- 1Institute of Biochemical Plant Pathology, Helmholtz Zentrum München, Neuherberg/Munich, Germany
- 2College of Life Sciences, Henan Normal University, Xinxiang, China
- 3Research Unit Environmental Simulation, Institute of Biochemical Plant Pathology, Helmholtz Zentrum München, Neuherberg/Munich, Germany
- 4Department of Agriculture, Food, Environment, and Forestry (DAGRI), University of Florence, Florence, Italy
- 5Department of Biology, Agriculture and Food Sciences, Institute for Sustainable Plant Protection, The National Research Council of Italy (CNR), Florence, Italy
- 6Institute of Meteorology and Climate Research — Institute of Atmospheric Environmental Research, Karlsruhe Institute of Technology, Garmisch-Partenkirchen, Germany
- 7Institute of Research on Terrestrial Ecosystems (IRET), National Research Council (CNR), Porano, Italy
- 8Chair of Biochemical Plant Pathology, Technische Universität München, Freising, Germany
Nitrogen oxides (NOx), mainly a mixture of nitric oxide (NO) and nitrogen dioxide (NO2), are formed by the reaction of nitrogen and oxygen compounds in the air as a result of combustion processes and traffic. Both deposit into leaves via stomata, which on the one hand benefits air quality and on the other hand provides an additional source of nitrogen for plants. In this study, we first determined the NO and NO2 specific deposition velocities based on projected leaf area (sVd) using a branch enclosure system. We studied four tree species that are regarded as suitable to be planted under predicted future urban climate conditions: Carpinus betulus, Fraxinus ornus, Fraxinus pennsylvanica and Ostrya carpinifolia. The NO and NO2 sVd were found similar in all tree species. Second, in order to confirm NO metabolization, we fumigated plants with 15NO and quantified the incorporation of 15N in leaf materials of these trees and four additional urban tree species (Celtis australis, Alnus spaethii, Alnus glutinosa, and Tilia henryana) under controlled environmental conditions. Based on these 15N-labeling experiments, A. glutinosa showed the most effective incorporation of 15NO. Third, we tried to elucidate the mechanism of metabolization. Therefore, we generated transgenic poplars overexpressing Arabidopsis thaliana phytoglobin 1 or 2. Phytoglobins are known to metabolize NO to nitrate in the presence of oxygen. The 15N uptake in phytoglobin-overexpressing poplars was significantly increased compared to wild-type trees, demonstrating that the NO uptake is enzymatically controlled besides stomatal dependence. In order to upscale the results and to investigate if a trade-off exists between air pollution removal and survival probability under future climate conditions, we have additionally carried out a modeling exercise of NO and NO2 deposition for the area of central Berlin. If the actually dominant deciduous tree species (Acer platanoides, Tilia cordata, Fagus sylvatica, Quercus robur) would be replaced by the species suggested for future conditions, the total annual NO and NO2 deposition in the modeled urban area would hardly change, indicating that the service of air pollution removal would not be degraded. These results may help selecting urban tree species in future greening programs.
Introduction
Urban air is posing a risk to health in most parts of the world, with emissions from industrial processes, residential heating, and heavy traffic based on fossil fuels being the principal causes. This results in high levels of particles, nitrogen oxides (NOx), and other dangerous compounds.
Particularly NOx, which is formed by the reaction of nitrogen and oxygen compounds as a result of combustion processes, is a pollutant of great concern since it is directly related to cardiovascular diseases and respiratory malfunctions (Mannucci et al., 2015) as well as being a precursor for ozone formation (Sillman, 1999). Additionally, they have also been found to increase the allergenicity of pollen (Zhao et al., 2016). In areas with heavy vehicle traffic, such as in large cities and conurbations, the amount of NOx emitted as an air pollutant into the lower troposphere is significant, and the resulting concentrations often exceed national regulation. For instance, in 2017, around 10% of all the air quality monitoring stations in Europe recorded average annual concentrations above the annual limit value of 40 μg m-3 (EEA, 2019). Plants can play an important role in mitigating the NOx related damages on health and environment because their large surface represents efficient “sinks” for air pollutants (Hill, 1971). In cities, the air phytoremediation abilities combined with other ecosystem services of trees (e.g., mitigate air temperature extremes) give urban greening the potential to improve human health while mitigating the effects of climate change (Salmond et al., 2016; Kabisch and van den Bosch, 2017). Plants remove gaseous air pollutants such as NOx and ozone mainly by uptake through the stomata of leaves, although some gaseous compounds may also be deposited on the plant surface (Elkiey et al., 1982; Jud et al., 2016). The ability to absorb NO2 has been reported for a variety of plant species, including many tree species, such as loblolly pine (Pinus taeda), white oak (Quercus alba), silver birch (Betula pendula), European beech (Fagus sylvatica), pedunculate oak (Quercus robur), holm oak (Quercus ilex), California oak (Quercus agrifolia), Scots pine (Pinus sylvestris), and Norway spruce (Picea abies) (Rogers et al., 1979; Geßler et al., 2002; Eller and Sparks, 2006; Chaparro-Suarez et al., 2011; Breuninger et al., 2013; Delaria et al., 2018). NO2 deposition is influenced by stomata aperture, nitrogen status, leaf development and -age, photosynthetic rate, and the position of leaves within the plant canopy (Morikawa et al., 1998; Sparks et al., 2001; Takahashi et al., 2005; Hu and Sun, 2010). Thus, a clear difference between tree species and dependence on vitality can be expected. In contrast to NO2 deposition, studies on NO uptake by plants are scarce in the literature. Nevertheless, measurements of atmospheric NO levels in the presence of horticultural crops, including lettuce, strawberry, apple, and banana, showed a significant decrease in atmospheric NO concentrations, indicating the ability of these plants to absorb NO (Hanson and Lindberg, 1991; Soegiarto et al., 2003).
If NOx is taken up through the stomata, it needs to be further processed or deposited into the plant structure. In fact, evidence exists that various enzymes have the ability to metabolize NOx. For example, phytoglobins (PGBs) are proteins regarded as important for the nitrogen metabolisms and are ubiquitously distributed in plants (Becana et al., 2020). These proteins play a major role in regulating many biological processes, such as normal growth and development, hypoxic stress, symbiotic nodulation and nitrogen fixation, and are activated in response to low mineral nutrient status and abiotic stress (Hebelstrup et al., 2006; Mira et al., 2016; Mira et al., 2017; Shankar et al., 2018; Becana et al., 2020; Berger et al., 2020). Particularly, PGBs can oxidize NO to nitrate during hypoxic stress, which is called the PGB/NO cycle (Igamberdiev and Hill, 2004; Igamberdiev et al., 2006; Becana et al., 2020). In previous publications on Arabidopsis and barley, we reported on the ability of PGBs (Kuruthukulangarakoola et al., 2017; Zhang et al., 2019) to fix atmospheric NO and incorporate N into the nitrogen metabolism of the plants. Atmospheric nitrogen supply has been formerly regarded as gaseous nitrogen fixation or ammonia uptake only in connection with microbial or fungal associations (Granhall and Lindberg, 1980; Papen et al., 2002). This new NO fixation process seems to be a new pathway in this cycle, which can potentially play an important role within the whole nitrogen cycle, which is essential for building up proteins, nucleic acids, chlorophyll and many other organic compounds. Although the ability for NOx uptake may be ubiquitous in plants, the actual uptake capacity of different species is likely to vary (Takahashi et al., 2005).
In the near future, tree species composition in urban areas is likely to change towards climate-change resilient species, which can cope with increases in intensity, frequency, and severity of abiotic stresses (Burley et al., 2019). In particular, drought and heat resistance are primary selection criteria for urban greening programs (e.g., Roloff et al., 2009). Therefore, stress-tolerant species such as C. betulus, F. ornus, F. pennsylvanica, O. carpinifolia, C. australis, Alnus x spaethii, A. glutinosa, and T. henryana are currently being proposed (e.g., Böll, 2017; Dickhaut and Eschenbach, 2019). However, it is known that different tree species have different pollution removal capacities that are related to various leaf traits that influence deposition velocity and to their stomatal behavior in response to drought (Grote et al., 2016). The ability to process NOx may thus be a further trait that influences the uptake of gaseous nitrogen compounds. Particularly trees that are considered suitable under future environmental conditions and which, therefore, might have a reduced stomatal conductance adapted to high temperatures and low water supply, could be assumed to have less NOx removal capacity. Therefore, tree species that are selected to withstand increasing heat and drought stress in urban areas need to be checked for their ability to provide the same degree of ecosystem services, i.e. air pollution removal.
To provide a quantitative estimate of pollution removal of current and potential future tree species, we determined the deposition rates of NO and NO2 in tree species that are regarded as suitable candidates for urban trees under future climatic conditions in Central Europe (Böll, 2017; Böll, 2018a; Böll, 2018b). Moreover, we used gas exchange measurements and followed the capacity of NO uptake and metabolization in eight different tree species fumigated with 15NO under controlled environmental conditions. Additionally, we demonstrated that the NO uptake could be enhanced in trees by introducing the Arabidopsis phytoglobin 1 and 2 (AtPGB1, AtPBG2) genes into poplars. Finally, using the newly determined deposition rates, we compared the potential NOx removal for a scenario that assumes a high abundance of tree species proposed for adaptation to climate change conditions with the removal capacities of the current urban tree distribution. This should indicate potential changes in NOx removal due to the selection of these species in future urban planning under “real world conditions”. For this exercise, we use state-of-the-art calculation processes, parameters from literature for the current tree species, and the boundary conditions for a Metropolitan area, Central Berlin.
Materials and Methods
Plants Material
All plant species with altered PGB expression used in this study are listed in Table 1. Arabidopsis thaliana (Columbia-0) with overexpressing class 1 PGB (AtPgb1+) or class 2 PGB (AtPgb2+), as well as plants with reduced (AtPgb1-) or knocked out (AtPgb2-) Pgb expression were obtained in Aarhus University as described in Hebelstrup et al. (2006). Transgenic hybrid poplars [Populus x canescens, syn. P. tremula x P. alba, number 7171-B4, Institute de la Recherche Agronomique (INRA), Nancy, France] were generated following the protocol of Bi et al. (2015). PcPgb1+ and PcPgb2+ lines are grey poplars with overexpressing Arabidopsis class 1 PGB gene (AtPgb1) and Arabidopsis class 2 PGB gene (AtPgb2). The different tree species Carpinus betulus ‘Frans Fontaine’, Fraxinus ornus ‘Loisa Lady’, Fraxinus pennsylvanica ‘Summit’, Ostrya carpinifolia, Celtis australis L., Alnus x spaethii (syn. A. japonica x A. subcordata), Alnus glutinosa ‘Imperialis’, and Tilia henryana were obtained from Wilhelm Ley Baumschule (Meckenheim, Germany) and plants were 2–4 years old. These climate-resilient tree species are tested for their suitability for future urban greening in Germany (http://www.lwg.bayern.de/landespflege/urbanes_gruen/085113/index.php; Böll, 2018a; Böll, 2018b).
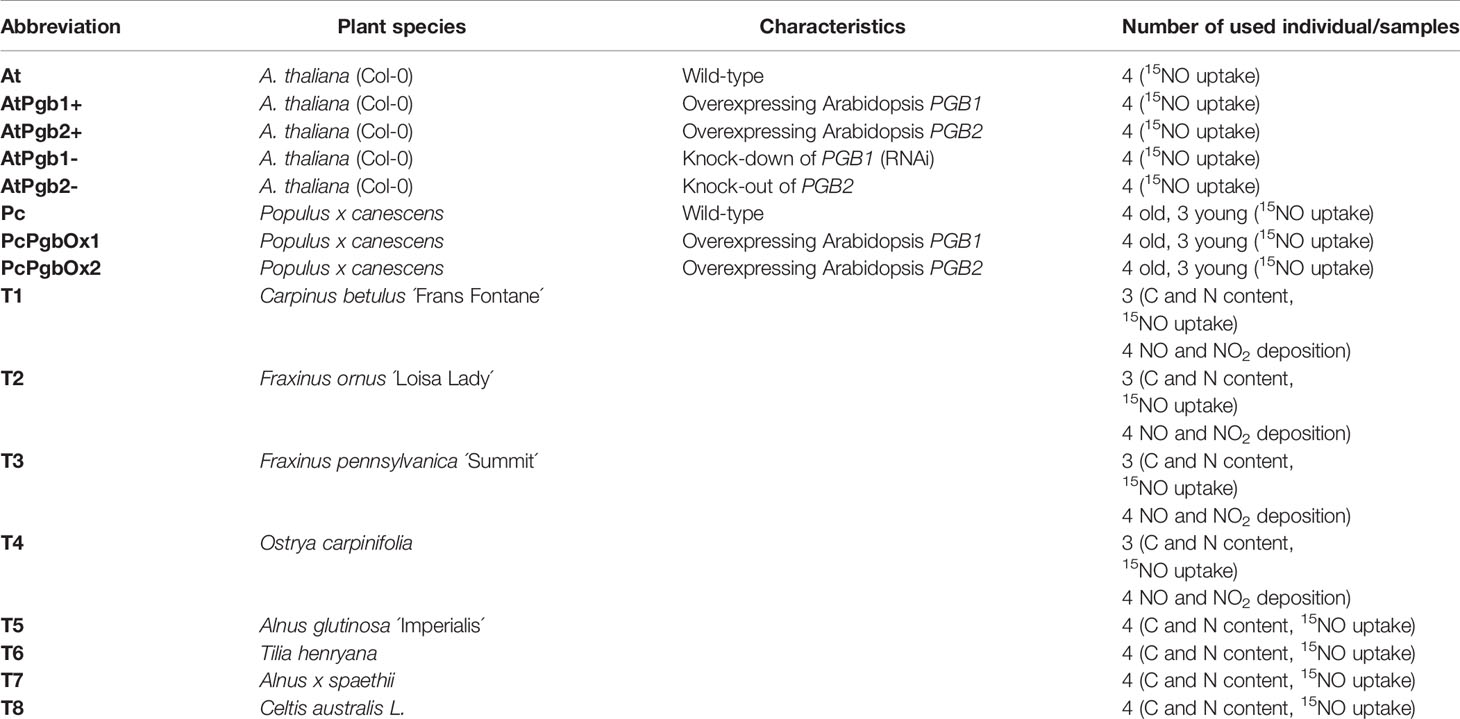
Table 1 Tree species and plants with altered PGB expression used for the different experiments in the study.
Experimental Setup and Determination of NO/NO2 Specific Leaf Deposition Velocities
All experiments were performed in the phytotron chambers of Helmholtz Center in Munich, under highly controlled conditions (for a detailed description of the chambers, see Ghirardo et al., 2020). In brief, the phytotron is composed of unique climate chambers for exposure experiments of reactive gasses (Kozovits et al., 2005), and analyses of gas-exchange of CO2, H2O (Vanzo et al., 2015) under a realistic simulation of the solar radiation spectra of UV-Vis-NIR (Seckmeyer and Payer, 1993; Döhring et al., 1996; Thiel et al., 1996).
To determinate the ability of plants to emit or remove NO/NO2 from the atmosphere, we performed NO and NO2 fumigation experiments on four different tree species (C. betulus, F. ornus, F. pennsylvanica, and O. carpinifolia) under steady-state conditions by using a dynamic branch enclosure system. Experiments were repeated using different trees to obtain four replicates (n = 4). Plants were moved inside the climate chambers two days before starting the fumigation experiment, and one tree branch containing 5–8 mature leaves was enclosed the day before the NO/NO2 experiment. The cuvette system consisted of eight odorless polyethylene terephthalate (PET) bags (size: 60x31cm) without plasticizer (Toppits Cofresco, Minden, Germany). All the line tubes (1/4"), fittings, and T-pieces were made of the inert material polytetrafluoroethylene (PTFE). The inlet air tube was placed on the side of the stem and tightened together. Each of the eight cuvettes was continuously flushed with 1,000 ml min-1 of humidified (60% RH) NOx-free air (Ghirardo et al., 2020) containing ambient CO2 concentrations (~ 400 ppm).
The environmental conditions of the enclosed branches were: leaf temperatures of 25/12°C and relative humidity (RH) of 60/80% (light/dark); light intensities of maximum incident photosynthetically active quantum flux density (PPFD) levels of 300 μmol m-2 s-1 and a photoperiod of 14 h. Experiments started four hours after switching on the light to ensure steady-state photosynthetic conditions (Ghirardo et al., 2010; Ghirardo et al., 2014). Overall, measurements followed the experimental procedures described elsewhere (Wildt et al., 1997; Chaparro-Suarez et al., 2011), although specific leaf deposition velocities of NO/NO2 were determinate on tree branches. Branches of healthy trees were exposed to six different mixing ratios of NO and NO2 of 0, 2.5, 12.5, 25, 45, 90 ppb. Clean air enriched in NO/NO2 was produced by dilution steps using mass flow controllers (MKS, Andover, USA), starting from a gas cylinder containing 2% NO in N2 (Air Liquide, Düsseldorf, Germany), and converting 50% NO to NO2 using pure O2 and reaction chambers as previously described (Mayer et al., 2018).
NO and NO2 concentrations at the inlet and outlet of the cuvettes were measured online throughout all experiments by chemiluminescence technique and using an ultra-high precision and sensitive NO/NO2 analyzer (limit of detection <0.025 ppb; model nCLD 899Y SupremeLine, Eco Physics AG, Duernten, Switzerland). Calibration of the instrument was achieved by using N2 (purity 5.0) for the zero measurements and certified NO standards at 850 ppb (Air Liquide) for the span calibration.
The cuvettes were run in parallel, and NO/NO2 were measured sequentially by switching automatically every 9 min using an automatic multiport valve in a similar manner as described before (Ghirardo et al., 2010; Ghirardo et al., 2020). The first 8 min of measurements were used as flushing time, and the corresponding acquisition data were disregarded from the data analysis to remove any interference from the previous cuvette measurement. The last 1 min containing six measurement points (10 s integration time) were averaged and used for calculation of gas-exchange based on projected leaf area (m2) as previously described (Ghirardo et al., 2011). As the reference of the fumigation levels, the inlet air was measured every four cuvettes. Therefore, the entire measurement cycle through all eight cuvettes and two references took 1 h and 30 min, before switching to the next concentration and waiting another 30 min for reaching the equilibrium of NO/NO2 concentrations.
Fluxes (F) of NO (FNO) and NO2 (FNO2) (nmol m-2 s-1) were calculated following Chaparro-Suarez et al. (2011) as:
based on the concentration differences between the outlet ports of the branch cuvette and the inlet air reference ([Cout] and [Cin], respectively, in nmol mol-1), the enclosed project leaf area (A, in m2), and the airflow rate through the cuvettes (Q, in mol s-1). The linear relationship was calculated between FNO/FNO2 and the fumigated NO/NO2 concentration:
(where x represents the fumigated NO/NO2 concentration; y, the net exchange rates of NO (FNO) or NO2 (FNO2), b, the leaf emission rate of NO/NO2 (in nmol m-2 s-1). The deposition potential (in nmol m-2 s-1 ppb-1) is the slope (k) value of eq. 2, and the compensation point is determined as the x value when y equals zero (i.e., the NO/NO2 air concentrations when leaf emission equals uptake and therefore net exchange rate is zero). Deposition potentials were converted to absolute values of specific deposition velocities (sVd, in m s-1) using the ideal gas equation for conditions of 1 atm and 20oC. Background measurements were conducted using empty cuvettes and all the data have been corrected, accordingly. All calculations were performed using data of stable leaf gas-exchange of NO/NO2 collected under steady-state conditions of photosynthesis. The total projected leaf area was determined from drawings of leaves on paper prior cuvette enclosure to allow an immediate harvest after the fumigation experiment.
Determination of 15N Content in Leaves
30 day-old Arabidopsis, 15 day-old grey poplar (the height was around 15 cm), 40 day-old grey poplar (the height was around 50 cm), and 8 different urban tree species were used in this fumigation experiment. All plants were transferred to the climate chamber two days before fumigation. 15NO (99 % atom isotopic abundance) was obtained from Linde (Pullach, Germany) and diluted to 2% (v/v) with nitrogen by Westfalen AG (Münster, Germany). Fumigation with 50 ppb of 15NO and 50 ppb of unlabeled NO (control) was performed for 4 days. After the experiment, plant leaf material was dried at 60°C for 48 h and ground to a homogenous powder using a ball mill (Tissue Lyser II, Qiagen, Venlo, Netherlands). Aliquots of about 2 mg leaf powder was transferred into tin capsules (IVA Analysentechnik, Meerbusch, Germany). 15N abundance as well as N and C contents were determined with an Isotope Ratio Mass Spectrometer (IRMS, delta V Advantage, Thermo Fisher, Dreieich, Germany) coupled to an Elemental Analyzer (Euro EA, Eurovector, Milano, Italy). IRMS measurements were always be performed in comparison with one or more standards with known isotope composition in the same range of the analyzed samples. For that purpose, a laboratory standard (acetanilide), being part of every sequence in intervals, was used. A series of working standards of different weights were measured to determine the isotope linearity of the system. All lab standard measurements were also the base for the calibration of N and C content calculation. The lab standard itself was calibrated against several suitable international isotope standards (International Atomic Energy Agency, Vienna, Austria). International and suitable laboratory isotope standards were also part of every sequence to create a final correction of 15N covering all 15N results of this sequence. The accuracy of the 15N measurements can be described by a coefficient of variation of less than 0.5%. That of the element analyses is less than 2%.
Modeling the NO2 and NO Deposition for Central Berlin
The deposition potentials measured for the four species (C. betulus, F. ornus, F. pennsylvanica and O. carpinifolia) were used to investigate the effect that planting these species may have on dry NOx deposition under realistic conditions. For this purpose, we have calculated total atmospheric NO and NO2 deposition fluxes into the street tree foliage (F, in g m-2 s-1) within the central district of Berlin (Mitte), simplified as the product of the deposition velocity (vd, m s-1) and the NO/NO2 concentration (C, in g m-3):
For this exercise, 78,000 trees within one district area of Berlin (Mitte, 39.47 km2) were considered, available from the city-tree inventory presented by Tigges et al. (2017). Species and dimension of each tree is known, indicating that the four most prominent genera in this area are Acer (26.2%, mostly A. platanoides), Tilia (25.7%, mostly T. cordata), Fagus sylvatica (17%), and Quercus (10.9%, mostly Q. robur) which together have a share of about 80%. The leaf area (LA) has been calculated using a formula based on the Beer-Lambert Law according to Nowak (1996), considering the crown dimension, which is available from the inventory.
In order to demonstrate the potential impact of the new parameters, we determined the deposition first by considering the prescribed species selection using a daytime vd value of 0.001 m s-1 for NO2 and 0.0001 m s-1 for NO for all trees (standard run). The NO2 value is the average of published measurements from tree species that are relevant for Central European urban areas, i.e., maple, oaks, and birches (Elkiey et al., 1982; Chaparro-Suarez et al., 2011). It is at the lower end of the range suggested by Lovett (1994) considering a wide range of plant species. For NO deposition velocity, we assumed a 10-fold smaller value as recommended by Hanson and Lindberg (1991), which is consistent with findings from Neubert et al. (1993). The simulation was carried out for the entire year 2014 using measured precipitation (data obtained from the German Weather Service) and air pollution data (from station “Mitte”, available from the BLUME network Berlin on request, https://www.berlin.de/senuvk/umwelt/umweltratgeber/de/spiu/luft.shtml). In a second simulation (scenario run), all trees of the aforementioned most abundant genera were replaced by the four species which had been investigated in the laboratory, using the experimentally measured sVd based on projected leaf area measurements that were converted into deposition velocity (m s-1) to leaf canopy according to:
Where LAI is the leaf area index, assumed a value of 3, commonly found for urban trees (Öztürk et al., 2015; Massetti et al., 2019). To have the largest effect, we replaced species in the order of descending vd for NO2, which is C. betulus (0.933 mm s-1) replacing Acer, O. carpinifolia (0.666 mm s-1) replacing Tilia, F. pennsylvanica (0.600 mm s-1) replacing Fagus and F. ornus (0.453 mm s-1) replacing Quercus trees. All other boundary conditions were the same as in the standard run. The respective vds for NO are 0.568 mm s-1 for C. betulus, 0.1152 mm s-1 for O. carpinifolia, 0.184 mm s-1 for F. pennsylvanica, and 0.169 mm s-1 for F. ornus. In addition, we considered a decrease in vd to one-fifth of the daytime value during night as suggested by Lovett (1994), assuming the stomata to be mostly closed.
Statistics
All experiments were performed using three or four different plants as independent replicates (n = 3-4, Table 1). Principal component analysis and group comparisons were done in R version 3.6.0 (R Core Team, 2019). Normality and homogeneity of variances were checked via the Shapiro-Wilk test (R Core Team, 2019) and Levene’s test with group medians (Fox and Weisberg, 2019), respectively. If both assumptions were not rejected (p > 0.05), ANOVA was applied, otherwise the non-parametric Kruskal-Wallis test. Raw p-values were Bonferroni-corrected across all variables of a data set. For posthoc analysis of significant ANOVA results, we applied Tukey’s test (Hothorn et al., 2008) to identify group differences. Letter assignment to groups was performed with multcompView (Graves et al., 2015).
Results
NOx Deposition Velocities and 15NO Labeling Studies in Different Tree Species
To determine the NO and NO2 specific deposition velocities to the leaf surface of Carpinus betulus, Fraxinus ornus, Fraxinus pennsylvanica, and Ostrya carpinifolia, we performed fumigation experiments and dynamic branch enclosure measurements. The experiment was performed under highly controlled environmental conditions of a phytotron. For each of the four plant species, one branch containing 5–8 mature leaves was enclosed in parallel into a respective odorless bag inside the climate chambers (Figure 1A). Increasing concentrations of NO and NO2 up to 90 ppb were applied via the inlet air, and the concentration-dependent capacity of the different tree species to remove atmospheric NO/NO2 was observed (Figures 1B, C). Then, deposition and compensation parameters were determined for NO and NO2. Experiments were repeated using four different trees (n = 4) per plant species. Although the different tree species did not fall into clearly distinct groups (Supplementary Figure S1), the foliage of C. betulus showed the highest NO deposition velocity, although not statistically different from that of the others (Figure 1D). Similary, the NO2 deposition velocity in C. betulus leaves tend to be higher compared to the other tree species (Figure 1D). Detected compensation points for NO were in the range of 1.8–2.6 ppb and for NO2 in the range of 0.8–1.5 ppb (Figure 1E).
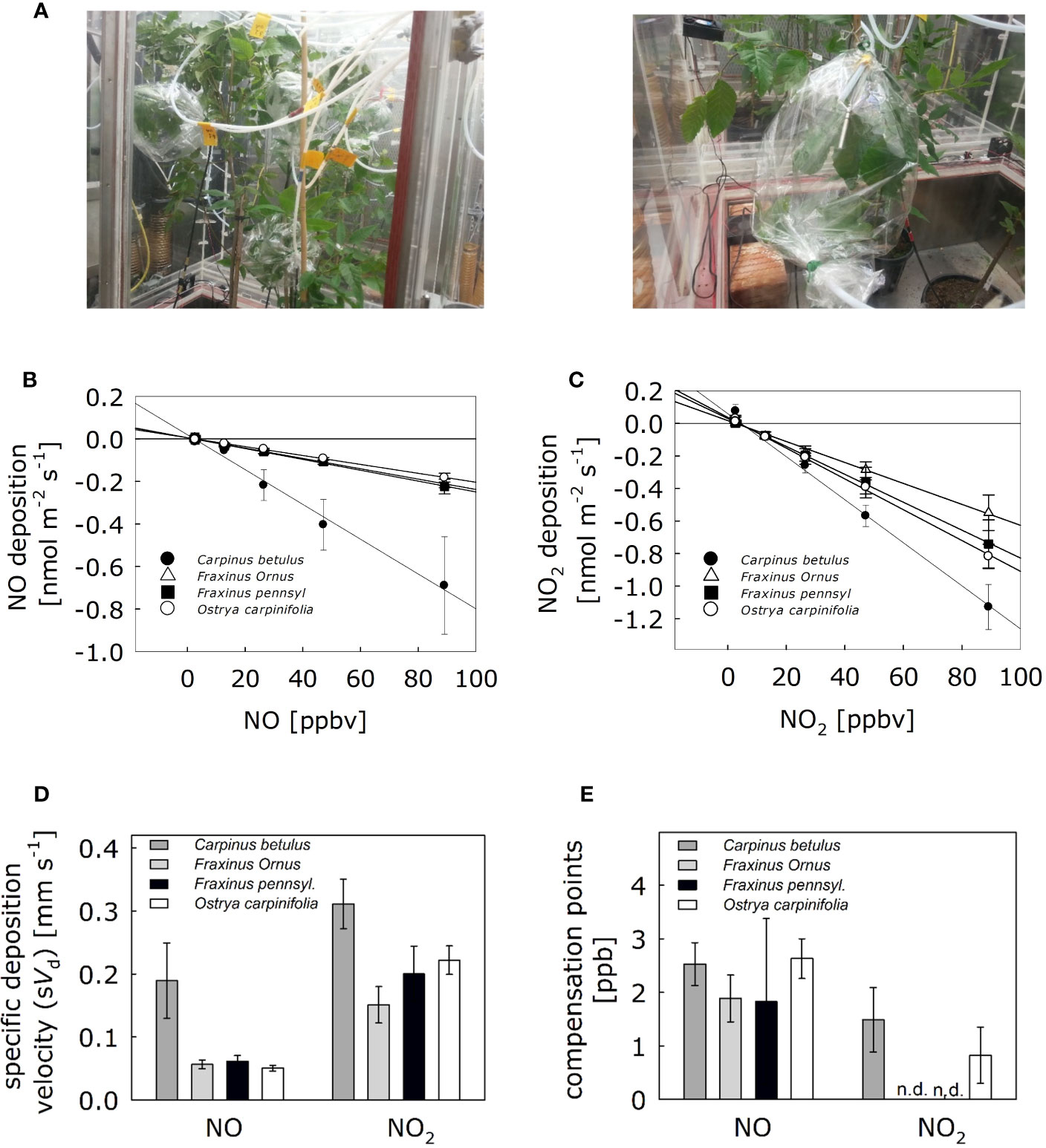
Figure 1 Exchange of NO and NO2 fluxes of four trees species resilient to heat and drought stresses suitable for urban greening. (A) Trees (C. betulus, F. ornus, F. pennsylvanica and O. carpinifolia) were placed into the climate chambers two days before fumigation to adapt to the environmental conditions. Fumigation experiments were performed with a dynamic branch enclosure system under steady-state conditions using NO and NO2. One branch was enclosed in odorless polyethylene terephthalate bags serving as cuvette system during fumigations and online measurements of NO/NO2. The inlet air tube was placed on the side of the stem and tightened together. Each cuvette was continuously flushed with 1,000 ml min-1 of humidified (60% RH) NOx-free air containing ambient CO2 concentrations (~ 400 ppm). NOx was measured sequentially by switching automatically every 9 min using an automatic multiport valve. (B) Linear regressions of NO deposition fluxes and (C) NO2 deposition fluxes are shown. (D) Specific deposition velocities based on projected leaf area for each tree species was calculated from the corresponding linear regressions. (E) Compensation points, the NO/NO2 air concentrations (in ppb) when leaf emission equals uptake (i.e., net exchange rate is zero). nd, not detectable. Bars in (C) represents means ± SD. Experiments were replicated with four different trees. None of the NO and NO2 parameters in (D) and (E) showed significant differences [Kruskal-Wallis test for NO in (D): p > 0.05; ANOVA for NO2 in (D) and for NO and NO2 in (E): p>0.05; Shapiro-Wilk test: p < 0.05 for NO in (D), p > 0.05 otherwise; Levene’s test: p < 0.001 for NO in (D), p > 0.05 otherwise].
To study the NO uptake capacity of tree foliage, a 15NO labeling experiment was performed with three trees of each species listed above. Moreover, four additional tree species (Celtis australis, Alnus spaethii, Alnus glutinosa, and Tilia henryana; four trees of each species) were included in the analysis. The trees were exposed to 50 ppb of 15NO for 5 days, while fumigation with 50 ppb of unlabeled NO was used as control. Then total N and C content, C/N ratio and 15N content were determined. Overall, the different tree species formed characteristic gradients, with Fraxinus ornus, Celtis australis, and Alnus glutinosa representing the extremes (Supplementary Figure S2). The trees differed in their total dry matter N content ranging from 0.016 g per g dry matter (F. ornus) to 0.036 g per g dry matter (C. australis) (Figure 2A). The total C content was in the range of 0.42 to 0.51 g per g of dry matter, with significantly larger values in both Alnus species than in C. australis, F. ornus and C. betulus (Figure 2B). Consequently, we observed a C/N ratio between 13 (C. australis) and 26 (F. ornus) (Figure 2C). The highest daily 15N uptake was found in A. glutinosa (3.6 mg per kg dry matter), followed by C. betulus and C. australis, respectively. The lowest daily uptake of 15N was detected in F. ornus (0.8 mg per kg dry matter) (Figure 2D).
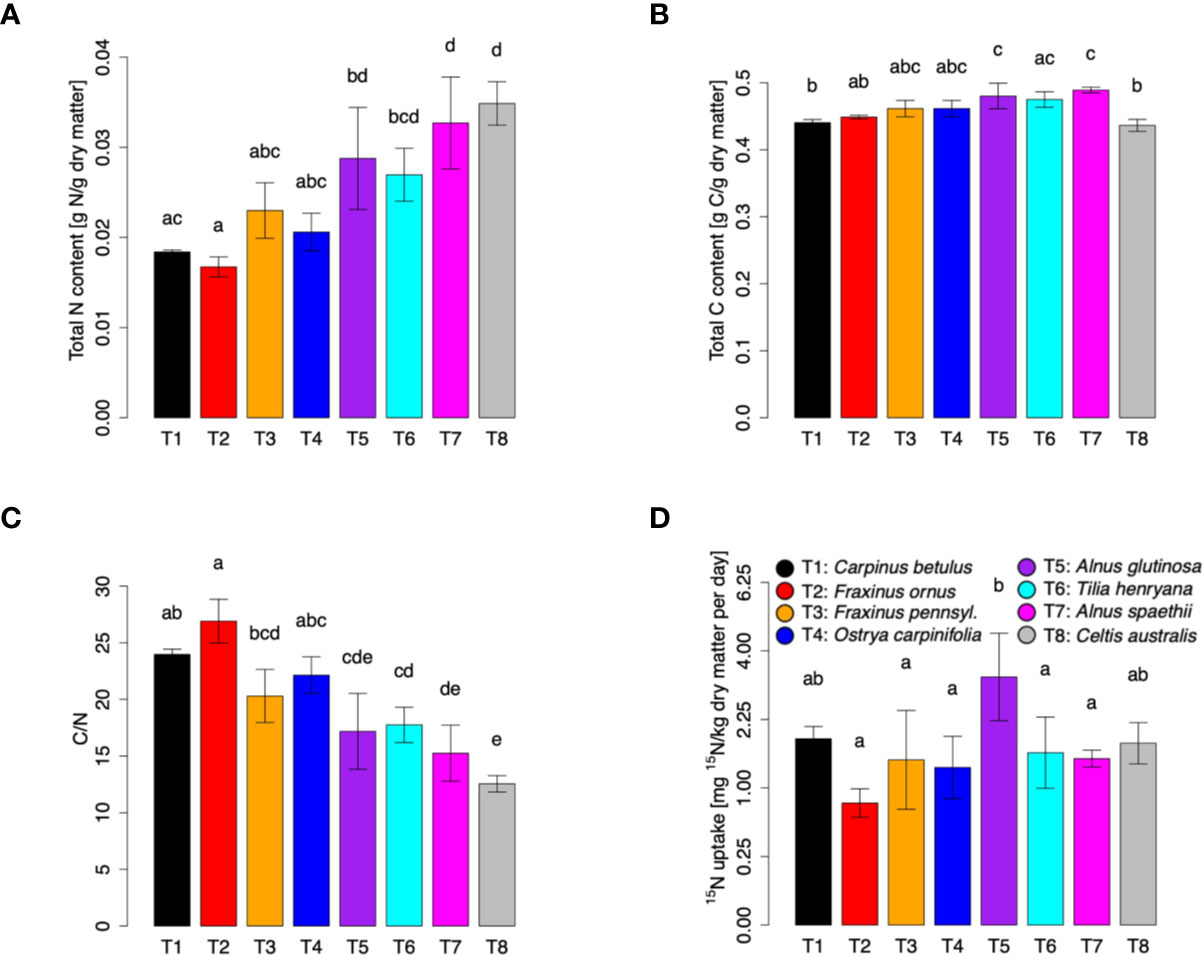
Figure 2 N, C, C/N ratio and 15N content in different tree species after fumigation with 50 ppb of 15NO for 5 days. Trees were exposed to 50 ppb of 15NO in climate chambers, and leaf samples were taken for 15N measurements after 4 days of treatment. Total N (A) and C (B) contents as well as 15N (D) content were determined with an Isotope Ratio Mass Spectrometer (IRMS) coupled to an Elemental Analyzer (EA). Calculated C/N ratio for each tree is shown in (C). 15N uptake values are shown after square root transformation. Each plot represents means ± SD. Three individuals of Carpinus betulus, Fraxinus ornus, Fraxinus pennsylvanica, and Ostrya carpinifolia were measured (n = 3). Four individuals of Celtis australis, Alnus spaethii, Alnus glutinosa, and Tilia henryana were measured (n = 4). Significant species differences were observed for N, C, C/N and 15N (ANOVA: p < 0.01; Shapiro-Wilk test: p > 0.05; Levene’s test: p > 0.05). Different letters indicate significant differences according to Tukey’s posthoc test (p < 0.05).
Modelling NO2 and NO Dry Deposition for Central Berlin.
The NOx specific deposition velocities are not plant-species dependent (Figure 1D), concluding that all the tree species investigated are suitable for urban greening. Based on our determined NOx deposition rates, we defined a tree population for the effective reduction of NOx in Central Berlin. Therefore, the four dominant tree genera in this area as depicted in Figure 3A, which represent the standard simulation, were replaced by C. betulus, F. ornus, F. pennsylvanica, and O. carpinifolia. For discussion, we also present the distribution of each tree species within the Berlin district Mitte in Figure 3B. The simulations indicate that the overall pollution removal of the newly investigated species was in the same range as that of the four currently dominant genera. According to our rough estimates that assume no changes in tree dimensions or tree positions and an equal share of the abundance of the new species, the total annual NO deposition would more than double, but the NO2 deposition would slightly decrease by ~23% (Figure 3C).
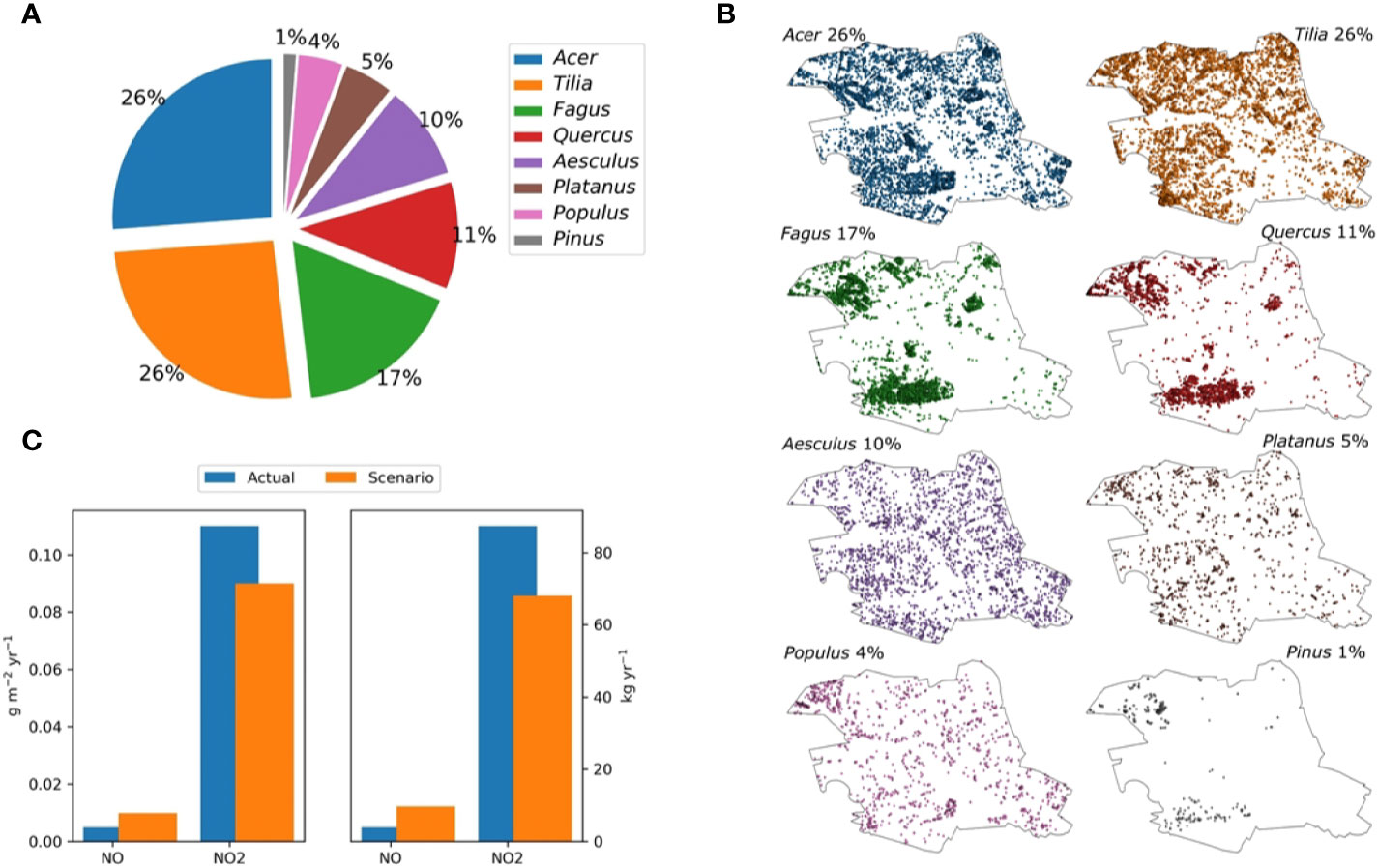
Figure 3 Tree population in Central Berlin and modeling of NOx deposition. The calculations are done with NO2 and NO concentrations of the year 2014 for the central district of Berlin (Mitte). This area hosts about 78,000 trees with the species composition indicated in (A) and the spatial distribution depicted in (B). The overall annual deposition of NO2 and NO per m2 regarding this species composition (standard) and alternative species composition (scenario) is shown in (C). For the scenario, the four urban climate-resilient tree-species (Carpinus betulus, Fraxinus ornus, Fraxinus pennsylvanica, Ostrya carpinifolia) replaced the four actual dominant tree genera as indicated by the senate of Berlin. For parameterization, we assume that the genera can be characterized by the most abundant species in each genera group (Acer platanoides, Tilia cordata, Fagus sylvatica, Quercus robur, Aesculus hypocastanum, Platanus hispanica, Populus nigra/alba, and Pinus sylvestris).
Improved NO Uptake in Phytoglobin Transgenic Arabidopsis and Poplar
As previously reported for Arabidopsis and barley, PGBs are able to fix atmospheric NO into nitrogen metabolites (Kuruthukulangarakoola et al., 2017; Zhang et al., 2019). The reaction mechanism of the NO-fixation by PGB is illustrated in Figure 4A. To investigate if an enhanced expression of PGBs can enhanced NO uptake in trees, we generated transgenic grey poplar overexpressing the Arabidopsis class 1 and class 2 PGB gene.
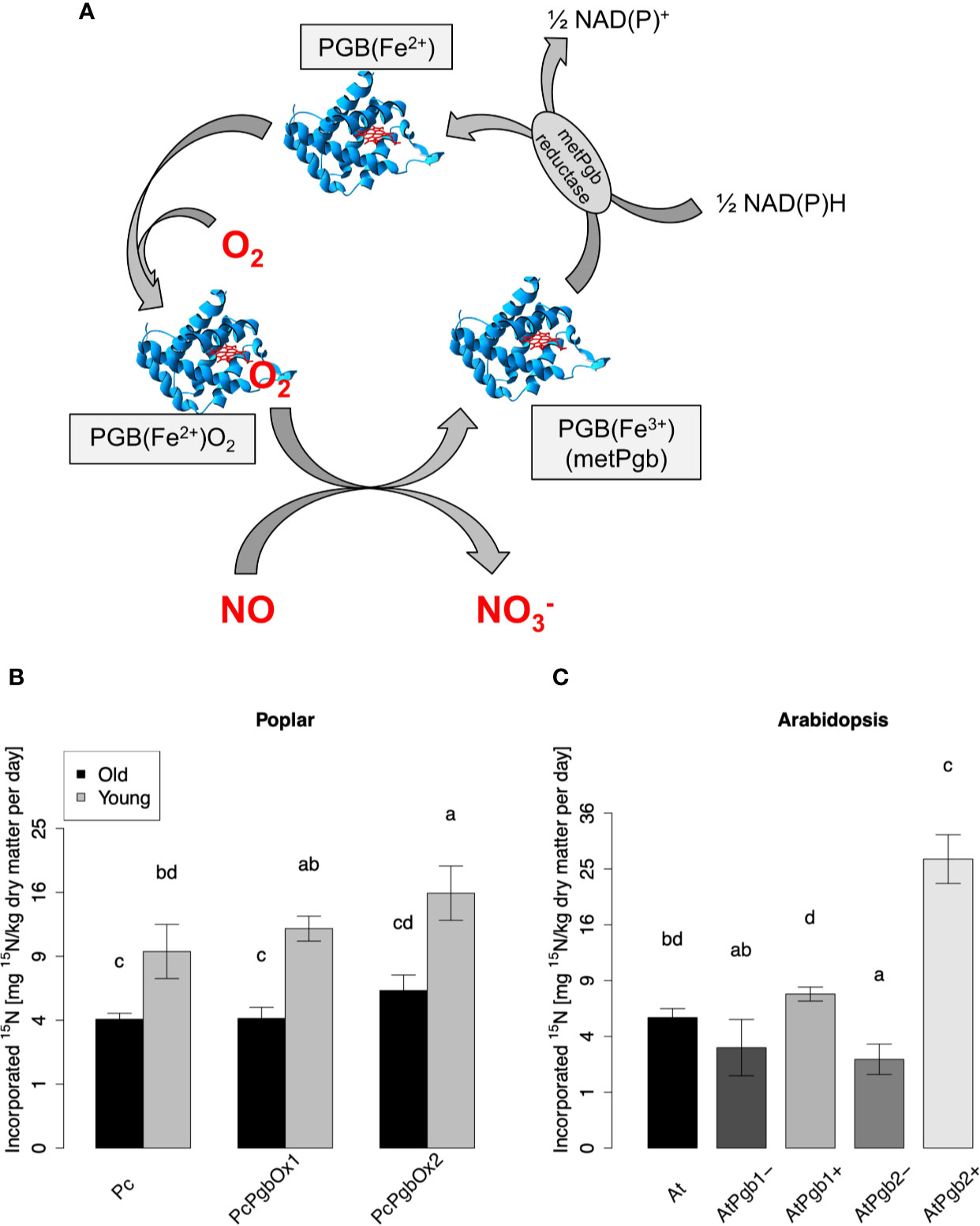
Figure 4 NO-fixation by plant phytoglobin. (A) Illustration of the biochemical NO-fixing reaction mechanism. NO is converted to nitrate (NO3-) by the oxygenated ferrous phytoglobin [PGB(Fe2+)], which turns to the metPgb form [PGB(Fe3+)]. The latter is reduced by a NAD(P)H-dependent reductase (metPGB) and then oxygenated again. (B) 15N content in transgenic poplar and (C) Arabidopsis determined 5 days after fumigation with 50 ppbv of 15NO. In (B) leaves of old (40 day-old) and young (15 day-old) poplar tree have been analyzed. Each plot depicts means ± SD. Four samples of Arabidopsis and old poplar trees were measured (n = 4) and three samples of the young poplar trees were measured (n = 3). 15N content values are shown after square root transformation. Different letters indicate significant differences according to Tukey’s posthoc test (p < 0.05) after significant ANOVA (p < 0.001; Shapiro-Wilk test: p > 0.05; Levene’s test: p > 0.05).
The enzymatically dependent NO uptake capacity of these transgenic lines was studied in four 40-day-old trees and three 15-day-old poplars. We exposed the trees to 50 ppbv of labeled 15NO for 5 consecutive days under controlled environmental conditions, and we studied the 15N label in the harvested leaf materials. Wild type poplar of the corresponding age, as well as transgenic Arabidopsis plants with enhanced and reduced expression of PGB genes, were used as controls (Figures 4B, C). 15N levels in AtPGB overexpressing plants were higher than in the corresponding WT and PGB knockdown/knockout mutants (Figure 4B), confirming that enhanced production of the protein PGB can significantly increase the enzymatic process towards higher foliage NO uptake capacity in trees. Interestingly, we observed a higher level of 15N incorporation in young poplars (15 day-old poplars) in comparison to older (40 day-old poplars) plants (Figure 4B).
Discussion
Our results showed that under well-watered conditions, A. glutinosa has the most effective NO uptake. Overall, specific deposition velocities measured in this study are in the same order of magnitude as observed in other tree species relevant for Central European urban areas such as A. platanoides, A. pseudoplatanus, Q. robur, Q. petraea, and Betula pendula (Elkiey et al., 1982; Chaparro-Suarez et al., 2011). However, some species seem to deviate from this average, as demonstrated in our study for C. betulus which at least tended to have higher sVd for NO and NO2 deposition rates. Assuming a standard transformation procedure, the resulting uptake/deposition velocities were between 0.15 and 0.56 mm s-1 for NO and 0.45 and 0.95 mm s-1 for NO2. This strongly agrees with previous studies (Hanson and Lindberg, 1991; Hereid and Monson, 2001; Teklemariam and Sparks, 2006; Breuninger et al., 2013; Delaria et al., 2018). The reasons for the considerable difference between NO and NO2 are manifold: The assimilation of NOx is controlled by several factors, including the resistance to the entry of NOx gas molecules through the stomata and mesophyll conductance, cuticle layer, and intercellular cavity to reach the surface of mesophyll cells (Morikawa et al., 1998). Overall, NO is low soluble in water, whereas NO2 quickly reacts in water to form nitrate and nitrite in the apoplast (Lee and Schwartz, 1981a; Lee and Schwartz, 1981b). Because air pollutants need to go through the extracellular aqueous covering plant cell when they enter the mesophyll cells, it is logical to expect much lower deposition velocities for NO than for NO2. Also, the permeability of nitrate and nitrite ions through cell walls and plasma membranes (Lee and Schwartz, 1981a; Lee and Schwartz, 1981b; Ramge et al., 1993; Ammann et al., 1995), as well as the activity in the primary nitrate assimilation pathway through which NO2-nitrogen is reported to be metabolized do play a role in NOx uptake (Rogers et al., 1979; Yoneyama and Sasakawa, 1979; Wellburn, 1990). The NO2 uptake by leaves of the same plant species is furthermore affected by stomatal dynamics, rate of photosynthesis, and position within the canopy (Sparks et al., 2001; Chaparro-Suarez et al., 2011). Altogether, these features can largely explain the different uptake/deposition rates for NO.
In addition to the physicochemical controls on NO/NO2 deposition velocities, we demonstrate that the fixation of NO is also under the control of an enzymatic process. The amount of PGB proteins and the activity of the NO-fixing machinery are important factors for an effective NO uptake and might differ between tree species. According to the biochemical activities of PGBs as NO dioxygenase [EC 1.14.12.17] (Perazzolli et al., 2004), nitrite reductase [EC 1.7.2.1] (Sturms et al., 2011a; Tiso et al., 2012; Kumar et al., 2016), and hydroxylamine reductase [EC 1.7.1.10] (Sturms et al., 2011b), they seem to be of general importance for the nitrogen metabolism. Since different PGB isoforms differ in their kinetic properties regarding oxygen and NO binding as well as NO deoxygenase activity (Smagghe et al., 2008; Calvo-Begueria et al., 2017; Eriksson et al., 2019), inducing the biosynthesis of PGBs and improving the biochemical features for NO-fixation might increase the uptake of atmospheric NO. We demonstrated that overexpression of Arabidopsis PGB 1 and 2 in grey poplar could indeed significantly enhance the NO uptake capacity. Therefore, producing and planting highly efficient NOx removing trees, transgenic ones or after derived from “natural” selection from phenotype screening, could be a potential means to reduce the atmospheric NOx level and improve air quality in urban areas.
Our modeling exercise resulted in only moderate to minor changes in overall NOx removal during a full year in a Metropolitan area. Nevertheless, differences between compounds exist, indicating that the total NO2 deposition would only slightly decrease while NO deposition would increase by a factor of more than two, if the four dominant tree genera grown in Central Berlin would be replaced by the four tree species considered for climate change adaptation. This is partly due to the differences between the investigated species but also due to the relatively rough estimate that the standard run was based upon. Indeed, this run is parametrized with very few deposition velocity data available for the tree species populating urban areas. Besides, the scaling of specific deposition velocities based on leaf area that is also estimated with a relatively crude method includes considerable uncertainties. As a criterion for deposition removal capacity, we used stomatal conductance that is easily scalable with LAI to obtain a canopy- or regional level result (Teklemariam and Sparks, 2006; Chaparro-Suarez et al., 2011; Breuninger et al., 2013; Delaria et al., 2018). Respective functions consider crown size and competition that are estimated based on site conditions (e.g., Pace et al., 2018). Since we did this estimation based on species-specific parameterization, the differences in LAI due to the new tree species are already considered in the calculations. Nevertheless, LAI estimates may still considerably deviate from reality because the estimation method provides high uncertainty and pruning intensity as well as frequency. Thus, crown size strongly depends on the management practice of the city, which may differ with species. In addition, the stomatal dependency of gas uptake implies that growing under dry conditions substantially decrease removal capacity. Different water-use strategies thus result in differences in gaseous uptake. For example, an isohydric species such as A. platanoides that establishes drought resistance by closing stomata early would perform less well under medium water supply compared to an anisohydric species such as Alnus or Carpinus (Li et al., 2016). Considering this behavior, we can assume that the removal capacity of the tree species resilient to drought episodes would be higher under realistic environmental conditions and even higher under projected future climate conditions, provided the drought episodes are moderate. The picture, however, might change under more severe drought that might deplete water reservoirs completely and may induce mortality.
It is, overall, desirable to choose city tree species that have a relatively high NO and NO2 uptake/deposition capacity since they could provide a viable means to reduce atmospheric NOx level and help meet clean air standards. The selection of appropriate tree species able to cope with increased heat and drought stress while keeping a high capacity to “clean” air may thus support urban planning strategies. Also, the NO-fixing capability of PGBs could be a valuable trait that might be increasingly applied to characterize tree species in the context of urban air quality.
Data Availability Statement
Datasets from this study are shown as graphs in the article/Supplementary Material. Original data are available upon request.
Author Contributions
JZ, AGh, AGo, RP, FB, and CL contributed conception and design of the study and performed the experiments/analysis. JZ AGh, and EG performed the statistical analysis. JZ and CL wrote the first draft of the manuscript. AGh, RG, EG, and J-PS wrote sections of the manuscript. All authors contributed to the article and approved the submitted version.
Funding
JZ gratefully acknowledges the financial support from China Scholarship Council (CSC, File No. 201406300083).
Conflict of Interest
The authors declare that the research was conducted in the absence of any commercial or financial relationships that could be construed as a potential conflict of interest.
Acknowledgments
We thank Felix Antritter, Elke Mattes, Lucia Gößl, and Rosina Ludwig for excellent technical assistance. Moreover, we thank Susanne Böll for selecting the tree species that are regarded suitable candidates for urban trees under future climatic conditions. This work was supported by the Bundesministerium für Bildung und Forschung (BMBF) and by the Graduate School for Climate and Environment (GRACE).
Supplementary Material
The Supplementary Material for this article can be found online at: https://www.frontiersin.org/articles/10.3389/fpls.2020.549913/full#supplementary-material
References
Ammann, M., von Ballmoos, P., Stalder, M., Suter, M., Brunold, C. (1995). Uptake and assimilation of atmospheric NO2 - N by spruce needles (Picea abies): A field study. Water Air Soil Pollut. 85, 1497–1502. doi: 10.1007/BF00477193
Becana, M., Yruela, I., Sarath, G., Catal, P., Hargrove, M. S. (2020). Plant hemoglobins: a journey from unicellular green algae to vascular plants. New Phytol. 227, 1618–1635. doi: 10.1111/nph.16444
Berger, A., Guinand, S., Boscari, A., Puppo, A., Brouquisse, R. (2020). Medicago truncatula Phytoglobin 1.1 controls symbiotic nodulation and nitrogen fixation via the regulation of nitric oxide concentration. New Phytol. 227, 84–98. doi: 10.1111/nph.16462
Bi, Z., Merl-Pham, J., Uehlein, N., Zimmer, I., Mü̈hlhans, S., Aichler, M., et al. (2015). RNAi-mediated downregulation of poplar plasma membrane intrinsic proteins (PIPs) changes plasma membrane proteome composition and affects leaf physiology. J. Proteomics 128, 321–332. doi: 10.1016/j.jprot.2015.07.029
Böll, S. (2017). 7 Jahre “Stadtgrün 2021” - Einfluss des regionalen Klimas auf das Baumwachstum an drei bayerischen Standorten (Verlag HAYMARKET, Braunschweig: Jahrbuch der Baumpflege), S. 91–S.114.
Böll, S. (2018a). Stadtbäume der Zukunft – Wichtige Erkenntnisse aus dem Forschungsprojekt (Stadtgrün 2021: Bayerische Landesanstalt für Weinbau und Gartenbau, Veitshöchheim). Available at: http://www.lwg.bayern.de/landespflege/urbanes_gruen/085113/index.php.
Böll, S. (2018b). “Projekt Stadtgrün 2021” Selektion, Anzucht und Verwendung von Gehölzen unter sich ändernden klimatischen Bedingungen (Abschlussbericht zum Forschungsvorhaben Nr: KL/17/03).
Breuninger, C., Meixner, F. X., Kesselmeier, J. (2013). Field investigations of nitrogen dioxide (NO2) exchange between plants and the atmosphere. Atmospheric Chem. Phys. 13, 773–790. doi: 10.5194/acp-13-773-2013
Burley, H., Beaumont, L. J., Ossola, A., Baumgartner, J. B., Gallagher, R., Laffan, S., et al. (2019). Substantial declines in urban tree habitat predicted under climate change. Sci. Total Environ. 685, 451–462. doi: 10.1016/j.scitotenv.2019.05.287
Calvo-Begueria, L., Cuypers, B., Van Doorslaer, S., Abbruzzetti, S., Bruno, S., Berghmans, H., et al. (2017). Characterization of the heme pocket structure and ligand binding kinetics of non-symbiotic hemoglobins from the model legume Lotus japonicus. Front. Plant Sci. 8, 407. doi: 10.3389/fpls.2017.00407. eCollection 2017.
Chaparro-Suarez, I. G., Meixner, F. X., Kesselmeier, J. (2011). Nitrogen dioxide (NO2) uptake by vegetation controlled by atmospheric concentrations and plant stomatal aperture. Atmospheric Environ. 45, 5742–5750. doi: 10.1016/j.atmosenv.2011.07.021
Delaria, E. R., Vieira, M., Cremieux, J., Cohen, R. C. (2018). Measurements of NO and NO2 exchange between the atmosphere and Quercus agrifolia. Atmospheric Chem. Phys. 18, 14161–14173. doi: 10.5194/acp-18-14161-2018
Dickhaut, W., Eschenbach, A. (2019). Entwicklungskonzept Stadtbäume - Anpassungsstrategien an sich verändernde urbane und klimatische Rahmenbedingungen (Hamburg: HafenCity Universität Hamburg).
Döhring, T., Köfferlein, M., Thiel, S., Seidlitz, H. K. (1996). Spectral shaping of artificial UV-B irradiation for vegetation stress research. J. Plant Physiol. 148, 115–119. doi: 10.1016/S0176-1617(96)80302-6
EEA (2019). Air quality in Europe 2019, European Environmental Agency, EEA report No 10/2018. Luxembourg: European Environmental Agency, Publications Office of the European Union.
Elkiey, T., Ormrod, D. P., Marie, B. (1982). Foliar sorption of sulfur dioxide, nitrogen dioxide and ozone by ornamental woody plants. HortScience 17, 358–359.
Eller, A. S. D., Sparks, J. P. (2006). Predicting leaf-level fluxes of O3 and NO2: The relative roles of diffusion and biochemical processes. Plant Cell Environ. 29, 1742–1750. doi: 10.1111/j.1365-3040.2006.01546.x
Eriksson, N. L., Reeder, B. J., Wilson, M. T., Bülow, L. (2019). Sugar beet hemoglobins: reactions with nitric oxide and nitrite reveal differential roles for nitrogen metabolism. Biochem. J. 476, 2111–2125. doi: 10.1042/BCJ20190154
Fox, J., Weisberg, S. (2019). An R Companion to Applied Regression. 3rd ed. (Thousand Oaks CA: Sage). Available at: https://socialsciences.mcmaster.ca/jfox/Books/Companion/.
Geßler, A., Rienks, M., Rennenberg, H. (2002). Stomatal uptake and cuticular adsorption contribute to dry deposition of NH3 and NO2 to needles of adult spruce (Picea abies) trees. New Phytol. 156, 179–194. doi: 10.1046/j.1469-8137.2002.00509.x
Ghirardo, A., Koch, K., Taipale, R., Zimmer, I., Schnitzler, J.-P., Rinne, J. (2010). Determination of de novo and pool emissions of terpenes from four common boreal/alpine trees by 13CO2 labelling and PTR-MS analysis. Plant Cell Environ. 33, 781–792. doi: 10.1111/j.1365-3040.2009.02104.x
Ghirardo, A., Gutknecht, J., Zimmer, I., Brüggemann, N., Schnitzler, J.-P. (2011). Biogenic volatile organic compound and respiratory CO2 emissions after 13C-labeling: online tracing of C translocation dynamics in poplar plants. PloS One 6, e17393. doi: 10.1371/journal.pone.0017393
Ghirardo, A., Wright, L. P., Bi, Z., Rosenkranz, M., Pulido, P., Rodríguez-Concepción, M., et al. (2014). Metabolic flux analysis of plastidic isoprenoid biosynthesis in poplar leaves emitting and nonemitting isoprene. Plant Physiol. 165, 37–51. doi: 10.1104/pp.114.236018
Ghirardo, A., Lindstein, F., Koch, K., Buegger, F., Schloter, M., Albert, A., et al. (2020). Origin of VOC emissions from subarctic ecosystems under global warming. Global Change Biol. 26, 1908–1925. doi: 10.1111/gcb.14935
Granhall, U., Lindberg, T. (1980). “Nitrogen input through biological nitrogen fixation,” in Structure and function of northern Coniferous forests - An ecosystem Study. Ed. Persson, T. (Stockholm: Ecol. Bull.).
Graves, S., Piepho, H.-P., Selzer, L., Dorai-Raj, S. (2015). multcompView: Visualizations of Paired Comparisons. R package version 0.1-7. Available at: https://CRAN.R-project.org/package=multcompView.
Grote, R., Samson, R., Alonso, R., Amorim, J. H., Cariñanos, P., Churkina, G., et al. (2016). Functional traits of urban trees in relation to their air pollution mitigation potential: A holistic discussion, Front. Ecol. Environ. 14, 543–550. doi: 10.1002/fee.1426
Hanson, P. J., Lindberg, S. E. (1991). Dry deposition of reactive nitrogen compounds: A review of leaf, canopy and non-foliar measurements. Atmos. Environ. 25, 1615–1634. doi: 10.1016/0960-1686(91)90020-8
Hebelstrup, K. H., Hunt, P., Dennis, E., Jensen, S. B., Jensen, E.Ø. (2006). Hemoglobin is essential for normal growth of Arabidopsis organs. Physiol. Plant. 127, 157–166. doi: 10.1111/j.1399-3054.2006.00653.x
Hereid, D. P., Monson, R. K. (2001). Nitrogen oxide fluxes between corn (Zea mays L.) leaves and the atmosphere. Atmospheric Environ. 35, 975–983. doi: 10.1016/S1352-2310(00)00342-3
Hill, A. C. (1971). Vegetation: A sink for atmospheric pollutants. J. Air Pollut. Control Assoc. 21, 341–346. doi: 10.1080/00022470.1971.10469535
Hothorn, T., Bretz, F., Westfall, P. (2008). Simultaneous Inference in General Parametric Models. Biometrical J. 50, 346–363. doi: 10.1002/bimj.200810425
Hu, Y., Sun, G. (2010). Leaf nitrogen dioxide uptake coupling apoplastic chemistry, carbon/sulfur assimilation, and plant nitrogen status. Plant Cell Rep. 29, 1069–1077. doi: 10.1007/s00299-010-0898-5
Igamberdiev, A. U., Hill, R. D. (2004). Nitrate, NO and haemoglobin in plant adaptation to hypoxia: An alternative to classic fermentation pathways. J. Exp. Bot. 55, 2473–2482. doi: 10.1093/jxb/erh272
Igamberdiev, A. U., Bykova, N. V., Hill, R. D. (2006). Nitric oxide scavenging by barley hemoglobin is facilitated by a monodehydroascorbate reductase-mediated ascorbate reduction of methemoglobin. Planta 223, 1033–1040. doi: 10.1007/s00425-005-0146-3
Jud, W., Fischer, L., Canaval, E., Wohlfahrt, G., Tissier, A., Hansel, A. (2016). Plant surface reactions: an ozone defence mechanism impacting atmospheric chemistry. Atmospheric Chem. Phys. 16, 277–292. doi: 10.5194/acp-16-277-2016
Kabisch, N., van den Bosch, M. A. (2017). “Urban green spaces and the potential for health improvement and environmental justice in a changing climate,” in Nature-Based Solutions to Climate Change Adaptation in Urban Areas. Theory and Practice of Urban Sustainability Transitions. Eds. Kabisch, N., Korn, H., Stadler, J., Bonn, A. (Cham: Springer).
Kozovits, A. R., Matyssek, R., Blaschke, H., Göttlein, A., Grams, T. E. E. (2005). Competition increasingly dominates the responsiveness of juvenile beech and spruce to elevated CO2/O3 concentrations throughout two subsequent growing seasons. Global Change Biol. 11, 1387–1401. doi: 10.1111/j.1365-2486.2005.00993.x
Kumar, N., Astegno, A., Chen, J., Giorgetti, A., Dominici, P. (2016). Residues in the distal heme pocket of Arabidopsis non-symbiotic hemoglobins: Implication for Nitrite Reductase Activity. Int. J. Mol. Sci. 17, 640–656. doi: 10.3390/ijms17050640
Kuruthukulangarakoola, G. T., Zhang, J., Albert, A., Winkler, J. B., Lang, H., Buegger, F., et al. (2017). Nitric oxide-fixation by non-symbiotic haemoglobin proteins in Arabidopsis thaliana under N-limited conditions. Plant Cell Environ. 40, 36–50. doi: 10.1111/pce.12773
Lee, Y. N., Schwartz, S. E. (1981a). Evaluation of the rate of uptake of nitrogen dioxide by atmospheric and surface liquid water. J. Geophys. Res. 86, 11971–11983. doi: 10.1029/JC086iC12p11971
Lee, Y. N., Schwartz, S. E. (1981b). Reaction kinetics of nitrogen dioxide with liquid water at low partial pressure. J. Phys. Chem. 85, 840–848. doi: 10.1021/j150607a022
Li, S., Feifel, M., Karimi, Z., Schuldt, B., Choat, B., Jansen, S. (2016). Leaf exchange performance and the lethal water potential of five European species during drought. Tree Physiol. 36, 179–192. doi: 10.1093/treephys/tpv117
Lovett, G. M. (1994). Atmospheric deposition of nutrients and pollutants in North America: an ecological perspective. Ecol. Appl. 4, 629–650. doi: 10.2307/1941997
Mannucci, P. M., Harari, S., Martinelli, I., Franchini, M. (2015). Effects on health of air pollution: a narrative review. Internal Emergency Med. 10, 657–662. doi: 10.1007/s11739-015-1276-7
Massetti, L., Petralli, M., Napoli, M., Brandani, G., Orlandini, S., Pearlmutter, D. (2019). Effects of deciduous shade trees on surface temperature and pedestrian thermal stress during summer and autumn. Int. J. Biometeorol. 63, 467–479. doi: 10.1007/s00484-019-01678-1
Mayer, D., Kanawati, B., Schmitt-Kopplin, P., Schnitzler, J.-P., Ghirardo, A., Georgii, E., et al. (2018). Short-term exposure to nitrogen dioxide provides basal pathogen resistance. Plant Physiol. 178, 468–487. doi: 10.1104/pp.18.00704
Mira, M. M., Hill, R. D., Stasolla, C. (2016). Pgbs improve hypoxic root growth by alleviating apical meristem cell death. Plant Physiol. 172, 2044–2056. doi: 10.1104/pp.16.01150
Mira, M. M., Huang, S., Kapoor, K., Hammond, C., Hill, R. D., Stasolla, C. (2017). Expression of Arabidopsis class 1 Pgb (AtPgb1) delays death and degradation of the root apical meristem during severe PEG-induced water deficit. J. Exp. Bot. 68, 5653–5668. doi: 10.1093/jxb/erx371
Morikawa, H., Higaki, A., Nohno, M., Takahashi, M., Kamada, M., Nakata, M., et al. (1998). More than a 600-fold variation in nitrogen dioxide assimilation among 217 plant taxa. Plant Cell Environ. 21, 180–190. doi: 10.1046/j.1365-3040.1998.00255.x
Neubert, A., Kley, D., Wildt, J., Segschneider, H., Förstel, H. (1993). Uptake of NO, NO2 and O3 by sunflower (Helianthus annuus) and tobacco plants (Nicotiana tabacum): Dependence on stomatal conductivity. Atmospheric Environ. 27A, 2137–2145. doi: 10.1016/0960-1686(93)90043-X
Nowak, D. J. (1996). Estimating leaf area and leaf biomass of open-grown deciduous urban trees. For. Sci. 42, 504–507. doi: 10.1093/forestscience/42.4.504
Öztürk, M., Bolat, İ., Ergün, A. (2015). Influence of air–soil temperature on leaf expansion and LAI of Carpinus betulus trees in a temperate urban forest patch. Agric. For. Meteorol. 200, 185–191. doi: 10.1016/j.agrformet.2014.09.014
Pace, R., Biber, P., Pretzsch, H., Grote, R. (2018). Modelling ecosystem services for park trees: Sensitivity of i-Tree Eco simulations to light exposure and tree species classification. Forests. 9, 89–106. doi: 10.3390/f9020089
Papen, H., Geßler, A., Zumbusch, E., Rennenberg, H. (2002). Chemolithoautotrophic nitrifiers in the phyllosphere of a spruce ecosystem receiving high atmospheric nitrogen input. Curr. Microbiol. 44, 56–60. doi: 10.1007/s00284-001-0074-9
Perazzolli, M., Dominici, P., Romero-Puertas, M. C., Zago, E., Zeier, J., Sonoda, M., et al. (2004). Arabidopsis nonsymbiotic hemoglobin AHb1 modulates nitric oxide bioactivity. Plant Cell 16, 2785–2794. doi: 10.1105/tpc.104.025379
R Core Team (2019). R: A language and environment for statistical computing (Vienna, Austria: R Foundation for Statistical Computing). Available at: https://www.R-project.org/.
Ramge, P., Badeck, F.-W., Plöchl, M., Kohlmaier, G. H. (1993). Apoplastic antioxidants as decisive elimination factors within the uptake process of nitrogen dioxide into leaf tissues. New Phytol. 125, 771–785. doi: 10.1111/j.1469-8137.1993.tb03927.x
Rogers, H. H., Campbell, J. C., Volk, R. J. (1979). Nitrogen-15 dioxide uptake and incorporation by Phaseolus vulgaris (L.). Science 206, 333–335. doi: 10.1126/science.206.4416.333
Roloff, A., Korn, S., Gillner, S. (2009). The Climate-Species-Matrix to select tree species for urban habitats considering climate change. Urban For. Urban Greening 8, 295–308. doi: 10.1016/j.ufug.2009.08.002
Salmond, J. A., Tadaki, M., Vardoulakis, S., Arbuthnott, K., Coutts, A., Demuzere, M., et al. (2016). Health and climate related ecosystem services provided by street trees in the urban environment. Environ. Health 15, S36. doi: 10.1186/s12940-016-0103-6
Seckmeyer, G., Payer, H. D. (1993). A new sunlight simulator for ecological research on plants. J. Photochem. Photobiol. B: Biol. 21, 175–181. doi: 10.1016/1011-1344(93)80180-H
Shankar, A., Fernandes, J. L., Kaur, K., Sharma, M., Kundu, S., Pandey, G. K. (2018). Rice Pgbs regulate responses under low mineral nutrients and abiotic stresses in Arabidopsis thaliana. Plant Cell Environ. 41, 215–230. doi: 10.1111/pce.13081
Sillman, S. (1999). The relation between ozone, NOx and hydrocarbons in urban and polluted rural environments. Atmospheric Environ. 33, 1821–1845. doi: 10.1016/S1352-2310(98)00345-8
Smagghe, B. J., Trent, J. T., III, Hargrove, M. S. (2008). NO dioxygenase activity in hemoglobins is ubiquitous in vitro, but limited by reduction in vivo. PloS One 3, e2039. doi: 10.1371/journal.pone.0002039
Soegiarto, L., Wills, R. B. H., Seberry, J. A., Leshem, Y. Y. (2003). Nitric oxide degradation in oxygen atmospheres and rate of uptake by horticultural produce. Postharvest Biol. Technol. 28, 327–331. doi: 10.1016/S0925-5214(02)00199-0
Sparks, J. P., Monson, R. K., Sparks, K. L., Lerdau, M. (2001). Leaf uptake of nitrogen dioxide (NO2) in a tropical wet forest: Implications for tropospheric chemistry. Oecologia 127, 214–221. doi: 10.1007/s004420000594
Sturms, R., DiSpirito, A. A., Hargrove, M. S. (2011a). Plant and cyanobacterial hemoglobins reduce nitrite to nitric oxide under anoxic conditions. Biochemistry 50, 3873–3878. doi: 10.1021/bi2004312
Sturms, R., DiSpirito, A. A., Fulton, D. B., Hargrove, M. S. (2011b). Hydroxylamine reduction to ammonium by plant and cyanobacterial hemoglobins. Biochemistry 50, 10829–10835. doi: 10.1021/bi201425f
Takahashi, M., Higaki, A., Nohno, M., Kamada, M., Okamura, Y., Matsui, K., et al. (2005). Differential assimilation of nitrogen dioxide by 70 taxa of roadside trees at an urban pollution level. Chemosphere 61, 633–639. doi: 10.1016/j.chemosphere.2005.03.033
Teklemariam, T. A., Sparks, J. P. (2006). Leaf fluxes of NO and NO2 in four herbaceous plant species: The role of ascorbic acid. Atmospheric Environ. 40, 2235–2244. doi: 10.1016/j.atmosenv.2005.12.010
Thiel, S., Döhring, T., Köfferlein, M., Kosak, A., Martin, P., Seidlitz, H. K. (1996). A phytotron for plants stress research: How far can artificial lighting compare to natural sunlight? J. Plant Physiol. 148, 456–463. doi: 10.1016/S0176-1617(96)80279-3
Tigges, J., Churkina, G., Lakes, T. (2017). Modeling above-ground carbon storage: a remote sensing approach to derive individual tree species information in urban settings. Urban Ecosyst. 20, 97–111. doi: 10.1007/s11252-016-0585-6
Tiso, M., Tejero, J., Kenney, C., Frizzell, S., Gladwin, M. T. (2012). Nitrite reductase activity of nonsymbiotic hemoglobins from Arabidopsis thaliana. Biochemistry 51, 5285–5292. doi: 10.1021/bi300570v
Vanzo, E., Jud, W., Li, Z., Albert, A., Domagalska, M. A., Ghirardo, A., et al. (2015). Facing the future: Effects of short-term climate extremes on isoprene-emitting and non-emitting poplar. Plant Physiol. 169, 560–575. doi: 10.1104/pp.15.00871
Wellburn, A. R. (1990). Why are atmnospheric oxides of nitrogen usually phytotoxic and not alternative fertilizers? New Phytol. 115, 395–429. doi: 10.1111/j.1469-8137.1990.tb00467.x
Wildt, J., Kley, D., Rockel, A., Rockel, P., Segschneider, H. J. (1997). Emission of NO from several higher plant species. J. Geophys. Res. 102, 5919–5927. doi: 10.1029/96JD02968
Yoneyama, T., Sasakawa, H. (1979). Transformation of atmospheric NO2 absorbed in spinach leaves. Plant Cell Physiol. 20, 263–266. doi: 10.1093/oxfordjournals.pcp.a075801
Zhang, J., Buegger, F., Albert, A., Ghirardo, A., Winkler, J. B., Schnitzler, J.-P., et al. (2019). Phytoglobin overexpression promotes barley growth in the presence of enhanced level of atmospheric nitric oxide. J. Exp. Bot. 70, 4521–4537. doi: 10.1093/jxb/erz249
Keywords: nitric oxide, nitrogen dioxide, 15N, phytoglobin, air pollution mitigation, urban trees
Citation: Zhang J, Ghirardo A, Gori A, Albert A, Buegger F, Pace R, Georgii E, Grote R, Schnitzler J-P, Durner J and Lindermayr C (2020) Improving Air Quality by Nitric Oxide Consumption of Climate-Resilient Trees Suitable for Urban Greening. Front. Plant Sci. 11:549913. doi: 10.3389/fpls.2020.549913
Received: 07 April 2020; Accepted: 10 September 2020;
Published: 29 September 2020.
Edited by:
Christiane Werner, University of Freiburg, GermanyReviewed by:
Lina Fusaro, Italian National Research Council, ItalyAstrid Moser-Reischl, Technical University of Munich, Germany
Copyright © 2020 Zhang, Ghirardo, Gori, Albert, Buegger, Pace, Georgii, Grote, Schnitzler, Durner and Lindermayr. This is an open-access article distributed under the terms of the Creative Commons Attribution License (CC BY). The use, distribution or reproduction in other forums is permitted, provided the original author(s) and the copyright owner(s) are credited and that the original publication in this journal is cited, in accordance with accepted academic practice. No use, distribution or reproduction is permitted which does not comply with these terms.
*Correspondence: Christian Lindermayr, bGluZGVybWF5ckBoZWxtaG9sdHotbXVlbmNoZW4uZGU=