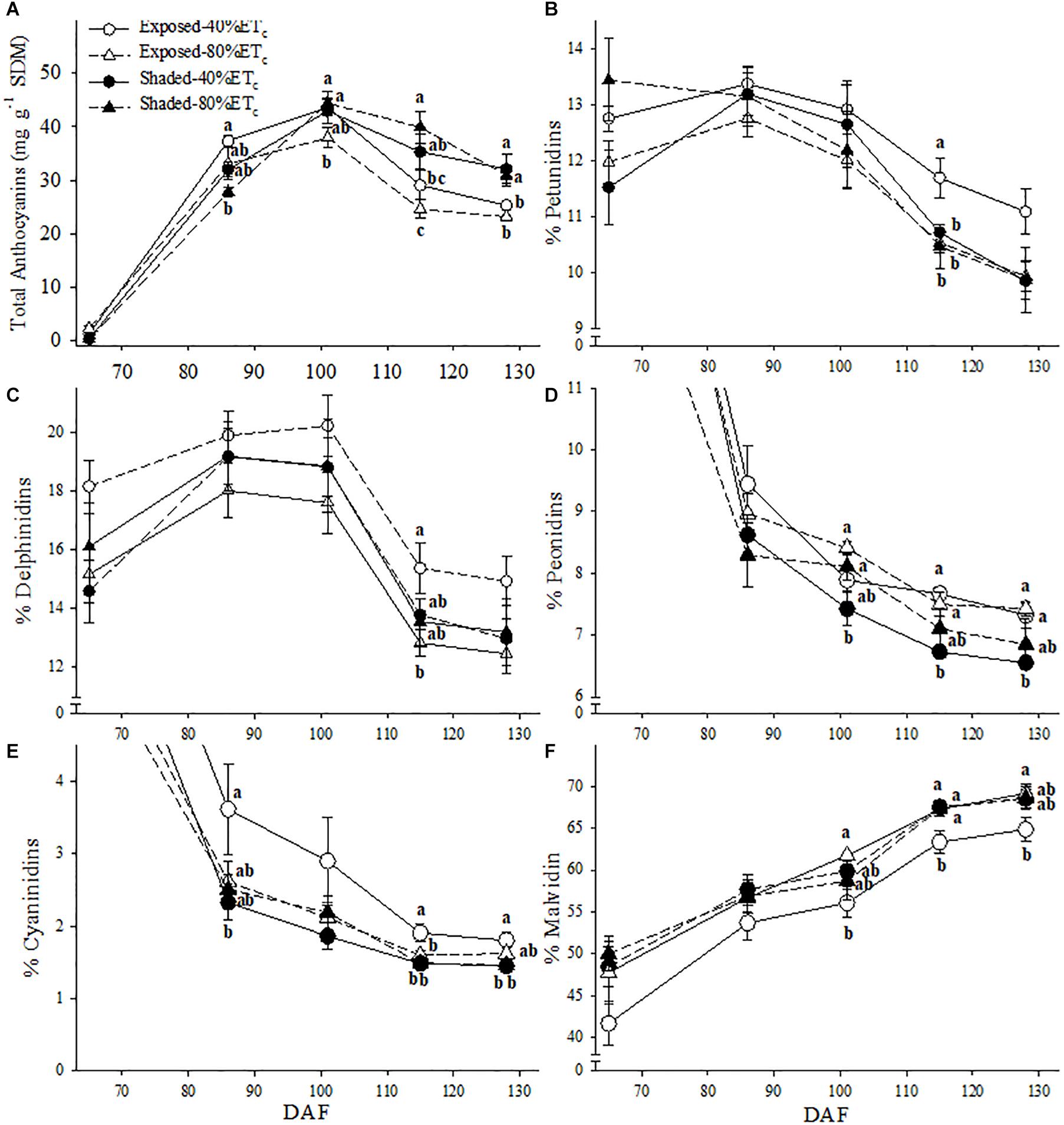
Figure 8. Berry skin anthocyanin content (A) and the relative abundance petunidin (B), delphinidin (C) peonidin (D) cyanidin (E) and malvidin (F) of in plants Exposed or Shaded 60% by nets at the fruit zone level combined with two applied water amounts, 40 and 80% replacement of crop evapotranspiration (ETc). Error bars represent standard errors (n = 4). Groups with no letters in common are statistically different (p < 0.05).
Berry skin flavonol concentration was more than 2 times greater in the Exposed berries at 86 and 102 DAF (Figure 9A). However, this difference decreased as flavonol concentration increased in the Shaded berries until 115 DAF. Meanwhile the Exposed berries reached their maximum flavonol concentration at 86 DAF. Flavonol profile was in most cases affected by the presence or absence of Shading rather than additional irrigation amounts. The proportion of myricetin and isorhamnetin was greater in the Shaded treatments for the last three berry sampling points (Figures 9B,E). Likewise, this corresponded to decreases in the proportion of quercetin (Figure 9C). The proportion of kaempferol was lower in Shaded treatments and this difference continued to increase through harvest regardless of irrigation amount (Figure 9D).
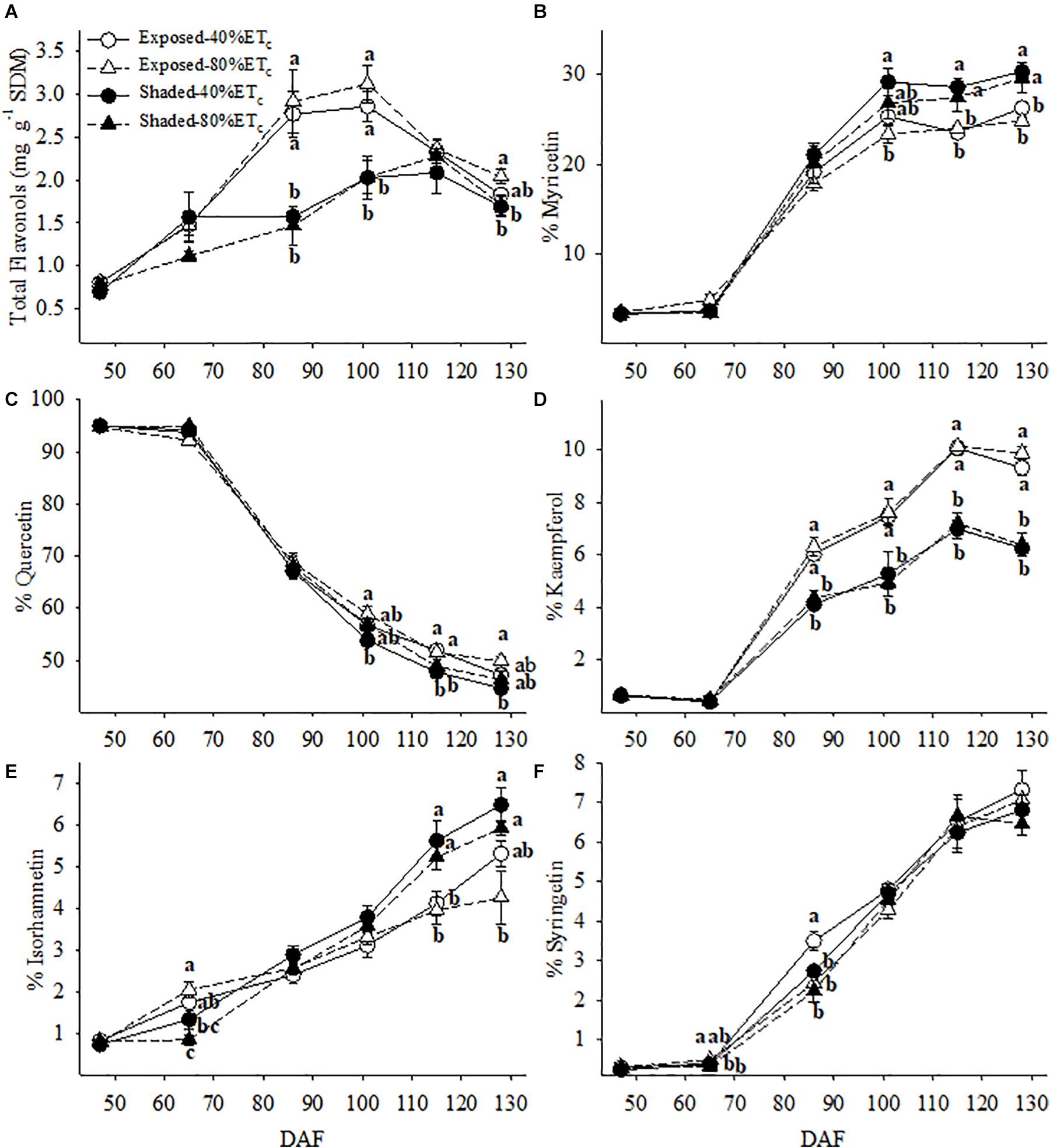
Figure 9. Berry skin flavonol content (A) and the relative abundance of myricetin (B), quercetin (C), kaempferol, (D), isorhamnetin (E) and syringetin (F) in plants Exposed or Shaded 60% by nets at the fruit zone level combined with two applied water amounts, 40 and 80% replacement of crop evapotranspiration (ETc). Error bars represent standard errors (n = 4). Groups with no letters in common are statistically different (p < 0.05).
During the most severe heat wave event of the year (103–106 DAF), anthocyanin loss occurred both in Exposed and Shaded berries. However, the loss was more pronounced in the Exposed berries (Table 2). We measured significant changes in the anthocyanin composition of berries which revealed themselves as reductions in the proportion of delphinidins, mainly in favor of malvidin in the Exposed treatment. Conversely, this modulation of anthocyanin profile was not observed in the Shaded berries. Likewise, flavonols in the Exposed treatment experienced a loss, as well. However the flavonol losses were less severe than the anthocyanins. During this most severe heat wave event, we measured a modulation of the flavonol profile, as well. After the heat wave, the proportion of kaempferol and laricitin were greater in detriment of myricetin.
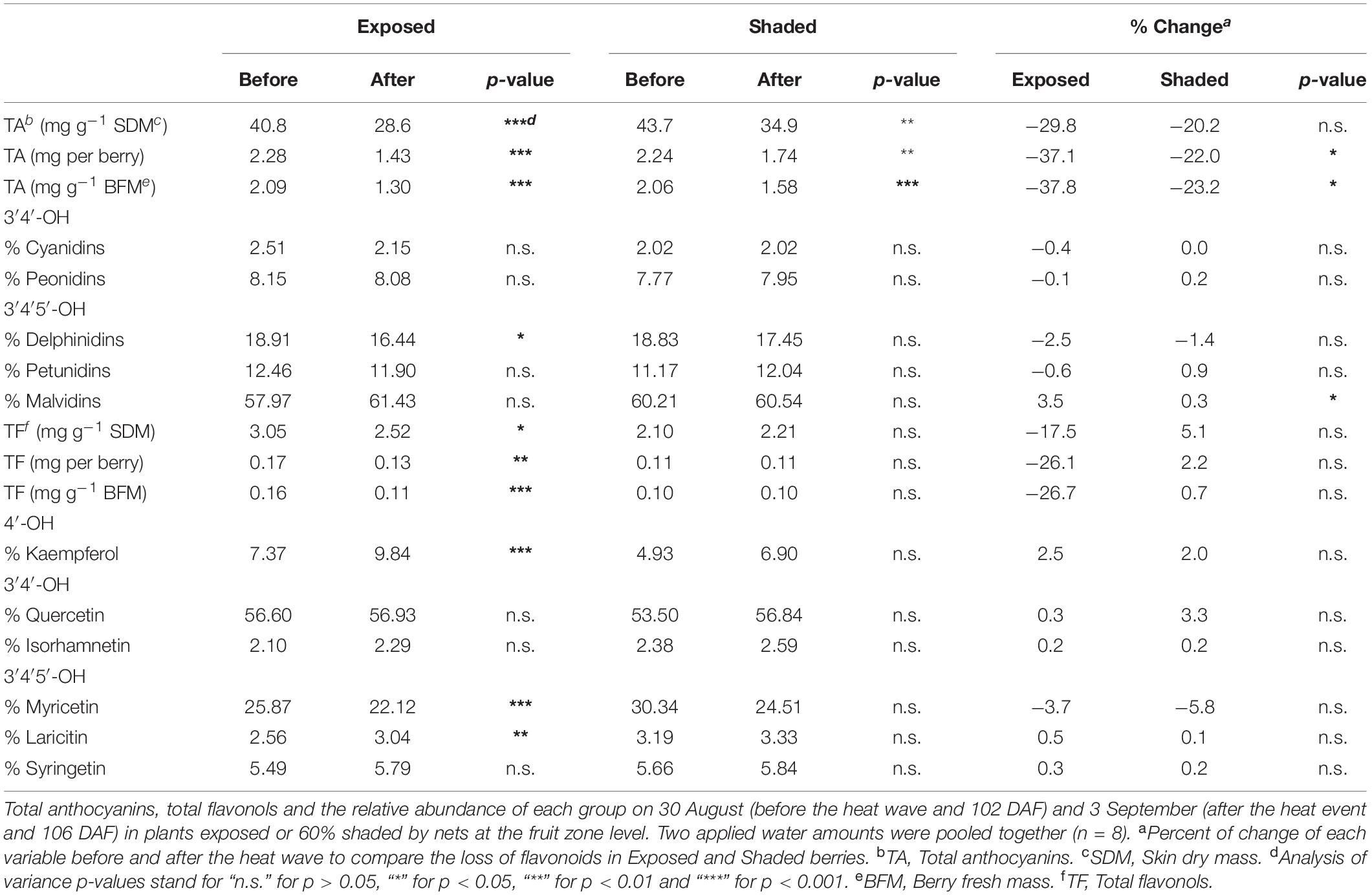
Table 2. Effects of a 4-day-long heat event with a maximum temperature of 43.4°C on anthocyanins and flavonols.
Yield Components and Heat Wave Damage at Harvest
Harvest commenced at 128 DAF. The cluster weight (Table 3) was slightly greater with the 80% ETc. However, this did not result in higher yield per grapevine. We measured a 15% decrease of dormant pruning mass in the Shaded treatment, and this resulted in a greater yield-to-pruning mass ratio. However, the yield-to-pruning mass ratio was not statistically different.
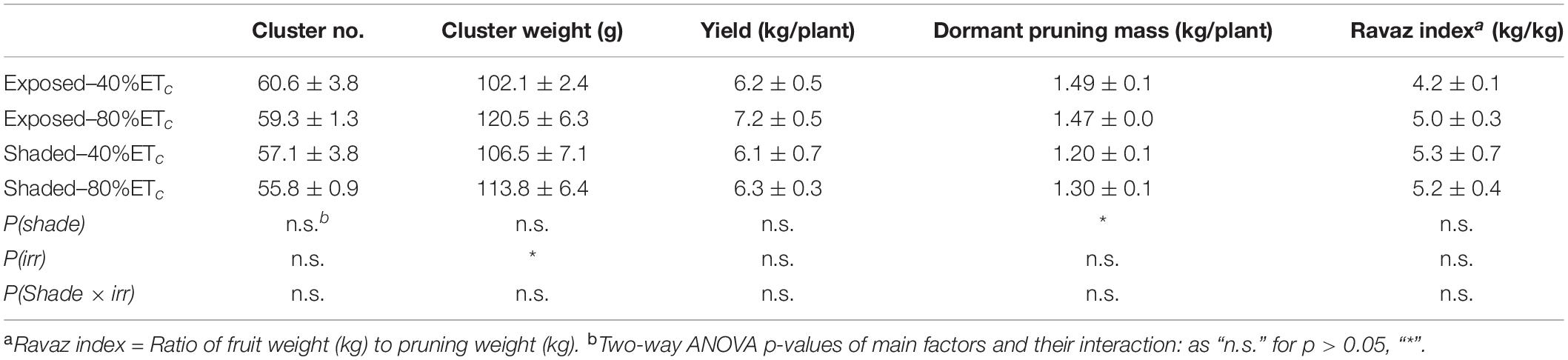
Table 3. Effects of partial solar radiation exclusion by Black-40 nets and replacement of 40% or 80% of estimated crop evapotranspiration replacement by irrigation on components of yield Cabernet Sauvignon grapevines.
The Southwest-facing clusters in the Exposed treatments showed evident signs of damage consisting in desiccated and aborted berries, affecting the appearance of clusters to a different extent (Supplementary Figure S2). In fact, the greater part of the berry abortion happened immediately after a heat wave with temperatures of 43.4°C and 42.8°C on 104 and 105 DAF, respectively. The proportion of damaged clusters was greater in the Exposed treatments when compared to Shaded (24 vs. 2%; Figure 10). Out of this damage, more than a third was severe; indicating that all the visible portions of the grape cluster, directly exposed were completely damaged. The 40%ETc treatments had slightly higher proportion of damaged clusters than 80%ETc but these were not significantly different. First signs of berry damage were observed 89 DAF on 17 August as a mild discoloration of the grape skins most oriented toward the sun only in well-exposed clusters on the Southwest aspect of the canopy. This damage progressed into flesh tissue death and berry mummification over the course of weeks (Supplementary Figures S2E,F). However, a heat shock phenomenon occurred the days of the heat wave, where sun-facing grapes within a cluster would discolor within hours and mummify over 1 or 2 days (Supplementary Figures S2A,B,G). A third phenomenon, appeared by harvest where whole clusters were found to be completely mummified regardless of their exposure. Previously, this phenomenon was referred to as bunch stem necrosis (Krasnow et al., 2010).
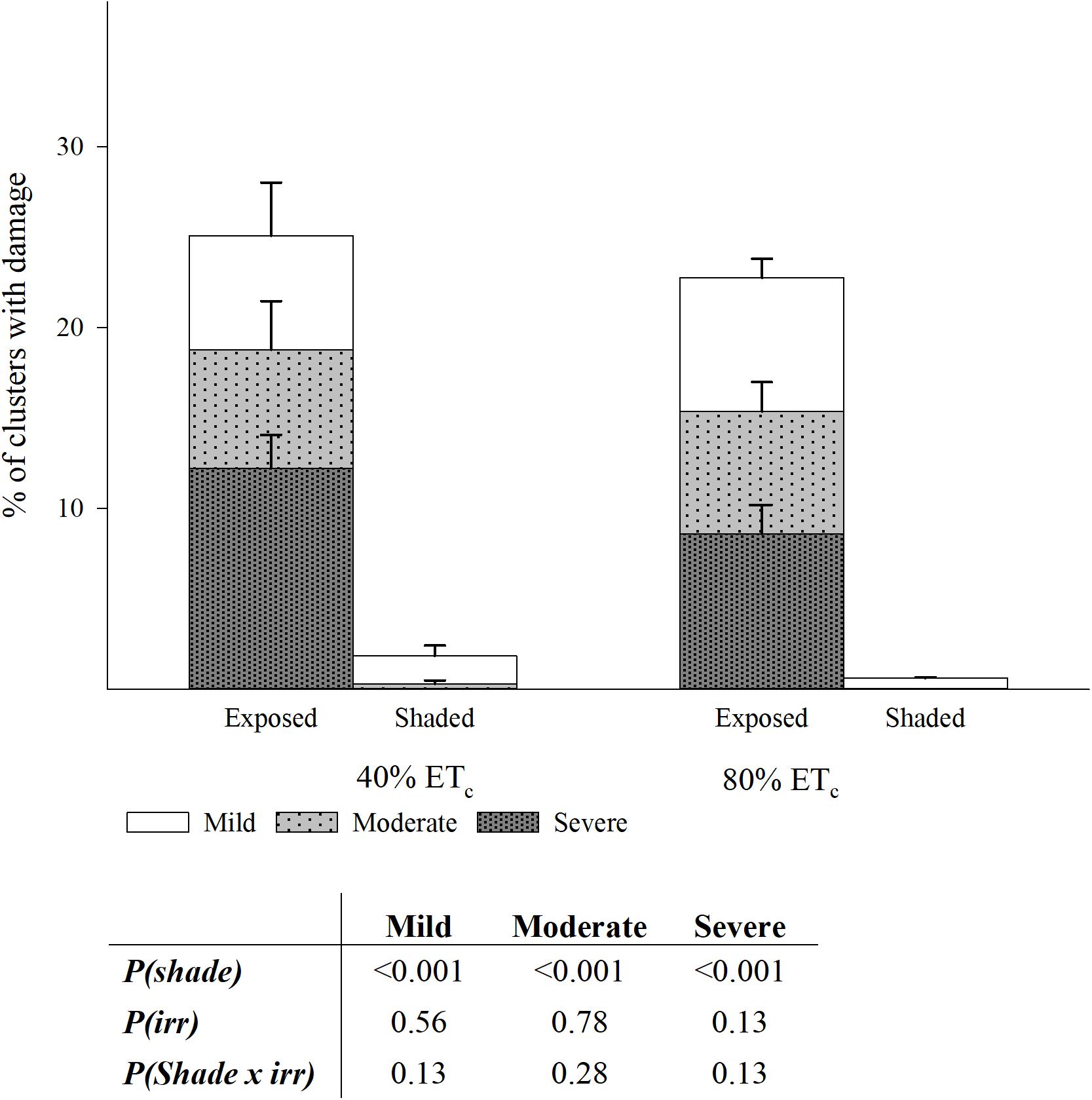
Figure 10. Percentage of clusters with three levels of damage in plants Exposed or Shaded 60% by nets at the fruit zone level combined with two applied water amounts, 40 and 80% replacement of crop evapotranspiration (ETc). Error bars represent standard errors (n = 4). Cut off sampling points did not present statistical differences. Table with two-way ANOVA p-values of main factors and their interaction for each of the damage categories.
Discussion
Environmental Conditions and Heat Waves
During the execution of this study, a 4-day heat wave affected the study site from 31 of August to the 3 of September (103-106 DAF). Although other high heat events occurred that year and the preceding years (Koch et al., 2012; Martínez-Lüscher et al., 2017b) at the study site, it was undeniable that this 4-day period with temperatures above 37°C with a maximum temperature of 43.4°C conditioned our results. These temperatures have not been observed at the study site since 2006. Therefore, results can be interpreted in the context of dealing with extreme climatic events that may become more frequent in many viticultural regions in the context of climate change predictions (Dosio et al., 2018). In regions that are accustomed to growing wine grapes under such high temperatures (Kurtural et al., 2013; Martínez-Lüscher et al., 2017a) events such as the one witnessed here are buffered with the use of different trellising systems. However, with the pre-planting decision of a vertical shoot positioning training system in coastal, but hot climate areas exacerbated the heat wave conditions even further with the monitored effects to cascading to plant primary metabolism.
Plant Primary Metabolism
Stem water potential (Ψstem) was greatly affected by doubling the amount of water applied. Customarily, vineyard operators would apply 40–50% of estimated ETc in the study area (Torres et al., 2020). Contrary to our expectations, 80%ETc did not incur higher stomatal conductance, transpiration or carbon assimilation for the instances measured. The relatively frequent (once a week) replacement of ETc fractions could have allowed maintaining stomatal conductance, and ultimately yields, while water stored in the soil profile and stem water potential decreases through the season. However, it should not be discarded that in this study, more frequent gas exchange measurements, at a different time of the day and further from the irrigation day may have resulted in differences in stomatal conductance according to the amounts of water applied. Shade nets reduced the solar radiation by 40% that reached the sides of the first 0.25 m of the canopy (composed of shoots 1.4 m or longer) and this reduced carbon assimilation in those shaded leaves, but not the rest. The lower carbon assimilation measured in Shaded treatments resulted in lower pruning mass (Greer and Weedon, 2013). Plant organ mass (in our case dormant shoot mass) is an important indicator of photomorphogenesis. Its cell division and expansion may be altered by sun exposure through photomorphogenic effects at low rates (Robson et al., 2015). In addition, shade nets led to slightly better water status under 40% ETc, which could be indicative of a slightly lower water consumption by plants with nets covering the leaves around the fruit-zone. In studies where shading nets were placed above the canopy, soluble solids may be reduced through lower carbon assimilation rates when shriveling berries were not accounted (Greer and Weedon, 2013).
The application of 80% ETc of water slightly increased berry and cluster mass. In fact, increases in berry size by both shade nets double irrigation were coupled to a reduction in TSS. Decreases in berry size and/or increases in berry TSS were observed in response to solar exposure by fruit-zone leaf removal (Poni et al., 2009). Solar overexposure may exacerbated transpiration loss in the fruit-zone, which has showed to affect cell expansion during leaf growth (Devi et al., 2015). However, in the last weeks of fruit development, higher dehydration/cell death may better explain the lower berry weight of Exposed–40%ETc (Bonada et al., 2013; Caravia et al., 2016). Changes in berry size are often one of the first events in a concomitant effect, in which berry soluble solids are concentrated, enhancing other processes intertwined to hexose signaling, transport and metabolism (Dai et al., 2014; Rienth et al., 2016). The Exposed–40%ETc berries, had higher TSS (+ 2°Brix) than the other treatments. However, berry acidity was mildly impacted and no significant effects were evident at harvest. Having similar titratable acidity values in berries exposed to higher temperatures was a result that was somewhat unexpected as warmer berry temperatures are associated with a higher respiration rate of malic acid (Sweetman et al., 2014). Contrarily to experiments in which ambient temperature was manipulated (e.g., open top chambers) that have a very homogeneous effect on fruit metabolism, overexposure is an extreme—although greatly patchy—factor depending on the position of each cluster and how these are covered by leaves and the time of the day. Therefore, the spatiotemporal variation in cluster temperature differences among treatments can be anything from similar (e.g., at night or clusters occluded by leaves) to + 12°C (i.e., at 1530 h in Southwest exposed clusters). This finding provided evidence that the greater berry temperature experienced by berries of Exposed vines—only experienced by some clusters on the Southwest aspect for a period of less than 4 h—has a milder impact on overall grape must pH and TA (Zarrouk et al., 2016) than persistent changes in ambient temperature reported elsewhere (Sweetman et al., 2014; Rienth et al., 2016; Torres et al., 2020).
Plant Secondary Metabolites
Berry skin anthocyanins were initially greater in Exposed–40%ETc 86 DAF but higher in the two shaded treatments (regardless of irrigation) during the last weeks prior to harvest. Whereas part of these results responded to a shift in berry development, mediated by the higher TSS of Exposed-40%ETc grapevines, the lower concentration of anthocyanin of Exposed grapevines at harvest responded to degradation. This result is corroborated by Torres et al. (2020) where optimal solar radiation exposure for Cabernet Sauvignon grape berry is in fact, less than 20% of the global radiation reaching the cluster. Furthermore, the first visual symptoms of berry color blanching were observed on 17 August, not long after veraison and during the first two heat waves of the year (Figure 3). At 102 DAF, before the 4-day heat wave, anthocyanin concentration was at its maximum. However, when anthocyanins were measured 4 days after this heat wave, anthocyanin concentration decreased at variable rates in each, and all treatments (Table 2). Doubling the irrigation amount did not change anthocyanin degradation rates in any case, thus only the factor of Shading was presented. Studies dealing with the effects of light and temperature on anthocyanins have proposed reduced synthesis (Yamane et al., 2006), chemical (Mori et al., 2007) and biological degradation (Sarni-Manchado et al., 1997) of these. For instance, anthocyanin degradation rates were 20–23% in Shaded treatments, which was interpreted as an effect of ambient temperatures above 40°C on berries at a stage (102 DAF) that synthesis was most certainly stopped (Castellarin et al., 2007). Exposed grapes also experienced extreme berry temperatures beyond 50°C for a few hours, and nearly doubled anthocyanin degradation rates (30–38%). Isothermal degradation kinetics studies with fruit extracts indicated half-life values of few days at 50°C (Cemeroğlu et al., 1994), which were enough to corroborate to a great extent the anthocyanin degradation rates in the present study.
Malvidin was the most abundant anthocyanin in all samples and its proportion increased in detriment of the other 4 anthocyanins as ripening progressed. Changes in anthocyanin profile have been reported before and attributed to differential synthesis concomitant to changes in expression of flavonoid hydroxylases (Castellarin et al., 2006; Martínez-Lüscher et al., 2014). A differential degradation of each of the anthocyanins could also explain this result based on the different antioxidant capacity of each compound (Arroyo-Currás et al., 2016; Csepregi and Hideg, 2018). However, the increase in the proportion of malvidin increased at a constant pace, regardless of net synthesis or degradation (i.e., before or after 102 DAF) and (Torres et al., 2020) reported similar findings in Cabernet Sauvignon to further corroborate these results.
Inhibition of flavonol synthesis is not as sensitive to temperature as anthocyanin synthesis (Mori et al., 2005). In contrast, flavonol synthesis is mainly regulated by the exposure to UV-B radiation (Martínez-Lüscher et al., 2014). Flavonols accumulate in the outer layers of plant tissues screening a great part of solar UV radiation. The involvement of flavonols in the signaling and alleviation of oxidative stress was suggested (Agati et al., 2013; Watkins et al., 2017). However, our results do not provide strong evidence that flavonols may help to cope with high temperatures witnessed during heat waves. Therefore, when grape berries under field conditions are exposed to solar radiation with a subsequent increase in fruit temperature, there was a net synthesis flavonols up to mid/high levels of exposure (∼48% canopy porosity). However, their concentration then rapidly decrease as berries become overexposed with the heat waves (Torres et al., 2020). Our results presented herein corroborated this finding, where grapes from Exposed and Shaded plants reached similar concentrations of flavonols at harvest through two different levels of exposure. The behavior of berries were statistically separated as such in the Exposed thorough higher rates of biosynthesis; and then degradation, and in the Shaded, through lower rates of biosynthesis. Furthermore, Exposed berries only displayed a 17–27% decrease in flavonols. The berries in the Shaded treatments did not experience a decrease in flavonols during the heat wave, providing evidence that flavonols have a slightly higher stability than anthocyanins under high temperature. This differential net synthesis/degradation left a footprint in flavonol profile, increasing the proportion of kaempferol and quercetin, in detriment of myricetin and isorhamnetin as reported previously (Pastore et al., 2017; Martínez-Lüscher et al., 2019a; Torres et al., 2020).
Heat Wave and Exposure Damage
In addition to the changes in chemical composition in the remaining fruit, a substantial part of the berries were completely mummified (at least 10%), and therefore, not sampled. During commercial harvest either through hand picking or with mechanical picking with on-board sorting, clusters and berries may be sorted and the grower may discard up to a 25% of the yield of this trial to optimize quality as desiccated berries may influence wine characteristics (Bonada et al., 2013). At the tissue level, the process of cell death has been characterized under elevated temperature and irrigation deficits (Bonada et al., 2013). It seems plausible that in our work, tissue temperatures of at least 50°C (berry temperature measurements while air temperature was 43.4°C were up to 54°C; data not shown) were enough to induce cell death, either progressively (Supplementary Figures S2E,F), or suddenly (Supplementary Figure S2G). However, to the best of our knowledge the estimation of certain temperature or irradiation thresholds for berry sunburn remain unexplored. In addition, cultural and environmental factors, featuring row orientation, trellis and training system, irrigation, and pre-exposure, may affect the incidence of sunburn for a given air temperature and irradiation (Webb et al., 2010; Zarrouk et al., 2016; Torres et al., 2020). Irrigating prior to an extreme heat event is meant by vineyard operators to increase leaf transpiration, leading to a cooling off effect at the fruit zone similar to overhead irrigation with sprinklers or misting system (Kliewer and Schultz, 1973). In our results, we had slightly higher berry temperatures in Exposed–40%ETc than in Exposed–80%ETc during the warmest part of the day on 114 DAF, but this was not associated to a reduced incidence of sunburn, less anthocyanin degradation or plant fitness. As both irrigation amounts tested in this study had similarly high transpiration rates, it still remains to be tested whether a under low transpiration rates, misting could have ameliorated berry temperatures. Before these results may be extrapolated, it must be noted that vertically shoot positioned trellis systems, compared to sprawling training systems, have few leaf layers around the fruit zone, and thus, increases in transpiration may not influence berry temperature as much. Therefore, the potential benefit of irrigation prior to extreme heat events should not be completely discarded.
Conclusion
After a great number of studies—mostly performed in cooler climates—reporting the beneficial effects of solar radiation and water deficits for fruit ripening and especially flavonoids biosynthesis, new research focus is needed about the detrimental effects of the extreme climatic events such as drought and heat waves. Partial shading can have a positive role in the retention of grape berry skin flavonoids in a scenario of high temperatures and reduced cloud-cover. Conversely, solar exposure did not have a great effect on must acidity as controlled increases in air temperature reported elsewhere. Direct exposure to solar radiation was a necessary cooperator together with extreme heat events to produce fruit damage as practically all the damage recorded in this study was found in Southwest aspects of canopies without shade nets. Doubling irrigation amount had some mild effects lowering berry temperature and avoiding berry dehydration during the last part of ripening. Although doubling the amount of irrigation used may not be justified by this little gain, some additional irrigation prior to the heat events may be of practical use in rain-fed vineyards or with minimal irrigation in a case such as this. This study provided evidence of the necessity of field studies performed during extreme weather events, such as heat waves, to help complement the information gained under constant elevated temperature for the adaptation of cropping systems to climate change.
Data Availability Statement
The raw data supporting the conclusions of this article will be made available by the authors, without undue reservation.
Author Contributions
SK acquired the funding. CC collected the field data. JM-L and LB oversaw the field and lab data collection. JM-L wrote the first version of the manuscript. All authors contributed to the article and approved the submitted version.
Funding
A graduate stipend was provided to CC by the Department of Viticulture and Enology at UC Davis, Horticulture and Agronomy Graduate Group at UC Davis, and American Society for Enology and Viticulture.
Conflict of Interest
The authors declare that the research was conducted in the absence of any commercial or financial relationships that could be construed as a potential conflict of interest.
Supplementary Material
The Supplementary Material for this article can be found online at: https://www.frontiersin.org/articles/10.3389/fpls.2020.579192/full#supplementary-material
Supplementary Figure 1 | Installation of Black-40 shade net 1 m (w) × 6 m (long) along the fruit zone of the experimental plot (A). Installed Black-40 shade net on the Northeast side of the fruit zone of the experimental along the experimental vines (B) In-row spacing: 2.0 m, row spacing 2.4 m.
Supplementary Figure 2 | Appearance of Exposed treatment clusters on the Southwest aspect of canopy (A,B). Clusters on the Southwest aspect of the canopy of Shaded plants with the nets on (C) and same clusters after removing the shade nets (D). Initial damage on clusters few weeks after veraison (E) and progression of the damage through harvest (F). Sudden berry abortion observed on during the heat wave (104 DAF; 1 September) (G).
References
Agati, G., Brunetti, C., Di Ferdinando, M., Ferrini, F., Pollastri, S., and Tattini, M. (2013). Functional roles of flavonoids in photoprotection: new evidence, lessons from the past. Plant Physiol. Biochem. 72, 35–45. doi: 10.1016/j.plaphy.2013.03.014
Arroyo-Currás, N., Rosas-García, V. M., and Videa, M. (2016). Substituent inductive effects on the electrochemical oxidation of flavonoids studied by square wave voltammetry and Ab initio calculations. Molecules 21:1422. doi: 10.3390/molecules21111422
Azuma, A., Yakushiji, H., Koshita, Y., and Kobayashi, S. (2012). Flavonoid biosynthesis-related genes in grape skin are differentially regulated by temperature and light conditions. Planta 236, 1067–1080. doi: 10.1007/s00425-012-1650-x
Bonada, M., Sadras, V., Moran, M., and Fuentes, S. (2013). Elevated temperature and water stress accelerate mesocarp cell death and shrivelling, and decouple sensory traits in Shiraz berries. Irrig. Sci. 31, 1317–1331. doi: 10.1007/s00271-013-0407-z
Brillante, L., Martínez-Luscher, J., Yu, R., Plank, C. M., Sanchez, L., Bates, T. L., et al. (2017). Assessing spatial variability of grape skin flavonoids at the vineyard scale based on plant water status mapping. J. Agric. Food Chem. 65, 5255–5265. doi: 10.1021/acs.jafc.7b01749
Caravia, L., Collins, C., Petrie, P. R., and Tyerman, S. D. (2016). Application of shade treatments during Shiraz berry ripening to reduce the impact of high temperature. Aust. J. Grape Wine Res. 22, 422–437. doi: 10.1111/ajgw.12248
Castellarin, S. D., Di Gaspero, G., Marconi, R., Nonis, A., Peterlunger, E., Paillard, S., et al. (2006). Colour variation in red grapevines (Vitis vinifera L.): genomic organisation, expression of flavonoid 3’-hydroxylase, flavonoid 3’,5’-hydroxylase genes and related metabolite profiling of red cyanidin-/blue delphinidin-based anthocyanins in berry skin. BMC Genomics 7:12. doi: 10.1186/1471-2164-7-12
Castellarin, S. D., Matthews, M. A., Di Gaspero, G., and Gambetta, G. A. (2007). Water deficits accelerate ripening and induce changes in gene expression regulating flavonoid biosynthesis in grape berries. Planta 227, 101–112. doi: 10.1007/s00425-007-0598-8
Castillo-Muñoz, N., Fernández-González, M., Gómez-Alonso, S., García-Romero, E., and Hermosín-Gutiérrez, I. (2009). Red-color related phenolic composition of Garnacha Tintorera (Vitis vinifera L.) grapes and red wines. J. Agric. Food Chem. 57, 7883–7891. doi: 10.1021/jf9002736
Cemeroğlu, B., Velioglu, S., and Isik, S. (1994). Degradation kinetics of anthocyanins in sour cherry juice and concentrate. J. Food Sci. 59, 1216–1218. doi: 10.1111/j.1365-2621.1994.tb14680.x
Chaves, M. M., Zarrouk, O., Francisco, R., Costa, J. M., Santos, T., Regalado, A. P., et al. (2010). Grapevine under deficit irrigation: hints from physiological and molecular data. Ann. Bot. 105, 661–676. doi: 10.1093/aob/mcq030
Cook, M. G., Zhang, Y., Nelson, C. J., Gambetta, G., Kennedy, J. A., and Kurtural, S. K. (2015). Anthocyanin composition of merlot is ameliorated by light microclimate and irrigation in central California. Am. J. Enol. Vitic. 66, 266–278. doi: 10.5344/ajev.2015.15006
Csepregi, K., and Hideg, É (2018). Phenolic compound diversity explored in the context of photo-oxidative stress protection. Phytochem. Anal. 29, 129–136. doi: 10.1002/pca.2720
Dai, Z. W., Meddar, M., Renaud, C., Merlin, I., Hilbert, G., Delrot, S., et al. (2014). Long-term in vitro culture of grape berries and its application to assess the effects of sugar supply on anthocyanin accumulation. J. Exp. Bot. 65, 4665–4677. doi: 10.1093/jxb/ert489
Daryanto, S., Wang, L., and Jacinthe, P.-A. (2016). Global synthesis of drought effects on maize and wheat production. PLoS One 11:e0156362. doi: 10.1371/journal.pone.0156362
Deryng, D., Conway, D., Ramankutty, N., Price, J., and Warren, R. (2014). Global crop yield response to extreme heat stress under multiple climate change futures. Environ. Res. Lett. 9:34011. doi: 10.1088/1748-9326/9/3/034011
Devi, M. J., Taliercio, E. W., and Sinclair, T. R. (2015). Leaf expansion of soybean subjected to high and low atmospheric vapour pressure deficits. J. Exp. Bot. 66, 1845–1850. doi: 10.1093/jxb/eru520
Dosio, A., Mentaschi, L., Fischer, E. M., and Wyser, K. (2018). Extreme heat waves under 1.5°C and 2°C global warming. Environ. Res. Lett. 13:54006. doi: 10.1088/1748-9326/aab827
Drake, J. E., Tjoelker, M. G., Vårhammar, A., Medlyn, B. E., Reich, P. B., Leigh, A., et al. (2018). Trees tolerate an extreme heatwave via sustained transpirational cooling and increased leaf thermal tolerance. Glob. Chang. Biol. 24, 2390–2402. doi: 10.1111/gcb.14037
Fischer, E. M., and Schär, C. (2010). Consistent geographical patterns of changes in high-impact European heatwaves. Nat. Geosci. 3, 398–403. doi: 10.1038/ngeo866
Fraga, H., García de Cortázar Atauri, I., Malheiro, A. C., and Santos, J. A. (2016). Modelling climate change impacts on viticultural yield, phenology and stress conditions in Europe. Glob. Chang. Biol. 22, 3774–3788. doi: 10.1111/gcb.13382
González, C. V., Fanzone, M. L., Cortés, L. E., Bottini, R., Lijavetzky, D. C., Ballaré, C. L., et al. (2015). Fruit-localized photoreceptors increase phenolic compounds in berry skins of field-grown Vitis vinifera L. cv. Malbec. Phytochemistry 110, 46–57. doi: 10.1016/j.phytochem.2014.11.018
Greer, D. H., and Weedon, M. M. (2013). The impact of high temperatures on Vitis vinifera cv. Semillon grapevine performance and berry ripening. Front. Plant Sci. 4:491. doi: 10.3389/fpls.2013.00491
Hall, G. E., Bondada, B. R., and Keller, M. (2011). Loss of rachis cell viability is associated with ripening disorders in grapes. J. Exp. Bot. 62, 1145–1153. doi: 10.1093/jxb/erq355
Hannah, L., Roehrdanz, P. R., Ikegami, M., Shepard, A. V., Shaw, M. R., and Tabor, G. (2013). Climate change, wine, and conservation. Proc. Natl. Acad. Sci. U.S.A. 110, 6907–6912. doi: 10.1073/pnas.1210127110
IPCC (2013). “Climate change 2013: the physical science basis,” in Contribution of Working Group I to the Fifth Assessment Report of the Intergovernmental Panel on Climate Change, eds T. F. Stocker, D. Qin, G.-K. Plattner, M. Tignor, S. K. Allen, J. Boschung, et al. (Cambridge: Cambridge University Press), doi: 10.1017/CBO9781107415324
Jackson, D. I., and Lombard, P. B. (1993). Environmental and management practices affecting grape composition and wine quality. - A Review. Am. J. Enol. Vitic. 44, 409–430.
Kliewer, W. M., and Schultz, H. B. (1973). Effect of sprinkler cooling of grapevines on fruit growth and composition. Am. J. Enol. Vitic. 24, 17–26.
Koch, A., Ebeler, S. E., Williams, L. E., and Matthews, M. A. (2012). Fruit ripening in Vitis vinifera: light intensity before and not during ripening determines the concentration of 2-methoxy-3-isobutylpyrazine in Cabernet Sauvignon berries. Physiol. Plant. 145, 275–285. doi: 10.1111/j.1399-3054.2012.01572.x
Krasnow, M., Matthews, M., Smith, R., Benz, J., Weber, E., and Shackel, K. (2010). Distinctive symptoms differentiate four common types of berry shrivel disorder in grape. Calif. Agric. 64, 155–159. doi: 10.3733/ca.v064n03p155
Kuhn, N., Guan, L., Dai, Z. W., Wu, B. H., Lauvergeat, V., Gomès, E., et al. (2014). Berry ripening: recently heard through the grapevine. J. Exp. Bot. 65, 4543–4559. doi: 10.1093/jxb/ert395
Kurtural, S. K., Wessner, L. F., and Dervishian, G. (2013). Vegetative compensation response of a procumbent grapevine (Vitis vinifera cv. Syrah) cultivar under mechanical canopy management. HortScience 48, 576–583. doi: 10.21273/HORTSCI.48.5.576
Levene, H. (1960). “Robust tests for equality of variances,” in Contributions to Probability and Statistics: Essays in Honor of Harold Hotelling, eds I. Olkin and H. Hotelling (Stanford: Stanford University Press), 278–292.
Martínez-Lüscher, J., Brillante, L., and Kurtural, S. K. (2019a). Flavonol profile is a reliable indicator to assess canopy architecture and the exposure of red wine grapes to solar radiation. Front. Plant Sci. 10:10. doi: 10.3389/fpls.2019.00010
Martínez-Lüscher, J., Plank, C. M., Brillante, L., Cooper, M. L., Smith, R. J., Al-Rwahnih, M., et al. (2019b). Grapevine red blotch virus may reduce carbon translocation leading to impaired grape berry ripening. J. Agric. Food Chem. 67, 2437–2448. doi: 10.1021/acs.jafc.8b05555
Martínez-Lüscher, J., Brillante, L., Nelson, C. C., Al-Kereamy, A. M., Zhuang, S., and Kurtural, S. K. (2017a). Precipitation before bud break and irrigation affect the response of grapevine ‘Zinfandel’ yields and berry skin phenolic composition to training systems. Sci. Hortic. 222, 153–161. doi: 10.1016/j.scienta.2017.05.011
Martínez-Lüscher, J., Chen, C. C. L., Brillante, L., and Kurtural, S. K. (2017b). Partial solar radiation exclusion with color shade nets reduces the degradation of organic acids and flavonoids of grape berry (Vitis vinifera L.). J. Agric. Food Chem. 65, 10693–10702. doi: 10.1021/acs.jafc.7b04163
Martínez-Lüscher, J., Sánchez-Díaz, M., Delrot, S., Aguirreolea, J., Pascual, I., and Gomès, E. (2014). Ultraviolet-B radiation and water deficit interact to alter flavonol and anthocyanin profile in grapevine berries through transcriptomic regulation. Plant Cell Physiol. 55, 1925–1936. doi: 10.1093/pcp/pcu121
Matus, J. T. (2016). Transcriptomic and metabolomic networks in the grape berry illustrate that it takes more than flavonoids to fight against ultraviolet radiation. Front. Plant Sci. 7:1337. doi: 10.3389/fpls.2016.01337
Mori, K., Goto-Yamamoto, N., Kitayama, M., and Hashizume, K. (2007). Loss of anthocyanins in red-wine grape under high temperature. J. Exp. Bot. 58, 1935–1945. doi: 10.1093/jxb/erm055
Mori, M., Yoshida, K., Ishigaki, Y., Matsunaga, T., Nikaido, O., Kameda, K., et al. (2005). UV-B protective effect of a polyacylated anthocyanin, HBA, in flower petals of the blue morning glory, Ipomoea tricolor cv. Heavenly Blue. Bioorg. Med. Chem. 13, 2015–2020. doi: 10.1016/j.bmc.2005.01.011
Ollat, N., Touzard, J.-M., and van Leeuwen, C. (2016). Climate change impacts and adaptations: new challenges for the wine industry. J. Wine Econ. 11, 139–149. doi: 10.1017/jwe.2016.3
Pastore, C., Allegro, G., Valentini, G., Muzzi, E., and Filippetti, I. (2017). Anthocyanin and flavonol composition response to veraison leaf removal on cabernet sauvignon, nero d’avola, raboso piave and sangiovese Vitis vinifera L. cultivars. Sci. Hortic. (Amsterdam). 218, 147–155. doi: 10.1016/j.scienta.2017.01.048
Pastore, C., Zenoni, S., Fasoli, M., Pezzotti, M., Tornielli, G. B., and Filippetti, I. (2013). Selective defoliation affects plant growth, fruit transcriptional ripening program and flavonoid metabolism in grapevine. BMC Plant Biol. 13:30. doi: 10.1186/1471-2229-13-30
Poni, S., Bernizzoni, F., Civardi, S., and Libelli, N. (2009). Effects of pre-bloom leaf removal on growth of berry tissues and must composition in two red Vitis vinifera L. cultivars. Aust. J. Grape Wine Res. 15, 185–193. doi: 10.1111/j.1755-0238.2008.00044.x
Restaino, C. M., Peterson, D. L., and Littell, J. (2016). Increased water deficit decreases Douglas fir growth throughout western US forests. Proc. Natl. Acad. Sci. U.S.A. 113, 9557–9562. doi: 10.1073/pnas.1602384113
Rienth, M., Torregrosa, L., Sarah, G., Ardisson, M., Brillouet, J.-M., and Romieu, C. (2016). Temperature desynchronizes sugar and organic acid metabolism in ripening grapevine fruits and remodels their transcriptome. BMC Plant Biol. 16:164. doi: 10.1186/s12870-016-0850-0
Robson, M. T., Klem, K., Urban, O., and Jansen, K. M. A. (2015). Re-interpreting plant morphological responses to UV-B radiation. Plant. Cell Environ. 38, 856–866. doi: 10.1111/pce.12374
Sadras, V. O., and Moran, M. A. (2012). Elevated temperature decouples anthocyanins and sugars in berries of Shiraz and Cabernet Franc. Aust. J. Grape Wine Res. 18, 115–122. doi: 10.1111/j.1755-0238.2012.00180.x
Sarni-Manchado, P., Cheynier, V., and Moutounet, M. (1997). Reactions of polyphenoloxidase generated caftaric acid o-quinone with malvidin 3-O-glucoside. Phytochemistry 45, 1365–1369. doi: 10.1016/s0031-9422(97)00190-8
Smith, M. D. (2011). The ecological role of climate extremes: current understanding and future prospects. J. Ecol. 99, 651–655. doi: 10.1111/j.1365-2745.2011.01833.x
Sweetman, C., Sadras, V. O., Hancock, R. D., Soole, K. L., and Ford, C. M. (2014). Metabolic effects of elevated temperature on organic acid degradation in ripening Vitis vinifera fruit. J. Exp. Bot. 65, 5975–5988. doi: 10.1093/jxb/eru343
Terry, D. B. D. B., and Kurtural, S. K. K. (2011). Achieving vine balance of Syrah with mechanical canopy management and regulated deficit irrigation. Am. J. Enol. Vitic. 62, 426–437. doi: 10.5344/ajev.2011.11022
Tinyane, P. P., Soundy, P., and Sivakumar, D. (2018). Growing ‘Hass’ avocado fruit under different coloured shade netting improves the marketable yield and affects fruit ripening. Sci. Hortic. 230, 43–49. doi: 10.1016/j.scienta.2017.11.020
Torres, N., Martínez-Lüscher, J., Porte, E., and Kurtural, S. K. (2020). Optimal ranges and thresholds of grape berry solar radiation for flavonoid biosynthesis in warm climates. Front. Plant Sci. 11:931. doi: 10.3389/fpls.2020.00931
Trenberth, K. E., and Fasullo, J. T. (2009). Global warming due to increasing absorbed solar radiation. Geophys. Res. Lett. 36:L07706. doi: 10.1029/2009gl037527
Watkins, J. M., Chapman, J. M., and Muday, G. K. (2017). Abscisic acid-induced reactive oxygen species are modulated by flavonols to control stomata aperture. Plant Physiol. 175, 1807–1825. doi: 10.1104/pp.17.01010
Webb, L., Whiting, J., Watt, A., Hill, T., Wigg, F., Dunn, G., et al. (2010). Managing grapevines through severe heat: a survey of growers after the 2009 summer heatwave in south-eastern Australia. J. Wine Res. 21, 147–165. doi: 10.1080/09571264.2010.530106
Williams, L. E., and Ayars, J. E. (2005). Grapevine water use and the crop coefficient are linear functions of the shaded area measured beneath the canopy. Agric. For. Meteorol. 132, 201–211. doi: 10.1016/j.agrformet.2005.07.010
Yamane, T., Jeong, S. T., Goto-Yamamoto, N., Koshita, Y., and Kobayashi, S. (2006). Effects of temperature on anthocyanin biosynthesis in grape berry skins. Am. J. Enol. Vitic. 57, 54–59.
Yu, R., Brillante, L., Martínez-Lüscher, J., and Kurtural, S. K. (2020). Spatial variability of soil and plant water status and their cascading effects on grapevine physiology are linked to berry and wine chemistry. Front. Plant Sci. 11:790. doi: 10.3389/fpls.2020.00790
Yu, R., Cook, M. G., Yacco, R. S., Watrelot, A. A., Gambetta, G., Kennedy, J. A., et al. (2016). Effects of leaf removal and applied water on flavonoid accumulation in grapevine (Vitis vinifera L. cv. Merlot) berry in a hot climate. J. Agric. Food Chem. 64, 8118–8127. doi: 10.1021/acs.jafc.6b03748
Yu, R., and Kurtural, S. K. (2020). Proximal sensing of soil electrical conductivity provides a link to soil-plant water relationships and supports the identification of plant water status zones in vineyards. Front. Plant Sci. 11:244. doi: 10.3389/fpls.2020.00244
Keywords: climate change, water stress, shade nets, flavonoids, heat wave, irrigation, fruit exposure, anthocyanin degradation
Citation: Martínez-Lüscher J, Chen CCL, Brillante L and Kurtural SK (2020) Mitigating Heat Wave and Exposure Damage to “Cabernet Sauvignon” Wine Grape With Partial Shading Under Two Irrigation Amounts. Front. Plant Sci. 11:579192. doi: 10.3389/fpls.2020.579192
Received: 01 July 2020; Accepted: 20 October 2020;
Published: 10 November 2020.
Edited by:
Chiara Pastore, University of Bologna, ItalyReviewed by:
Lars Hendrik Wegner, Foshan University, ChinaVirginia Ferrari, National Institute for Agricultural Research (INIA), Uruguay
Copyright © 2020 Martínez-Lüscher, Chen, Brillante and Kurtural. This is an open-access article distributed under the terms of the Creative Commons Attribution License (CC BY). The use, distribution or reproduction in other forums is permitted, provided the original author(s) and the copyright owner(s) are credited and that the original publication in this journal is cited, in accordance with accepted academic practice. No use, distribution or reproduction is permitted which does not comply with these terms.
*Correspondence: Sahap Kaan Kurtural, skkurtural@ucdavis.edu
†ORCID: Johann Martínez-Lüscher, orcid.org/0000-0002-3077-1346; Luca Brillante, orcid.org/0000-0002-5747-6312; Sahap Kaan Kurtural, orcid.org/0000-0001-9578-831X
‡Present address: Johann Martínez-Lüscher, SemiosBio Technologies, Vancouver, BC, Canada; Luca Brillante, Department of Viticulture and Enology, California State University Fresno, Fresno, CA, United States