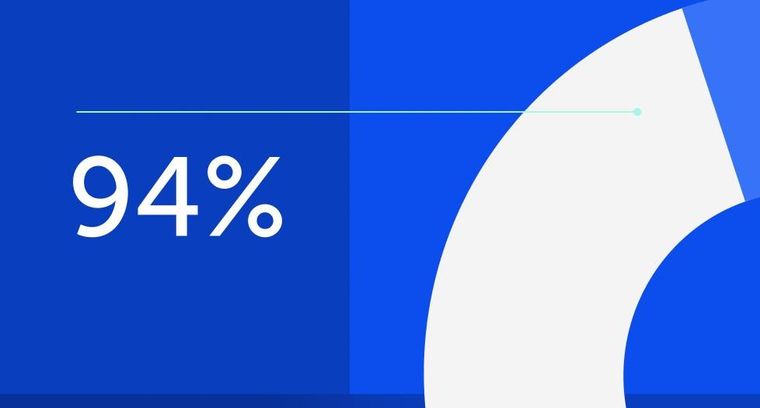
94% of researchers rate our articles as excellent or good
Learn more about the work of our research integrity team to safeguard the quality of each article we publish.
Find out more
ORIGINAL RESEARCH article
Front. Plant Sci., 12 November 2020
Sec. Plant Systematics and Evolution
Volume 11 - 2020 | https://doi.org/10.3389/fpls.2020.577536
This article is part of the Research TopicMobile Elements and Plant Genome Evolution, Comparative Analyses and Computational ToolsView all 8 articles
Traditionally, reference genomes in crop species rely on the assembly of one accession, thus occulting most of intraspecific diversity. However, rearrangements, gene duplications, and transposable element content may have a large impact on the genomic structure, which could generate new phenotypic traits. Comparing two Brassica rapa genomes recently sequenced and assembled using long-read technology and optical mapping, we investigated structural variants and repetitive content between the two accessions and genome size variation among a core collection. We explored the structural consequences of the presence of large repeated sequences in B. rapa ‘Z1’ genome vs. the B. rapa ‘Chiifu’ genome, using comparative genomics and cytogenetic approaches. First, we showed that large genomic variants on chromosomes A05, A06, A09, and A10 are due to large insertions and inversions when comparing B. rapa ‘Z1’ and B. rapa ‘Chiifu’ at the origin of important length differences in some chromosomes. For instance, lengths of ‘Z1’ and ‘Chiifu’ A06 chromosomes were estimated in silico to be 55 and 29 Mb, respectively. To validate these observations, we compared using fluorescent in situ hybridization (FISH) the two A06 chromosomes present in an F1 hybrid produced by crossing these two varieties. We confirmed a length difference of 17.6% between the A06 chromosomes of ‘Z1’ compared to ‘Chiifu.’ Alternatively, using a copy number variation approach, we were able to quantify the presence of a higher number of rDNA and gypsy elements in ‘Z1’ genome compared to ‘Chiifu’ on different chromosomes including A06. Using flow cytometry, the total genome size of 12 Brassica accessions corresponding to a B. rapa available core collection was estimated and revealed a genome size variation of up to 16% between these accessions as well as some shared inversions. This study revealed the contribution of long-read sequencing of new accessions belonging to different cultigroups of B. rapa and highlighted the potential impact of differential insertion of repeat elements and inversions of large genomic regions in genome size intraspecific variability.
Genetic and phenotypic diversity are the drivers of plant evolution and adaptation. While ecology and population genetics provide important knowledge on the molecular processes underlying the astonishing plant biodiversity, comparative genomic analyses are essential to understand the large-scale genomic variations that can be observed within species and how these structural variations may impact plant phenotypes. Resequencing of accessions and construction of pangenomes of plant species such as wheat (Montenegro et al., 2017), maize (Gage et al., 2019), rice (Schatz et al., 2014; Wang et al., 2018; Zhao et al., 2018), or soybean (Lam et al., 2010; Li et al., 2014) have revealed the involvement of structural variations in various agronomically relevant traits. In maize, structural variants are implicated in plant architecture, flowering, and disease resistance (Walker et al., 1995; Chia et al., 2012; Lu et al., 2015). Similarly, using pangenomes, numerous structural variations have been revealed in Brassica napus varieties correlating with genes and phenotypic traits such as silique length and seed weight (Gazave et al., 2016), disease resistance (Dolatabadian et al., 2020), as well as flowering time and vernalization (Song et al., 2020). Structural variants identified as underlying flowering time were associated with the insertion of transposable elements (TEs) in three Flowering Locus C (or FLC) genes (Song et al., 2020). However, structural diversity in the progenitors of this allopolyploid species has, so far, been particularly overlooked.
Only recently, the development of Brassica pangenomes has started to reveal the extensive number of structural variants within one of the two diploid progenitors Brassica oleracea (Golicz et al., 2016) and assessed in regard to the domestication process of the subsequent allotetraploids Brassica juncea (Paritosh et al., 2019) and B. napus (Song et al., 2020). These studies highlight the importance of structural variants and gene copy number variation (CNVs) in adaptive phenotypes. While core genes represent 80% of the assembled genomes, each new accession brings around 20% of novel genes. In most of these analyses, however, the occurrence and importance of the repeat compartment are undervalued.
TEs form an important internal source of genetic diversity as a result of their ability to create mutations, alter gene expression, and promote chromosomal mispairing (Kidwell and Lisch, 2000). These ancient, ubiquitous, and dynamic components of eukaryotic genomes comprise up to 58% of the allopolyploid genome of B. napus (Song et al., 2020). TEs comprise two classes that have contrasted mode of replication, each divided in several families: retrotransposons (Class I) transpose via reverse transcription of their messenger RNA and DNA transposons (Class II), encompassing all other types of TEs (Wicker et al., 2007). Overall, TEs have major impact on genome organization and function and have been associated with up-regulated genes in response to various abiotic stresses, environmental gradients, reproductive mode (asexuality, selfing vs. outcrossing), interspecific hybrid formation, and polyploidy (Wright and Schoen, 1999; Kalendar et al., 2000; Ungerer et al., 2006; Makarevitch et al., 2015; Vicient and Casacuberta, 2017). Several studies from different biological systems indicate that the patterns (position and number of copies) of a single TE family can vary within species (Quadrana et al., 2016). However, the study of TEs inserted in complex genomes of various populations is challenging and rely on the amplification of specific TE sequences (for instance using Sequence-Specific Amplification Polymorphism transposon display, Parisod et al., 2009) or low-coverage sequencing strategies (Ferreira de Carvalho et al., 2016). The utilization of long-read sequencing and optical mapping in several varieties of the same species is allowing fine comparative genomic analysis of the differential proliferation of TEs and other repeats. This overlooked component of plant genomes is getting accessible and provides important insights on the structural genomic diversity standing within species.
In the present study, we conducted a fine comparative genomic analysis of two varieties of Brassica rapa ‘Chiifu’ and ‘Z1.’ B. rapa (AA, 2n = 20) is one of the diploid progenitors of the important allotetraploid oilseed crops, B. juncea (AABB, 2n = 36), and B. napus (AACC, 2n = 38) (U, 1935). Therefore, B. rapa varieties can be used to broaden the genetic diversity of these domesticated species. Brassica diploid species arose from a whole genome triplication that occurred about 15–20 million years ago (Murat et al., 2015). Diploid Brassica are thus considered as paleohexaploids. Thereafter, their duplicated genomes rapidly underwent extensive gene deletion through a fractionation process (Murat et al., 2015). The majority of the genes are tending toward a return to single-copy status (Wang et al., 2011), and overall gene content in Brassica diploid genomes has so far been reduced by about half. Yet, different cultigroups within Brassica species have selected different alleles from the same duplicated genes explaining part of the astonishing and extensive number of morphotypes existing within each species (Cheng et al., 2014, 2016b; Golicz et al., 2016). B. rapa speciation started after the divergence with B. oleracea estimated 2.18 Mya (Li et al., 2017), but its diversification occurred mainly during the domestication process of B. rapa crops, 3,500 years BC in the Mediterranean basin with a secondary center of diversification in Asia (Guo et al., 2014). This resulted in genetically (Aissiou et al., 2018) and phenotypically diverse morphotypes (leafy, root or fodder, and oilseed types). We focus on two subspecies, ssp. pekinensis (Chinese cabbage with ‘Chiifu’) and ssp. trilocularis (yellow sarson with ‘Z1’). Chinese cabbage has originated in central China by natural hybridization between Pak-choi (ssp. chinensis) and turnip rape (ssp. oleifera) 1,200–2,100 years ago (Song et al., 1990; Qi et al., 2017). Yellow sarson originated from India, but its precise origin is still unknown. Overall, the underlying structural genomic diversity has never been explored within this important crop.
Using established protocols (flow cytometry and cytogenetics), along with state-of-the-art whole genome sequencing and optical mapping for assembly of complex Brassica genomes, we compared structural rearrangements between two varieties of B. rapa that have contrasted morphotypes, evolutionary history and reproductive mode. Specifically, we identified differential insertion of ribosomal DNA and LTR retrotransposons and reconstructed the evolution of these repeats during B. rapa domestication. Finally, we unraveled wide variation in DNA content among different accessions belonging to B. rapa core collection.
Two B. rapa genotypes, B. rapa var. pekinensis cabbage ‘Chiifu’ (pure line), and B. rapa var. trilocularis ‘Z1’ (doubled haploid line), as well as their F1 hybrid (‘Z1’ × ‘Chiifu’), were grown in semi-controlled conditions in a greenhouse. Seven to 10 individuals of each B. rapa accession representing biological replicates were harvested for leaves and roots. Leaf tissue was used for molecular work and flow cytometry, whereas root meristem samples were used to perform fluorescent in situ hybridization (FISH) experiments. In addition, 10 accessions representing the different groups of B. rapa core collection (Zhao et al., 2010; Cheng et al., 2016b) were chosen and grown in a similar environment for assessing genome size variation. Fresh leaves from three to eight individuals, used as biological replicates, were harvested per accession: from group 1, one accession of ssp. pekinensis; from group 2, one accession of ssp. narinosa; from group 3, one accession of ssp. perviridis and one accession of ssp. nipposinica; from group 4, two different accessions of ssp. oleifera; from group 5, one accession of ssp. trilocularis; from group 6, three different accessions of ssp. rapa (for thorough descriptions see Cheng et al., 2016c). Leaf tissue is then directly processed for flow cytometry analyses (Supplementary Table 1).
The latest genome assemblies available for B. rapa ‘Z1’ (Belser et al., 2018) and ‘Chiifu’ v.3.0 (Zhang et al., 2018) were retrieved from the Genoscope website1 and the Brassica database2, respectively. These genomes were subjected to the same TE annotation package REPET V2.5 (Quesneville et al., 2005; Flutre et al., 2011; Hoede et al., 2014). Briefly, the REPET pipeline was used for the detection, classification (TEdenovo) and annotation of TEs (TEannot). The TEdenovo pipeline identifies TE copies present on a reference genome using a curated database of TEs: Repbase (V 20.05 for both nucleotides and amino acid sequences). Then, TEdenovo creates de novo consensus sequences when at least five copies of the same TE family are retrieved within the genome. Next, TEannot pipeline uses these consensus sequences as well as Repbase to annotate the provided reference genomes. Default parameters were set for this last step of annotation. Finally, two files containing the structural annotation of ‘Z1’ and ‘Chiifu’ were output in GFF3 format (Generic Feature Format version 3). The resulting GFF3 files were hand curated using custom python scripts (Python version 2.7.12).
To evaluate large-scale genome structural rearrangements, the assembled genomes of B. rapa ‘Z1’ and ‘Chiifu,’ as well as the A subgenome of B. napus ‘Darmor Bzh’ V4.1, were retrieved from the Brassica database2 and compared. The package MUMmer V3.23 (Kurtz et al., 2004) was used on each of the 10 chromosomes using the following settings: -id 95, -l 4,000. Inversions or deletions observed in ‘Z1’ when compared to ‘Chiifu’ were further verified using BioNano raw data from Belser et al. (2018). To better characterize these genomic regions, gene annotations were retrieved from GFF files. Gene Ontology (GO) enrichment was performed on GO terms of the identified genes present in specifically inserted regions of ‘Z1’ using Agrigo V2 (Tian et al., 2017). Finally, we assessed the duplication status of these genes using proto-Calepineae karyotype (PCK; Murat et al., 2015) and custom-made python scripts. In addition, among all inversions, three showing defined breakpoints in both ‘Z1’ and ‘Chiifu’ were chosen and validated using custom-made polymerase chain reaction (PCR) primers designed in flanking regions of inversions and specific to either ‘Z1’ or ‘Chiifu’ accessions (Supplementary Table 2).
To validate the presence of repeated sequences on the chromosome A06 of B. rapa ‘Z1,’ FISH was carried out in ‘Z1,’ ‘Chiifu’ and the F1 hybrid ‘Z1’ × ‘Chiifu’ according to protocols detailed in Książczyk et al. (2011). The two BAC clones KBrB022P06 and KBrH003P24 hybridizing on each of the A06 chromosome arms in B. rapa (Xiong and Pires, 2011) were labeled by random priming with biotin-14-dUTP (Invitrogen, Life Technologies). The ribosomal probe used in this study was pTa-71 (Gerlach and Bedbrook, 1979), which contained a 9-kb EcoRI fragment of rDNA repeat unit (18S-5.8S-26S genes and spacers) isolated from Triticum aestivum. pTa-71 was labeled with Alexa-488 dUTP by random priming. Biotinylated probes were immunodetected by Texas red avidin DCS (Vector Laboratories), and the signal was amplified with biotinylated anti-avidin D (Vector Laboratories). The chromosomes were mounted and counterstained in Vectashield (Vector Laboratories) containing 2.5 μg/mL 4′,6-diamidino-2-phenylindole (DAPI). Fluorescence images were captured using an ORCA-Flash4 (Hamamatsu, Japan) on an Axioplan 2 microscope (Zeiss, Oberkochen, Germany) and analyzed using Zen 2 PRO software (Carl Zeiss, Germany). Finally, the lengths of both A06 chromosomes in the hybrid ‘Z1’ × ‘Chiifu’ were estimated on 14 cells at the mitotic metaphase stage and measured using the visualization software Zen 2 PRO (Carl Zeiss, Germany).
CNVs of 45S rDNA (otherwise called 25S or 35S rDNA) and gypsy LTR Retrotransposons were obtained using Shotgun data for B. rapa var. pekinensis ‘Chiifu’ (from2) and B. rapa var. trilocularis ‘Z1’ (from3), applying a method based on a calibration using the CNV (based on read depth approach) of single copy genes (scripts available at github/juboutte). First, 17,954 putative single copy genes in ‘Chiifu’ and ‘Z1’ were identified using custom-made python scripts based on PCK genes from B. rapa (Murat et al., 2015). Second, 38 rDNA sequences, corresponding to Brassica rDNA full-length sequences downloaded from the NCBI (last accessed August 5, 2019) and 96 gypsy sequences downloaded from the gypsy database (last accessed August 5, 2019)4, were retrieved as reference sequences. Then, rDNA and gypsy sequences of ‘Chiifu’ and ‘Z1’ were identified by performing BLASTn and tBLASTn algorithms (e-value threshold of 10–5; Altschul et al., 1997) between the rDNA and gypsy reference sequences and the two assembled genomes ‘Chiifu’ v.3.0 (Zhang et al., 2018) and ‘Z1’ (Belser et al., 2018). Finally, consensus sequences (two rDNA and 211 gypsy) were obtained overall for ‘Z1’ and ‘Chiifu’ using python custom script (sequences presenting more than 90% identity were combined together) and aligned with Mafft v.7.245; option –auto (Katoh and Standley, 2013). Visual checking of the alignments was done using Geneious v.10.0.9 (Kearse et al., 2012). ‘Chiifu’ and ‘Z1’ genomic reads were then mapped on the two 45S rDNA consensus, the 211 gypsy RT consensus and the 17,954 putative single copy genes using Bowtie 2, v.2.2.7 (Langmead and Salzberg, 2012) using the parameters G, 52, 6 and G, 58, 3 for ‘Chiifu’ and ‘Z1,’ respectively.
To study the evolutionary relationships of 45S rDNA and gypsy sequences of B. rapa ‘Chiifu’ and ‘Z1,’ phylogenetic analyses were performed. First, 45S rDNA and gypsy sequences (first translated to protein sequences and merged to the 96 gypsy database sequences) of ‘Chiifu’ and ‘Z1’ were aligned separately using Mafft alignment v.7.245; option –auto (Katoh and Standley, 2013). Second, beginning and end of the matrices with less than 25% of the sequences were deleted, as well as indels present in more than 25% of the sequences. Phylogenetic analyses were performed using Geneious tree builder with the Jukes–Cantor model and the neighbor-joining method (45S rDNA clade support was determined by 1,000 bootstrap replicates). To avoid Long Branch Attraction error, sequences presenting long branches were deleted, and new phylogenetic analyses were performed using the parameters described above.
In order to explore the intraspecific variability of genome size among the different varieties of B. rapa, we assessed by flow cytometry, the DNA content, and estimated genome size for the focal accessions ‘Chiifu’ and ‘Z1,’ as well as 10 other accessions representing B. rapa intraspecific diversity. Briefly, approximately 4 mg of fresh leaves of B. rapa and Pisum sativum (var. Victor; used as an internal reference standard) were harvested and transferred to a Petri dish. This material was chopped using a sharp razor blade in 500 μL of staining buffer (from Cystain PI OxProtect) and incubated at room temperature for 30–90 s. The solution was then filtered through a 50 μm nylon mesh, and 1.5 mL of solution (0.0166 mg of RNase A and 10 μL of propidium iodide) was added per sample. Incubation at room temperature was made for 30–60 min, protected from light. Estimation of genome size for each accession was obtained using a CyFlow space cytometer (Partec Inc.). This instrument was equipped with a 488 nm blue laser 50 mW and a band-pass filter LP590 used as an emission filter. Also, prior to running the samples, gain and linearity of the instrument were adjusted by using DNA control PI from Sysmex. Finally, G1 peaks in B. rapa accessions and P. sativum were collected for each sample to calculate nuclear DNA content (1C in pg) and haploid genome size (in Mbp).
Proportions of repeat elements in both B. rapa ‘Z1’ and B. rapa ‘Chiifu’ and proportions of sequences within each LTR gypsy clade were investigated using χ2-test. Genome size variations were investigated using analysis of variance (ANOVA) and Tukey pairwise comparisons [honestly significant difference (HSD)] tests. Normality of data was first tested by Shapiro test. Statistical tests were performed using R software v.3.5.1 (R Core Team, 2018).
Structural comparison of the B. rapa ‘Z1’ genome against both B. rapa ‘Chiifu’ genome and B. napus ‘Darmor Bzh’ A subgenome revealed six large sequences specific to B. rapa ‘Z1’ on A01, A05 (two regions), A06, A08, and A09 (Table 1, see also Figure 1). These regions were qualified as “specific” because no orthologous match could be found in the assembled Brassica genomes tested in this study. These six regions with a mean length of 12,179,136 bp (median = 9,811,381 bp) were composed of a mean number of TEs, rDNA, and genes of 4,244.5 (median = 2,878), 25.5 (median = 19), and 160.5 (median = 135.5), respectively (Table 1). A total of 963 genes were found in the regions specific to ‘Z1.’ On these 963 genes, 439 genes were only detected in the ‘Z1’ genome without any homologous similarity in ‘Chiifu’ genome. GO enrichment was performed for the genes localized in the ‘Z1’-specific regions; however, no specific pathway was revealed. Similarly, we were not able to detect enrichment of duplicated genes that may be localized in these regions. Moreover, these six ‘Z1’ regions contained an important percentage of “N” sequences (mean = 30.59%, median = 26.38%). The observed “N” percentage in these regions was associated with the scaffolding of long reads due to optical mapping. Thus, we might lack genetic information, but thanks to optical mapping, we are confident on the size of these structural variants.
Table 1. Position and length of the B. rapa ‘Z1’ genome-specific regions (in comparison to B. rapa ‘Chiifu’).
Figure 1. Dot plot comparing the assembly chromosome of B. rapa ‘Z1’ against B. rapa ‘Chiifu’ and B. napus ‘Darmor Bzh’ A subgenome for the chromosomes A05 (A), A06 (B), and A09 (C). Gray boxes corresponded to regions specific to ‘Z1’ genome. Only alignment blocks with greater than 95% of identity and a length greater than 4,000 bp were shown.
Additionally, 81 inverted regions were identified on all chromosomes between ‘Z1’ (cumulative length = 23,066,463 bp) and ‘Chiifu’ (cumulative length = 176,703,831 bp) reciprocally (Supplementary Table 3). Inversions in ‘Z1’ and ‘Chiifu’ had a mean length of 281,771 bp (median = 72,650 bp) and 2,181,528 bp (median = 107,269 bp), respectively. A total of 22,274 (mean = 274.99, median = 14) and 21,780 (mean = 268.89, median = 13) genes were identified within ‘Z1’ and ‘Chiifu’ inversions, respectively. A total of 9,240 (mean = 114.07, median = 35) and 112,578 (mean = 1,389.85, median = 63) TEs were identified within ‘Z1’ and ‘Chiifu’ inversions, respectively. The cumulative length of TEs within the inversions corresponded to 49.86 and 44.15% of the total length of the inversions in ‘Z1’ and ‘Chiifu,’ respectively. By contrast, a very low number of rDNA sequences were identified in these inversions (with overall three and five rDNA sequences in ‘Z1’ and ‘Chiifu,’ respectively; Supplementary Table 3). Among these inversions, we were able to validate three inversions between ‘Z1’ and ‘Chiifu,’ of which two were present on chromosome A05 (located from 6.48–6.50 and 6.65–7.03 Mb) and one on chromosome A10 (located from 18.24–19.57 Mb) using PCR (Supplementary Figure 1).
The proportions of repeat elements (including TEs and ribosomal DNA) were estimated in B. rapa ‘Z1’ and B. rapa ‘Chiifu.’ TEs were annotated along the 10 chromosomes of both genomes with higher proportions in the centromeric regions. Considering a genome size of 529 Mb for B. rapa ‘Z1’ and ‘Chiifu’ after Johnston et al. (2005), TEs may represent 31.73 and 22.16% of ‘Z1’ and ‘Chiifu’ genomes, respectively. However, considering the genome sizes determined in this study by flow cytometry (577 and 545 Mb on average for ‘Z1’ and ‘Chiifu,’ respectively, see below), TEs may represent 29.09 and 21.50% of ‘Z1’ and ‘Chiifu’ genomes respectively. LINEs and LTR (including Copia and gypsy) TEs represent 3.02 and 8.58% of the ‘Z1’ genome, respectively. These proportions were also determined for the ‘Chiifu’ genome with 2.92 and 4.32% for LINEs and LTR TEs, respectively.
In B. rapa ‘Z1,’ several chromosomes (especially the A05 and A06 chromosomes) had a higher proportion of TEs compared to ‘Chiifu’ assembled chromosomes. As expected, regions rich in TEs had a lower proportion of genes. Ribosomal sequences were observed in the 10 chromosomes of both genomes with a higher proportion on the A01, A05, A06, and A09 compared to other chromosomes of ‘Z1’ (Figure 2). As the short arm of the chromosome A03 (bearing the nucleolar organizer region, including a large domain of 45S rDNA) was not assembled, no rDNA was observed (Mun et al., 2010). These results were confirmed by CNV analysis, which allowed estimating the number of 45S rDNA copies to 1,416 and 1,750 in ‘Chiifu’ and ‘Z1,’ respectively. Interestingly, the regions presenting a high number of rDNA and TEs corresponded to the six regions specific to the ‘Z1’ genome (Table 1 and Figure 1). Proportions of each TE order in the two genomes were also investigated using χ2 test. No significant difference was observed between ‘Chiifu’ chromosomes; however, statistical differences were observed between ‘Z1’ chromosomes (χ2-test; α = 0.05). Indeed, chromosomes A05 and A06 had a higher percentage of LTR TEs in comparison to the other chromosomes (Figure 3 and Supplementary Table 4). CNV analysis confirmed that the proportion of LTR gypsy was higher in the ‘Z1’ genome (1,626 copies) compared to the ‘Chiifu’ genome (1,347 copies).
Figure 2. Circular diagrams of the chromosomes of (A) B. rapa ‘Chiifu’ and (B) B. rapa ‘Z1.’ Black boxes represent chromosomes centromeric regions. Rings represent: (i) density of transposable elements, (ii) density of LTR, (iii) density of genes, and (iv) presence–absence of ribosomal DNA (45S in blue and 5S in yellow).
Figure 3. Transposable element proportions in the 10 chromosomes of (A) B. rapa ‘Chiifu’ and (B) B. rapa ‘Z1.’ Class I and Class II corresponded to unclassified TEs within their respective Class.
As the large specific sequences of ‘Z1’ were made predominantly of rDNA and LTR gypsy, phylogenies of both repeated elements were performed to explore their evolutionary dynamics. Ribosomal DNA phylogeny of the ‘Chiifu’ and ‘Z1’ genomes allowed us to identify three clades, one common to the two B. rapa samples (clade 3) and two containing only ‘Z1’ sequences (clades 1 and 2). These two clades contained 67 and 96 ‘Z1’ rDNA sequences, respectively, coming from chromosomes A01, A05, A06, and A09 (Figure 4A). LTR gypsy phylogeny allowed us to identify six major clades (Figure 4B). Proportions of sequences within each LTR gypsy clade were not significantly different between ‘Chiifu’ and ‘Z1’ (χ2-test; a = 0.05).
Figure 4. (A) Neighbor-joining phylogram of the rDNA of 168 B. rapa ‘Chiifu’ sequences (in blue) and 271 B. rapa ‘Z1’ sequences (in red). Clades 1 and 2 in red included only ‘Z1’ sequences. Clade 3 in purple included ‘Chiifu’ and ‘Z1’ sequences. (B) Neighbor-joining phylogram and classification of LTR gypsy elements of 2,447 B. rapa ‘Chiifu’ sequences, 2,908 B. rapa ‘Z1’ sequences and 60 gypsy sequences from the gypsy database for identifying major clades of TEs.
Fluorescence in situ hybridization was performed on ‘Z1,’ ‘Chiifu,’ and F1 ‘Z1’ × ‘Chiifu’ individuals to compare their A06 chromosomes, presenting high length variation. The 45S probe (green fluorescence) on B. rapa marked five different chromosome pairs (Figure 5). The strong FISH signal located on the A03 chromosomes likely reflected a large number of genes in the array of the 45S rDNA sequence. A second rDNA-rich locus was located on A01 chromosome, close to the centromere. BAC-FISH analysis with two specific BACs of A06 chromosome arms (red fluorescence) revealed that the 45S rDNA probe hybridized to a major site on B. rapa ‘Chiifu’ (Figures 5A–C), a minor site on B. rapa ‘Z1’ (Figures 5D–F) and mixed-intensity sites, one major and one minor, on hybrid ‘Z1’ × ‘Chiifu’ (Figures 5G–I). Moreover, in B. rapa, the individual mitotic metaphase chromosome A06 ranged from 2.1 to 5.6 μm in length (observed from 14 metaphase sets). The chromosome A06 from ‘Chiifu’ was on average 17.6% (±6.96%) smaller compared to the chromosome A06 of ‘Z1’ (Supplementary Table 4).
Figure 5. Fluorescent in situ hybridization was carried out using 45S rDNA (in green) and KBrB022P06 + KBrH003P24 BAC probes (A06) (in red). FISH analyses of somatic metaphase chromosomes were carried out in B. rapa ‘Z1’ (A–C), B. rapa ‘Chiifu’ (D–F), and hybrid ‘Z1’× ‘Chiifu’ (G–I). The 45S rDNA probe on B. rapa marked five different chromosome pairs (stars). A06 chromosomes carrying 45S rDNA were indicated by a blue star in ‘Z1’ and an orange star in ‘Chiifu.’ Chromosomes were counterstained with DAPI (gray). Bars represent 5 μm.
DNA content was obtained for ‘Z1,’ ‘Chiifu,’ and 10 accessions representing B. rapa core collection. After checking normality of data (Shapiro test, P = 0.487), the significative impact of accessions was tested (ANOVA; df = 11, P = 4.19e-13), followed by Tukey pairwise comparisons and group assignment based on P < 0.05. Of particular interest, the two focal varieties ‘Chiifu’ and ‘Z1’ have significantly different 1C DNA content with 0.557 (median = 0.565) and 0.590 (median = 0.588), respectively (Tukey HSD; P = 0.019). All accessions can be divided in seven subspecies and six groups structured within the core collection (Figure 6). The variation in genome size does not seem to be associated with any of those phylogenetic structures.
Figure 6. Box plot of the DNA nuclear content (1C) of 12 B. rapa accessions with 5 to 10 individuals per accession. Colors indicate the Tukey pairwise comparisons and group assignment (Tukey HSD; α = 0.05). Accessions are classified according to their higher-order phylogeny (group and subspecies according to Cheng et al., 2016b).
Finally, we explored the presence of these inversions in the same core collection of B. rapa accessions (Supplementary Figure 1). Although it appeared that some nucleotide divergence might have prevented amplification, some accessions seem to share preponderantly either one or the other genetic structure (inversion on A05 zone II: populations 1, 2, 4, 5, and 10 might seem alike ‘Z1,’ whereas populations 3, 7, 8, and 9 seem alike ‘Chiifu’; inversion on A10: populations 3, 4, 5, 6, 7, 9, and 10 seem more alike ‘Z1’).
The large phenotypic diversity within B. rapa species has always triggered intensive investigations from the community. Ancient whole genome duplication followed by subgenome parallel selection has been shown to play a role in morphotype diversification in B. rapa (Cheng et al., 2016b). In addition, contrasted levels of cytosine methylations correlating with various TE densities have been proposed to shape subgenome dominance and fractionation levels within the genome of B. rapa (Cheng et al., 2016a). However, the extent of TE diversity within the species as well as the structural genomic variants that might additionally explain within-species diversity has merely been touched upon.
A recent study on whole-genome resequencing of eight B. napus accessions revealed millions of genomic variants, resulting in 3.2–6.8% (for a total of 77.2–149.6 Mb) of the genome presenting such variations (Song et al., 2020). Similarly, chromosome-level assemblies of seven Arabidopsis thaliana genomes unravel overall 13–17 Mb of rearranged genomic variants and up to 6.5 Mb (or 5.5% of the reference genome) of genome-specific sequences based on all possible pairwise comparisons (Jiao and Schneeberger, 2020). In the present study, we identified 23 Mb of inversions (representing 4.3% of the genome) and 73 Mb of specific insertions (representing 13.8% of the genome) in ‘Z1’ compared to ‘Chiifu.’ These results show the large unexplored genomic diversity present in only two accessions of B. rapa. Although we cannot directly address their role in phenotypic diversification within the species, these insertions include 963 coding regions for which almost half of them cannot find an orthologous copy in the ‘Chiifu’ genome. These genes might be part of the dispensable gene pool as explained in a study gathering 10 accessions of B. oleracea, where 81.3% of genes represent the core, whereas the remaining genes (18.7%) represent dispensable genes (Golicz et al., 2016).
Besides the functional consequences of such variants, the whole genome structure could be particularly impacted. The variation in genome sizes between our focal accessions has been previously addressed with estimations varying between 529 and 443 Mb for ‘Z1’ and ‘Chiifu,’ respectively (Belser et al., 2018; Zhang et al., 2018). However, the disparate methods used, either by flow cytometry or k-mer analysis, can reveal wide differences (see Zhang et al., 2018). Here, using internal control and repeated evaluations of DNA content by flow cytometry, we estimated an increase of ca. 6% of genome size between ‘Z1’ and ‘Chiifu.’ In addition, we were able to validate the difference of the A06 chromosome lengths between the focal accessions using a ribosomal DNA probe in a BAC-FISH experiment showing firsthand the possible additional impact of these elements on chromosome and genome size.
Extending our analysis to the B. rapa core collection, we explored the variation in genome sizes among different accessions of B. rapa representatives of the phenotypic diversity in the species. Interestingly, up to 16% variation in genome size was observed among the 12 accessions without visible structuration according to their phylogenetic relationships. These differences are probably due to intraspecific variation in repeat content and insertion/deletion of sequences as their expansion correlates with the diversification of morphotypes (ca. 1 Mya, Zhao et al., 2013).
Very few studies tried so far to identify intraspecific genome size variation in Brassicaceae (with same ploidy level and same number of chromosomes). In Johnston et al. (2005), B. rapa species (including unknown accessions) exhibited wider standard error than any other Brassicaceae species. By comparison in A. thaliana, the analyses of DNA content in 10 ecotypes showed little variation with less than 1% among the different accessions (Johnston et al., 2005), which seemed to be corroborated with recent assemblies of various A. thaliana accessions with low genome size variance (Jiao and Schneeberger, 2020).
Usually, genome size variation is often associated with TE content and ribosomal repeats as these elements could be highly dynamic. The large specific insertions identified in ‘Z1’ allowed improving our understanding of centromeric and pericentromeric regions that are usually difficult to sequence and assemble in complex genomes such as plant genomes, because of their high density in repeats. Interestingly, apart from some dispensable genes, specific ‘Z1’ insertions exhibited mostly both rDNA copies and LTR elements.
In Brassica species, the number of 45S rDNA loci is variable. For example, B. oleracea, B. nigra, and B. rapa have 4, 6, and 10 rDNA loci, respectively (Hasterok et al., 2001). However, the number of 45S (and 5S) rDNA loci varies among varieties within each species. Hasterok et al. (2006) identified 10 45S rDNA loci in all B. rapa, except in B. rapa subsp. oleifera (DC.) ‘Schneeball’ (six loci). Here, we investigated the 45S rDNA in two other B. rapa varieties, ‘Z1’ and ‘Chiifu.’ The number of 45S rDNA loci identified in these two varieties (ten loci, Figure 5) is identical to the number of 45S rDNA loci identified in the majority of other B. rapa varieties (Hasterok et al., 2006). However, based on the signal intensity, as shown by Xiong and Pires (2011) for the chromosome A05 of B. rapa ‘Chiifu’ vs. A05 of ‘IMB218,’ the loci on the chromosome A06 in B. rapa ‘Z1’ had low signal intensity but showed a decondensation, while the loci on the chromosome A06 in ‘Chiifu’ are fully condensed. As the A03 locus has been shown to be the only one carrying transcriptionally active ribosomal genes in the nucleolar organizer region (Hasterok and Maluszynska, 2000), it could be of interest to test if the 45S rDNA genes localized on the A06 in ‘Z1’ are transcribed and active.
Although the number of loci between ‘Z1’ and ‘Chiifu’ is identical, the number of 45S rDNA copies is different between these two varieties. The number of copies estimated in ‘Z1’ (1,750 copies) is highly similar to the number of rDNA copies previously estimated in this variety (1,709 copies, Sochorová et al., 2017) and is more abundant than the number of copies identified in ‘Chiifu’ (1,416 copies). This difference could be the result of rearrangements with loss of rDNA copies in B. rapa ‘Chiifu,’ as demonstrated in polyploid species such as B. napus or Nicotiana tabacum (Lim et al., 2000; Książczyk et al., 2011; Sochorová et al., 2017). However, rDNA phylogeny of ‘Chiifu’ and ‘Z1’ (Figure 4A) has shown that among the three clades identified, two included only ‘Z1’ sequences (163 sequences). Thus, the most parsimonious scenario would indicate that these two clades most probably result from duplication events in ‘Z1’ after the divergence between the two focal accessions and not from loss of copies in ‘Chiifu.’
Regarding TE content, assembly of the ‘Z1’ genome has allowed a first comparative exploration of the repetitive compartment between ‘Z1’ and ‘Chiifu’ (Belser et al., 2018); however, differences were mainly attributed to methodological dissimilarities between genome assemblies. Here, using a consistent analysis pipeline for TE detection and annotation in these two accessions, we can reveal the impact of TEs on B. rapa intraspecific diversity, taking into account the structural variations.
Using genome size estimation for B. rapa (i.e., 529 Mb according to Johnston et al., 2005), TEs represent 31.73 and 22.16% of ‘Z1’ and ‘Chiifu’ genomes, respectively. However, considering the genome sizes obtained in this study (577 and 545 Mb on average for ‘Z1’ and ‘Chiifu,’ respectively), TEs may represent 29.09 and 21.50% of the genome in ‘Z1’ and ‘Chiifu,’ respectively. Overall, our methodology allowed us to retrieve similar numbers of TEs compared to what is expected with 29 and 24% of TEs in accessions ‘Z1’ and ‘Chiifu,’ respectively (Belser et al., 2018). Interestingly, a tangible difference in TE content is consistently observed between these two accessions, and we proposed that this pattern is not widely distributed along the genome but focused on specific chromosomes and on identified structural variants.
The detailed analysis of the structural variants in ‘Z1’ showed that while inversions do not significantly sustain a higher proportion of TEs compared to the rest of the genome, specific insertions in ‘Z1’ carry a large proportion of LTR gypsy and LINEs; this is especially clear on chromosome A05 and A06 in ‘Z1,’ which harbor the largest genomic variants. TE proportions could be underestimated in these regions; as a large part of the inserted sequences were unsequenced data, we are nonetheless confident on the length of these sequences, thanks to reliable optical mapping scaffolds.
TE intraspecific diversity has been previously shown to be driven by an expansion of recent LTR retrotransposons in B. rapa with rapid nucleotide substitution (Zhao et al., 2013). However, we do not observe such patterns using a phylogenetic approach. Instead, all LTR gypsy clades seem to be represented in the inserted regions. This tendency seems to be particularly exacerbated in B. rapa and by extension in the A subgenome of B. napus compared to B. oleracea and the C subgenome of B. napus as demonstrated in Song et al. (2020). In addition, the inserted LTR retrotransposons do occur in clusters on chromosomes A05, A06, and A09, and their spreading predates the divergence between B. rapa and B. oleracea (Song et al., 2020).
Our results unveil the necessity for long-read sequencing strategies and developing various whole-genome references for agronomically important species (Danilevicz et al., 2020). This recent endeavor in constructing pangenomes is revealing the importance of structural variants and the diversity in repeat content within species. This is especially relevant in species showing broad phenotypic diversity such as Brassica sp. Besides the role of these variants in Brassica diversification, we can only wonder at their role in other processes such as recombination. In that case, structural rearrangements (especially inversions) act as modifiers of recombination that create different patterns of recombination rate across the genome (Ortiz-Barrientos et al., 2016), leading to segregation distortion and partial lethality (Lynch and Force, 2000; Fransz et al., 2016). Similarly, TEs have been shown to cause post-zygotic barriers and reproductive isolation in a diversity of taxa (reviewed in Serrato-Capuchina and Matute, 2018). First hints suggest that both structural variants and repeat content seem highly dynamic among the different accessions of B. rapa composing the core collection. Thus, exploring this diversity will deeply improve our understanding of the species adaptive processes and could be used in the creation of new interspecific and intraspecific Brassica hybrid varieties.
Publicly available datasets were analyzed in this study. This data can be found here: http://www.genoscope.cns.fr/plants and http://brassicadb.org.
JF and JB conceived and designed the experiments with inputs from LM. TC, SL, and LM implemented the REPET pipeline and performed the work on TE annotation. JB, LM, CF, and JM performed the bioinformatics analyses. J-MA and CB analyzed the generated optical maps and verified regions of interests. AB, VH, and OC performed the cytological analyses. GT performed the flow-cytometry experiments. GD and ML performed the DNA extractions and PCR to verify inversions. A-MC and MR-G helped supervise the project and participated in critical thinking of the results with LM, FB, CF, OC, JB, and JF. JF and JB wrote the manuscript. All authors approved the final manuscript.
This work was made possible by financial support of the European Union and a Marie Sklodowska-Curie grant (No. 791908) awarded to JF as well as a BOOST’EUROPE financed by the Conseil régional de Bretagne. FB was supported by a fellowship from BAP INRAE and Conseil régional de Bretagne. This work was additionally supported by the France Génomique National infrastructure, funded as part of the «Investissements d’Avenir » program managed by the Agence Nationale pour la Recherche (contract ANR-10-INBS-09).
The authors declare that the research was conducted in the absence of any commercial or financial relationships that could be construed as a potential conflict of interest.
We acknowledge the BrACySol BRC (INRA Ploudaniel, France) that provided us with the seeds of B. rapa accessions and Marie-Madeleine Gilet for growing and taking care of the accessions. We would also like to thank all the technical staff of the greenhouse for management of the plant material (especially L. Charlon, P. Rolland, J.-P. Constantin, J.-M. Lucas, and F. Letertre).
The Supplementary Material for this article can be found online at: https://www.frontiersin.org/articles/10.3389/fpls.2020.577536/full#supplementary-material
Supplementary Figure 1 | PCR amplifications and agarose gels validating inversions in three genomic regions on (A) chromosome A05 first inversion, (B) chromosome A05 second inversion and (C) chromosome A10. Both sides of each inversion have been investigated (L for left side and R for right side) in the focal accessions and B. rapa core collections (named here as PBD1 to PBD10), ordered on the gel as follows: ‘Chiifu,’ ‘Z1,’ PBD001 to PBD010.
Supplementary Table 1 | Accession identification and origin of the B. rapa accessions.
Supplementary Table 2 | Primer identifiers, sequences and target amplification.
Supplementary Table 3 | Position and length of the B. rapa ‘Chiifu’ vs. ‘Z1’ inversions. For each region, the number and cumulative length of genes, Transposable Elements and ribosomal DNA were identified.
Supplementary Table 4 | Transposable Element proportions in the 10 chromosomes of B. rapa ‘Chiifu’ and B. rapa ‘Z1.’ Class I and Class II correspond to TEs for which order’s annotation remains unknown.
Supplementary Table 5 | A06 individual chromosome lengths (in μm) at mitotic metaphase in F1 hybrid (‘Z1’ × ‘Chiifu’).
Aissiou, F., Laperche, A., Falentin, C., Lodé, M., Deniot, G., Boutet, G., et al. (2018). A novel Brassica rapa L. genetic diversity found in Algeria. Euphytica 214:241. doi: 10.1007/s10681-018-2318-9
Altschul, S. F., Madden, T. L., Schaffer, A. A., Zhang, J. H., Zhang, Z., Miller, W., et al. (1997). Gapped BLAST and PSI-BLAST: a new generation of protein database search programs. Nucleic Acids Res. 25, 3389–3402. doi: 10.1093/nar/25.17.3389
Belser, C., Istace, B., Denis, E., Dubarry, M., Baurens, F.-C., Falentin, C., et al. (2018). Chromosome-scale assemblies of plant genomes using nanopore long reads and optical maps. Nat. Plants 4, 879–887. doi: 10.1038/s41477-018-0289-4
Cheng, F., Sun, C., Wu, J., Schnable, J., Woodhouse, M. R., Liang, J., et al. (2016a). Epigenetic regulation of subgenome dominance following whole genome triplication in Brassica rapa. New Phytol. 211, 288–299. doi: 10.1111/nph.13884
Cheng, F., Sun, R., Hou, X., Zheng, H., Zhang, F., Zhang, Y., et al. (2016b). Subgenome parallel selection is associated with morphotype diversification and convergent crop domestication in Brassica rapa and Brassica oleracea. Nat. Genet. 48:1218. doi: 10.1038/ng.3634
Cheng, F., Wu, J., Cai, C., Fu, L., Liang, J., Borm, T., et al. (2016c). Genome resequencing and comparative variome analysis in a Brassica rapa and Brassica oleracea collection. Sci. Data 3:160119. doi: 10.1038/sdata.2016.119
Cheng, F., Wu, J., and Wang, X. (2014). Genome triplication drove the diversification of Brassica plants. Hortic. Res. 1:14024. doi: 10.1038/hortres.2014.24
Chia, J.-M., Song, C., Bradbury, P. J., Costich, D., de Leon, N., Doebley, J., et al. (2012). Maize HapMap2 identifies extant variation from a genome in flux. Nat. Genet. 44, 803–807. doi: 10.1038/ng.2313
Danilevicz, M. F., Tay Fernandez, C. G., Marsh, J. I., Bayer, P. E., and Edwards, D. (2020). Plant pangenomics: approaches, applications and advancements. Curr. Opin. Plant Biol. 54, 18–25. doi: 10.1016/j.pbi.2019.12.005
Dolatabadian, A., Bayer, P. E., Tirnaz, S., Hurgobin, B., Edwards, D., and Batley, J. (2020). Characterization of disease resistance genes in the Brassica napus pangenome reveals significant structural variation. Plant Biotechnol. J. 18, 969–982. doi: 10.1111/pbi.13262
Ferreira de Carvalho, J., de Jager, V., van Gurp, T. P., Wagemaker, N. C. A. M., and Verhoeven, K. J. F. (2016). Recent and dynamic transposable elements contribute to genomic divergence under asexuality. BMC Genomics 17:884. doi: 10.1186/s12864-016-3234-9
Flutre, T., Duprat, E., Feuillet, C., and Quesneville, H. (2011). Considering transposable element diversification in de novo annotation approaches. PLoS One 6:e16526. doi: 10.1371/journal.pone.0016526
Fransz, P., Linc, G., Lee, C.-R., Aflitos, S. A., Lasky, J. R., Toomajian, C., et al. (2016). Molecular, genetic and evolutionary analysis of a paracentric inversion in Arabidopsis thaliana. Plant J. 88, 159–178. doi: 10.1111/tpj.13262
Gage, J. L., Vaillancourt, B., Hamilton, J. P., Manrique-Carpintero, N. C., Gustafson, T. J., Barry, K., et al. (2019). Multiple maize reference genomes impact the identification of variants by genome-wide association study in a diverse inbred panel. Plant Genome 12, 1–12. doi: 10.3835/plantgenome2018.09.0069
Gazave, E., Tassone, E. E., Ilut, D. C., Wingerson, M., Datema, E., Witsenboer, H. M. A., et al. (2016). Population genomic analysis reveals differential evolutionary histories and patterns of diversity across subgenomes and subpopulations of Brassica napus L. Front. Plant Sci. 7:525. doi: 10.3389/fpls.2016.00525
Gerlach, W. L., and Bedbrook, J. R. (1979). Cloning and characterization of ribosomal RNA genes from wheat and barley. Nucleic Acids Res. 7, 1869–1885. doi: 10.1093/nar/7.7.1869
Golicz, A. A., Bayer, P. E., Barker, G. C., Edger, P. P., Kim, H., Martinez, P. A., et al. (2016). The pangenome of an agronomically important crop plant Brassica oleracea. Nat. Commun. 7:13390. doi: 10.1038/ncomms13390
Guo, Y., Chen, S., Li, Z., and Cowling, W. A. (2014). Center of origin and centers of diversity in an ancient crop, Brassica rapa (Turnip Rape). J. Hered. 105, 555–565. doi: 10.1093/jhered/esu021
Hasterok, R., Jenkins, G., Langdon, T., Jones, R. N., and Maluszynska, J. (2001). Ribosomal DNA is an effective marker of Brassica chromosomes. Theor. Appl. Genet. 103, 486–490. doi: 10.1007/s001220100653
Hasterok, R., and Maluszynska, J. (2000). Nucleolar dominance does not occur in root tip cells of allotetraploid Brassica species. Genome 43, 574–579. doi: 10.1139/g00-005
Hasterok, R., Wolny, E., Hosiawa, M., Kowalczyk, M., Kulak-Ksiazczyk, S., Ksiazczyk, T., et al. (2006). Comparative analysis of rDNA distribution in chromosomes of various species of brassicaceae. Ann. Bot. 97, 205–216. doi: 10.1093/aob/mcj031
Hoede, C., Arnoux, S., Moisset, M., Chaumier, T., Inizan, O., Jamilloux, V., et al. (2014). PASTEC: an automatic transposable element classification tool. PLoS One 9:e91929. doi: 10.1371/journal.pone.0091929
Jiao, W.-B., and Schneeberger, K. (2020). Chromosome-level assemblies of multiple Arabidopsis genomes reveal hotspots of rearrangements with altered evolutionary dynamics. Nat. Commun. 11:989. doi: 10.1038/s41467-020-14779-y
Johnston, J. S., Pepper, A. E., Hall, A. E., Chen, Z. J., Hodnett, G., Drabek, J., et al. (2005). Evolution of genome size in brassicaceae. Ann. Bot. 95, 229–235. doi: 10.1093/aob/mci016
Kalendar, R., Tanskanen, J., Immonen, S., Nevo, E., and Schulman, A. H. (2000). Genome evolution of wild barley (Hordeum spontaneum) by BARE-1 retrotransposon dynamics in response to sharp microclimatic divergence. Proc. Natl. Acad. Sci. U.S.A. 97, 6603–6607. doi: 10.1073/pnas.110587497
Katoh, K., and Standley, D. M. (2013). MAFFT multiple sequence alignment software version 7: improvements in performance and usability. Mol. Biol. Evol. 30, 772–780. doi: 10.1093/molbev/mst010
Kearse, M., Moir, R., Wilson, A., Stones-Havas, S., Cheung, M., Sturrock, S., et al. (2012). Geneious basic: an integrated and extendable desktop software platform for the organization and analysis of sequence data. Bioinformatics 28, 1647–1649. doi: 10.1093/bioinformatics/bts199
Kidwell, M. G., and Lisch, D. R. (2000). Transposable elements and host genome evolution. Trends Ecol. Evol. 15, 95–99. doi: 10.1016/s0169-5347(99)01817-0
Książczyk, T., Kovarik, A., Eber, F., Huteau, V., Khaitova, L., Tesarikova, Z., et al. (2011). Immediate unidirectional epigenetic reprogramming of NORs occurs independently of rDNA rearrangements in synthetic and natural forms of a polyploid species Brassica napus. Chromosoma 120, 557–571. doi: 10.1007/s00412-011-0331-z
Kurtz, S., Phillippy, A., Delcher, A. L., Smoot, M., Shumway, M., Antonescu, C., et al. (2004). Versatile and open software for comparing large genomes. Genome Biol. 5:R12. doi: 10.1186/gb-2004-5-2-r12
Lam, H.-M., Xu, X., Liu, X., Chen, W., Yang, G., Wong, F.-L., et al. (2010). Resequencing of 31 wild and cultivated soybean genomes identifies patterns of genetic diversity and selection. Nat. Genet. 42, 1053–1059. doi: 10.1038/ng.715
Langmead, B., and Salzberg, S. L. (2012). Fast gapped-read alignment with Bowtie 2. Nat. Methods 9, 357–359. doi: 10.1038/nmeth.1923
Li, P., Zhang, S., Li, F., Zhang, S., Zhang, H., Wang, X., et al. (2017). A phylogenetic analysis of chloroplast genomes elucidates the relationships of the six economically important Brassica species comprising the triangle of U. Front. Plant Sci. 8:111. doi: 10.3389/fpls.2017.00111
Li, Y., Zhou, G., Ma, J., Jiang, W., Jin, L., Zhang, Z., et al. (2014). De novo assembly of soybean wild relatives for pan-genome analysis of diversity and agronomic traits. Nat. Biotechnol. 32, 1045–1052. doi: 10.1038/nbt.2979
Lim, K. Y., Matyášek, R., Lichtenstein, C. P., and Leitch, A. R. (2000). Molecular cytogenetic analyses and phylogenetic studies in the Nicotiana section Tomentosae. Chromosoma 109, 245–258. doi: 10.1007/s004120000074
Lu, F., Romay, M. C., Glaubitz, J. C., Bradbury, P. J., Elshire, R. J., Wang, T., et al. (2015). High-resolution genetic mapping of maize pan-genome sequence anchors. Nat. Commun. 6:6914. doi: 10.1038/ncomms7914
Lynch, M., and Force, A. G. (2000). The origin of interspecific genomic incompatibility via gene duplication. Am. Nat. 156, 590–605. doi: 10.1086/316992
Makarevitch, I., Waters, A. J., West, P. T., Stitzer, M., Hirsch, C. N., Ross-Ibarra, J., et al. (2015). Transposable elements contribute to activation of maize genes in response to abiotic stress. PLoS Genet. 11:e1004915. doi: 10.1371/journal.pgen.1004915
Montenegro, J. D., Golicz, A. A., Bayer, P. E., Hurgobin, B., Lee, H., Chan, C.-K. K., et al. (2017). The pangenome of hexaploid bread wheat. Plant J. 90, 1007–1013. doi: 10.1111/tpj.13515
Mun, J.-H., Kwon, S.-J., Seol, Y.-J., Kim, J. A., Jin, M., Kim, J. S., et al. (2010). Sequence and structure of Brassica rapachromosome A3. Genome Biol. 11:R94. doi: 10.1186/gb-2010-11-9-r94
Murat, F., Louis, A., Maumus, F., Armero, A., Cooke, R., Quesneville, H., et al. (2015). Understanding Brassicaceae evolution through ancestral genome reconstruction. Genome Biol. 16:262. doi: 10.1186/s13059-015-0814-y
U, N. (1935). Genome analysis in Brassica with special reference to the experimental formation of B. napus and peculiar mode of fertilization. Jpn. J. Bot. 7, 389–452.
Ortiz-Barrientos, D., Engelstädter, J., and Rieseberg, L. H. (2016). Recombination rate evolution and the origin of species. Trends Ecol. Evol. 31, 226–236. doi: 10.1016/j.tree.2015.12.016
Parisod, C., Salmon, A., Zerjal, T., Tenaillon, M., Grandbastien, M., and Ainouche, M. (2009). Rapid structural and epigenetic reorganization near transposable elements in hybrid and allopolyploid genomes in Spartina. New Phytol. 184, 1003–1015. doi: 10.1111/j.1469-8137.2009.03029.x
Paritosh, K., Yadava, S. K., Singh, P., Bhayana, L., Mukhopadhyay, A., Gupta, V., et al. (2019). A chromosome-scale assembly of allotetraploid Brassica juncea (AABB) elucidates comparative architecture of the A and B genomes. bioRxiv[Preprint] doi: 10.1101/681080
Qi, X., An, H., Ragsdale, A. P., Hall, T. E., Gutenkunst, R. N., Pires, J. C., et al. (2017). Genomic inferences of domestication events are corroborated by written records in Brassica rapa. Mol. Ecol. 26, 3373–3388. doi: 10.1111/mec.14131
Quadrana, L., Bortolini Silveira, A., Mayhew, G. F., LeBlanc, C., Martienssen, R. A., Jeddeloh, J. A., et al. (2016). The Arabidopsis thaliana mobilome and its impact at the species level. eLife 5:e15716. doi: 10.7554/eLife.15716
Quesneville, H., Bergman, C. M., Andrieu, O., Autard, D., Nouaud, D., Ashburner, M., et al. (2005). Combined evidence annotation of transposable elements in genome sequences. PLoS Comput. Biol. 1:e22. doi: 10.1371/journal.pcbi.0010022
R Core Team (2018). R: A Language and Environment for Statistical Computing. Vienna: R Foundation for Statistical Computing.
Schatz, M. C., Maron, L. G., Stein, J. C., Wences, A. H., Gurtowski, J., Biggers, E., et al. (2014). Whole genome de novo assemblies of three divergent strains of rice, Oryza sativa, document novel gene space of aus and indica. Genome Biol. 15:506. doi: 10.1186/s13059-014-0506-z
Serrato-Capuchina, A., and Matute, D. R. (2018). The role of transposable elements in speciation. Genes (Basel) 9:6914. doi: 10.3390/genes9050254
Sochorová, J., Coriton, O., Kuderová, A., Lunerová, J., Chèvre, A.-M., and Kovařík, A. (2017). Gene conversion events and variable degree of homogenization of rDNA loci in cultivars of Brassica napus. Ann. Bot. 119, 13–26. doi: 10.1093/aob/mcw187
Song, J.-M., Guan, Z., Hu, J., Guo, C., Yang, Z., Wang, S., et al. (2020). Eight high-quality genomes reveal pan-genome architecture and ecotype differentiation of Brassica napus. Nat. Plants 6, 34–45. doi: 10.1038/s41477-019-0577-7
Song, K., Osborn, T. C., and Williams, P. H. (1990). Brassica taxonomy based on nuclear restriction fragment length polymorphisms. Theor. Appl. Genet. 79, 497–506. doi: 10.1007/bf00226159
Tian, T., Liu, Y., Yan, H., You, Q., Yi, X., Du, Z., et al. (2017). agriGO v2.0: a GO analysis toolkit for the agricultural community, 2017 update. Nucleic Acids Res. 45, W122–W129. doi: 10.1093/nar/gkx382
Ungerer, M. C., Strakosh, S. C., and Zhen, Y. (2006). Genome expansion in three hybrid sunflower species is associated with retrotransposon proliferation. Curr. Biol. 16, R872–R873. doi: 10.1016/j.cub.2006.09.020
Vicient, C. M., and Casacuberta, J. M. (2017). Impact of transposable elements on polyploid plant genomes. Ann. Bot. 120, 195–207. doi: 10.1093/aob/mcx078
Walker, E. L., Robbins, T. P., Bureau, T. E., Kermicle, J., and Dellaporta, S. L. (1995). Transposon-mediated chromosomal rearrangements and gene duplications in the formation of the maize R-r complex. EMBO J. 14, 2350–2363. doi: 10.1002/j.1460-2075.1995.tb07230.x
Wang, W., Mauleon, R., Hu, Z., Chebotarov, D., Tai, S., Wu, Z., et al. (2018). Genomic variation in 3,010 diverse accessions of Asian cultivated rice. Nature 557, 43–49. doi: 10.1038/s41586-018-0063-9
Wang, X., Wang, H., Wang, J., Sun, R., Wu, J., Liu, S., et al. (2011). The genome of the mesopolyploid crop species Brassica rapa. Nat. Genet. 43, 1035–1039. doi: 10.1038/ng.919
Wicker, T., Sabot, F., Hua-Van, A., Bennetzen, J. L., Capy, P., Chalhoub, B., et al. (2007). A unified classification system for eukaryotic transposable elements. Nat. Rev. Genet. 8, 973–982. doi: 10.1038/nrg2165
Wright, S. I., and Schoen, D. J. (1999). Transposon dynamics and the breeding system. Genetica 107, 139–148. doi: 10.1007/978-94-011-4156-7_16
Xiong, Z., and Pires, J. C. (2011). Karyotype and identification of all homoeologous chromosomes of allopolyploid Brassica napus and its diploid progenitors. Genetics 187, 37–49. doi: 10.1534/genetics.110.122473
Zhang, L., Cai, X., Wu, J., Liu, M., Grob, S., Cheng, F., et al. (2018). Improved Brassica rapa reference genome by single-molecule sequencing and chromosome conformation capture technologies. Hortic. Res. 5, 1–11. doi: 10.1038/s41438-018-0071-9
Zhao, J., Artemyeva, A., Del Carpio, D. P., Basnet, R. K., Zhang, N., Gao, J., et al. (2010). Design of a Brassica rapa core collection for association mapping studies. Genome 53, 884–898. doi: 10.1139/G10-082
Zhao, M., Du, J., Lin, F., Tong, C., Yu, J., Huang, S., et al. (2013). Shifts in the evolutionary rate and intensity of purifying selection between two Brassica genomes revealed by analyses of orthologous transposons and relics of a whole genome triplication. Plant J. 76, 211–222. doi: 10.1111/tpj.12291
Keywords: Brassica, genome evolution, transposable elements, LTR Gypsy, intraspecific diversity, ribosomal DNA
Citation: Boutte J, Maillet L, Chaussepied T, Letort S, Aury J-M, Belser C, Boideau F, Brunet A, Coriton O, Deniot G, Falentin C, Huteau V, Lodé-Taburel M, Morice J, Trotoux G, Chèvre A-M, Rousseau-Gueutin M and Ferreira de Carvalho J (2020) Genome Size Variation and Comparative Genomics Reveal Intraspecific Diversity in Brassica rapa. Front. Plant Sci. 11:577536. doi: 10.3389/fpls.2020.577536
Received: 29 June 2020; Accepted: 24 September 2020;
Published: 12 November 2020.
Edited by:
Ruslan Kalendar, University of Helsinki, FinlandReviewed by:
Ryo Fujimoto, Kobe University, JapanCopyright © 2020 Boutte, Maillet, Chaussepied, Letort, Aury, Belser, Boideau, Brunet, Coriton, Deniot, Falentin, Huteau, Lodé-Taburel, Morice, Trotoux, Chèvre, Rousseau-Gueutin and Ferreira de Carvalho. This is an open-access article distributed under the terms of the Creative Commons Attribution License (CC BY). The use, distribution or reproduction in other forums is permitted, provided the original author(s) and the copyright owner(s) are credited and that the original publication in this journal is cited, in accordance with accepted academic practice. No use, distribution or reproduction is permitted which does not comply with these terms.
*Correspondence: Julien Boutte, Ym91dHRlLmp1bGllbkBnbWFpbC5jb20=; Julie Ferreira de Carvalho, anVsaWUuZmVycmVpcmEtZGUtY2FydmFsaG9AaW5yYWUuZnI=
Disclaimer: All claims expressed in this article are solely those of the authors and do not necessarily represent those of their affiliated organizations, or those of the publisher, the editors and the reviewers. Any product that may be evaluated in this article or claim that may be made by its manufacturer is not guaranteed or endorsed by the publisher.
Research integrity at Frontiers
Learn more about the work of our research integrity team to safeguard the quality of each article we publish.