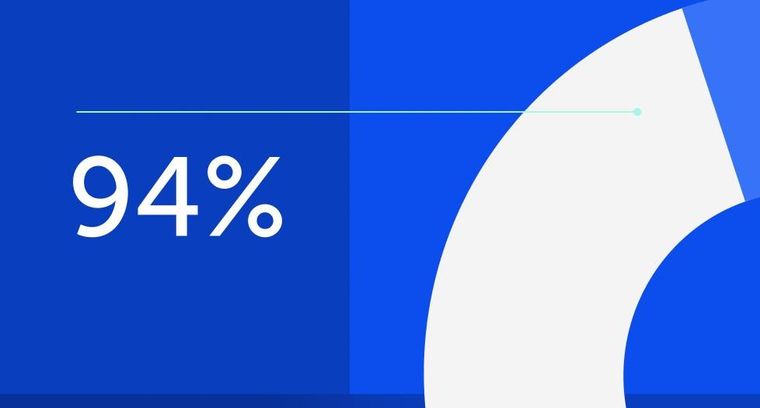
94% of researchers rate our articles as excellent or good
Learn more about the work of our research integrity team to safeguard the quality of each article we publish.
Find out more
ORIGINAL RESEARCH article
Front. Plant Sci., 30 October 2020
Sec. Plant Pathogen Interactions
Volume 11 - 2020 | https://doi.org/10.3389/fpls.2020.565745
Acibenzolar-S-methyl (ASM) is a well-known plant activator, which is a synthetic analog of salicylic acid (SA). Recently, copper fungicides and antibiotics are major strategies for controlling bacterial diseases. However, resistant strains have already been found. Therefore, there is an increasing demand for sustainable new disease control strategies. We investigated the ASM disease control effect against Pseudomonas cannabina pv. alisalensis (Pcal), which causes bacterial blight on Japanese radish. In this study, we demonstrated that ASM effectively suppressed Pcal disease symptom development associated with reduced bacterial populations on Japanese radish leaves. Interestingly, we also demonstrated that ASM activated systemic acquired resistance (SAR), including stomatal-based defense on ASM-untreated upper and lower leaves. Reactive oxygen species (ROS) are essential second messengers in stomatal-based defense. We found that ASM induced stomatal closure by inducing ROS production through peroxidase. These results indicate that stomatal closure induced by ASM treatment is effective for preventing Pcal pathogen invasion into plants, and in turn reduction of disease development.
Recently, several bacterial pathogens were identified as causal agents of severe worldwide epidemics. Pseudomonas cannabina pv. alisalensis (Pcal) causes bacterial blight of crucifer plants (Brassicaceae plants; Takikawa and Takahashi, 2014) and inflicts great damage on crucifer production including cabbage, Chinese cabbage, and Japanese radish. On Japanese radish, disease symptoms caused by a newly isolated Pcal were observed on leaves and roots (Takikawa and Takahashi, 2014). Discoloration of roots and necrotic leaf lesions has serious implications for the commercial value of Japanese radish. Chemical treatments such as copper fungicides and antibiotics are popular strategies for bacterial disease control. However, Pcal strains resistant against these chemicals were found (Takahashi et al., 2013). Therefore, the demand for developing sustainable alternative strategies has been increasing.
Acibenzolar-S-methyl (ASM), a synthetic analog of salicylic acid (SA), has been used to protect crops from several diseases by activating plant defense (Kunz et al., 1997; Oostendorp et al., 2001). We demonstrated that soil drench application with ASM induced systemic acquired resistance (SAR) against Pcal in cabbage (Ishiga et al., 2020). SAR processes can be divided into three steps: local immune activation, information relay from local to systemic tissues by mobile signals, and defense activation and priming in systemic tissues (Jung et al., 2009; Shah and Zeier, 2013; Wang et al., 2018). Thus, our previous results implied that mobile signals generated by ASM-triggered SAR in untreated leaves. Pre-treating cucumber plant first leaves with ASM protected whole plants from fungal infection with Colletotrichum orbiculare (Cools and Ishii, 2002). In cucumber plants, SAR genes were rapidly and highly expressed in upper leaves after first leaf treatment with ASM (Narusaka et al., 1999, 2001; Cools and Ishii, 2002). However, the ASM-induced SAR mechanism is still unclear. We also demonstrated that ASM protects cabbage plants from Pcal by activating stomatal-based defense (Ishiga et al., 2020). Furthermore, ASM induced expression of SAR marker genes including PR1, PR2, and PR5 in cabbage plants (Ishiga et al., 2020). Additionally, because many foliar bacterial pathogens target stomata as an entry site, ASM is expected to have a powerful disease control effect. However, whether ASM is effective at suppressing disease development against Pcal on other crops (in addition to cabbage) has not been evaluated.
ROS production at the cell surface, which is called oxidative burst, is one of the earliest defenses detectable during pathogen-associated molecular pattern (PAMP)-triggered immunity (PTI) and effector-triggered immunity (ETI). Doke (1983) first demonstrated that ROS members possibly function as the chemical signals required for hypersensitive response (HR) induction. The oxidative burst induced by pathogen-derived signals is essential to defense mechanisms throughout the plant (Fobert and Després, 2005). Apoplastic ROS are generated from plasma membrane-localized NADPH oxidases and cell wall-localized peroxidases (Zhao et al., 2019). ROS are known as antimicrobial molecules involved in cell wall reinforcement and callose deposition. ROS also act as local and systemic secondary messengers triggering additional immune responses, including stomatal movement. Stomatal closure in response to various stress is brought about by loss of guard cell turgor, which is caused by ion channel modulation (Thor et al., 2020; Wu et al., 2020). A complex signaling network involving ROS production regulates these channels (Kim et al., 2010; Kollist et al., 2014). ASM treatment of the first leaves via fungal inoculation caused rapid H2O2 accumulation below the penetration site on ASM-untreated third leaves (Lin and Ishii, 2009; Park et al., 2019). Endogenous SA is essential for both apoplastic ROS production and stomatal closure induced by chitosan as microbe-associated molecular patterns (MAMPs; Prodhan et al., 2017). Since ASM is a synthetic analog of SA, it is tempting to speculate that ASM induced stomatal closure is closely related to ROS production.
Here, we demonstrate that ASM successfully suppressed disease development and bacterial multiplication in Japanese radish by activating stomatal defense. Furthermore, to gain knowledge into the ASM action mechanism on stomata, we investigated its effect on ROS production. ASM activated ROS production mediated by peroxidases, which in turn activated stomatal-based defense. Moreover, ASM also induced PR gene expression on Japanese radish. Importantly, ASM controlled Japanese radish bacterial blight disease not only on the treated leaves, but also on upper and lower leaves. Thus, our results highly support that ASM can be an additional disease management tool to prevent crop disease losses against bacterial pathogens.
Japanese radish (Raphanus sativus var. longipinnatus) cv. Natsutsukasa plants were used for all experiments. Seedlings were grown in 9 cm pots at 24°C with a light intensity of 200 μmol/(m2s) and 16 h light/8 h dark in a growth chamber or were maintained in the greenhouse under a natural photoperiod at 21.2 ± 2.4°C. ASM (marketed as ACTIGARD®) was supplied courtesy of Syngenta as a 50% (a.i.) water-dispersible granular formulation and dissolved in water. To evaluate the effect of ASM on plant defense, Japanese radish fourth leaves were treated with ASM (100 ppm) by dip-treatment at 3 weeks after sowing or when fifth true leaves were unfolded. Salicylhydroxamic acid (SHAM) and diphenyleneiodonium chloride (DPI) were obtained from Sigma-Aldrich (Sigma-Aldrich, St Louis, MO, United States). Plants were treated with ASM 4 h, 1 day, and 1 week before Pcal inoculation (Supplementary Figure 1). Plants were dip-treated with water or mock-inoculated with water as controls.
Pathogenic Pseudomonas cannabina pv. alisalensis strain KB211 (Pcal; Sakata et al., 2019) was kindly provided by the Nagano Vegetable and Ornamental Crops Experiment Station, Nagano, Japan. Pcal was grown at 28°C for 24 h on Kings B (KB; King et al., 1954) agar medium. For inoculum, bacteria were suspended in sterile distilled H2O, and cell densities measured at 600 nm (OD600) using a Biowave CO8000 Cell Density Meter (biochrom, Cambridge, United Kingdom).
Plants were spray-inoculated with a bacterial suspension in sterile distilled water containing 0.025% Silwet L-77 (bio medical science, Tokyo, Japan). For Pcal lesion area and disease symptoms measurement, Japanese radish plants were spray-inoculated with a bacterial suspension [5 × 107 colony forming units (CFU)/ml] to runoff. Dip-inoculation method was used for stomatal assay. For bacterial growth assay, Japanese radish plants were spray-inoculated with a bacterial suspension (5 × 107 CFU/ml). The plants were then incubated in growth chambers at approximately 100% RH for the first 24 h, then at approximately 70% RH for the rest of the experiment. Lesion area and bacterial population were assessed 1-week post-inoculation (wpi).
To assess the percentage of diseased leaf area of each leaf by visually estimating the leaf area affected as a percentage of the whole leaf area. The lesion area was evaluated in four independent experiments.
To assess bacterial growth in Japanese radish, the internal bacterial population was measured after dip-inoculation. Inoculated plants were collected, and a whole inoculated leaf was used to quantify the bacterial population. The leaves were surface-sterilized with 10% H2O2 for 3 min. After washing three times with sterile distilled water, the leaves were homogenized in sterile distilled water, and diluted samples were plated onto solid KB agar medium. Two or three days after dilution sample plating, the bacterial CFUs were counted and normalized as CFU per gram using the total leaf weight. The bacterial populations were evaluated in four independent experiments.
For expression profiles of Japanese radish defense genes in response to ASM, plants were dip-treated with water as a control or ASM on fourth leaves. After 4 h, total RNA was extracted using RNAiso Plus (Takara Bio, Shiga, Japan) according to the manufacturer’s protocol and used for real-time quantitative RT-PCR (qRT-PCR) as described (Ishiga and Ichinose, 2016). Two micrograms of total RNA were treated with gDNA Remover (TOYOBO, Osaka, Japan) to eliminate genomic DNA, and the DNase-treated RNA was reverse transcribed using the ReverTra Ace® qPCR RT Kit (TOYOBO). The cDNA (1:50) was then used for RT-qPCR with the primers shown below with THUNDERBIRD® qPCR Mix (TOYOBO) on a Thermal Cycler Dice Real Time System (Takara Bio). Radish Glyceraldehyde 3-phosphate dehydrogenase (GAPDH) gene was used as an internal control. Average CT values, calculated using the second derivative maximum method from triplicate samples, were used to determine the fold expression relative to the controls (zero time). Primers used in gene-specific PCR amplification for PR1 were 5'‐ AAAGCTACGCCGACCGACTACGAG -3' and 5'‐ CCAGAAAAGTCGGCGCTACTCCA -3'; for PR2, 5'‐ GTACGCTCTGTTCAAACCGACCC -3' and 5'‐ TTTCCAACGATCCTCCGCCTGA -3'; for PR3, 5'‐ TCTTTGGTCAGACTTCCCACGAG -3' and 5'‐ GATGGCTCTTCCACACTGTCCGTA -3'; and for GAPDH, 5'‐ CGCTTCCTTCAACATCATTCCCA -3' and 5'‐ TCAGATTCCTCCTTGATAGCCTT -3' according to a previous study (Alkooranee et al., 2015).
A modified method was used to assess stomatal response as described previously (Chitrakar and Melotto, 2010). Briefly, Japanese radish plants were grown for 3 weeks after sowing as described previously. Pcal was grown at 28°C for 24 h on KB agar medium, then suspended in sterile distilled water to 1 × 108 CFU/ml. Dip-inoculated or water mock-inoculated radish leaves were directly imaged at 4 h post-inoculation (hpi) using a Nikon optical microscope (Eclipse 80i). Additionally, 1 week after ASM treatment, leaves were treated with SHAM (1 mM) and DPI (10 μM) with and without Pcal. The aperture width of at least 100 stomata was measured 4 hpi and treatment (Supplementary Figure 1). The average and SE for the stomatal aperture width were calculated. The stomatal apertures were evaluated in three independent experiments.
All data are expressed as the mean with SE. All statistical analyses were performed using EZR (Saitama Medical Centre, Jichi Medical University, Saitama, Japan; Kanda, 2013), a graphical user interface for R (version 3.6.3; R Foundation for statistical Computing, Vienna, Austria). Two-way ANOVA and Tukey’s honestly significant difference (HSD) test were used to analyze stomatal aperture width. Differences of p < 0.05 were considered statistically significant.
To investigate if ASM effectively suppresses Pcal disease development in Japanese radish plants, plants were inoculated with Pcal, 4 h, 1 day, and 1 week after ASM dip-treatment on only the fourth leaf. Then, disease symptoms were observed at 7 day post-inoculation (dpi; Supplementary Figure 1). The ASM-treated Japanese radish plants showed less disease symptoms than the control water-treated plants inoculated with Pcal in all the time points after the ASM treatment (Supplementary Figure 2). We also evaluated the disease development by measuring lesion areas. The Pcal lesion area on the ASM-treated Japanese radish plants was significantly smaller than that of the control water-treated plants even if the ASM-pretreatment period was only 4 h (Figures 1A–C). Furthermore, the lesion area was reduced not only on the ASM-treated leaves, but also on the untreated upper and lower leaves (Figures 1A–C). To investigate whether ASM suppresses bacterial growth as well as lesion area, we also measured bacterial population. In the Japanese radish, fourth leaves pretreated with ASM for 4 h, the Pcal populations were around 10 times less compared to those of the water-treated control (Figure 2A). In addition, at 1 day post-treatment (dpt) and 1 week post-treatment (wpt) with ASM, the Pcal populations were around 100 times less compared to those of the water-treated control (Figures 2B,C). Moreover, in untreated third and fifth leaves, the Pcal populations were also less compared to those of the water-treated control (Figures 2A–C). These results indicate that ASM is able to suppress the symptom development and the bacterial multiplication in Japanese radish after Pcal inoculation, and this effect is acquired systemically.
Figure 1. Lesion area (%) of acibenzolar-S-methyl (ASM)-treated and untreated leaves of Japanese radish after Pseudomonas cannabina pv. alisalensis (Pcal) inoculation. Greenhouse-grown Japanese radish plants were spray-inoculated with Pcal (5 × 107 CFU/ml) 4 h (A), 1 day (B), and 1 week (C) after ASM dip-treatment (100 ppm) on fourth leaves. Disease symptoms of the ASM-treated fourth leaves and untreated upper (fifth) and lower (third) leaves were monitored by measuring lesion areas at 1-week post inoculation (wpi). Vertical bars indicate the SE for four independent replications. Asterisks indicate a significant difference from the water treatment control in a t-test (*p < 0.05; **p < 0.01).
Figure 2. Bacterial populations of ASM-treated and untreated systemic leaves of Japanese radish after Pcal inoculation. Three-week-old Japanese radish plants were spray-inoculated with Pcal suspensions (5 × 107 CFU/ml) 4 h (A), 1 day (B), and 1 week (C) after ASM dip-treatment (100 ppm) on fourth leaves. Bacterial populations in the ASM-treated fourth leaves and untreated systemic upper (fifth) and lower (third) leaves were determined by dilution plating on selective medium as described in the methods section at 1-wpi. Vertical bars indicate the SE for three independent experiments. Asterisks indicate a significant difference from the water treatment control in a t-test (*p < 0.05).
To examine whether ASM activates stomatal-based defense on Japanese radish, we observed stomatal response after Pcal inoculation of the ASM-treated fourth leaves and their untreated third and fifth leaves. At 4 h after the ASM treatment, all the tested leaves (the ASM-treated forth leaves as well as the untreated upper and lower leaves) exhibited stomatal closure upon Pcal inoculation unlike the ASM-untreated control plants (Figures 3A–C). Meanwhile, at 1 dpt and 1 wpt after the ASM treatment, all tested leaves except for the third leaves at 1 dpt exhibited stomatal closure regardless of Pcal inoculation (Figures 3D–I). These results indicate that the ASM treatment suppressed stomatal opening at least 1 dpt and the effect lasted for a week. Furthermore, the effect extended to untreated systemic leaves (Figures 3A,C,D,F,G,I). At 1 dpt and 1 wpt after the ASM treatment, all the tested leaves exhibited similar or enhanced stomatal closure level upon Pcal inoculation compared with the uninoculated controls. These results indicate that ASM activates stomatal-based defense against Pcal on Japanese radish locally and systemically.
Figure 3. Stomatal aperture width (μm) of ASM-treated and untreated systemic leaves of Japanese radish plants after Pcal inoculation. The fourth leaves of Japanese radish were dipped with ASM (100 ppm), and leaves were dip inoculated with Pcal suspensions (1 × 108 CFU/ml) 4 h (A–C), 1 day (D–F), and 1 week (G–I) after ASM treatment. The microscope images of the ASM-treated fourth leaves (B,E,H), and the untreated systemic third (A,D,G), and fifth (C,F,I) leaves were taken 4 h post-inoculation (hpi) of Pcal using a Nikon optical microscope (Eclipse 80i). Then, stomatal aperture width (μm) were analyzed by image J using at least 100 stomata. In all bar graphs, vertical bars indicate the SE for three biological replicates. Significant factors (SF) indicate whether the two independent factors, ASM treatment (A) and Pcal inoculation (P), and/or their interaction, I (A × P), were statistically significant (two way ANOVA, p < 0.05). When the interaction was significant, Tukey’s honestly significant difference (HSD) test was performed. Different letters indicate significant differences between treatment/inoculation (p < 0.05). p values of the two-way ANOVA are shown in Supplemental Table 1.
Reactive oxygen species (ROS) are essential second messengers in stomatal immunity (Joshi-Saha et al., 2011). Stomatal closure by the phytohormone SA requires ROS production by cell wall peroxidases (Mori et al., 2001; Khokon et al., 2011). Since ASM is a synthetic analog of SA, we investigated whether the signaling of ASM-induced stomatal closure is mediated by ROS by using a peroxidase inhibitor, SHAM, and an NADPH oxidase inhibitor, diphenylene iodonium (DPI). At 1 week after the ASM dip-treatment on forth leaves, the ASM-treated leaves and the untreated third and fifth leaves were dip-treated with SHAM (1 mM) or DPI (10 μM). Then, stomatal aperture of each leaf was examined 4 h after the chemical treatment. ASM-induced stomatal closure was suppressed by SHAM (Figures 4A–C), indicating that cell wall peroxidases might be involved in mediating ROS production during ASM-induced stomatal closure. Conversely, exogenous DPI application did not inhibit the ASM-induced stomatal closure (Figures 4A–C). We obtained similar results in the ASM-treated leaves and their untreated systemic leaves simultaneously inoculated with Pcal when SHAM or DPI was treated (Supplementary Figures 3A–C). These results suggest that ROS produced by cell wall peroxidases is likely to at least in part mediate signaling in the ASM-induced stomatal closure.
Figure 4. Stomatal aperture width (μm) in ASM-treated Japanese radish plants after application with SHAM or DPI. Fourth leaves of Japanese radish were dip-treated with ASM (100 ppm) and after 1 week, SHAM (1 mM), and DPI (10 μM) was dip-treated to the ASM-treated fourth leaves, and the untreated systemic upper (fifth) and lower (third) leaves. The microscope images of third (A), fourth (B), and fifth (C) leaves were taken 4 h after treatment of the chemicals using a Nikon optical microscope (Eclipse 80i). Then, stomatal aperture width (μm) were analyzed by image J using at least 100 stomata. In all bar graphs, vertical bars indicate the SE for three biological replicates. Significant differences (p < 0.05) are indicated by different letters based on a Tukey’s HSD test.
ASM also induced defense-related gene expression, including PR proteins in Arabidopsis thaliana, Brachypodium distachyon, tobacco, cucumber, and cabbage (Wendehenne et al., 1998; Nakashita et al., 2002; Yasuda et al., 2008; Kouzai et al., 2018a,b; Ishiga et al., 2020). To investigate the effect of ASM treatment on Japanese radish defense gene expression, we investigated the expression profiles of PR1, PR2, and PR3 in response to ASM. The expression of PR1, PR2, and PR3 was significantly induced in fourth leaves 4 h after ASM treatment (Figures 5A–C). Furthermore, in both untreated upper and lower leaves, all gene expression was induced or tended to be induced (Figures 5A–C). These results indicate that ASM induced defense-related gene expression in both local and systemic leaves in Japanese radish.
Figure 5. Gene expression profiles of PR1, PR2, and PR3 of Japanese radish plant in ASM-treated and untreated systemic leaves. Forth leaves of 3-week-old Japanese radish plants were treated with ASM (100 ppm) or water as a control. After 4 h expression of PR1 (A), PR2 (B), and PR3 (C) in the ASM-treated fourth leaves, and the untreated systemic upper (fifth) and lower (third) leaves were determined using real-time quantitative reverse transcription-PCR (RT-qPCR) with gene-specific primer sets. Expression was normalized using glyceraldehyde 3-phosphate dehydrogenase (GAPDH). Vertical bars indicate the SE for three biological replicates. Asterisks indicate a significant difference from the water control in a t-test (*p < 0.05; **p < 0.01).
There is an increased demand for developing sustainable disease control strategies. ASM is predicted as an additional disease management tool to protect numerous crops from pathogens without the occurrence of chemically resistant strains. Our results clearly showed that an ASM dip-treatment effectively suppressed Pcal lesion formation and bacterial multiplication on Japanese radish plants (Figures 1, 2; Supplementary Figure 2). Previously, we also demonstrated that an ASM soil drench suppressed Pcal disease development associated with reduced bacterial population in cabbage (Ishiga et al., 2020). These results indicate that ASM is a powerful tool against Pcal, which is the causal agent of bacterial blight of cruciferous plants.
The most important finding was that ASM greatly enhanced the systemic activation of defense response. The reduction of disease development and bacterial growth was observed not only on treated fourth leaves, but also on untreated third and fifth leaves (Figures 1, 2; Supplementary Figure 2). Additionally, ASM triggered stomatal-based defense and SAR-related gene expression induction on both treated and untreated leaves (Figures 3, 5). Cools and Ishii (2002) demonstrated that ASM pre-treatment of cucumber first leaves protected whole plants from infection with the virulent fungal pathogen Colletotrichum orbiculare. Three hours post-ASM treatment on first leaves, ASM-induced systemic priming of PAL1 expression in cucumber plants occurred rapidly, with enhanced expression in the third leaves (Cools and Ishii, 2002). Interestingly, our results showed ASM-induced systemic defense was acquired not only on untreated upper leaves, but also on untreated lower leaves (Figure 5). Kubota and Abiko (2000) demonstrated that 7 days after a first inoculation with Colletotrichum lagenarium and Pythium ultimum on cucumber cotyledons and primary leaves, induced resistance was observed against the challenge inoculation with those pathogenic fungi in hypocotyl. These results implied that foliar leaf treatment can prevent disease of lower plant parts, including hypocotyls and roots (Kubota and Abiko, 2000). Importantly, Pcal causes discoloration of Japanese radish hypocotyl and root (Takikawa and Takahashi, 2014). It is expected that ASM-induced systemic defense could be a powerful disease management tool against bacterial blight on Japanese radish.
The gene expression profiles of PR1, PR2, and PR3 were induced at least at 4 h after ASM-treatment in both treated and untreated leaves (Figure 5). ASM induced the expression of PR1, PR2, and PR5 in A. thaliana and cabbage (Uknes et al., 1992; Lawton et al., 1996; Ishiga et al., 2020). ASM also induced PR1, PR3, and PR5 expression in tobacco (Mandal et al., 2008). In Raphanus alboglabra, PR1, PR2, and PR3 expressions were increased by fungal pathogen, Erysiphe cruciferarum (Alkooranee et al., 2015). These results suggest that PR1, PR2, and PR3 can serve as SAR marker genes in Japanese radish.
In our results, the ASM-induced disease control effect was obvious at 4 hpt (Figures 1, 2), and SAR-related gene expression was also induced at 4 hpt (Figure 5). Benzo (1,2,3) thiadiazole-7-carboxylic acid (acibenzolar), but not ASM itself was detected in untreated third leaves 3 dpt on first leaves in cucumber (Ishii et al., 2002). SABP2 catalyzes ASM conversion into acibenzolar to induce SAR (Tripathi et al., 2010). Therefore, acibenzolar may function similar to SA to activate NPR1 and SAR. Given that acibenzolar was not detected in untreated leaves 1 dpt in cucumber (Ishii et al., 2002), it is tempting to speculate that putative signal molecules are rapidly translocated from ASM-treated leaves to untreated leaves in Japanese radish. Several candidates for this long-distance signal have been identified, including methyl salicylate (MeSA), an SFD1/GLY1-derived G3P-dependent signal, the lipid-transfer protein DIR1, the dicarboxylic acid azelaic acid (AzA), the abietane diterpenoid dehydroabirtinal (DA), jasmonic acid (JA), and N-hydroxy-pipecolic acid (N-hydroxy-Pip; Dempsey and Klessig, 2012; Chen et al., 2018; Hartmann et al., 2018). Among them, N-hydroxy-Pip acts as primary regulator of SAR (Návarová et al., 2012; Zeier, 2013; Chen et al., 2018; Hartmann et al., 2018). In A. thaliana, these responses include camalexin (phytotoxin) accumulation and expression of defense-related genes, including ALD1, FMO1, and PR1 (Bernsdorff et al., 2016). Further analysis will be needed to elucidate the mobile signals which induce SAR in Japanese radish.
ASM induced stomatal-based defense against Pcal within 4 hpt in both cabbage and Japanese radish (Figure 3; Ishiga et al., 2020). We also showed that the ASM-triggered stomatal closure was suppressed by a peroxidase inhibitor, SHAM but not by an NADPH oxidase inhibitor, DPI (Figure 4; Supplementary Figure 3). Khokon et al. (2011) reported that SA-induced stomatal closure accompanied ROS production mediated by peroxidase in A. thaliana. Therefore, these results suggest that the ASM-induced stomatal closure is closely related to ROS production through peroxidase. Our results also showed that the ASM induced stomatal-based defense was observed not only on treated leaves, but also on untreated leaves (Figure 3). Lin and Ishii (2009) demonstrated that rapid H2O2 accumulation occurred in cucumber stem xylem fluids during ASM-induced SAR. Takahashi and Shinozaki (2019) reported that light stress-mediated ABA accumulation induced the ROS/Ca2+ wave propagated from local leaves to systemic leaves with a rapid transmission speed, suggesting that local ROS/Ca2+ wave functions as long-distance signal to activate stomatal closure during light stress conditions. Therefore, it is possible that ROS induced by ASM treatment activates stomatal closure in a systemic response during pathogen infection.
Based on our results, we propose a stomatal closure model induced by ASM treatment. ASM induces ROS production mediated by SHAM-sensitive peroxidase, leading to stomatal closure. Then, local ROS functions as a long-distance signal to induce stomatal closure in a systemic response after ASM treatment. In addition to ROS, it is expected that some mobile signals are possibly rapidly transported to induce ROS production leading to stomatal closure. Further analysis will be needed to understand the ASM-induced SAR mechanism.
We clearly demonstrated that ASM dip-treatment protects Japanese radish plants against Pcal, a causal agent of bacterial blight disease, by activating SAR, such as stomatal-based defense. Furthermore, we observed that ASM-induced defense response was acquired not only on treated leaves, but also untreated upper and lower leaves. These results highlight the role of ASM as an efficient sustainable disease control strategy.
The datasets presented in this study can be found in online repositories. The names of the repository/repositories and accession number(s) can be found at: https://figshare.com/s/439806eb8e944c0f4dbb.
NS, ST, and YI designed the study and wrote the manuscript. NS, TI, ST, and YI performed experiments and collected and analyzed data. All authors contributed to the article and approved the submitted version.
This work was supported in part by Sustainable Food Security Research Project in the form of an operational grant from the National University Corporation and by the Japan Science and Technology Agency (JST) Exploratory Research for Advanced Technology (ERATO) NOMURA Microbial Community Control Project, Grant Number: JPMJER1502.
YI has received research funds from Syngenta Japan.
The remaining authors declare that the research was conducted in the absence of any commercial or financial relationships that could be construed as a potential conflict of interest.
We thank Dr. Christina Baker for editing the manuscript. Pcal KB211 was kindly provided by the Nagano Vegetable and Ornamental Crops Experiment Station, Nagano, Japan.
The Supplementary Material for this article can be found online at: https://www.frontiersin.org/articles/10.3389/fpls.2020.565745/full#supplementary-material
Alkooranee, J. T., Yin, Y., Aledan, T. R., Jiang, Y., Lu, G., Wu, J., et al. (2015). Systemic resistance to powdery mildew in Brassica napus (AACC) and Raphanus alboglabra (RRCC) by Trichoderma harzianum TH12. PLoS One 10:e0142177. doi: 10.1371/journal.pone.0142177
Bernsdorff, F., Döring, A. C., Gruner, K., Schuck, S., Bräutigam, A., and Zeier, J. (2016). Pipecolic acid orchestrates plant systemic acquired resistance and defense priming via salicylic acid-dependent and-independent pathways. Plant Cell 28, 102–129. doi: 10.1105/tpc.15.00496
Chen, Y. C., Holmes, E. C., Rajniak, J., Kim, J. G., Tang, S., Fischer, C. R., et al. (2018). N-hydroxy-pipecolic acid is a mobile metabolite that induces systemic disease resistance in Arabidopsis. Proc. Natl. Acad. Sci. U. S. A. 115, E4920–E4929. doi: 10.1073/pnas.1805291115
Chitrakar, R., and Melotto, M. (2010). Assessing stomatal response to live bacterial cells using whole leaf imaging. J. Vis. Exp. 44:2185. doi: 10.3791/2185
Cools, H. J., and Ishii, H. (2002). Pre-treatment of cucumber plants with acibenzolar-S-methyl systemically primes a phenylalanine ammonia lyase gene (PAL1) for enhanced expression upon attack with a pathogenic fungus. Physiol. Mol. Plant Pathol. 61, 273–280. doi: 10.1006/pmpp.2003.0439
Dempsey, D. A., and Klessig, D. F. (2012). SOS—too many signals for systemic acquired resistance? Trends Plant Sci. 17, 538–545. doi: 10.1016/j.tplants.2012.05.011
Doke, N. (1983). Generation of superoxide anion by potato tuber protoplasts during the hypersensitive response to hyphal wall components of Phytophthora infestans and specific inhibition of the reaction by suppressors of hypersensitivity. Physiol. Plant Pathol. 23, 359–367. doi: 10.1016/0048-4059(83)90020-6
Fobert, P. R., and Després, C. (2005). Redox control of systemic acquired resistance. Curr. Opin. Plant Biol. 8, 378–382. doi: 10.1016/j.pbi.2005.05.003
Hartmann, M., Zeier, T., Bernsdorff, F., Reichel-Deland, V., Kim, D., Hohmann, M., et al. (2018). Flavin monooxygenase-generated N-hydroxypipecolic acid is a critical element of plant systemic immunity. Cell 173, 456.e16–469.e16. doi: 10.1016/j.cell.2018.02.049
Ishiga, Y., and Ichinose, Y. (2016). Pseudomonas syringae pv. tomato OxyR is required for virulence in tomato and Arabidopsis. Mol. Plant Microbe Interact. 29, 119–131. doi: 10.1094/MPMI-09-15-0204-R
Ishiga, T., Iida, Y., Sakata, N., Ugajin, T., Hirata, T., Taniguchi, S., et al. (2020). Acibenzolar-S-methyl activates stomatal-based defense against Pseudomonas cannabina pv. alisalensis in cabbage. J. Gen. Plant Pathol. 86, 48–54. doi: 10.1007/s10327-019-00883-5
Ishii, H., Kato, T., Yamanaka, M., Cools, H., and Sugiyama, T. (2002). Induction activity of systemic disease resistance and metabolism of acibenzolar-S-methyl in cucumber (abstract in Japanese). Abstr. 27th Ann. Meet. Pesticide Sci. Soc. Jpn.
Joshi-Saha, A., Valon, C., and Leung, J. (2011). A brand new START: abscisic acid perception and transduction in the guard cell. Sci. Signal. 4:re4. doi: 10.1126/scisignal.2002164
Jung, H. W., Tschaplinski, T. J., Wang, L., Glazebrook, J., and Greenberg, J. T. (2009). Priming in systemic plant immunity. Science 324, 89–91. doi: 10.1126/science.1170025
Kanda, Y. (2013). Investigation of the freely available easy-to-use software “EZR” for medical statistics. Bone Marrow Transplant. 48, 452–458. doi: 10.1038/bmt.2012.244
Khokon, A. R., Okuma, E., Hossain, M. A., Munemasa, S., Uraji, M., Nakamura, Y., et al. (2011). Involvement of extracellular oxidative burst in salicylic acid-induced stomatal closure in Arabidopsis. Plant Cell Environ. 34, 434–443. doi: 10.1111/j.1365-3040.2010.02253.x
Kim, T. H., Böhmer, M., Hu, H., Nishimura, N., and Schroeder, J. I. (2010). Guard cell signal transduction network: advances in understanding abscisic acid, CO2, and Ca2+ signaling. Annu. Rev. Plant Biol. 61, 561–591. doi: 10.1146/annurev-arplant-042809-112226
King, E. O., Ward, M. K., and Raney, D. E. (1954). Two simple media for the demonstration of pyocyanin and fluorescin. J. Lab. Clin. Med. 44, 301–307.
Kollist, H., Nuhkat, M., and Roelfsema, M. R. (2014). Closing gaps: linking elements that control stomatal movement. New Phytol. 203, 44–62. doi: 10.1111/nph.12832
Kouzai, Y., Kimura, M., Watanabe, M., Kusunoki, K., Osaka, D., Suzuki, T., et al. (2018a). Salicylic acid-dependent immunity contributes to resistance against Rhizoctonia solani, a necrotrophic fungal agent of sheath blight, in rice and Brachypodium distachyon. New Phytol. 217, 771–783. doi: 10.1111/nph.14849
Kouzai, Y., Noutoshi, Y., Inoue, K., Shimizu, M., Onda, Y., and Mochida, K. (2018b). Benzothiadiazole, a plant defense inducer, negatively regulates sheath blight resistance in Brachypodium distachyon. Sci. Rep. 8:17358. doi: 10.1038/s41598-018-35790-w
Kubota, M., and Abiko, K. (2000). Induced resistance in hypocotyl of cucumber by infection with Colletotrichum lagenarium in leaves. J. Gen. Plant Pathol. 66, 128–131. doi: 10.1007/PL00012933
Kunz, W., Schurter, R., and Maetzke, T. (1997). The chemistry of benzothiadiazole plant activators. Pestic. Sci. 50, 275–282. doi: 10.1002/(SICI)1096-9063(199708)50:4<275::AID-PS593>3.0.CO;2-7
Lawton, K. A., Friedrich, L., Hunt, M., Weymann, K., Delaney, T., Kessmann, H., et al. (1996). Benzothiadiazole induces disease resistance in Arabidopsis by activation of the systemic acquired resistance signal transduction pathway. Plant J. 10, 71–82. doi: 10.1046/j.1365-313X.1996.10010071.x
Lin, T., and Ishii, H. (2009). Accumulation of H2O2 in xylem fluids of cucumber stems during ASM-induced systemic acquired resistance (SAR) involves increased LOX activity and transient accumulation of shikimic acid. Eur. J. Plant Pathol. 125, 119–130. doi: 10.1007/s10658-009-9464-9
Mandal, B., Mandal, S., Csinos, A. S., Martinez, N., Culbreath, A. K., and Pappu, H. R. (2008). Biological and molecular analyses of the acibenzolar-S-methyl-induced systemic acquired resistance in flue-cured tobacco against tomato spotted wilt virus. Phytopathology 98, 196–204. doi: 10.1094/PHYTO-98-2-0196
Mori, I. C., Pinontoan, R., Kawano, T., and Muto, S. (2001). Involvement of superoxide generation in salicylic acid-induced stomatal closure in Vicia faba. Plant Cell Physiol. 42, 1383–1388. doi: 10.1093/pcp/pce176
Nakashita, H., Yoshioka, K., Yasuda, M., Nitta, T., Arai, Y., Yoshida, S., et al. (2002). Probenazole induces systemic acquired resistance in tobacco through salicylic acid accumulation. Physiol. Mol. Plant Pathol. 61, 197–203. doi: 10.1006/pmpp.2002.0426
Narusaka, Y., Narusaka, M., Horio, T., and Ishii, H. (1999). Induction of disease resistance in cucumber by acibenzolar-S-methyl and expression of resistance-related genes. Jpn. J. Phytopathol. 65, 119–122. doi: 10.3186/jjphytopath.65.116
Narusaka, Y., Narusaka, M., and Ishii, H. (2001). Searching by differential display for new genes implicated in induced disease resistance of cucumber treated with acibenzolar-S-methyl. J. Gen. Plant Pathol. 67, 207–211. doi: 10.1007/PL00013013
Návarová, H., Bernsdorff, F., Döring, A. C., and Zeier, J. (2012). Pipecolic acid, an endogenous mediator of defense amplification and priming, is a critical regulator of inducible plant immunity. Plant Cell 24, 5123–5141. doi: 10.1105/tpc.112.103564
Oostendorp, M., Kunz, W., Dietrich, B., and Staub, T. (2001). Induced disease resistance in plants by chemicals. Eur. J. Plant Pathol. 107, 19–28. doi: 10.1023/A:1008760518772
Park, P., Kurihara, T., Nita, M., Ikeda, K., Inoue, K., and Ishii, H. (2019). Application of energy-filtering electron microscopy (EFEM) for analysis of hydrogen peroxide and lignin in epidermal walls of cucumber leaves triggered by acibenzolar-S-methyl treatment prior to inoculation with Colletotrichum orbiculare. Plant Pathol. 68, 1624–1634. doi: 10.1111/ppa.13095
Prodhan, M. Y., Issak, M., Nakamura, T., Munemasa, S., Nakamura, Y., and Murata, Y. (2017). Chitosan signaling in guard cells requires endogenous salicylic acid. Biosci. Biotechnol. Biochem. 81, 1536–1541. doi: 10.1080/09168451.2017.1332979
Sakata, N., Ishiga, T., Saito, H., Nguyen, V. T., and Ishiga, Y. (2019). Transposon mutagenesis reveals Pseudomonas cannabina pv. alisalensis optimizes its virulence factors for pathogenicity on different hosts. PeerJ 7:e7698. doi: 10.7717/peerj.7698
Shah, J., and Zeier, J. (2013). Long-distance communication and signal amplification in systemic acquired resistance. Front. Plant Sci. 4:30. doi: 10.3389/fpls.2013.00030
Takahashi, F., Ochiai, M., Ikeda, K., and Takikawa, Y. (2013). Streptomycin and copper resistance in Pseudomonas cannabina pv. alisalensis (abstract in Japanese). Jpn. J. Phytopathol. 35.
Takahashi, F., and Shinozaki, K. (2019). Long-distance signaling in plant stress response. Curr. Opin. Plant Biol. 47, 106–111. doi: 10.1016/j.pbi.2018.10.006
Takikawa, Y., and Takahashi, F. (2014). Bacterial leaf spot and blight of crucifer plants (Brassicaceae) caused by Pseudomonas syringae pv. maculicola and P. cannabina pv. alisalensis. J. Gen. Plant Pathol. 80, 466–474. doi: 10.1007/s10327-014-0540-4
Thor, K., Jiang, S., Michard, E., George, J., Scherzer, S., Huang, S., et al. (2020). The calcium-permeable channel OSCA1.3 regulates plant stomatal immunity. Nature 585, 569–573. doi: 10.1038/s41586-020-2702-1
Tripathi, D., Jiang, Y. L., and Kumar, D. (2010). SABP2, a methyl salicylate esterase is required for the systemic acquired resistance induced by acibenzolar-S-methyl in plants. FEBS Lett. 584, 3458–3463. doi: 10.1016/j.febslet.2010.06.046
Uknes, S., Mauch-Mani, B., Moyer, M., Potter, S., Williams, S., Dincher, S., et al. (1992). Acquired resistance in Arabidopsis. Plant Cell 4, 645–656. doi: 10.1105/tpc.4.6.645
Wang, Y., Schuck, S., Wu, J., Yang, P., Döring, A. C., Zeier, J., et al. (2018). A MPK3/6-WRKY33-ALD1-pipecolic acid regulatory loop contributes to systemic acquired resistance. Plant Cell 30, 2480–2494. doi: 10.1105/tpc.18.00547
Wendehenne, D., Durner, J., Chen, Z., and Klessig, D. F. (1998). Benzothiadiazole, an inducer of plant defenses, inhibits catalase and ascorbate peroxidase. Phytochemistry 47, 651–657. doi: 10.1016/S0031-9422(97)00604-3
Wu, F., Chi, Y., Jiang, Z., Xu, Y., Xie, L., Huang, F., et al. (2020). Hydrogen peroxide sensor HPCA1 is an LRR receptor kinase in Arabidopsis. Nature 578, 577–581. doi: 10.1038/s41586-020-2032-3
Yasuda, M., Ishikawa, A., Jikumaru, Y., Seki, M., Umezawa, T., Asami, T., et al. (2008). Antagonistic interaction between systemic acquired resistance and the abscisic acid-mediated abiotic stress response in Arabidopsis. Plant Cell 20, 1678–1692. doi: 10.1105/tpc.107.054296
Zeier, J. (2013). New insights into the regulation of plant immunity by amino acid metabolic pathways. Plant Cell Environ. 36, 2085–2103. doi: 10.1111/pce.12122
Keywords: Acibenzolar-S-methyl, Pseudomonas cannabina pv. alisalensis, Japanese radish, stomatal-based defense, systemic acquired resistance, plant defense activator, peroxidase, reactive oxygen species
Citation: Sakata N, Ishiga T, Taniguchi S and Ishiga Y (2020) Acibenzolar-S-Methyl Activates Stomatal-Based Defense Systemically in Japanese Radish. Front. Plant Sci. 11:565745. doi: 10.3389/fpls.2020.565745
Received: 26 May 2020; Accepted: 07 October 2020;
Published: 30 October 2020.
Edited by:
Dirk Albert Balmer, Syngenta, SwitzerlandReviewed by:
Heriksen Puerari, Universidade Federal de Goiás, BrazilCopyright © 2020 Sakata, Ishiga, Taniguchi and Ishiga. This is an open-access article distributed under the terms of the Creative Commons Attribution License (CC BY). The use, distribution or reproduction in other forums is permitted, provided the original author(s) and the copyright owner(s) are credited and that the original publication in this journal is cited, in accordance with accepted academic practice. No use, distribution or reproduction is permitted which does not comply with these terms.
*Correspondence: Yasuhiro Ishiga, aXNoaWdhLnlhc3VoaXJvLmttQHUudHN1a3ViYS5hYy5qcA==
Disclaimer: All claims expressed in this article are solely those of the authors and do not necessarily represent those of their affiliated organizations, or those of the publisher, the editors and the reviewers. Any product that may be evaluated in this article or claim that may be made by its manufacturer is not guaranteed or endorsed by the publisher.
Research integrity at Frontiers
Learn more about the work of our research integrity team to safeguard the quality of each article we publish.