- Department of Biological Sciences, National University of Singapore, Singapore, Singapore
Hybridization is a core element in modern rice breeding as beneficial combinations of two parental genomes often result in the expression of heterosis. On the contrary, genetic incompatibility between parents can manifest as hybrid necrosis, which leads to tissue necrosis accompanied by compromised growth and/or reduced reproductive success. Genetic and molecular studies of hybrid necrosis in numerous plant species revealed that such self-destructing symptoms in most cases are attributed to autoimmunity: plant immune responses are inadvertently activated in the absence of pathogenic invasion. Autoimmunity in hybrids predominantly occurs due to a conflict involving a member of the major plant immune receptor family, the nucleotide-binding domain and leucine-rich repeat containing protein (NLR; formerly known as NBS-LRR). NLR genes are associated with disease resistance traits, and recent population datasets reveal tremendous diversity in this class of immune receptors. Cases of hybrid necrosis involving highly polymorphic NLRs as major causes suggest that diversified R gene repertoires found in different lineages would require a compatible immune match for hybridization, which is a prerequisite to ensure increased fitness in the resulting hybrids. In this review, we overview recent genetic and molecular findings on hybrid necrosis in multiple plant species to provide an insight on how the trade-off between growth and immunity is equilibrated to affect hybrid performances. We also revisit the cases of hybrid weakness in which immune system components are found or implicated to play a causative role. Based on our understanding on the trade-off, we propose that the immune system incompatibility in plants might play an opposite force to restrict the expression of heterosis in hybrids. The antagonism is illustrated under the plant fitness equilibrium, in which the two extremes lead to either hybrid necrosis or heterosis. Practical proposition from the equilibrium model is that breeding efforts for combining enhanced disease resistance and high yield shall be achieved by balancing the two forces. Reverse breeding toward utilizing genomic data centered on immune components is proposed as a strategy to generate elite hybrids with balanced immunity and growth.
Introduction
Hybridization between individuals of the same or different plant species leads to the formation of hybrids that contain a copy of each parental genome. This heterozygosity results in novel genetic combinations that were never found in the respective parental lines, and thus distinct phenotypes can be sometimes expressed in the hybrid (Mallet, 2007). Genetic interactions in the hybrid can result in beneficial phenotypes, of which the phenomenon is termed as heterosis or hybrid vigor (Shull, 1948; Schnable and Springer, 2013). On the contrary, such interactions can lead to detrimental phenotypes, as found in hybrid weakness or hybrid necrosis cases. Hybrid necrosis is a common phenomenon observed in plant hybrids featuring immune-related deleterious phenotypes (Bomblies and Weigel, 2007; Bomblies, 2009). The term reflects phenotypically recognizable damages in plant tissues with visible necrosis resulting from uncontrolled cell death and/or autoimmune-like symptoms including dwarfism, stunted growth, leaf crinkling, reduced fertility, and in severe instances death before transitioning to the reproductive phase (Bomblies and Weigel, 2007; Chae et al., 2014). The overall compromise in plant performances observed in hybrid necrosis cases is believed to reflect trade-offs between immunity and growth (Todesco et al., 2014; Chae et al., 2016; Świadek et al., 2017). Due to its drastic effect on survival and prevalence in F1 generations, hybrid necrosis is postulated to provide plant populations with a conducive mechanism to create post-zygotic barriers toward eventual speciation (Bomblies and Weigel, 2007).
Hybrid Necrosis Under the Bateson-Dobzhansky-Muller Model and Negative Epistasis
Hybrid necrosis arises from novel genetic interactions in heterozygous backgrounds. This phenomenon often manifests in the F1 generation, but cases in the F2 have also been described. Numerous examples of hybrid necrosis in plants demonstrate that the dynamics of genetic incompatibilities found in F1 and F2 hybrids conform to the simple Bateson-Dobzhansky-Muller (BDM) model for two-locus interaction that had been formulated to explain the speciation mechanism (Figure 1A; Orr, 1996). The BDM model posits that independently evolved genetic variants are harmless in their respective parental lineages but the combination of a pair becomes incompatible in the hybrid (Figure 1A). Since inviable, inferior or infertile offspring are the outcome of such incompatibilities, such genetic mechanisms are considered to establish major postzygotic reproductive barriers between different lineages, hence promoting speciation (Orr, 1996).
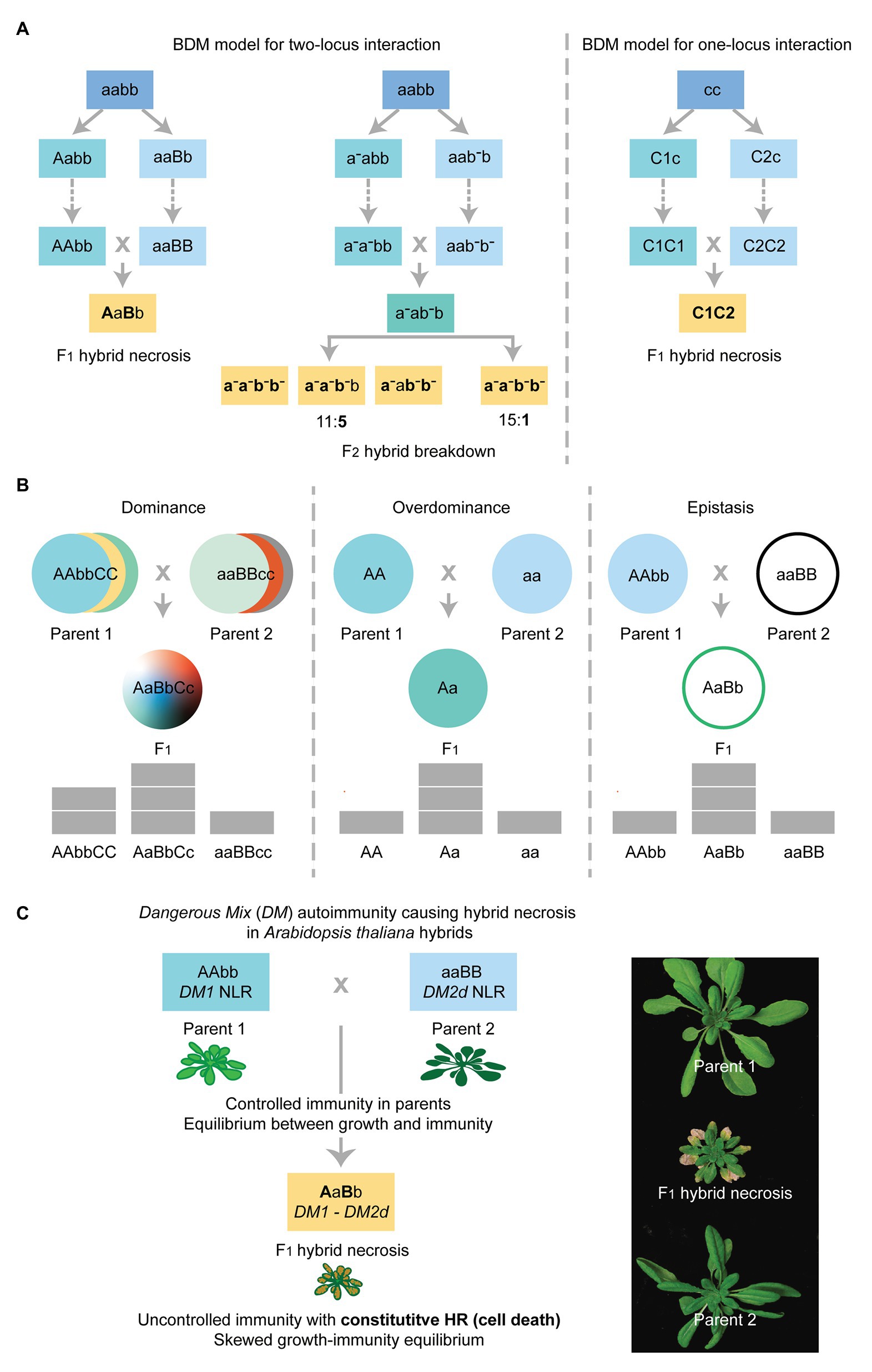
Figure 1. The genetic mechanisms of hybrid necrosis caused by negative epistasis explained by the Bateson-Dobzhansky-Muller (BDM) model. (A) The BDM model for two-locus or one-locus interaction triggering F1 hybrid necrosis and F2 hybrid breakdown in hybrid necrosis cases (yellow text-boxes). Ancestral status of alleles at indicated loci is uncapitalized and derived allelic status is indicated as capitalized for hybrid necrosis cases. Boldface letters indicate alleles that are under deleterious epistasis for hybrid necrosis and hybrid breakdown cases. The dotted arrows in each scheme indicate that numerous generations are required before the different allelic combinations represented in the colored boxes are fixed in independent lineages. In the cases of hybrid necrosis locus/loci, derived alleles behave as dominant or semi-dominant (Bomblies et al., 2007; Alcázar et al., 2009; Chae et al., 2014). In F2 hybrid breakdown, ancestral status and derived allelic status differ from each other by the presence of the upper dash for derived recessive alleles. Note that classical F2 hybrid breakdown cases manifest when the homozygous recessive state at two loci is met with the derived alleles (a-a-;b-b-) or the homozygous recessive state of one locus is combined with the heterozygous status of the other locus (a-a-;b-b or aa-;b-b-) in 15:1 or 11:5 segregation (Oka, 1957; Fukuoka et al., 1998; Okuno, 1999; Matsubara et al., 2007, 2014; Ichitani et al., 2012). (B) Genetic mechanisms of dominance, overdominance, and epistasis leading to phenotypes that respond to both qualitative (color) or quantitative (pyramid) traits. The first two mechanisms attribute the observed phenotype in the hybrid to additive effects, arising from allelic interactions between dominant over recessive alleles at various loci (dominance) or at a single locus (overdominance). Epistasis attributes any phenotype that deviates from the expected additive effect of two alleles to non-allelic interactions (i.e., two different loci). Note that the BDM model for two-locus interaction shown in (A) corresponds to epistasis, while overdominance explains the BDM model for one-locus interaction shown in (A). (C) Left: Autoimmunity triggered by the DM1/DM2d hybrid necrosis pair. The two loci, DM1 and DM2d, are innocuous in their respective parental lineages. The combination of DM1 and DM2d in the Arabidopsis thaliana hybrid triggers a constitutive activation of defense mechanisms in the absence of pathogen attack, which results in the hypersensitive response (HR) and hybrid necrosis (Bomblies et al., 2007; Tran et al., 2017). Right: F1 hybrid displaying cell death in somatic tissues and the comparison to the respective parents.
A wider spectrum of phenotypes are found in hybrids as compared to the parents, including heterosis and hybrid necrosis, and the underlying causes can be generally explained by genetic interactions following the three schemes, namely (i) dominance, (ii) overdominance, and (iii) epistasis (Figure 1B). These three mechanisms can operate simultaneously, but independently, to allow the expression of different traits (Li et al., 2008; Huang et al., 2016). The dominance hypothesis is often favored to explain heterotic phenotypes, which attributes the expression of heterosis to the heterozygous allelic status at multiple loci in hybrids. The premise is that in parental lines phenotypic expression for a given trait is constrained by the sum of deleterious, recessive alleles. In heterotic hybrids, the introduction of beneficial or dominant alleles from both parents at these multiple loci breaks the recessiveness that had caused inferiority in the respective parental backgrounds, thus improving hybrid performance (Figure 1B, left; Hochholdinger and Hoecker, 2007). The overdominance hypothesis, on the other hand, attributes superior- or under-performance in the hybrid to the heterozygosity at a single locus as compared to the homozygous status in parents (Figure 1B, middle; Hochholdinger and Hoecker, 2007). Lastly, epistasis refers to non-additive genetic interactions deviating from the summation of allelic effects at the involved loci (Figure 1B, right; Fisher, 1919). It is necessary to point out, however, that other mechanisms such as epigenetics can control the expression of traits in hybrids (Fujimoto et al., 2018). For instance, experiments in Arabidopsis thaliana using epigenetic recombinant inbred lines (Epi-RILs) have shown that epialleles are inheritable and can explain phenotypic variation in certain developmental traits (Cortijo et al., 2014; Zhang et al., 2018). The BDM model for hybrid necrosis is certainly a well spelled-out case of negative epistasis involving two derived alleles from the involved genetic loci. In most hybrid necrosis cases, the derived alleles act as dominant or semi-dominant, in a dosage-dependent manner. There are hybrid necrosis cases only visible in the F2 generation, of which genetic scheme can be similar to the classical F2 hybrid breakdown, albeit with strong dose-dependent effects (Figure 1A). Genetic studies on hybrid necrosis cases have demonstrated that a pairwise epistatic relationship between alleles at two distinct loci inherited from each of the parents does condition autoimmunity in both F1 and F2 hybrid generations (Figure 1A, left; Bomblies et al., 2007; Alcázar et al., 2009; Chae et al., 2014). In addition, cases of hybrid necrosis involving different alleles at a single locus have also been identified. Such negative epistasis can be easily explained by a simple modification of the BDM model with a replacement of one unlinked locus with the different alleles of the same locus (Figure 1A, right; Alcázar et al., 2014; Chae et al., 2014). To conclude, the BDM model explains the majority of F1 or F2 hybrid necrosis cases as a result of negative epistasis. Due to the deleterious effect on hybrid performance, hybrid necrosis can serve as a conducive mechanism to create a reproductive barrier between two independently evolving parental lineages (Figure 1A).
Autoimmunity as an Underlying Mechanism for Hybrid Necrosis
Numerous hybrid necrosis cases have been identified in crops of economic relevance such as wheat (Hermsen, 1963), interspecific hybrids of Triticum-Aegilops (Mizuno et al., 2010), rye (Ren and Lelley, 1988), lettuce (Jeuken et al., 2009), rice (Yamamoto et al., 2010), cotton (Phillips, 1977; Deng et al., 2019), and in natural germplasms of A. thaliana and Capsella species (Bomblies et al., 2007; Chae et al., 2014; Sicard et al., 2015). Because hybrid necrosis has been observed in a broad range of species (see review Bomblies et al., 2007), it is of major interest to define the causal genetic components and investigate if there is a common underlying mechanism. Accumulating fine-mapping data obtained from various hybrid necrosis cases in different species point out that this phenomenon arises from the uncontrolled activation of immune responses in the absence of pathogens. Such links to autoimmunity had already been suggested by Kostoff in 1930, who indicated that hybrid necrosis phenotypes resemble those triggered by pathogens and therefore suggested autoimmunity as a major cause of hybrid necrosis (Kostoff, 1930). However, it has only been over a decade since the causality was identified to the R protein families by molecular cloning (Krüger et al., 2002; Rooney et al., 2005; Bomblies et al., 2007; Alcázar et al., 2009; Chae et al., 2014). Nucleotide-binding and leucine-rich repeat (NLR) immune receptors in the majority and in some cases receptor-like proteins (RLPs) or receptor-like kinases (RLKs) families contribute to trigger autoimmunity in hybrids. NLRs constitute the major class of resistance genes (hereafter R genes) characterized to date, and plant genomes often carry hundreds of R gene homologs, some of which are highly variable even within a species (Sarris et al., 2016; Kourelis and van der Hoorn, 2018; Van de Weyer et al., 2019). It is thought that such variability reflects the need for the gene-for-gene hypothesis, in which a specific R protein recognizes a matching avirulent effector that can be highly variable in pathogen populations (Flor, 1971; Chen and Ronald, 2011). Because pathogens evolve rapidly, plant NLRs in a given population are under the pressure of diversifying to provide sufficient recognition-specificity for effectors, of which coevolution is best explained under the concept of an arms race between host and parasites (Holub, 2001; Borrelli et al., 2018). Such diversity, however, can result in a collateral conflict involving NLRs; when divergently evolved NLRs are found in combination with other immune components, one can miss-recognize the other and trigger autoimmunity (Chae et al., 2014).
In the past years, thanks to fine-scale genetics and well-annotated genomes, it has been made possible to identify hybrid necrosis triggering epistatic loci down to individual genes. The first causal link between hybrid necrosis and autoimmunity has been established in A. thaliana with the epistatic interaction between DANGEROUS MIX1 (DM1) and DANGEROUS MIX2 (DM2; Bomblies et al., 2007). Both DM genes are located in the polymorphic, multi-gene clusters encoding multiple NLRs, with DM2 homologs having known isolate-specific resistance function to the adapted pathogen Hyaloperonospora arabidopsidis (Hpa; Figure 1C). Later, a systematic study carried out with over 6000 F1 hybrids generated from 80 A. thaliana parental accessions corroborated the notion that hybrid necrosis is commonly mediated by negative epistasis between highly polymorphic components of the immune system, such as NLRs (Chae et al., 2014). This large-scale investigation provides a species- and genome-wide insight on which immune components are more prone to generate predisposed alleles for autoimmunity. Interestingly, not all NLR loci turned out to contribute to the generation of autoimmunity-risk alleles but there exist particular genome loci recurrently spawning the risk alleles (Chae et al., 2014). Among such hybrid necrosis hot spots in the A. thaliana genome, the DM2 cluster is the most prominent one producing multiple hybrid necrosis alleles interacting with a broad range of loci, including other NLR-containing clusters and the loci encoding enzymes (Alcázar et al., 2010; Chae et al., 2014; Tran et al., 2017). For instance, hybrids between Ler and Kas-2 accessions of A. thaliana display mild-F1 hybrid necrosis with obvious phenotypes found in F2 and later generations, and the causal BDM pair is an NLR encoded by the DM2 locus from Ler and the SRF3 RLK from Kas-2 (Alcázar et al., 2009, 2010). There also seems to be a loyal partnership between BDM epistatic loci: multiple allelic pairs of DM6 and DM7 have been discovered to cause distinct classes of hybrid necrosis (Chae et al., 2014; Barragan et al., 2019). It has also been revealed that deleterious interactions can occur between different alleles at a single locus, such as DM8 and DM9, as illustrated in Figure 1A (right; Chae et al., 2014). Recent analyses on the complex NLR encoding loci, of which dataset had been much improved by long-read sequences compared to Illumina short read-mapping based NLR assembly, revealed that the DM loci are definitely characterized with a high propensity of genomic rearrangements, including asymmetric cluster expansion and contraction in the A. thaliana natural accessions (Van de Weyer et al., 2019; Jiao and Schneeberger, 2020; Lee and Chae, 2020). Thus, a high correlation between the DM locus predisposition and structural variation involving copy number variations (CNVs) emerges as an obvious feature. Given that the causality often maps to a single gene in the DM multi-gene clusters, it is likely that the cluster expansion and contraction events promote to generate such deleterious alleles. Importantly, the large-scale hybrid necrosis survey in A. thaliana has not only provided the incidence rate of hybrid necrosis at about 2% but also established high-throughput platforms for identifying causal allelic variants under epistatic interaction. Once an allele frequency contributing to the same type of hybrid necrosis is determined under a large-scale crossing scheme, such as diallelic crosses, genome-wide association studies (GWAS) can be utilized to identify single-nucleotide polymorphisms (SNPs) tagging the causal variants (Barragan et al., 2019, 2020). It has been also found that geographic distances between two parental lines do not necessarily correlate with the chances of running into immune system incompatibilities, as was demonstrated by DM1 and DM2d carriers, Uk-3/Uk-6 and Uk-1 coming from a small town in Germany, Umkirch (Mouchel et al., 2004; Bomblies et al., 2007).
In crops, a systematic study focused on autoimmune hybrids has not been carried out obviously due to its seemingly low economic and agricultural potential, but there are certainly a massive number of examples reported from breeding panels. In an interspecific hybrid of lettuce, a deleterious epistatic interaction involving RPM1 INTERACTING PROTEIN4 (RIN4) and potentially an R gene has been identified to lead to a dosage-dependent hybrid necrosis (Jeuken et al., 2009). Later, the causal interacting R locus, DM39, was mapped to an NLR gene cluster (Christopoulou et al., 2015; Parra et al., 2016). The protein RIN4 is one of the most well studied immune components (guardee, see section Diversity of NLRs in Sequence and Function for details) in plants, of which integrity is being monitored by multiple R proteins. Importantly, the most extreme case of hybrid necrosis has been reported in cotton in which an NLR gene, Le4 leads to F1 interspecific hybrid lethality in the cross between Gossypium barbadense and Gossypium hirsutum (Deng et al., 2019). The relevance of these findings in crops lies in the fact that a functional correlation could be made between hybrid necrosis alleles and disease resistance traits in the field. One of the most important unanswered questions is whether hybrid necrosis alleles can arise through the course of enhancing disease resistance either from selective breeding or through evolution in the wild. This connection is to be best addressed when hybrid necrosis research is carried out in crops with well-defined pathosystems and available breeding history such as in rice.
In this review article, we overview recent progress in hybrid necrosis research focusing on genetic and molecular details. Although initial works were favorably discussed under speciation mechanism, current research outcomes are heavily detailed in inbreeding species for obvious practicality in the pursuit of identifying causal genes. With a strong link to the plant immune system, we take an initiative to extend our view of hybrid necrosis to study plant performance in hybrids under the equilibrium of growth and immunity. We also attempt to revisit the cases of rice genetic incompatibilities responsible for various deleterious phenotypes found in F1 and F2 hybrids to examine if any of the reported criteria falls under the definition of immune system incompatibility. We propose that hybrid necrosis as a result of immune system incompatibility illustrates different levels of balancing act between immunity and growth. Thus, the characterization of hybrid necrosis in crops can guide breeding efforts to equilibrate resistance and yield to produce elite hybrids. This strategy will be highly informative in crops relying on hybridization as a major breeding method such as in rice. To this end, we explain in detail the molecular mechanism governing the autoimmunity in hybrids and suggest a way to mitigate autoimmunity to simultaneously enhance growth and resistance in breeding lines. Finally, we present the possible application of reverse breeding, a breeding technique that can rapidly deliver different types of mapping populations useful for the study of hybrid necrosis and heterosis.
Hybrid Necrosis and Heterosis: A Matter of Extremes
Trade-off Between Immunity and Growth
The trade-off between immunity and growth in plants is thought to occur as a result of prioritizing resource allocation to either of the two processes. An optimized equilibrium in response to external or internal factors would be achieved to ensure plant fitness and health (Huot et al., 2014). Optimal immune responses certainly promote the survival of plants in the time of needs, such as to fend off pathogen attack. For instance, under a certain avirulent bacterial pathogen load, it has been demonstrated that A. thaliana plants carrying the matching NLR will initialize an acute immune response, also known as effector-triggered immunity (ETI). Despite the fact that such a response disposes infection sites via activating local cell death, known as the hypersensitive response (HR), these plants display overall enhanced fitness as compared to those that do not carry the corresponding NLR. Indeed, regarding lifetime fitness, resistant lines can significantly outperform susceptible individuals when both are infected with pathogens. When total silique length was used as a proxy for lifetime fitness, it has been demonstrated that resistant A. thaliana isolines carrying an allele of RPS5 (RPS5+) NLR that confers resistance to the avirulent Pseudomonas syringae strain show enhanced values by 9.6–32% in overall fitness measurement compared to susceptible lines (RPS5−) under the semi-controlled glasshouse condition (Gao et al., 2009). These findings support the idea that robust resistance conferred by an NLR for a matching pathogen enhances fitness if plants are under pathogenic attack.
The fact that energetic resources will be consumed once ETI is activated can logically explain a growth penalty. The complexity of this trade-off, however, largely resides in the fact that a fitness cost is associated with resistance even in the absence of obvious pathogen load. The pioneering work from Bergelson’s group provided strong quantitative evidence for this (Tian et al., 2003). The RPM1 NLR is an R gene in A. thaliana that confers resistance against the P. syringae strain DC3000 carrying matching avirulence (Avr) effectors (Dangl et al., 1992; Innes et al., 1993; Bisgrove et al., 1994). To examine the cost that RPM1 resistance would exact, susceptible host lines were transformed with the RPM1 resistant allele to create a total of four isogenic lines (RPM1+). When RPM1+ lines were compared against susceptible lines (RPM1−) in a field trial where the avirulent strain was absent, RPM1+ showed lower shoot biomass and an average of 9% seed-set reduction as compared to RPM1−. Interestingly, resistant and susceptible alleles of RPM1 coexist in the global populations of A. thaliana, indicating that balancing selection acts on to maintain both alleles. The signature of balancing selection endorses the premise that RPM1 confers both a fitness advantage and a fitness cost depending on the presence and absence of the matching pathogens (Tian et al., 2003). A similar experimental setup has shown that carriers of an NLR, RPS5 (RPS5+), that confers resistance to P. syringae carrying AvrPphB or its homologs, suffer from a yield penalty in comparison to lines carrying the susceptibility allele (RPS5−; Karasov et al., 2014). Field trials performed with the RPS5+ and RPS5− that were not exposed to P. syringae showed a reduction in the seed-set between 5 and 10.2% in the RPS5+ lines in comparison to RPS5− lines. Supporting the fitness cost associated with RPS5 resistance, a balanced polymorphism for RPS5 has also been observed (Tian et al., 2002). In both cases, fitness costs associated with the NLRs led to about 9–10% decrease in seed production in the absence of obvious pathogen infection, and such costs are not likely to be explained merely by the metabolic cost of RPM1 and RPS5 synthesis. There can be alternative reasons for the observed fitness costs in both experimental setups. First, although the particular pathogen that is thought to trigger the HR was not found during the field trials, it is possible that other, yet unknown, effectors from several pathogen species can interact with RPS5 and RPM1, triggering a constitutive defense response that would negatively impact growth (Karasov et al., 2014). RPM1 in particular has been shown to be a common target of multiple effectors in laboratory conditions (Grant et al., 1995; Kapos et al., 2019; Ray et al., 2019). Such diffused R-Avr interactions, as opposed to Flor’s gene-for-gene hypothesis, would also explain relatively high frequencies of RPM1+ and RPS5+ natural accessions of A. thaliana despite the cost (Stahl et al., 1999; Rose et al., 2012; Karasov et al., 2014). Second, RPM1 and RPS5 may negatively interact with other R genes in the introduced background (Tian et al., 2003). Such type of interaction could very well correspond to negative epistasis following the BDM model, but further research is required to confirm whether there are partners for RPM1 or RPS5 exerting mild autoimmunity. Intriguingly, RIN4, an important host partner of RPM1, has been identified as a hybrid necrosis gene in lettuce interacting potentially with an NLR gene, indicating the BDM epistasis may occur through a host protein that such costly NLRs surveil. Overall, these findings imply that the composition of R gene repertoire in a given population determines the cost exerted to affect overall fitness because a fraction of costly R genes would bring down yield even under seemingly non-pathogenic conditions. In addition, these results highlight the importance of controlling such costly R genes in the host germplasm.
Since a well-managed trade-off can reward breeding efforts, there have been substantial research endeavors to uncover regulatory mechanisms that coordinate growth and immune responses (Karasov et al., 2017). Immune responses supposedly divert substantial amounts of resources that otherwise would be invested in growth, and multiple hormonal pathways have been under investigation to establish the link between growth and immunity (Purrington, 2000; Huot et al., 2014). Several studies have pointed out that crosstalks between different hormonal pathways are instrumental in shifting the balance between growth and defense (Glazebrook, 2005). Generally, but not exclusively, it is considered that growth-promoting hormones, including cytokinins, brassinosteroids (BR), auxins and gibberellins (GA), act against defense-promoting hormones such as ethylene (ET), salicylic acid (SA), and jasmonates (JA; Liu et al., 2008; Tsuda et al., 2009; Clouse, 2011; Emenecker and Strader, 2020). However, direct molecular links between an individual or a suit of hormonal pathways and immune responses still remain to be investigated in-depth (An and Mou, 2011; Chen and Ronald, 2011; De Vleesschauwer et al., 2014; Lozano-Durán and Zipfel, 2015). A mechanistic insight about the trade-off involving NLR-mediated immunity has been provided by the work in rice, demonstrating functional dependency between the fungal resistance NLR Pik-H4 and its interacting protein OsBIHD1 that encodes a homeodomain transcription factor (Liu et al., 2017). Physical interaction between Pik-H4 and OsBIHD1 is necessary for ETI, supporting the importance of OsBIHD1-mediated control in immunity. During infection, OsBIHD1 launches a growth-inhibiting process by activating reactions that catabolize the growth-promoting BR. OsBIHD1, in addition, facilitates ET synthesis, which in turn enhances the NLR-mediated immunity. Interestingly, not only the overexpression but also the knockout of OsBIHD1 leads to dwarfism, suggesting that OsBIHD1 is a critical factor coordinating growth potential through the regulation of other growth-promoting hormones or processes (Liu et al., 2017). This work elegantly describes how a single factor is able to modulate ET-BR crosstalk in response to NLR-mediated defense and how such modulation affects plant growth. Engagement of such an important growth regulator into the NLR action during infection appears to be a way to effectively reallocate resources.
On the other hand, untimely or uncontrolled activation of immunity often results in a decrease in plant fitness. Indeed, numerous lesion-mimic mutants identified in A. thaliana and rice illustrate a skewed balance toward constitutive activation of immune responses, resulting in growth compromise typically expressed with dwarfism, leaf curling, stunted roots, and death (Heil and Baldwin, 2002; Zhu et al., 2020). Some of these lesion-mimic mutants in A. thaliana were further characterized to reveal the contribution of NLR to such autoimmunity (van Wersch et al., 2016). For instance, a gain-of-function mutation in one of the DM1 homolog is named SSI4 (SUPPRESSOR OF SALICYLIC ACID INSENSITIVITY OF NPR1-5, 4) characterized with obvious autoimmune symptoms (Shirano et al., 2002). The most well-characterized autoimmune mutant would be snc1 (suppressor of npr1-1, constitutive 1) in A. thaliana, which had been identified with its dominant allele suppressing defects of npr1-1 plants in SA pathway regulation (Li et al., 2001; Zhang et al., 2003). Both dominant mutations fall in the domain of the two NLRs important for regulating its activity. Another lesion mimic mutant in A. thaliana, acd11 clearly shows autoimmunity-related compromise in growth due to a mutation in the gene involved in sphingolipid modification (Brodersen et al., 2002). However, later it has been demonstrated that the cell-death observed in acd11 can be suppressed by mutations in LAZ2, a gene that encodes a histone-methyltransferase, which in turn controls the expression of an NLR named LAZ5 (Palma et al., 2010). Likewise, ACD6-mediated growth compromise commonly observed in naturally occurring autoimmune accessions has recently been shown to require particular natural alleles of SNC1 (Todesco et al., 2010; Zhu et al., 2018). Similar contributions of SNC1 and other NLR alleles had been observed to regulate the class of BONZAI mutants characterized by dwarf phenotypes (Hua et al., 2001; Yang and Hua, 2004; Li et al., 2009). The involvement of NLRs in these lesion-mimic mutants strongly suggest that cell death execution in these mutants likely is mediated by NLRs, and such mutants indeed reveal regulatory mechanisms tightly controlling NLR activity to balance the equilibrium with growth promotion.
The above-described findings hint us to assess NLR function in lesion-mimic mutants in other species. For instance, a rice lesion-mimic mutant collection includes Spl26 and spl17 exhibiting chlorosis and cell death, as well as conferring resistance to both fungal blast and bacterial blight (Wu et al., 2008). The penalty on plant fitness is high in these mutants such that homozygosity of the dominant lesion-mimic mutations prevents them from reaching maturity and that the trans-heterozygosity between them makes plants lethal (Wu et al., 2008). Due to the enhanced resistance conferred by these rice mutants, one can easily hypothesize a link to NLR-mediated strong immune responses as the cause for the severe autoimmunity in rice. It would be a rewarding research agenda to further investigate the lesion-mimic mutants in rice with beneficial field resistance traits, which will allow us to examine how such enhanced resistance is achieved and examine the relative importance of causal factors in the plant immune system. In rice, constitutive activation of defense signaling and growth inhibition can also be simultaneously mediated by WRKY transcription factors. Overexpressing lines of OsWRKY31 show constitutive activation of defense-related genes and enhanced resistance to rice blast, however, growth penalty is observed in lateral root formation and elongation (Zhang et al., 2008). The growth-deficient phenotype has been attempted for rescue by the administration of exogenous auxin, but due to a reduced auxin sensitivity in this line the trial failed. The result suggests that OsWRKY31 could act in the signal transduction of both the auxin and defense responses (Zhang et al., 2008), offering promising grounds to investigate the role OsWRKY31 in growth and immunity. WRKY transcription factors are known for its general function in defense in plants such as OsWRKY03 acting upstream of NPR1 (Liu et al., 2005), WRKY70 in A. thaliana mediating crosstalk between JA and SA (Li et al., 2004), and most importantly, decoy WRKY domains integrated to NLRs (Cesari et al., 2014a; see NLR-IDs in section Mechanisms of NLR-Mediated Autoimmunity Responsible for Hybrid Necrosis for detail). An additional functional link between WRKY and NLRs in rice has been established with five rice NLR proteins (Piz-t, Pib, Pi36, Pit, and Pita) interacting with WRKY45 and WRKY66 (Liu et al., 2016). Thus, it seems that growth-affecting defense pathways known in rice shall be revisited to further refine their molecular details to reveal regulatory mechanisms for the trade-off between growth and immunity.
Yield Penalty Accompanying Disease Resistance Traits Reflects the Trade-off
In breeding panels, the above-mentioned trade-off has been conceptualized under yield penalties associated with disease resistance traits. Low yield, due to reduced grain weight and/or a general reduction in plant growth, reflects a skewed balance favoring the maintenance of resistance traits that would protect crops from pathogenic infection at the expense of yield (Ning et al., 2017). Rice yield has steadily increased since the early 1960s in Asian countries like China, Vietnam, Indonesia, and India (Qian et al., 2016). The success of hybridization programs and the identification of major quantitative trait loci (QTLs) for heterosis along with their systematic incorporation to the existing elite lines are certainly responsible for major increases in rice production (Qian et al., 2016). Despite breeding success in yield traits per se, sustaining high yield in the deployed lines is evidently facing problems due to pest management. Indeed, rice production is being seriously affected by several pathogens such as the insect brown planthopper (Nilaparvata lugens), rice blast fungus (Magnaporthe oryzae), rice bacterial leaf blight (Xanthomonas oryzae pv. oryzae), rice bacterial leaf strike (X. oryzae pv. oryzicola), and Tungro virus (Azzam and Chancellor, 2002; Bottrell et al., 2012; Liu et al., 2014). Climate change is predicted to escalate yield losses as both disease resistance and yield traits are optimized for certain environmental conditions (Qian et al., 2016; Muehe et al., 2019). Thus, engineering robust and durable disease resistance traits with a minimized yield penalty in elite rice hybrids is an urgent matter.
Unfortunately, the catalog of field-effective R genes in rice rather remains short-listed. Although hundreds of QTLs for resistance against different pathogens across taxa have been identified in experimental populations, only a handful of R genes have been used for hybrid breeding (Liu et al., 2014; Qian et al., 2016; Li et al., 2019). For instance, among ~30 genes cloned to date, most of which encoding NLRs (Wang et al., 2017), only a limited number of loci, for example, the locus encoding Pi9/Pi2/PigmR, have been under frequent use due to their ability to confer durable and/or broad-spectrum resistance for rice blast isolates (Cesari and Kroj, 2017; Deng et al., 2017; Tian et al., 2019). Although recent reports have successfully identified over a hundred potential new R genes against different isolates of rice blast out of hundreds of cloned rice NLRs present in Tetep and other blast-resistant cultivars (Zhang et al., 2015; Wang et al., 2019c), introgression of the newly discovered in elite lines have not yet been reported. Given that Xa2, a favorable blight R gene with no obvious yield penalty is of Tetep origin (Ogawa et al., 1991), genome-assisted R gene discovery from a cultivar with broad-spectrum resistance offers a promising path. In addition, Xa4 and Xa21, along with Xa2, are non-NLR R proteins and characterized by their durable nature and least yield penalty (Ogawa et al., 1991; He et al., 2006; Kottapalli et al., 2010; Ning et al., 2017), suggesting potential routes to broaden our search for functional R proteins other than NLRs. On the other hand, achieving durable resistance for pathogens like rice blast is still challenging as resistance conferred by single R genes would often break down within 3–5 years due to rapid cycling of pathogens that outpace the host immune system adaptation (Devi et al., 2015). To broaden the spectrum and durability of blast resistance, several R genes have been combined in the same background (Wang et al., 2019c). Although the current endeavor focuses on pyramiding R genes to promote both broad-spectrum and durable resistance in commercial lines (Hu et al., 2012; Miah et al., 2013; Xiao et al., 2017; Sabar et al., 2019), pyramiding resistance genes for any of the major rice pathogens in a novel genetic background is expected to encounter negative epistasis between newly assembled R gene combinations to a certain extent (Figure 1C). In addition, usually each set of R genes has been cloned based on the specific resistance to a single pathogen species or even to an isolate (Flor, 1971). Furthermore, it is technically tedious and costly to incorporate several R genes in new breeding lines every few years (Li et al., 2019). The introgression of R genes from donor lines does not always result in enhanced resistance in the recipient background due to an interference in immunity from the existing immune components (Hurni et al., 2014; Stirnweis et al., 2014; Kim et al., 2019). Finally, only several R genes are known not to affect yield after introgression in the commercial lines (Ning and Wang, 2018).
Negative effects on yield born by R gene pyramiding in crops are well documented (Ning and Wang, 2018). For this reason, introgression of new R genes in rice elite varieties is very often accompanied by a thorough evaluation of possible yield penalties (Xiao et al., 2017). Due to the correlation observed between R gene pyramiding with a decrease in yield, it is tempting to speculate that rice elite varieties that produce high-yielding hybrids have not only accumulated heterotic traits but also dispensed a suit of costly or unnecessary R genes during the breeding. Therefore, it is plausible that these lines have been selected for R gene loci that had accumulated mutations abrogating or compromising the resistance function. Supporting this proposal, an impressive large-scale GWAS on rice heterosis revealed that breeding strategies for increasing yield inadvertently selected for susceptibility alleles for blast and leaf-blight resistance genes in rice. Huang et al. (2015) analyzed 1,495 elite hybrid and their respective inbred parental lines of Oryza sativa spp. indica and O. sativa ssp. japonica to investigate the genetic architecture governing heterosis in rice hybrids mainly bred in 1990s and 2000s. This study has identified major loci contributing to heterosis with large effects on certain traits, including disease resistance and grain quality together with multiple small-effect loci for grain yield, thus presenting a comprehensive view on trait fixation during domestication. One of the most striking results from this work regarding the link between immunity and growth is that high yield in hybrids is associated with the accumulation of susceptibility alleles. In the evaluated hybrids, the frequency of the resistant alleles for the locus Pi2/Pi9 for rice blast and a locus associated with resistance to leaf-blight in chromosome 6 was 3.7 and 2.6%, respectively, which implies that the accumulation of susceptibility alleles had resulted in positive effects on yield production (Huang et al., 2015).
Accumulating susceptibility alleles on known R genes for rice blast and leaf-blight during breeding suggests that there had been a selection pressure favoring susceptibility alleles at those loci. Potentially, the absence or low number of avirulent strains for these resistances as well as fitness cost innate in such alleles might have rendered the resistant carrier generating low performing hybrids than the ones carrying a susceptible allele when used as parents. Unlike natural populations where such resistant and susceptible alleles are being maintained by balancing selection due to stochastic selective pressure imposed by pathogens (Tian et al., 2003; Karasov et al., 2014), selective breeding would favor seemingly higher-yielding ones at the expense of resistant alleles. These examples suggest that the trade-off between growth and immunity had played a critical role in directing the R gene repertoire in crops to be streamlined only for an immediate purpose. The artificial selection against resistant alleles of no immediate benefits would make the elite germplasms susceptible to diseases that can be easily resisted by their wild relatives. Less diversity in R genes in a population would increase the vulnerability to new pathogens, while the introduction of a new immune component such as an R gene becomes cumbersome. Problems in readapting R genes from wild relatives in the introgressed cultivated lines are evident, which caught not only breeders attention but also researchers who investigated complexity in the plant immune system. The first scientific report for a clashing immune component with the introgressed R gene appeared with the identification of Rcr3, an important immune papain-like cysteine endoprotease in tomato, that conflicted with the Cf-2 R gene from wild tomato Lycopersicon pimpinellifolium (Krüger et al., 2002). Negative epistasis clearly explains the incompatibility between Rcr3 and Cf-2, triggering uncontrolled cell death and compromise in growth. The fine genetic analysis revealing epistasis in tomato made a foundation for hybrid necrosis studies to be established under the BDM model.
Hybrid Necrosis Exemplifies a Trade-off Between Immunity and Growth
An obvious trend observed for hybrid necrosis cases in A. thaliana and other species is that uncontrolled immune responses negatively impacts on overall plant performance. The effects could range from mild compromise in growth to severe tissue necrosis and distortion in developmental programming (Alcázar et al., 2009; Jeuken et al., 2009; Yamamoto et al., 2010; Chae et al., 2014; Todesco et al., 2014; Sicard et al., 2015; Deng et al., 2019). In most hybrid necrosis cases, plants do not reach maturity to produce seeds. The characterization of overt hybrid necrosis in multiple plant species thus faces challenges in obtaining viable seeds to generate mapping populations unless advanced crossing schemes are engaged. Nonetheless, genetic architecture and molecular mechanisms underlying hybrid necrosis have been discovered since the detrimental autoimmune symptoms are temperature sensitive; changes in temperature can drastically suppress the symptoms and F2 progeny becomes available (Bomblies et al., 2007; Jeuken et al., 2009; Muralidharan et al., 2014). Our current understanding on the link between uncontrolled immunity and developmental defects and growth compromise still remains to be descriptive and further molecular characterization to define the connection is in need. Considering that the major mechanism underlying hybrid necrosis has been unequivocally attributed to autoimmunity only in recent years, it is very likely that the incidence of hybrid necrosis cases in rice and other crop species have been so far overlooked. The lettuce RIN4 is an example of the discovery of epistasis upon revisiting the previous QTL mapping results for disease resistance traits under scrutiny (Jeuken et al., 2009). Therefore, to estimate the frequency of hybrid necrosis in crops, it would be necessary to reassess the data available from the literature and examine the cases of deleterious phenotypes observed in F1 and F2 hybrids to address how many of those could be contributed by immune genes and thus categorized under hybrid necrosis stemming from negative epistatic loci.
Deleterious phenotypes in rice hybrids have been reported as early as in 1957 by Oka, who described two different phenomena: hybrid inviability in the F1 generation and hybrid breakdown in F2. Oka established that dominant alleles cause plant death (Oka, 1957), which essentially conveys the concept of an allelic interaction following deleterious epistasis. In addition, he demonstrated that the genetic cause of the F2 hybrid breakdown was due to a set of duplicated genes in which the combination of recessive alleles leads to poor growth, proving a classic Michael Lynch’s prediction of hybrid incompatibilities arising from the combination of duplicate genes that had followed the independent fate (Lynch and Conery, 2000). Hybrid incompatibility due to negative epistasis between duplicated copies of the histidinol-phosphate aminotransferase gene which codes for HPA, a protein important in the biosynthesis pathway of histidine, has also been reported in A. thaliana (Bikard et al., 2009). The framework of hybrid weakness in rice prompted researchers in the following years to pursue more cases and characterize pairs of loci responsible for the range of hybrid weakness phenotypes (Table 1). Such research endeavors have identified some of the incompatibility loci to be mapped or linked to the R gene family (Table 1). For instance, Hw4 was found to induce hybrid weakness when interacting with Hw3, which encodes for a putative calmodulin-binding protein (CaMBPs). We found it intriguing as CaMBPs are involved, among other processes, in plant defense mechanisms (Ali et al., 2003; Fu et al., 2013). Interestingly, further linkage-mapping analysis defined that Hw3 was linked to Pi1, an allele of the Pika locus that confers resistance to blast, although it turned out that Pi1 and Hw3 was not the same gene (Hua et al., 2012; Fu et al., 2013). These findings show that Hw3 and Hw4 are both the causal genes of the hybrid weakness phenotype observed in rice, although the role played by components of the immune system in this genetic background and by the linkage between Hw3 and the R gene is yet to be investigated. Moreover, the work of Yamamoto et al. (2007, 2010) has undoubtedly established the correlation between hybrid necrosis and autoimmunity in an indica-japonica F2 rice hybrid. Indeed, this particular genetic incompatibility case was investigated under the BDM-epistasis model, revealing that the interaction between two recessive genes, hbd2 that codes for a casein kinase I (CKI1) and hbd3 that is mapped to an NLR gene cluster region, is responsible for the reduced height and number of tillers in the autoimmune lines (Yamamoto et al., 2010). Interestingly, the deleterious allele effect of hbd2 has been attributed to one amino acid change, indicating that a reproductive barrier between varieties of rice can be easily established due to autoimmunity (Yamamoto et al., 2010).
The number of underperforming hybrids described in the literature (Table 1) indicates that this phenomenon has an appreciable incidence in rice, which requires further attention for various reasons. First, we cannot exclude the possibility that hybrid necrosis has played a role in the observed underperformance. During rice domestication, disease resistance traits have been primarily selected within breeding panels of a region. This suggests that an imminent local pathogen load may have imposed a strong pressure selecting for a robust R gene in the bred lines. As has been demonstrated in hybrid necrosis cases, a new combination of R repertoire could result in underperforming hybrids, which may have not yet been rigorously classified under the hybrid necrosis category. As genetic architecture is simple, cases under BDM epistasis would easily generate lists of causal loci. In addition, it has been shown that in permissive or close-to-permissive temperature ranges the inferior hybrid phenotypes are alleviated (Saito et al., 2007; Fu et al., 2013; Chen et al., 2014; Muralidharan et al., 2014). Therefore, the characterization of underperforming hybrids under different environmental conditions and genetic identification of causality are relevant for improving hybrid performance in general. Finally, hybrid necrosis, not only severe but also mild ones, would preclude the expression of heterotic phenotypes. We believe that cryptic heterosis can be easily released if deleterious effects from a degree of autoimmunity are managed. We speculate that the negative correlation between immunity and plant performance, in particular growth and yield, is an important bottleneck for achieving heterosis in hybrid rice, which shall be extensively investigated with the underperforming hybrids. Not all underperformance would be attributed to the trade-off between growth and immunity, but addressing the proportional contribution made by elevated immunity to a decrease in growth can be effectively carried out using such rice hybrid germplasms. For this reason, we propose that the heterotic expression of agricultural traits can be improved when a degree of autoimmunity triggered by immune system conflicts is properly defined and mitigated.
Fitness Equilibrium Model in Plants: Anti-hybrid Necrosis as Part of Heterosis?
The idea of mitigating autoimmunity in hybrids to increase overall fitness posits that the equilibrium between growth and immunity is an important factor determining plant performances. How such an equilibrium can be regulated and how the overall immunity of an individual limit the manifestation of heterotic traits remains to be determined. One might easily question if dampening residual or basal immunity level by systematically knocking down prominent immune receptor genes would indeed increase plant performances and yield under no obvious pathogenic loads. Such a systematic approach has not been embarked on; however, it appears that nature has embraced the needs to evolve certain microRNAs that can target multiple NLRs at a time to reduce NLR activity by and large (Zhai et al., 2011; Shivaprasad et al., 2012; Zhang et al., 2016). In addition, the expression of a cohort of NLRs clustered in a multigene cluster can be coregulated through the action of small-RNAs produced within the cluster (Yi and Richards, 2007). The complexity of such regulatory mechanisms as well as the genome studies focused on NLR evolution in different species at least provides us with the breadth and intricacy of NLR diversity present in natural populations (Van de Weyer et al., 2019; Lee and Chae, 2020; Seong et al., 2020). Although cataloging NLRs present in a species would not pinpoint which NLRs and how many of them are cost-exacting or predisposed to trigger autoimmunity in hybrids, detailed analysis of the pan-NLRome might inform us which of them had undergone either positive or negative selection and are associated with other traits than disease resistance, such as yield. Due to the poor-mapping quality of short reads, GWAS studies or other SNP-based genome-wide scans certainly have missed information covering complex NLR loci, often tandemly repeated with a massive structural variation. An improved assembly by whole genome sequencing with longer reads as well as the targeted assembly of NLR genes will greatly improve the resolution of trait mapping and genotype-phenotype association. In this regard, rice hybrids on the other hand offer a great system to investigate the potential contribution of hybrid incompatibilities of the plant immune system to limit the expression of heterosis. The exceptional amount of field-collected phenotype data in rice are not comparable to those existing in any other species, which will become a crucial component in the attempts to associate the phenome to immune-centered genotype platforms in rice.
Here, we illustrate an equilibrium model that represents different manifestations of the trade-off between growth and immunity in natural populations, crop inbred lines, and elite hybrids on the basis of genetic trait distribution for disease resistance and heterosis (Figure 2). We propose that hybrid necrosis and heterosis lie in the extreme end of such equilibrium as the result of disproportionate phenotypic manifestations of uncontrolled immunity and exacerbated growth, respectively. As explained above, the genetic architecture regarding the R repertoire affects plant fitness both in the presence and absence of pathogens. In addition, the accumulation of heterotic alleles corresponds to an increase in growth and yield, as long as they contain an optimum R repertoire that does not cause yield penalty. In a given natural population (gray) where multiple types of immune alleles are being maintained, we envision that the fitness level of individuals in the population would show a wide distribution with a peak that reaches the point optimally balanced with the two opposing forces, immunity and growth (Figure 2). Under a pathogen attack, immune responses in the natural population are activated only among the individuals carrying the cognate immune receptors that can mount robust immunity. On the other hand, non-carriers would suffer serious disease symptoms which eventually decrease the allele frequency of the susceptibility alleles in the population. However, when the pathogenic load is low or absent, the discrepancy in allele frequencies between the carriers and non-carriers becomes rebalanced because the fitness cost in the carriers would render them as unfavorable ones over the non-carriers (Tian et al., 2003; Karasov et al., 2014). For instance, stable natural populations of A. thaliana would experience bouts of such fluctuating events given that pathogenic loads in nature are believed to be stochastic (Karasov et al., 2018). In our model, as the immunity and growth traits are simplified to manifest as antagonistic forces, the high genetic diversity in the immune system will result in high diversity in growth traits. Thus, compared to selectively bred materials, natural populations would show the widest genetic trait distribution (gray; Figure 2). On the other hand, in the inbreeding crop parents (yellow) that are developed to generate elite hybrids, the peak of equilibrium shifts toward favoring growth as selective breeding has accumulated heterosis alleles (Figure 2). Some inbred lines could even reach a point exceeding the fitness equilibrium, resulting in enhanced resistance for certain pathogens yet with a minimized yield penalty. This has been achieved through domestication efforts that had largely reconstructed R gene repertoires in the genomes (Stein et al., 2018) and through breeding strategies successfully pyramiding a few R genes with the least yield penalty (Xiao et al., 2017; Chen et al., 2020). Under the breeding goal of enhancing yield and resistance in hybrids, R genes in inbred lines predisposed to show extreme deleterious phenotypes in later generations (i.e., hybrid necrosis) should have been eliminated or largely reduced. Additionally, a common breeding practice had favorably used only a small number of R genes which show broad and/or durable resistance for dominant field pathogens (Li et al., 2019). Thus, inbred individuals would show a much narrower distribution for resistant and heterotic traits as compared to ones found in natural populations (Figure 2). Hybridization can easily disrupt the fitness equilibrium to a large extent due to the presence of novel allelic combinations and genetic interactions (Figure 1) that often leads to antagonistic events: hybrid necrosis (red) or heterosis (blue; Figure 2). Elite hybrids (purple) would occupy space in this equilibrium reaching toward far right with the expression of maximum heterotic traits and of least number of resistance traits only suited for a purpose. Maximization of heterotic traits has been shown to be achieved due to the concomitant accumulation of heterotic and/or susceptible alleles in rice breeding (Huang et al., 2015, 2016; Lin et al., 2020). Therefore, this group is skewed toward increased growth and yield reaching a much higher level for optimum fitness if we consider that those plants are grown under controlled environments in the absence of pathogens or in the presence of only a known pathogen that can be resisted (Figure 2). Looking at the bigger picture, however, losses in rice production worldwide are certainly caused by a generalized compromise in immunity in elite hybrids, as no one can predict the next wave of pathogenic attack. We then propose that elite hybrids display an underperforming R gene repertoire with insufficient diversity to face a wide range of pathogenic strains. Such underrepresentation of optimum R repertoires in elite hybrids can be explained by the trade-off favoring growth and selection against hybrid necrosis alleles that might have great resistance potential.
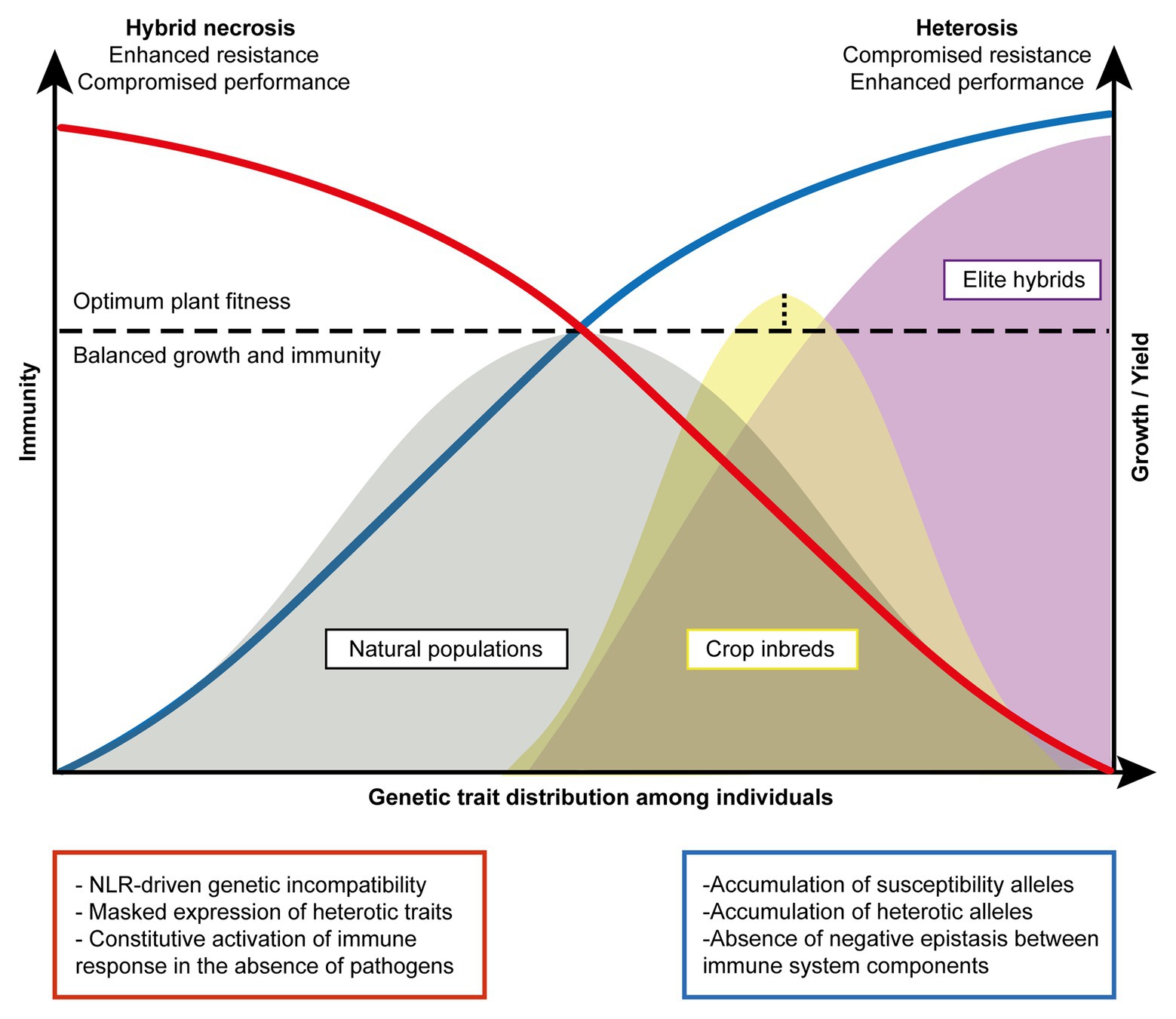
Figure 2. Phenotypic expression of hybrid necrosis vs. heterosis. The model represents an equilibrium between immunity and growth and/or yield achieved essentially to ensure optimum plant fitness (horizontal dotted line). The expression of two extreme phenotypes, heterosis (blue) and hybrid necrosis (red), results from drastic disruption of the equilibrium. The optimum fitness equilibrium is predominantly reached by natural populations (gray area) with robust immune systems characterized by a broad and controlled immune response to biotic stresses. The total frequency of resistant alleles in the natural population potential limits overall growth and yield as compared to commercial lines. Crop inbred lines (yellow area) show a disproportioned accumulation of heterotic alleles. In optimal growth conditions, the fitness of these lines may be superior to the one reached by natural populations (vertical dotted line). Predominant accumulation of heterotic traits shifts the distribution of these lines toward the right, prioritizing the expression of heterosis over a robust immune system. Elite hybrids (purple area) show the highest accumulation of heterotic alleles accompanied by the accumulation of susceptibility alleles for blast rice and leaf-blight (Huang et al., 2015). The performance for elite hybrids can surpass the optimum fitness point under controlled conditions. Nonetheless, in three scenarios, the expression of heterosis may be compromised being: (i) hybrid necrosis, (ii) introduction of R genes to the cost of yield, and (iii) susceptibility to pathogen attack.
Molecular Mechanisms Underlying Hybrid Necrosis
One of the breeding goals in crops lies in broadening the spectrum of disease resistance. Understanding how plants have evolved the immune system to defend multiple pathogens is the first step to deploy different disease resistance traits into breeding panels. Although phytopathogens utilize diverse infection strategies, plants employ seemingly common yet complex immune pathways for defense (Mukhtar et al., 2011; Dou and Zhou, 2012; Weßling et al., 2014; Zhou and Zhang, 2020). The dedicated immune network is featured with prominent classes of immune receptors that perceive invasive events and transduce immune signaling. Coevolution between plants and pathogens drives the diversification of plant immune networks that immune receptors participate in; the system found in a given plant individual is supposedly optimized for its own, but not always function properly when combined in hybrids (Bomblies and Weigel, 2007; Chae et al., 2016). Hybrid necrosis is an extreme outcome of the immune system dysfunction resulting from newly combined immune components independently evolved from each parental lineage. In this section, we summarize recent advances that revealed the mechanism of plant immune receptor action and explain how such mechanistic understanding rationalizes the action of NLR variants predisposed for hybrid necrosis. From the molecular framework, we propose how uncontrolled autoimmune signaling could be derived due to the combination of incompatible immune components involving NLRs. Our mechanistic view on the NLR-based autoimmunity provides a strategy to optimize the growth-immunity equilibrium toward breeding elite hybrids.
Diversity of NLRs in Sequence and Function
Faced with constant threats from pathogens, plants use a suit of membrane-bound and intracellular receptors to initiate defense signaling pathways to confer disease resistance (Kourelis and van der Hoorn, 2018). Most cell surface receptors are pattern-recognition receptors (PRRs), which include RLKs and RLPs. RLKs contain an extracellular LRR domain, a single-pass transmembrane (TM) domain, and an intracellular kinase domain. RLPs are structurally similar to RLKs except they do not contain a kinase domain (Boutrot and Zipfel, 2017). They could perceive the conserved non-self molecules, termed as pathogen-associated molecular patterns (PAMPs) or self-molecule damage-associated molecular patterns (DAMPs), and activate PAMP-triggered immunity (PTI; Boller and Felix, 2009; Boutrot and Zipfel, 2017). Some pathogens could yet evade from the PTI-based host immunity by secreting effectors that promote pathogenic virulence by suppressing immune perception or modifying host physiology (Toruño et al., 2016). NLRs are the family of immune receptors that sense the effectors or effector-driven modifications in the plant cell to initiate an acute immune response, ETI. Reflecting the effectiveness of ETI, NLRs constitute a major fraction of R proteins discovered to date across plant species (Cui et al., 2015; Kourelis and van der Hoorn, 2018). Depending on whether an effector and the matching NLR physically associate, the NLR recognition strategy could be divided into “direct” and “indirect” modes. The indirect recognition mode could be further refined to engage a third protein that can be classified either as “guard” or “decoy” in the plant cell (Van der Biezen and Jones, 1998; Dangl and Jones, 2001; van der Hoorn and Kamoun, 2008; Cesari, 2018). Homeostasis in the plant cell is gauged by the status of such host proteins, for which the matching NLRs monitor. Those host proteins become effector-targets upon infection, and NLRs sense such events to trigger ETI. Here, decoys are considered to have been generated to mimic guardees that have important immune functions. Under arms race between rapidly evolving pathogen effectors and host proteins, plants seem to have widened the net to catch the effectors by duplicating effector targets as decoys that in essence have no other roles affecting plant fitness (van der Hoorn and Kamoun, 2008; Ilyas et al., 2015).
NLRs are multidomain proteins with high diversity in structure and sequence. Based on the N-terminal structure, plant NLRs could be divided into three groups; substantial numbers of NLRs, in particular in eudicots, contain the Toll/Interleukin-1 receptor (TIR) domain at their N-terminus, termed as TIR-NLR or TNL in short; NLRs carrying predicted coiled-coil (CC) domains, albeit more variable than TIR in domain definition, are named CC-NLR or CNL; a less-abundant NLR group carries a non-canonical CC that bears similarity to RESISTANCE TO POWDERY MILDEW8 (RPW8) and named as RPW8-NLR or RNL (Shao et al., 2016). The small RPW8 proteins themselves serve as atypical non-NLR R proteins for conferring broad-spectrum resistance to powdery mildew, while some RPW8 domains experimentally cloned out from RNLs have been demonstrated to trigger cell death on its own when expressed in Nicotiana benthamiana (Xiao et al., 2001; Collier et al., 2011). Interestingly, one of the hybrid necrosis DM loci, DM7, encodes multiple members of RPW8s and HOMOLOG OF RPW8s (HRs), highlighting their connection to cell death activity (Barragan et al., 2019). The central part of NLRs is occupied by the nucleotide-binding and Apaf-1, R protein and CED4 homology (NB-ARC) domain followed by the C-terminal leucine-rich repeat (LRR) domain. The NB-ARC domain offers a conserved nucleotide-binding pocket, while LRR plays an essential role in effector recognition and features the highest sequence variability compared to other domains (Collier and Moffett, 2009; Takken and Goverse, 2012; Cesari, 2018; Wang et al., 2019a,b). The modular nature in domain structure, as well as high variability in sequence and copy number, are the key NLR features which presumably promote its versatility in the plant immune system.
Beyond a classical role of NLRs conferring a strain-specific disease resistance, recent findings highlight functional diversity of NLRs in the plant immune system. It is apparent that some NLRs form a pair, commonly encoded in head-to-head orientation in the genome, and require each other to fulfill resistance functions (Sinapidou et al., 2004; Narusaka et al., 2009; Okuyama et al., 2011; van Wersch and Li, 2019). Another group of NLRs assists immune signaling initiated from other NLRs, which are named as helpers. Well-characterized helpers in N. benthamiana and A. thaliana belong to RNLs (Peart et al., 2005; Bonardi et al., 2011; Collier et al., 2011; Castel et al., 2019), while helpers found in multiple Solanaceae species belong to a clade of CNL (Wu et al., 2016, 2017). Diversity in NLR function is likely to reflect the evolutionary processes followed by the massive expansion of NLRs in the plant lineage. Indeed, a recent pan-NLRome analysis carried out in A. thaliana has shown that distinct patterns of sequence polymorphisms exist for the different functional NLR groups (Van de Weyer et al., 2019). Paired NLRs evolve as one functional unit, while classical NLRs conferring resistance to adapted pathogens show the highest sequence diversity in populations, indicating diversifying forces imposed on such NLRs. On the other hand, helpers remain relatively conserved, meeting the needs to work with multiple sensors. A surprising versatility of NLR is also found in its modularity incorporating extra domains into its classical tripartite domains. In particular, domains found in decoys are integrated as part of NLRs as a single translation unit. This type of NLR is called NLR-ID with ID standing for the “integrated decoy” (Cesari et al., 2014a; Wu et al., 2015). Decoy domains of NLR-IDs are known to readily sense the effector presence through physical association, and thus NLR-IDs are considered as sensor NLRs (Cesari, 2018; Adachi et al., 2019). The concept of the sensor has been elaborated through the identification of functionally dependent paired NLRs in rice. Rice NLRs, the RGA4/RGA5 and Pi5-1/Pi5-2 pairs require each other to confer resistance to rice blast fungus M. oryzae through one NLR of the pair, the sensor, which directly binds with the matching effectors through its ID (Lee et al., 2009; Okuyama et al., 2011; Cesari et al., 2013, 2014b). Functional dependency is explained by their ability of forming a heterodimer, in which effector sensing through the ID in the sensor NLR instigates the other to activate ETI to constrain rice blast growth (Cesari et al., 2014b; Figure 3A, middle). In this partnership, the matching NLR to the sensor is considered as an executor NLR due to its ability to transduce immune signaling leading to the HR, of which action appears to be under a negative control of the partnering sensor in the absence of pathogenic effectors. Up to date, a handful of NLR pairs are found under the same working mechanism: in A. thaliana RPS4/RRS1, CHS3/CSA1 NLRs are characterized as pairs with one NLR as a sensor with ID and the other as an executor (Cesari et al., 2014b; Huh et al., 2017; Ma et al., 2018). Although the initially characterized NLR-IDs haven been discovered by their head-to-head configuration in the genomic loci, later systematic search for NLR-IDs across plant genomes revealed that such configuration is not always the case, implying that a gained functional dependency might have promoted a genomic relocation of non-paralogous NLRs to be placed in tandem under linkage to facilitate coevolution of the two.
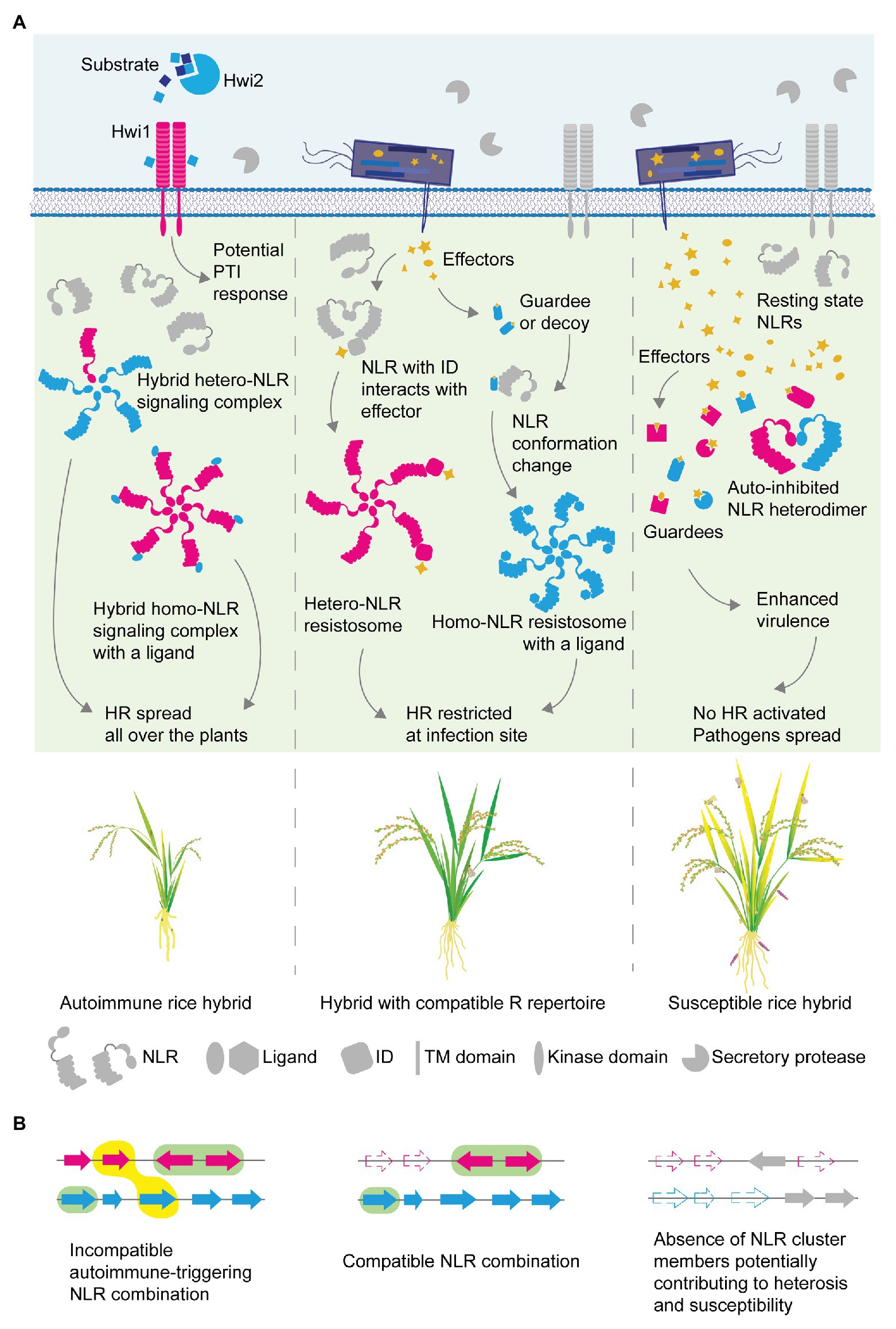
Figure 3. Molecular mechanisms for disease resistance, hybrid necrosis and heterosis in the view of NLR receptor complex formation. (A) NLR complex assembly illustrated in the plant cell of autoimmune (left), normal (middle) or heterotic (right) hybrids. Immune components are color-coded as magenta or cyan to indicate parental origins. Different architecture in NLR illustration, such as a fusion of ID at the C-terminal end, was used to differentiate distinct NLR proteins in the hetero-complex. Domain illustrations constituting the membrane-bound receptor-like kinase (RLK)/receptor-like proteins (RLPs) and intracellular NLRs are indicated at the bottom. The resting state is indicated with gray fill while the active state is with cyan or magenta. Effectors are filled with orange. In a rice hybrid with compatible immune components (middle), effector-modified host proteins are perceived by NLRs, which induces the assembly of a signaling complex, so-called resistosome. NLR-IDs with a decoy domain are hypothesized to form a resistosome with their pair NLRs, forming a heteromeric complex (middle). Conformation changes occurring upon perception are indicated with the closed-form of NLRs and linear form of NLRs. In an autoimmune hybrid (left), signaling NLR complexes form constitutively without effector perception. The complex may consist of NLRs of different parental origins, or of NLR and other host proteins that function as ligands to activate the NLR to initiate and complete the assembly. RLKs of one parent could be constitutively activated by ligands from the other parent to trigger pathogen-associated molecular pattern (PAMP)-triggered immunity (PTI). Accumulation of susceptibility alleles or interference between NLRs in breeding lines (right) can lead to hybrids with compromised resistance. NLR underrepresentation may facilitate effector action toward disease. (B) Schematic diagram of NLR cluster composition assembled in hybrids. The incompatible NLR combination (left) is highlighted with a yellow-filled object. Compatible combinations are marked with green-filled objects (left, middle). Each filled arrow indicates an NLR, while pseudogenization or locus deletion is represented with dashed-lined arrows. Arrows are colored to indicate different parental origins.
Sometimes, NLRs residing within a cluster can impede the signaling capacity of the paralogous NLRs. One example is the Pigm multigene cluster in rice that has been utilized for conferring broad-spectrum resistance to M. oryzae in many regions in China over the decades of breeding (Deng et al., 2017). The enhanced R trait is conferred by PigmR encoding a CNL, while one of the closely related paralogs PigmS in the same cluster opposes the PigmR’s function in a tissue-specific manner. As being a strong R, PigmR shows clear yield penalty as its uncontrolled function in the absence of antagonizing PigmS could cause a reduction in grain weight and size, potentially through autoimmunity. It has been shown that PigmS and PigmR could form a heterocomplex through their CC domains and thus it is plausible that the heterocomplex formation might inhibit the formation of PigmR homo-signaling complexes required for strong immunity. In the extant high-yielding rice lines, the PigmS promoter is highly methylated and the expression is suppressed in foliar tissues but not in the pollen. The tissue-specific expression would condition the trade-off to be balanced differently. In the vegetative tissue, PigmR homo-complexes would form to confer broad-spectrum resistance to M. oryzae when infected or to exert some degree of yield penalty when not infected. However, the net yield penalty is much alleviated as the epigenetic repression of the yield-saving PigmS expression is released in the pollen: supposedly PigmR/PigmS heterocomplex would reduce PigmR-mediated detrimental effects in the pollen, such as cell death or residual autoimmunity, and healthy pollen improve grain quality. This is a great example of how epigenetic regulation, potentially selected by rice breeding, contributes to achieve a balance between resistance and yield (Cesari and Kroj, 2017; Deng et al., 2017). While antagonistic paralogs could positively regulate the trade-off through tissue specificity, it can also hinder efforts to pyramid paralogous NLRs. When an introgressed paralogous NLR find its partner in the recipient germplasms to form a non-functional heteromeric NLR complex, the desired immunity from the introgressed R traits would be suppressed (Figure 3A, right), of which molecular examples are reported in wheat breeding (Hurni et al., 2014; Stirnweis et al., 2014).
Contrary to NLRs requiring or assisting others for full functionality, there are NLRs self-sufficient to complete its function without the need of other NLRs (Adachi et al., 2019). ZAR1 in A. thaliana is the most well-characterized NLR of which structure is recently resolved to reveal successive events accompanying NLR activation and signaling complex formation (Wang et al., 2019a,b). The ZAR1 structure revealed by cryo-EM explains how the previously postulated “on” and “off” status influence NLR activity and signaling complex assembly. The resolved complex includes not only ZAR1 but also resistance-related kinase 1 (RKS1) bound to the LRR domain of ZAR1. When ADP is bound to the NB-ARC of ZAR1, the ZAR1-RKS1 complex remains at resting state and inactive. Yet, when the effector-driven uridylation on PBL2 occurs, RKS1 tethers it to form a ZAR1-RKS1-PBL2UMP complex and ADP/dATP exchange occurs, which is a key process ensuing conformational changes of ZAR1 and successive oligomerization. The resulting full signaling ZAR1 (or ZAR1-RKS1-PBL2) complex appears as a wheel-like pentamer, which is referred as ZAR1 resistosome that is sufficient to fully trigger the HR to restrict Xanthomonas campestris bacterial growth (Wang et al., 2019a,b; Figure 3A, middle). The mechanism underlying the NLR assembly offers an excellent template to envision NLR action and (auto)immunity, in general, to explain how plant performance is affected by controlled or uncontrolled immunity in hybrid plants, which we discuss in the next section.
Mechanisms of NLR-Mediated Autoimmunity Responsible for Hybrid Necrosis
Hybrid necrosis caused by immune system incompatibility appears to result from too much variability in the NLR sequences and potentially in function. Almost all NLRs predisposed to hybrid necrosis are encoded by tandem clusters (Figure 3B); a recently cloned DM10 is encoded by a single locus, however, the locus had been generated through a recent chromosomal relocation event of a multigene TNL cluster in A. thaliana (Barragan et al., 2020). Moreover, the most prominent DM2 cluster along with other large DM clusters show great expandability in cluster size with high CNV detected in the 64 natural accessions (Lee and Chae, 2020). An interesting finding from the pattern analysis of NLR cluster expansion is that not all NLRs in the cluster contribute to the expansion in the global A. thaliana population, suggesting that differentiated functionality within a given NLR cluster may contribute to shaping the NLR repertoire available in the population. Potentially deleterious hybrid necrosis NLR alleles may rise during cluster expansion and contraction, which in some clusters may not be easily purged but maintained due to the linkage to beneficial R alleles within the cluster.
The first cloned BDM pair for hybrid necrosis in A. thaliana is the two NLR-encoding genes, DM1 and DM2d, and these NLRs have been shown to form a heteromeric oligomerized complex in plants through their TIR domains (Tran et al., 2017). A recent molecular investigation on DM6/DM7 hybrid necrosis also has shown that DM6/RPP7 NLR oligomeric status is augmented by the presence of the matching hybrid necrosis pair DM7, RPW8/HR protein through physical interaction between the two proteins (Li et al., 2020). Based on the ZAR1 resistosome structure, we postulate that in the autoimmune hybrids the causal NLR pairs are likely to formulate a hetero-signaling complex consisting of NLRs from different parents. If the BDM pair involves a non-NLR protein and an NLR, the NLR complex would include the host protein such as in RPP7/RPW8-mediated autoimmunity, which is also reflective of the ZAR1 NLR complex including decoys (Figure 3A, left and middle). The hybrid NLR signaling complex exerts immune responses in an uncontrolled manner since the completion of the signaling complex would not depend on an effector-trigger but on their own conformation of participating NLRs. Naturally evolved NLR variants with high sequence similarity have been experimentally demonstrated to potentiate different degrees of signaling (Bernoux et al., 2016). Based on the comparative analysis of closely related flax NLR L6 and L7, Bernoux et al. (2016) proposed that NLRs are present both in active vs. inactive status under equilibrium, which becomes readily shifted toward the active state when the effector is bound. We hypothesize that naturally occurring hybrid necrosis NLR variants adopt conformations close to the active status in a way that the equilibrium is already pulled close to fully active status. Independently evolved NLRs from the reciprocal parent would mistakenly recognize this form as an effector-bound conformation and assemble a fully functional NLR signaling complex. This proposition reminds a situation of paired NLR, where one is an effector-bound sensor and the other is a signaling executer (Figure 3B, middle). Although we proposed that DM1 and DM2d fit under this molecular category, with DM2d masquadering an effector-bound conformation and DM1 recognizing this form to help signaling, none of them carries IDs (Tran et al., 2017). It would be interesting to uncover molecular details of more hybrid necrosis cases, which will expand our knowledge on the functional diversity of NLRs in complex assembly and signaling. The new findings on RPW8/HR4 facilitating a higher-order NLR assembly also reminds of the ZAR1 complex assembly initiated by decoy binding (Figure 3B, left and middle). Whether or not RPW8 proteins are effector targets and how this class of proteins potentiate cell death remain as an active area of research. Our proposition of anti-hybrid necrosis potentially explaining heterosis could be illustrated with the molecular scenarios in the view of NLR complex formation. In the heterotic hybrids, NLR repertoires inherited from parents are supposedly much reduced as a result of selective breeding and the chance of formulating a hybrid NLR complex or decoy-assisted assembly of NLR complex is low (Figures 3A,B, right). Even worse scenario would be the auto-inhibiting NLR complex formation, which can suppress the particular disease resistance traits bred in the respective parents as was discussed in the above section with examples in wheat breeding (Hurni et al., 2014; Stirnweis et al., 2014).
In rice, most hybrid dysfunction has been categorized under hybrid weakness. The case involving Hwi1/Hwi2 caught our attention as Hwi1 encodes two tandemly repeated RLKs from wild rice and Hwi2 encodes a secreted putative subtilisin-like protease in the indica rice variety Teqing (Chen et al., 2013, 2014). With the established contribution of RLKs to PTI, we could hypothesize that Hwi1 from wild origin mistakenly perceives Hwi2-generated products as PAMPs or DAMPs in the hybrid, presumably activating uncontrolled PTI at the cost of yield in the hybrid rice (Figure 3A, upper left). Another immune-related factor contributing to hybrid performance in rice is S5, a disease resistance-related aspartic protease (AP). Allelic conflict at S5 contributes to set up a reproductive barrier between indica and japonica by interfering with the functional embryo-sac production (Chen et al., 2008; Ji et al., 2012; Yang et al., 2012). Although the AP family has not yet been investigated in line with NLR activity, members of this family are involved in defense responses with activated SA in addition to pollen and ovule development in multiple plant species, including rice, grape and A. thaliana (Xia et al., 2004; Ge et al., 2005; Prasad et al., 2009; Huang et al., 2013; Guo et al., 2016; Gao et al., 2017). Given that immune incompatibility between the protease Rcr3 from domesticated tomatoes and R protein Cf-2 from wild tomatoes are known, it will be interesting to see if the rice AP important for setting up the reproductive barrier would engage an immune system incompatibility under BDM epistasis. Breeding history and already existing indica-japonica rice hybrids that overcame the AP-mediated barrier would provide a hint on the involvement of immune components as well as the possibility of APs as host targets that an NLR monitors.
Reverse Breeding Application to Hybrid Necrosis and Heterosis
To improve the combining ability of antagonistic traits such as growth and immunity, understanding genetic architectures underlying heterosis and hybrid necrosis is the first step. With the advances in crop genomics in recent years, it is becoming feasible to map responsible loci associated with such traits and retrieve relevant SNP sets, which will guide the design of a crop based on genomic information. However, challenges still remain to precisely and efficiently incorporate new traits into elite varieties. Given that fine-mapping resolution in crops still span a region including multiple loci and that immune genes particularly tend to form a cluster in the genome, substituting a responsible chromosome segment can be a useful genetic solution both to detect causal loci in bulk and to incorporate allele replacement when causal genes had not been cloned (Balakrishnan et al., 2019). Reverse breeding is an effective way to fix heterosis from hybrids expressing a suit of beneficial traits (Dirks et al., 2009). It first decomposes traits by generating numerous homozygous lines from a given heterozygous plant, from which desirable phenotypic traits can be selected in combination or in part. The crossing of complementing homozygous lines for the desired traits yields a hybrid which resembles fully or partially the genome of the starting heterozygous (Wijnker et al., 2012; Calvo-Baltanás et al., 2020; Figure 4A).
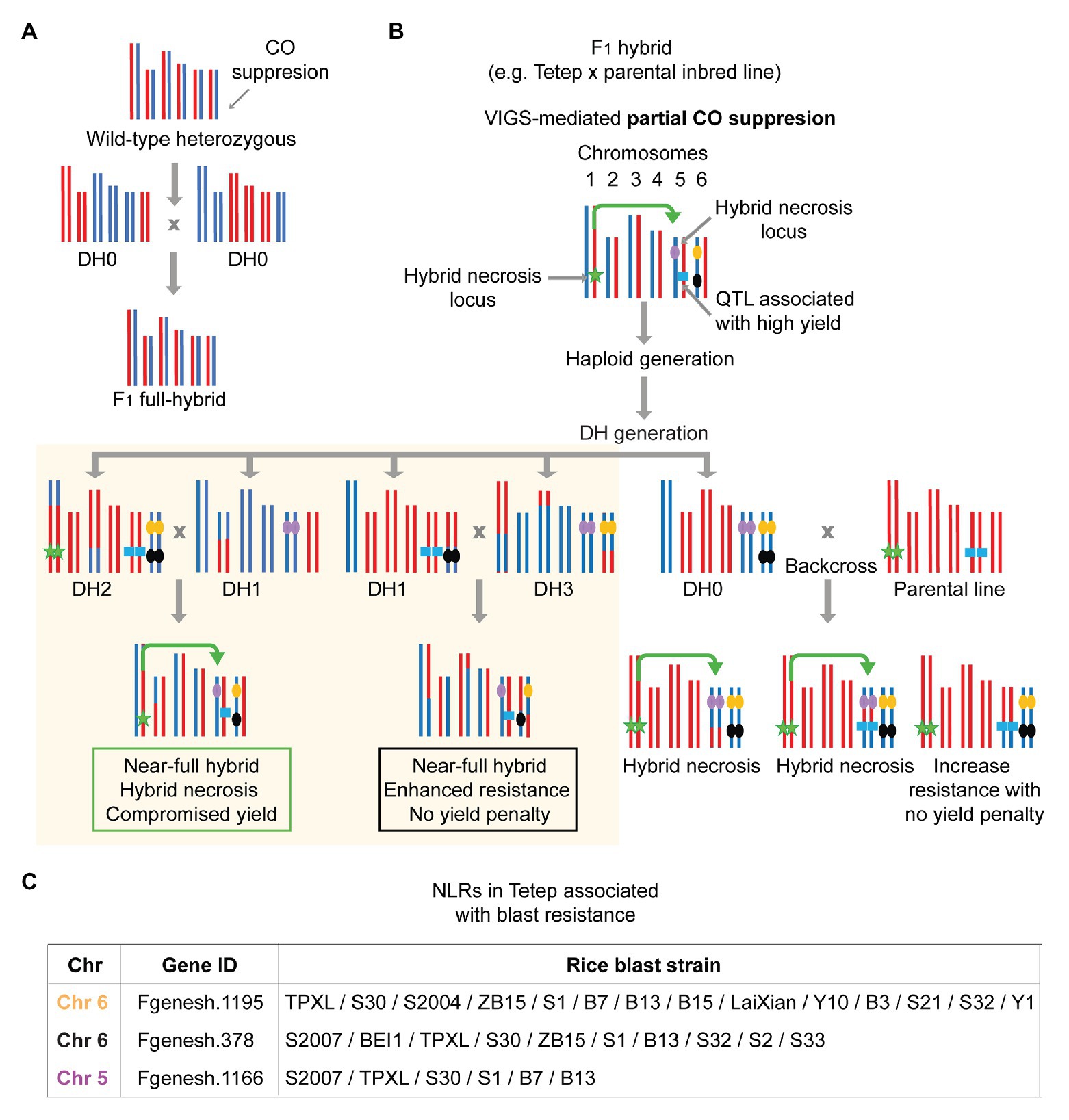
Figure 4. Reverse breeding through virus-induced gene silencing (VIGS)-mediated partial crossover suppression in a rice hybrid. (A) Reverse breeding allows the recreation of a heterozygous plant as an F1 hybrid through the generation of genetic complementing parental lines. (B) A schematic reverse breeding strategy in rice. Only the first six chromosomes of a putative rice hybrid between Tetep (blue) and a hypothetical parental inbred line (red) are presented for simplicity. Three NLRs found in the Tetep genome are represented with purple, yellow, and black circles in chromosomes 5 and 6. The quantitative trait locus (QTL) associated with high yield is represented by the light blue square in chromosome 5. Hypothetical genetic interaction between two loci (green star and purple circle) results in hybrid necrosis, thus affecting yield. Reverse breeding based on partial suppression of meiotic recombination will yield plants producing gametes carrying chromosomes with 0, 1, 2, or 3 crossover (COs) which will be grown into DH0, DH1, DH2, and DH3, respectively. Different DHs can be crossed to produce near-full hybrids. The homozygous regions found in one near-full hybrid eliminate one of the hybrid necrosis loci (green star) while retaining the QTL for increased yield and three NLRs from Tetep, hence allowing the generation of a resistant and high-yielding hybrid. The DH0 lines [chromosome-substitution lines (CSLs)] can be used as parental donors to introduce R genes in a recipient background. Note that the lines obtained in this scheme can be instrumental to map hybrid necrosis loci (C). List of NLRs in the Tetep represented in (B) and the list of pathogens they confer resistance against (Wang et al., 2019c). The font color corresponds to the NLR represented in each chromosome in (B).
The technical application of reverse breeding demands two conditions: (1) the downregulation of meiotic recombination to preserve the two parental haplotypes that were combined in the starting hybrid and (2) the regeneration of the resulting gametes as a double-haploid (hereafter DH) population to obtain homozygous lines (Dirks et al., 2009; Wijnker et al., 2012; Figure 4). To date, reverse breeding has been shown in the model organism A. thaliana through complete and partial crossover (hereafter CO) suppression by silencing genes essential for meiotic recombination (Wijnker et al., 2012; Calvo-Baltanás et al., 2020). In the latest design, a meiotic gene required for about 83–87% of the total number of COs, MSH5 (MUT-S HOMOLOG 5; Higgins et al., 2004, 2008; Lu et al., 2008), was silenced in a wild-type hybrid using virus-induced gene silencing (VIGS; Calvo-Baltanás et al., 2020). The silencing of MSH5 resulted in non-recombinant and low-recombinant chromosomes segregating to gametes. These gametes are later grown into DH lines which show variable recombination rates, from zero COs (DH0) to three COs (thus generating DH1, DH2, or DH3; Figure 4B). If gametes carrying non-recombinant chromosomes are grown into DHs (DH0), chromosome-substitution lines (CSLs) will be obtained. The combination of two fully complementing CSLs will reconstitute the initial heterozygous genotype (Figure 4A). Gametes carrying low-recombinant chromosomes, however, can also be of use for reverse breeding. The combination of DH1 and DH2 lines, with one and two CO, respectively, will generate near-full hybrids (Figure 4B). Near-full hybrids largely retain the heterozygosity of the full hybrid with the exception of certain homozygous regions (Figure 4B). These hybrids, therefore, can be used to experimentally assess the effect that such homozygous regions have on hybrid performance (Calvo-Baltanás et al., 2020).
Pyramiding R genes in elite-hybrids is challenging, especially when it entails R gene cloning, which is particularly complicated due to massive structural variation often associated with the encoding loci (Jiao and Schneeberger, 2020). Alternatively, a backcrossing scheme between a donor and recipient line can facilitate the introduction of R genes in breeding lines. However, this is a time-consuming process and unknown deleterious combinations can lead to hybrid necrosis when parents containing newly assembled R genes sets are used to produce hybrids. To increase the efficiency of this process, one can use reverse breeding as an alternative technique to NLR cloning or to assist in backcrossing schemes (Figure 4B). In an ideal scenario, the genome of the initial (wild-type) heterozygote for a reverse breeding experiment will contain loci associated with hybrid necrosis, heterosis, and resistance. Reverse breeding based on partial CO suppression applied to this heterozygote will deliver DHs that will segregate for the desired number of R genes, heterotic loci and hybrid necrosis loci (Figure 4B). The combination of these lines will then produce hybrids with different genetic composition. Because DHs are grown from gametes that contain recombinant and non-recombinant chromosomes, depending on the combination of DHs used, some regions will become homozygous in the near-full hybrids (Figure 4B). In the event that such homozygous regions are not associated with yield, the expression of this trait should not be compromised in near-full hybrids. Some works already showed that the accumulation of several positive alleles was responsible for the heterotic traits in rice and for this reason, the reconstitution of the full hybrid genotype would not be essential to obtain elite heterozygous lines from a reverse breeding experiment (Huang et al., 2015, 2016). In addition, homozygous regions present in the near-full hybrids may be necessary to eliminate hybrid necrosis loci while retaining all the other desirable traits (i.e., R genes and heterotic loci; Figure 4B).
The use of reverse breeding offspring has further advantages. DH0s are also CSLs, a type of mapping population useful to dissect epistasis (Nadeau et al., 2000; Spiezio et al., 2012; Ziolkowski et al., 2017; Wijnen et al., 2018). CSLs can also be used as donor lines for single or entire sets of R genes; crossing CSLs to an existing breeding line allows the sequential introduction of desirable R genes into that background (Figure 4B). Because only specific chromosomes segregate, the associated genotyping effort to identify the lines with the desired introgression is largely reduced as compared to traditional backcrossing schemes. Moreover, if CSLs are crossed to an inbred parental line, in later generations, individuals segregating for specific loci will be obtained (Figure 4B). Such lines may contain a single chromosome segment from a donor parent introduced to a second, recurrent background. These genetic stocks are valuable tools for crop improvement, as they allow to map QTLs, causal genes, and gene interactions (Balakrishnan et al., 2019). Therefore, the rapid generation of CSLs through reverse breeding could be instrumental to facilitate the study of the genetic mechanisms of hybrid necrosis in rice.
Practical Application of Reverse Breeding in Rice
For the application of reverse breeding in rice, silencing of genes essential for CO formation shall be achieved in hybrids. To this end, different approaches such as microRNA, RNAi, or VIGS can be used. There are, however, benefits of using VIGS over other silencing methods that require stable transformation. First, for a gene to be silenced by RNAi or microRNAs in the hybrid, at least one of the parental lines needs to be previously transformed with dominantly acting transgenes. Consequently, inbred lines for that particular hybrid need to be available. VIGS, however, allows direct silencing of the target in the chosen hybrid, even if this is a heterozygote from outcrossing populations. Therefore, VIGS represents a convenient, broad, rapid method over the use of stable transgenes. In rice, the rice tungro bacilliform virus (RTBV)-VIGS system has been shown to reduce gene expression by 80% (Kant and Dasgupta, 2017). The silencing efficiency of meiotic genes, however, is yet to be assessed using this system. The most obvious target for VIGS in a reverse breeding experiment would be OsMSH5/OsMSH4 and/or other proteins involved in class I CO formation, which would lead to a comparable decrease in CO numbers as for the A. thaliana mutants defective in this CO pathway (Luo et al., 2013; Zhang et al., 2014, 2019; Wang et al., 2016; Calvo-Baltanás et al., 2020).
The effect of CO reduction on gamete viability can be calculated as (1/2)x, where “x” represents the species’ chromosome number. In rice, the average number of COs in wild-type meiosis has been estimated to be between 20.1 and 33.9 (Si et al., 2015; Zhang et al., 2019). The estimated 83% reduction in CO formation when class I CO formation is suppressed and considering 33.9 or 20.1 COs would result in about six or three residual COs per meiosis, respectively. While complete CO suppression would yield (1/2)12 × 100% = 0.02% of viable gametes, in the event that either six or three residual COs are present, and assuming the occurrence of one CO per chromosome pair this frequency will vary greatly, which will equal to (1/2)12–6 × 100% = 1.6% or (1/2)12–3 × 100% = 0.2%. Therefore, partial COs suppression represents an 80 to 10-fold increase in gamete viability over complete CO suppression, depending on the residual number of COs present. In addition, it is important to consider the number of gametes produced by a single plant, as this will affect the number of viable gametes obtained from a reverse breeding experiment in rice. The number of pollen grains produced by rice plants is rather variable among varieties, and it is influenced by anther-length and environmental conditions such as temperature (Fukushima et al., 2017). Under optimal growth conditions, each anther may contain 1,000–2,000 pollen grains (Fukushima et al., 2017). If COs are reduced by 83% and six or three residual COs are present, each anther will produce between 16 and 32 or between 2 and 4 viable pollen grains, respectively. Such pollen viability rate a priori may seem low, but it would certainly enable the recovery of reverse breeding offspring if several silenced anthers from each plant are used for DH regeneration.
The last step requires haploid and/or DH technology to be established. Although haploid-induction efficiency and DH regeneration in rice has long remained largely genotype-dependent, these constraints have been greatly overcome in recent years (Bishnoi et al., 2000; Maharani et al., 2020). Indeed, different anther culture techniques allow DH-recovery frequency that ranges from 30 to 60% (Mishra and Rao, 2016; Ren et al., 2017; Sarao and Gosal, 2018). Interestingly, one of the latest protocols available for anther-culture using an elite indica rice hybrid even reports a regeneration of exclusively DHs from callus (Naik et al., 2017). Such frequencies integrated in a reverse breeding scheme implies that useful DHs and near-full hybrids can be obtained in a maximum of two generations from the starting hybrid (Figure 4B).
Conclusion
In this review, we put forward our proposition that hybrid necrosis resulting from immune incompatibility would function as a potential opposing force to the expression heterosis in hybrids. Our reasoning bases on recent molecular and genetic findings on hybrid necrosis in plant species covering both natural and selectively bred germplasms. Autoimmunity is a major mechanism underlying hybrid necrosis arising from a new combination of highly diversified immune components from different parental origins, which obligatorily results in compromise in growth and yield. Thus, hybrid necrosis illustrates an extreme degree of trade-off manifestation between growth and immunity. In the plant performance equilibrium model that we propose, the shifted equilibrium to the extremes is expressed as either hybrid necrosis or heterosis. Although the degree of contribution made from anti-hybrid necrosis to determining heterosis is yet to be examined, yield penalty associated with enhanced resistance observed throughout breeding history, including hybrid breeding in rice, clearly points to the link between heterosis and disease resistance traits. We encourage rice researchers to revisit the cases of underperforming hybrids in the breeding panel under the new concept of hybrid necrosis as an opposing force to heterosis. Despite the seemingly low breeding interests, such underperformance, when addressed under epistatic interactions and environmental variables affecting performances, might reveal an underestimated contribution of hybrid necrosis to restrict the full manifestation of heterosis. The findings will not only shed light on guided breeding strategies in the post-genome era but also greatly inform us of evolutionary processes shaping up an immune system including the valuable R gene repertoire. The more we understand the downside of hybrid performance, the better could we combine beneficial traits of yield and disease resistance. Valuable rice germplasms bred throughout history await a new wave of immune-centered breeding for heterosis.
Author Contributions
VCB, JW, and EC formulated concepts and wrote the manuscript. All authors contributed to the article and approved the submitted version.
Funding
The work was supported by Academic Research Fund from Singapore Ministry of Education (MOE 2019-T2-1-134: MOE Tier1 funds R-154-000-B20-114 and R-154-000-B33-114) and Intramural Research Fund from National University of Singapore (R-154-000-A79-133:startup fund for EC).
Conflict of Interest
The authors declare that the research was conducted in the absence of any commercial or financial relationships that could be construed as a potential conflict of interest.
Acknowledgments
We are grateful for reviewers for constructive comments. We also thank Wei Yuan Cher for the critical reading and comments on this manuscript. We also acknowledge that the term “anti-hybrid necrosis” was coined by Detlef Weigel.
References
Adachi, H., Derevnina, L., and Kamoun, S. (2019). NLR singletons, pairs, and networks: evolution, assembly, and regulation of the intracellular immunoreceptor circuitry of plants. Curr. Opin. Plant Biol. 50, 121–131. doi: 10.1016/j.pbi.2019.04.007
Alcázar, R., García, A. V., Kronholm, I., de Meaux, J., Koornneef, M., Parker, J. E., et al. (2010). Natural variation at Strubbelig Receptor Kinase 3 drives immune-triggered incompatibilities between Arabidopsis thaliana accessions. Nat. Genet. 42, 1135–1139. doi: 10.1038/ng.704
Alcázar, R., García, A. V., Parker, J. E., and Reymond, M. (2009). Incremental steps toward incompatibility revealed by Arabidopsis epistatic interactions modulating salicylic acid pathway activation. Proc. Natl. Acad. Sci. U. S. A. 106, 334–339. doi: 10.1073/pnas.0811734106
Alcázar, R., von Reth, M., Bautor, J., Chae, E., Weigel, D., Koornneef, M., et al. (2014). Analysis of a plant complex resistance gene locus underlying immune-related hybrid incompatibility and its occurrence in nature. PLoS Genet. 10:e1004848. doi: 10.1371/journal.pgen.1004848
Ali, G. S., Reddy, V. S., Lindgren, P. B., Jakobek, J. L., and Reddy, A. S. N. (2003). Differential expression of genes encoding calmodulin-binding proteins in response to bacterial pathogens and inducers of defense responses. Plant Mol. Biol. 51, 803–815. doi: 10.1023/A:1023001403794
An, C., and Mou, Z. (2011). Salicylic acid and its function in plant immunity. J. Integr. Plant Biol. 53, 412–428. doi: 10.1111/j.1744-7909.2011.01043.x
Azzam, O., and Chancellor, T. C. B. (2002). The biology, epidemiology, and management of rice tungro disease in Asia. Plant Dis. 86, 88–100. doi: 10.1094/PDIS.2002.86.2.88
Balakrishnan, D., Surapaneni, M., Mesapogu, S., and Neelamraju, S. (2019). Development and use of chromosome segment substitution lines as a genetic resource for crop improvement. Theor. Appl. Genet. 132, 1–25. doi: 10.1007/s00122-018-3219-y
Barragan, A. C., Collenberg, M., Wang, J., Lee, R. R. Q., Cher, W. Y., Rabanal, F. A., et al. (2020). A truncated singleton NLR causes hybrid necrosis in Arabidopsis thaliana. Mol. Biol. Evol. doi: 10.1093/molbev/msaa245 [Epub ahead of print]
Barragan, C. A., Wu, R., Kim, S. -T., Xi, W., Habring, A., Hagmann, J., et al. (2019). RPW8/HR repeats control NLR activation in Arabidopsis thaliana. PLoS Genet. 15:e1008313. doi: 10.1371/journal.pgen.1008313
Bernoux, M., Burdett, H., Williams, S. J., Zhang, X., Chen, C., Newell, K., et al. (2016). Comparative analysis of the flax immune receptors l6 and l7 suggests an equilibrium-based switch activation model. Plant Cell 28, 146–159. doi: 10.1105/tpc.15.00303
Bikard, D., Patel, D., Le Metté, C., Giorgi, V., Camilleri, C., Bennett, M. J., et al. (2009). Divergent evolution of duplicate genes leads to genetic incompatibilities within A. thaliana. Science 323, 623–626. doi: 10.1126/science.1165917
Bisgrove, S. R., Simonich, M. T., Smith, N. M., Sattler, A., and Innes, R. W. (1994). A disease resistance gene in Arabidopsis with specificity for two different pathogen avirulence genes. Plant Cell 6, 927–933. doi: 10.1105/tpc.6.7.927
Bishnoi, U., Jain, R. K., Rohilla, J. S., Chowdhury, V. K., Gupta, K. R., and Chowdhury, J. B. (2000). Anther culture of recalcitrant indica × Basmati rice hybrids. Euphytica 114, 93–101. doi: 10.1023/A:1003915331143
Boller, T., and Felix, G. (2009). A renaissance of elicitors: perception of microbe-associated molecular patterns and danger signals by pattern-recognition receptors. Annu. Rev. Plant Biol. 60, 379–406. doi: 10.1146/annurev.arplant.57.032905.105346
Bomblies, K. (2009). Too much of a good thing? Hybrid necrosis as a by-product of plant immune system diversification. Botany 87, 1013–1022. doi: 10.1139/B09-072
Bomblies, K., Lempe, J., Epple, P., Warthmann, N., Lanz, C., Dangl, J. L., et al. (2007). Autoimmune response as a mechanism for a Dobzhansky-Muller-type incompatibility syndrome in plants. PLoS Biol. 5:e236. doi: 10.1371/journal.pbio.0050236
Bomblies, K., and Weigel, D. (2007). Hybrid necrosis: autoimmunity as a potential gene-flow barrier in plant species. Nat. Rev. Genet. 8, 382–393. doi: 10.1038/nrg2082
Bonardi, V., Tang, S., Stallmann, A., Roberts, M., Cherkis, K., and Dangl, J. L. (2011). Expanded functions for a family of plant intracellular immune receptors beyond specific recognition of pathogen effectors. Proc. Natl. Acad. Sci. U. S. A. 108:16463. doi: 10.1073/pnas.1113726108
Borrelli, G. M., Mazzucotelli, E., Marone, D., Crosatti, C., Michelotti, V., Valè, G., et al. (2018). Regulation and evolution of NLR genes: a close interconnection for plant immunity. Int. J. Mol. Sci. 19:1662. doi: 10.3390/ijms19061662
Boutrot, F., and Zipfel, C. (2017). Function, discovery, and exploitation of plant pattern recognition receptors for broad-spectrum disease resistance. Annu. Rev. Phytopathol. 55, 257–286. doi: 10.1146/annurev-phyto-080614-120106
Bottrell, D. G., and Schoenly, K. G. (2012). Resurrecting the ghost of green revolutions past: the brown planthopper as a recurring threat to high-yielding rice production in tropical Asia. J. Asia Pac. Entomol. 15, 122–140. doi: 10.1016/j.aspen.2011.09.004
Brodersen, P., Petersen, M., Pike, H. M., Olszak, B., Skov, S., Odum, N., et al. (2002). Knockout of Arabidopsis accelerated-cell-death11 encoding a sphingosine transfer protein causes activation of programmed cell death and defense. Genes Dev. 16, 490–502. doi: 10.1101/gad.218202
Calvo-Baltanás, V., Wijnen, C. L., Yang, C., Lukhovitskaya, N., Snoo, C. B.d., Hohenwarter, L., et al. (2020). Meiotic crossover reduction by virus-induced gene silencing enables the efficient generation of chromosome substitution lines and reverse breeding in Arabidopsis thaliana. Plant J. 104, 1437–1452. doi: 10.1111/tpj.14990
Castel, B., Ngou, P. -M., Cevik, V., Redkar, A., Kim, D. -S., Yang, Y., et al. (2019). Diverse NLR immune receptors activate defence via the RPW8-NLR NRG1. New Phytol. 222, 966–980. doi: 10.1111/nph.15659
Cesari, S. (2018). Multiple strategies for pathogen perception by plant immune receptors. New Phytol. 219, 17–24. doi: 10.1111/nph.14877
Cesari, S., Bernoux, M., Moncuquet, P., Kroj, T., and Dodds, P. N. (2014a). A novel conserved mechanism for plant NLR protein pairs: the “integrated decoy” hypothesis. Front. Plant Sci. 5:606. doi: 10.3389/fpls.2014.00606
Cesari, S., Kanzaki, H., Fujiwara, T., Bernoux, M., Chalvon, V., Kawano, Y., et al. (2014b). The NB-LRR proteins RGA4 and RGA5 interact functionally and physically to confer disease resistance. EMBO J. 33, 1941–1959. doi: 10.15252/embj.201487923
Cesari, S., and Kroj, T. (2017). Transposon-mediated NLR exile to the pollen allows rice blast resistance without yield penalty. Mol. Plant 10, 665–667. doi: 10.1016/j.molp.2017.04.005
Cesari, S., Thilliez, G., Ribot, C., Chalvon, V., Michel, C., Jauneau, A., et al. (2013). The rice resistance protein pair RGA4/RGA5 recognizes the Magnaporthe oryzae effectors AVR-Pia and AVR1-CO39 by direct binding. Plant Cell 25, 1463–1481. doi: 10.1105/tpc.112.107201
Chae, E., Bomblies, K., Kim, S. -T., Karelina, D., Zaidem, M., Ossowski, S., et al. (2014). Species-wide genetic incompatibility analysis identifies immune genes as hot spots of deleterious epistasis. Cell 159, 1341–1351. doi: 10.1016/j.cell.2014.10.049
Chae, E., Tran, D. T., and Weigel, D. (2016). Cooperation and conflict in the plant immune system. PLoS Pathog. 12:e1005452. doi: 10.1371/journal.ppat.1005452
Chen, C., Chen, H., Lin, Y. S., Shen, J. B., Shan, J. X., Qi, P., et al. (2014). A two-locus interaction causes interspecific hybrid weakness in rice. Nat. Commun. 5:3357. doi: 10.1038/ncomms4357
Chen, C., Chen, H., Shan, J. X., Zhu, M. Z., Shi, M., Gao, J. P., et al. (2013). Genetic and physiological analysis of a novel type of interspecific hybrid weakness in rice. Mol. Plant 6, 716–728. doi: 10.1093/mp/sss146
Chen, J., Ding, J., Ouyang, Y., Du, H., Yang, J., Cheng, K., et al. (2008). A triallelic system of S5 is a major regulator of the reproductive barrier and compatibility of indica–japonica hybrids in rice. Proc. Natl. Acad. Sci. U. S. A. 105:11436. doi: 10.1073/pnas.0804761105
Chen, X., and Ronald, P. C. (2011). Innate immunity in rice. Trends Plant Sci. 16, 451–459. doi: 10.1016/j.tplants.2011.04.003
Chen, Q., Zeng, G., Hao, M., Jiang, H., and Xiao, Y. (2020). Improvement of rice blast and brown planthopper resistance of PTGMS line C815S in two-line hybrid rice through marker-assisted selection. Mol. Breed. 40:21. doi: 10.1007/s11032-020-1098-9
Christopoulou, M., McHale, L. K., Kozik, A., Reyes-Chin Wo, S., Wroblewski, T., and Michelmore, R. W. (2015). Dissection of two complex clusters of resistance genes in lettuce (Lactuca sativa). Mol. Plant-Microbe Interact. 28, 751–765. doi: 10.1094/MPMI-06-14-0175-R
Chu, Y. -E., and Oka, H. -I. (1970). The genetic basis of crossing barriers between Oryza perennis subsp. Barthii and its related taxa. Evolution 24, 135–144. doi: 10.1111/j.1558-5646.1970.tb01746.x
Clouse, S. D. (2011). Brassinosteroid signal transduction: from receptor kinase activation to transcriptional networks regulating plant development. Plant Cell 23, 1219–1230. doi: 10.1105/tpc.111.084475
Collier, S. M., Hamel, L. -P., and Moffett, P. (2011). Cell death mediated by the N-terminal domains of a unique and highly conserved class of NB-LRR protein. Mol. Plant-Microbe Interact. 24, 918–931. doi: 10.1094/MPMI-03-11-0050
Collier, S. M., and Moffett, P. (2009). NB-LRRs work a “bait and switch” on pathogens. Trends Plant Sci. 14, 521–529. doi: 10.1016/j.tplants.2009.08.001
Cortijo, S., Wardenaar, R., Colomé-Tatché, M., Gilly, A., Etcheverry, M., Labadie, K., et al. (2014). Mapping the epigenetic basis of complex traits. Science 343, 1145–1148. doi: 10.1126/science.1248127
Cui, H., Tsuda, K., and Parker, J. E. (2015). Effector-triggered immunity: from pathogen perception to robust defense. Annu. Rev. Plant Biol. 66, 487–511. doi: 10.1146/annurev-arplant-050213-040012
Dangl, J. L., and Jones, J. D. G. (2001). Plant pathogens and integrated defence responses to infection. Nature 411, 826–833. doi: 10.1038/35081161
Dangl, J. L., Ritter, C., Gibbon, M. J., Luis, A. J. M., Wood, J. R., Goss, S., et al. (1992). Functional homologs of the Arabidopsis RPM1 disease resistance gene in bean and pea. Plant Cell 4, 1359–1369. doi: 10.2307/3869508.a
De Vleesschauwer, D., Xu, J., and Höfte, M. (2014). Making sense of hormone-mediated defense networking: from rice to Arabidopsis. Front. Plant Sci. 5:611. doi: 10.3389/fpls.2014.00611
Deng, J., Fang, L., Zhu, X., Zhou, B., and Zhang, T. (2019). A CC-NBS-LRR gene induces hybrid lethality in cotton. J. Exp. Bot. 70, 5145–5156. doi: 10.1093/jxb/erz312
Deng, Y., Zhai, K., Xie, Z., Yang, D., Zhu, X., Liu, J., et al. (2017). Epigenetic regulation of antagonistic receptors confers rice blast resistance with yield balance. Science 355, 962–965. doi: 10.1126/science.aai8898
Devi, S. J. S. R., Singh, K., Umakanth, B., Vishalakshi, B., Renuka, P., Sudhakar, K. V., et al. (2015). Development and identification of novel rice blast resistant sources and their characterization using molecular markers. Rice Sci. 22, 300–308. doi: 10.1016/j.rsci.2015.11.002
Dirks, R., Van Dun, K., De Snoo, C. B., Van Den Berg, M., Lelivelt, C. L. C., Voermans, W., et al. (2009). Reverse breeding: a novel breeding approach based on engineered meiosis. Plant Biotechnol. J. 7, 837–845. doi: 10.1111/j.1467-7652.2009.00450.x
Dou, D., and Zhou, J. M. (2012). Phytopathogen effectors subverting host immunity: different foes, similar battleground. Cell Host Microbe 12, 484–495. doi: 10.1016/j.chom.2012.09.003
Emenecker, R. J., and Strader, L. C. (2020). Auxin-abscisic acid interactions in plant growth and development. Biomolecules 10:281. doi: 10.3390/biom10020281
Fisher, R. A. (1919). The correlation between relatives on the supposition of mendelian inheritance. Trans. R. Soc. Edinb. 52, 399–433. doi: 10.1017/S0080456800012163
Flor, H. H. (1971). Current status of the gene-for-gene concept. Annu. Rev. Phytopathol. 9, 275–296. doi: 10.1146/annurev.py.09.090171.001423
Fu, C. -Y., Wang, F., Sun, B. -R., Liu, W. -G., Li, J. -H., Deng, R. -F., et al. (2013). Genetic and cytological analysis of a novel type of low temperature-dependent intrasubspecific hybrid weakness in rice. PLoS One 8:e73886. doi: 10.1371/journal.pone.0073886
Fujimoto, R., Uezono, K., Ishikura, S., Osabe, K., Peacock, W. J., and Dennis, E. S. (2018). Recent research on the mechanism of heterosis is important for crop and vegetable breeding systems. Breed. Sci. 68, 145–158. doi: 10.1270/jsbbs.17155
Fukuoka, S., Namai, H., and Okuno, K. (1998). RFLP mapping of the genes controlling hybrid breakdown in rice (Oryza sativa L.). Theor. Appl. Genet. 97, 446–449. doi: 10.1007/s001220050915
Fukushima, A., Hayashi, T., Ohta, H., Kaji, R., Yokogami, N., and Tsuda, N. (2017). Effects of the number of pollen grains on cold tolerance at the booting stage in rice lines with QTLs for cold tolerance. Plant Prod. Sci. 20, 149–155. doi: 10.1080/1343943X.2016.1245103
Gao, L., Roux, F., and Bergelson, J. (2009). Quantitative fitness effects of infection in a gene-for-gene system. New Phytol. 184, 485–494. doi: 10.1111/j.1469-8137.2009.02959.x
Gao, H., Zhang, Y., Wang, W., Zhao, K., Liu, C., Bai, L., et al. (2017). Two membrane-anchored aspartic proteases contribute to pollen and ovule development. Plant Physiol. 173:219. doi: 10.1104/pp.16.01719
Ge, X., Dietrich, C., Matsuno, M., Li, G., Berg, H., and Xia, Y. (2005). An Arabidopsis aspartic protease functions as an anti-cell-death component in reproduction and embryogenesis. EMBO Rep. 6, 282–288. doi: 10.1038/sj.embor.7400357
Glazebrook, J. (2005). Contrasting mechanisms of defense against biotrophic and necrotrophic pathogens. Annu. Rev. Phytopathol. 43, 205–227. doi: 10.1146/annurev.phyto.43.040204.135923
Grant, M., Godiard, L., Straube, E., Ashfield, T., Lewald, J., Sattler, A., et al. (1995). Structure of the Arabidopsis RPM1 gene enabling dual specificity disease resistance. Science 269, 843–846. doi: 10.1126/science.7638602
Guo, R., Tu, M., Wang, X., Zhao, J., Wan, R., Li, Z., et al. (2016). Ectopic expression of a grape aspartic protease gene, AP13, in Arabidopsis thaliana improves resistance to powdery mildew but increases susceptibility to Botrytis cinerea. Plant Sci. 248, 17–27. doi: 10.1016/j.plantsci.2016.04.006
He, Q., Li, D., Zhu, Y., Tan, M., Zhang, D., and Lin, X. (2006). Fine mapping of Xa2, a bacterial blight resistance gene in rice. Mol. Breed. 17, 1–6. doi: 10.1007/s11032-005-8698-2
Heil, M., and Baldwin, I. T. (2002). Fitness costs of induced resistance: emerging experimental support for a slippery concept. Trends Plant Sci. 7, 61–67. doi: 10.1016/S1360-1385(01)02186-0
Hermsen, J. G. T. (1963). The genetic basis of hybrid necrosis in wheat. Genetica 33, 245–287. doi: 10.1007/BF01725765
Higgins, J. D., Armstrong, S. J., Franklin, F. C. H., and Jones, G. H. (2004). The Arabidopsis MutS homolog AtMSH4 functions at an early step in recombination: evidence for two classes of recombination in Arabidopsis. Genes Dev. 18, 2557–2570. doi: 10.1101/gad.317504
Higgins, J. D., Vignard, J., Mercier, R., Pugh, A. G., Franklin, F. C. H., and Jones, G. H. (2008). AtMSH5 partners AtMSH4 in the class I meiotic crossover pathway in Arabidopsis thaliana, but is not required for synapsis. Plant J. 55, 28–39. doi: 10.1111/j.1365-313X.2008.03470.x
Hochholdinger, F., and Hoecker, N. (2007). Towards the molecular basis of heterosis. Trends Plant Sci. 12, 427–432. doi: 10.1016/j.tplants.2007.08.005
Holub, E. B. (2001). The arms race is ancient history in Arabidopsis, the wildflower. Nat. Rev. Genet. 2, 516–527. doi: 10.1038/35080508
Hu, J., Li, X., Wu, C., Yang, C., Hua, H., Gao, G., et al. (2012). Pyramiding and evaluation of the brown planthopper resistance genes Bph14 and Bph15 in hybrid rice. Mol. Breed. 29, 61–69. doi: 10.1007/s11032-010-9526-x
Hua, J., Grisafi, P., Cheng, S. H., and Fink, G. R. (2001). Plant growth homeostasis is controlled by the Arabidopsis BON1 and BAP1 genes. Genes Dev. 15, 2263–2272. doi: 10.1101/gad.918101
Hua, L., Wu, J., Chen, C., Wu, W., He, X., Lin, F., et al. (2012). The isolation of Pi1, an allele at the Pik locus which confers broad spectrum resistance to rice blast. Theor. Appl. Genet. 125, 1047–1055. doi: 10.1007/s00122-012-1894-7
Huang, X., Yang, S., Gong, J., Zhao, Y., Feng, Q., Gong, H., et al. (2015). Genomic analysis of hybrid rice varieties reveals numerous superior alleles that contribute to heterosis. Nat. Commun. 6:6258. doi: 10.1038/ncomms7258
Huang, X., Yang, S., Gong, J., Zhao, Q., Feng, Q., Zhan, Q., et al. (2016). Genomic architecture of heterosis for yield traits in rice. Nature 537, 629–633. doi: 10.1038/nature19760
Huang, J., Zhao, X., Cheng, K., Jiang, Y., Ouyang, Y., Xu, C., et al. (2013). OsAP65, a rice aspartic protease, is essential for male fertility and plays a role in pollen germination and pollen tube growth. J. Exp. Bot. 64, 3351–3360. doi: 10.1093/jxb/ert173
Huh, S. U., Cevik, V., Ding, P., Duxbury, Z., Ma, Y., Tomlinson, L., et al. (2017). Protein-protein interactions in the RPS4/RRS1 immune receptor complex. PLoS Pathog. 13:e1006376. doi: 10.1371/journal.ppat.1006376
Huot, B., Yao, J., Montgomery, B. L., and He, S. Y. (2014). Growth-defense tradeoffs in plants: a balancing act to optimize fitness. Mol. Plant 7, 1267–1287. doi: 10.1093/mp/ssu049
Hurni, S., Brunner, S., Stirnweis, D., Herren, G., Peditto, D., McIntosh, R. A., et al. (2014). The powdery mildew resistance gene Pm8 derived from rye is suppressed by its wheat ortholog Pm3. Plant J. 79, 904–913. doi: 10.1111/tpj.12593
Ichitani, K., Namigoshi, K., Sato, M., Taura, S., Aoki, M., Matsumoto, Y., et al. (2007). Fine mapping and allelic dosage effect of Hwc1, a complementary hybrid weakness gene in rice. Theor. Appl. Genet. 114, 1407–1415. doi: 10.1007/s00122-007-0526-0
Ichitani, K., Takemoto, Y., Iiyama, K., Taura, S., and Sato, M. (2012). Chromosomal location of HCA1 and HCA2 hybrid chlorosis genes in rice. Int. J. Plant Genomics 2012:649081. doi: 10.1155/2012/649081
Ichitani, K., Taura, S., Tezuka, T., Okiyama, Y., and Kuboyama, T. (2011). Chromosomal location of HWA1 and HWA2, complementary hybrid weakness genes in rice. Rice 4, 29–38. doi: 10.1007/s12284-011-9062-2
Ilyas, M., Horger, A. C., Bozkurt, T. O., van den Burg, H. A., Kaschani, F., Kaiser, M., et al. (2015). Functional divergence of two secreted immune proteases of tomato. Curr. Biol. 25, 2300–2306. doi: 10.1016/j.cub.2015.07.030
Innes, R. W., Bisgrove, S. R., Smith, N. M., Bent, A. F., Staskawicz, B. J., and Liu, Y. -C. (1993). Identification of a disease resistance locus in Arabidopsis that is functionally homologous to the RPG1 locus of soybean. Plant J. 4, 813–820. doi: 10.1046/j.1365-313X.1993.04050813.x
Jeuken, M. J. W., Zhang, N. W., McHale, L. K., Pelgrom, K., den Boer, E., Lindhout, P., et al. (2009). Rin4 causes hybrid necrosis and race-specific resistance in an interspecific lettuce hybrid. Plant Cell 21, 3368–3378. doi: 10.1105/tpc.109.070334
Ji, Q., Zhang, M., Lu, J., Wang, H., Lin, B., Liu, Q., et al. (2012). Molecular basis underlying the S5-dependent reproductive isolation and compatibility of indica/japonica rice hybrids. Plant Physiol. 158:1319. doi: 10.1104/pp.111.189571
Jiao, W. B., and Schneeberger, K. (2020). Chromosome-level assemblies of multiple Arabidopsis genomes reveal hotspots of rearrangements with altered evolutionary dynamics. Nat. Commun. 11:989. doi: 10.1038/s41467-020-14779-y
Kant, R., and Dasgupta, I. (2017). Phenotyping of VIGS-mediated gene silencing in rice using a vector derived from a DNA virus. Plant Cell Rep. 36, 1159–1170. doi: 10.1007/s00299-017-2156-6
Kapos, P., Devendrakumar, K. T., and Li, X. (2019). Plant NLRs: from discovery to application. Plant Sci. 279, 3–18. doi: 10.1016/j.plantsci.2018.03.010
Karasov, T. L., Almario, J., Friedemann, C., Ding, W., Giolai, M., Heavens, D., et al. (2018). Arabidopsis thaliana and pseudomonas pathogens exhibit stable associations over evolutionary timescales. Cell Host Microbe 24, 168.e164–179.e164. doi: 10.1016/j.chom.2018.06.011
Karasov, T. L., Chae, E., Herman, J. J., and Bergelson, J. (2017). Mechanisms to mitigate the trade-off between growth and defense. Plant Cell 29:666. doi: 10.1105/tpc.16.00931
Karasov, T. L., Kniskern, J. M., Gao, L., DeYoung, B. J., Ding, J., Dubiella, U., et al. (2014). The long-term maintenance of a resistance polymorphism through diffuse interactions. Nature 512, 436–440. doi: 10.1038/nature13439
Kim, M. -S., Ouk, S., Jung, K. -H., Song, Y., Van Trang, L., Yang, J. -Y., et al. (2019). Breeding hybrid rice with genes resistant to diseases and insects using marker-assisted selection and evaluation of biological assay. Plant Breed. Biotechnol. 7, 272–286. doi: 10.9787/PBB.2019.7.3.272
Kostoff, D. (1930). Ontogeny, genetics, and cytology of Nicotiana hybrids. Genetica 12, 33–139. doi: 10.1007/BF01508072
Kottapalli, K. R., Lakshmi Narasu, M., and Jena, K. K. (2010). Effective strategy for pyramiding three bacterial blight resistance genes into fine grain rice cultivar, Samba Mahsuri, using sequence tagged site markers. Biotechnol. Lett. 32, 989–996. doi: 10.1007/s10529-010-0249-1
Kourelis, J., and van der Hoorn, R. A. L. (2018). Defended to the nines: 25 years of resistance gene cloning identifies nine mechanisms for R protein function. Plant Cell 30, 285–299. doi: 10.1105/tpc.17.00579
Krüger, J., Thomas, C. M., Golstein, C., Dixon, M. S., Smoker, M., Tang, S., et al. (2002). A tomato cysteine protease required for Cf-2-dependent disease resistance and suppression of autonecrosis. Science 296, 744–747. doi: 10.1126/science.1069288
Kubo, T., Takashi, T., Ashikari, M., Yoshimura, A., and Kurata, N. (2016). Two tightly linked genes at the hsa1 locus cause both F1 and F2 hybrid sterility in rice. Mol. Plant 9, 221–232. doi: 10.1016/j.molp.2015.09.014
Kubo, T., and Yoshimura, A. (2002). Genetic basis of hybrid breakdown in a japonica/indica cross of rice, Oryza sativa L. Theor. Appl. Genet. 105, 906–911. doi: 10.1007/s00122-002-1059-1
Kubo, T., and Yoshimura, A. (2005). Epistasis underlying female sterility detected in hybrid breakdown in a japonica-indica cross of rice (Oryza sativa L.). Theor. Appl. Genet. 110, 346–355. doi: 10.1007/s00122-004-1846-y
Kuboyama, T., Saito, T., Matsumoto, T., Wu, J., Kanamori, H., Taura, S., et al. (2009). Fine mapping of HWC2, a complementary hybrid weakness gene, and haplotype analysis around the locus in rice. Rice 2, 93–103. doi: 10.1007/s12284-009-9026-y
Lee, R. R. Q., and Chae, E. (2020). Variation patterns of NLR clusters in Arabidopsis thaliana genomes. Plant Commun. 1:100089. doi: 10.1016/j.xplc.2020.100089
Lee, S. K., Song, M. Y., Seo, Y. S., Kim, H. K., Ko, S., Cao, P. J., et al. (2009). Rice Pi5-mediated resistance to Magnaporthe oryzae requires the presence of two coiled-coil-nucleotide-binding-leucine-rich repeat genes. Genetics 181, 1627–1638. doi: 10.1534/genetics.108.099226
Li, J., Brader, G., and Palva, E. T. (2004). The WRKY70 transcription factor: a node of convergence for jasmonate-mediated and salicylate-mediated signals in plant defense. Plant Cell 16, 319–331. doi: 10.1105/tpc.016980
Li, W., Chern, M., Yin, J., Wang, J., and Chen, X. (2019). Recent advances in broad-spectrum resistance to the rice blast disease. Curr. Opin. Plant Biol. 50, 114–120. doi: 10.1016/j.pbi.2019.03.015
Li, X., Clarke, J. D., Zhang, Y., and Dong, X. (2001). Activation of an EDS1-mediated R-gene pathway in the snc1 mutant leads to constitutive, NPR1-independent pathogen resistance. Mol. Plant-Microbe Interact. 14, 1131–1139. doi: 10.1094/MPMI.2001.14.10.1131
Li, L., Habring, A., Wang, K., and Weigel, D. (2020). Atypical resistance protein RPW8/HR triggers oligomerization of the NLR immune receptor RPP7 and autoimmunity. Cell Host Microbe 27, 405.e406–417.e406. doi: 10.1016/j.chom.2020.01.012
Li, L., Lu, K., Chen, Z., Mu, T., Hu, Z., and Li, X. (2008). Dominance, overdominance and epistasis condition the heterosis in two heterotic rice hybrids. Genetics 180, 1725–1742. doi: 10.1534/genetics.108.091942
Li, Y., Pennington, B. O., and Hua, J. (2009). Multiple R-like genes are negatively regulated by BON1 and BON3 in Arabidopsis. Mol. Plant-Microbe Interact. 22, 840–848. doi: 10.1094/MPMI-22-7-0840
Lin, Z., Qin, P., Zhang, X., Fu, C., Deng, H., Fu, X., et al. (2020). Divergent selection and genetic introgression shape the genome landscape of heterosis in hybrid rice. Proc. Natl. Acad. Sci. U. S. A. 117:4623. doi: 10.1073/pnas.1919086117
Liu, X. Q., Bai, X. Q., Qian, Q., Wang, X. J., Chen, M. S., and Chu, C. C. (2005). OsWRKY03, a rice transcriptional activator that functions in defense signaling pathway upstream of OsNPR1. Cell Res. 15, 593–603. doi: 10.1038/sj.cr.7290329
Liu, H., Dong, S., Gu, F., Liu, W., Yang, G., Huang, M., et al. (2017). NBS-LRR protein Pik-H4 interacts with OsBIHD1 to balance rice blast resistance and growth by coordinating ethylene-brassinosteroid pathway. Front. Plant Sci. 8:127. doi: 10.3389/fpls.2017.00127
Liu, X., Inoue, H., Hayashi, N., Jiang, C. -J., and Takatsuji, H. (2016). CC-NBS-LRR-type R proteins for rice blast commonly interact with specific WRKY transcription factors. Plant Mol. Biol. Report. 34, 533–537. doi: 10.1007/s11105-015-0932-4
Liu, W., Liu, J., Triplett, L., Leach, J. E., and Wang, G. -L. (2014). Novel insights into rice innate immunity against bacterial and fungal pathogens. Annu. Rev. Phytopathol. 52, 213–241. doi: 10.1146/annurev-phyto-102313-045926
Liu, H., Wang, Y., Xu, J., Su, T., Liu, G., and Ren, D. (2008). Ethylene signaling is required for the acceleration of cell death induced by the activation of AtMEK5 in Arabidopsis. Cell Res. 18, 422–432. doi: 10.1038/cr.2008.29
Lozano-Durán, R., and Zipfel, C. (2015). Trade-off between growth and immunity: role of brassinosteroids. Trends Plant Sci. 20, 12–19. doi: 10.1016/j.tplants.2014.09.003
Lu, X., Liu, X., An, L., Zhang, W., Sun, J., Pei, H., et al. (2008). The Arabidopsis MutS homolog AtMSH5 is required for normal meiosis. Cell Res. 18, 589–599. doi: 10.1038/cr.2008.44
Luo, D., Xu, H., Liu, Z., Guo, J., Li, H., Chen, L., et al. (2013). A detrimental mitochondrial-nuclear interaction causes cytoplasmic male sterility in rice. Nat. Genet. 45, 573–577. doi: 10.1038/ng.2570
Lynch, M., and Conery, J. S. (2000). The evolutionary fate and consequences of duplicate genes. Science 290, 1151–1155. doi: 10.1126/science.290.5494.1151
Ma, Y., Guo, H., Hu, L., Martinez, P. P., Moschou, P. N., Cevik, V., et al. (2018). Distinct modes of derepression of an Arabidopsis immune receptor complex by two different bacterial effectors. Proc. Natl. Acad. Sci. U. S. A. 115, 10218–10227. doi: 10.1073/pnas.1811858115
Maharani, A., Fanata, W. I. D., Laeli, F. N., Kim, K. -M., and Handoyo, T. (2020). Callus induction and regeneration from anther cultures of indonesian indica black rice cultivar. J. Crop. Sci. Biotechnol. 23, 21–28. doi: 10.1007/s12892-019-0322-0
Matsubara, K., Ando, T., Mizubayashi, T., Ito, S., and Yano, M. (2007). Identification and linkage mapping of complementary recessive genes causing hybrid breakdown in an intraspecific rice cross. Theor. Appl. Genet. 115, 179–186. doi: 10.1007/s00122-007-0553-x
Matsubara, K., Yamamoto, E., Mizobuchi, R., Yonemaru, J. -I., Yamamoto, T., Kato, H., et al. (2014). Hybrid breakdown caused by epistasis-based recessive incompatibility in a cross of rice (Oryza sativa L.). J. Hered. 106, 113–122. doi: 10.1093/jhered/esu065
Miah, G., Rafii, M. Y., Ismail, M. R., Puteh, A. B., Rahim, H. A., Asfaliza, R., et al. (2013). Blast resistance in rice: a review of conventional breeding to molecular approaches. Mol. Biol. Rep. 40, 2369–2388. doi: 10.1007/s11033-012-2318-0
Mishra, R., and Rao, G. J. N. (2016). In-vitro androgenesis in rice: advantages, constraints and future prospects. Rice Sci. 23, 57–68. doi: 10.1016/j.rsci.2016.02.001
Miura, K., Yamamoto, E., Morinaka, Y., Takashi, T., Kitano, H., Matsuoka, M., et al. (2008). The hybrid breakdown 1(t) locus induces interspecific hybrid breakdown between rice Oryza sativa cv. Koshihikari and its wild relative O. nivara. Breed. Sci. 58, 99–105. doi: 10.1270/jsbbs.58.99
Mizuno, N., Hosogi, N., Park, P., and Takumi, S. (2010). Hypersensitive response-like reaction is associated with hybrid necrosis in interspecific crosses between tetraploid wheat and Aegilops tauschii Coss. PLoS One 5:e11326. doi: 10.1371/journal.pone.0011326
Mouchel, C. F., Briggs, G. C., and Hardtke, C. S. (2004). Natural genetic variation in Arabidopsis identifies BREVIS RADIX, a novel regulator of cell proliferation and elongation in the root. Genes Dev. 18, 700–714. doi: 10.1101/gad.1187704
Muehe, E. M., Wang, T., Kerl, C. F., Planer-Friedrich, B., and Fendorf, S. (2019). Rice production threatened by coupled stresses of climate and soil arsenic. Nat. Commun. 10:4985. doi: 10.1038/s41467-019-12946-4
Mukhtar, M. S., Carvunis, A. -R., Dreze, M., Epple, P., Steinbrenner, J., Moore, J., et al. (2011). Independently evolved virulence effectors converge onto hubs in a plant immune system network. Science 333, 596–601. doi: 10.1126/science.1203659
Muralidharan, S., Box, M. S., Sedivy, E. L., Wigge, P. A., Weigel, D., and Rowan, B. A. (2014). Different mechanisms for Arabidopsis thaliana hybrid necrosis cases inferred from temperature responses. Plant Biol. 16, 1033–1041. doi: 10.1111/plb.12164
Nadeau, J. H., Singer, J. B., Matin, A., and Lander, E. S. (2000). Analysing complex genetic traits with chromosome substitution strains. Nat. Genet. 24, 221–225. doi: 10.1038/73427
Nadir, S., Li, W., Zhu, Q., Khan, S., Zhang, X. L., Zhang, H., et al. (2019). A novel discovery of a long terminal repeat retrotransposon-induced hybrid weakness in rice. J. Exp. Bot. 70, 1197–1207. doi: 10.1093/jxb/ery442
Naik, N., Rout, P., Umakanta, N., Verma, R. L., Katara, J. L., Sahoo, K. K., et al. (2017). Development of doubled haploids from an elite indica rice hybrid (BS6444G) using anther culture. Plant Cell Tissue Organ Cult. 128, 679–689. doi: 10.1007/s11240-016-1149-4
Narusaka, M., Shirasu, K., Noutoshi, Y., Kubo, Y., Shiraishi, T., Iwabuchi, M., et al. (2009). RRS1 and RPS4 provide a dual resistance-gene system against fungal and bacterial pathogens. Plant J. 60, 218–226. doi: 10.1111/j.1365-313X.2009.03949.x
Ning, Y., Liu, W., and Wang, G. -L. (2017). Balancing immunity and yield in crop plants. Trends Plant Sci. 22, 1069–1079. doi: 10.1016/j.tplants.2017.09.010
Ning, Y., and Wang, G. -L. (2018). Breeding plant broad-spectrum resistance without yield penalties. Proc. Natl. Acad. Sci. U. S. A. 115, 2859–2861. doi: 10.1073/pnas.1801235115
Ogawa, T., Yamamoto, T., Khush, G. S., and Mew, T. -W. (1991). Breeding of near-isogenic lines of rice with single genes for resrstance to bacterial blight pathogen (Xanthomonas campestris pv. oryzae). Jap. J. Breed. 41, 523–529. doi: 10.1270/jsbbs1951.41.523
Oka, H. -I. (1957). Phylogenetic differentiation of cultivated rice. XV. Complementary lethal genes in rice. Jap. J. Genet. 32, 83–87. doi: 10.1266/jjg.32.83
Okuno, K. (1985). Complementary recessive genes controlling hybrid breakdown found in a varietal cross of rice. RGN 2, 52–54.
Okuno, K. (1999). Geographical distribution of genes causing hybrid breakdown in varietal crosses of Asian cultivated rice. Genet. Resour. Crop. Evol. 46, 13–17. doi: 10.1023/A:1008631600311
Okuno, K., and Fukuoka, S. (1999). Distribution and RFLP mapping of complementary genes causing hybrid breakdown in Asian cultivated rice, Oryza sativa L. Jpn. Agric. Res. Q. 33, 1–6.
Okuyama, Y., Kanzaki, H., Abe, A., Yoshida, K., Tamiru, M., Saitoh, H., et al. (2011). A multifaceted genomics approach allows the isolation of the rice Pia-blast resistance gene consisting of two adjacent NBS-LRR protein genes. Plant J. 66, 467–479. doi: 10.1111/j.1365-313X.2011.04502.x
Palma, K., Thorgrimsen, S., Malinovsky, F. G., Fiil, B. K., Nielsen, H. B., Brodersen, P., et al. (2010). Autoimmunity in Arabidopsis acd11 is mediated by epigenetic regulation of an immune receptor. PLoS Pathog. 6:e1001137. doi: 10.1371/journal.ppat.1001137
Parra, L., Maisonneuve, B., Lebeda, A., Schut, J., Christopoulou, M., Jeuken, M., et al. (2016). Rationalization of genes for resistance to Bremia lactucae in lettuce. Euphytica 210, 309–326. doi: 10.1007/s10681-016-1687-1
Peart, J. R., Mestre, P., Lu, R., Malcuit, I., and Baulcombe, D. C. (2005). NRG1, a CC-NB-LRR protein, together with N, a TIR-NB-LRR protein, mediates resistance against tobacco mosaic virus. Curr. Biol. 15, 968–973. doi: 10.1016/j.cub.2005.04.053
Phillips, L. L. (1977). Interspecific incompatibility in gossypium. IV. Temperature-conditional lethality in hybrids of G. klotzschianum. Am. J. Bot. 64, 914–915. doi: 10.1002/j.1537-2197.1977.tb11935.x
Prasad, B. D., Creissen, G., Lamb, C., and Chattoo, B. B. (2009). Overexpression of rice (Oryza sativa L.) OsCDR1 leads to constitutive activation of defense responses in rice and Arabidopsis. Mol. Plant-Microbe Interact. 22, 1635–1644. doi: 10.1094/MPMI-22-12-1635
Purrington, C. B. (2000). Costs of resistance. Curr. Opin. Plant Biol. 3, 305–308. doi: 10.1016/S1369-5266(00)00085-6
Qian, Q., Guo, L., Smith, S. M., and Li, J. (2016). Breeding high-yield superior quality hybrid super rice by rational design. Natl. Sci. Rev. 3, 283–294. doi: 10.1093/nsr/nww006
Ray, S. K., Macoy, D. M., Kim, W. Y., Lee, S. Y., and Kim, M. G. (2019). Role of RIN4 in regulating PAMP-triggered immunity and effector-triggered immunity: current status and future perspectives. Mol. Cell 42, 503–511. doi: 10.14348/molcells.2019.2433
Ren, Z. L., and Lelley, T. (1988). Genetics of hybrid necrosis in rye. Plant Breed. 100, 173–180. doi: 10.1111/j.1439-0523.1988.tb00237.x
Ren, J., Wu, P., Trampe, B., Tian, X., Lübberstedt, T., and Chen, S. (2017). Novel technologies in doubled haploid line development. Plant Biotechnol. J. 15, 1361–1370. doi: 10.1111/pbi.12805
Rooney, H. C., van’t Klooster, J. W., van der Hoorn, R. A., Joosten, M. H., Jones, J. D., and de Wit, P. J. (2005). Cladosporium Avr2 inhibits tomato Rcr3 protease required for Cf-2-dependent disease resistance. Science 308, 1783–1786. doi: 10.1126/science.1111404
Rose, L., Atwell, S., Grant, M., and Holub, E. (2012). Parallel loss-of-function at the RPM1 bacterial resistance locus in Arabidopsis thaliana. Front. Plant Sci. 3:287. doi: 10.3389/fpls.2012.00287
Sabar, M., Akhter, M., Bibi, T., Riaz, A., Haider, Z., Khan, A. R., et al. (2019). Basmati rice lines development carrying multiple bacterial blight resistance genes pyramided using the marker-assisted backcross breeding approach. Mol. Breed. 39:155. doi: 10.1007/s11032-019-1047-7
Saito, T., Ichitani, K., Suzuki, T., Marubashi, W., and Kuboyama, T. (2007). Developmental observation and high temperature rescue from hybrid weakness in a cross between Japanese rice cultivars and Peruvian rice cultivar ‘Jamaica’. Breed. Sci. 57, 281–288. doi: 10.1270/jsbbs.57.281
Sarao, N. K., and Gosal, S. S. (2018). “In vitro androgenesis for accelerated breeding in rice” in Biotechnologies of crop improvement: Cellular approaches. Vol. 1. eds.S. S. Gosal and S. H. Wani (Cham: Springer International Publishing), 407–435.
Sarris, P. F., Cevik, V., Dagdas, G., Jones, J. D., and Krasileva, K. V. (2016). Comparative analysis of plant immune receptor architectures uncovers host proteins likely targeted by pathogens. BMC Biol. 14:8. doi: 10.1186/s12915-016-0228-7
Sato, Y. I., and Morishima, H. (1988). Distribution of the genes causing F2 chlorosis in rice cultivars of the Indica and Japonica types. Theor. Appl. Genet. 75, 723–727. doi: 10.1007/BF00265594
Schnable, P. S., and Springer, N. M. (2013). Progress toward understanding heterosis in crop plants. Annu. Rev. Plant Biol. 64, 71–88. doi: 10.1146/annurev-arplant-042110-103827
Seong, K., Seo, E., Witek, K., Li, M., and Staskawicz, B. (2020). Evolution of NLR resistance genes with noncanonical N-terminal domains in wild tomato species. New Phytol. 227, 1530–1543. doi: 10.1111/nph.16628
Shao, Z. -Q., Xue, J. -Y., Wu, P., Zhang, Y. -M., Wu, Y., Hang, Y. -Y., et al. (2016). Large-scale analyses of angiosperm nucleotide-binding site-leucine-rich repeat genes reveal three anciently diverged classes with distinct evolutionary patterns. Plant Physiol. 170, 2095–2109. doi: 10.1104/pp.15.01487
Shiragaki, K., Iizuka, T., Ichitani, K., Kuboyama, T., Morikawa, T., Oda, M., et al. (2019). HWA1- and HWA2-mediated hybrid weakness in rice involves cell death, reactive oxygen species accumulation, and disease resistance-related gene upregulation. Plants 8:450. doi: 10.3390/plants8110450
Shirano, Y., Kachroo, P., Shah, J., and Klessig, D. F. (2002). A gain-of-function mutation in an Arabidopsis toll interleukin1 receptor–nucleotide binding site–leucine-rich repeat type R gene triggers defense responses and results in enhanced disease resistance. Plant Cell 14, 3149–3162. doi: 10.1105/tpc.005348
Shivaprasad, P. V., Chen, H. -M., Patel, K., Bond, D. M., Santos, B. A. C. M., and Baulcombe, D. C. (2012). A microRNA superfamily regulates nucleotide binding site–leucine-rich repeats and other mRNAs. Plant Cell 24, 859–874. doi: 10.1105/tpc.111.095380
Si, W., Yuan, Y., Huang, J., Zhang, X., Zhang, Y., Zhang, Y., et al. (2015). Widely distributed hot and cold spots in meiotic recombination as shown by the sequencing of rice F2 plants. New Phytol. 206, 1491–1502. doi: 10.1111/nph.13319
Sicard, A., Kappel, C., Josephs, E. B., Lee, Y. W., Marona, C., Stinchcombe, J. R., et al. (2015). Divergent sorting of a balanced ancestral polymorphism underlies the establishment of gene-flow barriers in Capsella. Nat. Commun. 6:7960. doi: 10.1038/ncomms8960
Sinapidou, E., Williams, K., Nott, L., Bahkt, S., Tor, M., Crute, I., et al. (2004). Two TIR:NB:LRR genes are required to specify resistance to Peronospora parasitica isolate Cala2 in Arabidopsis. Plant J. 38, 898–909. doi: 10.1111/j.1365-313X.2004.02099.x
Spiezio, S. H., Takada, T., Shiroishi, T., and Nadeau, J. H. (2012). Genetic divergence and the genetic architecture of complex traits in chromosome substitution strains of mice. BMC Genet. 13:38. doi: 10.1186/1471-2156-13-38
Stahl, E. A., Dwyer, G., Mauricio, R., Kreitman, M., and Bergelson, J. (1999). Dynamics of disease resistance polymorphism at the Rpm1 locus of Arabidopsis. Nature 400, 667–671. doi: 10.1038/23260
Stein, J. C., Yu, Y., Copetti, D., Zwickl, D. J., Zhang, L., Zhang, C., et al. (2018). Genomes of 13 domesticated and wild rice relatives highlight genetic conservation, turnover and innovation across the genus Oryza. Nat. Genet. 50, 285–296. doi: 10.1038/s41588-018-0040-0
Stirnweis, D., Milani, S. D., Brunner, S., Herren, G., Buchmann, G., Peditto, D., et al. (2014). Suppression among alleles encoding nucleotide-binding-leucine-rich repeat resistance proteins interferes with resistance in F1 hybrid and allele-pyramided wheat plants. Plant J. 79, 893–903. doi: 10.1111/tpj.12592
Świadek, M., Proost, S., Sieh, D., Yu, J., Todesco, M., Jorzig, C., et al. (2017). Novel allelic variants in ACD6 cause hybrid necrosis in local collection of Arabidopsis thaliana. New Phytol. 213, 900–915. doi: 10.1111/nph.14155
Takken, F. L. W., and Goverse, A. (2012). How to build a pathogen detector: structural basis of NB-LRR function. Curr. Opin. Plant Biol. 15, 375–384. doi: 10.1016/j.pbi.2012.05.001
Tian, D., Araki, H., Stahl, E., Bergelson, J., and Kreitman, M. (2002). Signature of balancing selection in Arabidopsis. Proc. Natl. Acad. Sci. U. S. A. 99, 11525–11530. doi: 10.1073/pnas.172203599
Tian, D., Guo, X., Zhang, Z., Wang, M., and Wang, F. (2019). Improving blast resistance of the rice restorer line, Hui 316, by introducing Pi9 or Pi2 with marker-assisted selection. Biotechnol. Biotechnol. Equip. 33, 1195–1203. doi: 10.1080/13102818.2019.1649095
Tian, D., Traw, M. B., Chen, J. Q., Kreitman, M., and Bergelson, J. (2003). Fitness costs of R-gene-mediated resistance in Arabidopsis thaliana. Nature 423, 74–77. doi: 10.1038/nature01588
Todesco, M., Balasubramanian, S., Hu, T. T., Traw, M. B., Horton, M., Epple, P., et al. (2010). Natural allelic variation underlying a major fitness trade-off in Arabidopsis thaliana. Nature 465, 632–636. doi: 10.1038/nature09083
Todesco, M., Kim, S. T., Chae, E., Bomblies, K., Zaidem, M., Smith, L. M., et al. (2014). Activation of the Arabidopsis thaliana immune system by combinations of common ACD6 alleles. PLoS Genet. 10:1004459. doi: 10.1371/journal.pgen.1004459
Toruño, T. Y., Stergiopoulos, I., and Coaker, G. (2016). Plant-pathogen effectors: cellular probes interfering with plant defenses in spatial and temporal manners. Annu. Rev. Phytopathol. 54, 419–441. doi: 10.1146/annurev-phyto-080615-100204
Tran, D. T. N., Chung, E. -H., Habring-Müller, A., Demar, M., Schwab, R., Dangl, J. L., et al. (2017). Activation of a plant NLR complex through heteromeric association with an autoimmune risk variant of another NLR. Curr. Biol. 27, 1148–1160. doi: 10.1016/j.cub.2017.03.018
Tsuda, K., Sato, M., Stoddard, T., Glazebrook, J., and Katagiri, F. (2009). Network properties of robust immunity in plants. PLoS Genet. 5:e1000772. doi: 10.1371/journal.pgen.1000772
Van de Weyer, A. L., Monteiro, F., Furzer, O. J., Nishimura, M. T., Cevik, V., Witek, K., et al. (2019). A species-wide inventory of NLR genes and alleles in Arabidopsis thaliana. Cell 178, 1260.e1214–1272.e1214. doi: 10.1016/j.cell.2019.07.038
Van der Biezen, E. A., and Jones, J. D. (1998). Plant disease-resistance proteins and the gene-for-gene concept. Trends Biochem. Sci. 23, 454–456. doi: 10.1016/s0968-0004(98)01311-5
van der Hoorn, R. A., and Kamoun, S. (2008). From guard to decoy: a new model for perception of plant pathogen effectors. Plant Cell 20, 2009–2017. doi: 10.1105/tpc.108.060194
van Wersch, S., and Li, X. (2019). Stronger when together: clustering of plant NLR disease resistance genes. Trends Plant Sci. 24, 688–699. doi: 10.1016/j.tplants.2019.05.005
van Wersch, R., Li, X., and Zhang, Y. (2016). Mighty dwarfs: Arabidopsis autoimmune mutants and their usages in genetic dissection of plant immunity. Front. Plant Sci. 7:1717. doi: 10.3389/fpls.2016.01717
Wang, B. -H., Ebbole, D. J., and Wang, Z. -H. (2017). The arms race between Magnaporthe oryzae and rice: diversity and interaction of Avr and R genes. J. Integr. Agric. 16, 2746–2760. doi: 10.1016/S2095-3119(17)61746-5
Wang, J., Hu, M., Wang, J., Qi, J., Han, Z., Wang, G., et al. (2019a). Reconstitution and structure of a plant NLR resistosome conferring immunity. Science 364:eaav5870. doi: 10.1126/science.aav5870
Wang, C., Wang, Y., Cheng, Z., Zhao, Z., Chen, J., Sheng, P., et al. (2016). The role of OsMSH4 in male and female gamete development in rice meiosis. J. Exp. Bot. 67, 1447–1459. doi: 10.1093/jxb/erv540
Wang, J., Wang, J., Hu, M., Wu, S., Qi, J., Wang, G., et al. (2019b). Ligand-triggered allosteric ADP release primes a plant NLR complex. Science 364:eaav5868. doi: 10.1126/science.aav5868
Wang, L., Zhao, L., Zhang, X., Zhang, Q., Jia, Y., Wang, G., et al. (2019c). Large-scale identification and functional analysis of NLR genes in blast resistance in the Tetep rice genome sequence. Proc. Natl. Acad. Sci. U. S. A. 116, 18479–18487. doi: 10.1073/pnas.1910229116
Weßling, R., Epple, P., Altmann, S., He, Y., Yang, L., Henz, S. R., et al. (2014). Convergent targeting of a common host protein-network by pathogen effectors from three kingdoms of life. Cell Host Microbe 16, 364–375. doi: 10.1016/j.chom.2014.08.004
Wijnen, C. L., Botet, R., van de Belt, J., Deurhof, L., de Jong, H., de Snoo, C. B., et al. (2018). A complete chromosome substitution mapping panel reveals genome-wide epistasis in Arabidopsis. bioRxiv [Preprint], 436154. doi: 10.1101/436154
Wijnker, E., van Dun, K., de Snoo, C. B., Lelivelt, C. L. C., Keurentjes, J. J. B., Naharudin, N. S., et al. (2012). Reverse breeding in Arabidopsis thaliana generates homozygous parental lines from a heterozygous plant. Nat. Genet. 44, 467–470. doi: 10.1038/ng.2203
Wu, C. -H., Abd-El-Haliem, A., Bozkurt, T. O., Belhaj, K., Terauchi, R., Vossen, J. H., et al. (2017). NLR network mediates immunity to diverse plant pathogens. Proc. Natl. Acad. Sci. U. S. A. 114, 8113–8118. doi: 10.1073/pnas.1702041114
Wu, C. -H., Belhaj, K., Bozkurt, T. O., Birk, M. S., and Kamoun, S. (2016). Helper NLR proteins NRC2a/b and NRC3 but not NRC1 are required for Pto-mediated cell death and resistance in Nicotiana benthamiana. New Phytol. 209, 1344–1352. doi: 10.1111/nph.13764
Wu, C., Bordeos, A., Madamba, M. R., Baraoidan, M., Ramos, M., Wang, G. L., et al. (2008). Rice lesion mimic mutants with enhanced resistance to diseases. Mol. Gen. Genomics. 279, 605–619. doi: 10.1007/s00438-008-0337-2
Wu, C. -H., Krasileva, K. V., Banfield, M. J., Terauchi, R., and Kamoun, S. (2015). The “sensor domains” of plant NLR proteins: more than decoys? Front. Plant Sci. 6:134. doi: 10.3389/fpls.2015.00134
Xia, Y., Suzuki, H., Borevitz, J., Blount, J., Guo, Z., Patel, K., et al. (2004). An extracellular aspartic protease functions in Arabidopsis disease resistance signaling. EMBO J. 23, 980–988. doi: 10.1038/sj.emboj.7600086
Xiao, S., Ellwood, S., Calis, O., Patrick, E., Li, T., Coleman, M., et al. (2001). Broad-spectrum mildew resistance in Arabidopsis thaliana mediated by RPW8. Science 291, 118–120. doi: 10.1126/science.291.5501.118
Xiao, N., Wu, Y., Pan, C., Yu, L., Chen, Y., Liu, G., et al. (2017). Improving of rice blast resistances in japonica by pyramiding major R genes. Front. Plant Sci. 7:1918. doi: 10.3389/fpls.2016.01918
Yamamoto, E., Takashi, T., Morinaka, Y., Lin, S., Kitano, H., Matsuoka, M., et al. (2007). Interaction of two recessive genes, hbd2 and hbd3, induces hybrid breakdown in rice. Theor. Appl. Genet. 115, 187–194. doi: 10.1007/s00122-007-0554-9
Yamamoto, E., Takashi, T., Morinaka, Y., Lin, S., Wu, J., Matsumoto, T., et al. (2010). Gain of deleterious function causes an autoimmune response and Bateson-Dobzhansky-Muller incompatibility in rice. Mol. Gen. Genomics. 283, 305–315. doi: 10.1007/s00438-010-0514-y
Yanagihara, S., McCouch, S. R., Ishikawa, K., Ogi, Y., Maruyama, K., and Ikehashi, H. (1995). Molecular analysis of the inheritance of the S-5 locus, conferring wide compatibility in indica/japonica hybrids of rice (O. sativa L.). Theor. Appl. Genet. 90, 182–188. doi: 10.1007/BF00222200
Yang, S., and Hua, J. (2004). A haplotype-specific resistance gene regulated by BONZAI1 mediates temperature-dependent growth control in Arabidopsis. Plant Cell 16, 1060–1071. doi: 10.1105/tpc.020479
Yang, J., Zhao, X., Cheng, K., Du, H., Ouyang, Y., Chen, J., et al. (2012). A killer-protector system regulates both hybrid sterility and segregation distortion in rice. Science 337, 1336–1340. doi: 10.1126/science.1223702
Yi, H., and Richards, E. J. (2007). A cluster of disease resistance genes in Arabidopsis is coordinately regulated by transcriptional activation and RNA silencing. Plant Cell 19, 2929–2939. doi: 10.1105/tpc.107.051821
Yoshimura, S., Yamanouchi, U., Katayose, Y., Toki, S., Wang, Z. X., Kono, I., et al. (1998). Expression of Xa1, a bacterial blight-resistance gene in rice, is induced by bacterial inoculation. Proc. Natl. Acad. Sci. U. S. A. 95, 1663–1668. doi: 10.1073/pnas.95.4.1663
Zhai, J., Jeong, D. -H., De Paoli, E., Park, S., Rosen, B. D., Li, Y., et al. (2011). MicroRNAs as master regulators of the plant NB-LRR defense gene family via the production of phased, trans-acting siRNAs. Genes Dev. 25, 2540–2553. doi: 10.1101/gad.177527.111
Zhang, Y., Goritschnig, S., Dong, X., and Li, X. (2003). A gain-of-function mutation in a plant disease resistance gene leads to constitutive activation of downstream signal transduction pathways in suppressor of npr1-1, constitutive 1. Plant Cell 15, 2636–2646. doi: 10.1105/tpc.015842
Zhang, Y. -Y., Latzel, V., Fischer, M., and Bossdorf, O. (2018). Understanding the evolutionary potential of epigenetic variation: a comparison of heritable phenotypic variation in epiRILs, RILs, and natural ecotypes of Arabidopsis thaliana. Heredity 121, 257–265. doi: 10.1038/s41437-018-0095-9
Zhang, J., Peng, Y., and Guo, Z. (2008). Constitutive expression of pathogen-inducible OsWRKY31 enhances disease resistance and affects root growth and auxin response in transgenic rice plants. Cell Res. 18, 508–521. doi: 10.1038/cr.2007.104
Zhang, L., Tang, D., Luo, Q., Chen, X., Wang, H., Li, Y., et al. (2014). Crossover formation during rice meiosis relies on interaction of OsMSH4 and OsMSH5. Genetics 198:1447. doi: 10.1534/genetics.114.168732
Zhang, J., Wang, C., Higgins, J. D., Kim, Y. J., Moon, S., Jung, K. H., et al. (2019). A multiprotein complex regulates interference-sensitive crossover formation in rice. Plant Physiol. 181, 221–235. doi: 10.1104/pp.19.00082
Zhang, Y., Xia, R., Kuang, H., and Meyers, B. C. (2016). The diversification of plant NBS-LRR defense genes directs the evolution of microRNAs that target them. Mol. Biol. Evol. 33, 2692–2705. doi: 10.1093/molbev/msw154
Zhang, X., Yang, S., Wang, J., Jia, Y., Huang, J., Tan, S., et al. (2015). A genome-wide survey reveals abundant rice blast R genes in resistant cultivars. Plant J. 84, 20–28. doi: 10.1111/tpj.12955
Zhou, J. M., and Zhang, Y. (2020). Plant immunity: danger perception and signaling. Cell 181, 978–989. doi: 10.1016/j.cell.2020.04.028
Zhu, W., Zaidem, M., Van de Weyer, A. L., Gutaker, R. M., Chae, E., Kim, S. T., et al. (2018). Modulation of ACD6 dependent hyperimmunity by natural alleles of an Arabidopsis thaliana NLR resistance gene. PLoS Genet. 14:e1007628. doi: 10.1371/journal.pgen.1007628
Zhu, X., Ze, M., Chern, M., Chen, X., and Wang, J. (2020). Deciphering rice lesion mimic mutants to understand molecular network governing plant immunity and growth. Rice Sci. 27, 278–288. doi: 10.1016/j.rsci.2020.05.004
Keywords: hybrid necrosis, autoimmunity, anti-hybrid necrosis, heterosis, immunity, trade-off, NLR, growth
Citation: Calvo-Baltanás V, Wang J and Chae E (2021) Hybrid Incompatibility of the Plant Immune System: An Opposite Force to Heterosis Equilibrating Hybrid Performances. Front. Plant Sci. 11:576796. doi: 10.3389/fpls.2020.576796
Edited by:
Kazuki Matsubara, Institute of Crop Science (NARO), JapanReviewed by:
Rubén Alcázar, University of Barcelona, SpainKatsuyuki Ichitani, Kagoshima University, Japan
Copyright © 2021 Calvo-Baltanás, Wang and Chae. This is an open-access article distributed under the terms of the Creative Commons Attribution License (CC BY). The use, distribution or reproduction in other forums is permitted, provided the original author(s) and the copyright owner(s) are credited and that the original publication in this journal is cited, in accordance with accepted academic practice. No use, distribution or reproduction is permitted which does not comply with these terms.
*Correspondence: Eunyoung Chae, ZGJzY2VAbnVzLmVkdS5zZw==
†ORCID: Vanesa Calvo-Baltanás orcid.org/0000-0003-1348-8503
Jinge Wang orcid.org/0000-0002-6359-2133
Eunyoung Chae orcid.org/0000-0002-0889-9837