- 1Department of Genomics, Institute of Plant Genetics of the Polish Academy of Sciences, Poznañ, Poland
- 2Institute of Biological, Environmental and Rural Sciences, Aberystwyth University, Aberystwyth, United Kingdom
- 3Department of Genetics and Plant Breeding, Poznañ University of Life Sciences, Poznañ, Poland
Alien chromosome introgression has become a valuable tool to broaden the genetic variability of crop plants via chromosome engineering. This study details the procedure to obtain monosomic addition and monosomic substitution lines of the triticale carrying 2Sk chromosome from Aegilops kotchyi Boiss., which harbors Lr54 + Yr37 leaf and stripe rust-resistant gene loci, respectively. Initially, A. kotschyi × Secale cereale artificial amphiploids (2n = 6x = 42 chromosomes, UUSSRR) were crossed with triticale cv. “Sekundo” (2n = 6x = 42, AABBRR) in order to obtain fertile offspring. Cyto-molecular analyses of five subsequent backcrossing generations revealed that 2Sk chromosome was preferentially transmitted. This allowed for the selection of monosomic 2Sk addition (MA2Sk) lines of triticale. Finally, the 2Sk(2R) substitution plants were obtained by crossing MA2Sk with the nullisomic (N2R) plants of triticale. The presence of 2Sk chromosome in subsequent generations of plants was evaluated using SSR markers linked to Lr54 + Yr37 loci. Disease evaluation of the monosomic 2Sk(2R) substitution plants for the reaction to leaf and stripe rust infection were carried out under controlled conditions in a growth chamber. The results showed significant improvement of leaf rust resistance severity of monosomic substitution plants compared with control (“Sekundo”). In contrast, the introgression of the Lr54 + Yr37 loci did not lead to improvement of stripe rust resistance. In summary, the creation of monosomic addition and monosomic substitution lines of triticale is the starting point for the precise and guided transfer of Lr54 + Yr37 loci. The results showed that the developed materials could be exploited for the development of triticale varieties with resistance to leaf rust.
Introduction
Wild relatives and related species with homoeologous genomes are important for broadening the genetic variability of crop plants. For many crops, wild relatives provide a vast reservoir for most of agronomically important traits. A large number of the highest yielding wheat (Triticum aestivum L.) cultivars carry portions of alien chromatin introgression from related crops (Secale cereale L.) or weedy species (Aegilops sp., Dasypyrum sp., Elymus sp. Haynaldia sp.) (Gill et al., 2011). The Aegilops genus is the closest wild relative of bread wheat (T. aestivum; 2n = 6x = 42 chromosomes; AABBDD) or triticale (× Triticosecale Wittmack; 2n = 6x = 42; AABBRR). It contains 11 diploid, 10 tetraploid, and 2 hexaploid species and provides a vast reservoir of valuable genes, which were eliminated during the domestication and breeding of cultivated cereals.
Aegilops kotschyi Boiss. (UkUkSkSk, 2n = 4x = 28) is valuable from genetic and breeding point of view as it carries genes associated with disease resistance, drought, heat, salt tolerance, as well as other beneficial traits (Schneider et al., 2008). Rawat et al. (2009) utilized A. kotschyi as a source of genes to increase the iron and zinc content in seeds of wheat. A. kotschyi was crossed as a male parent with bread wheat line “Chinese Spring” lacking the Ph1 locus, which is a suppressor of homeologous pairing in wheat (Riley and Chapman, 1958). The aim of their study was to bring the genes associated with increased iron and zinc content in seeds, harbored in the 2Sk and 7Uk chromosomes of A. kotschyi, into the genome of wheat. They obtained only a 2Sk and 7Uk chromosome substitution line, which were not very suitable for commercial exploitation due to linkage drag. It is also reported that chromosome 2Sk of A. kotschyi possess leaf and stripe rust-resistant loci of Lr54 and Yr37 genes (Marais et al., 2005; Prażak and Paczos-Grzęda, 2013). Marais et al. (2005) have introduced A. kotschyi-derived leaf and stripe rust-resistant genes Lr54 and Yr37 to the wheat genome by the induction of 2DS.2SkL chromosome translocation.
Polyploids, such as of cultivated forms wheat or triticale, contain multiple sets of chromosomes and have highly buffered genotypes that are more permissive to benefit from alien introgression than diploids (Qi et al., 2007). Monosomic alien addition lines (MAALs) contain only one alien chromosome in addition to the receptor background chromosomes. The production of monosomic addition lines rely on backcrosses with an acceptor crop (Faris et al., 2002; Brar and Dhaliwal, 2004; Wulff and Moscou, 2014; Kwiatek and Nawracała, 2018). In traditional breeding of intraspecific hybrids, the recurrent backcrossing is commonly employed to transfer alleles at one or more loci from a donor to an elite variety (Veatch-Blohm, 2007). The expected recurrent parent genome recovery would be 99.2% at the end of six backcrossing, which is most similar to improved variety. The proportion of the recurrent parent genome is recovered at a rate of 1 − (1/2)t + 1 for each of the generations of backcrossing (Singh and Singh, 2015). When generating interspecific hybrids, backcrossing is required to generate addition, substitution, and translocation lines. In wheat and triticale, chromosome pairing between homologous chromosomes is strictly regulated by the expression of Ph1 and Ph2 loci (Riley and Chapman, 1958; Sears, 1977). Thus, subsequent backcrosses eliminate the alien chromatin gradually. However, the alien chromosome can be transmitted to the offspring preferentially, which contributes to the saturation of specific chromosomes (Endo, 2007).
Some Aegilops chromosomes were reported to be transmitted preferentially to the offspring in the process of backcrossing to produce alien chromosome addition lines of wheat (Endo, 2007) and triticale (Kwiatek et al., 2016c). The gametocidal activity of genes located on chromosome 4Ssh from A. sharonensis Eig is an example of when a chromosome is preferentially eliminated (Endo, 1990). Such chromosomes were reported to carry gametocidal factors (Gcs) (Endo, 1985). These chromosomes are known to remain in host plants in a “selfish” way. The preferential transmission of Gc chromosomes result from the elimination of gametes that lack them, while the gametes with the Gc chromosomes remain fertile (Nasuda et al., 1998). Gc factors cause extensive chromosome breakage, which results in the induction of non-functional gametes and exclusive transmission of the Gc chromosome to the offspring (Nasuda et al., 1998). Gcs have been reported in A. geniculata, A. triuncialis, A. caudata, A. cylindrica, A. longissima, A. sharonensis, and A. speltoides. Their activity is mostly confined to 2, 3, and 4 homeologous groups of C, S, S1, Ssh, and Mg genomes. It has been reported that Gc genes can be constructively utilized for development of addition, deletion and translocation stocks in wheat (Endo, 2007) and triticale (Kwiatek et al., 2017b).
Monosomic alien addition and substitution lines (MAALs and MASLs) are widely used as linking bridges for the transfer of desirable genes from wild species into elite cultivars. For example, wheat-rye addition, substitution, and translocation lines are used as pre-breeding materials for the improvement of wheat (Lukaszewski, 2000, 2016) and triticale (Lukaszewski, 2006). MASLs can be used to identify favorable genes in wild species, allowing for more accurate and rapid transfer of such genes to create introgression lines. Such initiatives provide the opportunity to examine the effect of specific alien (Edet et al., 2018) and to construct the physical maps of specific chromosomes (Kynast et al., 2001). MASLs are produced by crossing monosomic or nullisomic acceptors with appropriate addition lines (Sears, 1972). The missing chromosome of acceptor plant is substituted by the donor homoeologue. The production of substitution lines for alien chromosomes is the initial step for direct introgression of specific alien chromosome segments into the acceptor genome (Lukaszewski, 2000, 2017). One of the most common procedures that reduce the amount of alien chromosome present is the induction of crop-alien Robertsonian translocations (RobTs). This approach requires a set of specific genetic stock plants, including MAALs or MASLs as a donor, and monosomic plants with a single acceptor chromosome (Kwiatek et al., 2017b).
The main aim of this study was to generate the monosomic addition and substitution line of triticale with introgression of alien 2Sk chromosome derived from A. kotschyi, which could be used for efficient study and transfer of genes responsible for leaf and stripe rust resistance.
Materials and Methods
Plant Material
An artificial amphidiploid line of Aegilops kotschyi × Secale cereale (2n = 6x = 42; UkUkSkSkRR) (Figure 1) was obtained by Wojciechowska and Pudelska (2002) from a cross between A. kotschyi Boiss. (no. 14,808; kindly provided from Prof. Taihachi Kawahara, Kyoto University, Kyoto, Japan; Figures 2a,b), and diploid rye “Dankowskie Złote” (Danko Hodowla Roślin sp. z o.o.). Triticale “Sekundo” (Danko Hodowla Roślin Sp. z o.o.) was used as a pollinator for F1 seed production and five subsequent backcrosses (Figure 3). Nullisomic (N2R) line of triticale were developed by Kwiatek et al. (2016c) and used for crossing with monosomic alien addition of 2Sk chromosome to produce monosomic 2Sk(2R) substitution plants of triticale (Figure 3). Cross-hybridization was made in greenhouse of the Institute of Plant Genetics of the Polish Academy of Sciences. Anthers of maternal plants were emasculated and spikes isolated using paper bags, in order to avoid uncontrolled pollination. Mature stigmas were pollinated with the pollen of triticale “Sekundo.” Crossing efficiency percentage was calculated by dividing of the total amount of seeds with total number of pollinated flowers.
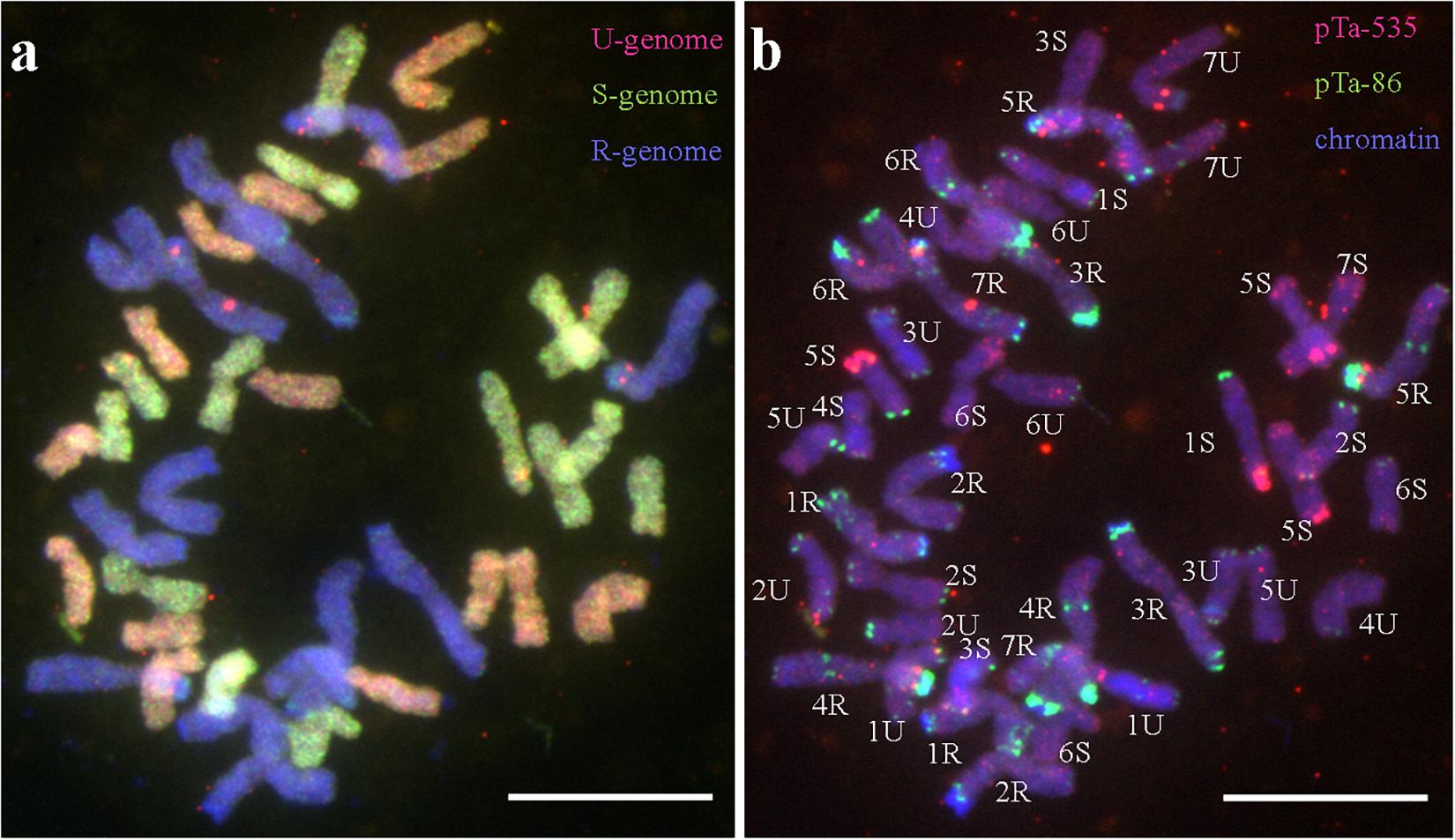
Figure 1. Flourescence/genomic in situ hybridization performed on mitotic chromosomes of A. kotschyi × S. cereale amphiploid. (a) Uk- and Sk-genome chromosomes are labeled with Atto-550 (red) and Atto-488 (green), respectively. R-genome chromosomes labeled with DAPI (blue). (b) Probes pTa-535 and pTa-86 are labeled with Atto-550 (red) and Atto-488 (green), respectively. Chromosomes counterstained with DAPI (blue). Scale bar, 10 μm.
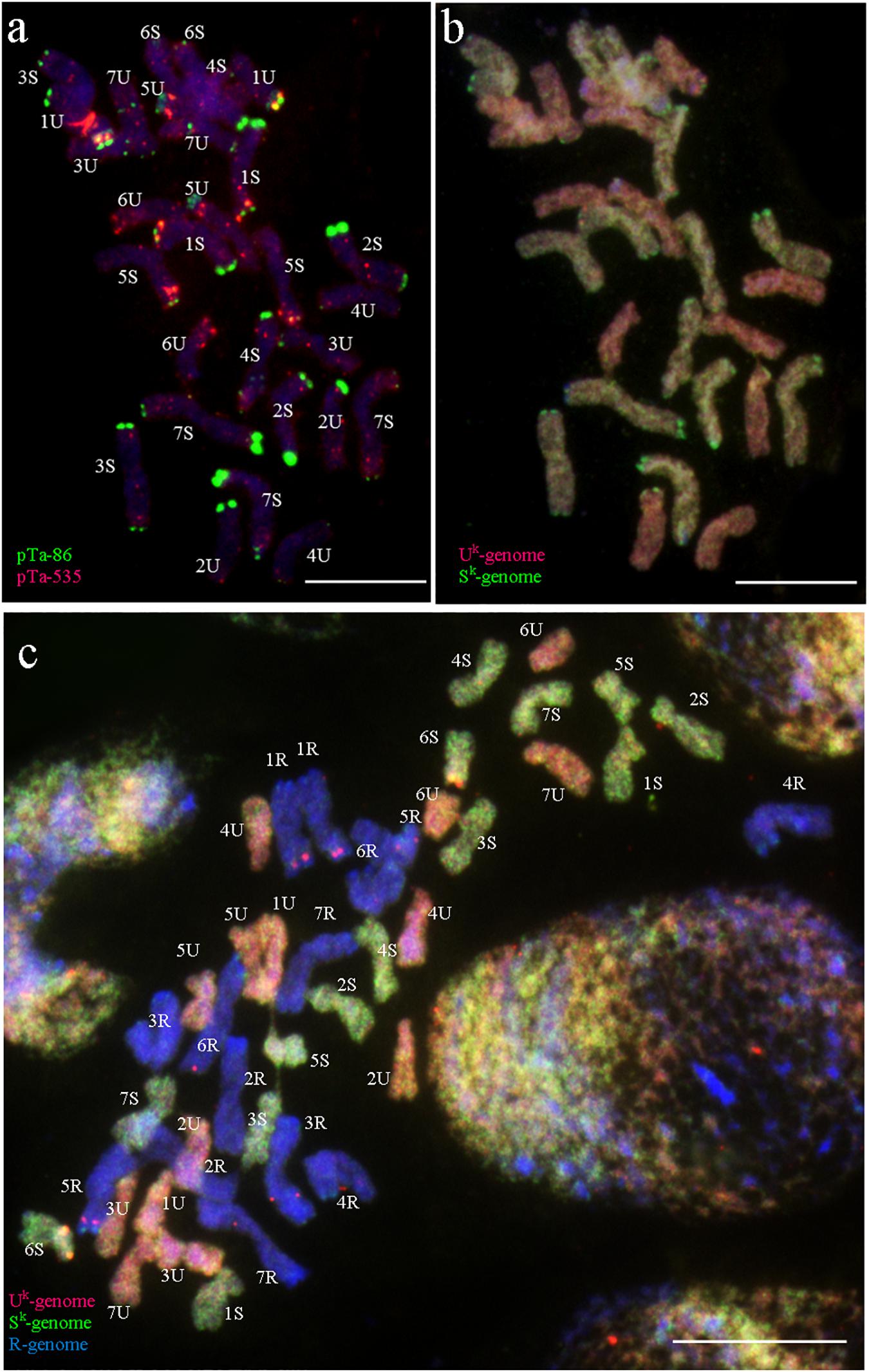
Figure 2. Fluorescence/genomic in situ hybridization performed on mitotic chromosomes of A. kotschyi Boiss (2n = 6x = 42; UkUkSkSk; no. 14808 using (a) pTa-86 (Atto-488, green), pTa-535 (Atto-550, red). Genomic in situ hybridization using U-genome (Atto-550, red) and S-genome (Atto-488, green) probes performed on mitotic chromosomes of (b) A. kotschyi and (c) Aegilops kotschyi × Secale cereale (2n = 6x = 42; UUMMRR). (a,c) Chromosomes counterstained with DAPI (blue). Scale bar, 10 μm.
Cytogenetic Studies
Accumulation of cells at mitotic metaphase and fixation was carried out according to Kwiatek et al. (2017b). Root meristems were digested at 37°C for 2 h and 40 min in an enzymes solution containing 0.2% (v/v) Cellulase Onozuka R-10 and Calbiochem cytohelicase (1:1 ratio) and 20% pectinase (Sigma), in 10 mM citrate buffer (pH 4.6). Digested root tips were placed on slides with a drop of ice-cold 60% acetic acid and squashed. The coverslips were removed with a razor after liquid nitrogen treatment.
Probe Preparation and Fluorescence in situ Hybridization
Total genomic DNA was purified using GeneMATRIX Plant & Fungi DNA Purification Kit (EURx, Gdańsk, Poland). DNA of Aegilops sharonensis Eig (a progenitor of the Sk-genome of A. kotschyi; PI 551020, U.S. National Plant Germplasm System) and A. umbellulata (a progenitor of the Uk-genome of A. kotschyi; PI 298905, U.S. National Plant Germplasm System) were labeled by nick translation with Atto-488NT and Atto-550NT kits (Jena Bioscience, Jena, Germany), respectively. Blocking DNA from triticale “Sekundo” was prepared by boiling for 30–45 min (1:50 probe:block ratio). Chromosomes were identified using fluorescence in situ hybridization (FISH) with the repetitive sequences from pTa-86, pTa-535, pTa-103 (centromere specific), and pTa-k374 (homologous to ribosomal DNA sequence 28S) clones described by Komuro et al. (2013). Clones were amplified from genomic DNA of “Chinese Spring” wheat (Kwiatek et al., 2016b; Goriewa-Duba et al., 2018) and labeled using Atto-488NT, Atto-550NT, and Atto-647NT nick translation kits (Jena Bioscience, Germany). FISH/genomic in situ hybridization (GISH) experiments were performed according to Kwiatek et al. (2017b). Slides were examined with the Olympus BX 61 automatic epifluorescence microscope equipped with Olympus XM10 CCD camera. Image processing was performed using Olympus Cell-F (version 3.1; Olympus Soft Imaging Solutions GmbH: Münster, Germany). Chromosomes of Aegilops and triticale were identified by comparing the signal patterns of the probes (Badaeva et al., 2004; Komuro et al., 2013; Kwiatek et al., 2013; Ruban and Badaeva, 2018).
SSR Marker Analysis
The SSR marker S14-410 (forward primer: 5′ -ACCAATT CAACTTGCCAAGAG-3′; reverse primer: 5′-GAGTAACATG CAGAAAACGACA-3; Smit, 2013) closely linked to the Lr54 + Yr37 loci on the 2Sk chromosome was used to genotype genomic DNA of the plant materials. Genomic DNA of Aegilops kotschyi, triticale “Sekundo” and the hybrid plants were isolated using Plant DNA Purification Kit (EurX Ltd., Gdañsk, Poland). PCR reactions were performed in a LabCycler thermal cycler (SensoQuest Biomedizinische Elektronik, Goettingen, Germany). The PCR reaction consisted of 150 nM each primer (Merck KGaA, Darmstadt, Germany), 0.2 mM of each nucleotide, 1.5 mM MgCl2, 0.2 units of Taq-DNA hot-start polymerase (TaqNovaHS, Blirt, Gdańsk, Poland), and 50 ng of genomic DNA as a template. The PCR conditions were as follows: 5 min at 95°C, then 35 cycles of 30 s at 94°C, 30 s at 61°C, 1 min at 72°C, and 5 min at 72°C. Midori Green Direct (Nippon Genetics Europe) was added to each amplification product and analyzed by gel electrophoresis on 2% agarose gel (LabEmpire, Poznań, Poland). Gels were visualized and photographed using EZ GelDoc System (BioRad, Hercules, CA, United States).
Evaluation of Leaf Rust Symptoms in Growth Chamber
Evaluation of the response of monosomic substitution plants on leaf rust infection was conducted in the growth chamber (at IPG PAS) using leaf and stripe rust urediniospores, which were collected separately from triticale fields in three localizations in Wielkopolska region: IPG PAS Experimental Station in Cerekwica, Poland (52° 31° 41°41°04° 25° 54 Figure 4). Both experiments were repeated. Each experiment repeat included forty monosomic 2Sk(2R) substitution plants and forty control plants (“Sekundo”), which were sprayed with leaf rust urediniospore solution containing 0.1% Tween 20, at three-leaf stage. Another 160 plants were sprayed with stripe rust urediniospore solution. The inoculated plants were then incubated in a humid growth chamber free from light for 10 days. After inoculation, the plants were maintained under a day/night photoperiod of 18/6 h, a temperature of 16–22°C. Winter triticale cv. “Sekundo” was taken as the susceptible control. The infection type of each individual was scored at three timepoints [10, 15, and 20 days post-inoculation (dpi)] using an infection scale adapted from Roelfs (1988) and transformed into nine-grade scale (1, high resistance; 9, susceptibility, Tables 2, 3; McNeal et al., 1971). The phenotypic data were analyzed using analysis of variance (ANOVA) and Tukey’s highest significant difference (HSD) test.
Results
Production of Monosomic 2Sk Addition Plants From Triticale-A. kotschyi Hybrids
Seven F1 plants were produced from a cross between hexaploid triticale “Sekundo” (2n = 6x = 42 chromosomes; AABBRR) and hexaploid A. kotschyi × S. cereale (2n = 6x = 42; UUSSRR; Figure 2c) hybrid, as a pollen donor. All seven F1 plants possessed 42 chromosomes including a complete haploid set of chromosomes of A. kotschyi (Figure 5a and Table 1). The number of R-genome chromosomes was 14. Fifteen BC1F1 seeds were obtained from 375 flowers of F1 plants, which were backcrossed using triticale pollen. FISH experiments revealed that chromosomes 1Uk, 2Uk, and 7Sk were eliminated (Figure 5b and Table 1). Fiftyone BC2F1 seeds were obtained by subsequent backcrossing of BC1F1 with triticale “Sekundo.” Fifty BC2F1 plants were karyotyped (Table 1), with the progressive elimination of Aegilops chromosomes was observed. The number of U-genome chromosomes varied between 1 and 2. The number of Sk-genome chromosomes was much more diversified and varied between 2 and 6 (Figure 5c and Table 1). However, two chromosomes (2Sk and 4Sk) were present in all 50 BC2F1 plants (Table 1). The BC2F1 plants were pollinated with triticale “Sekundo” pollen, and a total number of 234 seeds were obtained. FISH was carried out on 100 BC3F1, revealing that chromosome 7Uk was present in all plants. Chromosome number of Sk-genome varied between 2 and 3 (Figure 5d). As before, chromosomes 2Sk and 4Sk were present in all 100 BC3F1 plants (Table 1). Fifty randomly selected plants of 93 BC4F1 hybrids were karyotyped using FISH and GISH techniques. Chromosomes of Uk-genome were completely eliminated, but surprisingly chromosomes 2Sk and 4Sk were present in all 50 BC4F1 plants (Figure 5e and Table 1). The last backcross resulted in formation of 216 BC5F1 seeds (Table 1). One hundred plants were karyotyped and 99 of them carried additional 2Sk chromosomes (2n = 43) (Figure 5f and Table 1). Chromosome 2Sk was not present in one BC5F1 plant (2n = 42) (Table 1), and this plant showed centric breaks in three pairs chromosomes (Figure 6). Therefore, an investigation of six generations of triticale Sekundo × (A. kotschyi × S. cereale) hybrids (F1 to BC5F1) by means of molecular cytogenetic methods (FISH and GISH) revealed subsequent elimination of Aegilops chromosomes.
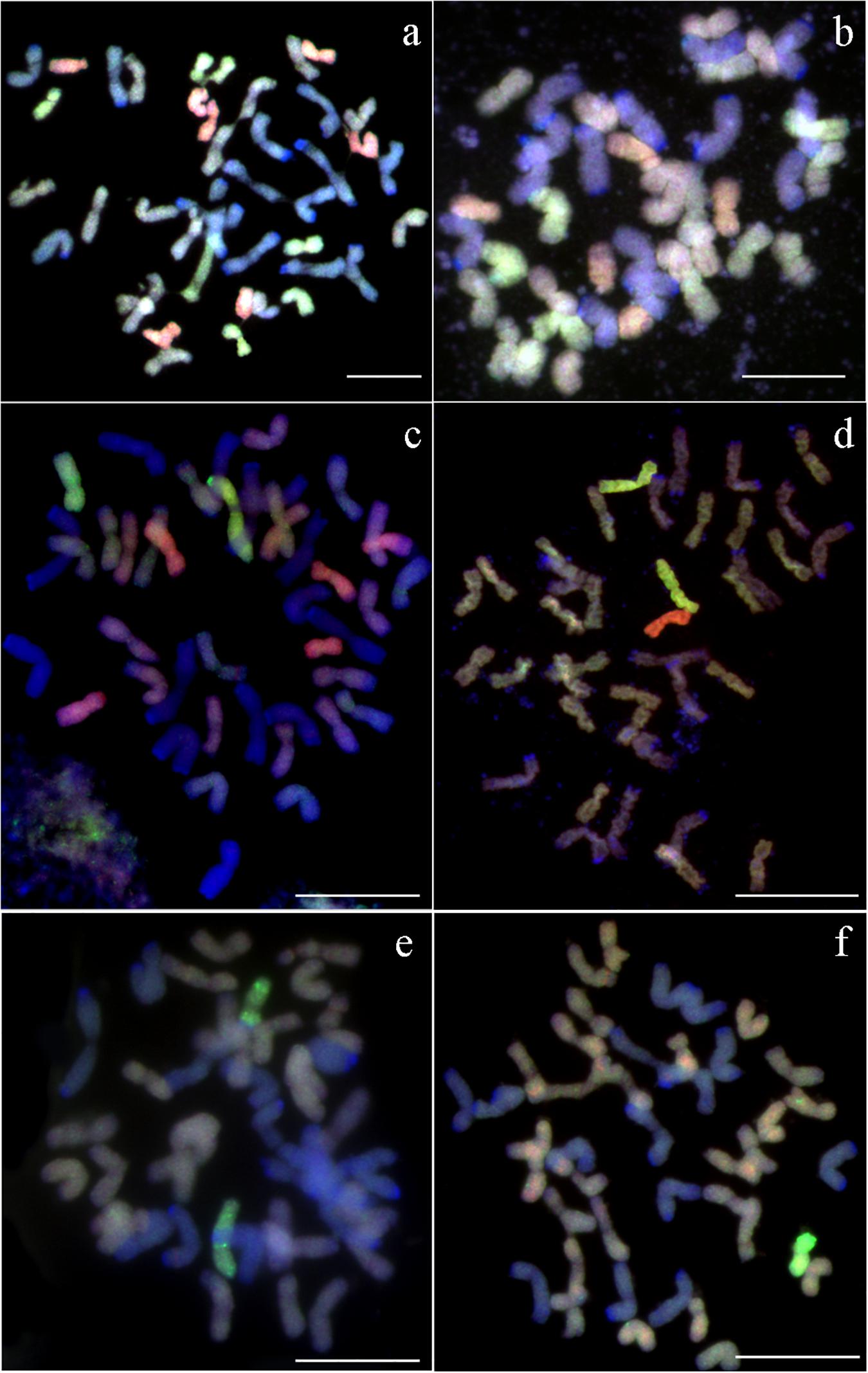
Figure 5. Genomic in situ hybridization performed on mitotic chromosomes of (a) F1, (b) BC1F1, (c) BC2F1, (d) BC3F1, (e) BC4F1, and (f) BC5F1 triticale-Aegilops introgression plants. A- and B-genome chromosomes are labeled with DAPI (light blue), as well as R-genome chromosomes (dark blue). Uk- and Sk-genome chromosomes are labeled with Atto-550 (red) and Atto-488 (green), respectively. Scale bar, 10 μm.
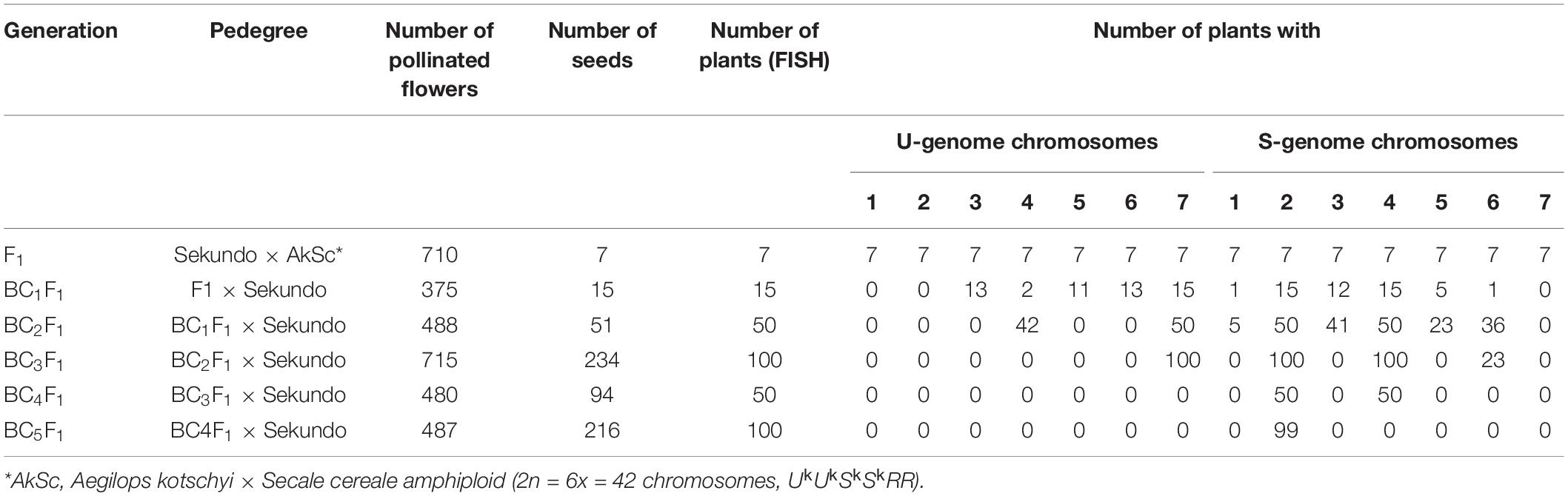
Table 1. Frequencies of individual Aegilops kotschyi chromosomes in five subsequent generations of backcrossing with triticale.
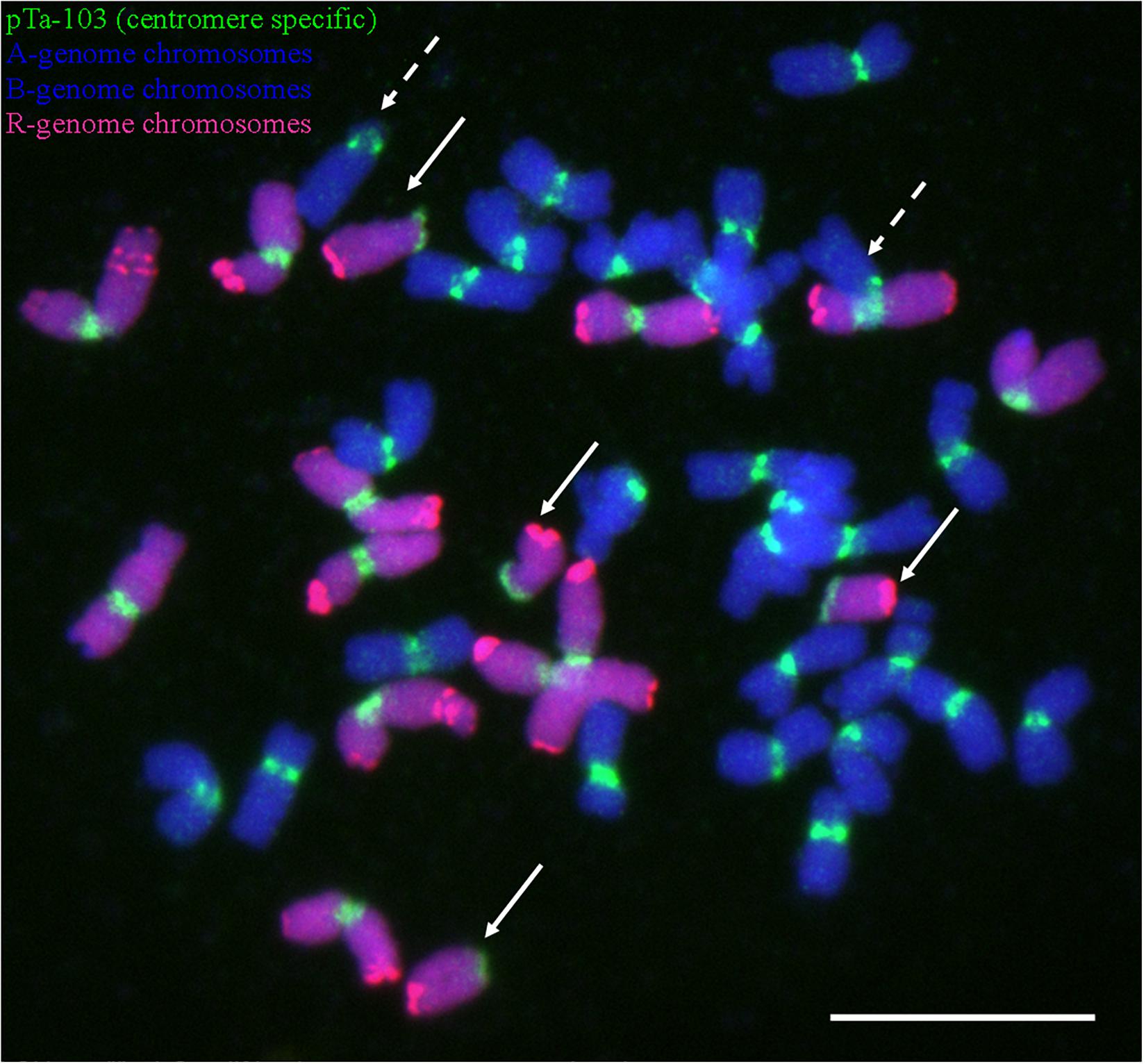
Figure 6. Centric breaks of chromosomes in BC5F1 plant screened using multi-color GISH/FISH. Centromere regions were mapped using pTa-103 (Atto-488, green) probe. Dotted arrows, chromosome aberrations of A- or B-genome (DAPI, blue) of triticale; solid arrows, chromosome aberrations of R-genome (Atto-550, red) of triticale. Scale bar, 10 μm.
Production of Monosomic 2Sk(2R) Substitution Plants From Triticale-A. kotschyi Hybrids
A total number of 468 seeds were obtained from a cross between nullisomic N2R triticale plants (2n = 40; Figures 7a,b) and monosomic 2Sk addition line (MA2SkAL) of triticale (Figures 7c,d), as a pollen donor. 6,486 flowers of N2R plants were emasculated and pollinated with MA2Sk pollen. The crossing efficiency was 7.22%, and 468 plants were obtained. The FISH/GISH analyses revealed that each offspring plant carried 42 chromosomes, including 2R and 2Sk chromosomes, which were in monosomic condition (Figure 7e,f). The presence of monosomic or nullisomic 2R chromosome was not observed.
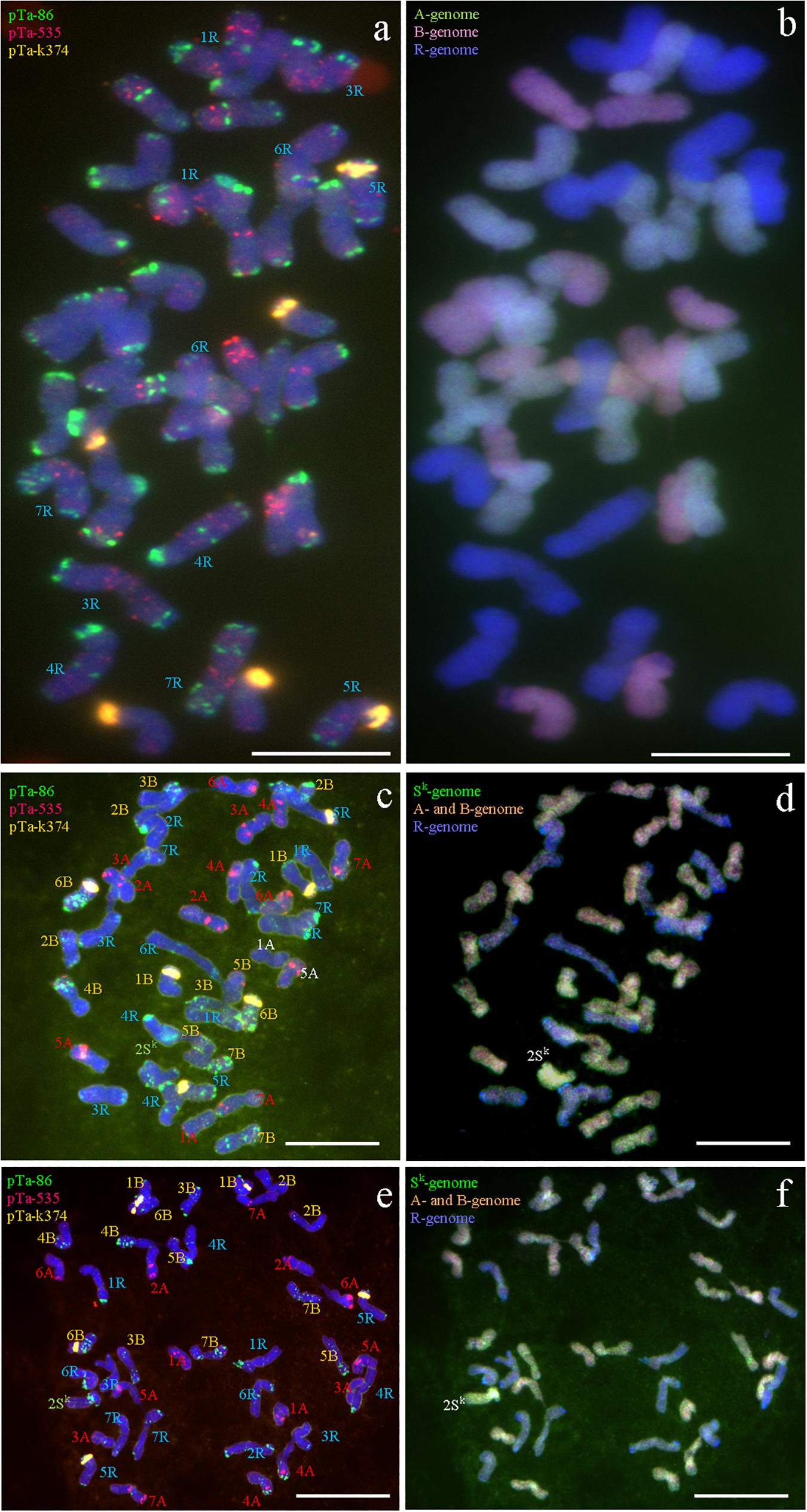
Figure 7. Fluorescence and genomic in situ hybridization performed on mitotic chromosomes of: (a,b) nullisomic 2R plant of triticale (2n = 40); (c,d) monosomic 2Sk addition plant of triticale (2n = 43); (e,f) monosomic 2Sk(2R) substitution plant of triticale (2n = 42). pTa-86 (Atto-488; green), pTa-535 (Atto-550; red), and pTa-k374 (Atto-647; yellow) probes were used for FISH. Genomic probes of A-, B-, R-, and Sk-genome were used for GISH. Scale bar, 10 μm.
Lr54 + Yr37 SSR Markers Analysis
PCR with SSR marker S14-410, linked to Lr54 + Yr37 loci, was conducted on A. kotschyi, triticale “Sekundo,” the monosomic substitution M2Sk (M2R) line and nullisomic N2R triticale plants (2n = 40). The same protocol was used to examine the offspring derived by subsequent backcrossing. A 410-bp amplicon for the S14-410 marker was identified in the control sample of A. kotschyi and in all hybrid plants of F1 to BC4F1 generations (Figure 8). Screening for the presence of the S14-410 marker in 100 BC5F1 plants showed that 410 bp amplicon was present in 99 plants. Similar screening was carried out in 468 plants, created by cross-hybridization of N2R triticale plants (2n = 40) and M2SkAL plants (2n = 43). PCR reaction with the genomic DNA of all recombinant plants yielded a 410-bp fragment.
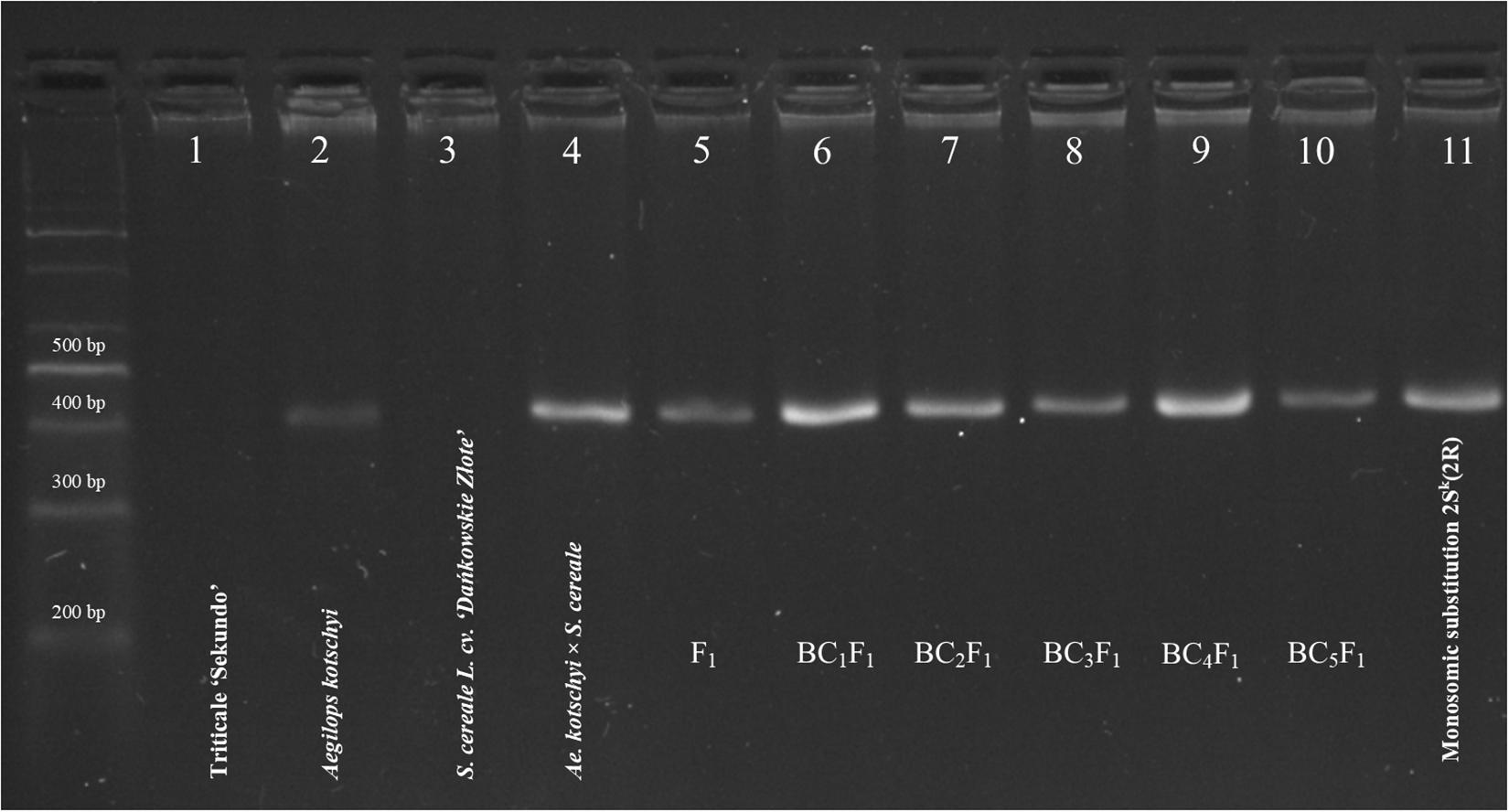
Figure 8. Amplification products (410 bp) of S14-410 marker linked to loci of Lr54 + Lr37 leaf and stem rust resistance genes on 2Sk chromosome of Ae. kotschyi. (1) triticale “Sekundo” (AABBRR); (2) Aegilops kotschyi (UkUkSkSk); (3) Secale cereale L. “Dañkowskie Złote”; (4) Aegilops kotschyi × Secale cereale (2n = 6x = 42; UUMMRR); (5) F1; (6) BC1F1; (7) BC2F1; (8) BC3F1; (9) BC4F1; (10) BC5F1; and (11) monosomic 2Sk(2R) substitution plant of triticale (2n = 42). Size standard: Thermo ScientificTM GeneRulerTM 100 bp DNA Ladder.
Evaluation of Leaf Rust Symptoms
The leaf and stripe rust response of two sets of 80 plants of triticale monosomic 2Sk(2R) substitution line was evaluated at the seedling stage in the growth chamber and compared with phenotypes of acceptor cultivar “Sekundo” as controls (Table 2). The mean scores for two replications of control plants (6.40 ± 0.73 and 6.43 ± 0.75) showed that the inoculation solution was effective for induction of the leaf rust infection. The mean score of three independent evaluations of infection level (10, 15, and 20 dpi) of two replications of monosomic 2Sk(2R) substitution plants was similar (2.96 ± 0.42 and 2.97 ± 0.48) (Table 2). The differences were significant at α = 0.01 (Supplementary Table 1). The scores for two replications of the leaf rust response experiment did not differ significantly (Supplementary Table 1). In contrast, the stripe rust responses of the monosomic 2Sk(2R) substitution plants and the control triticale cv. “Sekundo” at the three successive points did not differ significantly (α = 0.01) (Table 3). Plants of cv. “Sekundo” showed moderate stripe rust resistance (3.24 ± 0.79 and 3.33 ± 0.85), as well as monosomic substitution plants (3.21 ± 0.79 and 3.29 ± 0.77). ANOVA test for all thee timepoints showed that there is no statistically significant differences between stripe rust response of monosomic substitution plants compared with triticale “Sekundo” controls (Supplementary Table 2).
Discussion
Alien and cultivated species are closely related and possess homeologous genomes (or sub-genomes). Hence, it is possible to introduce the desirable variation into crop plants using a backcrossing program. However, isolation barriers exist that prevent the formation of interspecific hybridization, and present a significant obstacle for the exploration of alien genetic variation in crop improvement. The generation of artificial amphidiploids can facilitate the transmission of desirable genetic material from wild species to crops. In this study, synthetic A. kotschyi × S. cereale (2n = 6x = 42, UkUkSkSkRR) amdiphiploid plants were used as a donor of wild genetic material to widen the genetic variation in hexaploid triticale “Sekundo” (2n = 6x = 42, AABBRR).
Similar artificial amphidiploid forms were used to transfer Lr32 and Lr39 leaf rust resistance genes from A. tauschii × S. cereale (2n = 4x = 28; DDRR) (Kwiatek et al., 2015) and Pm13 powdery mildew resistance gene from A. variabilis × S. cereale (2n = 6x = 42, UvUvSvSvRR) (Kwiatek et al., 2016a) into cultivated triticale. It was reported that the presence of an R-genome chromosome set resulted in semi-fertile F1 plants that were capable of producing an F1 via cross-hybridization of artificial Aegilops biuncialis × S. cereale (2n = 6x = 42, UbUbMbMbRR) amphiploid and hexaploid triticale (Kwiatek et al., 2017a). In this study, seven F1 plants were obtained via cross-hybridization of A. kotschyi × S. cereale and triticale. The first backcross with triticale “Sekundo” resulted in rapid elimination of the Aegilops chromosomes. The elimination range of the Uk-genome chromosomes was far higher when compared with the rate of Sk-genome chromosome loss (Table 1). The elimination of alien chromosomes was also observed in the BC2F1 generation. Interestingly, only 7Uk, 4Uk, 2Sk, and 4Sk chromosomes were present in all of BC2F1 plants. In subsequent generations of triticale hybrids, obtained via successive backcrosses, the number of Aegilops chromosomes was highly reduced and the plants of BC4F1 carried 2Sk and 4Sk chromosomes only.
This pattern could be a result of gametocidal action of the Gc locus on 2Ssh of Aegilops sharonensis (Endo, 1985). Moreover, similar “breaker” element described in A. sharonensis (Maan, 1975). These loci have been mapped to a region proximal to a block of sub-telomeric heterochromatin on chromosome 4SshL (Knight et al., 2015). The diploid A. sharonensis (2n = 2x = 14; SshSsh) is a direct ancestor and a donor of Sk-genome of A. kotschyi (Kihara, 1954; Badaeva et al., 2004; Ruban and Badaeva, 2018). Investigations into the origin of A. kotschyi showed that the Uk and Sk subgenomes are very similar to the diploid progenitors: A. umbellulata (2n = 2x = 14; UU) and A. sharonensis, respectively (Zohary and Feldman, 1962; Zohary, 1999; Ozkan et al., 2001; Feldman and Levy, 2012). It is a distinct possibility that there are gametocidal loci on 2Sk and 4Sk chromosomes of A. kotschyi, and those loci could be orthologous to A. sharonensis analogs.
In the presented study, 99% of BC5F1 monosomic alien addition plants of triticale carried an additional 2Sk chromosome. Endo (2007) suggested, that when the gametocidal action is intense, gametes without the alien chromosome may suffer severe chromosome damages, resulting in sterility. In this study, one BC5F1 plant without 2Sk chromosomes suffered minor chromosome aberrations (Figure 3). It could be hypothesized that gametes without 2Sk can be fertile and develop into plants with chromosome aberrations as no chromosome fragment has been lost. This genetic phenomenon is of particular interest to breeders. Linking Gc loci with Lr54 + Yr37 leaf and stripe rust-resistant genes in triticale varieties would ensure maintenance of these traits in subsequent generations without the need for selection. But, on the other hand, triticale plants with centric chromosome breaks can be selected and used for the induction of Robertsonian translocations (RobTs) (Kwiatek and Nawracała, 2018).
The molecular analyses with the S14-410 marker confirmed that the preferential transmission of the 2Sk chromosome occurred in all hybrid plants, from the F1 generation to BC4F1. The Lr54 + Yr17 marker identification reflected the results of cytogenetic analysis of BC5F1 plants. The key result of this study was the development of monosomic 2Sk(2R) substitution lines, which can be used for induction of chromosome translocations. There are several reports describing the methodology used for the production of monosomic substitutions. According to Faris et al. (2002), this can be achieved by crossing an alien chromosome addition line containing the gene of interest with a line that is monosomic for a homoeologous wheat chromosome. This approach was applied for development of 1RS.1DL chromosome translocation in triticale “Rhino” (Lukaszewski and Curtis, 2006). The 1RS.1DL translocation was later used for the induction of multi-breakpoint translocation lines (Lukaszewski, 2006, 2017).
It is reported that triticale is infected by the races specific to both: wheat and rye; however, it was noticed that triticale is more easily attacked by the wheat physiological forms of the rusts than by the rye ones (Arseniuk, 1996). After inoculation, a significant improvement of the resistance level was observed in introgressed plants in comparison with triticale cv. Sekundo plants. The mean level of leaf rust resistance was high for two independent replications of the experiment (2.96 ± 0.42 and 2.97 ± 0.48). Such low infection rate can be considered as a result of Lr54 gene expression. In a similar study, Marais et al. (2005) developed a 2DS.2SkL wheat-A. kotschyi line (called S14 translocation). The authors reported 96% resistant plants (72 tested) which were tested for resistance to eight Pt pathotypes (UVPrt2, UVPrt3, UVPrt4, UVPrt5, UVPrt8, UVPrt9, UVPrt10 and UVPrt13) and two Pst pathotypes (6E16A- and 6E22A-) endemic to South Africa. Moreover, it was shown that the S14 translocation evidently had preferential transmission (Marais et al., 2005). Intriguingly, monosomic 2Sk(2R) substitution triticale plants, as well as control plants revealed the same, moderate resistance against stripe rust. In general, it is reported that triticale is more resistant against stripe rust as wheat. Moreover, triticale infection by P. stiiformis is highly dependent on plant growth stage and pathogen race (Rodriguez-Algaba et al., 2020). Hence, it could be possible that triticale “Sekundo” possesses a partial resistance against stripe rust and Yr37 did not increase it.
In this study, the monosomic 2Sk(2R) substitution plants were obtained through crossing between monosomic 2Sk addition line of triticale (M2SkAL), containing Lr54 loci, with another triticale line that was nullisomic for 2R chromosome pair (N2R). The cross-hybridization between M2SkAL and N2R plants resulted in no offspring with the 2R chromosome in a monosomic condition (M2R). Hence, it can be hypothesized that gametocidal action of 2Sk chromosome killed gametes lacking it. Moreover, it could be possible that the inhibiting or suppressing factor of the 2Sk gametocidal action in triticale is located on the 2R chromosome. It is already known that some cultivars of common wheat possess genes that suppress the function of the Gc factors. Tsujimoto and Tsunewaki (1985) reported that that a suppressor gene (Igc1), that controls the suppression of Gc gene action on chromosome 3C of A. truncialis, is located on 3B chromosome of wheat “Norin 26.” Similarly, Endo (1988) postulated that gametocidal action of the 4S chromosome of A. sharonensis or A. longissima is suppressed by the presence of 4B chromosome of wheat. It could be plausible that both the Gc gene and the suppressor loci are located on chromosomes of the same homoeologous group; however, these hypotheses require future investigation.
The classical methods basing on backcrossing and selection are still significant in plant breeding. There are several countries, which are considered as key crop producers (for example, France, Germany, Poland, etc.), place a strict set of regulations on the cultivation of genetically modified (GM) crops. Hence, classical breeding methods, enriched by the molecular (and cyto-molecular)-assisted selection and chromosome manipulation are still worthy endeavors. In addition, the genetic mechanisms that regulate the process of segregation distortion should be seriously taken into consideration as a natural tool for chromosome manipulation and engineering.
Data Availability Statement
The raw data supporting the conclusions of this article will be made available by the authors, without undue reservation, to any qualified researcher.
Author Contributions
MK: conceptualization, methodology, formal analysis, writing original draft preparation, project administration, and funding acquisition. MK, WU, JB, and HW: validation. MK, JB, WU, RS, and AN: investigation. MK and HW: resources and supervision. MK and WU: data curation. MK and DP: writing—review and editing. All authors contributed to the article and approved the submitted version.
Funding
This research and the APC were funded by the National Centre for Research and Development, Poland (Narodowe Centrum Badań i Rozwoju, Polska), grant number LIDER/3/0004/L-8/16/NCBR/2017.
Conflict of Interest
The authors declare that the research was conducted in the absence of any commercial or financial relationships that could be construed as a potential conflict of interest.
Acknowledgments
We would like to acknowledge and thank Dr. Zofia Banaszak at the Danko Breeding Station, for providing seeds of triticale cultivars. In addition, we would like to thank all of the reviewers and manuscript editor for their careful review of the manuscript and for their excellent suggestions for improving our initial work.
Supplementary Material
The Supplementary Material for this article can be found online at: https://www.frontiersin.org/articles/10.3389/fpls.2020.509481/full#supplementary-material
References
Arseniuk, E. (1996). “Triticale diseases - a review,” in Triticale: today and tomorrow, eds V. P. Carnide, H. Guedes-Pinto, and N. Darvey (Netherlands: Springer), 499–525. doi: 10.1007/978-94-009-0329-6_65
Badaeva, E. D., Amosova, A. V., Samatadze, T. E., Zoshchuk, S. A., Shostak, N. G., Chikida, N. N., et al. (2004). Genome differentiation in Aegilops. 4. Evolution of the U-genome cluster. Plant Syst. Evol. 246, 45–76. doi: 10.1007/s00606-003-0072-4
Brar, D. S., and Dhaliwal, H. S. (2004). ““Chromosome manipulations for crop improvement.”,” in Plant Breeding: Mendelian to Molecular Approaches, eds H. K. Jain and M. C. Kharkwal (Dordrecht: Springer Netherlands), 65–96. doi: 10.1007/978-94-007-1040-5_4
Edet, O. U., Kim, J.-S., Okamoto, M., Hanada, K., Takeda, T., Kishii, M., et al. (2018). Efficient anchoring of alien chromosome segments introgressed into bread wheat by new Leymus racemosus genome-based markers. BMC Genet. 19:18. doi: 10.1186/s12863-018-0603-1
Endo, T. R. (1985). Two types of gametocidal chromosome of Aegilops sharonensis and Ae. longissima. Jap. J. Genet. 60, 125–135. doi: 10.1266/jjg.60.125
Endo, T. R. (1990). Gc chromosomes and their induction of chromosome mutations in wheat. Jpn. J. Genet. 65, 135–152.
Endo, T. R. (1988). Induction of chromosomal structural changes by a chromosome Aegilops cylindrica L. in common wheat. J. Hered. 79, 366–370. doi: 10.1093/oxfordjournals.jhered.a110529
Endo, T. R. (2007). The gametocidal chromosome as a tool for chromosome manipulation in wheat. Chromosom. Res. 15, 67–75. doi: 10.1007/s10577-006-1100-3
Faris, J. D., Friebe, B., and Gill, B. S. (2002). Wheat Genomics: Exploring the Polyploid Model. Curr. Genomics 3, 577–591. doi: 10.2174/1389202023350219
Feldman, M., and Levy, A. A. (2012). Genome evolution due to allopolyploidization in wheat. Genetics 192, 763–774. doi: 10.1534/genetics.112.146316
Gill, B. S., Friebe, B. R., and White, F. F. (2011). Alien introgressions represent a rich source of genes for crop improvement. PNAS 108, 7657–7658. doi: 10.1073/pnas.1104845108
Goriewa-Duba, K., Duba, A., Kwiatek, M., Wiśniewska, H., Wachowska, U., and Wiwart, M. (2018). Chromosomal distribution of pTa-535, pTa-86, pTa-713, 35S rDNA repetitive sequences in interspecific hexaploid hybrids of common wheat (Triticum aestivum L.) and spelt (Triticum spelta L.). PLoS One 13:192862. doi: 10.1371/journal.pone.0192862
Kihara, H. (1954). Considerations on the evolution and distribution of Aegilops Species based on the analyser-method. Cytologia 19, 336–357. doi: 10.1508/cytologia.19.336
Knight, E., Binnie, A., Draeger, T., Moscou, M., Rey, M.-D., Sucher, J., et al. (2015). Mapping the ‘breaker’ element of the gametocidal locus proximal to a block of sub-telomeric heterochromatin on the long arm of chromosome 4Ssh of Aegilops sharonensis. Theor. Appl. Genet. 128, 1049–1059. doi: 10.1007/s00122-015-2489-x
Komuro, S., Endo, R., Shikata, K., and Kato, A. (2013). Genomic and chromosomal distribution patterns of various repeated DNA sequences in wheat revealed by a fluorescence in situ hybridization procedure. Genome 56, 131–137. doi: 10.1139/gen-2013-0003
Kwiatek, M. T., and Nawracała, J. (2018). Chromosome manipulations for progress of triticale (×Triticosecale) breeding. Plant Breed. 137, 823–831. doi: 10.1111/pbr.12652
Kwiatek, M. T., Majka, J., Majka, M., Belter, J., and Wisniewska, H. (2017a). Adaptation of the pivotal-differential genome pattern for the induction of intergenomic chromosome recombination in hybrids of synthetic amphidiploids within Triticeae tribe. Front. Plant Sci. 8:1300. doi: 10.3389/fpls.2017.01300
Kwiatek, M. T., Wiśniewska, H., Ślusarkiewicz-Jarzina, A., Majka, J., Majka, M., Belter, J., et al. (2017b). Gametocidal factor transferred from Aegilops geniculata Roth can be adapted for large-scale chromosome manipulations in cereals. Front. Plant Sci. 8:409. doi: 10.3389/fpls.2017.00409
Kwiatek, M., Belter, J., Majka, M., and Wiśniewska, H. (2016a). Allocation of the S-genome chromosomes of Aegilops variabilis Eig. carrying powdery mildew resistance in triticale (× Triticosecale Wittmack). Protoplasma 253, 329–343. doi: 10.1007/s00709-015-0813-6
Kwiatek, M., Majka, M., Majka, J., Belter, J., Suchowilska, E., Wachowska, U., et al. (2016b). Intraspecific polymorphisms of cytogenetic markers mapped on chromosomes of Triticum polonicum L. PLoS One 11:158883. doi: 10.1371/journal.pone.0158883
Kwiatek, M., Majka, M., Ślusarkiewicz-Jarzina, A., Ponitka, A., Pudelska, H., Belter, J., et al. (2016c). Transmission of the Aegilops ovata chromosomes carrying gametocidal factors in hexaploid triticale (×Triticosecale Wittm.) hybrids. J. Appl. Genet. 57, 305–315. doi: 10.1007/s13353-015-0332-3
Kwiatek, M., Majka, M., Wiśniewska, H., Apolinarska, B., and Belter, J. (2015). Effective transfer of chromosomes carrying leaf rust resistance genes from Aegilops tauschii Coss. into hexaploid triticale (X Triticosecale Witt.) using Ae. tauschii × Secale cereale amphiploid forms. J. Appl. Genet. 56, 163–168. doi: 10.1007/s13353-014-0264-3
Kwiatek, M., Wiśniewska, H., and Apolinarska, B. (2013). Cytogenetic analysis of Aegilops chromosomes, potentially usable in triticale (X Triticosecale Witt.) breeding. J. Appl. Genet. 54, 147–155. doi: 10.1007/s13353-013-0133-5
Kynast, R. G., Riera-Lizarazu, O., Vales, M. I., Okagaki, R. J., Maquieira, S. B., Chen, G., et al. (2001). A complete set of maize individual chromosome additions to the oat genome. Plant Physiol. 125, 1216–1227. doi: 10.1104/pp.125.3.1216
Lukaszewski, A. J. (2000). Manipulation of the 1RS.1BL translocation in wheat by induced homoeologous recombination. Crop Sci. 40, 216–225. doi: 10.2135/cropsci2000.401216x
Lukaszewski, A. J. (2006). Cytogenetically engineered rye chromosomes 1R to improve bread-making quality of hexaploid triticale. Crop Sci. 46, 2183–2194. doi: 10.2135/cropsci2006.03.0135
Lukaszewski, A. J. (2016). “Manipulation of homologous and homoeologous chromosome recombination in wheat,” in Plant Cytogenetics: Methods and Protocols, eds S. F. Kianian and P. M. A. Kianian (New York, NY: Springer New York), 77–89. doi: 10.1007/978-1-4939-3622-9_7
Lukaszewski, A. J. (2017). A set of new 1RS translocations from wheat cv. Amigo in a uniform genetic background. Euphytica 213:214. doi: 10.1007/s10681-017-2008-z
Lukaszewski, A., and Curtis, C. (2006). Transfer of the Glu−D1 gene from chromosome 1D of bread wheat to chromosome 1R in hexaploid triticale. Plant Breed. 109, 203–210. doi: 10.1111/j.1439-0523.1992.tb00174.x
Maan, S. S. (1975). Exclusive preferential transmission of an alien chromosome in common wheat 1. Crop Sci. 15, 287–292. doi: 10.2135/cropsci1975.0011183X001500030002x
Marais, G. F., McCallum, B., and Marais, A. S. (2005). Leaf rust and stripe rust resistance genes Lr54 and Yr37 transferred to wheat from Aegilops kotschyi. Plant Breed. 124, 538–541. doi: 10.1007/s10681-006-9092-9
McNeal, F. H., Koznak, C. F., Smith, E. P., Tate, W. S., and Russell, T. S. (1971). A uniform system for recording and processing cereal research data. USDA-ARS Bull. 42, 34–121.
Nasuda, S., Friebe, B., and Gill, B. S. (1998). Gametocidal genes induce chromosome breakage in the interphase prior to the first mitotic cell division of the male gametophyte in wheat. Genetics 149, 1115–1124.
Ozkan, H., Levy, A. A., and Feldman, M. (2001). Allopolyploidy-induced rapid genome evolution in the wheat (Aegilops – Triticum) Group. Plant Cell 13, 1735–1747. doi: 10.1105/TPC.010082
Prażak, R., and Paczos-Grzęda, E. (2013). Characterization of Aegilops kotschyi Boiss. x Triticum aestivum L. hybrid lines. Acta Agrobot. 66, 109–120. doi: 10.5586/aa.2013.057
Qi, L., Friebe, B., Zhang, P., and Gill, B. S. (2007). Homoeologous recombination, chromosome engineering and crop improvement. Chromosom. Res. 15, 3–19. doi: 10.1007/s10577-006-1108-8
Rawat, N., Tiwari, V. K., Singh, N., Randhawa, G. S., Singh, K., Chhuneja, P., et al. (2009). Evaluation and utilization of Aegilops and wild Triticum species for enhancing iron and zinc content in wheat. Genet. Resour. Crop Evol. 56, 53–64. doi: 10.1007/s10722-008-9344-8
Riley, R., and Chapman, V. (1958). Genetic control of the cytologically diploid behaviour of hexaploid wheat. Nature 182, 713–715. doi: 10.1038/182713a0
Rodriguez-Algaba, J., Sørensen, C. K., Labouriau, R., Justesen, A. F., and Hovmøller, M. S. (2020). Susceptibility of winter wheat and triticale to yellow rust influenced by complex interactions between vernalisation, temperature, plant growth stage and pathogen race. Agronomy 10:13. doi: 10.3390/agronomy10010013
Roelfs, A. P. (1988). An international system of nomenclature for Puccinia graminis f. sp. tritici. Phytopathology 78, 526–533. doi: 10.1094/phyto-78-526
Ruban, A. S., and Badaeva, E. D. (2018). Evolution of the S-Genomes in Triticum-Aegilops alliance: evidences from chromosome analysis. Front. Plant Sci. 9:1756. doi: 10.3389/fpls.2018.01756
Schneider, A., Molnár, I., and Molnár-Láng, M. (2008). Utilisation of Aegilops (goatgrass) species to widen the genetic diversity of cultivated wheat. Euphytica 163, 1–19. doi: 10.1007/s10681-007-9624-y
Sears, E. R. (1972). “Chromosome engineering in wheat,” in Stadler Genet. Symp, eds G. Kimber and G. P. Redei (Columbia: University of Missouri), 23–38.
Sears, E. R. (1977). An induced mutant with homoeologous pairing in wheat. Can. J. Genet. Cytol. 19, 585–593. doi: 10.1139/g77-063
Singh, B. D., and Singh, A. K. (2015). Marker-assisted plant breeding: principles and practices. India: Springer. doi: 10.1007/978-81-322-2316-0
Smit, C. (2013). Pyramiding of novel rust resistance genes in wheat, utilizing marker assisted selection and doubled haploid technology. Master of Science Thesis, Stellenbosch: Stellenbosch University, 1–123.
Tsujimoto, H., and Tsunewaki, K. (1985). Gametocidal genes in wheat and its relatives. II. Suppressor of the chromosome 3C gametocidal gene of Aegilops triuncialis. Can. J. Genet. Cytol. 27, 178–185. doi: 10.1139/g85-027
Veatch-Blohm, M. E. (2007). Principles of plant genetics and breeding. Crop Sci. 47:1763. doi: 10.2135/cropsci2007.05.0002br
Wojciechowska, B., and Pudelska, H. (2002). Production and morphology of the hybrids Aegilops kotschyi × Secale cereale and Ae. biuncialis × S. cereale. Genet. Plant Acad. 43, 279–285.
Wulff, B. B. H., and Moscou, M. J. (2014). Strategies for transferring resistance into wheat: from wide crosses to GM cassettes. Front. Plant Sci. 5:692. doi: 10.3389/fpls.2014.00692
Zohary, D. (1999). Monophyletic vs. polyphyletic origin of the crops on which agriculture was founded in the Near East. Genet. Resour. Crop Evol. 46, 133–142. doi: 10.1023/A:1008692912820
Keywords: back-cross, fluorescence in situ hybridization, molecular markers, monosomic, resistance genes, triticale 2, Aegilops kotschyi
Citation: Kwiatek MT, Ulaszewski W, Belter J, Phillips D, Skowrońska R, Noweiska A and Wiśniewska H (2020) Development and Cytomolecular Identification of Monosomic Alien Addition and Substitution Lines of Triticale (×Triticosecale Wittmack) With 2Sk Chromosome Conferring Leaf Rust Resistance Derived From Aegilops kotschyi Boiss. Front. Plant Sci. 11:509481. doi: 10.3389/fpls.2020.509481
Received: 01 November 2019; Accepted: 14 October 2020;
Published: 14 December 2020.
Edited by:
Geoffrey Meru, University of Florida, United StatesReviewed by:
Zujun Yang, University of Electronic Science and Technology of China, ChinaRudolph Fredua-Agyeman, University of Alberta, Canada
Copyright © 2020 Kwiatek, Ulaszewski, Belter, Phillips, Skowrońska, Noweiska and Wiśniewska. This is an open-access article distributed under the terms of the Creative Commons Attribution License (CC BY). The use, distribution or reproduction in other forums is permitted, provided the original author(s) and the copyright owner(s) are credited and that the original publication in this journal is cited, in accordance with accepted academic practice. No use, distribution or reproduction is permitted which does not comply with these terms.
*Correspondence: Michał T. Kwiatek, bWljaGFsLmt3aWF0ZWtAdXAucG96bmFuLnBs; orcid.org/0000-0001-9442-3124