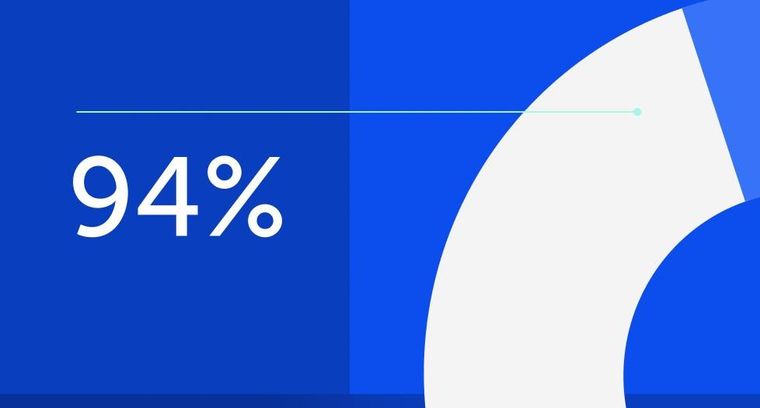
94% of researchers rate our articles as excellent or good
Learn more about the work of our research integrity team to safeguard the quality of each article we publish.
Find out more
REVIEW article
Front. Plant Sci., 07 January 2021
Sec. Plant Nutrition
Volume 11 - 2020 | https://doi.org/10.3389/fpls.2020.545453
This article is part of the Research TopicHeavy Metal Toxicity in Plants: Recent Insights on Physiological and Molecular AspectsView all 14 articles
Improving growth and productivity of plants that are vulnerable to environmental stresses, such as heavy metals, is of significant importance for meeting global food and energy demands. Because heavy metal toxicity not only causes impaired plant growth, it has also posed many concerns related to human well-being, so mitigation of heavy metal pollution is a necessary priority for a cleaner environment and healthier world. Hydrogen sulfide (H2S), a gaseous signaling molecule, is involved in metal-related oxidative stress mitigation and increased stress tolerance in plants. It performs multifunctional roles in plant growth regulation while reducing the adverse effects of abiotic stress. Most effective function of H2S in plants is to eliminate metal-related oxidative toxicity by regulating several key physiobiochemical processes. Soil pollution by heavy metals presents significant environmental challenge due to the absence of vegetation cover and the resulting depletion of key soil functions. However, the use of stress alleviators, such as H2S, along with suitable crop plants, has considerable potential for an effective management of these contaminated soils. Overall, the present review examines the imperative role of exogenous application of different H2S donors in reducing HMs toxicity, by promoting plant growth, stabilizing their physiobiochemical processes, and upregulating antioxidative metabolic activities. In addition, crosstalk of different growth regulators with endogenous H2S and their contribution to the mitigation of metal phytotoxicity have also been explored.
Heavy metals are a group of metal elements having peculiar physical and chemical properties, which are also known to possess higher specific gravity > 4 g cm–3 in nature (Grant and Grant, 1987; Duffus, 2002). Environmental occurrence of heavy metal can be of both natural and anthropogenic origin; however, unprecedented release and their strong ecological persistence have now become a serious toxicological and public health challenge worldwide (Arif et al., 2019; Zheng et al., 2020). Under natural conditions, heavy metals are the intrinsic component of earth crust and are often dispersed in soil, water, and atmosphere as a result of many geological processes, i.e., forest fire and volcanic eruption (Lado et al., 2008). A range of anthropogenic activities, such as intensive pesticides, as well as fertilizers use, vehicular emissions, mining activities, and industrial wastes, are the main contributors of heavy metal pollution across various domains of environment. Besides this, heavy environmental loading of metal toxicants also emanates from different wastewater sources. Globally, large volume of untreated wastewater is being discharged directly into waterways and soil, where they pose serious concerns for ecosystem stability (Mataka et al., 2006).
In soil, level of individual heavy metal concentration is a primary indicator often used to determine the degree of ecotoxicological effects on plants. For instance, some metals, such as nickel (Ni), molybdenum (Mo), zinc (Zn), and copper (Cu), are plant micronutrients and are phytotoxic only if their concentration is higher in soil (Lasat, 2002), whereas few other metallic elements, in particular chromium (Cr), lead (Pb), and cadmium (Cd), are hazardous to plants even at low soil concentrations. Heavy metals can either be found in dissolved or immobilized form; however, high immobilization rates can cause stronger detrimental effects on plants because of their in situ persistence and concentration buildup over time (Alloway, 1995, 2013). Consequently, they tend to impair various growth attributes of plants (Ahmad et al., 2012). Furthermore, some of these metals, such as Cr, Cd, Mn, and Zn, are also recognized to exert hormetic responses, which are reflected by a positive growth response at low concentrations and by phytotoxicity at higher metal concentrations (Azevedo and Lea, 2005). Detrimental effects of heavy metals on plants may involve oxidative stress, stunted growth, and toxicity-induced metabolic anomalies.
After nitric oxide and carbon monoxide, hydrogen sulfide (H2S) is the third most important naturally occurring gaseous molecule known for its cellular signaling in biology (Yang et al., 2008). In plants, synthesis and release of H2S typically occur during different stages of metabolic activities. It is generally formed in cut branches, tissue cultures, and leaf discs, whereas it is discharged into the surrounding environment from green cells of the plants (Rennenberg et al., 1990). Under normal growth conditions, numerous plants such as soybean, pumpkin, cotton, cantaloupe, squash, corn, and cucumber were found to release H2S from leaf into the exterior environment (Wilson et al., 1978). Besides increasing enzymatic activity of vigorous cysteine (Cys), H2S secretion is also recognized to enhance sulfite and sulfate metabolic activities (Rennenberg, 1983, 1984). However, numerous reports have observed paradoxical functions of H2S in the regulation of plants physiological and biochemical traits. For instance, H2S has been established to act as sulfur source at lower concentration, while promoting phytotoxic effects on plant growth at elevated level (De Kok et al., 2002; Li, 2013; Hancock and Whiteman, 2016; Li et al., 2016; Hancock, 2017; Huo et al., 2018). In most plants, elongated exposure to higher H2S level eventually caused leaf removal after developing leaf injury, which can lead to overall deterioration of plant growth. Likewise, H2S triggered O2 obstruction and subsequent impaired nutrient acquisition in rice seedlings were reported by Wang et al. (2012). Positive influence of H2S on various plants has also been reported in literature. Shoot deposited sulfur (S) from H2S appears to provide major site-specific S regulation for plants to improve plant growth, particularly under sulfur-deprived conditions. In many plants, H2S is reported to be involved in the regulation of key physiological and growth functions, such as formation of adventitious root in cucumber, stomatal conductance in Arabidopsis thaliana, enhanced tolerance against salinity in alfalfa during seed germination, and regulation of thiol levels in A. thaliana (Riemenschneider et al., 2005a,b; Lisjak et al., 2010; Lin et al., 2012).
The H2S is a convenient stress signaling molecule, as its biosynthesis process can take place in various cellular components once plants experience stress such as heavy metal exposure (Zulfiqar and Hancock, 2020). Upon its production, H2S can be highly mobile across plant membranes and can either be influxed in or effluxed out of the plant system as a way out against heavy metal stress (Shivaraj et al., 2020). Plant stress adaptation against heavy metal in H2S-treated plant is initiated via antioxidant activities (Kushwaha and Singh, 2019), accumulation of osmoregulators (Tian et al., 2016), cell signaling protein (He et al., 2019), and by different gene expressions (Pandey and Gautam, 2019). Overall, it enables plants to combat against stress factor such as heavy metals via effective removal of reactive oxygen species (ROS) by adjusting intracellular redox balance.
A plant experiment with nickel spiking has demonstrated that H2S can enhance rice nickel tolerance prompted mainly by preventing chloroplast damage as a result of improved N metabolism under excessive nickel contamination (Rizwan et al., 2019). However, the role of H2S as a signaling molecule in plants is still not fully understood despite the fact that the release of H2S has been demonstrated in many plant species. Notably, desulfhydrases (H2S-releasing enzymes) have functionally been endorsed as key H2S volatile in plants (Riemenschneider et al., 2005b). In another case, enhanced L-Cys desulfhydrase (LCD) activity under biotic stress further reaffirms its significant potency as an adaptive defense approach under stress agriculture (Rausch and Wachter, 2005). H2S was also involved in a promotional role of superior root organogenesis in Ipomoea batatas, Salix matsudana, and Glycine max L. (Zhang et al., 2009).
The antioxidant enzymes are another type of stress mediator in plants, which are often activated as a key defense response after recognition of given stressor, including heavy metals (Zhang et al., 2010a,b). Foliar application of sodium hydrosulfide (NaHS), an H2S donor, led to an upscale induction response of different antioxidant enzymes and reduced the concentration of H2O2 in wheat seedlings to enhance resistance against heat stress. Also, Zhang et al. (2011) emphasized that H2S can have an effective role in plant protection against different types of oxidative stress. Water-soluble antioxidants, such as ascorbic acid and glutathione, were expressed at enhanced level upon fumigation with H2S, which consequently delivered higher water stress tolerance in wheat plants (Shan et al., 2011). As NaHS is characterized as key antioxidant inducer; therefore, plant growth promotive activities including root organogenesis (Zhang et al., 2009), stomatal regulation (Lisjak et al., 2010), and seed germination (Zhang et al., 2010a,b) were substantially improved in response to heavy metal stresses. Interestingly, exogenously applied H2S (100 ppb) has shown striking effect on plant growth improvement of beet, alfalfa, and lettuce (Thompson and Kats, 1978). Furthermore, H2S foliar spraying has also improved the accumulation of vital nutrients in plant (Wang et al., 2012).
As a secondary messenger, nitric oxide and H2S can collectively trigger signal transduction resulting in higher intracellular buildup of these molecules, which are indeed a plant’s necessity to cope with metal-induced oxidative stress. In this way, use of H2S with nitric oxide could exert a regulatory response to transporters and antioxidant systems to alleviate phytotoxic effects of heavy metals (Li et al., 2012; Wang et al., 2019). Considering all the background information about the role of H2S in biological system and, most importantly, its interaction with crop plants under stress agriculture, we aimed to get an advanced overview of H2S-related growth promotive effects on crop plants. Soil contamination by heavy metals imposes greater ecological concern because of lack of plant cover and resultant land degradation. However, use of stress alleviators, such as H2S, along with suitable crop plant holds a great potential for the successful restoration of these contaminated soils. We also focus on how the use of H2S and other precursor compounds interacts with plants to counteract heavy metal toxicity. Moreover, our discussion also focuses H2S-mediated response mechanisms exhibited by plants toward heavy metal toxicity.
H2S is a weak acid with good water solubility and commonly exists in neutral molecular form (H2S). Although HS– is a major ionic form of H2S involved in most of its biological reactions, S2– also exists in minor concentration due to higher dissociation constant for second ionization (Filipovic et al., 2018). Despite being a highly water-soluble compound, H2S tends to be unstable under natural conditions as it slowly oxidizes to elemental sulfur having a weak solubility in aqueous solution. Moreover, the volatile nature of H2S exacerbates its experimental use in the environment. For instance, nearly half-dose of H2S could be lost in 5 min from open cell culture wells (DeLeon et al., 2012). Consequently, H2S handling imposes greater challenge of its precise measurement under field conditions (Wang et al., 2014; Peng and Xian, 2015).
There has been a proposition long ago that plants can produce and release H2S themselves, particularly when exposed to external sulfur (S) stimuli, i.e., Cys, sulfate, sulfite, or SO2 (Wilson et al., 1978; Sekiya et al., 1982a,b). This was thought to be a mechanism for regulating sulfur homeostasis (Calderwood and Kopriva, 2014). However, mechanistic understanding of H2S generation in plants and its interaction with other cellular components remains elusive. In higher plants, H2S biosynthesis pathway emerges in different subcellular compartments, where main enzymes linked to sulfur metabolism have the potential to initiate H2S biogenesis (Corpas et al., 2019b; Chen et al., 2020). In plants, most common enzymes involved in the H2S biosynthesis enzymes include LCD, D-Cys desulfhydrase (DCD), l-3-cyanoalaninesynthase, sulfite reductase, and Cys synthase (Yamasaki and Cohen, 2016). Among various plant cellular organelles, chloroplast serves as the major H2S production site due to localization of sulfite reductase enzyme, which catalyzes the reduction of sulfite to sulfide during sulfate assimilation pathway. In addition to this, cytosol can also generate H2S by the action of DCD and LCD, accompanied by ammonia and pyruvate production. In chloroplast, sulfide concentration is two times greater than that found into the cytosol (Krueger et al., 2009). However, this sulfide is dissociated into its ionized forms due to the basic physiological conditions and therefore unable to pass through the membranes to the cytosol (Kabil and Banerjee, 2010). In mitochondria, the synthesis of H2S can be regulated by β-cyanoalanine synthase (a pyridoxal phosphate-dependent enzyme), which transforms both cyanide and L-Cys into β-cyanoalanine and H2S in order to degrade toxic cyanogen (Gotor et al., 2019). Recently, Corpas et al. (2019a) also provided an evidence of H2S generation in the peroxisomes of Arabidopsis; however, it is still unclear whether its generation pathway is endogenous or recruited from other cellular compartments (e.g., cytosol). Additionally, the expression of plant cellular proteins [L-Cys desulfurase such as O-acetylserine thiol lyase (OAS-TL) and Nifs-like proteins] also processes the H2S synthesis in different cellular organelles (Gotor et al., 2019). These enzymes, with their varied expression, are therefore involved in controlling the production of H2S across various cellular compartments of the plant. On the other hand, occurrence of H2S in these cellular organelles with strong lipophilic characteristic promotes its translocation in the lipid bilayer of cell membranes (Cuevasanta et al., 2017).
H2S is a flammable-toxic gaseous molecule that is often distinguished by stinky rotten eggs smell. It has also shown strong concentration dependent affinity for reactions as it can disrupt mitochondrial cytochrome activity and even reduce mitochondrial respiration (Mancardi et al., 2009). Surprisingly, research in recent years has unraveled the significance of H2S as a gasotransmitter that promotes plant growth and development at various stages of plant life cycle (Xuan et al., 2020). Other than algae, fungi, and few prokaryotes, plants are known to take leverage of taking up the naturally occurring sulfate (SO42–) source of S from soil and incorporate it into organic forms (Takahashi et al., 2011). In S assimilation process, sulfate taken up by plant roots is initially reduced to H2S by catalytic activity of adenosine 5′-phosphosulfate reductase and sulfite reductase and eventually transformed into Cys via O-OAS-TL. Therefore, H2S is an extremely important intermediate in the thiometabolism pathway.
The use of H2S as signaling molecule has now become very common; thus, basic mechanism of its functional activities has been decrypted (Mustafa et al., 2009; Aroca et al., 2015). Some recent proteomic analyses have described a new posttranslational modification of proteins, where reactive Cys residues (as an H2S signal) can modify protein function by converting the thiol group (-SH) into a persulfide group (-SSH) known as persulfidation. In most cases, persulfide adducts exhibit higher nucleophilicity relative to the thiol group, and as a result, modified Cys displayed highly complex reactivity (Paul and Snyder, 2012). This might be the rationale of widespread persulfidation in nature, which largely affects protein over O2 and N species (Ida et al., 2014) (Figure 1). Overall, complex functional interactions of H2S based on its donor, concentration gradient, and plant section tend to describe actions of specific protein after translational modification.
The nature of H2S-mediated specific cellular modifications still lacks clarity, because thermodynamic reaction involving H2S and a thiol is unfavorable. Sulfane sulfur is a sulfur atom that has the peculiar ability to bind reversibly to other sulfur atoms to form hydropersulfides (R-S-SH) and polysulfides (-S-Sn-S-). These polysulfides tend to be far more efficient in persulfidation, as they are more nucleophilic than H2S (Toohey, 2011). Recently, new molecular weight persulfides were identified as possible mediator of sulfide signaling. In this relation, Cys-persulfide (Cys-SSH), glutathione persulfide, and its persulfurated species Cys-SSnH and GSSnH have been designated as redox regulators (Kasamatsu et al., 2016; Kimura et al., 2017). Recently, the endogenous Cys-SSH production synthetized by prokaryotic and mammalian cysteinyl-tRNA synthetases using L-Cys as substrate has been described. The Cys polysulfides bound to tRNA are incorporated into polypeptides that are synthesized de novo in the ribosomes, suggesting that these enzymes are the principal Cys persulfide synthases in vivo (Akaike et al., 2017).
Of various abiotic stresses in environment, heavy metal-triggered stress always has very serious repercussions for plant growth and productivity. Like other abiotic stress, heavy metal stress is also associated with unregulated overproduction of ROS, which can influence plant metabolism and physiological activities by inflicting range of oxidative stress damages. Among the signaling molecules, H2S is now an established regulator of growth in plants exposed to plethora of abiotic stresses, including heavy metal stress (Rather et al., 2020).
Given a suite of heavy metal mitigation approaches competing for an effective stress management in agriculture, it is extremely pertinent to consider only those measures that can provide plant benefits only in an eco-friendly way. Although H2S is toxic for many living organisms as evidenced by mitochondrion inhibition (Wang et al., 2019), its central role in key plant physiological functions and therapeutic use in various human ailments have been well developed in recent times (Aroca et al., 2020). The H2S in gaseous form is the simplest method of its usage in the laboratory; however, it is not realistic practice due to non-targeted ecotoxicological consequences both for the humans and environment (Rubright et al., 2017). In most cases, NaHS and/or sodium sulfide (Na2S) are used as H2S donor molecules specifically due to their higher dissolution, resulting in a short albeit sustained pulse of H2S (Table 1).
Table 1. Effects of exogenous application of different H2S sources on plant growth regulations under heavy metals stress.
Production of ROS, by-product of physiological metabolism, is a typical plant response under abiotic stress including heavy metal stress. Nevertheless, plants vary in their response against the different oxidative stresses linked to ROS in species-cultivar-specific ways. In plants, a robust antioxidative defense system (enzymatic and non-enzymatic) has been evolved to scavenge the excessive ROS accumulation, which can in turn counteract the harmful impacts of oxidative stress. Increasing evidence demonstrated that NaHS treatment can ameliorate and repair oxidative stress caused by heavy metal toxicity (Luo et al., 2020). Exogenous application of H2S can suppress the burst of ROS by activating enzymatic and non-enzymatic defense components of ascorbate–glutathione cycle and eventually avert oxidative stress damage to plants.
Legume plants are of considerable significance for the remediation of heavy metal-polluted soil due to the unique symbiotic assemblage of N-fixing bacteria (rhizobia) with leguminous host plant (Reichman, 2007; Shen et al., 2019). In alfalfa, exogenous application of NaHS (100 μM) has mitigated the compounding effects of dual metal stress (Pb/Cd) on legume-rhizobium symbiosis (Table 1; Fang et al., 2020). Application of H2S donor molecule boosted up the survival rate of rhizobia by enhancing soil enzyme activity, facilitating nutrient transformations, and shifting both composition and diversity of soil microbial population. This study concludes that H2S-mediated symbiosis has resulted in development of greater plant resistance to metal-induced toxicity as evident by increased antioxidant enzyme activity and reduced metal ion absorption. Similar to these findings, Mostofa et al. (2015) investigated a rice–cadmium interaction model, where H2S provided further evidence of being an efficient growth regulator to mitigate Cd-related growth suppression and reduction in biomass. Moreover, rice growth revitalization performance was primarily triggered by a three-way Cd alleviation process, including low Cd uptake/accumulation, mineral nutrient upregulation, and photosynthetic functions and timely induction of antioxidant response.
Heavy metals, such as Ni, are known to cause disruption in the absorption and utilization of key mineral elements in plants (Sharma and Dhiman, 2013). Importantly, Ni metal ions demonstrate a strong competitive affinity for bivalent cations, i.e., Ca, Mg, Mn, Fe, Zn, which could reduce uptake of these essential elements and obstruct normal plant growth and development (Ahmad et al., 2011). In hydroponic culture, NaHS (100 μM)-treated young seedlings of Zucchini plant showed a decrease in Ni accumulation by reviving essential mineral homeostasis (Valivand and Amooaghaie, 2020). Furthermore, this study validates the role of H2S in osmotic adjustment, as indicated by the proline and sugar content of plant exposed to heavy metal stress. Clearly, NaHS facilitated an increase in flavonoid and phenolic secretions likely to reduce the oxidative damage due to ROS scavenging, which in turn led to the improved Ni tolerance in Zucchini seedlings.
Phenolic and flavonoid are not the solitary metabolites those are exuded by heavy metal–stressed plants. Ahmad et al. (2020) demonstrated that NaHS treatment can upregulate antioxidant enzyme activity in Cr-exposed cauliflower seedlings, which is central to the amelioration of Cr-related oxidative damages, as well as reduction in metal ion translocation, to aerial plant parts. Root growth is an important predictor of plant productivity in agriculture, while impaired root growth has often been distinguished as one of the most common and earliest symptoms of plant exposure to metal toxicity (Liu et al., 2018). In foxtail millet, Cd-induced root growth inhibition was reversed by an SO2 derivative compound Na2SO3 (Han et al., 2020). This study concludes the existence of crosstalk between SO2 and nitric oxide (NO) for nitrate reductase (NR)–nitric oxide synthase-dependent endogenous NO signaling that prompted an upregulated antioxidant enzyme activity and suppressed genes associated with the Cd uptake (SiNRAMP1, SiNRAMP6, SiIRT1, and SiIRT2).
Plant cell wall is a first architectural barrier to avert transmembrane movements of toxic materials, such as heavy metals (Krzesłowska, 2011), as it can allow cellular compartmentation of the metal ions and reduce the phytotoxic effects of heavy metal exposure (Lai, 2015). Metal-tolerant proteins such as metallothionein and phytochelatins are crucial metal-binding ligand that regulates cationic homeostasis of plant cell wall (Yu et al., 2018; Zhi et al., 2020). In Woad plants, Jia et al. (2020) identified strict connection between Cd chelation and S metabolic products based on the weakening of metal ion translocation from root to shoot. The study confirmed that NaHS stimulated the endogenous metal binding proteins, i.e., metallothionein 1A and phytochelatins, which in turn promoted Cd accumulation in the cell wall by modifying its contents, thus reducing intracellular metal ion mobility for detoxification. Previously, Jia et al. (2016) established an intertwining effect of H2S and Cys in A. thaliana L. They found that sulfur metabolism has key role to play in the growth and development of plants exposed to Cd toxicity. Collectively, plant stress alleviators such as H2S and Cys have been shown to resurrect root growth, as well as to increase plant Cd tolerance via S metabolite feedback loop. An active synergy between H2S and proline pulls together millet plant from negative effects of Cd toxicity, as evidenced by induced Cd tolerance and stimulated biomass production (Tian et al., 2016). Apart from higher proline accumulation, as well as proline dehydrogenase (PDH) and proline-5-carboxylate reductase (P5CR) activities, the transcript levels of PDH and P5CR were also enhanced after H2S treatment.
Also, Yu et al. (2019) have shown that exogenous NaHS application can increase Cd retention into the root cell wall of oilseed rape by stimulating LCD activity. Furthermore, NaHS led to increase in cellular pectin, and root methylesterase activity validates higher metal-binding capacity of root cell that repressed Cd translocation to aerial plant sections. In another study, downregulated metal homeostasis and uptake were recorded when young seedlings of black night shade plant were treated with NaHS under Zn stress (Liu et al., 2016). It has been noted that expression of the metallothionein was increased, leading to an improvement in plant Zn tolerance as shown by the chelation of excessive Zn in the cytoplasm. Moreover, elevated expression of antioxidant enzyme, CAT2, also prevented oxidative stress damages in metal-stressed plants as a result of H2S treatment.
Seed viability is one of the crucial determinants of healthy plant growth and increased production. Seed germination and its emergence and subsequent seedling establishment contribute proportionally to achieve sustainability, growth, and productivity. Exogenous NaHS application has improved both seed germination and seedling emergence in cauliflower by scavenging Pb-induced ROS (Chen et al., 2018). Interestingly, H2S-led exhibition of plant protection against Pb stress was comparable to ROS scavengers, i.e., 4,5-dihydroxy-1,3-benzene disulfonic acid and N, N′-dimethylthiourea. They concluded that non-enzymatic antioxidants, such as non-protein thiol and total glutathione, were upregulated by H2S and improved Pb tolerance by ROS-scavenging and/or directly chelating metal ions. Plant exposure to metal ions often represses the activity of transporters, resulting in ionic imbalance and reduced nutrients assimilation in plants (Vaculík et al., 2020). In Zn-exposed pepper plants, exogenous NaHS treatment reduced Zn plant accumulation and enhanced the absorption of key mineral elements, i.e., Fe, N, P (Kaya et al., 2020). Moreover, mitigating effects of NaHS have been further up-scaled by antioxidant activity and osmotic adjustment to minimize membrane oxidative damage. Methylglyoxal (MG), a cytotoxic metabolic by-product of glycolytic pathways, usually interacts with macromolecules to trigger protein inactivation and induces oxidative damages to plant under abiotic stress, such as heavy metals (Hoque et al., 2016; Bhuyan et al., 2020). In maize, Cr tolerance of young seedlings was linked to the suppression of NADPH oxidase activity, resulting in restricted ROS accumulation following exogenous application of NaHS (Kharbech et al., 2020). This study illustrated the potential function of glutathione in minimizing Cr toxicity by reducing MG content while preserving glutathione–ascorbate homeostasis for additional S metabolism, as demonstrated by the activity of glutathione S-transferase and reductase enzymes. Similarly, NaHS pretreatment also reduced the lipid peroxidation and electrolyte leakage in coriander seedling exposed to Cu toxicity (Karam and Keramat, 2017). These results further substantiate that NaHS-led changes in endogenous H2S were presumably involved in the prevention of oxidative damages via cellular ascorbate–glutathione cycle. Similarly, mitigation of Cu toxicity in wheat has been linked to H2S-related ascorbate–glutathione cycle (Shan et al., 2012). Moreover, decrease in lipid peroxidation and electrolyte leakage further implies a systemic defense response activated by NaHS application.
Photosynthesis is a key physiological process for plant productivity and directly provides energy for plant growth. Photosynthetic pigments are very sensitive to various abiotic stress including heavy metals, which can negatively affect rate of photosynthesis via chlorophyll and carotenoid degradation (Amari et al., 2017). RuBISCO (a multi-meric photosynthetic enzyme), reflects potential for plant productivity and the efficiency of resource use by its net C assimilation rate. There is some substantial evidence in literature that described about H2S role of being a key regulator of plant photosynthetic apparatus (Carmo-Silva et al., 2015; Cummins et al., 2018). In spinach, Chen et al. (2011) found that NaHS-treated plants had significant increase in chlorophyll content alongside higher soluble protein content and biomass yield. This possibly highlights the significance of RuBISCO activity, which promotes chloroplast biogenesis, photosynthetic enzyme expression, and thiol redox alterations. Also, Bharwana et al. (2014) reported that NaHS application could regulate the photosynthetic activity of cotton seedlings under Pb toxicity. They concluded that higher photosynthesis is obviously a defense mechanism intended to minimize metal toxicity by accelerating rate of photosynthesis, which ultimately contribute to cope with predictable oxidative stress. Further results showed that H2S also promoted the reversal of electrolyte leakage in cotton seedlings caused by Pb toxicity, as shown by reduced H2O2 and MDA contents. In another study, Rizwan et al. (2019) reported NaHS-induced upregulation of chloroplast biogenesis and N metabolism in rice plant exposed to Ni stress. The NaHS application has been found to increase the activities of various N-related enzymes, i.e., NR, nitrite reductase, glutamate synthase, glutamate oxaloacetate transaminase, glutamine synthetase, and glutamate pyruvate transaminase. Furthermore, key involvement of H2S in Ni stress regulation of rice plant was also validated by the expression of genes abundance associated with N metabolism. Also, Chen et al. (2016) revealed the molecular basis of rice stress adaptation against mercury (Hg) contamination. In their study, H2S pretreatment extended membrane transcriptional expression of bZIP60 and OsMT-1, which were involved in Hg localization in roots. In addition, Hg-related plant membrane damages were attenuated by scavenging ROS and downregulation of H2O2 and MDA, which eventually led to growth promotion of rice seedlings. The redox status of plant cell can be disrupted by accumulation of ROS associated with metal toxicity, resulting in a cascade of retarded physiological and morphological functions (Schutzendubel and Polle, 2002). In pea seedlings, H2S application restored the cellular redox status of pea suffering from arsenate toxicity (Singh et al., 2015). It appears that H2S-mediated recovery of ascorbate–glutathione enzymes pool was a pivotal contributor to plant defense, as depicted by suppression of oxygen free radicals and membrane damage.
In some recent reports, enrolment of different plant growth regulators has also unveiled endogenous H2S synthesis in plant that eventually provide a protective role in crop plants under stress (Table 2). NO has been deemed to be an important signaling compound that can stimulate plant growth and development in agriculture. A plethora of studies has investigated the key involvement of NO in activating plant defense response to heavy metal stress (Bai et al., 2014; Rizwan et al., 2018; Hu et al., 2019; Sharma et al., 2020a). A strong synergic association between exogenously applied NO and resultant endogenous synthesis of H2S was involved in enhanced Cr resistance of tomato seedlings (Alamri et al., 2020). It appears that increased S assimilation and related enzyme metabolic activities have mitigated the depressing effect of metal ions in both cellular and molecular levels. Salicylic acid (SA), a phenolic compound, has been known to control a broad range of physiological and biochemical functions in plants to combat stressful conditions, including heavy metals (Hasanuzzaman et al., 2019; Sharma et al., 2020b). The exogenous application of Na2SiO3, a silicon derivative compound, endorsed a functional relationship between NO and H2S in regulating the Cd stress of pepper plant (Kaya et al., 2020). It was evident that upregulation of endogenous NO and H2S was central to metal stress alleviation as confirmed by lower Cd content of leaves. In addition, improvement in plant antioxidant activities, nutrients uptake (e.g., Ca, K), photosynthesis activity, and water relations upscaled the plant metal tolerance. In pepper experiment, SA prompted 66% higher buildup of endogenous H2S in Pb-exposed leaves over untreated control (Kaya, 2020). It was further elucidated that efficient crosstalk between SA and H2S averted induced phytotoxicity effect by minimizing Cd accumulation in leaves via upregulated metabolisms of the enzymes involved in ascorbate–glutathione cycle. Furthermore, SA and H2S augmented leaf relative water, water potential, and proline content, which has key contribution in restoring the phenotypic appearance of Pb-stressed plants. In another study with maize exposed to Pb stress, Zanganeh et al. (2018) pointed out the synergic effect of SA and H2S, which contributed to the relegation of metal-induced phytotoxicity by augmenting glycine-betaine and NO signaling. It was found that SA and NO signaling play an instrumental role in plant growth regulation as they boosted the S-assimilation via arginine–methionine metabolism and thus prevented Pb-induced oxidative stress injury in plants.
Table 2. Use of different stress alleviators and their interactions with endogenous H2S for higher metal tolerance in plants.
Thiamine (THI) is another unique biomolecules that can effectively control plant growth by facilitating the synthesis of carbohydrate, nucleic acids, adenosine triphosphate, and nicotinamide adenine dinucleotide phosphate (Nosaka, 2006). At the same time, it can also activate defensive responses in plants as a non-cofactor (Bettendorff and Wins, 2013; Yusof et al., 2015). Recent work utilizing THI as growth regulators in strawberry transplants exposed to Cd stress has revealed a 1.7-fold increase in endogenous H2S levels and displayed enhanced metal tolerance (Kaya and Aslan, 2020). They found that endogenous NO levels have also demonstrated the similar rise following THI treatment. The increase in leaf H2S concentration caused the upregulation of MDA and H2O2, while antioxidant enzyme activities were downregulated to overcome Cd toxicity. Furthermore, endogenous H2S improved mechanical stability and physiological functions of strawberry plants by markedly increasing the uptake of key nutrient elements, i.e., calcium and potassium. Although calcium (Ca) is typically an essential macronutrient required for normal plant growth, it can act as a universal messenger to establish systemic defense response and tolerance acquisition under stressful conditions (Jalmi et al., 2018). Several studies confirmed that exogenous Ca application can lead to higher stress tolerance in plants upon heavy metal exposure (Gonzalez et al., 2012; Ahmad et al., 2015; Aziz et al., 2015). According to Valivand et al. (2019), two-sided crosstalk between exogenous Ca and endogenous H2S appears to mediate defense response in Zucchini plant exposed to Ni stress. The Ca signaling cascade from roots to leaves has been involved in endogenous H2S synthesis that contributes toward a systemic acclimation against Ni stress. At the same time, antioxidant enzyme activities and genetic expression of calmodulin (CaM) protein further strengthen the metal ion tolerance of young seedlings, as demonstrated by reduced electrolyte leakage and oxidative injury. This study further supports the fact that both intracellular and extracellular Ca-based complexes are important for the synthesis of endogenous H2S to improve plant metal tolerance. Ascorbate–glutathione metabolism involves antioxidant defense system that perceives stress and regulates plant growth by coordinating the activities of detoxification of ROS by its key enzymes–ascorbate peroxidase, monodehydroascorbate reductase, dehydroascorbate reductase, and glutathione reductase (Hasanuzzaman et al., 2017). In maize, Zanganeh et al. (2019) showed that endogenous H2S positively regulated early growth of young seedlings via SA-mediated Pb detoxification. Plant exposure to metal ion significantly deteriorated chlorophyll content and restricted nutrients uptake, whereas H2S-SA crosstalk was instrumental in reversal of selected plant physiological and biochemical attributes, indicated by higher plant expression of ascorbic acid–glutathione metabolic activities. Methyl jasmonate (MeJA), a volatile derivative of jasmonic acid, serves as main cell signaling molecule mediating various key plant processes and also triggers plant defense in response to wide array of abiotic stresses (Raza et al., 2020). In foxtail millet, exogenous MeJA and NaHS showed an increase in endogenous H2S (75–120 nm g FW–1) and restore seedling growth under Cd stress (Tian et al., 2017). The results suggest that endogenous H2S buildup was a pivotal component of ROS mitigation, thus hindering the accumulation of Cd in young seedlings. In addition, positive interplay between MeJA and H2S augmented Cd-induced expression of the homeostasis-related genes (MTP1, MTP12, CAX2, and ZIP4).
In agriculture, abiotic stress involving heavy metals contributes to major production losses globally. It is broadly acknowledged that H2S can mitigate the abiotic stresses including heavy metal stress. This review provides insight into beneficial aspects of H2S on plant biochemical and physiological responses against heavy metal stress. After a thorough review of the available literature, we found that heavy metals inhibit plant growth, which unfortunately disturbs food production and eventually leads to food shortfall. Application of H2S has shown effective mitigation of heavy metals related by strengthening the biochemical and physiological functions of plants. Treatment of H2S devotedly leads to the enhancement in plant growth, photosynthetic pigments, biomass, nutrient uptake, gas exchange parameters, and antioxidant enzymes of plants.
Taking due account of the above findings and studies, it is strongly concluded that H2S treatment effectively reduces the harmful effects of many heavy metals (Al, Cd, B, Pb, Cr, and Cu) by obstructing the accumulation of heavy metals in various plants. Such results strongly indicate that H2S treatment could be used effectively as a signal molecule to inhibit the oxidative stress caused by heavy metal contamination. Recently, interaction of certain signal molecules and growth regulators with H2S application has been investigated in order to regulate plant growth against heavy metal stress. Nonetheless, future work on other emerging signaling molecules and phytohormones is highly desirable, which can provide us a better understanding of these signaling molecules that affect the concentration of plant hormones and thus control the toxicity of metals in plants. Moreover, further investigations at genomics, transcriptomic, and metabolomics scale are required to explore the particular H2S-generated tolerance mechanism in various plants against heavy metal stress. On the other hand, effective, widespread, and ongoing field trials using organic amendments and/or metal-tolerant microbial inoculant can also be tested for their protective role against the exposed contaminants, which can also upscale the effectiveness of H2S in heavy metal-contaminated degraded land.
All authors listed have made a substantial, direct and intellectual contribution to the work, and approved it for publication.
This research was funded by the Deanship of Scientific Research at Princess Nourah Bint Abdulrahman University through the Fast-track Research Funding Program.
The authors declare that the research was conducted in the absence of any commercial or financial relationships that could be construed as a potential conflict of interest.
The authors highly acknowledge the Government College University, Faisalabad, Pakistan for its support. This work was financially supported by Fast-track Research Funding Program under the Deanship of Scientific Research at Princess Nourah Bint Abdulrahman University.
Ahmad, I., Akhtar, M. J., Zahir, Z. A., and Jamil, A. (2012). Effect of cadmium on seed germination and seedling growth of four wheat (Triticum aestivum L.) cultivars. Pak. J. Bot. 5, 1569–1574.
Ahmad, M. S., Ashraf, M., and Hussain, M. (2011). Phytotoxic effects of nickel on yield and concentration of macro- and micro-nutrients in sunflower (Helianthus annuus L.) achenes. J. Hazard. Mater. 185, 1295–1303. doi: 10.1016/j.jhazmat.2010.10.045
Ahmad, P., Sarwat, M., Bhat, N. A., Wani, M. R., Kazi, A. G., Tran, L. S., et al. (2015). Alleviation of cadmium toxicity in Brassica juncea L. by calcium application involves various physiological and biochemical strategies. PLoS One 10:e0114571. doi: 10.1371/journal.pone.0114571
Ahmad, R., Ali, S., Rizwan, M., Dawood, M., Farid, M., Hussain, A., et al. (2020). Hydrogen sulfide alleviates chromium stress on cauliflower by restricting its uptake and enhancing antioxidative system. Physiol. Plant. 168, 289–300.
Akaike, T., Ida, T., Wei, F.-Y., Nishida, M., Kumagai, Y., Alam, M. M., et al. (2017). Cysteinyl-tRNA synthetase governs cysteine polysulfidation and mitochondrial bioenergetics. Nat. Commun. 8:1177.
Alamri, S., Ali, H. M., Khan, M. I. R., Singh, V. P., and Siddiqui, M. H. (2020). Exogenous nitric oxide requires endogenous hydrogen sulfide to induced the resilience through sulfur assimilation in tomato seedlings under hexavalent chromium toxicity. Plant Physiol. Biochem. 155, 20–34. doi: 10.1016/j.plaphy.2020.07.003
Alloway, B. J. (2013). Heavy Metals in Soils: Trace Metals and Metalloids in Soils and Their Bioavailability, 3rd Edn. Glasgow: Blackie Academic and Professional.
Amari, T., Ghnaya, T., and Abdelly, C. (2017). Nickel, cadmium and lead phytotoxicity and potential of halophytic plants in heavy metal extraction. South Afr. J. Bot. 111, 99–110. doi: 10.1016/j.sajb.2017.03.011
Arif, M. S., Yasmeen, T., Shahzad, S. M., Riaz, M., Rizwan, M., Iqbal, S., et al. (2019). Lead toxicity induced phytotoxic effects on mung bean can be relegated by lead tolerant Bacillus subtilis (PbRB3). Chemosphere 234, 70–80. doi: 10.1016/j.chemosphere.2019.06.024
Aroca, A., Gotor, C., Bassham, D. C., and Romero, L. C. (2020). Hydrogen sulfide: from a toxic molecule to a key molecule of cell life. Antioxidants. 9:621. doi: 10.3390/antiox9070621
Aroca, A., Serna, A., Gotor, C., and Romero, L. C. (2015). S-sulfhydration: a cysteine posttranslational modification in plant systems. Plant Physiol. 168, 334–342. doi: 10.1104/pp.15.00009
Azevedo, R. A., and Lea, P. J. (2005). Toxic metals in plants. Br. J. Plant Physiol. 17:1. doi: 10.1590/s1677-04202005000100001
Aziz, H., Sabir, M., Ahmad, H. R., Aziz, T., Zia-ur-Rehman, M., Hakeem, K. R., et al. (2015). Alleviating effect of calcium on nickel toxicity in rice. Clean Soil Air Water 43, 787–866.
Bai, X. Y., Dong, Y. J., Wang, Q. H., Xu, L. L., Kong, J., and Liu, S. (2014). Effects of lead and nitric oxide on photosynthesis, antioxidative ability, and mineral element content of perennial ryegrass. Biol. Plant. 59, 163–170. doi: 10.1007/s10535-014-0476-8
Bettendorff, L., and Wins, P. (2013). Thiamine triphosphatase and the CYTH superfamily of proteins. FEBS J. 280, 6443–6455. doi: 10.1111/febs.12498
Bharwana, S. A., Ali, S., Farooq, M. A., Ali, B., Iqbal, N., Abbas, F., et al. (2014). Hydrogen sulfide ameliorates lead-induced morphological, photosynthetic, oxidative damages and biochemical changes in cotton. Environ. Sci. Pollut. Res. 21, 717–731. doi: 10.1007/s11356-013-1920-6
Bhuyan, M. H. M. B., Parvin, K., Mohsin, S. M., Mahmud, J. A., Hasanuzzaman, M., and Fujita, M. (2020). Modulation of cadmium tolerance in rice: insight into vanillic acid-induced upregulation of antioxidant defense and glyoxalase systems. Plants 9:188. doi: 10.3390/plants9020188
Calderwood, A., and Kopriva, S. (2014). Hydrogen sulfide in plants: from dissipation of excess sulfur to signaling molecule. Nitric Oxide 41, 72–78. doi: 10.1016/j.niox.2014.02.005
Carmo-Silva, E., Scales, J. C., Madgwick, P. J., and Parry, M. A. J. (2015). Optimizing Rubisco and its regulation for greater resource use efficiency. Plant Cell Environ. 38, 1817–1832. doi: 10.1111/pce.12425
Chen, J., Wu, F. H., Wang, W. H., Zheng, C. J., Lin, G. H., Dong, X. J., et al. (2011). Hydrogen sulfide enhances photosynthesis through promoting chloroplast biogenesis, photosynthetic enzyme expression, and thiol redox modification in Spinacia oleracea seedlings. J. Exp. Bot. 62, 4481–4493. doi: 10.1093/jxb/err145
Chen, T., Tian, M., and Han, Y. (2020). Hydrogen sulfide: a multi-tasking signal molecule in the regulation of oxidative stress responses. J. Exp. Bot. 71, 2862–2869. doi: 10.1093/jxb/eraa093
Chen, Z., Chen, M., and Jiang, M. (2016). Hydrogen sulfide alleviates mercury toxicity by sequestering it in roots or regulating reactive oxygen species productions in rice seedlings. Plant Physiol. Biochem. 111, 179–192. doi: 10.1016/j.plaphy.2016.11.027
Chen, Z., Yang, B., Hao, Z., Zhu, J., Zhang, Y., and Xu, T. (2018). Exogenous hydrogen sulfide ameliorates seed germination and seedling growth of cauliflower under lead stress and its antioxidant role. J. Plant Growth Regul. 37, 5–15. doi: 10.1007/s00344-017-9704-8
Corpas, F. J., Barroso, J. B., Gonzalez-Gordo, S., Munoz-Vargas, M. A., and Palma, J. M. (2019a). Hydrogen sulfide: a novel component in Arabidopsis peroxisomes which triggers catalase inhibition. J. Integr. Plant Biol. 61, 871–883.
Corpas, F. J., González-Gordo, S., Cañas, A., and Palma, J. M. (2019b). Nitric oxide and hydrogen sulfide in plants: which comes first? J. Exp. Bot. 70, 4391–4404. doi: 10.1093/jxb/erz031
Cuevasanta, E., Moller, M. N., and Alvarez, B. (2017). Biological chemistry of hydrogen sulfide and persulfides. Arch. Biochem. Biophys. 617, 9–25. doi: 10.1016/j.abb.2016.09.018
Cummins, P. L., Kannappan, B., and Gready, J. E. (2018). Directions for optimization of photosynthetic carbon fixation: RuBisCO’s efficiency may not be so constrained after all. Front. Plant Sci. 9:183.
De Kok, L. J., Castro, A., Durenkamp, M., Stuiver, C. E. E., Westerman, S., Yang, L., et al. (2002). “Sulphur in plant physiology,” in Proceedings No 500, The International Fertiliser Society, New York, NY. doi: 10.1016/j.plaphy.2016.12.024
DeLeon, E. R., Stoy, G. F., and Olson, K. R. (2012). Passive loss of hydrogen sulfide in biological experiments. Anal. Biochem. 421, 203–207. doi: 10.1016/j.ab.2011.10.016
Duffus, J. H. (2002). “Heavy metals” a meaningless term? (IUPAC Technical Report). Pure appl. Chem. 74, 793–807. doi: 10.1351/pac200274050793
Fang, L., Ju, W., Yang, C., Jin, X., Liu, D., Li, M., et al. (2020). Exogenous application of signaling molecules to enhance the resistance of legume-rhizobium symbiosis in Pb/Cd-contaminated soils. Environ. Pollut. 265:114744. doi: 10.1016/j.envpol.2020.114744
Filipovic, M. R., Zivanovic, J., Alvarez, B., and Banerjee, R. (2018). Chemical biology of H2S signaling through persulfidation. Chem. Rev. 118, 1253–1337. doi: 10.1021/acs.chemrev.7b00205
Gonzalez, A., Cabrera, M., de, L., Henriquez, M. J., Contreras, R. A., Morales, B., et al. (2012). Cross talk among calcium, hydrogen peroxide, and nitric oxide and activation of gene expression involving calmodulins and calcium-dependent protein kinases in Ulva compressa exposed to copper excess. Plant Physiol. 158, 1451–1462. doi: 10.1104/pp.111.191759
Gotor, C., García, I., Aroca, Á, Laureano-Marín, A. M., Arenas-Alfonseca, L., Jurado-Flores, A., et al. (2019). Signaling by hydrogen sulfide and cyanide through post-translational modification. J. Exp.Bot. 70, 4251–4265. doi: 10.1093/jxb/erz225
Han, Y., Yin, Y., and Yi, H. (2020). Decreased endogenous nitric oxide contributes to sulfur dioxide derivative alleviated cadmium toxicity in Foxtail millet roots. Environ. Exp. Bot. 177:104144. doi: 10.1016/j.envexpbot.2020.104144
Hancock, J. T. (2017). Harnessing evolutionary toxins for signaling: reactive oxygen species, nitric oxide and hydrogen sulfide in plant cell regulation. Front. Plant. Sci. 8:189.
Hancock, J. T., and Whiteman, M. (2016). Hydrogen sulfide signaling: interactions with nitric oxide and reactive oxygen species. Ann. N. Y. Acad. Sci. 1365, 5–14. doi: 10.1111/nyas.12733
Hasanuzzaman, M., Matin, M. A., Fardus, J., Hasanuzzaman, M., Hossain, M. S., and Parvin, K. (2019). Foliar application of salicylic acid improves growth and yield attributes by upregulating the antioxidant defense system in Brassica campestris plants grown in lead-amended soils. Acta Agro. Bot. 72:1765.
Hasanuzzaman, M., Nahar, K., Anee, T. I., and Fujita, M. (2017). Exogenous silicon attenuates cadmium-induced oxidative stress in Brassica napus L. by modulating AsA-GSH pathway and glyoxalase system. Front. Plant Sci. 8:1061.
He, H., Li, Y., and He, L. F. (2019). Role of nitric oxide and hydrogen sulfide in plant aluminum tolerance. Biometals 32, 1–9. doi: 10.1007/s10534-018-0156-9
Hoque, T. S., Hossain, M. A., Mostofa, M. G., Burritt, D. J., Fujita, M., and Tran, L. S. P. (2016). Methylglyoxal: an emerging signaling molecule in plant abiotic stress responses and tolerance. Front. Plant Sci. 7:1341.
Hu, Y., Lu, L., Tian, S., Li, S., Liu, X., Gao, X., et al. (2019). Cadmium-induced nitric oxide burst enhances Cd tolerance at early stage in roots of a hyperaccumulator Sedum alfredii partially by altering glutathione metabolism. Sci. Total Environ. 650, 2761–2770. doi: 10.1016/j.scitotenv.2018.09.269
Huo, J., Huang, D., Zhang, J., Fang, H., Wang, B., Wang, C., et al. (2018). Hydrogen sulfide: a gaseous molecule in postharvest freshness. Front. Plant Sci. 27:1172.
Ida, T., Sawa, T., Ihara, H., Tsuchiya, Y., Watanabe, Y., Kumagai, Y., et al. (2014). Reactive cysteine persulfides and S-polythiolation regulate oxidative stress and redox signaling. Proc. Natl. Acad. Sci. U.S.A. 111, 7606–7611. doi: 10.1073/pnas.1321232111
Jalmi, S. K., Bhagat, P. K., Verma, D., Noryang, S., Tayyeba, S., Singh, K., et al. (2018). Traversing the links between heavy metal stress and plant signaling. Front. Plant Sci. 9:12.
Jia, H., Wang, X., Dou, Y., Liu, D., Si, W., Fang, H., et al. (2016). Hydrogen sulfide - cysteine cycle system enhances cadmium tolerance through alleviating cadmium-induced oxidative stress and ion toxicity in Arabidopsis roots. Sci. Rep. 6:39702.
Jia, H., Wang, X., Shi, C., Guo, J., Ma, P., Wei, T., et al. (2020). Hydrogen sulfide decreases Cd translocation from root to shoot through increasing Cd accumulation in cell wall and decreasing Cd2+ influx in Isatis indigotica. Plant Physiol. Biochem. 155, 605–612. doi: 10.1016/j.plaphy.2020.08.033
Kabil, O., and Banerjee, R. (2010). Redox biochemistry of hydrogen sulfide. J. Biol. Chem. 285, 21903–21907. doi: 10.1074/jbc.r110.128363
Karam, E. A., and Keramat, B. (2017). Hydrogen sulfide protects coriander seedlings against copper stress by regulating the ascorbate-glutathione cycle in leaves. J. Plant Process Funct. 5, 59–64.
Kasamatsu, S., Nishimura, A., Morita, M., Matsunaga, T., Abdul Hamid, H., and Akaike, T. (2016). Redox signaling regulated by cysteine persulfide and protein polysulfidation. Molecules 21:E1721.
Kaya, C. (2020). Salicylic acid-induced hydrogen sulphide improves lead stress tolerance in pepper plants by upraising the ascorbate-glutathione cycle. Physiol. Plant [Epub ahead of print]. doi: 10.1111/ppl.13159
Kaya, C., Akram, N. A., Ashraf, M., Alyemeni, M. N., and Ahmad, P. (2020). Exogenously supplied silicon (Si) improves cadmium tolerance in pepper (Capsicum annuum L.) by up-regulating the synthesis of nitric oxide and hydrogen sulfide. J. Biotechnol. 316, 35–45. doi: 10.1016/j.jbiotec.2020.04.008
Kaya, C., Ashraf, M., and Akram, N. A. (2018). Hydrogen sulfide regulates the levels of key metabolites and antioxidant defense system to counteract oxidative stress in pepper (Capsicum annuum L.) plants exposed to high zinc regime. Environ. Sci. Pollut. Res. 25, 12612–12618. doi: 10.1007/s11356-018-1510-8
Kaya, C., and Aslan, M. (2020). Hydrogen sulphide partly involves in thiamine-induced tolerance to cadmium toxicity in strawberry (Fragaria x ananassa Duch) plants. Environ. Sci. Pollut. Res. 27, 941–953. doi: 10.1007/s11356-019-07056-z
Kharbech, O., Massoud, M. B., Sakouhi, L., Djebali, W., Mur, L. A. J., and Chaoui, A. (2020). Exogenous application of hydrogen sulfide reduces chromium toxicity in maize seedlings by suppressing NADPH oxidase activities and methylglyoxal accumulation. Plant Physiol. Biochem. 154, 646–656. doi: 10.1016/j.plaphy.2020.06.002
Kimura, Y., Koike, S., Shibuya, N., Lefer, D., Ogasawara, Y., and Kimura, H. (2017). 3-Mercaptopyruvate sulfurtransferase produces potential redox regulators cysteine- and glutathione-persulfide (Cys-SSH and GSSH) together with signaling molecules H2S2, H2S3 and H2S. Sci. Rep. 7:10459.
Krueger, S., Niehl, A., Martin, M. C., Steinhauser, D., Donath, A., Hildebrandt, T., et al. (2009). Analysis of cytosolic and plastidic serine acetyltransferase mutants and subcellular metabolite distributions suggests interplay of the cellular compartments for cysteine biosynthesis in Arabidopsis. Plant Cell Environ. 32, 349–367. doi: 10.1111/j.1365-3040.2009.01928.x
Krzesłowska, M. (2011). The cell wall in plant cell response to trace metals: polysaccharide remodeling and its role in defense strategy. Acta Physiol. Plant. 33, 35–51. doi: 10.1007/s11738-010-0581-z
Kushwaha, B. K., and Singh, V. P. (2019). Glutathione and hydrogen sulfide are required for sulfur-mediated mitigation of Cr (VI) toxicity in tomato, pea and brinjal seedlings. Physiol. Plant 168, 406–421.
Lado, L. R., Hengl, T., and Reuter, H. I. (2008). Heavy metals in European soils: a geostatistical analysis of the FOREGS geochemical database. Geoderma 148, 189–199. doi: 10.1016/j.geoderma.2008.09.020
Lai, H. Y. (2015). Subcellular distribution and chemical forms of cadmium in Impatiens walleriana in relation to its phytoextraction potential. Chemosphere 138, 370–376. doi: 10.1016/j.chemosphere.2015.06.047
Lasat, M. M. (2002). Phytoextraction of toxic metals A review of biological mechanism. J Environ. Qual. 31, 109–120. doi: 10.2134/jeq2002.1090
Li, L., Wang, Y., and Shen, W. (2012). Roles of hydrogen sulphide and nitric oxide in the alleviation of cadmium-induced oxidative damage in alfalfa seedling roots. Biometals 25, 617–631. doi: 10.1007/s10534-012-9551-9
Li, Z. G. (2013). Hydrogen sulfide: a multifunctional gaseous molecule in plants. Russ. J. Plant Physiol. 60, 733–740. doi: 10.1134/s1021443713060058
Li, Z. G., Min, X., and Zhou, Z. H. (2016). Hydrogen sulfide: a signal molecule in plant cross-adaptation. Front. Plant Sci. 7:1621.
Lin, Y. T., Li, M. Y., Cui, W. T., Lu, W., and Shen, W. B. (2012). Haem oxygenase-1 is involved in hydrogen sulfide-induced cucumber adventitious root formation. J. Plant Growth Regul. 3, 519–528. doi: 10.1007/s00344-012-9262-z
Lisjak, M., Srivastava, N., Teklic, T., Civale, L., Lewandowski, K., Wilson, I., et al. (2010). A novel hydrogen sulfide donor causes stomatal opening and reduces nitric oxide accumulation. Plant Physiol. Biochem. 48, 931–935. doi: 10.1016/j.plaphy.2010.09.016
Liu, T. J., Liu, X. N., Liu, M. L., and Wu, L. (2018). Evaluating heavy metal stress levels in rice based on remote sensing phenology. Sensors 18:860. doi: 10.3390/s18030860
Liu, X., Chen, J., Wang, G.-H., Wang, W.-H., Shen, Z.-J., Luo, M.-R., et al. (2016). Hydrogen sulfide alleviates zinc toxicity by reducing zinc uptake and regulating genes expression of antioxidative enzymes and metallothioneins in roots of the cadmium/zinc hyperaccumulator Solanum nigrum L. Plant Soil 400, 177–192. doi: 10.1007/s11104-015-2719-7
Luo, S., Calderón-Urrea, A., Yu, J., Liao, W., Xie, J., Lv, J., et al. (2020). The role of hydrogen sulfide in plant alleviates heavy metal stress. Plant Soil 449, 1–10. doi: 10.1007/s11104-020-04471-x
Mancardi, D., Penna, C., Merlino, A., Del Soldato, P., Wink, D. A., and Pagliaro, P. (2009). Physiological and pharmacological features of the novel gasotransmitter: hydrogen sulfide. BBA Bioenerget. 1787, 864–872. doi: 10.1016/j.bbabio.2009.03.005
Mataka, L. M., Henry, E. M. T., Masamba, W. R. L., and Sajidu, S. M. (2006). Lead remediation of contaminated water using Moringa stenopetala sp., and Moringa oleifera sp., seed powder. Inter. J. Environ. Sci. Tech. 2, 131–139. doi: 10.1007/bf03325916
Mostofa, M. G., Rahman, A., Ansary, M. M. U., Watanabe, A., Fujita, M., and Tran, L. S. P. (2015). Hydrogen sulfide modulates cadmium-induced physiological and biochemical responses to alleviate cadmium toxicity in rice. Sci. Rep. 5:14078.
Mustafa, A. K., Gadalla, M. M., Sen, N., Kim, S., Mu, W., Gazi, S. K., et al. (2009). H2S signals through protein S-sulfhydration. Sci. Signal. 2:ra72. doi: 10.1126/scisignal.2000464
Nosaka, K. (2006). Recent progress in understanding thiamin biosynthesis and its genetic regulation in Saccharomyces cerevisiae. Appl. Microbiol. Biotechnol. 72, 30–40. doi: 10.1007/s00253-006-0464-9
Pandey, A. K., and Gautam, A. (2019). Stress responsive gene regulation in relation to hydrogen sulfide in plants under abiotic stress. Physiol. Plant 168, 511–525.
Paul, B. D., and Snyder, S. H. (2012). H2S signalling through protein sulfhydration and beyond. Nat. Rev. Mol. Cell Biol. 13, 499–507. doi: 10.1038/nrm3391
Peng, B., and Xian, M. (2015). Hydrogen sulfide detection using nucleophilic substitution-cyclization-based fluorescent probes. Methods Enzymol. 554, 47–62. doi: 10.1016/bs.mie.2014.11.030
Rather, B. A., Mir, I. R., Sehar, Z., Anjum, N. A., Masood, A., and Khan, N. A. (2020). The outcomes of the functional interplay of nitric oxide and hydrogen sulfide in metal stress tolerance in plants. Plant Physiol. Biochem. 155, 523–534. doi: 10.1016/j.plaphy.2020.08.005
Rausch, T., and Wachter, A. (2005). Sulfur metabolism: a versatile platform for launching defence operations. Trends Plant Sci. 10, 503–509. doi: 10.1016/j.tplants.2005.08.006
Raza, A., Charagh, S., Zahid, Z., Mubarik, M. S., Javed, R., Siddiqui, M. H., et al. (2020). Jasmonic acid: a key frontier in conferring a biotic stress tolerance in plants. Plant Cell Rep. [Epub ahead of print]. doi: 10.1007/s00299-020-02614-z
Reichman, S. M. (2007). The potential use of the legume-rhizobium symbiosis for the remediation of arsenic contaminated sites. Soil Biol. Biochem. 39, 2587–2593. doi: 10.1016/j.soilbio.2007.04.030
Rennenberg, H. (1983). Role of O-acetylserine in hydrogen sulfide emission from pumpkin leaves in response to sulfate. Plant Physiol. 73, 560–565. doi: 10.1104/pp.73.3.560
Rennenberg, H. (1984). The fate excess of sulfur in higher plants. Annu. Rev. Plant Physiol. 35, 121–153. doi: 10.1146/annurev.pp.35.060184.001005
Rennenberg, H., Huber, B., Schroder, P., Stahl, K., Haunold, W., Georgii, H. W., et al. (1990). Emission of volatile sulfur compounds from spruce trees. Plant Physiol. 92, 560–564. doi: 10.1104/pp.92.3.560
Riemenschneider, A., Nikiforova, V., Hoefgen, R., De Kok, L. J., and Papenbrock, J. (2005a). Impact of elevated H2S on metabolite levels, activity of enzymes and expression of genes involved in cysteine metabolism. Plant Physiol. Biochem. 43, 473–448. doi: 10.1016/j.plaphy.2005.04.001
Riemenschneider, A., Riedel, K., Hoefgen, R., Papenbrok, J., and Hesse, H. (2005b). Impact of reduced O-acetylserine (thiol) lyase iso-form contents on potato plant metabolism. Plant Physiol. 137, 892–900. doi: 10.1104/pp.104.057125
Rizwan, M., Mostofa, M. G., Ahmad, M. Z., Imtiaz, M., Mehmood, S., Adeel, M., et al. (2018). Nitric oxide induces rice tolerance to excessive nickel by regulating nickel uptake, reactive oxygen species detoxification and defense- related gene expression. Chemosphere 191, 23–35. doi: 10.1016/j.chemosphere.2017.09.068
Rizwan, M., Mostofa, M. G., Ahmad, M. Z., Zhou, Y., Adeel, M., Mehmood, S., et al. (2019). Hydrogen sulfide enhances rice tolerance to nickel through the prevention of chloroplast damage and the improvement of nitrogen metabolism under excessive nickel. Plant Physiol. Biochem. 138, 100–111. doi: 10.1016/j.plaphy.2019.02.023
Rubright, S. L. M., Pearce, L. L., and Peterson, J. (2017). Environmental toxicology of hydrogen sulfide Nitric Oxide. Biol. Chem. 71:1. doi: 10.1016/j.niox.2017.09.011
Schutzendubel, A., and Polle, A. (2002). Plant responses to abiotic stresses: heavy metal-induced oxidative stress and protection by mycorrhization. J. Exp. Bot. 53, 1351–1365. doi: 10.1093/jexbot/53.372.1351
Sekiya, J., Schmidt, A., Wilson, L. G., and Filner, P. (1982a). Emission of hydrogen sulfide by leaf tissue in response to L-cysteine. Plant Physiol. 70, 430–436. doi: 10.1104/pp.70.2.430
Sekiya, J., Wilson, L. G., and Filner, P. (1982b). Resistance to injury by sulfur dioxide: correlation with its reduction to, and emission of, hydrogen sulfide in Cucurbitaceae. Plant Physiol. 70, 437–441. doi: 10.1104/pp.70.2.437
Shan, C., Dai, H., and Sun, Y. (2012). Hydrogen sulfide protects wheat seedlings against copper stress by regulating the ascorbate and glutathione metabolism in leaves. Aust. J. Crop Sci. 6, 248–254.
Shan, C. J., Zhang, S. L., Li, D. F., Zhao, Y. Z., Tian, X. L., Zhao, X. L., et al. (2011). Effects of exogenous hydrogen sulfide on the ascorbate and glutathione metabolism in wheat seedlings leaves under water stress. Acta. Physiol. Plant. 33, 2533–2540. doi: 10.1007/s11738-011-0746-4
Sharma, A., and Dhiman, A. (2013). Nickel and cadmium toxicity in plants. J. Pharm. Sci. Innov. 2, 20–24. doi: 10.7897/2277-4572.02213
Sharma, A., Sidhu, G. P. S., Araniti, F., Bali, A. S., Shahzad, B., Tripathi, D. K., et al. (2020b). The role of salicylic acid in plants exposed to heavy metals. Molecules 25:540. doi: 10.3390/molecules25030540
Sharma, A., Soares, C., Sousa, B., Martins, M., Kumar, V., Shahzad, B., et al. (2020a). Nitric oxide-mediated regulation of oxidative stress in plants under metal stress: a review on molecular and biochemical aspects. Physiol. Planta. 168, 318–344. doi: 10.1111/ppl.13004
Shen, G. T., Ju, W. L., Liu, Y. Q., Guo, X. B., Zhao, W., and Fang, L. C. (2019). Impact of urea addition and rhizobium inoculation on plant resistance in metal contaminated soil. Int. J. Environ. Res. Publ. Health 16:1955. doi: 10.3390/ijerph16111955
Shivaraj, S. M., Vats, S., Bhat, J. A., Dhakte, P., Goyal, V., Khatri, P., et al. (2020). Nitric oxide and hydrogen sulfide crosstalk during heavy metal stress in plants. Physiol. Planta 168, 437–455.
Singh, V. P., Singh, S., Kumar, J., and Prasad, S. M. (2015). Hydrogen sulfide alleviates toxic effects of arsenate in pea seedlings through up-regulation of the ascorbate-glutathione cycle: possible involvement of nitric oxide. J. Plant Physiol. 181, 20–29. doi: 10.1016/j.jplph.2015.03.015
Takahashi, H., Kopriva, S., Giordano, M., Saito, K., and Hell, R. (2011). Sulfur assimilation in photosynthetic organisms: molecular functions and regulations of transporters and assimilatory enzymes. Annu. Rev. Plant Biol. 62, 157–184. doi: 10.1146/annurev-arplant-042110-103921
Thompson, C. R., and Kats, G. (1978). Effects of continuous hydrogen sulphide fumigation on crop and forest plants. Environ. Sci. Technol. 12, 550–553. doi: 10.1021/es60141a001
Tian, B., Qiao, Z., Zhang, L., Li, H., and Pei, Y. (2016). Hydrogen sulfide and proline coperate to alleviate cadmium stress in foxtail millet seedlings. Plant Physiol. Biochem. 109, 293–299. doi: 10.1016/j.plaphy.2016.10.006
Tian, B., Zhang, Y., Jin, Z., Liu, Z., and Pei, Y. (2017). Role of hydrogen sulfide in the methyl jasmonate response to cadmium stress in Foxtail millet. Front. Biosci. Landmark 225:30–538.
Toohey, J. I. (2011). Sulfur signaling: is the agent sulfide or sulfane? Anal. Biochem. 413, 1–7. doi: 10.1016/j.ab.2011.01.044
Vaculík, M., Lukačová, Z., Bokor, B., Martinka, M., Tripathi, D. K., and Lux, A. (2020). Alleviation mechanisms of metal(loid) stress in plants by silicon: a review. J. Exp. Bot. 71, 6744–6757. doi: 10.1093/jxb/eraa288
Valivand, M., and Amooaghaie, R. (2020). Sodium hydrosulfide modulates membrane integrity, cation homeostasis, and accumulation of phenolics and osmolytes in Zucchini under nickel stress. J. Plant Growth Regul. [Epub ahead of print]. doi: 10.1007/s00344-020-10101-8
Valivand, M., Amooaghaie, R., and Ahadi, A. M. (2019). Interplay between hydrogen sulfide and calcium/calmodulin enhances systemic acquired acclimation and antioxidative defense against nickel toxicity in zucchini. Environ. Exp. Bot. 158, 40–50. doi: 10.1016/j.envexpbot.2018.11.006
Wang, H., Ji, F., Zhang, Y., Hou, J., Liu, W., Huang, J., et al. (2019). Interactions between hydrogen sulphide and nitric oxide regulate two soybean citrate transporters during the alleviation of aluminium toxicity. Plant Cell Environ. 42, 2340–2356. doi: 10.1111/pce.13555
Wang, K., Peng, H., and Wang, B. (2014). Recent advances in thiol and sulfide reactive probes. J. Cell Biochem. 115, 1007–1022. doi: 10.1002/jcb.24762
Wang, S., Chi, Q., Hu, X., Cong, Y., and Li, S. (2019). Hydrogen sulfide-induced oxidative stress leads to excessive mitochondrial fission to activate apoptosis in broiler myocardia. Ecotoxicol. Environ. Saf. 183, 1–9.
Wang, Y. Q., Li, L., Cui, W. T., Xu, S., Shen, W. B., and Wang, R. (2012). Hydrogen sulfide enhances alfalfa (Medicago sativa) tolerance against salinity during seed germination by nitric oxide pathway. Plant Soil 351, 107–119. doi: 10.1007/s11104-011-0936-2
Wilson, L. G., Bressan, R. A., and Filner, P. (1978). Light-dependent emission of hydrogen sulfide from plants. Plant Physiol. 61, 184–189. doi: 10.1104/pp.61.2.184
Xuan, L., Li, J., Wang, X., and Wang, C. (2020). Crosstalk between hydrogen sulfide and other signal molecules regulates plant growth and development. Int. J. Mol. Sci. 21:4593. doi: 10.3390/ijms21134593
Yamasaki, H., and Cohen, M. F. (2016). Biological consilience of hydrogen sulfide and nitric oxide in plants: gases of primordial earth linking plant, microbial and animal physiologies. Nitric Oxide 55, 91–100. doi: 10.1016/j.niox.2016.04.002
Yang, G., Wu, L., Jiang, B., Yang, W., Qi, J., Cao, K., et al. (2008). H2S as a physiologic vasorelaxant, hypertension in mice with deletion of cystathionine γ-lyase. Science 322, 587–590. doi: 10.1126/science.1162667
Yu, X. Z., Ling, Q. L., Li, Y. H., and Lin, Y. J. (2018). mRNA analysis of genes encoded with Phytochelatin Synthase (PCS) in rice seedlings exposed to chromium: the role of phytochelatins in Cr detoxification. Bull. Environ. Contam. Toxicol. 101, 257–261. doi: 10.1007/s00128-018-2362-0
Yu, Y., Zhou, X. Y., Zhu, Z. H., and Zhou, K. J. (2019). Sodium hydrosulfide mitigates cadmium toxicity by promoting cadmium retention and inhibiting its translocation from roots to shoots in Brassica napus. J. Agric. Food Chem. 67, 433–440. doi: 10.1021/acs.jafc.8b04622
Yusof, Z. N. B., Borhan, F. P., Mohamad, F. A., and Rusli, M. H. (2015). The effect of Ganoderma boninense infection on the expressions of thiamine (vitamin B1) biosynthesis genes in oil palm. J. Oil Palm Res. 27, 12–18.
Zanganeh, R., Jamei, R., and Rahmani, F. (2018). Impacts of seed priming with salicylic acid and sodium hydrosulfide on possible metabolic pathway of two amino acids in maize plant under lead stress. Mol. Biol. Res. Comm. 7, 83–87.
Zanganeh, R., Jamei, R., and Rahmani, F. (2019). Role of salicylic acid and hydrogen sulfide in promoting lead stress tolerance and regulating free amino acid composition in Zea mays L. Acta Physiol. Plant. 41:94.
Zhang, H., Hua, S. L., Zhang, Z. J., Hua, L. Y., Jiang, C. X., Wei, Z. J., et al. (2011). Hydrogen sulfide acts as a regulator of flower senescence in plants. Postharvest Biol. Technol. 60, 251–257. doi: 10.1016/j.postharvbio.2011.01.006
Zhang, H., Hu, L. Y., Hu, K. D., Jiang, C. X., and Luo, J. P. (2010a). Hydrogen sulfide alleviated chromium toxicity in wheat. Biol. Plant. 54, 743–747. doi: 10.1007/s10535-010-0133-9
Zhang, H., Tan, Z. Q., Hu, L. Y., Wang, S. H., Luo, J. P., and Jones, R. L. (2010b). Hydrogen sulfide alleviates aluminum toxicity in germinating wheat seedlings. J. Integr. Plant Biol. 52, 556–567. doi: 10.1111/j.1744-7909.2010.00946.x
Zhang, H., Tang, J., Liu, X. P., Wang, Y., Yu, W., Peng, W. Y., et al. (2009). Hydrogen sulfide promotes root organogenesis in Ipomoea batatas, Salix matsudana and Glycine max. J. Integr. Plant Biol. 51, 1084–1092.
Zheng, S., Wang, Q., Yuan, Y., and Sun, W. (2020). Human health risk assessment of heavy metals in soil and food crops in the Pearl River Delta urban agglomeration of China. Food Chem. 316:126213. doi: 10.1016/j.foodchem.2020.126213
Zhi, J., Liu, X., Yin, P., Yang, R., Liu, J., and Xu, J. (2020). Overexpression of the metallothionein gene PaMT3-1 from Phytolacca americana enhances plant tolerance to cadmium. Plant Cell Tissue Organ. Cult. 143, 211–218. doi: 10.1007/s11240-020-01914-2
Keywords: biochemical properties, physiological activities, heavy metal stress, hydrogen sulfide, signaling molecule, oxidative impairment
Citation: Arif MS, Yasmeen T, Abbas Z, Ali S, Rizwan M, Aljarba NH, Alkahtani S and Abdel-Daim MM (2021) Role of Exogenous and Endogenous Hydrogen Sulfide (H2S) on Functional Traits of Plants Under Heavy Metal Stresses: A Recent Perspective. Front. Plant Sci. 11:545453. doi: 10.3389/fpls.2020.545453
Received: 25 March 2020; Accepted: 27 November 2020;
Published: 07 January 2021.
Edited by:
Mukesh Kumar Kanwar, Zhejiang University, ChinaReviewed by:
Elke Bloem, Julius Kühn-Institut, GermanyCopyright © 2021 Arif, Yasmeen, Abbas, Ali, Rizwan, Aljarba, Alkahtani and Abdel-Daim. This is an open-access article distributed under the terms of the Creative Commons Attribution License (CC BY). The use, distribution or reproduction in other forums is permitted, provided the original author(s) and the copyright owner(s) are credited and that the original publication in this journal is cited, in accordance with accepted academic practice. No use, distribution or reproduction is permitted which does not comply with these terms.
*Correspondence: Tahira Yasmeen, cmlkYV9ha2FzaEBob3RtYWlsLmNvbQ==; dGFoaXJheWFzbWVlbkBnY3VmLmVkdS5waw==; Shafaqat Ali, c2hhZmFxYXRhbGlnaWxsQHlhaG9vLmNvbQ==; c2hhZmFxYXRhbGlnaWxsQGdjdWYuZWR1LnBr
Disclaimer: All claims expressed in this article are solely those of the authors and do not necessarily represent those of their affiliated organizations, or those of the publisher, the editors and the reviewers. Any product that may be evaluated in this article or claim that may be made by its manufacturer is not guaranteed or endorsed by the publisher.
Research integrity at Frontiers
Learn more about the work of our research integrity team to safeguard the quality of each article we publish.