- 1Gene Discovery Research Group, RIKEN Center for Sustainable Resource Science, Tsukuba, Japan
- 2Gene Discovery Research Group, RIKEN Center for Sustainable Resource Science, Wako, Japan
- 3Laboratory of Plant Molecular Physiology, Graduate School of Agricultural and Life Sciences, The University of Tokyo, Bunkyo-ku, Japan
The drought stress responses of vascular plants are complex regulatory mechanisms because they include various physiological responses from signal perception under water deficit conditions to the acquisition of drought stress resistance at the whole-plant level. It is thought that plants first recognize water deficit conditions in roots and that several molecular signals then move from roots to shoots. Finally, a phytohormone, abscisic acid (ABA) is synthesized mainly in leaves. However, the detailed molecular mechanisms of stress sensors and the regulators that initiate ABA biosynthesis in response to drought stress conditions are still unclear. Another important issue is how plants adjust ABA propagation, stress-mediated gene expression and metabolite composition to acquire drought stress resistance in different tissues throughout the whole plant. In this review, we summarize recent advances in research on drought stress responses, focusing on long-distance signaling from roots to shoots, ABA synthesis and transport, and metabolic regulation in both cellular and whole-plant levels of Arabidopsis and crops. We also discuss coordinated mechanisms for acquiring drought stress adaptations and resistance via tissue-to-tissue communication and long-distance signaling.
Introduction
Environmental stresses have multiple effects on plant growth. Extreme stresses often inflict severe damage during the production of plant biomass. Environmental stress conditions change either rapidly or incrementally. Therefore, plants must recognize and respond to stress conditions with a variety of biological signals at appropriate times and speeds for their survival (Takahashi and Shinozaki, 2019). Higher plants achieve sophisticated responses and adaptations to abiotic stresses, including drought, to maintain optimal growth under stress conditions. For these complex physiological responses in plants, a variety of cellular and molecular regulatory mechanisms are required for short-term responses to prevent water loss via transpiration from guard cells and for long-term adaptations to acquire stress resistance at the whole-plant level (Nakashima et al., 2014; Takahashi et al., 2018a).
Abscisic acid (ABA) is a key phytohormone that mediates drought stress responses and resistance by regulating stomatal closure and stress-responsive gene expression (Addicott et al., 1968; Cutler et al., 2010). In response to drought stress conditions, ABA accumulation is enhanced mainly in the vasculature of the leaves because almost all ABA biosynthesis enzymes are expressed in vascular tissues (Kuromori et al., 2018). In addition, several cellular-membrane-located ABA transporters are predominantly expressed at vascular tissues. Although it is not completely understood how ABA is regulated to move in tissue-to-tissue manner, the vasculature and apoplastic areas among tissues are described as the typical ABA route for the systemic signaling in classical textbooks. Therefore, accumulated ABA is supposed to spread from the vasculature to all tissues to mediate stomatal movements and gene expression related to drought stress resistance.
In general, the environmental conditions in the soil and atmosphere are completely different under drought stress conditions. Plants recognize the change of water deficit conditions in the soil appropriately under drought stress and transmit the water deficit signal from the roots to the leaves to adapt to drought stress conditions through ABA accumulation. Drought stress responses are also regulated by both ABA-dependent and ABA-independent regulatory systems (Nakashima et al., 2014; Takahashi et al., 2018a). Unlike animals, plants do not have a central nervous system, but their vascular system connects the roots and shoots and plays important roles in integrating stress information from the underground and aerial parts of plants. Many studies have reported that hydraulic signals, electric currents, calcium waves, reactive oxygen species (ROS), mRNAs, and phytohormone movements mediate drought stress responses (Christmann et al., 2013; Notaguchi and Okamoto, 2015; Choi et al., 2017; Kudla et al., 2018). Recent studies reported novel roles of hormone-like peptides as signaling molecules that mediate drought stress responses (Takahashi et al., 2018b; Takahashi et al., 2019). These novel findings suggest that peptides function as mobile molecules in the plant vasculature for the integration of water deficit stress signals in long-distance organ-to-organ communication. Recently, the regulatory mechanisms of both intracellular and intercellular responses have been analyzed in phytohormone signaling and transport, stress-mediated gene expression and metabolite production during drought stress responses.
In this review, we summarize recent knowledge of how long-distance signaling, ABA transport and metabolic regulation mediate drought stress responses and resistance in Arabidopsis and crops. These findings imply that plants have developed unique and complex mechanisms that connect various organs to resist environmental stresses and optimize growth. A comprehensive understanding of plant responses to drought stress will help to elucidate the mechanism by which local and long-distance responses are integrated at the whole-plant level.
Long-Distance Communication in Drought Stress Responses and Resistance
Dehydration Responses in Leaves via Root-to-Shoot Signaling
Vascular plants have developed some tissue-to-tissue communication systems by which various organs transmit the information of environmental stress conditions (Figure 1). As for one example, the decreasing water potential status is an initial step that plants recognize dehydration stress conditions (Christmann et al., 2013). Plants perceive water deficit status in their roots and integrate the information among distant organs. The hydraulic change signal can transmit through the vasculature at a speed of 1 m/min. Previous report indicates that lycophytes and ferns regulate stomatal closure in an ABA-independent manner (Brodribb and McAdam, 2011). Vitis vinifera regulates stomatal closure primarily in response to water deficit conditions, and then accumulates ABA to maintain the status of closed stomata (Tombesi et al., 2015).
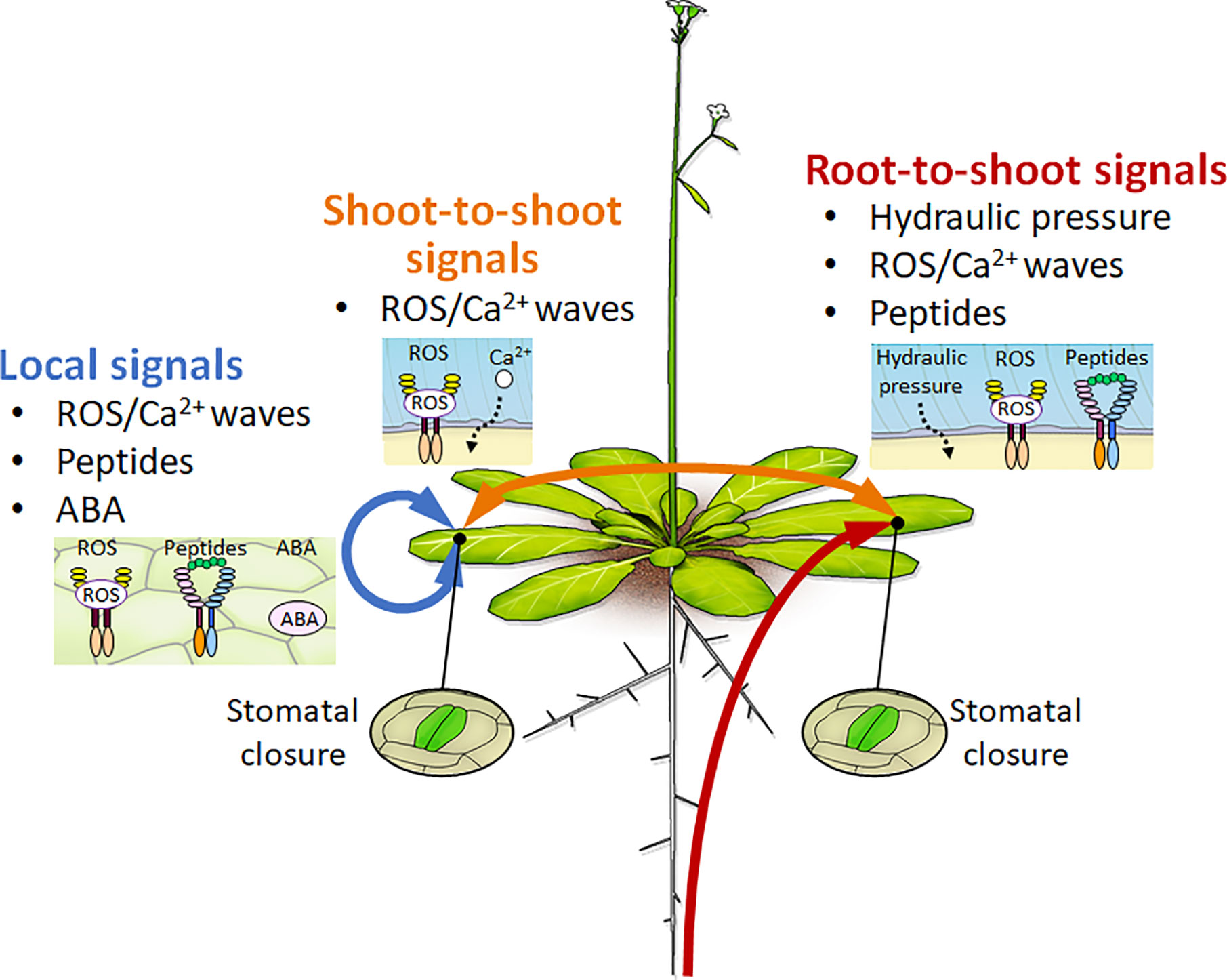
Figure 1 Conceptual diagram of root-to-shoot, shoot-to-shoot, and local signals in response to drought stress conditions. Mobile signals such as hydraulic pressure, ROS/Ca2+ waves, peptides, and phytohormones mediate tissue-to-tissue and long-distance communication for the acquisition of drought stress resistance at the whole-plant level. The red line indicates the root-to-shoot signals such as hydraulic pressure, ROS/Ca2+ waves, and peptides signals under dehydration stress conditions. The orange line indicates the shoot-to-shoot signals of ROS/Ca2+ waves to mediate stomatal closure under stress conditions. The blue line indicates the local signals of ROS/Ca2+ waves, peptide, or ABA signals that mediate stomatal control under stress conditions.
On the other hand, previous studies indicated that dehydrated plants accumulate ABA in their bodies for regulating stomatal closure (Munemasa et al., 2015). The gene for a key enzyme in ABA biosynthesis, NINE CIS EPOXYCAROTENOID DIOXYGENASE3 (NCED3), is highly expressed in the vasculature of dehydrated leaves (Iuchi et al., 2001; Endo et al., 2008). Grafting experiments showed that the accumulation of ABA is more important in the leaves than in the roots for regulating stomatal closure (Holbrook et al., 2002; McAdam et al., 2016). In addition, the hydraulic stress caused by turgor loss activates ABA biosynthesis in the leaves (Christmann et al., 2007). Recently, the CLAVATA3/EMBRYO-SURROUNDING REGION-RELATED25 (CLE25) peptide was shown to function as a root-derived long-distance signal for ABA production in the leaves (Christmann and Grill, 2018; McLachlan et al., 2018; Takahashi et al., 2018b; Yoshida and Fernie, 2018). Cysteine produced from sulfate can mediate the stomatal closure through the accumulation of ABA and ROS (Batool et al., 2018). These results indicate that plants regulate stomatal aperture via various signals that are generated at different times during prolonged dehydration stress conditions (Figure 1). Further analyses are needed to understand how plants recognize water-deficit conditions at each organ. In addition, spaciotemporal analyses will reveal detailed stepwise responses for integrating complex dehydration stress information into long-distance communication at the cellular, organ, and whole-plant levels.
CLE Peptides Mediate Drought Stress Responses in Long-Distance Signaling
Recent studies have suggested that some peptides mediate cell-to-cell and/or long-distance signaling to cover the phytohormones functions (Motomitsu et al., 2015; Notaguchi and Okamoto, 2015; Takahashi and Shinozaki, 2019). As a functional feature of these mobile peptides, the peptide-receptor modules in each tissue enable to transmit environmental stimuli precisely from the sensing tissues to the target tissues (Figure 1). Recent study reported that the CLE25 peptide modulates ABA biosynthesis to regulate stomatal closure during root-to-shoot signaling under dehydration stress conditions (Takahashi et al., 2018b). Root-derived CLE25 moves from the roots to the leaves and enhances ABA accumulation through the induction of NCED3 expression in the leaves. BARELY ANY MERISTEM (BAM) 1 and BAM3 Receptor-like Protein Kinases (RLKs) perceive CLE25 in the leaves. Therefore, the CLE25–BAM1 and BAM3 systems control ABA accumulation and responses, including stomatal closure and stress-inducible gene expression. These results indicate that plants can integrate the water deficit information into their roots and leaves and optimize stress adaptations in those tissues.
The NGATHA1 (NGA1) transcription factor mediates dehydration stress-induced NCED3 expression (Sato et al., 2018). The expression of NGA1 gene is observed in the leaf vasculature. This indicates a physiological relationship between the CLE25–BAM1 and BAM3 signaling systems and the NGA1 in the regulation of NCED3 expression.
cle25 mutants show a normal root growth phenotype. On the other hand, overexpression of a partial dominant-negative mutant of CLE25 (with a G6T change in the amino acid sequence) inhibited differentiation of the protophloem sieve element (Ren et al., 2019). Systematic analyses revealed that almost all CLE peptide genes are expressed in roots (Jun et al., 2010). Exogenous applications of synthetic CLE peptides inhibit root growth elongation (Fiers et al., 2005; Ito et al., 2006; Kinoshita et al., 2007), indicating that several CLE peptides, including CLE25, redundantly mediate the regulation of root differentiation and development. It is generally known that plants retard shoot and root growth to save energy effectively under environmental stress conditions and acquire stress resistance. Taken together, these reports on CLE25 show that the function of CLE25 in the leaves and roots may be advantageous in adaptation to drought stress conditions in higher plants.
Signaling Crosstalk for Stomatal Regulation
The acquisition of drought stress resistance occurs as a result of complex physiological signals, such as hydraulic, peptide, ABA, ROS, and calcium ion (Ca2+) current signals, in plants. ABA triggers an increase in Ca2+ currents through the activation of hydrogen peroxide (H2O2), which is a major component of ROS in guard cells (Hamilton et al., 2000; Pei et al., 2000).
Recently, HYDROGEN-PEROXIDE-INDUCED Ca2+ INCREASE (HPCA) was reported as a sensor of H2O2 in guard cells (Wu et al., 2020). HPCA1 belongs to subclass VIII-1 of Leucine-Rich-Repeat Receptor-Like Kinase (LRR-RLK), which is localized to the plasma membrane. The activation of HPCA1 occurs via the oxidization of two cysteine residue pairs that are present in the extracellular domain of HPCA1 by H2O2, which leads to the influx of Ca2+ currents in guard cells. As a result, hpca1 mutants impair ABA- and H2O2-induced stomatal closure, indicating that HPCA1 is a key component of H2O2 signaling for stomatal control in guard cells.
Excessive light stress rapidly regulates stomatal closure through changes in photosynthesis and transpiration in plants. Light stress enhances ABA accumulation and then causes the generation of ROS/Ca2+ waves through the RESPIRATORY BURST OXIDASE HOMOLOG D (RBOHD). Both light stress-induced ABA and ROS/Ca2+ mediate stomatal closure in local leaves (Devireddy et al., 2018). The ROS/Ca2+ wave that is generated by light stress propagates rapidly from local leaves to systemic leaves at a speed of 100 μm/s and, in turn, mediates the initiation of ABA and jasmonic acid (JA) biosynthesis. ROS/Ca2+ wave-generated ABA and JA mediate stomatal closure with the activation of GUARD CELL HYDROGEN PEROXIDE-RESISTANT1 (GHR1) and the S-type anion channel SLAC1 in systemic leaves. Therefore, local ROS/Ca2+ waves function as long-distance signals to regulate ABA responses and stomatal closure in systemic leaves under (Devireddy et al., 2018; Yoshida and Fernie, 2018; Kollist et al., 2019) (Figure 1).
Sensing Systems in Water Deficit Conditions
It is generally thought that membrane proteins, including RLKs, histidine kinases, and integrin-like proteins, function as osmotic stress sensors based on research in yeasts as well as in plants. Arabidopsis thaliana histidine kinase (ATHK) 1/Arabidopsis histidine kinase (AHK) 1 is localized at the plasma membrane of the cells and isolated as a homolog of the yeast two-component system in plants (Urao et al., 1999). ATHK1/AHK1 complements the function of SLN1 that encodes osmosensing histidine protein kinase in the yeast, indicating that ATHK1/AHK1 can acts as an osmotic stress sensor. Gain and loss of function of ATHK1/AHK1 showed that ATHK1/AHK1 mediates drought stress responses and resistance through ABA accumulation and drought stress-induced gene expression (Tran et al., 2007; Wohlbach et al., 2008). On the other hand, other T-DNA mutant lines of athk1 did not show a significant difference in ABA accumulation compared to that of control plants under low water potential and instead displayed an increased density of stomata (Kumar et al., 2013). Compared to the control phenotype, the morphological phenotype of athk1 mutants led to higher relative water loss from the leaves on soil under long-term drought stress conditions. The alteration of stomatal conductance caused by rapid changes in water potential did not mediate the ATHK1/AHK1 mutation (Sussmilch et al., 2017). Other ATHK/AHKs, such as AHK2, AHK3, and AHK4, mediate osmotic stress responses negatively through cytokinin signaling (Jeon et al., 2010; Nishiyama et al., 2011). ATHK/AHKs are intricately linked to both stress responses and optimal growth under drought stress conditions. Further analyses including the isolation of molecules directly downstream of ATHK/AHKs will help us understand ATHK/AHK signaling in plants.
Calcium channels are also thought to be possible osmosensor candidates in the drought stress response. The Ca2+ uptake channels, Ca2+-permeable mechanosensitive channel (MCA) 1 and MCA2, mediate optimal growth under stress conditions (Nakagawa et al., 2007; Yamanaka et al., 2010). The MCA1 and MCA2 act as sensors of cell wall tension in plants because MCA1 and MCA2 functionally complemented the lethal phenotype of the mid1 mutant in yeast. These results imply that MCA1 and MCA2 may mediate the perception of the change of hydraulic pressure caused by water potential under dehydration stress conditions.
Local Signals That Mediate Dehydration Stress Responses and Stomatal Control
The guard cell–expressed CLE9 gene was also reported to mediate dehydration stress responses (Zhang et al., 2019). Gain-of-function analyses indicated that CLE9 triggers both the activation of mitogen-activated protein kinase (MAPK) and the accumulation of ROS and enhances SLOW ANION CHANNEL ASSOCIATED1 (SLAC1) activity in guard cells to regulate stomatal closure. cle9 mutants show the dehydration sensitive phenotype. The CLE9-induced stomatal closure is not inhibited in the bam1 and bam3 mutants, indicating that some other RLKs mediate the CLE9 signaling in guard cells.
REDUCED HYPEROSMOLALITY INDUCED Ca2+ INCREASE1 (OSCA1) mediates osmotic stress-induced Ca2+ influx in guard cells and root cells (Yuan et al., 2014). osca1 mutants showed higher water loss than did control plants in response to osmotic stress treatment of the roots because the osca1 mutant did not regulate stomatal closure under osmotic stress conditions. The detached leaves of osca1 mutants also displayed enhanced water loss compared to that of control plants. On the other hand, ABA-induced stomatal closure occurred in the leaves of osca1 mutants as well as control plants, indicating that OSCA1 mediates stomatal closure only with osmotic stress-induced Ca2+ influx in guard cells (Figure 1). CALCIUM-PERMEABLE STRESS-GATED CATION CHANNEL1 (CSC1)/OSCA1.2 is a homolog in the OSCA family and has been reported as a hyperosmolality-gated calcium-permeable channel protein (Hou et al., 2014). CSC1/OSCA1.2 also mediated calcium fluxes in plant cells in response to osmotic stress conditions, although the detailed function of CSC1/OSCA1.2 in plant tissues remains unclear (Liu et al., 2018; Maity et al., 2019).
As a matter of course, ROS and hydroxyl radicals, turgor pressure and Ca2+ also mediate stomatal regulation to prevent water loss through local and/or long-distance signaling under drought stress conditions (Christmann et al., 2013; Kollist et al., 2019). These results indicate that the mechanisms by which osmotic stress is perceived in plants may be complicated and that spatiotemporal responses precisely transmit information to tissues throughout the whole plant.
ABA Transporters in Plants
ABA is a mobile signal in plants. Membrane transporters are important regulators of the intercellular networks of signaling molecules across cellular membranes. Certain kinds of membrane transporters have actually been reported as ABA membrane transporters in recent decades (Boursiac et al., 2013; Kuromori et al., 2018). Selected transporter families and protein members described in this section are listed in Table 1. Various membrane proteins function in ABA transport in different tissues, which indicates that complex systems are involved in ABA transport in plant physiology.
ABC Transporter Family
Several ABA transporters reported to date belong to the ATP-binding cassette (ABC) transporter family. ABC transporters are highly conserved in most organisms, and terrestrial plants have two to four times more ABC transporter genes in their genomes than do animals, suggesting that some ABC transporters may have plant-specific functions in development and physiological regulation (Hwang et al., 2016; Do et al., 2018). Soon after the discovery that cytosolic ABA receptors are located inside of cells in Arabidopsis thaliana (Ma et al., 2009; Park et al., 2009), two ABC transporters, AtABCG25 and AtABCG40, were identified as ABA transporters (Kang et al., 2010; Kuromori et al., 2010). Both of them were originally found in Arabidopsis tagged-mutant screening approaches based on ABA-related phenotypes. The AtABCG25 promoter was predominantly active in the vascular tissue, and the AtABCG40 promoter had the highest expression in guard cells. Both AtABCG25 and AtABCG40 localized to the plasma membrane when expressed as fluorescent fusion proteins in plant cells. An additional study showed that plasma membrane localization of AtABCG25 was regulated by abiotic stress and ABA (Park et al., 2016). Experimental assays of the ABA transport activity of each transporter finally revealed that AtABCG25 mediates ABA export from the cell and AtABCG40 mediates ABA import into the cell. This is consistent with distant ABA mobility and supports the hypothesis that ABA may serve as a mobile signal between vascular tissues and epidermal tissues, including guard cells, to control stomatal appearance (Kuromori and Shinozaki, 2010; Kuromori et al., 2014). AtABCG22 is a closely related member of the same subfamily that was reported to be involved in stomatal closure in Arabidopsis. AtABCG22 is strongly expressed in guard cells. However, its transporter activity has not yet been elucidated (Kuromori et al., 2011).
In addition to stomatal regulation, ABA also substantially controls germination arrest in seeds. AtABCG31 and AtABCG30, as well as AtABCG25 and AtABCG40 described above, were shown to serve as ABA exporters and importers, respectively, in Arabidopsis seeds (Kang et al., 2015). In mature seeds, ABA is released from the endosperm toward the embryo to repress seed germination (Lee et al., 2012). AtABCG31 was expressed at high levels and AtABCG25 was mainly expressed in the endosperm; in contrast, AtABCG30 and AtABCG40 were strongly expressed in dissected embryos. Additionally, plasma membrane localization of GFP-fusion proteins of AtABCG31 and AtABCG30 was shown in Arabidopsis mesophyll protoplasts. The absence of these transporters in knockout mutants resulted in disruption of the ABA distribution within seeds and a shortened germination time. These four ABC transporters act in concert to deliver ABA from the endosperm to the embryo to control germination in Arabidopsis; AtABCG25 and AtABCG31 export ABA from the endosperm, whereas AtABCG30 and AtABCG40 import ABA into the embryo (Kang et al., 2015).
More recently, the identification of ABA membrane transporters in the ABC transporter family has been extended to other plant species beyond the model plant Arabidopsis thaliana. For example, in Medicago truncatula, MtABCG20 was reported as an ABA transporter (Pawela et al., 2019). This gene was initially identified as strongly inducible under drought stress-mimicking conditions or ABA treatment. MtABCG20 was expressed at the hypocotyl-radicle transition zone in seeds and along vascular bundles, lateral root (LR) primordium sites and nodules in roots. Consistent with these expression sites, mtabcg20 mutants were more sensitive to ABA upon germination of seeds. Furthermore, the mutants had two root phenotypes: fewer LRs and more nodules than wild-type roots under drought stress-mimicking conditions. While ABA signaling largely inhibits LR development in Arabidopsis, ABA primarily plays a positive role in LR development in Medicago truncatula (Gonzalez et al., 2015). Nodulation is a unique process in legumes, and ABA acts as a negative regulator of nodule primordium formation in the root cortex tissue (Ding et al., 2008). The mtabcg20 mutant phenotypes might suggest that ABA accumulates at its place of biosynthesis as a consequence of impaired ABA transport. Actually, ABA export activity of MtABCG20 was shown by a transport assay using MtABCG20-overexpressing Nicotiana tabacum BY2 cells. Additionally, MtABCG20 showed subcellular localization at the plasma membrane via homodimer formation in Arabidopsis mesophyll protoplasts. Together, these data indicate that MtABCG20 is an ABA transporter that influences root morphology and nodulation in Medicago truncatula (Pawela et al., 2019).
In legumes, another ABC transporter encoded by the Arachis hypogaea ABA transporter-like 1 (AhATL1) gene has been reported as a potential ABA transporter (Ge et al., 2017). In peanut plants, AhATL1 was upregulated by water stress and exogenous ABA treatment. Overexpression of AhATL1 in Arabidopsis decreased ABA sensitivity and drought resistance by inhibiting drought-induced AtABCG40 expression in guard cells. While the ABA transport activity of AhATL1 has not yet been shown, the function of AhATL1 was postulated to modulate ABA sensitivity by specifically influencing ABA import into cells (Ge et al., 2017).
An ABA membrane transporter in the ABC transporter family has also been reported in monocot plants, including important agricultural crops. For example, in Triticum aestivum, Lr34 was identified as an ABA transporter (Hulbert et al., 2007). This factor was initially identified as a gene that confers durable adult plant resistance against multiple fungal diseases (Ellis et al., 2014). In the wheat gene pool, the Lr34 gene was present in two different alleles, called Lr34sus and Lr34res, which were characterized by their susceptible and resistant phenotypes, respectively, to biotrophic fungal pathogens. Lr34res-responsive genes in wheat have been associated with ABA inducibility (Hulbert et al., 2007). In addition, in Lr34res-expressing transgenic rice plants, Lr34res induced transcripts reminiscent of an ABA-regulated stress response. Lr34res-expressing transgenic rice plants also showed alterations in biological processes that are controlled by ABA (Hulbert et al., 2007). In transgenic rice seedlings, increased ABA accumulation was specific to the Lr34res-expressing lines, which correlated with the induction of ABA-regulated genes, physiological alterations, and disease resistance. In vitro yeast accumulation assays demonstrated that the Lr34res protein resulted in increased ABA uptake in yeast cells, suggesting that LR34res might act as an ABA importer. Because of its durability and broad-spectrum specificity, the Lr34 gene has been applied as one of the most frequently used disease resistance genes in wheat breeding. Moreover, Lr34res has been shown to be functionally transferrable as a transgene in all major cereals, including barley, rice, maize, and sorghum (Hulbert et al., 2007).
NPF, MATE, and Other Transporter Families
In addition to the ABC transporter family, several ABA transporters in different families have been reported thus far. For example, Arabidopsis NPF4.6, which was originally named ABA-IMPORTING TRANSPORTER1 (AIT1), and several members of the NITRATE TRANSPORTER 1/PEPTIDE TRANSPORTER FAMILY (NPF) have been identified as ABA transporters (Kanno et al., 2012; Chiba et al., 2015; Tal et al., 2016; Leran et al., 2020). NPF4.6 and related family members were successfully identified using a modified yeast two-hybrid system screen of Arabidopsis cDNAs to identify proteins capable of inducing interactions between the ABA receptor PYR/PYL/RCAR and the PP2C protein phosphatase under low ABA concentrations in yeast cells. Overall, transport assays in insect cells expressing NPF4.6 demonstrated that this transporter mediated cellular ABA uptake into cultured cells (Kanno et al., 2012). Fluorescent protein-fused NPF4.6 proteins were detected predominantly at the plasma membrane. Less sensitivity to exogenously applied ABA during seed germination and initial growth in seedlings was observed in npf4.6 mutants than in wild-type plants. In contrast, overexpression of NPF4.6 resulted in ABA hypersensitivity in seeds and during the initial stages of growth. In adult plants, the inflorescence stems of npf4.6 mutants had a lower surface temperature than did those of wild-type plants. NPF4.6 promoter activity was detected in imbibed seeds and vascular tissues of cotyledons, true leaves, hypocotyls, roots, and inflorescence stems using a promoter-reporter system. These data suggest that the function of NPF4.6 as an ABA importer in vascular regions affects the regulation of stomatal aperture in shoots (Kanno et al., 2012).
NPF transporters have been functionally characterized as nitrate transporters, but many members may also act as dual-affinity transporters (Chiba et al., 2015). One of the NPF members of Medicago truncatula, MtNPF6.8, has been reported to be involved in nitrate-mediated inhibition of primary root growth in a manner dependent on ABA signaling (Pellizzaro et al., 2014). In addition to nitrate influx activity, MtNPF6.8 was detected to have ABA uptake activity, although at a low rate, in the Xenopus oocyte assay, suggesting an additional role in ABA translocation as well as nitrate relocation (Pellizzaro et al., 2014).
In another transporter family, Arabidopsis thaliana DETOXIFICATION EFFLUX CARRIER 50 (AtDTX50), which belongs to the multidrug and toxin efflux transporter (MATE) family, has also been identified as an ABA transporter in Arabidopsis (Zhang et al., 2014). This gene was first found in a comprehensive reverse genetic study of MATE transporters in Arabidopsis. A mutant defective in AtDTX50 exhibited growth defects, possibly due to its higher sensitivity to ABA or higher endogenous ABA levels. Fluorescence-tagged AtDTX50 proteins were localized predominantly to the plasma membrane, and AtDTX50 facilitated ABA efflux from inside to outside of cells in Escherichia coli and Xenopus oocytes. AtDTX50 was preferentially expressed in vascular tissues, indicating that it might regulate ABA export from vascular tissues in Arabidopsis, possibly in association with AtABCG25 and NPF4.6 because of their similar expression sites. On the other hand, AtDTX50 was also expressed in guard cells, and the atdtx50 mutant plants were more tolerant to drought, with lower stomatal conductance. These data indicate that AtDTX50 might modify ABA transport in both vascular cells and guard cells (Zhang et al., 2014).
In the Arabidopsis MATE transporter family, AtMATE45 has been reported as a candidate ABA transporter (Kovinich et al., 2018). AtMATE45 was first identified as one of four transporter genes found by genetic screening to observe anthocyanin pigmentation under anthocyanin induction conditions (AICs). Anthocyanin pigmentation related to ABA levels and ABA signaling has been demonstrated (Adams et al., 1999). Related to another ABA transporter described above in the ABC family, AtABCG25 was also included among four transporter genes found by genetic screening in AICs (Kovinich et al., 2018). AtMATE45 is mostly expressed at growing meristem sites and in the vasculature in Arabidopsis. The atmate45 mutant exhibited delayed germination of seeds, reduced rosette biomass, increased numbers of inflorescence stems, and shorter siliques. These phenotypes were ABA-dependent but not due to the anthocyanin level, as shown by the double-mutant analysis. The observation of GFP-fusion proteins with subcellular markers and BFA treatment, which blocks vesicle trafficking, revealed that AtMATE45 was localized to the subcellular trans-Golgi network (TGN) and Golgi apparatus. The subcellular localization of AtMATE45 is extremely unique because every other ABA transporter referred to in this article is a plasma membrane protein. Slight but significant ABA uptake activity of AtMATE45 was detected in E. coli cells expressing AtMATE45. These results suggest that AtMATE45 may antagonize meristematic ABA signaling, although it is unlikely to function as a bona fide ABA transporter (Kovinich et al., 2018).
Additionally, a novel type of membrane protein has been reported as a rice ABA transporter (Yao et al., 2018). Oryza sativa PLASMA MEMBRANE PROTEIN 1 (OsPM1) belongs to a protein family named ABA-induced Wheat Plasma Membrane polypeptide-19 (AWPM-19), whose functions were previously unknown. OsPM1 showed strong induction in response to drought in all developmental stages. The promoter-GUS transgenic rice showed that the gene was expressed weakly in vascular tissues, guard cells, and mature embryos under normal conditions and strongly induced by ABA at all these sites. The ospm1 knockout mutants and knockdown RNAi lines showed greater drought sensitivity than did wild-type plants. By contrast, the OsPM1-overexpression lines showed an obvious drought resistance phenotype, and their survival rates were much higher than those of wild-type plants under water stress conditions, probably because OsPM1 might be involved in ABA-induced stomatal closure. Furthermore, many ABA-responsive genes were upregulated more in the OsPM1-overexpression lines but less in the RNAi lines compared to wild-type plants (Yao et al., 2018). The YFP-OsPM1 fusion proteins clearly appeared in the plasma membrane portion but not in the cytoplasm. The yeast cells expressing OsPM1 accumulated more ABA than the control cells, and ABA substrate specificity and pH dependency were observed. Consistently, a FRET sensor plasmid (Jones et al., 2014) revealed that yeast cells expressing OsPM1 showed more ABA uptake due to stronger induced fluorescence changes than cells transformed with the empty vector control (Yao et al., 2018). All these data indicate that OsPM1 has a physiological function as an ABA influx transporter and plays a significant role in drought responses.
Metabolic Regulation Mediating Drought Stress Responses and Resistance
The metabolic profiles of plants change in response to environmental stress factors such as drought, high salinity, and temperature (Urano et al., 2010; Cramer et al., 2011) (Figure 2). Metabolic profiles have been analyzed to study the function of the plant environmental stress response and tolerance (Levitt, 1972; Bartels and Sunkar, 2005; Schauer and Fernie, 2006; Sweetlove et al., 2008). It has been proposed that soluble sugars and other charged metabolites, such as proline and glycine-betaine, accumulate throughout the plant body, and function as compatible solutes during stress responses. Stress-induced accumulation of these metabolites lowers the water potential of the cell, promoting water retention in the plant without interfering with normal metabolism. This process, known as osmotic adjustment, enables the maintenance of cell turgor for plant growth and survival under stress conditions (Bartels and Sunkar, 2005; Verslues and Sharma, 2010; Krasensky and Jonak, 2012). In addition, these compatible solutes stabilize proteins and cell structures, particularly when osmotic stress becomes severe or persists for longer periods (Hoekstra et al., 2001). These metabolites also act as free radical scavengers, protecting against oxidation by removing excess ROS and reestablishing the cellular redox balance (Couee et al., 2006; Miller et al., 2010). On the other hand, specialized/secondary metabolites such as flavonoids have defense functions against both biotic and abiotic stress (Dixon and Paiva, 1995). Major flavonoids, such as flavonols (kaempferols and quercetins) and anthocyanins (cyanidins), increase in response to drought stress to enhance stress tolerance (Nakabayashi et al., 2014a; Nakabayashi et al., 2014b). These specialized compounds also act as free radical scavengers to mitigate oxidative and drought stress in plants (Figure 2).
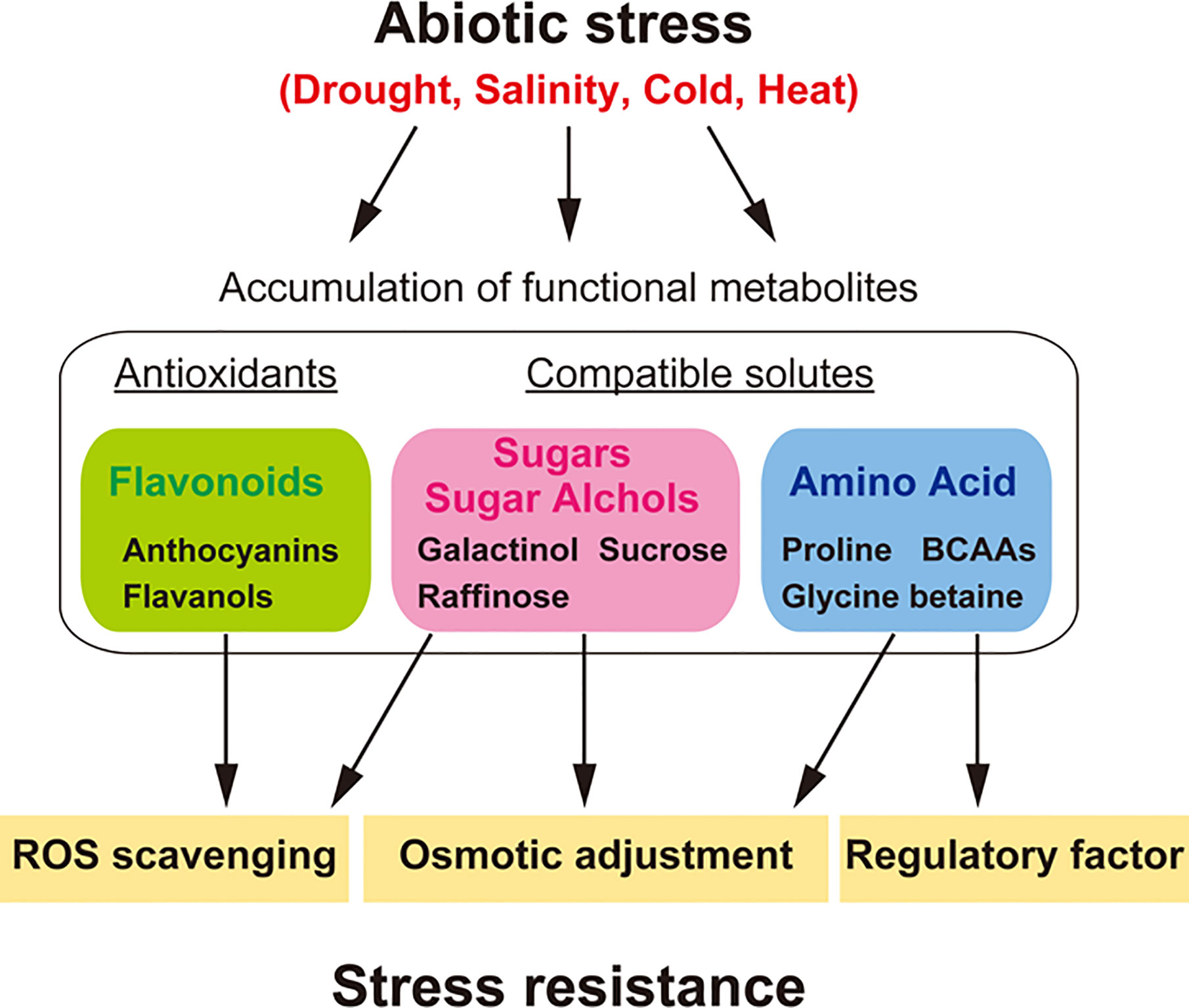
Figure 2 Metabolites and their functions in abiotic stress tolerance, especially under drought, salinity, cold and heat stress. Plants show a variety of metabolic responses to diverse abiotic stresses. Stress-induced accumulation of compatible solutes such as sugars (e.g., raffinose and sucrose), sugar alcohols (e.g., galactinol), and amino acids (e.g., BCAAs, proline, and glycine-betaine) functions in osmotic adjustment, enabling the maintenance of cell turgor for plant growth and survival under stress conditions. These compatible solutes, especially galactinol and raffinose, can act as free radical (ROS) scavengers, protecting against oxidation by removing excess ROS and reestablishing the cellular redox balance. BCAAs can act as regulatory factors in the production of specialized/secondary metabolites as a defense response against biotic stress during abiotic stress. Stress-induced accumulation of antioxidants such as flavonoids (e.g., anthocyanins and flavonols) allows them to act as free radical scavengers to mitigate oxidative and drought stress in plants. Genes involved in metabolite synthesis in diverse abiotic stress responses are useful for application in the metabolic engineering of stress resistance in dry field conditions.
Since 1998, the development of metabolomics technologies using highly sensitive mass spectrometry (MS), nuclear magnetic resonance (NMR), and bioinformatics has enhanced the comprehensive analysis of diverse plant metabolites and the changes in their profiles that occur in response to environmental stress factors (Rizhsky et al., 2002; Cook et al., 2004; Hirai et al., 2004; Kaplan et al., 2004; Gong et al., 2005; Kaplan et al., 2007; Brautigam et al., 2009; Maruyama et al., 2009; Tschoep et al., 2009; Urano et al., 2009; Wulff-Zottele et al., 2010; Caldana et al., 2011; Kusano et al., 2011; Krasensky and Jonak, 2012). Integrated analysis of transcriptome and metabolome analyses in Arabidopsis is important not only for the comprehensive analysis of metabolic networks but also for the analyses of specific regulatory networks in stress-related metabolism, especially ABA-dependent and independent pathways (Urano et al., 2009; Urano et al., 2010; Cramer et al., 2011). Here, we focus on recent progress in the characterization of metabolic responses in environmental stress and the application of metabolic approaches for drought-tolerant crops in the field.
Integrated Metabolomics Reveals the ABA-Dependent and ABA-Independent Metabolic Networks in Response to Abiotic Stress
Integrated analyses of the transcriptome and the metabolome in Arabidopsis successfully demonstrated connections between genes and primary metabolites, elucidating a wide range of signal outputs from ABA under dehydration (Urano et al., 2009). Metabolite profiling by GC/MS and CE/MS analyses revealed that ABA accumulates during dehydration, regulating the accumulation of various amino acids and sugars such as glucose and fructose. In particular, the dehydration-inducible accumulation of branched-chain amino acids (BCAAs), saccharopine, proline, and agmatine is correlated with the dehydration-inducible expression of their key biosynthetic genes (branch-chain aminotransferase 2; AtBCAT2, lysine ketoglutarate reductase/saccharopine dehydrogenase; AtLKR/SDH, delta 1-pyrroline-5-carboxylase 1; AtP5CS1, and arginine decarboxylase 2; and AtADC2, respectively), which are regulated by endogenous ABA (Urano et al., 2009).
In addition, analyses of the metabolic profiles of mutants of core regulators of ABA signaling, bZIP transcription factor ABA-RESPONSIVE ELEMENT BINDING PROTEIN/ABA-RESPONSIVE ELEMENT BINDING FACTOR (AREB/ABF) and subclass III SNF1-related protein kinase 2 (SnRK2s), could provide more detailed and significant information about the ABA-regulated metabolome in the context of plant stress tolerance and growth (Thalmann et al., 2016; Yoshida et al., 2019). The metabolic profile of starch degradation in mutants for ABA biosynthesis and signaling revealed that the ABA-subclass III SnRK2-AREB/ABF signaling pathway is important for the transcriptional regulation of α-AMYLASE3 and β-AMYLASE1 to release sugars and sugar-derived compatible solutes from starch degradation in response to osmotic stress (Thalmann et al., 2016). The subclass III SnRK2 signaling pathway is important for the modulation of organic acid and amino acid metabolism and leaf growth under nonstress conditions by fine-tuning flux through the tricarboxylic acid (TCA) cycle (Yoshida et al., 2019).
On the other hand, metabolome analysis of transgenic Arabidopsis overexpressing AtDREB1A/CBF3 revealed an ABA-independent metabolic network that is strikingly similar to the low-temperature regulated metabolome (monosaccharides, disaccharides, oligosaccharides, and sugar alcohols) and is regulated by the transcription factor AtDREB1A/CBF3 (Cook et al., 2004; Maruyama et al., 2009). In particular, the low-temperature-inducible accumulation of galactinol and raffinose is correlated with the expression of the galactinol synthase 3 (AtGols3) gene, which is a direct target of AtDREB1A/CBF3 (Cook et al., 2004; Maruyama et al., 2009). Maruyama et al. also analyzed AtDREB2A overexpression, which did not increase the level of any low-temperature regulated metabolites in transgenic plants. Overexpression of AtDREB2A in transgenic plants increased their tolerance to dehydration stress but only slightly increased their tolerance to freezing stress (Sakuma et al., 2006). These results indicate that the increased tolerance to freezing stress in transgenic plants overexpressing AtDREB1A may depend on the accumulation of low-temperature regulated metabolites, especially sucrose, raffinose, galactinol, and myo-inositol. Similarly, transcriptomics and metabolomics analyses of the PSEUDO RESPONSE REGULATOR (PRR) arrhythmic triple mutant revealed that the AtDREB1A/CBF gene and raffinose accumulation are regulated by the circadian clock in anticipation of colder night temperatures (Fukushima et al., 2009).
Comprehensive Overview of Metabolic Profiles in Environmental Stress Responses
Metabolic profiling of Arabidopsis under various environmental conditions, such as dehydration, salinity, heat, cold, light, and nutrient limitation, enables us to both obtain a comprehensive overview of the metabolic network and understand crucial components involved in the response to abiotic stress. The metabolites that respond to various stresses can act as compatible solutes and play a role in fundamental abiotic stress tolerance. A stress-specific response at the metabolite level can be the result of transient inhibition or activation of a specific metabolic pathway due to the properties of enzymes under stress conditions, such as temperature, oxidation, and ion concentrations (Obata and Fernie, 2012). As expected, some metabolites, such as pyruvate- (alanine, isoleucine, leucine, and valine), oxaloacetate- (asparagine, lysine, methionine, and threonine), amine-containing metabolites (β-alanine, GABA, and putrescine), and carbohydrates (galactinol, maltose, sucrose, trehalose, and raffinose), were reported to increase in response to various abiotic stresses in metabolome analyses (Rizhsky et al., 2002; Cook et al., 2004; Hirai et al., 2004; Kaplan et al., 2004; Gong et al., 2005; Kaplan et al., 2007; Brautigam et al., 2009; Maruyama et al., 2009; Tschoep et al., 2009; Urano et al., 2009; Wulff-Zottele et al., 2010; Caldana et al., 2011; Kusano et al., 2011; Krasensky and Jonak, 2012). Metabolome analyses showed that a variety of primary metabolites act collectively as compatible solutes. They are very soluble in water and nontoxic at high concentrations, and they function to sustain the ordered vicinal water around proteins by decreasing protein-solvent interactions at low water activities (Bartels and Sunkar, 2005). The combination of compatible solutes exerts additive or synergistic effects under abiotic stress. In addition, these metabolites act as not only compatible solutes but also signaling molecules, antioxidants, or defenses against pathogens (Kaplan et al., 2004).
Although proline and carbohydrates have been characterized as functional metabolites under osmotic stress (Yoshiba et al., 1995; Nanjo et al., 1999; Taji et al., 2002; Li et al., 2011), metabolomics studies have contributed to the identification of novel groups of metabolites that generally accumulate in response to various stress conditions. In particular, BCAAs, such as isoleucine, leucine, and valine, were found to show ABA-dependent regulation at the transcript level under dehydration stress (Urano et al., 2009) and to function as compatible solutes due to their high fold increases in various plant tissues (Joshi et al., 2010; Obata and Fernie, 2012). BCAAs also serve as precursors for cyanogenic glycosides (Vetter, 2000). The accumulation of BCAAs supports the increased production of secondary metabolites as a defense response against biotic stress during abiotic stress. Such stress responses function as preemptive defense responses against pathogen attack on stressed host plants (Figure 2).
Proline is known as a major compatible solute in various plants under environmental stresses (Bartels and Sunkar, 2005; Verslues and Sharma, 2010; Krasensky and Jonak, 2012). However, comparison of various metabolome analyses under abiotic stress revealed that proline has been reported in a limited number of studies on plant abiotic stress responses (Obata and Fernie, 2012). Therefore, proline is thought to mainly function in response to osmotic stress. This was supported by the expression of key genes for proline biosynthesis in Arabidopsis, delta 1-AtP5CS1 and AtP5CS2. Although AtP5CSs are induced by cold, dehydration, mannitol, and salt treatments (Yoshiba et al., 1995; Knight et al., 1997; Urano et al., 2009), they are not altered by heat, methyl viologen, and UV-B treatments in the Arabidopsis eFP Browser (https://bar.utoronto.ca/efp/cgi-bin/efpWeb.cgi) (Winter et al., 2007). Several studies have suggested that the intermediate of proline biosynthesis, delta 1-pyrroline-5-carboxylate (P5C), is toxic to plant cells (Hellmann et al., 2000; Deuschle et al., 2001; Mani et al., 2002). Because proline biosynthesis and degradation occur in mitochondria, the accumulation of toxic compounds such as P5C may cause damage to plant cells under severe abiotic stress (Rizhsky et al., 2002; Rizhsky et al., 2004). Plants are subjected to complex abiotic stress conditions in their natural habitats. Metabolite profiling of Arabidopsis responses to combined dehydration and heat stress (Rizhsky et al., 2004) has revealed that proline shows the highest accumulation among all metabolites under dehydration stress. On the other hand, sucrose showed the highest accumulation under combined dehydration and heat stress. These results revealed that sucrose replaces proline in plants as the major compatible solute during severe abiotic stress with both osmotic and oxidative damage. Sucrose, glucose, and fructose contents are distributed throughout the plant body upon exposure to drought stress in soybean (Maruyama et al., 2020). In contrast, other sugars and related polyol, raffinose, galactinol, and trehalose were highly accumulated in roots compared with those in shoots under drought stress. Pinitol and ononitol that are polyols detected in legume were specifically accumulated in drought-treated leaves of soybean. Distribution of sugars in plant is altered in response to drought stress, and function as a compatible solute in various organs. In additions, sugars such as sucrose, glucose, and trehalose also act as signal molecules to regulate gene expression in plant growth and stress response (Maruyama et al., 2014). To improve plant tolerance under field conditions, enhancement of the synthetic pathways of metabolites such as raffinose and/or sucrose is thought to be useful for metabolic engineering of drought tolerance, as shown in the next section.
Metabolic Engineering Approaches Have Contributed to the Development of Stress-Tolerant Crops in the Field
The development of drought-tolerant crops seems to be a promising solution to increase crop yield under water-limited conditions to fulfill the food requirements of increasing world populations (Soni et al., 2015). Drought stress has often caused significant decreases in crop production, which is caused by continuous global warming. Enhancing drought tolerance without a grain yield penalty has been a great challenge in crop improvement. Many genes have been tested in greenhouses, but few of them have proven to be useful in the field. Due to the complexity of stress conditions in the field, the enhancement of stress-related metabolites with no effect on plant growth seems to be a good strategy for the metabolic engineering of drought tolerance.
Recently, engineering of the raffinose biosynthetic pathway was shown to be successful in breeding drought stress tolerance in dry field conditions (Honna et al., 2016; Selvaraj et al., 2017). Arabidopsis genes for raffinose biosynthesis were identified by Taji et al., 2002. Galactinol synthase (AtGolS) is a key gene for galactinol and raffinose accumulation under drought, heat, and cold stress conditions. Among them, the AtGolS2 gene is specifically induced by drought stress. The expression of AtGolS2 was also increased under oxidative stress and regulated by heat-shock transcription factor A2 (AtHsfA2) (Nishizawa et al., 2008). Overexpression of AtGolS2 in transgenic Arabidopsis and Brachypodium caused an increase in galactinol and raffinose levels, improved drought stress tolerance (Taji et al., 2002; Himuro et al., 2014) and protected plants from oxidative stress (Nishizawa et al., 2008). These findings support that galactinol and raffinose have a strong ability to protect cells as compatible solutes and ROS scavengers under several types of environmental stresses, such as dry field conditions. Metabolome analyses in rice and soybean revealed that galactinol and raffinose levels were increased in response to dehydration stress (Maruyama et al., 2014; Maruyama et al., 2020). The application of AtGolS2 for rice and soybean transformation has been attempted to validate stress responses and phenotypes under dry field conditions (Honna et al., 2016; Selvaraj et al., 2017). Overexpression of AtGolS2 in transgenic rice and soybean not only improved drought tolerance but also increased grain yield in dry field conditions (Honna et al., 2016; Selvaraj et al., 2017). These reports showed that metabolic engineering of AtGolS2 is a useful biotechnological tool to reduce grain yield losses under drought stress in the field (Figure 2).
Conclusions and Future Perspectives
Plants have evolved to respond and adapt properly to drought stress conditions through spatiotemporal and stepwise signals in tissues throughout the whole plant to acquire drought stress resistance. The phytohormone ABA regulates drought stress responses and resistance at the cellular and intercellular levels in plants. However, it remains unclear how plants perceive drought stress conditions and transmit that information into the cell to regulate ABA accumulation for the acquisition of drought stress resistance. Regulatory factors involved in tissue-to-tissue communication and long-distance signaling have been focused recently because these mobile molecules complement the movement of ABA from the synthesis tissues to the target tissues. There are ABA receptors in almost cells. On the other hand, the receptors that bind with peptides are expressed specifically in each tissue. These diverse expressions allow the peptide-receptor modules to transmit the information of environmental conditions accurately from the sensing tissues to the target tissues than does the ABA regulatory system. Advances in molecular biology have improved our understanding of not only cellular signaling and gene expression but also intercellular communication and long-distance integration of stress signals as a whole system during abiotic stress responses. Systematic knowledge of drought stress responses in whole plants can provide novel insights for agriculture and biotechnology. The critical information on drought stress resistance mechanisms merits further research for future crop innovation. For this purpose, phenomics analysis will be important in addition to other omics analyses to measure whole-plant phenotypes under stress conditions. Data science and imaging technology are powerful tools for the integration of these complex data to understand the complex systems underlying plant responses to environmental changes as a whole.
Author Contributions
FT and KS designed work. FT, TK, KU, KY-S, and KS collected information from literature and wrote the manuscript. All authors contributed to the article and approved the submitted version.
Conflict of Interest
The authors declare that the research was conducted in the absence of any commercial or financial relationships that could be construed as a potential conflict of interest.
Acknowledgments
We apologize to the authors whose work could not be cited due to space limitations. This work was supported by the Project of the NARO Bio-oriented Technology Research Advancement Institution under Grant Number 29002A (to FT), by JSPS KAKENHI Grant Numbers JP18H04792 (to FT), JP19H03255 (to FT), JP17K07458 (to TK), JP15H05960 (to KY-S), and JP18H03996 (to KY-S), by research grants from the Toray Science Foundation (to FT), by The Research Grant against Global Warming of the Ichimura Foundation for New Technology (to TK), and by the Fuji Foundation for Protein Research (to TK).
References
Adams, M. A., Chen, Z., Landman, P., Colmer, T. D. (1999). Simultaneous determination by capillary gas chromatography of organic acids, sugars, and sugar alcohols in plant tissue extracts as their trimethylsilyl derivatives. Anal. Biochem. 266 (1), 77–84. doi: 10.1006/abio.1998.2906
Addicott, F. T., Lyon, J. L., Ohkuma, K., Thiessen, W. E., Carns, H. R., Smith, O. E., et al. (1968). Abscisic Acid: a New Name for Abscisin 2 (Dormin). Science 159 (3822), 1943. doi: 10.1126/science.159.3822.1493
Bartels, D., Sunkar, R. (2005). Drought and Salt Tolerance in Plants. Crit. Rev. Plant Sci. 24, 23–58. doi: 10.1080/07352680590910410
Batool, S., Uslu, V. V., Rajab, H., Ahmad, N., Waadt, R., Geiger, D., et al. (2018). Sulfate is Incorporated into Cysteine to Trigger ABA Production and Stomatal Closure. Plant Cell 30 (12), 2973–2987. doi: 10.1105/tpc.18.00612
Boursiac, Y., Leran, S., Corratge-Faillie, C., Gojon, A., Krouk, G., Lacombe, B. (2013). ABA transport and transporters. Trends Plant Sci. 18 (6), 325–333. doi: 10.1016/j.tplants.2013.01.007
Brautigam, K., Dietzel, L., Kleine, T., Stroher, E., Wormuth, D., Dietz, K. J., et al. (2009). Dynamic plastid redox signals integrate gene expression and metabolism to induce distinct metabolic states in photosynthetic acclimation in Arabidopsis. Plant Cell 21 (9), 2715–2732. doi: 10.1105/tpc.108.062018
Brodribb, T. J., McAdam, S. A. (2011). Passive origins of stomatal control in vascular plants. Science 331 (6017), 582–585. doi: 10.1126/science.1197985
Caldana, C., Degenkolbe, T., Cuadros-Inostroza, A., Klie, S., Sulpice, R., Leisse, A., et al. (2011). High-density kinetic analysis of the metabolomic and transcriptomic response of Arabidopsis to eight environmental conditions. Plant J. 67 (5), 869–884. doi: 10.1111/j.1365-313X.2011.04640.x
Chiba, Y., Shimizu, T., Miyakawa, S., Kanno, Y., Koshiba, T., Kamiya, Y., et al. (2015). Identification of Arabidopsis thaliana NRT1/PTR FAMILY (NPF) proteins capable of transporting plant hormones. J. Plant Res. 128 (4), 679–686. doi: 10.1007/s10265-015-0710-2
Choi, W. G., Miller, G., Wallace, I., Harper, J., Mittler, R., Gilroy, S. (2017). Orchestrating rapid long-distance signaling in plants with Ca2+, ROS and electrical signals. Plant J. 90 (4), 698–707. doi: 10.1111/tpj.13492
Christmann, A., Grill, E. (2018). Peptide signal alerts plants to drought. Nature 556 (7700), 178–179. doi: 10.1038/d41586-018-03872-4
Christmann, A., Weiler, E. W., Steudle, E., Grill, E. (2007). A hydraulic signal in root-to-shoot signalling of water shortage. Plant J. 52 (1), 167–174. doi: 10.1111/j.1365-313X.2007.03234.x
Christmann, A., Grill, E., Huang, J. (2013). Hydraulic signals in long-distance signaling. Curr. Opin. Plant Biol. 16 (3), 293–300. doi: 10.1016/j.pbi.2013.02.011
Cook, D., Fowler, S., Fiehn, O., Thomashow, M. F. (2004). A prominent role for the CBF cold response pathway in configuring the low-temperature metabolome of Arabidopsis. Proc. Natl. Acad. Sci. U. States America 101 (42), 15243–15248. doi: 10.1073/pnas.0406069101
Couee, I., Sulmon, C., Gouesbet, G., El Amrani, A. (2006). Involvement of soluble sugars in reactive oxygen species balance and responses to oxidative stress in plants. J. Exp. Bot. 57 (3), 449–459. doi: 10.1093/jxb/erj027
Cramer, G. R., Urano, K., Delrot, S., Pezzotti, M., Shinozaki, K. (2011). Effects of abiotic stress on plants: a systems biology perspective. BMC Plant Biol. 11:163. doi: 10.1186/1471-2229-11-163
Cutler, S. R., Rodriguez, P. L., Finkelstein, R. R., Abrams, S. R. (2010). Abscisic acid: emergence of a core signaling network. Annu. Rev. Plant Biol. 61, 651–679. doi: 10.1146/annurev-arplant-042809-112122
Deppe, J. P., Rabbat, R., Hortensteiner, S., Keller, B., Martinoia, E., Lopez-Marques, R. L. (2018). The wheat ABC transporter Lr34 modifies the lipid environment at the plasma membrane. J Biol Chem. 293 (48), 18667–18679. doi: 10.1074/jbc.RA118.002532
Deuschle, K., Funck, D., Hellmann, H., Daschner, K., Binder, S., Frommer, W. B. (2001). A nuclear gene encoding mitochondrial Delta-pyrroline-5-carboxylate dehydrogenase and its potential role in protection from proline toxicity. Plant J. 27 (4), 345–356. doi: 10.1046/j.1365-313x.2001.01101.x
Devireddy, A. R., Zandalinas, S. I., Gomez-Cadenas, A., Blumwald, E., Mittler, R. (2018). Coordinating the overall stomatal response of plants: Rapid leaf-to-leaf communication during light stress. Sci. Signaling 11 (518), eaam9514. doi: 10.1126/scisignal.aam9514
Ding, Y., Kalo, P., Yendrek, C., Sun, J., Liang, Y., Marsh, J. F., et al. (2008). Abscisic acid coordinates nod factor and cytokinin signaling during the regulation of nodulation in Medicago truncatula. Plant Cell 20 (10), 2681–2695. doi: 10.1105/tpc.108.061739
Dixon, R. A., Paiva, N. L. (1995). Stress-Induced Phenylpropanoid Metabolism. Plant Cell 7 (7), 1085–1097. doi: 10.1105/tpc.7.7.1085
Do, T. H. T., Martinoia, E., Lee, Y. (2018). Functions of ABC transporters in plant growth and development. Curr. Opin. Plant Biol. 41, 32–38. doi: 10.1016/j.pbi.2017.08.003
Ellis, J. G., Lagudah, E. S., Spielmeyer, W., Dodds, P. N. (2014). The past, present and future of breeding rust resistant wheat. Front. Plant Sci. 5:641:641. doi: 10.3389/fpls.2014.00641
Endo, A., Sawada, Y., Takahashi, H., Okamoto, M., Ikegami, K., Koiwai, H., et al. (2008). Drought induction of Arabidopsis 9-cis-epoxycarotenoid dioxygenase occurs in vascular parenchyma cells. Plant Physiol. 147 (4), 1984–1993. doi: 10.1104/pp.108.116632
Fiers, M., Golemiec, E., Xu, J., van der Geest, L., Heidstra, R., Stiekema, W., et al. (2005). The 14-amino acid CLV3, CLE19, and CLE40 peptides trigger consumption of the root meristem in Arabidopsis through a CLAVATA2-dependent pathway. Plant Cell 17 (9), 2542–2553. doi: 10.1105/tpc.105.034009
Fukushima, A., Kusano, M., Nakamichi, N., Kobayashi, M., Hayashi, N., Sakakibara, H., et al. (2009). Impact of clock-associated Arabidopsis pseudo-response regulators in metabolic coordination. Proc. Natl. Acad. Sci. U. States America 106 (17), 7251–7256. doi: 10.1073/pnas.0900952106
Ge, K., Liu, X., Li, X., Hu, B., Li, L. (2017). Isolation of an ABA Transporter-Like 1 Gene from Arachis hypogaea That Affects ABA Import and Reduces ABA Sensitivity in Arabidopsis. Front. Plant Sci. 8, 1150. doi: 10.3389/fpls.2017.01150
Gong, Q., Li, P., Ma, S., Indu Rupassara, S., Bohnert, H. J. (2005). Salinity stress adaptation competence in the extremophile Thellungiella halophila in comparison with its relative Arabidopsis thaliana. Plant J. 44 (5), 826–839. doi: 10.1111/j.1365-313X.2005.02587.x
Gonzalez, A. A., Agbevenou, K., Herrbach, V., Gough, C., Bensmihen, S. (2015). Abscisic acid promotes pre-emergence stages of lateral root development in Medicago truncatula. Plant Signaling Behav. 10 (1), e977741. doi: 10.4161/15592324.2014.977741
Hamilton, D. W., Hills, A., Kohler, B., Blatt, M. R. (2000). Ca2+ channels at the plasma membrane of stomatal guard cells are activated by hyperpolarization and abscisic acid. Proc. Natl. Acad. Sci. U. States America 97 (9), 4967–4972. doi: 10.1073/pnas.080068897
Hellmann, H., Funck, D., Rentsch, D., Frommer, W. B. (2000). Hypersensitivity of an Arabidopsis sugar signaling mutant toward exogenous proline application. Plant Physiol. 123 (2), 779–789. doi: 10.1104/pp.123.2.779
Himuro, Y., Ishiyama, K., Mori, F., Gondo, T., Takahashi, F., Shinozaki, K., et al. (2014). Arabidopsis galactinol synthase AtGolS2 improves drought tolerance in the monocot model Brachypodium distachyon. J. Plant Physiol. 171 (13), 1127–1131. doi: 10.1016/j.jplph.2014.04.007
Hirai, M. Y., Yano, M., Goodenowe, D. B., Kanaya, S., Kimura, T., Awazuhara, M., et al. (2004). Integration of transcriptomics and metabolomics for understanding of global responses to nutritional stresses in Arabidopsis thaliana. Proc. Natl. Acad. Sci. U. States America 101 (27), 10205–10210. doi: 10.1073/pnas.0403218101
Hoekstra, F. A., Golovina, E. A., Buitink, J. (2001). Mechanisms of plant desiccation tolerance. Trends Plant Sci. 6 (9), 431–438. doi: 10.1016/s1360-1385(01)02052-0
Holbrook, N. M., Shashidhar, V. R., James, R. A., Munns, R. (2002). Stomatal control in tomato with ABA-deficient roots: response of grafted plants to soil drying. J. Exp. Bot. 53 (373), 1503–1514. doi: 10.1093/jexbot/53.373.1503
Honna, P. T., Fuganti-Pagliarini, R., Ferreira, L. C., Molinari, M. D. C., Marin, S. R. R., de Oliveira, M. C. N., et al. (2016). Molecular, physiological, and agronomical characterization, in greenhouse and in field conditions, of soybean plants genetically modified with AtGolS2 gene for drought tolerance. Mol. Breed. 36 (11), 157. doi: 10.1007/s11032-016-0570-z
Hou, C., Tian, W., Kleist, T., He, K., Garcia, V., Bai, F., et al. (2014). DUF221 proteins are a family of osmosensitive calcium-permeable cation channels conserved across eukaryotes. Cell Res. 24 (5), 632–635. doi: 10.1038/cr.2014.14
Hulbert, S. H., Bai, J., Fellers, J. P., Pacheco, M. G., Bowden, R. L. (2007). Gene expression patterns in near isogenic lines for wheat rust resistance gene lr34/yr18. Phytopathology 97 (9), 1083–1093. doi: 10.1094/PHYTO-97-9-1083
Hwang, J. U., Song, W. Y., Hong, D., Ko, D., Yamaoka, Y., Jang, S., et al. (2016). Plant ABC Transporters Enable Many Unique Aspects of a Terrestrial Plant’s Lifestyle. Mol. Plant 9 (3), 338–355. doi: 10.1016/j.molp.2016.02.003
Ito, Y., Nakanomyo, I., Motose, H., Iwamoto, K., Sawa, S., Dohmae, N., et al. (2006). Dodeca-CLE peptides as suppressors of plant stem cell differentiation. Science 313 (5788), 842–845. doi: 10.1126/science.1128436
Iuchi, S., Kobayashi, M., Taji, T., Naramoto, M., Seki, M., Kato, T., et al. (2001). Regulation of drought tolerance by gene manipulation of 9-cis-epoxycarotenoid dioxygenase, a key enzyme in abscisic acid biosynthesis in Arabidopsis. Plant J. 27 (4), 325–333. doi: 10.1046/j.1365-313x.2001.01096.x
Jeon, J., Kim, N. Y., Kim, S., Kang, N. Y., Novak, O., Ku, S. J., et al. (2010). A subset of cytokinin two-component signaling system plays a role in cold temperature stress response in Arabidopsis. J. Biol. Chem. 285 (30), 23371–23386. doi: 10.1074/jbc.M109.096644
Jones, A. M., Danielson, J. A. H., ManojKumar, S. N., Lanquar, V., Grossmann, G., Frommer, W. B. (2014). Abscisic acid dynamics in roots detected with genetically encoded FRET sensors. Elife 3, e01741. doi: 10.7554/eLife.01741
Joshi, V., Joung, J. G., Fei, Z., Jander, G. (2010). Interdependence of threonine, methionine and isoleucine metabolism in plants: accumulation and transcriptional regulation under abiotic stress. Amino Acids 39 (4), 933–947. doi: 10.1007/s00726-010-0505-7
Jun, J., Fiume, E., Roeder, A. H., Meng, L., Sharma, V. K., Osmont, K. S., et al. (2010). Comprehensive analysis of CLE polypeptide signaling gene expression and overexpression activity in Arabidopsis. Plant Physiol. 154 (4), 1721–1736. doi: 10.1104/pp.110.163683
Kang, J., Hwang, J. U., Lee, M., Kim, Y. Y., Assmann, S. M., Martinoia, E., et al. (2010). PDR-type ABC transporter mediates cellular uptake of the phytohormone abscisic acid. Proc. Natl. Acad. Sci. U. S. A. 107 (5), 2355–2360. doi: 10.1073/pnas.0909222107
Kang, J., Yim, S., Choi, H., Kim, A., Lee, K. P., Lopez-Molina, L., et al. (2015). Abscisic acid transporters cooperate to control seed germination. Nat. Commun. 6, 8113. doi: 10.1038/ncomms9113
Kanno, Y., Hanada, A., Chiba, Y., Ichikawa, T., Nakazawa, M., Matsui, M., et al. (2012). Identification of an abscisic acid transporter by functional screening using the receptor complex as a sensor. Proc. Natl. Acad. Sci. U. S. A. 109 (24), 9653–9658. doi: 10.1073/pnas.1203567109
Kaplan, F., Kopka, J., Haskell, D. W., Zhao, W., Schiller, K. C., Gatzke, N., et al. (2004). Exploring the temperature-stress metabolome of Arabidopsis. Plant Physiol. 136 (4), 4159–4168. doi: 10.1104/pp.104.052142
Kaplan, F., Kopka, J., Sung, D. Y., Zhao, W., Popp, M., Porat, R., et al. (2007). Transcript and metabolite profiling during cold acclimation of Arabidopsis reveals an intricate relationship of cold-regulated gene expression with modifications in metabolite content. Plant J. 50 (6), 967–981. doi: 10.1111/j.1365-313X.2007.03100.x
Kinoshita, A., Nakamura, Y., Sasaki, E., Kyozuka, J., Fukuda, H., Sawa, S. (2007). Gain-of-function phenotypes of chemically synthetic CLAVATA3/ESR-related (CLE) peptides in Arabidopsis thaliana and Oryza sativa. Plant Cell Physiol. 48 (12), 1821–1825. doi: 10.1093/pcp/pcm154
Knight, H., Trewavas, A. J., Knight, M. R. (1997). Calcium signalling in Arabidopsis thaliana responding to drought and salinity. Plant J. 12 (5), 1067–1078. doi: 10.1046/j.1365-313x.1997.12051067.x
Kollist, H., Zandalinas, S. I., Sengupta, S., Nuhkat, M., Kangasjarvi, J., Mittler, R. (2019). Rapid Responses to Abiotic Stress: Priming the Landscape for the Signal Transduction Network. Trends Plant Sci. 24 (1), 25–37. doi: 10.1016/j.tplants.2018.10.003
Kovinich, N., Wang, Y., Adegboye, J., Chanoca, A. A., Otegui, M. S., Durkin, P., et al. (2018). Arabidopsis MATE45 antagonizes local abscisic acid signaling to mediate development and abiotic stress responses. Plant Direct 2 (10), e00087. doi: 10.1002/pld3.87
Krasensky, J., Jonak, C. (2012). Drought, salt, and temperature stress-induced metabolic rearrangements and regulatory networks. J. Exp. Bot. 63 (4), 1593–1608. doi: 10.1093/jxb/err460
Krattinger, S. G., Kang, J., Braunlich, S., Boni, R., Chauhan, H., Selter, L. L., et al. (2019). Abscisic acid is a substrate of the ABC transporter encoded by the durable wheat disease resistance gene Lr34. New Phytol. 223 (2), 853–866. doi: 10.1111/nph.15815
Kudla, J., Becker, D., Grill, E., Hedrich, R., Hippler, M., Kummer, U., et al. (2018). Advances and current challenges in calcium signaling. New Phytol. 218 (2), 414–431. doi: 10.1111/nph.14966
Kumar, M. N., Jane, W. N., Verslues, P. E. (2013). Role of the putative osmosensor Arabidopsis histidine kinase1 in dehydration avoidance and low-water-potential response. Plant Physiol. 161 (2), 942–953. doi: 10.1104/pp.112.209791
Kuromori, T., Shinozaki, K. (2010). ABA transport factors found in Arabidopsis ABC transporters. Plant Signaling Behav. 5 (9), 1124–1126. doi: 10.4161/psb.5.9.12566
Kuromori, T., Miyaji, T., Yabuuchi, H., Shimizu, H., Sugimoto, E., Kamiya, A., et al. (2010). ABC transporter AtABCG25 is involved in abscisic acid transport and responses. Proc. Natl. Acad. Sci. U. States America 107 (5), 2361–2366. doi: 10.1073/pnas.0912516107
Kuromori, T., Sugimoto, E., Shinozaki, K. (2011). Arabidopsis mutants of AtABCG22, an ABC transporter gene, increase water transpiration and drought susceptibility. Plant J. 67 (5), 885–894. doi: 10.1111/j.1365-313X.2011.04641.x
Kuromori, T., Sugimoto, E., Shinozaki, K. (2014). Intertissue signal transfer of abscisic acid from vascular cells to guard cells. Plant Physiol. 164 (4), 1587–1592. doi: 10.1104/pp.114.235556
Kuromori, T., Seo, M., Shinozaki, K. (2018). ABA Transport and Plant Water Stress Responses. Trends Plant Sci. 23 (6), 513–522. doi: 10.1016/j.tplants.2018.04.001
Kusano, M., Tohge, T., Fukushima, A., Kobayashi, M., Hayashi, N., Otsuki, H., et al. (2011). Metabolomics reveals comprehensive reprogramming involving two independent metabolic responses of Arabidopsis to UV-B light. Plant J. 67 (2), 354–369. doi: 10.1111/j.1365-313X.2011.04599.x
Lee, K. P., Piskurewicz, U., Tureckova, V., Carat, S., Chappuis, R., Strnad, M., et al. (2012). Spatially and genetically distinct control of seed germination by phytochromes A and B. Genes Dev. 26 (17), 1984–1996. doi: 10.1101/gad.194266.112
Leran, S., Noguero, M., Corratge-Faillie, C., Boursiac, Y., Brachet, C., Lacombe, B. (2020). Functional Characterization of the Arabidopsis Abscisic Acid Transporters NPF4.5 and NPF4.6 in Xenopus Oocytes. Front. Plant Sci. 11:144:144. doi: 10.3389/fpls.2020.00144
Levitt, E. (1972). Higher-order and lower-order reading responses of mentally retarded and nonretarded children at the first-grade level. Am. J. Ment. Deficiency 77 (1), 13–20.
Li, H. W., Zang, B. S., Deng, X. W., Wang, X. P. (2011). Overexpression of the trehalose-6-phosphate synthase gene OsTPS1 enhances abiotic stress tolerance in rice. Planta 234 (5), 1007–1018. doi: 10.1007/s00425-011-1458-0
Liu, X., Wang, J., Sun, L. (2018). Structure of the hyperosmolality-gated calcium-permeable channel OSCA1.2. Nat. Commun. 9 (1), 5060. doi: 10.1038/s41467-018-07564-5
Ma, Y., Szostkiewicz, I., Korte, A., Moes, D., Yang, Y., Christmann, A., et al. (2009). Regulators of PP2C phosphatase activity function as abscisic acid sensors. Science 324 (5930), 1064–1068. doi: 10.1126/science.1172408
Maity, K., Heumann, J. M., McGrath, A. P., Kopcho, N. J., Hsu, P. K., Lee, C. W., et al. (2019). Cryo-EM structure of OSCA1.2 from Oryza sativa elucidates the mechanical basis of potential membrane hyperosmolality gating. Proc. Natl. Acad. Sci. U. States America 116 (28), 14309–14318. doi: 10.1073/pnas.1900774116
Mani, S., Van De Cotte, B., Van Montagu, M., Verbruggen, N. (2002). Altered levels of proline dehydrogenase cause hypersensitivity to proline and its analogs in Arabidopsis. Plant Physiol. 128 (1), 73–83. doi: 10.1104/pp.010572
Maruyama, K., Takeda, M., Kidokoro, S., Yamada, K., Sakuma, Y., Urano, K., et al. (2009). Metabolic pathways involved in cold acclimation identified by integrated analysis of metabolites and transcripts regulated by DREB1A and DREB2A. Plant Physiol. 150 (4), 1972–1980. doi: 10.1104/pp.109.135327
Maruyama, K., Urano, K., Yoshiwara, K., Morishita, Y., Sakurai, N., Suzuki, H., et al. (2014). Integrated analysis of the effects of cold and dehydration on rice metabolites, phytohormones, and gene transcripts. Plant Physiol. 164 (4), 1759–1771. doi: 10.1104/pp.113.231720
Maruyama, K., Urano, K., Kusano, M., Sakurai, T., Takasaki, H., Kishimoto, M., et al. (2020). Metabolite/phytohormone-gene regulatory networks in soybean organs under dehydration conditions revealed by integration analysis. Plant J. 103 (1), 197–211. doi: 10.1111/tpj.14719
McAdam, S. A., Brodribb, T. J., Ross, J. J. (2016). Shoot-derived abscisic acid promotes root growth. Plant Cell Environ. 39 (3), 652–659. doi: 10.1111/pce.12669
McLachlan, D. H., Pridgeon, A. J., Hetherington, A. M. (2018). How Arabidopsis Talks to Itself about Its Water Supply. Mol. Cell 70 (6), 991–992. doi: 10.1016/j.molcel.2018.06.011
Miller, G., Suzuki, N., Ciftci-Yilmaz, S., Mittler, R. (2010). Reactive oxygen species homeostasis and signalling during drought and salinity stresses. Plant Cell Environ. 33 (4), 453–467. doi: 10.1111/j.1365-3040.2009.02041.x
Motomitsu, A., Sawa, S., Ishida, T. (2015). Plant peptide hormone signalling. Essays Biochem. 58, 115–131. doi: 10.1042/bse0580115
Munemasa, S., Hauser, F., Park, J., Waadt, R., Brandt, B., Schroeder, J. I. (2015). Mechanisms of abscisic acid-mediated control of stomatal aperture. Curr. Opin. Plant Biol. 28, 154–162. doi: 10.1016/j.pbi.2015.10.010
Nakabayashi, R., Mori, T., Saito, K. (2014a). Alternation of flavonoid accumulation under drought stress in Arabidopsis thaliana. Plant Signaling Behav. 9 (8), e29518. doi: 10.4161/psb.29518
Nakabayashi, R., Yonekura-Sakakibara, K., Urano, K., Suzuki, M., Yamada, Y., Nishizawa, T., et al. (2014b). Enhancement of oxidative and drought tolerance in Arabidopsis by overaccumulation of antioxidant flavonoids. Plant J. 77 (3), 367–379. doi: 10.1111/tpj.12388
Nakagawa, Y., Katagiri, T., Shinozaki, K., Qi, Z., Tatsumi, H., Furuichi, T., et al. (2007). Arabidopsis plasma membrane protein crucial for Ca2+ influx and touch sensing in roots. Proc. Natl. Acad. Sci. U. States America 104 (9), 3639–3644. doi: 10.1073/pnas.0607703104
Nakashima, K., Yamaguchi-Shinozaki, K., Shinozaki, K. (2014). The transcriptional regulatory network in the drought response and its crosstalk in abiotic stress responses including drought, cold, and heat. Front. Plant Sci. 5:170:170. doi: 10.3389/fpls.2014.00170
Nanjo, T., Kobayashi, M., Yoshiba, Y., Kakubari, Y., Yamaguchi-Shinozaki, K., Shinozaki, K. (1999). Antisense suppression of proline degradation improves tolerance to freezing and salinity in Arabidopsis thaliana. FEBS Lett. 461 (3), 205–210. doi: 10.1016/s0014-5793(99)01451-9
Nishiyama, R., Watanabe, Y., Fujita, Y., Le, D. T., Kojima, M., Werner, T., et al. (2011). Analysis of cytokinin mutants and regulation of cytokinin metabolic genes reveals important regulatory roles of cytokinins in drought, salt and abscisic acid responses, and abscisic acid biosynthesis. Plant Cell 23 (6), 2169–2183. doi: 10.1105/tpc.111.087395
Nishizawa, A., Yabuta, Y., Shigeoka, S. (2008). Galactinol and raffinose constitute a novel function to protect plants from oxidative damage. Plant Physiol. 147 (3), 1251–1263. doi: 10.1104/pp.108.122465
Notaguchi, M., Okamoto, S. (2015). Dynamics of long-distance signaling via plant vascular tissues. Front. Plant Sci. 6:161:161. doi: 10.3389/fpls.2015.00161
Obata, T., Fernie, A. R. (2012). The use of metabolomics to dissect plant responses to abiotic stresses. Cell. Mol. Life Sci. 69 (19), 3225–3243. doi: 10.1007/s00018-012-1091-5
Park, S. Y., Fung, P., Nishimura, N., Jensen, D. R., Fujii, H., Zhao, Y., et al. (2009). Abscisic acid inhibits type 2C protein phosphatases via the PYR/PYL family of START proteins. Science 324 (5930), 1068–1071. doi: 10.1126/science.1173041
Park, Y., Xu, Z. Y., Kim, S. Y., Lee, J., Choi, B., Kim, H., et al. (2016). Spatial Regulation of ABCG25, an ABA Exporter, Is an Important Component of the Mechanism Controlling Cellular ABA Levels. Plant Cell 28 (10), 2528–2544. doi: 10.1105/tpc.16.00359
Pawela, A., Banasiak, J., Biala, W., Martinoia, E., Jasinski, M. (2019). MtABCG20 is an ABA exporter influencing root morphology and seed germination of Medicago truncatula. Plant J. 93 (3), 511–523. doi: 10.1111/tpj.14234
Pei, Z. M., Murata, Y., Benning, G., Thomine, S., Klusener, B., Allen, G. J., et al. (2000). Calcium channels activated by hydrogen peroxide mediate abscisic acid signalling in guard cells. Nature 406 (6797), 731–734. doi: 10.1038/35021067
Pellizzaro, A., Clochard, T., Cukier, C., Bourdin, C., Juchaux, M., Montrichard, F., et al. (2014). The nitrate transporter MtNPF6.8 (MtNRT1.3) transports abscisic acid and mediates nitrate regulation of primary root growth in Medicago truncatula. Plant Physiol. 166 (4), 2152–2165. doi: 10.1104/pp.114.250811
Ren, S. C., Song, X. F., Chen, W. Q., Lu, R., Lucas, W. J., Liu, C. M. (2019). CLE25 peptide regulates phloem initiation in Arabidopsis through a CLERK-CLV2 receptor complex. J. Integr. Plant Biol. 61 (10), 1043–1061. doi: 10.1111/jipb.12846
Rizhsky, L., Liang, H., Mittler, R. (2002). The combined effect of drought stress and heat shock on gene expression in tobacco. Plant Physiol. 130 (3), 1143–1151. doi: 10.1104/pp.006858
Rizhsky, L., Liang, H., Shuman, J., Shulaev, V., Davletova, S., Mittler, R. (2004). When defense pathways collide. The response of Arabidopsis to a combination of drought and heat stress. Plant Physiol. 134 (4), 1683–1696. doi: 10.1104/pp.103.033431
Sakuma, Y., Maruyama, K., Osakabe, Y., Qin, F., Seki, M., Shinozaki, K., et al. (2006). Functional analysis of an Arabidopsis transcription factor, DREB2A, involved in drought-responsive gene expression. Plant Cell 18 (5), 1292–1309. doi: 10.1105/tpc.105.035881
Sato, H., Takasaki, H., Takahashi, F., Suzuki, T., Iuchi, S., Mitsuda, N., et al. (2018). Arabidopsis thaliana NGATHA1 transcription factor induces ABA biosynthesis by activating NCED3 gene during dehydration stress. Proc. Natl. Acad. Sci. U. States America 115 (47), E11178–E11187. doi: 10.1073/pnas.1811491115
Schauer, N., Fernie, A. R. (2006). Plant metabolomics: towards biological function and mechanism. Trends Plant Sci. 11 (10), 508–516. doi: 10.1016/j.tplants.2006.08.007
Selvaraj, M. G., Ishizaki, T., Valencia, M., Ogawa, S., Dedicova, B., Ogata, T., et al. (2017). Overexpression of an Arabidopsis thaliana galactinol synthase gene improves drought tolerance in transgenic rice and increased grain yield in the field. Plant Biotechnol. J. 15 (11), 1465–1477. doi: 10.1111/pbi.12731
Soni, P., Nutan, K. K., Soda, N., Nongpiur, R. C., Roy, S., Singla-Pareek, S. L., et al. (2015). “Towards Understanding Abiotic Stress Signaling in Plants: Convergence of Genomic, Transcriptomic, Proteomic, and Metabolomic Approaches,” in Elucidation of Abiotic Stress Signaling in Plants: Functional Genomics Perspectives, Volume 1. Ed. Pandey, G. K. (New York, NY: Springer New York), 3–40.
Sussmilch, F. C., Brodribb, T. J., McAdam, S. A. M. (2017). Up-regulation of NCED3 and ABA biosynthesis occur within minutes of a decrease in leaf turgor but AHK1 is not required. J. Exp. Bot. 68 (11), 2913–2918. doi: 10.1093/jxb/erx124
Sweetlove, L. J., Fell, D., Fernie, A. R. (2008). Getting to grips with the plant metabolic network. Biochem. J. 409 (1), 27–41. doi: 10.1042/BJ20071115
Taji, T., Ohsumi, C., Iuchi, S., Seki, M., Kasuga, M., Kobayashi, M., et al. (2002). Important roles of drought- and cold-inducible genes for galactinol synthase in stress tolerance in Arabidopsis thaliana. Plant J. 29 (4), 417–426. doi: 10.1046/j.0960-7412.2001.01227.x
Takahashi, F., Shinozaki, K. (2019). Long-distance signaling in plant stress response. Curr. Opin. Plant Biol. 47, 106–111. doi: 10.1016/j.pbi.2018.10.006
Takahashi, F., Kuromori, T., Sato, H., Shinozaki, K. (2018a). Regulatory Gene Networks in Drought Stress Responses and Resistance in Plants. Adv. Exp. Med. Biol. 1081, 189–214. doi: 10.1007/978-981-13-1244-1_11
Takahashi, F., Suzuki, T., Osakabe, Y., Betsuyaku, S., Kondo, Y., Dohmae, N., et al. (2018b). A small peptide modulates stomatal control via abscisic acid in long-distance signalling. Nature 556 (7700), 235–238. doi: 10.1038/s41586-018-0009-2
Takahashi, F., Hanada, K., Kondo, T., Shinozaki, K. (2019). Hormone-like peptides and small coding genes in plant stress signaling and development. Curr. Opin. Plant Biol. 51, 88–95. doi: 10.1016/j.pbi.2019.05.011
Tal, I., Zhang, Y., Jorgensen, M. E., Pisanty, O., Barbosa, I. C., Zourelidou, M., et al. (2016). The Arabidopsis NPF3 protein is a GA transporter. Nat. Commun. 7:11486. doi: 10.1038/ncomms11486
Thalmann, M., Pazmino, D., Seung, D., Horrer, D., Nigro, A., Meier, T., et al. (2016). Regulation of Leaf Starch Degradation by Abscisic Acid Is Important for Osmotic Stress Tolerance in Plants. Plant Cell 28 (8), 1860–1878. doi: 10.1105/tpc.16.00143
Tombesi, S., Nardini, A., Frioni, T., Soccolini, M., Zadra, C., Farinelli, D., et al. (2015). Stomatal closure is induced by hydraulic signals and maintained by ABA in drought-stressed grapevine. Sci. Rep. 5:12449. doi: 10.1038/srep12449
Tran, L. S., Urao, T., Qin, F., Maruyama, K., Kakimoto, T., Shinozaki, K., et al. (2007). Functional analysis of AHK1/ATHK1 and cytokinin receptor histidine kinases in response to abscisic acid, drought, and salt stress in Arabidopsis. Proc. Natl. Acad. Sci. U. States America 104 (51), 20623–20628. doi: 10.1073/pnas.0706547105
Tschoep, H., Gibon, Y., Carillo, P., Armengaud, P., Szecowka, M., Nunes-Nesi, A., et al. (2009). Adjustment of growth and central metabolism to a mild but sustained nitrogen-limitation in Arabidopsis. Plant Cell Environ. 32 (3), 300–318. doi: 10.1111/j.1365-3040.2008.01921.x
Urano, K., Maruyama, K., Ogata, Y., Morishita, Y., Takeda, M., Sakurai, N., et al. (2009). Characterization of the ABA-regulated global responses to dehydration in Arabidopsis by metabolomics. Plant J. 57 (6), 1065–1078. doi: 10.1111/j.1365-313X.2008.03748.x
Urano, K., Kurihara, Y., Seki, M., Shinozaki, K. (2010). ‘Omics’ analyses of regulatory networks in plant abiotic stress responses. Curr. Opin. Plant Biol. 13 (2), 132–138. doi: 10.1016/j.pbi.2009.12.006
Urao, T., Yakubov, B., Satoh, R., Yamaguchi-Shinozaki, K., Seki, M., Hirayama, T., et al. (1999). A transmembrane hybrid-type histidine kinase in Arabidopsis functions as an osmosensor. Plant Cell 11 (9), 1743–1754. doi: 10.1105/tpc.11.9.1743
Verslues, P. E., Sharma, S. (2010). Proline metabolism and its implications for plant-environment interaction. Arabidopsis Book 8, e0140. doi: 10.1199/tab.0140
Vetter, J. (2000). Plant cyanogenic glycosides. Toxicon 38 (1), 11–36. doi: 10.1016/s0041-0101(99)00128-2
Winter, D., Vinegar, B., Nahal, H., Ammar, R., Wilson, G. V., Provart, N. J. (2007). An “Electronic Fluorescent Pictograph” browser for exploring and analyzing large-scale biological data sets. PloS One 2 (1), e718. doi: 10.1371/journal.pone.0000718
Wohlbach, D. J., Quirino, B. F., Sussman, M. R. (2008). Analysis of the Arabidopsis histidine kinase ATHK1 reveals a connection between vegetative osmotic stress sensing and seed maturation. Plant Cell 20 (4), 1101–1117. doi: 10.1105/tpc.107.055871
Wu, F., Chi, Y., Jiang, Z., Xu, Y., Xie, L., Huang, F., et al. (2020). Hydrogen peroxide sensor HPCA1 is an LRR receptor kinase in Arabidopsis. Nature 578 (7796), 577–581. doi: 10.1038/s41586-020-2032-3
Wulff-Zottele, C., Gatzke, N., Kopka, J., Orellana, A., Hoefgen, R., Fisahn, J., et al. (2010). Photosynthesis and metabolism interact during acclimation of Arabidopsis thaliana to high irradiance and sulphur depletion. Plant Cell Environ. 33 (11), 1974–1988. doi: 10.1111/j.1365-3040.2010.02199.x
Yamanaka, T., Nakagawa, Y., Mori, K., Nakano, M., Imamura, T., Kataoka, H., et al. (2010). MCA1 and MCA2 that mediate Ca2+ uptake have distinct and overlapping roles in Arabidopsis. Plant Physiol. 152 (3), 1284–1296. doi: 10.1104/pp.109.147371
Yao, L., Cheng, X., Gu, Z., Huang, W., Li, S., Wang, L., et al. (2018). The AWPM-19 Family Protein OsPM1 Mediates Abscisic Acid Influx and Drought Response in Rice. Plant Cell 30 (6), 1258–1276. doi: 10.1105/tpc.17.00770
Yoshiba, Y., Kiyosue, T., Katagiri, T., Ueda, H., Mizoguchi, T., Yamaguchi-Shinozaki, K., et al. (1995). Correlation between the induction of a gene for delta 1-pyrroline-5-carboxylate synthetase and the accumulation of proline in Arabidopsis thaliana under osmotic stress. Plant J. 7 (5), 751–760. doi: 10.1046/j.1365-313x.1995.07050751.x
Yoshida, T., Fernie, A. R. (2018). Remote Control of Transpiration via ABA. Trends Plant Sci. 23 (9), 755–758. doi: 10.1016/j.tplants.2018.07.001
Yoshida, T., Obata, T., Feil, R., Lunn, J. E., Fujita, Y., Yamaguchi-Shinozaki, K., et al. (2019). The Role of Abscisic Acid Signaling in Maintaining the Metabolic Balance Required for Arabidopsis Growth under Nonstress Conditions. Plant Cell 31 (1), 84–105. doi: 10.1105/tpc.18.00766
Yuan, F., Yang, H., Xue, Y., Kong, D., Ye, R., Li, C., et al. (2014). OSCA1 mediates osmotic-stress-evoked Ca2+ increases vital for osmosensing in Arabidopsis. Nature 514 (7522), 367–371. doi: 10.1038/nature13593
Zhang, H., Zhu, H., Pan, Y., Yu, Y., Luan, S., Li, L. (2014). A DTX/MATE-type transporter facilitates abscisic acid efflux and modulates ABA sensitivity and drought tolerance in Arabidopsis. Mol. Plant 7 (10), 1522–1532. doi: 10.1093/mp/ssu063
Keywords: drought stress, abscisic acid, peptides, transporters, protein kinases, metabolites, tissue-to-tissue communication
Citation: Takahashi F, Kuromori T, Urano K, Yamaguchi-Shinozaki K and Shinozaki K (2020) Drought Stress Responses and Resistance in Plants: From Cellular Responses to Long-Distance Intercellular Communication. Front. Plant Sci. 11:556972. doi: 10.3389/fpls.2020.556972
Received: 30 April 2020; Accepted: 25 August 2020;
Published: 10 September 2020.
Edited by:
Andrew Charles Cuming, University of Leeds, United KingdomReviewed by:
Pil Joon Seo, Seoul National University, South KoreaJuan Manuel Ruiz-Lozano, Consejo Superior de Investigaciones Científicas (CSIC), Spain
Copyright © 2020 Takahashi, Kuromori, Urano, Yamaguchi-Shinozaki and Shinozaki. This is an open-access article distributed under the terms of the Creative Commons Attribution License (CC BY). The use, distribution or reproduction in other forums is permitted, provided the original author(s) and the copyright owner(s) are credited and that the original publication in this journal is cited, in accordance with accepted academic practice. No use, distribution or reproduction is permitted which does not comply with these terms.
*Correspondence: Fuminori Takahashi, ZnVtaW5vcmkudGFrYWhhc2hpQHJpa2VuLmpw