- Department of Plants and Crops, Faculty of Bioscience Engineering, Ghent University, Ghent, Belgium
Botrytis cinerea, a fungal pathogen that causes gray mold, displays a high degree of phenotypic diversity. Light emitting diodes (LEDs) with specific light spectrum are increasingly used as lighting resource for plant greenhouse production. The chosen light spectrum can also have an effect on the pathogens in this production system. In this study, we investigated the phenological diversity in 15 B. cinerea isolates upon different light treatments. Daylight, darkness, and LED lights with different wavelengths (white, blue, red, blue+red) were chosen as treatments. The 15 Botrytis isolates differed in their mycelial growth rate, conidia production, and sclerotia formation. Light quality had a limited effect on growth rate. All isolates sporulated under daylight treatment, red light resulted in lower sporulation, while white, blue, and blue+red light inhibited sclerotia formation in all isolates, and sporulation in most, but not all isolates. Pathogenicity of the Botrytis isolates was studied on 2-week-old strawberry (Fragaria × ananassa ‘Elsanta’) leaves grown under white, blue, and red LED lights. The isolates differed in virulence on strawberry leaves, and this was positively correlated to oxalic acid production by B. cinerea in vitro. Red LED light improved leaf basal resistance to all the tested Botrytis isolates. Blue light pretreatment resulted in decreased leaf resistance to some isolates. Furthermore, we used image analysis to quantify the virulence of the different Botrytis isolates based on changes in photosynthetic performance of the strawberry leaves: chlorophyll fluorescence (Fv/Fm), chlorophyll index (ChlIdx) and anthocyanin content (modified anthocyanin reflection index, mAriIdx). Fv/Fm showed a strong negative correlation with disease severity and can be an indicator for the early detection of gray mold on strawberry leaves.
Introduction
Botrytis cinerea Pers.:Fr the causal agent of gray mold disease is a filamentous, heterothallic fungus with a necrotrophic life style (Alfonso et al., 2000). This pathogen has a wide host range and infects more than 1,000 plant species worldwide including vegetables, ornamentals, and fruits, leading to important yield and quality losses (Elad et al., 2016). B. cinerea is a highly versatile pathogen. As a necrotroph, it can extract nutrients from dead or senescent plant material, but it can also infect living tissues via direct penetration or through natural openings or wounds (Williamson et al., 2007). Additionally, it can also grow saprophytically. Botrytis can be isolated from different plant species in nature and infection can be reproduced in the laboratory on a wide range of hosts. Yet, a certain degree of host specialization exists in this pathogen (Thompson and Latorre, 1999; Muñoz et al., 2002). For example, Cotoras and Silva (2005) reported that B. cinerea strains isolated from tomato were more virulent on tomato leaves than isolates from grapes. Furthermore, a study of 490 isolates from open-field crops by microsatellite loci suggested the occurrence of host-specific divergence of B. cinerea in perennial hosts (Asadollahi et al., 2013).
B. cinerea isolates show phenotypic and genetic variability. Differences in colony morphology, mycelial growth, sporulation intensity, sclerotia formation, and pathogenicity have been described (Di Lenna et al., 1981; Martinez et al., 2003; Khazaeli et al., 2010; Pande et al., 2010; Kuzmanovska et al., 2012; Kumari et al., 2014). B. cinerea produces a battery of extracellular enzymes, including pectinases and pectin methylesterases (Reignault et al., 1994). Botrytis isolates with different pathogenic capabilities on various host produced different amounts of extracellular pectic enzymes (Di Lenna et al., 1981). High genetic diversity in B. cinerea populations has been revealed using a multiplicity of molecular techniques, such as PCR detection of transposable elements (Martinez et al., 2008), restriction fragment length polymorphism (RFLP) analysis of PCR-amplified loci (Baraldi et al., 2002; Muñoz et al., 2002), PCR amplification of microsatellite loci (Isenegger et al., 2008; Asadollahi et al., 2013), and randomly amplified polymorphic DNA (RAPD) analysis (Alfonso et al., 2000; Pande et al., 2010). Disease control is difficult because the pathogen has a broad host range and it can survive as mycelium and/or conidia or as sclerotia for extended periods. In addition, B. cinerea isolates differ in their sensitivity to fungicides and fungicide resistance is quickly obtained (Kretschmer and Hahn, 2008).
B. cinerea has 11 photoreceptors including 2 cryptochromes, 4 LOVs (light, oxygen, voltage), 2 opsins, and 3 phytochromes to respond to different light conditions varying from near-UV, blue, green, red, and far-red light (Schumacher, 2017). Blue light is sensed by the proteins that bind flavin via LOV or FAD (flavin adenine dinucleotide) domains such as BcWCL1 and BcWCL2 (the orthologs of white collar complex in B. cinerea), and BcVVD1 (the orthologs of vivid in B. cinerea) (Rodriguez-Romero et al., 2010). BcWCL1 interacts with BcWCL2 in the nuclei, forming the white collar complex (WCC), which is required to respond to white light (Schumacher, 2012). Opsins are transmembrane proteins using retinal to sense green light, and phytochromes are histidine kinases using bilin to perceive red/far-red light (Rodriguez-Romero et al., 2010). Based on the light perception by these photoreceptors, B. cinerea senses surrounding light as a decision-tool for morphogenesis, as a guide for directed growth, as a stress factor for protection, and also as a time giver for the circadian clock (Schumacher, 2017). Studies have described the impact of light on mycelial growth, conidiation, sclerotial development, and tropic response, however, unclear results have been reported caused by B. cinerea isolate variability and different experimental conditions (Schumacher, 2017).
Strawberry (Fragaria × ananassa) is an important soft fruit crop that is popular all over the world. Gray mold is a serious disease in strawberry production and leads to important economic losses (Debode et al., 2015; Petrasch et al., 2019). B. cinerea can infect all plant parts of strawberry including leaves, fruits, flowers, petioles, and stems at every growth stage (Williamson et al., 2007; Petrasch et al., 2019). In year-round greenhouse strawberry production, light emitting diodes (LED) are increasingly applied to increase the day length as well as the light intensity in winter. LED lighting offers the possibility of spectral modulation thus influencing both morphology and metabolite content of the leaves. Commercial LED lamps typically combine blue and red wavelengths as these are highly absorbed by chlorophyll and thus promote photosynthesis and biomass production (Okamoto et al., 1996). Application of LED lighting also opens the possibility to increase the strawberry’s resistance to B. cinerea. Indeed, we previously showed that leaves that develop under monochromatic red light are more resistant to Botrytis infection compared to white, blue, and blue+red lights (Meng et al., 2019). Yet, in aforementioned study only one Botrytis strain was investigated. Hence, the investigation of the diversity in light-response of different B. cinerea isolates is of importance for the LED light application in greenhouse production.
Given the facts described above, we hypothesized that B. cinerea isolates will differentially respond to different light qualities. Therefore, we investigated the light-modulated phenotypic diversity of 15 B. cinerea isolates. We characterized their pathogenicity on strawberry leaves under white, blue, and red LED lights and hypothesized that a pretreatment of leaves with red light would reduce disease susceptibility irrespective of the isolate. To assess the virulence of the different Botrytis isolates on strawberry leaves in an objective way, we investigated the potential of image-based early detection to quantify changes in the photosynthetic performance of the leaves (quantum efficiency of photosystem II, chlorophyll index) and/or leaf defense compounds (modified anthocyanin reflection index).
Materials and Methods
Botrytis cinerea Isolates
Fifteen B. cinerea isolates were used in this study (Table 1). Four B. cinerea isolates (B1, B2, B6, and B7) are well-documented laboratory strains. Eleven gray mold isolates were collected from tomato, lettuce, apple, and grape in Belgium. Purified isolates were cultivated on potato dextrose agar (PDA, Becton, Dickinson, and Company) and subjected to long-term storage in 20% glycerol at −80°C.
Light Quality Treatments to Study Botrytis Phenotypes
To investigate mycelial growth, sporulation, and sclerotia production, the 15 B. cinerea isolates were grown on PDA under different spectral light qualities at 20°C. Six different light regimes were established using 1) daylight, 2) white LEDs (300–800 nm, 29% B, 39% G, 27% R, and 5% FR, Philips, the Netherlands), 3) blue LEDs (400–500 nm, peak at 460 nm), 4) red LEDs (600–700 nm, peak at 660 nm), 5) red + blue LEDs (75/25%, peak at 660 nm and 460 nm) (Supplementary Figure 1) and (6) dark as control. A photoperiod of 16 h and a photon flux density of 40 µmol m−2 s−1 were provided by the LEDs. Natural daylight was provided at a lab bench (18°C), with a natural day length of 15 h and average photosynthetic photon flux density (PPFD) of 10 µmol m−2 s−1. The spectral light distribution and the light intensity were measured using a spectroradiometer (JAZ-ULM-200, Ocean Optics, US).
Mycelial plugs with 6 mm in diameter were inoculated in the center of Petri dishes (90 mm in diameter). The Petri dishes were assigned to one of the six light treatments. Six replicates per isolate and per light treatment were used. The radial growth per colony was measured daily on two perpendicular axes for 5 days or until it reached the edge of the plate. The growth rate (cm day−1) was calculated as the average growth length increase per day.
To assess the sporulation and sclerotia production, PDA plates inoculated with 6 mm-mycelial plugs remained under the six light treatments for 15 days. Sporulation and sclerotia production were assessed visually and by microscopy. The class system was set as: class 0 (no spores/sclerotia formation); class I; (very few spores/sclerotia formation); class II (sparse sporulation/sclerotia formation); class III (average amount spores/sclerotia formation); class IV (many spores/sclerotia formation); class V (abundant formation of spores/sclerotia). The classification system for sclerotia is illustrated in Supplementary Figure 2. This was repeated three times with six replicates each time (n = 18).
Oxalic Acid Detection Assay
Plugs of B. cinerea isolates were inoculated on complete medium (Canessa et al., 2013) and always maintained in the dark at 24°C. This pH-indicating medium contains 0.1% bromothymol blue as indicator, and the medium color changes from green to yellow when an acid compound such as oxalic acid is produced. Medium acidification was evaluated after 7 days by its effect on the medium pH. The pH was measured on the outside of the yellow circle using a flat pH electrode (SF113, VWR, Germany) which can test the pH directly via the surface of the medium. Four measurements were conducted for each plate and averaged, this was done in four replications per isolate.
Pathogenicity Test on Strawberry
Strawberry (Fragaria × ananassa ‘Elsanta’) leaves that developed under different light qualities were used for the pathogenicity tests with the 15 B. cinerea isolates. The plants were potted in peat substrate (Van Israel nv, Belgium) and raised in a growth chamber with 70% relative humidity at 20°C. The growth chamber was equipped with three light qualities: white (W, 300–800 nm, Philips, the Netherlands), blue (B, peak at 460 nm, Philips, the Netherlands), and red (R, peak at 660 nm, Philips, the Netherlands) LED lights (Supplementary Figure 1). A photoperiod of 16 h with the photosynthetic photon flux density at 100 µmol m−2 s−1 was provided. The plants were fertilized with Soluplant (N:P:K:Ca 19-8-16-4, Haifa, the Netherlands, EC=1.5 dS/m, pH=5.7) three times per week.
All the 15 isolates were tested on strawberry leaves grown under white LED light, and a subset of 10 isolates was tested on leaves that developed under blue and red LED lights. Botrytis isolates were cultivated on PDA in Petri dishes. After 7–10 days, conidia were washed from the plates with ¼ potato dextrose broth (PDB) solution containing 0.01% (v/v) Tween 20. After removing the mycelium fragments, spore titers were determined microscopically using a Thoma counting chamber. A final concentration of 5×105 spore ml−1 was used for inoculation. Leaf discs of 1-cm in diameter from 2-week-old strawberry leaves were cut the day before inoculation and placed in disposable 24-well plates with water. Each leaf disc was inoculated with 10 µl droplets of conidial suspension on the adaxial leaf surface. Incubation was at 22°C under dark conditions. Disease symptoms were scored after 3 days. A 0–3 ordinal rating scale was employed for disease rating and disease index was calculated. Six leaf discs per leaf with four biological replicates were used in this study.
Image Analysis
Non-invasive spectral phenotyping was applied to monitor the strawberry disease development by a platform that allows to visualize diverse physiological traits in real time, based on specific absorption, reflection, and fluorescence patterns in visible and near-infrared (NIR) wavelengths. The central part of the platform comprises a 3CCD 6 Mp—16 bit camera mounted on a Cartesian coordinate robot, equipped with 12 optical interference filters (CropReporter, PhenoVation B.V., Wageningen, the Netherlands).
This multispectral imaging platform was used daily to record the lesion development of inoculated leaf discs floating in 24-well plates (24 replicates per light quality treatment and Botrytis isolate) from the day of inoculation until 4 days post-inoculation (dpi).
RGB (red green blue) images, reflectance spectra to calculate the anthocyanin index and chlorophyll index and the minimal fluorescence, F0, and the maximum fluorescence, Fm, are captured by the camera. Images obtained from the phenotyping platform were processed via the “Data Analysis Software” program (PhenoVation B.V., Wageningen, the Netherlands).
The modified anthocyanin reflectance index (mAriIdx) was determined using following formula (Gitelson et al., 2009):
The chlorophyll index (ChlIdx) was calculated using following formula (Gitelson et al., 2009):
where ρ550 is the reflectance in the first spectral band, which is maximally sensitive to anthocyanin content; ρ710 the reflectance in the second spectral band, which is maximally sensitive to chlorophyll content but not sensitive to anthocyanin content; and ρ770 the reflectance of the third spectral band, which compensates for leaf thickness and density.
The maximum quantum efficiency of photosystem II (Fv/Fm) was calculated using following formula (Baker, 2008):
Between measurements, the 24-well plates were placed in the dark allowing immediate quantification of the minimal (F0), then saturating red light flashes of 3,000 µmol m−2 s−1 were given. This allows the imaging of the OJIP induction curve at 12 images per second at a resolution of 1.5 Mp. This resolution gives the optimal signal to noise with respect to the detail in the image (PhenoVation B.V., Wageningen, the Netherlands). From the F0 and Fm image measurements based on the OJIP induction curve the variable fluorescence Fv/Fm is calculated (Björkman and Demmig, 1987; Kalaji et al., 2014). This yields images of Fv/Fm and from these images corresponding average values including standard deviation of the whole image are calculated (imaging software and algorithms by PhenoVation B.V., Wageningen, the Netherlands).
Statistical Analysis
Data were tested for normal distribution using the Kolmogorov–Smirnov test and for homoscedasticity of variances using Levene’s test. The mycelial growth rate and disease rating among B. cinerea isolates were compared by the non-parametric Kruskal-Wallis test with Dunn test as the post hoc test. The effect of light quality on mycelial growth rate was analyzed by one-way ANOVA. If significant differences were found, the Tukey test (p ≤ 0.05) was carried out to establish significant differences between means, here a Bonferroni correction was applied when n ≥ 10. The virulence of the Botrytis isolates assessed by the multispectral camera (Fv/Fm, ChlIdx, mAriIdx) were analyzed by one-way ANOVA for each light quality. As the light pretreatment affects both chlorophyll and polyphenol content (Meng et al., 2019), the effects of the light quality pretreatments were analyzed by ANCOVA using the images at the start (day 0) as covariate, adjusted means were calculated using Bonferroni for confidence interval adjustment. All assumptions for performing ANCOVA including homogeneity of regression slopes were checked. All analyses were performed using SPSS version 26 (SPSS Inc., Chicago, USA).
Results
Phenotypic Characterization of B. cinerea Isolates
Significant differences in the mycelial growth rate were observed between the 15 B. cinerea isolates, this for the dark control treatment (Figure 1, Supplementary Table 1). B12 resulted in the lowest growth rate (0.45 cm day−1) while B2 and B8 had a ± three-fold higher growth rate (respectively 1.31 and 1.32 cm day−1).
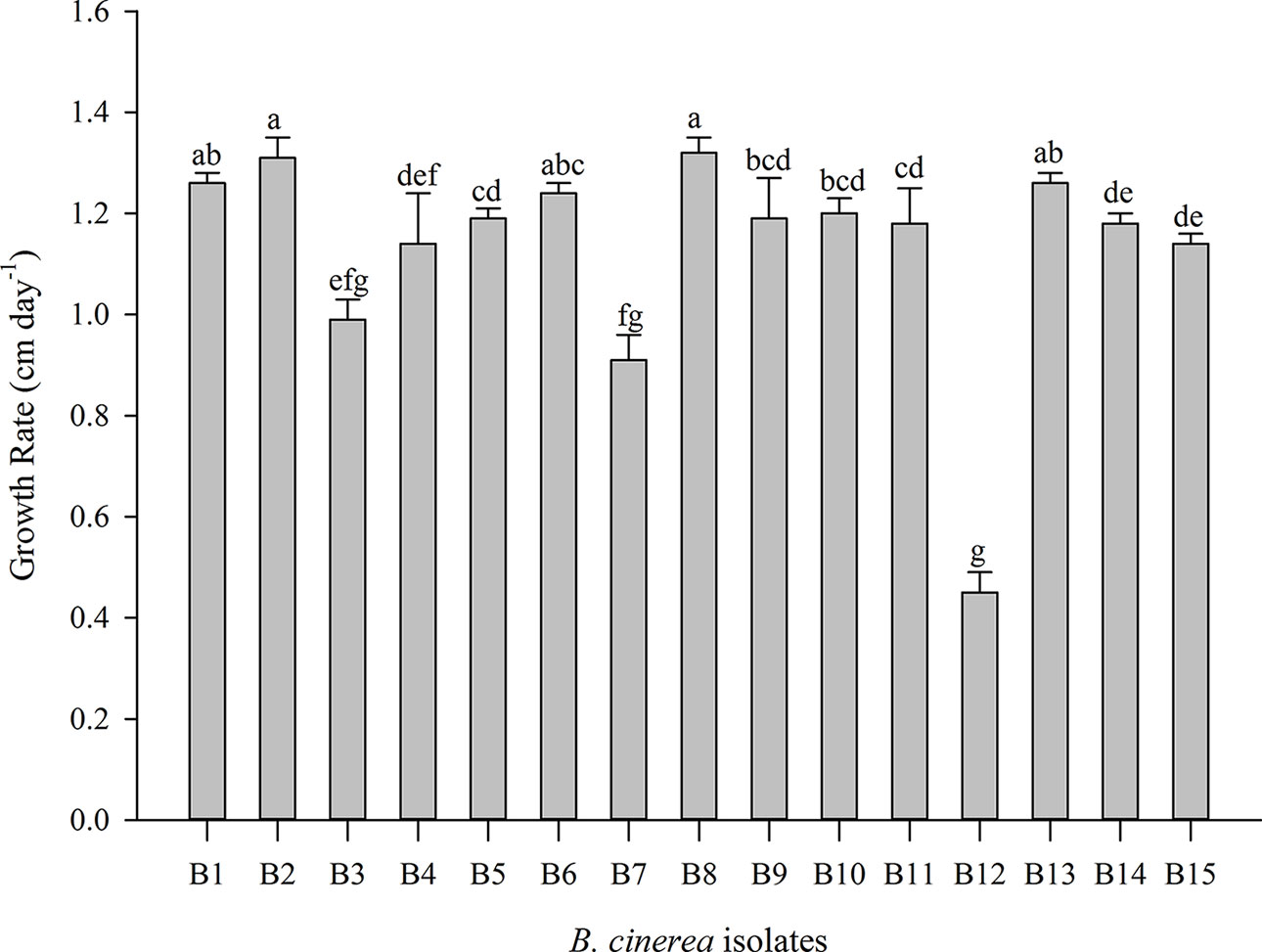
Figure 1 Mycelial growth rate of 15 Botrytis cinerea isolates in dark condition. Data are presented as mean of six replicates with standard deviation. Different letters indicate statistical differences among B. cinerea isolates based on Kruskal-Wallis test with Bonferroni correction (Dunn test, p ≤ 0.0033).
Light conditions greatly influenced the growth rate of B. cinerea (Figure 2, Supplementary Table 1). For the isolates B2, B3, B4, B5, B7, B12, and B14, the mycelial growth was considerable higher under LED lights (white, blue, red, blue+red) compared to the dark and daylight treatment. The spectral quality of the LED treatment did not affect the growth rate of B2, B5, B7, B9, B10, B12, B13, and B15. However, compared to white LED light, a significant increase was observed in the growth rate of B8 under blue light, while red light enhanced the growth rate of B1, B11, and B14. The combination blue+red, decreased the mycelial growth of B3 and B11 considerably, while an increase was observed in B1. Additionally, B1 was the only isolate where the highest growth rate was observed in the dark while all other isolates had higher or equal growth rates than the dark treatment.
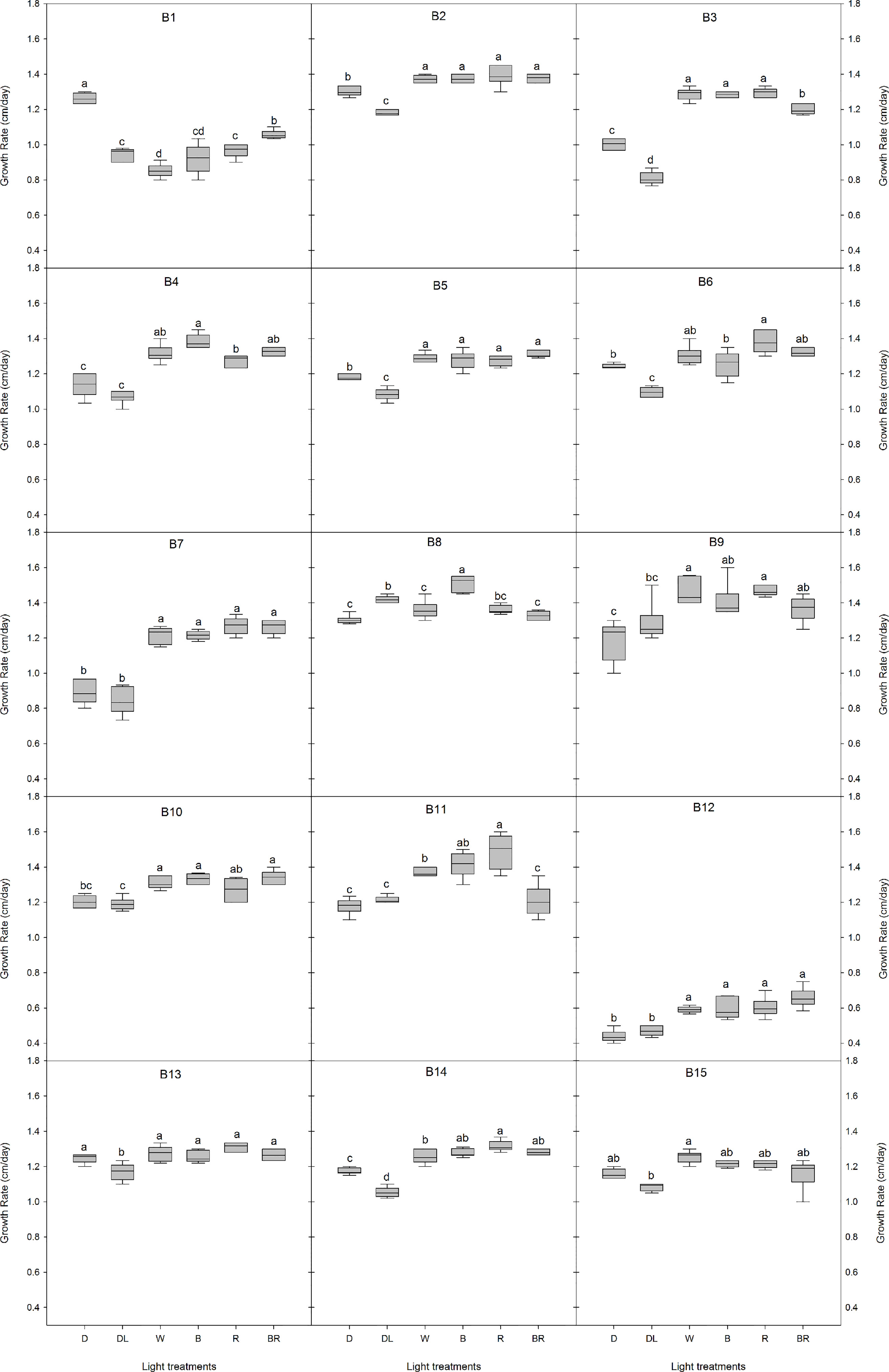
Figure 2 Effects of light quality on the growth rate of 15 Botrytis cinerea isolates. Six light treatments were analyzed namely dark (D), daylight (DL), white (W), blue (B), red (R), and blue+red (BR). Different letters indicate statistical differences between light treatments for each isolate based on Tukey’s test (p ≤ 0.05), except for B2 and B15 where a non-parametric Kruskal-Wallis followed by a post-hoc Dunn’s test (p ≤ 0.05) was performed.
Sporulation and sclerotia formation varied among the 15 B. cinerea isolates, and both were considerably affected by the light treatments used in this study (Figure 3). The 15 isolates were grouped according to the presence/absence of sporulation and of sclerotia formation under the light treatments (Figure 3). Four groups were defined based on their sporulation response. A first group clusters two lettuce and two tomato isolates (B3, B4, B13, B15) as they only produced spores under daylight and red LED light. A second group, including three lettuce and one grape isolate (B5, B8, B10, B14) sporulated in dark condition as well as under daylight and red light. The third group (grape isolates B2 and B11) developed spores under daylight, red and blue light. The fourth group, including grape and lettuce isolates (B1, B6, B9, and B12) exhibited great variability in sporulation compared to the other isolates under the considered light conditions. Finally, the nonpathogenic mutant B7 (A366) forms a fifth group and produced spores in all six treatments.
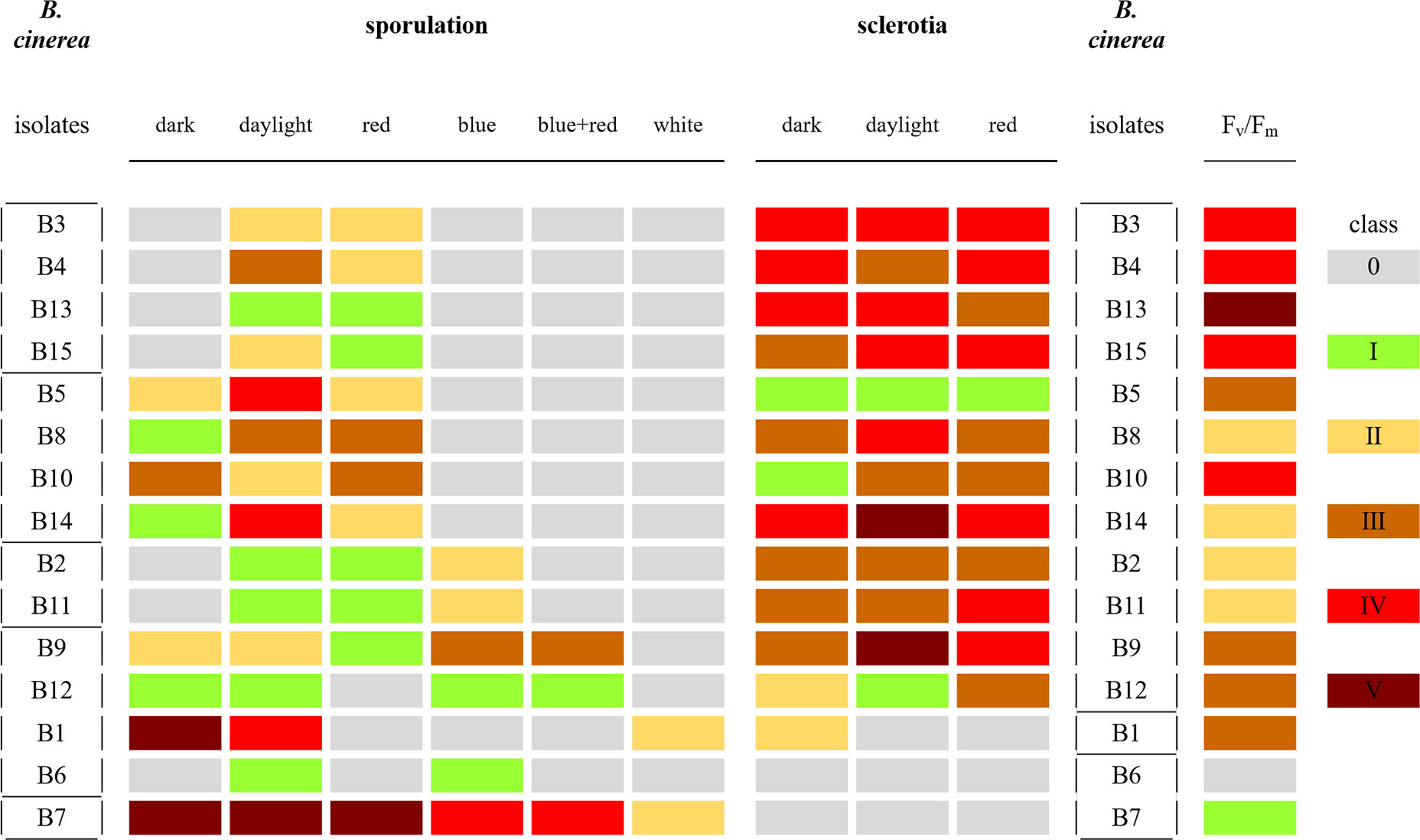
Figure 3 Classification of sporulation and sclerotia formation in the 15 Botrytis cinerea isolates after 15 days under six light treatments: dark, daylight, white, blue, red, and blue+red LED, and classification based on Fv/Fm values at 4 dpi from infection in white-light-leaves. Classes of sporulation and sclerotia presented are from three replications with six plates for each replicate (n = 18). The classes of sporulation and sclerotia formation are: class 0 (no spores/sclerotia formation); class I (very few spores/sclerotia formation); class II (sporulation/sclerotia formation sparse); class III (average amount of spores/sclerotia formation); class IV (many spores/sclerotia formation); class V (abundant spore/sclerotia formation). The class of virulence is based on the significant letters of Fv/Fm at 4 dpi (Table 2) with exclusion of B6 which is indicated by a light gray color. The classes are: class I (significant letters starting with a); class II (significant letters starting with b); class III (significant letters starting with c); class IV (significant letters starting with d); class V (significant letters starting with e). These classes are shown in colors (see the color bar).
No sclerotia formation was observed under white, blue, and blue+red LED lights, this for all isolates. Three groups could be distinguished based on the formation of sclerotia in dark, under daylight or under red light (Figure 3). No sclerotia were produced in B6 (B05.10) and B7 (A366) irrespectively the light or dark treatment, resulting in a first group. B1 (R16) formed a second group with only sclerotia formation in the dark. The third group is formed by the remaining twelve isolates including lettuce, tomato, apple, and grape isolates, as they all produced sclerotia under dark, daylight, and red LEDs.
Oxalic acid formation can be indicative as a virulence factor for Botrytis (Williamson et al., 2007; Sun et al., 2019). Media acidification due to organic acid formation was strongest for B4, B6, B12, B13, and B15, resulting in a decrease of more than 1.5 pH units compared to a non-inoculated control. The nonpathogenic mutant B7 (A366) did not acidify the medium in comparison to the control. All other isolates caused an intermediate acidification reducing the medium with 1.0 pH unit in comparison to the control (Figure 4). White, blue, and red LED irradiation had no significant effect on media acidification caused by different Botrytis isolates (data are not shown).
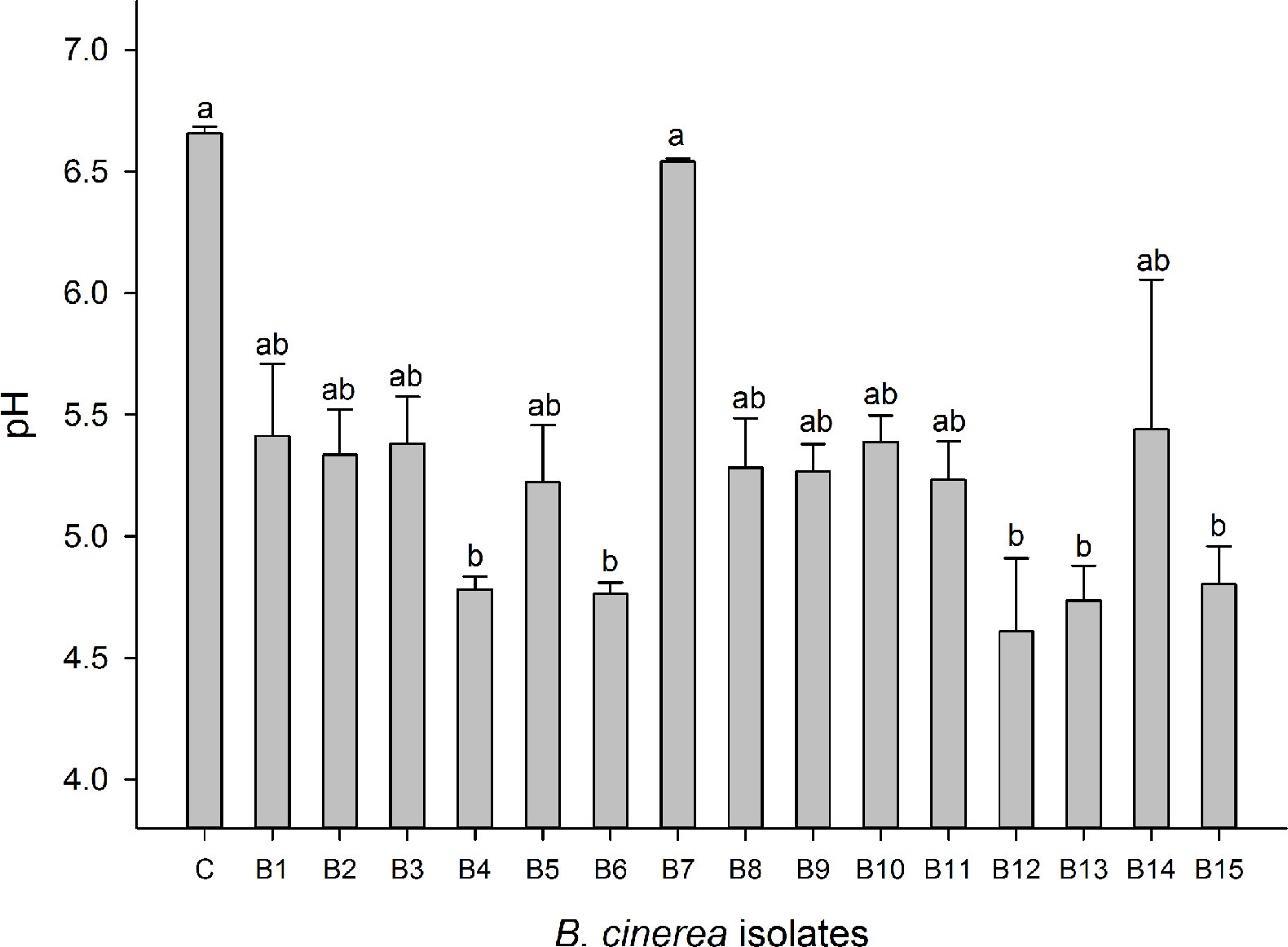
Figure 4 Oxalic acid production of Botrytis cinerea isolates was assessed based on changes in the medium pH. Control treatment (C) is medium without Botrytis inoculation. Data are shown as means ± SE (n=4). Different letters indicate statistical differences between B. cinerea isolates. Non-parametric Kruskal-Wallis followed by a post-hoc Dunn’s test was performed with p ≤ 0.05.
Inoculation Assays on Strawberry Leaves
First, 14 Botrytis isolates were tested by spore inoculation on white LED light-developed strawberry leaves. B6 could not be included because of its poor sporulation (Figure 5). Different degrees of virulence on strawberry leaves were observed between these 14 isolates. B4, B10, B13, and B15 were the most virulent, followed by B1, B2, B3, B5, B9, and B14. An intermediate virulence was observed for B8, B11, and B12. As expected, strawberry leaves were hardly infected by the non-pathogenic B7 (A366) strain.
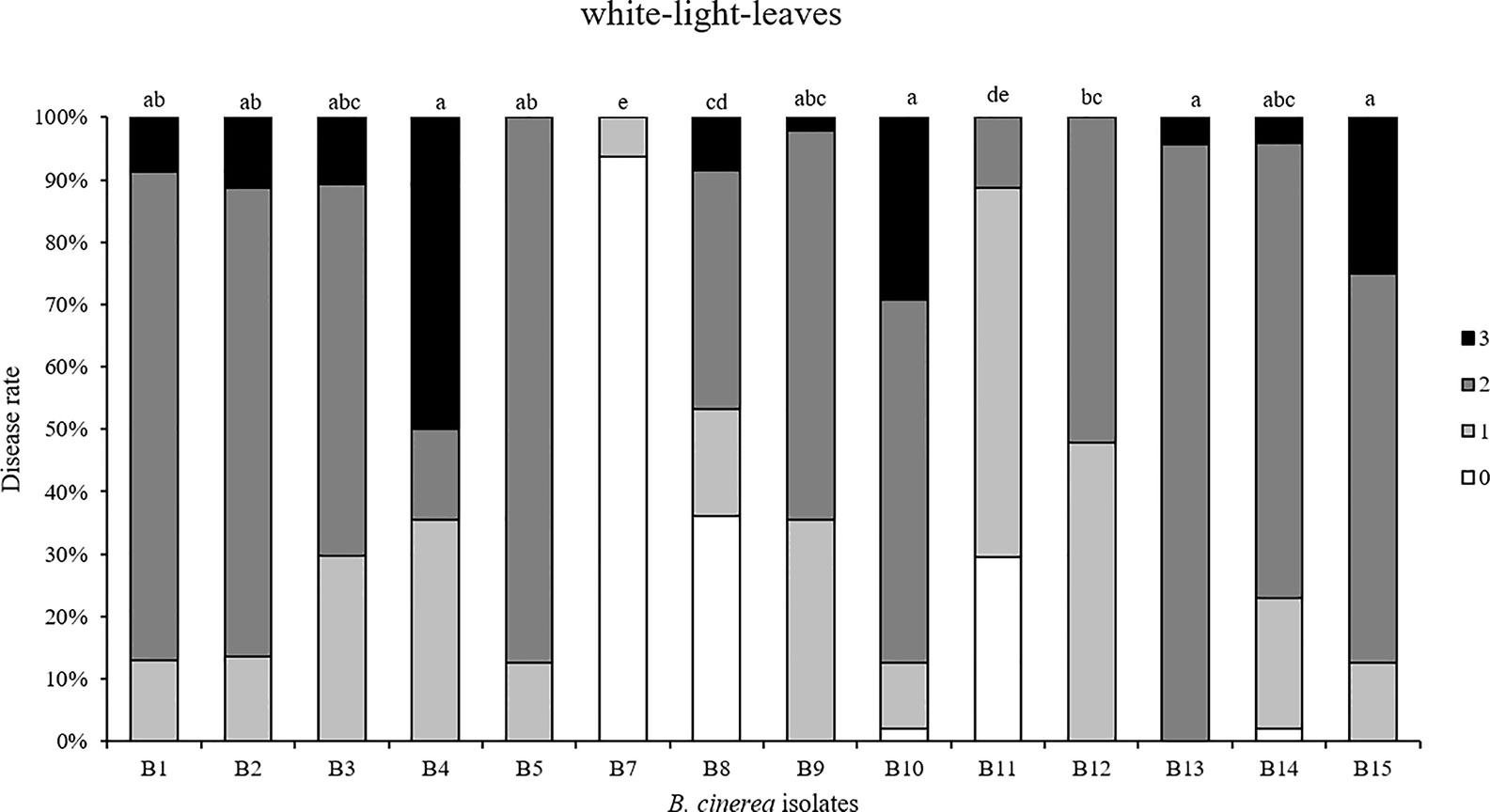
Figure 5 Disease severity caused by 14 Botrytis cinerea isolates spore-inoculated on strawberry leaves grown under white LED lights. Disease rating was scored 3 days post-inoculation using four scoring categories (0, resistant; 1, slightly spreading lesion; 2, moderately spreading lesion; 3, severely spreading lesion). B6 was not tested because not enough spores were produced. Different letters indicate statistical differences among the isolates performed by Kruskall-Wallis test followed by a post-hoc Dunn’s test with Bonferroni correction (p ≤ 0.0036, n = 4).
Second, ten Botrytis isolates were tested on strawberry leaves that were developed under blue and red LED lights. The isolates showed significant variations in their virulence (Supplementary Figures 3A, B). B7 (A366) was again the least aggressive, while B1, B2, and B13 were the most aggressive isolates and B15 resulted in moderate disease rating on the leaves that had developed either under blue or red light. Strawberry leaves that originated from red light were more resistant against the tested Botrytis isolates compared to leaves from white or blue light (Figure 6). Blue-light-developed leaves were more susceptibility to B1, B2, B9, B12, B13, while no remarkable difference was noted for isolates B3, B8, B10, B15, when compared to white-light-developed leaves. The isolate B7 (A366) also remained non-virulent on blue or red light-developed leaves (Supplementary Figures 3A, B).
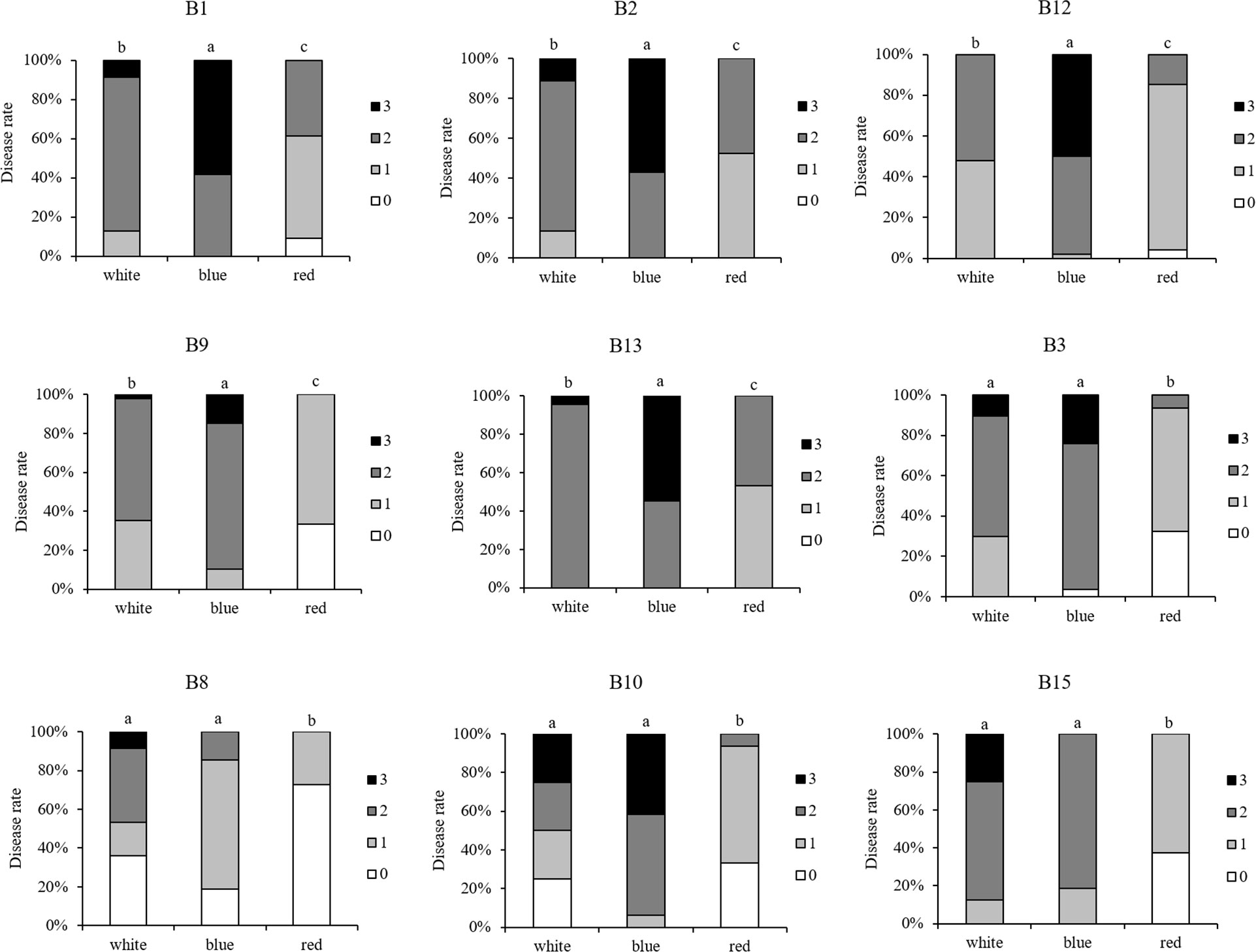
Figure 6 Comparison of virulence per isolate (including B1, B2, B3, B8, B9, B10, B12, B13, B15) with respect to the light pretreatments of the strawberry leaves. For each Botrytis isolate, virulence was tested on strawberry leaves grown under white, blue, and red LED lights, spore-inoculated leaf discs were dark incubated after inoculation and disease severity was compared between white-, blue-, and red-light-developed leaves. Disease rating was scored 3 days post-inoculation using four scoring categories (0, resistant; 1, slightly spreading lesion; 2, moderately spreading lesion; 3, severely spreading lesion). Different letters indicate statistical differences among the isolates performed by Kruskall-Wallis followed by a post-hoc Dunn’s test (p ≤ 0.05).
Overall, B8 (lettuce isolate) was the least aggressive Botrytis isolate on strawberry leaves irrespective of light treatments, while B13 (tomato isolate) was the most aggressive one. Interestingly, B15 (lettuce isolate) resulted in the strongest disease symptoms on leaves from white light, however, blue- and red-light developed leaves displayed moderate resistance to B15.
Image-Based Assessment of Botrytis Infection on Strawberry Leaves
For the image-based assessments of the progress rate of the disease after spore inoculation, changes in both chlorophyll fluorescence imaging (Fv/Fm) and stress indices (ChlIdx and mAriIdx) were assessed during 5 days (Figure 7, Supplementary Figures 4-6). The phenotyping of the plant resistance is shown from 0 to 4 dpi for three representative Botrytis isolates: the non-pathogenic B7 strain, the intermediate aggressive B12, and the most aggressive B13 isolate as determined by visual scoring on white-light-developed leaves (Figure 5). The non-pathogenic B7 displayed a minor but significant decrease in Fv/Fm from 0.755 at 0 dpi to 0.637 at 4 dpi and hardly any change in ChlIdx and mAriIdx (Figure 7A). For B12 a strong decline was observed in Fv/Fm from 3 dpi on, with values decreasing from 0.755 at 0 dpi to 0.263 at 4 dpi. Correspondingly, also ChlIdx displayed a significant decrease, while mAriIdx increased considerably from 0 to 4 dpi (Figure 7B). The isolates B8, B9, B11, and B14 showed similar temporal changes as the intermediate aggressive isolate B12 for Fv/Fm, ChlIdx, and mAriIdx, except for the mAriIdx of B14 (Supplementary Figure 4).
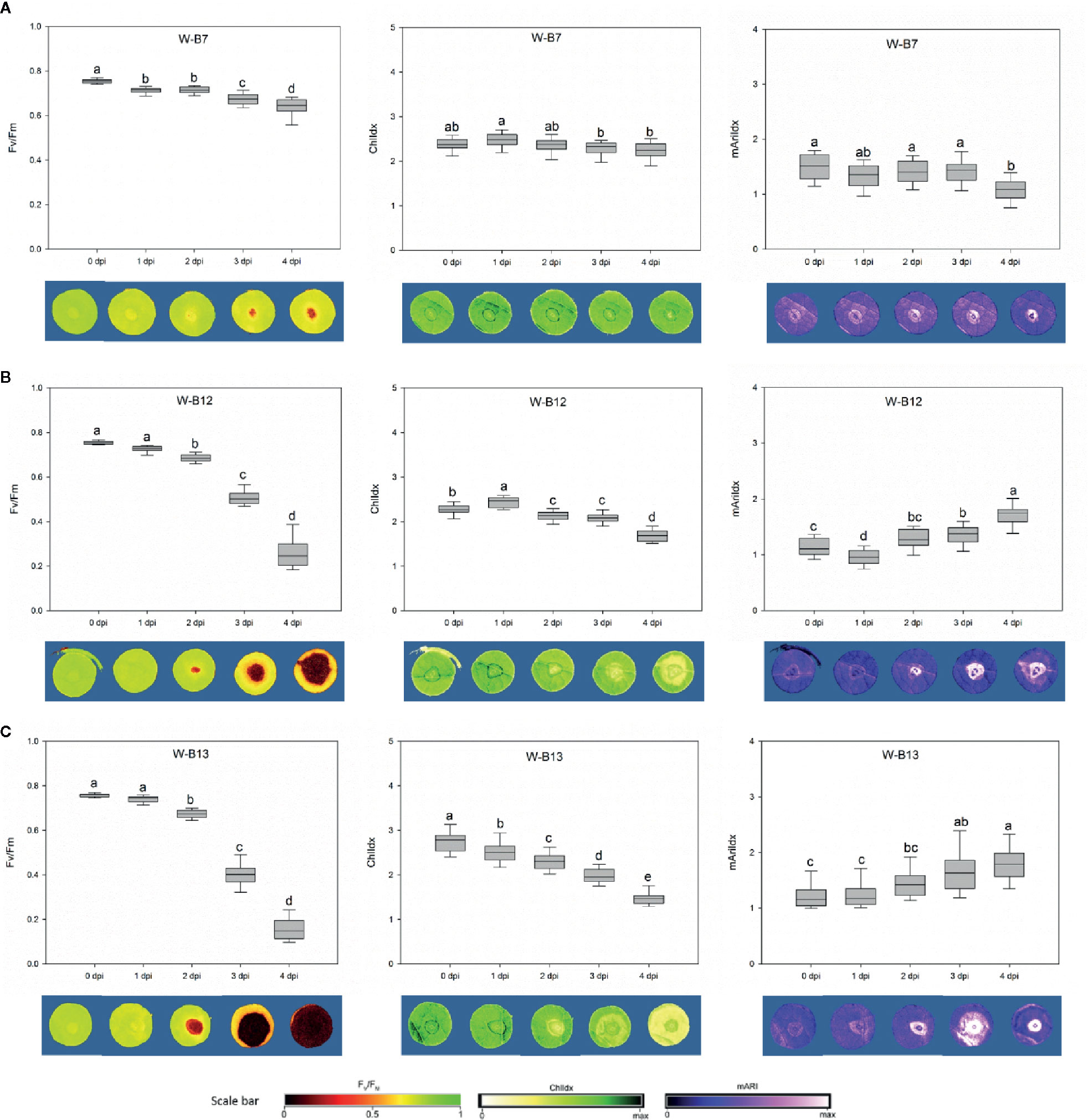
Figure 7 The variations of Fv/Fm, ChlIdx, and mAriIdx from 0 to 4 dpi caused by Botrytis cinerea isolates B7 (A), B12 (B), and B13 (C) were correlated with the development of disease lesion on white-light-leaves. The corresponding images are presented underneath the figures. Disease lesion led to darker Fv/Fm image with lower value, yellower ChlIdx image with lower value, and brighter mAriIdx image with higher level. One-way ANOVA was applied for the statistical analysis (Tukey test, p ≤ 0.05), and data are shown by box plots with median. Different letters indicates significant differences among the time points.
The most virulent isolate B13 caused the greatest decrease of Fv/Fm, this was already very strong at 3 dpi, while values further decreased to 0.172 at 4 dpi. Simultaneously a clear decrease in ChlIdx and increase in mAriIdx was found (Figure 7C). B1, B2, B3, B4, B10, and B15 grouped with B13 based on their virulence on strawberry leaves and they caused the same trends in Fv/Fm and ChlIdx. However, no significant increase was observed in mAriIdx for B1, B3, and B15.
The Botrytis isolates resulted in considerable variations in Fv/Fm, ChlIdx, and mAriIdx of the inoculated leaves at 4 dpi, this for all the pre-inoculation light quality regimes of the leaves (Table 2).
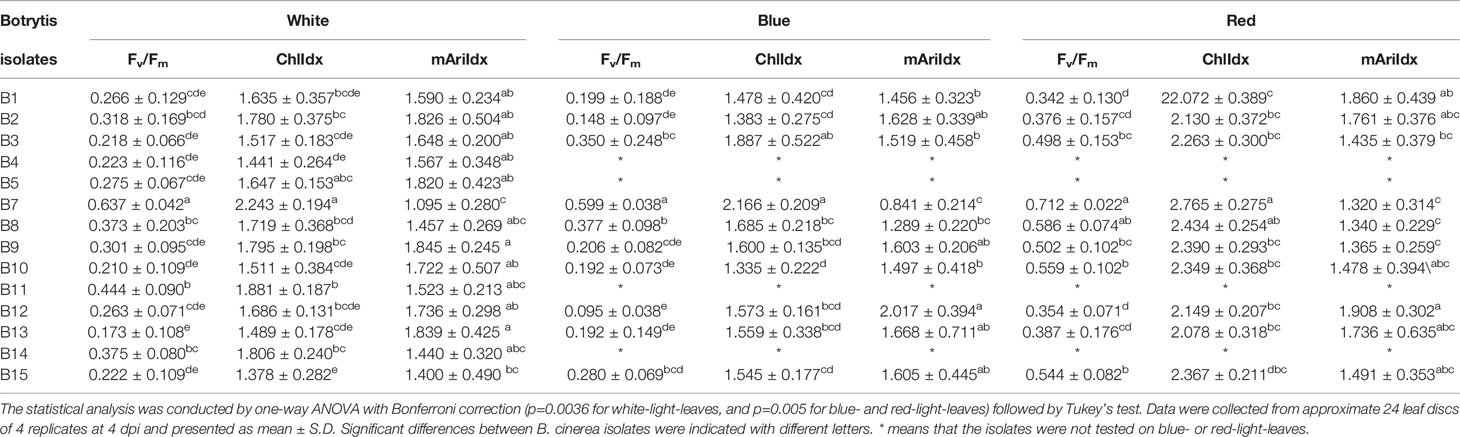
Table 2 Effect of inoculation with different Botrytis isolates at 4 dpi on Fv/Fm, ChlIdx, and mAriIdx in strawberry leaves grown under white, blue, and red LED lights.
Leaves inoculated with the nonpathogenic strain B7 (A366) maintained the highest level of Fv/Fm and ChlIdx, while the lowest levels of mAriIdx were observed, this irrespective of the light quality treatments. Leaf inoculation with the virulent B13 resulted in the strongest decrease of Fv/Fm for white-light-leaves, but not for the other light quality pretreatments. Here, Fv/Fm was lowest after B12 inoculation of leaves grown under both blue and red light. Leaf yellowing and chlorophyll content, assessed by ChlIdx was lowest after B15 inoculation of white-light-leaves, B10 inoculation of blue-light-developed leaves, and B1 inoculation of red-light-developed leaves. Increase of anthocyanins (mAriIdx) was highest in both B9 and B13 in white-light-developed leaves, and in B12 in both blue- and red-light leaves (Table 2).
Light pretreatment effects are thus clearly present and these effects are shown in Table 3. Overall red pretreated leaves have a significant higher Fv/Fm (p < 0.001) and ChlIdx (p < 0.001), while no difference between blue and white pretreated leaves is found. Only for strains B1 and B13 this positive effect of red light to maintain higher Fv/Fm levels was not observed, while this was the case of B2 with respect to ChlIdx. Overall no significant effect of light pretreatment was found for mAriIdx (p = 0.41), indeed only for 3 out of 10 isolates an effect of light pretreatment of the leaves was present, in B1 and B7 mAriIdx increased significantly while for B9 a significant decrease was found.
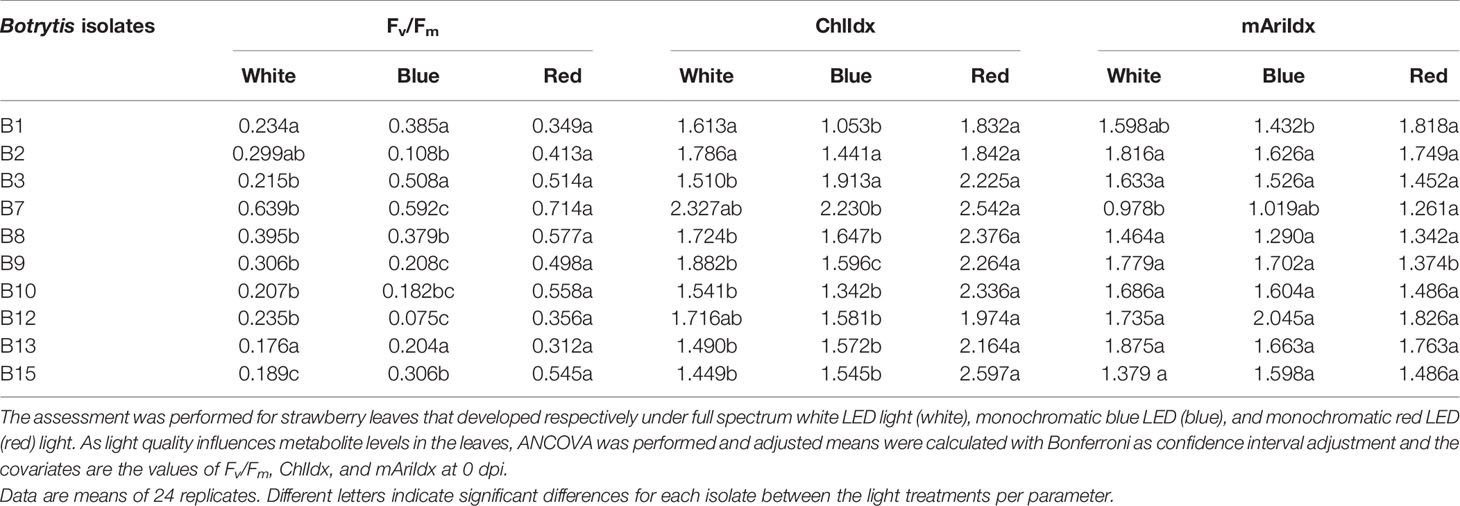
Table 3 Effect of light quality on the virulence of the B. cinerea isolates assessed by chlorophyll fluorescence imaging (Fv/Fm) and image-based indices (ChlIdx, and mAriIdx) at 4 dpi.
Additionally, overall comparison of anthocyanins with respect to the different light qualities (Supplementary Table 2) or more specifically for three representative B. cinerea isolates (Supplementary Table 3) were performed from 0 to 4 dpi. Blue light-developed leaves resulted in significant lower basal anthocyanin levels compared to leaves developed under white and red lights (Supplementary Table 2). A higher anthocyanin content was observed in Botrytis isolates with higher virulence (Supplementary Table 3).
As virulence of the isolates differed, correlations between the disease index and Fv/Fm, ChlIdx, and mAriIdx were explored (Figure 8). High correlations between disease index and Fv/Fm, were found, this irrespective of the light quality pretreatment (all r ≥ 0.978, Figure 8A). Stress indices resulted in lower though still significant correlations between the disease index and ChlIdx although the correlation was lowest for red pretreated leaves (r > 0.917 for leaves from white light and blue light, while r is 0.824 for leaves from red light, Figure 8B). Correlations between disease index and mAriIdx were much lower (r < 0.699, Figure 8C).
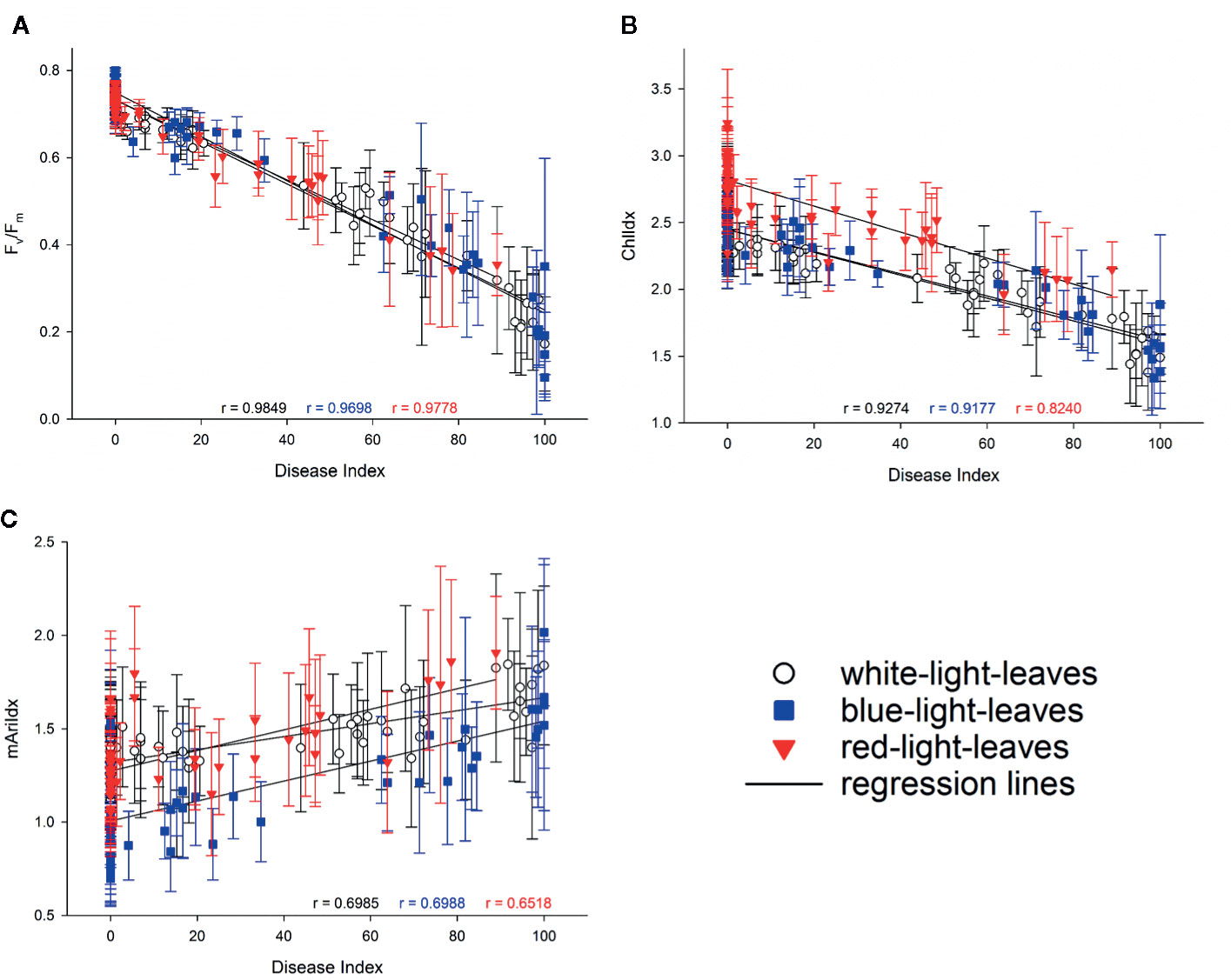
Figure 8 Correlations between disease index and Fv/Fm (A), ChlIdx (B), and mAriIdx (C) on strawberry leaves from white (in white), blue (in blue), and red (in red) LED lights. Values are presented as means with standard deviation shown by vertical bar. The Pearson correlation r is shown in colors to indicate the corresponding light treatments.
We also checked the correlations between Fv/Fm and phenotypic characteristics of the different isolates such as growth rate (Figure 1) and medium acidification (Figure 4). Virulence and medium acidification were highly correlated (Supplementary Figure 7A), while no correlation between virulence and mycelial growth rate was observed (Supplementary Figure 7B).
Discussion
Phenotypic Variability of Botrytis cinerea Isolates Is Influenced by Light Quality
The 15 Botrytis isolates in this study differed in their mycelial growth rate and in their ability to reproduce by conidia or form sclerotia (Figures 1–3, Supplementary Table 1). This intraspecies variation in B. cinerea is well documented (Martinez et al., 2003; Mirzaei et al., 2009) and can be influenced by environmental factors. As Botrytis is an important pathogen in greenhouse plant production, changes in the greenhouse environment might also lead to phenotypic variations. Here, we specifically focused on the increasing application of monochromatic red and blue light and its combination in lighting strategies with LED. Indeed, B. cinerea, possesses 11 photoreceptors to sense the surrounding light environment, which triggers various photoresponses influencing vegetative growth, sporulation, germination of spores, and sclerotia formation (Schumacher, 2017). Generally, the short UV A and B (300–420 nm) wavelengths retard the mycelial growth of B. cinerea (Tan and Epton, 1973). Here, the shortest wavelength we investigated was monochromatic blue light (460 nm) though this wavelength did not retard the growth rate of the studied isolates, in comparison to the full-spectrum white light. Light quality had a limited effect on the growth rate. Canessa et al. (2013) found that light reduced the daily growth rate of B05.10 (= B6) and attributed this to light-induced stress. It seems that our applied lower light intensities did not induce this light stress except for the strain B1 which showed the highest mycelial growth rate under dark condition. The reduced mycelial growth rate under daylight might be explained by the lower temperature (18°C while 20°C in the climate rooms) as indicated by Tan and Epton (1973).
Early studies showed that sporulation happens exclusively in light, it is strongly stimulated by near-UV light and slightly stimulated by red light, while blue light is ineffective (Tan and Epton, 1973). This light-dependent effect on sporulation was later confirmed for strain B05.10 (= B6) by Canessa et al. (2013) where broad spectrum light (400–720 nm) induced sporulation but blue light inhibited the formation of conidiophores. Also in our research all isolates sporulated under full spectrum daylight (from class I to class V). Monochromatic red light resulted in similar or lower sporulation in most isolates (12 out of the 15), this coincides with the slight stimulation of sporulation by red light reported by Tan and Epton (1973). Sporulation under white LEDs was low, only B1 and B7 sporulated under this light source (both class II) although the light spectrum is very similar to daylight. However, daylight is rich in far-red light (700–800 nm, Supplementary Figure 1), which promotes sporulation (Tan, 1975).
Here, the capacity of blue light to inhibit sporulation was only observed in 9 of the 15 isolates. Blue light stimulated sporulation in B6 (B05.10) and two other grape isolates (B2 and B11), in the nonpathogenic strain B7 (A366), and in two lettuce isolates (B9 and B12). The fact that B05.10 (= B6) produced very few spores (class I) under blue light but also hardly sporulated under daylight, might indicate that despite its genetic stability (Canessa et al., 2013) the strain has mutated. This is also supported by the fact that this strain did not form sclerotia in the dark. Adding red light (600–700 nm) to the blue spectrum did not enhance sporulation as only three isolates sporulated under these conditions. Stewart and Long (1987) reported that Botrytis strains could also show varying degrees of sporulation in the dark. This is also confirmed in our study where 8 out of 15 isolates sporulated in the dark. Although blind strains that exhibit the same phenotype under light and darkness are found in nature, this was not the case for our field collections. Only B7, the nonpathogenic mutant A366 produced conidia under each light quality as well as under dark condition and, as described by Kunz et al. (2006), was not able to form sclerotia.
Early studies indicated that sclerotia formation for survival exclusively occurs in cultures in constant darkness. Yet, this can be affected by small broad light dosages. Less sclerotia were formed when irradiated for 30 min compared to 15 min, and none were observed when irradiated for more than 60 min. Furthermore, sclerotia formation was found to be promoted by red and infrared light (Tan and Epton, 1973). In this study, blue, blue+red, and white lights inhibited sclerotia formation in the 15 B. cinerea isolates. This inhibition is due to the blue light fraction as under red light sclerotia were formed in 12 out of the 15 isolates. In this study also daylight at a very low light fluence (10 µmol m−2 s−1) induced sclerotia promotion which can be caused by the enrichment of the longer wavelengths (red light or the far-red light) in the indoor daylight spectrum (Tan and Epton, 1973).
It is clear that phenotypic responses of B. cinerea isolates to light quality are very diverse and sometimes conflicting with earlier publications. These conflicting results might be due to the fact that limited strains were studied though probably also light intensity might be an interacting factor with light quality. Also day length effects cannot be excluded, most studies apply a photoperiod of 12 h while in this research a photoperiod of 16 h, based on greenhouse lighting duration was applied.
Virulence Variation in Botrytis cinerea
B. cinerea isolates in this study displayed significant variations in their virulence. Virulence diversity in B. cinerea isolates has been studied in various locations and on various plants around the world (Kerssies et al., 1997; Martinez et al., 2005; Mirzaei et al., 2009; Kumari et al., 2014). Variation in virulence of B. cinerea is often due to differences in cell wall degrading enzymatic activities and in the secretion of other virulence factors such as oxalic acid (Derckel et al., 1999). Higher oxalic acid accumulation leads to higher aggressiveness of the pathogen and reversely, lower secretion of oxalic acid is associated with lower virulence (Kunz et al., 2006; Sun et al., 2019). Various degrees of medium acidification caused by oxalic acid production of B. cinerea isolates were also observed in this study (Figure 4). B4, B13, and B15 with highest virulence on strawberry leaves secreted more oxalic acid. In contrast, B7 (A336), the nonpathogenic mutant, showed no oxalic acid production and did not cause disease. Medium acidification and virulence were highly correlated (Supplementary Figure 7A). Yet also other virulence factors such as cell wall degrading enzymes, toxins, and secondary metabolites (Sharma and Kapoor, 2017) can explain differences in virulence. However, it seems that B. cinerea isolates with strong virulence favored less sporulation and produced more sclerotia (Figure 3).
Strawberry Leaves Developed Under Red Light Displayed Enhanced Resistance to Botrytis cinerea Isolates
Higher resistance to all the tested B. cinerea isolates (except for the non-pathogenic B7) was found in strawberry leaves grown under red LEDs (Figure 6). Meng et al. (2019) showed that red LED light increased strawberry leaf basal resistance against R16 (B1 in this study). Resistance improvement by red light was associated with lower hydrogen peroxide levels in the red-light-developed leaves of strawberry (Meng et al., 2019). It might also be linked to phytochrome modulated defense signaling where low R:FR ratios repress the jasmonate response to Botrytis (Cerrudo et al., 2012; Ballaré, 2014). In contrast blue-light-developed leaves were more susceptible to some of the isolates, including B1, B2, B9, B12, and B13 (Figure 6) and had lower level of anthocyanins compared to leaves grown under white and red lights (Supplementary Table 2). Here, both the increased hydrogen peroxide level as well as the reduced anthocyanin level might explain the increased susceptibility (Bassolino et al., 2013; Meng et al., 2019).
Fv/Fm Is the Best Indicator for Early Detection of Botrytis cinerea on Strawberry Leaves
Virulence of the Botrytis isolates on strawberry leaves derived from different light quality pretreatments was also assessed by imaging. The decrease in Fv/Fm synchronized with the development of lesion size, which was visualized on fluorescence images in dark red (Figure 7, Supplementary Figures 4 and 5). Strong correlations between Fv/Fm and disease index were observed in strawberry leaves derived from white (r = 0.985), blue (r = 0.97), and red (r = 0.978) LEDs (Figure 8).
Fv/Fm responded well to the development of Botrytis disease in strawberry leaves. Both biotic and abiotic stress factors decrease the efficiency of photosynthesis and suppress the variable fluorescence of dark-adapted chlorophyll-containing leaves correspondingly. Fv/Fm decreases along with the increasing effect of stresses (Rolfe and Scholes, 2010; Gorbe and Calatayud, 2012). This reduction in Fv/Fm suggests destructive changes in chloroplasts and photosystem II caused by B. cinerea infection. Here ChlIdx was clearly not as sensitive as Fv/Fm, only at higher disease indices (> 50%) this parameter decreased. Furthermore, Fv/Fm could discriminate virulence of the B. cinerea isolates. Higher virulence resulted in a stronger decrease in Fv/Fm, and lower virulence in smaller Fv/Fm reduction. In this study, the infectious symptom by B. cinerea were predominantly observed at 2 dpi based on chlorophyll fluorescence image. Chaerle et al. (2007) also showed that chlorophyll fluorescence imaging can be used for early detection of Botrytis infection. Autofluorescence signals (F440/F740) after Botrytis inoculation on grape berries could only be recorded 4 days after infection (Bélanger et al., 2011), which is a delay of 2 days in comparison with Fv/Fm and 1 day in comparison with ChlIdx response.
Anthocyanins are water-soluble pigments responsible for the red colors in leaves. Different abiotic stresses enhance the biosynthesis of anthocyanins in leaves, and anthocyanins act as antioxidant and reactive oxygen scavengers (Landi et al., 2015). Heim et al. (1983) described that zones of anthocyanin accumulation often surround restricted lesions where a plant disease has been successfully contained, whereas low anthocyanin levels often occur in susceptible combinations of maize. Additionally, anthocyanins levels are negatively correlated with the susceptibility to B. cinerea in tomato fruit (Bassolino et al., 2013). B. cinerea infection causes hydrogen peroxide accumulation which leads to the accumulation of anthocyanin at the infectious site (Chalker-Scott, 1999; Govrin and Levine, 2000). In this study, foliar anthocyanins are indicated by mAriIdx. Along with the pathogen invasion, anthocyanins accumulated at the infectious site as visualized in Figure 7 and Supplementary Figures 4 and 5. Despite the antioxidative role of anthocyanins responding to stresses, few study focuses on its development along with pathogen infection by imaging technology. Here the blue-light-leaves which had an increased susceptibility to Botrytis, showed significant lower basal anthocyanin level compared to leaves derived from white and red lights (Supplementary Table 2). On the other hand, B. cinerea isolates with higher virulence induced a higher anthocyanin content (Supplementary Table 3), this was due to the higher accumulation of hydrogen peroxide caused by Botrytis infection. Furthermore, weaker correlations between Botrytis disease index and anthocyanin level are observed, compared to Fv/Fm and ChlIdx. Therefore, it seems that AriIdx is more a supporting observation, but less useful for the early detection.
Horticultural Implications
B. cinerea causes significant losses in plant greenhouse production, storage, shipping, and marketing, which makes control of gray mold very important (Michailides and Elmer, 2000; Karchani-Balma et al., 2008; González et al., 2009). To control this pathogen cultural, chemical, and biological methods, as well as plant resistance breeding are used (Pande et al., 2006). However, the complexity and variability of this pathogen are reasons that make control difficult (Mirzaei et al., 2009). Awareness of the existence of pathogen variability together with the new insights in photobiological responses of both pathogen and plants may contribute to more efficient non-chemical methods of control. Here it is confirmed that red LED irradiance improved leaf resistance not only to one B. cinerea strain, but to all tested isolates in this study. Therefore, red LEDs have potential to be used in plant production system to control gray mold but this should be further investigated in greenhouse conditions. Moreover, effects of red LED irradiance on Botrytis infections on strawberry flowers and fruits need to be assessed.
Many biotic stress symptoms start as spots or patches within a crop. Such symptoms could be discovered with imaging techniques at an early stage, when no visible symptoms are yet apparent. The possibility of early stress detection allows timely treatment to prevent pathogen spread within the crop and greenhouse, which would result in limited yield loss and reduced chemicals usage. Today robots equipped with sensors are under investigation in greenhouse production. A UV-Robot with UV-C radiation is developed to control powdery mildew in horticulture (Mazar et al., 2018). This could also be equipped with imaging sensors, as early detection of disease is beneficial from both economic and environmental perspectives. In this study we evaluated imaging sensors in a highly controlled environment, without interference from other environmental factors such as wind, fluctuating light intensities, and temperature. Moreover, in horticultural production systems, not only environmental factors change in a dynamic way but also crop-dependent factors such as leaf morphology and orientation, leaf waxes and hairs, and leaf density might influence the response. Therefore, the potential of early detection of gray mold by chlorophyll fluorescence imaging in horticulture needs further validation in a greenhouse environment (Gorbe and Calatayud, 2012; Mahlein, 2016).
Conclusion
Here it is clearly shown that B. cinerea isolates differently respond to different light qualities in phenotypes such as mycelial growth, sporulation, and sclerotia formation. Despite differences in virulence, red light considerably improved leaf basal resistance against all the tested B. cinerea isolates, while blue light pretreatment increased leaf susceptibility to some of them. Disease development caused by different B. cinerea isolates is highly correlated with Fv/Fm (maximal PSII quantum efficiency), meaning that this indicator can be used to objectively quantify B. cinerea disease severity and may also be useful for early detection of plant stress related to gray mold infection. Overall, red LED light has potential to control gray mold in greenhouse production, and image sensors could be developed into a new technology for early disease detection.
Data Availability Statement
The raw data supporting the conclusions of this article will be made available by the authors, without undue reservation.
Author Contributions
LM, HM, MH, and M-CL conceived and designed the experiments. HM performed the experiments of light quality effects on phenotypic variations in the 15 Botrytis isolates. LM conducted the experiments of pathogenic test of Botrytis isolates on strawberry leaves developed under white, blue, and red LED lights and of imaging from the phenotyping platform. MA and KA guided the experiments on the phenotyping platform and image data analysis. LM analyzed the data and drafted the manuscript. HM, MA, KA, MH, and M-CL critically revised the manuscript. LM, MH, and M-CL reviewed and approved the final manuscript.
Funding
The first author has a grant of the China Scholarship Council (CSC).
Conflict of Interest
The authors declare that the research was conducted in the absence of any commercial or financial relationships that could be construed as a potential conflict of interest.
Supplementary Material
The Supplementary Material for this article can be found online at: https://www.frontiersin.org/articles/10.3389/fpls.2020.01233/full#supplementary-material
References
Alfonso, C., Raposo, R., Melgarejo, P. (2000). Genetic diversity in Botrytis cinerea populations on vegetable crops in greenhouses in south-eastern Spain. Plant Pathol. 49 (2), 243–251. doi: 10.1046/j.1365-3059.2000.00452.x
Asadollahi, M., Fekete, E., Karaffa, L., Flipphi, M., árnyasi, M., Esmaeili, M., et al. (2013). Comparison of Botrytis cinerea populations isolated from two open-field cultivated host plants. Microbiol. Res. 168 (6), 379–388. doi: 10.1016/j.micres.2012.12.008
Baker, N. R. (2008). Chlorophyll Fluorescence: A Probe of Photosynthesis In Vivo. Annu. Rev. Plant Biol. 59 (1), 89–113. doi: 10.1146/annurev.arplant.59.032607.092759
Ballaré, C. L. (2014). Light regulation of plant defense. Annu. Rev. Plant Biol. 65, 335–363. doi: 10.1146/annurev-arplant-050213-040145
Baraldi, E., Bertolini, P., Chierici, E., Trufelli, B., Luiselli, D. (2002). Genetic diversity between Botrytis cinerea isolates from unstored and cold stored kiwi fruit. J. Phytopathol. 150 (11–12), 629–635. doi: 10.1046/j.1439-0434.2002.00809.x
Bassolino, L., Zhang, Y., Schoonbeek, H. J., Kiferle, C., Perata, P., Martin, C. (2013). Accumulation of anthocyanins in tomato skin extends shelf life. New Phytol. 200 (3), 650–655. doi: 10.1111/nph.12524
Bélanger, M. C., Roger, J. M., Cartolaro, P., Fermaud, M. (2011). Autofluorescence of grape berries following Botrytis cinerea infection. Int. J. Remote Sens. 32 (14), 3835–3849. doi: 10.1080/01431161003782064
Björkman, O., Demmig, B. (1987). Photon yield of O2 evolution and chlorophyll fluorescence characteristics at 77 K among vascular plants of diverse origins. Planta 170 (4), 489–504. doi: 10.1007/BF00402983
Canessa, P., Schumacher, J., Hevia, M. A., Tudzynski, P., Larrondo, L. F. (2013). Assessing the effects of light on differentiation and virulence of the plant pathogen botrytis cinerea: Characterization of the white collar complex. PloS One 8 (12), e84223. doi: 10.1371/journal.pone.0084223
Cerrudo, I., Keller, M. M., Cargnel, M. D., Demkura, P. V., de Wit, M., Patitucci, M. S., et al. (2012). Low red/far-red ratios reduce Arabidopsis resistance to Botrytis cinerea and jasmonate responses viaa COI1-JAZ10-dependent, salicylic acid-independent mechanism. Plant Physiol. 158 (4), 2042–2052. doi: 10.1104/pp.112.193359
Chaerle, L., Hagenbeek, D., Vanrobaeys, X., Van Der Straeten, D. (2007). Early detection of nutrient and biotic stress in Phaseolus vulgaris. Int. J. Remote Sens. 28 (16), 3479–3492. doi: 10.1080/01431160601024259
Chalker-Scott, L. (1999). Environmental significance of anthocyanins in plant stress responses. Photochem. Photobiol. 70 (1), 1–9. doi: 10.1111/j.1751-1097.1999.tb01944.x
Cotoras, M., Silva, E. (2005). Differences in the initial events of infection of Botrytis cinerea strains isolated from tomato and grape. Mycologia 97 (2), 485–492. doi: 10.3852/mycologia.97.2.485
Debode, J., Van Hemelrijck, W., Xu, X. M., Maes, M., Creemers, P., Heungens, K. (2015). Latent entry and spread of Colletotrichum acutatum (species complex) in strawberry fields. Plant Pathol. 64 (2), 385–395. doi: 10.1111/ppa.12247
Derckel, J. P., Baillieul, F., Manteau, S., Audran, J. C., Haye, B., Lambert, B., et al. (1999). Differential induction of grapevine defenses by two strains of Botrytis cinerea. Phytopathology 89 (3), 197–203. doi: 10.1094/PHYTO.1999.89.3.197
Di Lenna, P., Marciano, P., Magro, P. (1981). Comparative Investigation on Morphological and Physiological Features of Three Isolates of Botrytis cinerea. J. Phytopathol. 100 (3), 203–211. doi: 10.1111/j.1439-0434.1981.tb03293.x
Elad, Y., Pertot, I., Cotes Prado, A. M., Stewart, A. (2016). Plant hosts of Botrytis spp. Botrytis - the fungus, the pathogen and its management in agricultural systems. Switzerland: Springer International Publishing. doi: 10.1007/978-3-319-23371-0_20
Faretra, F., Pollastro, S. (1991). Genetics of resistance to benzimidazole and dicarboximide fungicides in isolates of Botryotinia fuckeliana (Botrytis cinerea). Mycol. Res. 95 (8), 943–951. doi: 10.1111/j.1365-3059.1993.tb02933.x
Gitelson, A. A., Chivkunova, O. B., Merzlyak, M. N. (2009). Nondestructive estimation of anthocyanins and chlorophylls in anthocyanic leaves. Am. J. Bot. 96 (10), 1861–1868. doi: 10.3732/ajb.0800395
González, G., Moya, M., Sandoval, C., Herrera, R. (2009). Genetic diversity in Chilean strawberry (Fragaria chiloensis): differential response to Botrytis cinerea infection. Spanish J. Agric. Res. 7 (4), 886. doi: 10.5424/sjar/2009074-1102
Gorbe, E., Calatayud, A. (2012). Applications of chlorophyll fluorescence imaging technique in horticultural research: A review. Sci. Hortic. 138, 24–35. doi: 10.1016/j.scienta.2012.02.002
Govrin, E. M., Levine, A. (2000). The hypersensitive response facilitates plant infection by the necotrophic pathogen Botrytis cinerea. Curr. Biol. 10, 751–757. doi: 10.1016/S0960-9822(00)00560-1
Hamada, W., Reignault, P., Bompeix, G., Boccara, M. (1994). Transformation of Botrytis cinerea with the hygromycin B resistance gene, hph. Curr. Genet. 26 (3), 251–255. doi: 10.1007/BF00309556
Heim, D., Nicholson, R. L., Pascholati, S. F., Hagerman, A. E., Billett, W. (1983). Etiolated Maize Mesocotyls: A Tool for Investigating Disease Interactions. Phytopathology 73, 424–428. doi: 10.1094/phyto-73-424
Isenegger, D. A., MacLeod, W. J., Ford, R., Taylor, P. W. J. (2008). Genotypic diversity and migration of clonal lineages of Botrytis cinerea from chickpea fields of Bangladesh inferred by microsatellite markers. Plant Pathol. 57 (5), 967–973. doi: 10.1111/j.1365-3059.2008.01885.x
Kalaji, H. M., Schansker, G., Ladle, R. J., Goltsev, V., Bosa, K., Allakhverdiev, S., II, et al. (2014). Frequently asked questions about in vivo chlorophyll fluorescence: Practical issues. Photosyn. Res. 122 (2), 121–158. doi: 10.1007/s11120-014-0024-6
Karchani-Balma, S., Gautier, A., Raies, A., Fournier, E. (2008). Geography, plants, and growing systems shape the genetic structure of Tunisian Botrytis cinerea populations. Phytopathology 98 (12), 1271–1279. doi: 10.1094/PHYTO-98-12-1271
Kerssies, A., Bosker-van Zessen, A., II, Wagemakers, C. A. M., Van Kan, J. A. L. (1997). Variation in pathogenicity and DNA polymorphism among Botrytis cinerea isolates sampled inside and outside a glasshouse. Plant Dis. 81 (7), 781–786. doi: 10.1094/PDIS.1997.81.7.781
Khazaeli, P., Zamanizadeh, H., Morid, B., Bayat, H. (2010). Morphological and Molecular Identification of Botrytis Cinerea Causal Agent of Gray Mold in Rose Greenhouses in Centeral Regions of Iran. Int. J. Agric. Sci. Res. 1 (1), 20–24.
Kretschmer, M., Hahn, M. (2008). Fungicide resistance and genetic diversity of Botrytis cinerea isolates from a vineyard in Germany. J. Plant Dis. Prot. 115 (5), 214–219. doi: 10.1007/bf03356266
Kumari, S., Tayal, P., Sharma, E., Kapoor, R. (2014). Analyses of genetic and pathogenic variability among Botrytis cinerea isolates. Microbiol. Res. 169 (11), 862–872. doi: 10.1016/j.micres.2014.02.012
Kunz, C., Vandelle, E., Rolland, S., Poinssot, B., Bruel, C., Cimerman, A., et al. (2006). Characterization of a new, nonpathogenic mutant of Botrytis cinerea with impaired plant colonization capacity. New Phytol. 170 (3), 537–550. doi: 10.1111/j.1469-8137.2006.01682.x
Kuzmanovska, B., Rusevski, R., Jankuloski, L., Jankulovska, M., Ivic, D., Bandzo, K. (2012). Phenotypic and genetic characterization of Botrytis cinerea isolates from tomato. Genetika 44 (3), 633–647. doi: 10.2298/GENSR1203663K
Landi, M., Tattini, M., Gould, K. S. (2015). Multiple functional roles of anthocyanins in plant-environment interactions. Environ. Exp. Bot. 119, 4–17. doi: 10.1016/j.envexpbot.2015.05.012
Mahlein, A.-K. (2016). Present and Future Trends in Plant Disease Detection. Plant Dis. 100 (2), 1–11. doi: 10.1007/s13398-014-0173-7.2
Martinez, F., Blancard, D., Lecomte, P., Levis, C., Dubos, B., Fermaud, M. (2003). Phenotypic differences between vacuma and transposa subpopulations of Botrytis cinerea. Eur. J. Plant Pathol. 109 (5), 479–488. doi: 10.1023/A:1024222206991
Martinez, F., Dubos, B., Fermaud, M. (2005). The role of saprotrophy and virulence in the population dynamics of Botrytis cinerea in vineyards. Phytopathology 95 (6), 692–700. doi: 10.1094/PHYTO-95-0692
Martinez, F., Corio-Costet, M. F., Levis, C., Coarer, M., Fermaud, M. (2008). New PCR primers applied to characterize distribution of Botrytis cinerea populations in french vineyards. Vitis - J. Grapevine Res. 47 (4), 217–226. doi: 10.5073/vitis.2008.47.217-226
Mazar, M., Sahnoun, M., Bettayeb, B., Klement, N. (2018). Optimization of Robotized Tasks for the UV-C Treatment of Diseases in Horticulture. Int. Conf. Afr. Fed. Oper. Res. Soc. 1–4.
Meng, L., Höfte, M., Van Labeke, M.-C. (2019). Leaf age and light quality influence the basal resistance against Botrytis cinerea in strawberry leaves. Environ. Exp. Bot. 157, 35–45. doi: 10.1016/j.envexpbot.2018.09.025
Michailides, T. J., Elmer, P. A. G. (2000). Botrytis gray mold of Kiwifruit caused by Botrytis cinerea in the United States and New Zealand. Plant Dis. 84 (3), 208–223. doi: 10.1094/PDIS.2000.84.3.208
Mirzaei, S., Mohammadi Goltapeh, E., Shams-Bakhsh, M., Safaie, N., Chaichi, M. (2009). Genetic and phenotypic diversity among botrytis cinerea isolates in Iran. J. Phytopathol. 157 (7–8), 474–482. doi: 10.1111/j.1439-0434.2008.01518.x
Muñoz, G., Hinrichsen, P., Brygoo, Y., Giraud, T. (2002). Genetic characterisation of Botrytis cinerea populations in Chile. Mycol. Res. 106 (5), 594–601. doi: 10.1017/S0953756202005981
Okamoto, K., Yanagi, T., Takita, S., Tanaka, M., Higuchi, T., Ushida, Y., et al. (1996). Development of plant growth apparatus using blue and red LED as artificial light source. Acta Hortic. 440, 111–116. doi: 10.17660/ActaHortic.1996.440.20
Pande, S., Galloway, J., Gaur, P. M., Siddique, K. H. M., Tripathi, H. S., Taylor, P., et al. (2006). Botrytis grey mould of chickpea: A review of biology, epidemiology, and disease management. Aust. J. Agric. Res. 57 (11), 1137–1150. doi: 10.1071/AR06120
Pande, S., Sharma, M., Kishore, G. K., Shivram, L., Mangala, U. N. (2010). Characterization of Botrytis cinerea isolates from chickpea: DNA polymorphisms, cultural, morphological and virulence characteristics. Afr. J. Biotechnol. 9 (46), 7961–7967. doi: 10.5897/ajb10.1361
Petrasch, S., Knapp, S. J., van Kan, J. A. L., Blanco-Ulate, B. (2019). Grey mould of strawberry, a devastating disease caused by the ubiquitous necrotrophic fungal pathogen Botrytis cinerea. Mol. Plant Pathol. 20 (6), 877–892. doi: 10.1111/mpp.12794
Quidde, T., Osbourn, A. E., Tudzynski, P. (1998). Detoxification of α-tomatine by Botrytis cinerea. Physiol. Mol. Plant Pathol. 52 (3), 151–165. doi: 10.1006/pmpp.1998.0142
Reignault, P., Mercier, M., Bompeix, G., Boccara, M. (1994). Pectin methylesterase from Botrytis cinerea: Physiological, biochemical and immunochemical studies. Microbiology 140 (12), 3249–3255. doi: 10.1099/13500872-140-12-3249
Rodriguez-Romero, J., Hedtke, M., Kastner, C., Uller, S., Fischer, R. (2010). Fungi, Hidden in Soil or Up in the Air: Light Makes a Difference. Annu. Rev. Microbiol. 64, 585–610. doi: 10.1146/annurev.micro.112408.134000
Rolfe, S. A., Scholes, J. D. (2010). Chlorophyll fluorescence imaging of plant-pathogen interactions. Protoplasma 247 (3), 163–175. doi: 10.1007/s00709-010-0203-z
Schumacher, J. (2012). Tools for Botrytis cinerea: New expression vectors make the gray mold fungus more accessible to cell biology approaches. Fungal Genet. Biol. 49 (6), 483–497. doi: 10.1016/j.fgb.2012.03.005
Schumacher, J. (2017). How light affects the life of Botrytis. Fungal Genet. Biol. 106, 26–41. doi: 10.1016/j.fgb.2017.06.002
Sharma, E., Kapoor, R. (2017). Insights into the molecular interplay of virulence factors in Botrytis cinerea. Australas. Plant Pathol. 46 (6), 551–561. doi: 10.1007/s13313-017-0519-7
Stewart, T. M., Long, P. G. (1987). Sporulation of Botrytis cinerea in the dark. New Z. J. Exp. Agric. 15 (3), 389–392. doi: 10.1080/03015521.1987.10425587
Sun, G., Feng, C., Zhang, A., Zhang, Y., Chang, D., Wang, Y., et al. (2019). The dual role of oxalic acid on the resistance of tomato against Botrytis cinerea. World J. Microbiol. Biotechnol. 35 (2), 1–7. doi: 10.1007/s11274-019-2603-3
Tan, K. K., Epton, H. A. S. (1973). Effect of light on the growth and sporulation of Botrytis cinerea. Trans. Br. Mycol. Soc. 61 (1), 145–157. doi: 10.1016/S0007-1536(73)80096-8
Tan, B. K. K. (1975). Interaction of near-ultraviolet, blue, red, and far-red light in sporulation of Botrytis cinerea. Trans. Br. Mycol. Soc. 64 (2), 215–222. doi: 10.1016/S0007-1536(75)80105-7
Thompson, J. R., Latorre, B. A. (1999). Characterization of Botrytis cinerea from table grapes in Chile using RAPD-PCR. Plant Dis. 83 (12), 1090–1094. doi: 10.1094/PDIS.1999.83.12.1090
Keywords: gray mold, phenotypical variability, pathogenicity, strawberry, red light, image-based early detection
Citation: Meng L, Mestdagh H, Ameye M, Audenaert K, Höfte M and Van Labeke M-C (2020) Phenotypic Variation of Botrytis cinerea Isolates Is Influenced by Spectral Light Quality. Front. Plant Sci. 11:1233. doi: 10.3389/fpls.2020.01233
Received: 30 April 2020; Accepted: 28 July 2020;
Published: 13 August 2020.
Edited by:
Lucia Guidi, University of Pisa, ItalyReviewed by:
Marco Landi, University of Pisa, ItalyMario Serrano, National Autonomous University of Mexico, Mexico
Copyright © 2020 Meng, Mestdagh, Ameye, Audenaert, Höfte and Van Labeke. This is an open-access article distributed under the terms of the Creative Commons Attribution License (CC BY). The use, distribution or reproduction in other forums is permitted, provided the original author(s) and the copyright owner(s) are credited and that the original publication in this journal is cited, in accordance with accepted academic practice. No use, distribution or reproduction is permitted which does not comply with these terms.
*Correspondence: Marie-Christine Van Labeke, TWFyaWVDaHJpc3RpbmUuVmFuTGFiZWtlQFVHZW50LmJl