- 1Faculty of Agriculture, Iwate University, Morioka, Japan
- 2Graduate School of Agricultural Science, Kobe University, Nada-ku, Japan
- 3Graduate School of Agricultural Science, Tohoku University, Aoba-ku, Japan
Photorespiration coupled with CO2 assimilation is thought to act as a defense system against photoinhibition caused by osmotic stress. In the present study, we examined whether such a mechanism is operative for the protection of photosystem I (PSI) in rice (Oryza sativa L.) including transgenic plants with decreased and increased Rubisco content (RBCS-antisense and RBCS-sense plants, respectively). All plants were hydroponically grown and moderate osmotic stress was imposed using hydroponic culture solutions containing poly(ethylene glycol) (PEG) at 16% or 20% (w/v) for 2 d. In wild-type plants, the rates of CO2 assimilation (A) were significantly decreased by the PEG treatment, whereas the photorespiration activity estimated from the rates of electron transport in photosystem II (PSII) and A were not affected. The maximal quantum efficiency of PSII (Fv/Fm) and the maximal activity of PSI (Pm) were also not affected. In RBCS-antisense plants, A and the estimated photorespiration activity were considerably lower than those in wild-type plants in the presence or absence of the PEG treatment. Pm and both Fv/Fm and Pm decreased in the 16% PEG-treated and 20% PEG-treated RBCS-antisense plants, respectively. Thus, the decrease in Rubisco content led to the photoinhibition of PSI and PSII, indicating the importance of photorespiration coupled with CO2 assimilation for the protection of PSI from moderate PEG-induced osmotic stress. It was also shown that PSI was more sensitive to osmotic stress than PSII. In the PEG-treated wild-type and RBCS-antisense plants, osmotic-stress responses of the photosynthetic electron transport reactions upstream of PSI led to the oxidation of P700, which is thought to prevent PSI from over-reduction. Although such a defense system operated, it was not sufficient for the protection of PSI in RBCS-antisense plants. In addition, there were no large differences in the parameters measured between wild-type and RBCS-sense plants, as overproduction of Rubisco did not increase photorespiration activity.
Introduction
Drought stress is one of the most harmful environmental stresses on plant productivity. Stomatal closure in response to drought stress prevents water loss via transpiration but decreases CO2 availability within a leaf and energy consumption by the Calvin-Benson cycle (Lawlor and Tezara, 2009). The resulting excess light energy can over-reduce the photosynthetic electron transport (PET) chain (Cruz de Carvalho, 2008; Xu et al., 2010) and generate reactive oxygen species (ROS) around photosystem II and I (PSII and PSI, respectively) (Asada, 1999; Müller et al., 2001; Krieger-Liszkay, 2005), leading to photoinhibition of these photosystems. PSI photoinhibition requires a long period of recovery and severely decreases photosynthesis and plant growth (Kudoh and Sonoike, 2002; Sonoike, 2011), whereas PSII photoinhibition is repaired efficiently in a short period of time (Demmig-Adams and Adams, 1992; Murata et al., 2007). It has been observed that PSI suffered from photoinhibition under severe drought stress, whereas PSII was not largely affected in some tropical tree species (Huang et al., 2013). Similar phenomena were observed when rice plants were subjected PEG-induced osmotic stress, which are widely used to mimic drought stress (Wada et al., 2019). These results show that PSI is more sensitive to drought or osmotic stress than PSII. Therefore, PSI photoinhibition would be harmful under such stress conditions.
It has been reported that the PET reactions responded to drought or osmotic stress in a manner that limits the electron flow toward PSI. Such responses include the non-photochemical quenching (NPQ) of light energy at PSII (Golding and Johnson, 2003; Zhou et al., 2007; Lawlor and Tezara, 2009; Huang et al., 2012; Zivcak et al., 2013; Zivcak et al., 2014; Wada et al., 2019) and limitation of the electron flow at the cytochrome b6/f complex (Kohzuma et al., 2009). These events were accompanied by the oxidation of the reaction center chlorophyll of PSI, P700 (Golding and Johnson, 2003; Huang et al., 2012; Zivcak et al., 2013; Zivcak et al., 2014; Wada et al., 2019), which was suggested to suppress the production of ROS in PSI (Sejima et al., 2014; Takagi et al., 2017a). These results strongly suggest that these drought- or osmotic-stress responses of the PET reactions protect PSI from over-reduction and photoinhibition by ROS.
In addition to these responses of the PET reactions, processes downstream of PSI can also contribute to the protection of PSI under drought stress. One such process is photorespiration, a large and energy-consuming pathway that salvages byproducts of the reaction of Rubisco in the Calvin-Benson cycle (Ogren, 1984). Rubisco catalyzes not only the carboxylation of ribulose 1,5-bisphosphate, which generates two molecules of 3-phosphoglycerate for CO2 assimilation, but also its oxygenation, which generates one molecule each of 2-phosphoglycolate and 3-phosphoglycerate. The photorespiratory pathway converts 2-phosphoglycolate to 3-phosphoglycerate while consuming reducing equivalents and ATP. Rubisco oxygenase activity and photorespiration are relatively active under CO2-limited conditions according to the C3 photosynthesis model of Farquhar and co-workers (Farquhar et al., 1980; von Caemmerer, 2000). It was suggested that the rate of CO2 and O2 uptake by carboxylation and oxygenation reactions, respectively, is at the ratio of 1:2 under the CO2 compensation point, and that the Calvin-Benson cycle and the photorespiratory pathway operate in a balanced state. Photorespiration was estimated to consume a large portion of light energy under such conditions (Sejima et al., 2016; Hanawa et al., 2017). The rates of energy consumption by photorespiration were reported to increase in response to drought or osmotic stress (Cornic and Briantais, 1991; Wingler et al., 1999; Haupt-Herting and Fock, 2002; Galmés et al., 2007; Zivcak et al., 2013; Chastain et al., 2014; Wada et al., 2019). It was also found that drought-stress induced NPQ, and that NPQ was further stimulated in barley mutants with decreased activity of a photorespiratory enzyme, suggesting that photorespiration consumes excess light energy under drought stress (Wingler et al., 1999).
However, it remains unclear whether photorespiration coupled with CO2 assimilation protects PSI under drought or osmotic stress. In the present study, this was explored in transgenic rice (Oryza sativa L.) plants with decreased Rubisco content (RBCS-antisense plants; Makino et al., 2000). We have recently reported that the PET chain was over-reduced in RBCS-antisense plants under the combination of high irradiance and CO2-compensated conditions (Wada et al., 2018). PSI also became susceptible to excess light energy imposed by repetitive illumination of saturated pulse-light, which is thought to generate ROS in PSI (Sejima et al., 2014; Zivcak et al., 2015). Transgenic rice plants with increased Rubisco content (RBCS-8sense plants; Suzuki et al., 2007) were also used as control plants. We have previously observed that the activities of photorespiration and CO2 assimilation were not substantially enhanced in RBCS-sense plants (Makino and Sage, 2007; Suzuki et al., 2007; Suzuki et al., 2009; Wada et al., 2018). Plants were exposed to moderate osmotic-stress treatments using poly(ethylene glycol) (PEG)-containing culture solutions. The maximal quantum efficiency of PSII (Fv/Fm) and the maximal P700 signal of PSI (Pm) were determined as indices of photoinhibition and are discussed in relation to the activities of photorespiration and CO2 assimilation. In addition, osmotic-stress responses of the PET reactions were also examined by measuring chlorophyll fluorescence and P700 absorbance and its relationship with the activities of photorespiration and CO2 assimilation are discussed.
Materials and Methods
Plant Culture
Rice (Oryza sativa L. “Notohikari”) plants were used as wild-type plants and the background cultivar for the previously generated Rubisco-transgenic plants. T4 progenies of RBCS-antisense plants (line AS-71; Makino et al., 2000) and BC2 progenies of RBCS-sense plants (line Sr-26-8; Suzuki et al., 2007) were used. Each plant was grown hydroponically in a growth chamber (NC-441HC, NKsystem, Osaka, Japan) operated under the conditions of photon flux density of 400–500 μmol photon m−2 s−1, a photoperiod of 14 h, and day/night temperature regime of 27/22°C. Pre-soaked seeds were sown and germinated on a net floating on tap water, whose pH was adjusted to 5.3–5.5 with 1 M HCl. After 2 weeks, seedlings were transplanted into 1.1 L plastic pots filled with the culture solution. The composition of the culture solution is described in Makino et al. (1988). The culture solution was renewed once a week. The concentration of the culture solution was increased depending on plant growth.
Osmotic-Stress Treatments Using PEG
Plants grown for approximately 60 d after sowing were subjected to osmotic stress treatments using PEG with an average molecular weight of 6,000 (PEG, Sigma-Aldrich, St. Louis, MO, USA). The culture solution containing PEG at the concentration of 16 or 20% (w/v) was supplied instead of the regular culture solution for 2 d in the growth chamber described above. After the treatments, the uppermost, fully expanded leaves were used for the measurement of photosynthesis and biochemical assays.
Measurements of Photosynthesis
The rate of CO2 assimilation (A), chlorophyll fluorescence, and P700 absorbance were simultaneously measured using the combination system of GFS-3000 and DUAL-PAM-100 (Heinz Walz GmbH, Effeltrich, Germany). The detailed conditions are described in Wada et al. (2019). Briefly, Fv/Fm and Pm were measured after the leaves were dark-adapted, followed by the measurements of chlorophyll fluorescence and P700 absorbance under the conditions of an actinic light intensity of 1,200 μmol photon m−2 s−1, an ambient CO2 partial pressure of 40 Pa, a leaf temperature of 27°C, and a relative humidity of 60–70%. The quantum efficiency of PSII [Y(II)], the quantum yields of the NPQ [Y(NPQ)] and of the non-regulated and non-photochemical energy dissipation [Y(NO)], and the index for the reduction of the primary plastoquinone electron acceptor in PSII (QA) (1-qL) were calculated following the methods described by Kramer et al. (2004) and Baker (2008). Three complementary quantum yields were defined: Y(II) + Y(NO) + Y(NPQ) = 1. The rate of electron transport in PSII (ETRII) was calculated as Y(II) × photon flux density × α × 0.5. The absorptance (α) was adopted to be 0.84 in this study. The quantum efficiency of PSI [Y(I)] and the quantum yields of the donor side limitation of PSI [Y(ND)] and of the acceptor side limitation of PSI [Y(NA)] were calculated according to the methods described by Klughammer and Schreiber (1994) and Schreiber and Klughammer (2008). Three complementary quantum yields were defined: Y(I) + Y(NA) + Y(ND) = 1. The rate of electron flow donated for photorespiration (JPR) was evaluated using the equation of JPR = 2/3 × [ETRII – 4 (A + Rd)] (Valentini et al., 1995; Zivcak et al., 2013). Rd was the rate of respiration under illumination and was assumed to be 1 μmol m−2 s−1 as in our previous study (Suzuki et al., 2007).
Measurements of the Relative Water Content of Leaves
The relative water content of the leaves (RWC) was determined after the stress treatment, following the methods of Zhou et al. (2007), as described in Wada et al. (2019), using leaf fresh weight measured just after the stress treatment, leaf weight after overnight immersion in deionized water at 4°C, and leaf dry weight.
Biochemical Assays
Leaves were collected after the measurement of photosynthesis, frozen using liquid nitrogen, and kept at −80°C until use. Total leaf-N, chlorophyll, and Rubisco content were determined as described in Makino et al. (1997). Briefly, total leaf-N content was determined using Nessler’s reagent after Kjeldahl digestion. Arnon’s method (Arnon, 1949) was used for chlorophyll determination. Rubisco content was determined by formamide extraction of Coomassie Brilliant Blue R-250-stained bands corresponding to the large and small subunits of Rubisco separated by SDS-PAGE (Makino et al., 1985), except that bovine serum albumin was used to prepare the calibration curves.
Statistical Analysis
Three to five biological replicates were analyzed using the Tukey-Kramer’s HSD test using JMP 14 (SAS Institute Japan, Tokyo, Japan). The Pearson correlation coefficients of the measured parameters were calculated using Microsoft Excel 2013.
Results
Table 1 shows the amounts of Rubisco protein, chlorophyll, and total leaf-N in leaves of the PEG-untreated wild-type, RBCS-sense, and RBCS-antisense plants. The amounts of Rubisco in RBCS-sense and RBCS-antisense plants were 120% and 43%, respectively, of the levels in the wild-type plants. The amount of chlorophyll in the RBCS-sense plants tended to be slightly lower than that in wild-type plants, whereas the amount of total leaf-N was not different. In RBCS-antisense plants, the amounts of chlorophyll and total leaf-N were lower than those in wild-type plants. Such trend was also observed previously (Makino and Sage, 2007; Suganami et al., 2018; Wada et al., 2018). The magnitude of these changes was smaller than that in the amount of Rubisco. Thus, the amounts of Rubisco were greatly affected by genetic manipulation.
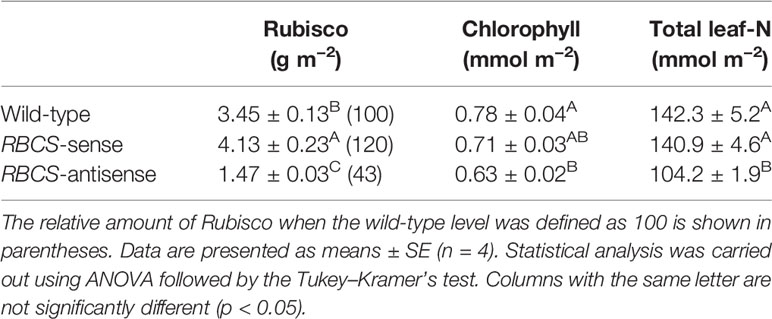
Table 1 Amounts of Rubisco protein, chlorophyll, and total leaf-nitrogen in the uppermost, fully expanded leaves in wild-type, RBCS-sense, and RBCS-antisense rice plants.
The culture solutions containing PEG at concentrations of 16 and 20% (w/v) were used to impose osmotic stress to the plants. We have previously observed that values of the relative water content of leaves of wild-type rice plants only marginally decreased under these PEG treatments (Wada et al., 2019). In the present study, the relative water content of leaves was not significantly affected by the PEG treatments, and did not significantly differ among genotypes (Figure 1).
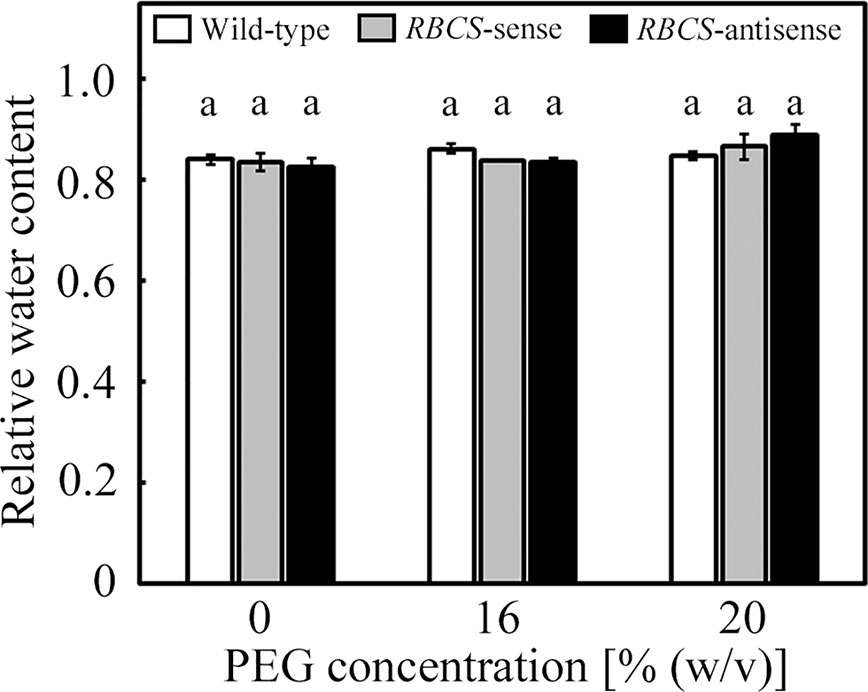
Figure 1 Relative water content of leaves after water stress treatment in transgenic rice plants with an increased (RBCS-sense) or decreased (RBCS-antisense) Rubisco content. Wild-type plants were used as a control. Sixty days after germination, hydroponically grown plants were water-stressed using culture solutions containing PEG at 0, 16, and 20% (w/v) for 2 d under an irradiance of 400–500 μmol photon m−2 s−1 and day/night air-temperatures of 27/22°C, followed by the measurements of relative water content of leaves. White, gray, and black bars denote wild-type, RBCS-sense, and RBCS-antisense plants, respectively. Data are presented as average ± standard error (n = 3–4). Statistical analysis was carried out using ANOVA with a post-hoc Tukey’s HSD test. Columns with the same letter are not significantly different (p < 0.05). Statistically significant differences were not observed.
Effects of the PEG treatments on the fitness of the photosynthetic system were evaluated using Fv/Fm and Pm, which are the indices of the photoinhibition of PSII and PSI, respectively. It has been previously shown that Fv/Fm and Pm were not affected under these PEG treatments in wild-type rice plants. In the PEG-untreated plants, there were no differences in Fv/Fm and Pm between wild-type and RBCS-sense plants (Figure 2). There was no statistical difference between wild-type plants and RBCS-antisense plants, although Fv/Fm and Pm in the latter tended to be marginally lower. Similar trend has been observed in RBCS-antisense plants previously (Hirotsu et al., 2004). Neither Fv/Fm nor Pm changed in the PEG-treated wild-type and RBCS-sense plants, indicating that these genotypes did not suffer from the photoinhibition of PSII or PSI. In contrast, Fv/Fm substantially decreased to 0.59 in the 20% PEG-treated RBCS-antisense plants (Figure 2A). Pm in the 16% PEG-treated plants decreased to 78% of the level of the PEG-untreated RBCS-antisense plants, and further decreased to 51% in the 20% PEG-treated plants (Figure 2B). These results indicate that PSI and both PSII and PSI underwent photoinhibition in RBCS-antisense plants under the 16%- and 20%-PEG treatments, respectively. It is also indicated that PSI in RBCS-antisense plants was more sensitive to the PEG treatments than PSII.
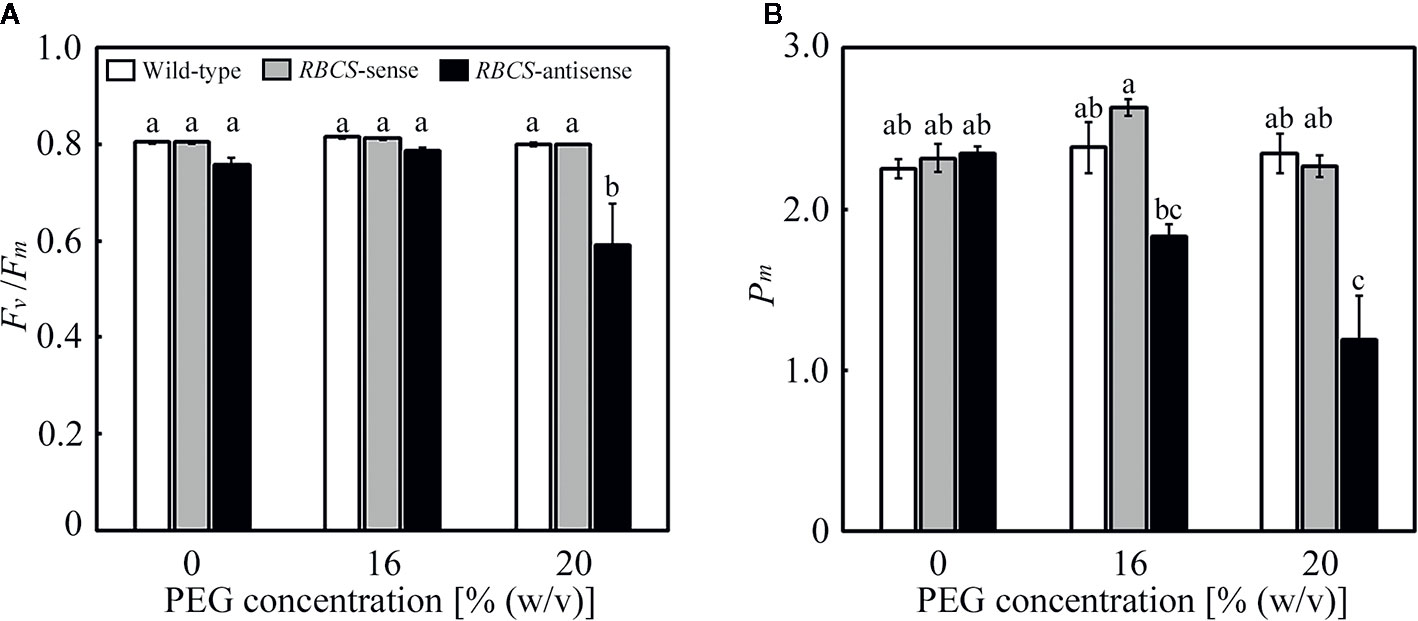
Figure 2 Maximum quantum efficiency of PSII photochemistry (Fv/Fm) (A) and the maximal P700 signal (Pm) (B) after water stress treatment in transgenic rice plants with an increased (RBCS-sense) or decreased (RBCS-antisense) Rubisco content. Wild-type plants were used as a control. Sixty days after germination, hydroponically grown plants were water-stressed using culture solutions containing PEG at 0, 16, and 20% (w/v) for 2 d under an irradiance of 400–500 μmol photon m−2 s−1 and day/night air-temperatures of 27/22°C, followed by the measurements of Fv/Fm and Pm. White, gray, and black bars denote wild-type, RBCS-sense, and RBCS-antisense plants, respectively. Data are presented as average ± standard error (n = 4–5). Statistical analysis was carried out using ANOVA with a post-hoc Tukey’s HSD test. Columns with the same letter are not significantly different (p < 0.05).
Changes in leaf gas-exchange parameters were examined (Figure 3). In all genotypes, A, stomatal conductance (gs), and intercellular CO2 partial pressure (pCi) tended to decrease in the PEG-treated plants. Although the relative water content in leaves was not affected (Figure 1), the PEG treatment was shown to lead to partial stomatal closure and concomitant changes in the leaf gas-exchange parameters. In wild-type plants, A in the PEG-treated plants decreased to 41–53% of the levels in the PEG-untreated control plants (Figure 3A). Similar trends were observed in gs. The values of pCi decreased by more than 30 ppm in the PEG-treated wild-type plants. The decreases in pCi were not as much as the decrease in both A and gs (Figure 2C; Lawlor and Tezara, 2009). The values of A, gs, and pCi in RBCS-sense plants were not largely different from those in wild-type plants irrespective of (PEG) in the culture solutions, although slight decreases in gs or pCi were observed in some cases (Figures 3A–C). In contrast, A in RBCS-antisense plants was lower than in other genotypes (Figure 3A). When not treated with PEG, A was 41% that of the wild-type level, corresponding to the magnitude of decreases in the amount of Rubisco (Table 1). Decreases in A were primarily accounted for by decreases in Rubisco content as observed in our previous studies (Hirotsu et al., 2004; Makino and Sage, 2007; Suganami et al., 2018; Wada et al., 2018). Therefore, it was unlikely that RBCS-antisense plants were suffering from PSII photoinhibition that affected A despite of decreases in chlorophyll content and marginal decreases in Fv/Fm (Table 1 and Figure 2). Although the level of gs was lower than that in the wild-type plants (Figure 3B), pCi was higher by 44 ppm owing to the greatly decreased A (Figure 3C). In the 16% and 20% PEG-treated RBCS-antisense plants, the values of A were 76% and 28%, respectively, that of the PEG-untreated RBCS-antisense plants. These values were 59% and 28% of those in the wild-type plants treated with the same (PEG), respectively. As gs decreased in the PEG-treated RBCS-antisense plants (Figure 3B), the values of pCi decreased by 12 and 58 ppm in the 16% and 20% PEG-treated plants, respectively. The pCi in the PEG-treated RBCS-antisense plants was still higher than that in the wild-type plants treated with the same (PEG) (Figure 3C).
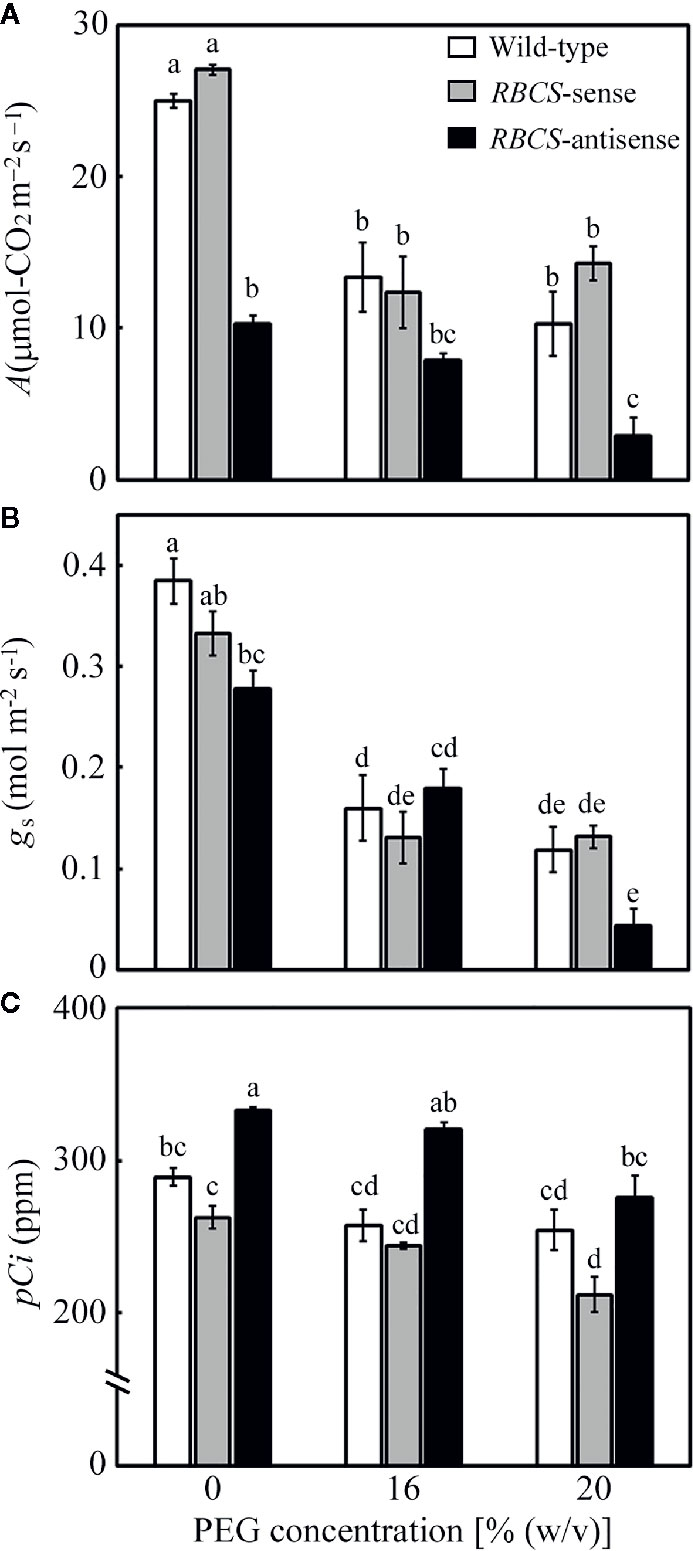
Figure 3 Rate of CO2 assimilation (A) (A), stomatal conductance (gs) (B), and intercellular CO2 partial pressure (pCi) (C) after water stress treatment in transgenic rice plants with an increased (RBCS-sense) or decreased (RBCS-antisense) Rubisco content. Wild-type plants were used as a control. Sixty days after germination, hydroponically grown plants were water-stressed using culture solutions containing PEG at 0, 16, and 20% (w/v) for 2 d under an irradiance of 400–500 μmol photon m−2 s−1 and day/night air-temperatures of 27/22°C. A, gs, and pCi were measured under the conditions of an actinic light intensity of 1,200 μmol photon m−2 s−1, an ambient CO2 partial pressure of 40 Pa, leaf temperature of 27°C, and relative humidity of 60–70%. White, gray, and black bars denote wild-type, RBCS-sense, and RBCS-antisense plants, respectively. Data are presented as average ± standard error (n = 4–5). Statistical analysis was carried out using ANOVA followed by the Tukey–Kramer’s test. Columns with the same letter are not significantly different (p < 0.05).
The consumption of electrons by photorespiration, JPR, was calculated from A and ETRII (Valentini et al., 1995; Zivcak et al., 2013). The values of JPR in the PEG-untreated wild-type plants and RBCS-sense plants were similar and did not change when treated by PEG (Figure 4A). In these genotypes, ratios of JPR to ETRII were about 0.35 when not treated with PEG and tended to increase to 0.42–0.46 when treated with PEG (Figure 4B), indicating that the rate of consumption of electrons by photorespiration increased. JPR/ETRII was less than 0.5, showing that CO2 assimilation acted as a relatively greater electron sink, probably because stomata were still partially open and pCi was not greatly decreased under the present experimental conditions (Figures 3B, C). JPR in RBCS-antisense plants was 41% of that in wild-type plants when not treated with PEG (Figure 4A). The magnitude of decreases in JPR was similar to that in the amount of Rubisco (Table 1), as observed in the case of A (Figure 3A). JPR further decreased in the 16% and 20% PEG treated RBCS-antisense plants. The values of JPR in these plants corresponded to 34% and 20% of those in the wild-type plants treated with the same (PEG), respectively. These results show that the consumption of electrons by photorespiration and CO2 assimilation was greatly restricted owing to the decreased Rubisco content in RBCS-antisense plants. Ratios of JPR to ETRII in RBCS-antisense plants were similar to those in wild-type plants when not treated with PEG (Figure 4B). In contrast to other genotypes, ratios of JPR to ETRII in RBCS-antisense plants were relatively unchanged when treated with PEG, showing that the rate of consumption of electrons by photorespiration did not change.
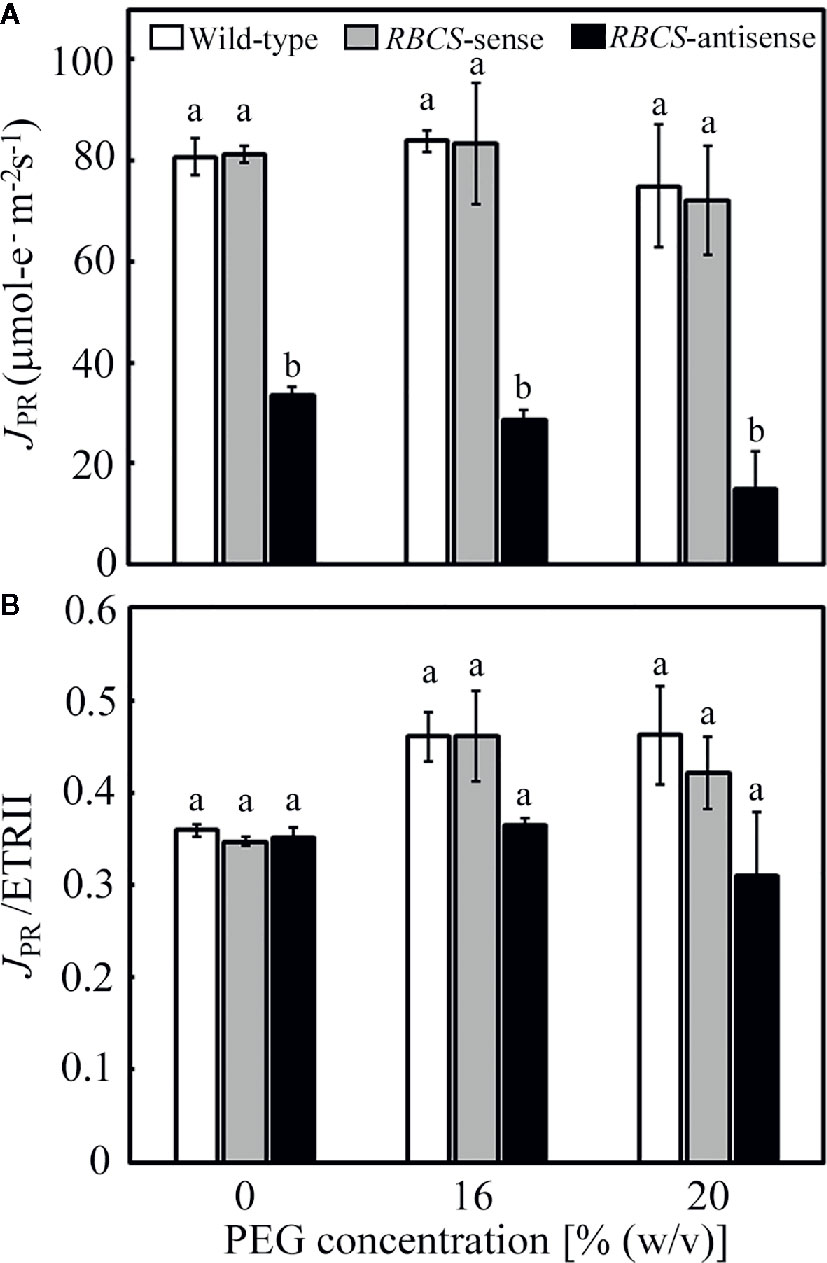
Figure 4 Rate of electron flow donated for photorespiration (JPR) (A) and its ratio to the electron transport rate in PSII (JPR/ETRII) (B) in transgenic rice plants with an increased (RBCS-sense) or decreased (RBCS-antisense) Rubisco content. Wild-type plants were used as a control. JPR was evaluated with the equation JPR = 2/3 × [ETRII – 4 (A + Rd)] (Valentini et al., 1995; Zivcak et al., 2013). Rd was the rate of respiration under illumination and was assumed to be 1 μmol m−2 s−1 as in our previous study (Suzuki et al., 2007). ETRII was calculated as Y(II) × PFD × α × 0.5, where absorptance (α) was adopted to be 0.84. Data of A and Y(II) were taken from Figures 3 and 5, respectively. White, gray, and black bars denote wild-type, RBCS-sense, and RBCS-antisense plants, respectively. Data are presented as average ± standard error (n = 4–5). Statistical analysis was carried out using ANOVA followed by the Tukey–Kramer’s test. Columns with the same letter are not significantly different (p < 0.05).
Changes in the photochemistry of PSII were examined in response to the PEG treatments. In wild-type plants, Y(II) decreased slightly and gradually as the (PEG) in the culture solution increased (Figure 5A). The magnitude of the decreases was smaller than that in A (Figure 3A). Slight decreases in Y(NO), which is an index for the dissipation of light energy in a non-regulated manner (Kramer et al., 2004), were also observed (Figure 5C). These changes were reflected in increases in Y(NPQ) (Figure 5B). The 1−qL indicates the fraction of PSII centers in closed states (Kramer et al., 2004), which is thought to reflect the extent of the reduction of the plastoquinone pool (Miyake et al., 2009). The 1−qL is also thought to be an index for lumenal acidification, as a decrease in qL was accompanied by lumenal acidification in transgenic or transplastomic tobacco plants with decreases in the amounts of the chloroplastic ATP synthase (Rott et al., 2011). The values of 1−qL tended to slightly increase in the PEG-treated wild-type plants (Figure 5D), suggesting the reduction of the plastoquinone pool and/or lumenal acidification. In RBCS-sense plants, the values of these parameters and their response to the PEG treatments were similar to those in wild-type plants (Figures 5A–D). In RBCS-antisense plants, Y(II) decreased to 43% of that in wild-type plants when not treated with PEG, while slight decreases in Y(NO) were also observed (Figures 5A, C). In contrast, Y(NPQ) increased to 2.2-fold higher than that in the PEG-untreated wild-type plants (Figure 5B), suggesting that light energy that became excessive because of the decrease in Rubisco content was dissipated primarily by NPQ (Hirotsu et al., 2004; Wada et al., 2018). At the same time, the values of 1−qL tended to be higher than in other genotypes (Figure 5D). Y(II) further decreased in the PEG-treated RBCS-antisense plants and was lower than that in the wild-type plants treated with the same (PEG) (Figure 4A). In the 16% PEG-treated plants, a decrease in Y(NO) and an increase in Y(NPQ) were observed as in other genotypes (Figures 5B, C). In the 20% PEG-treated plants, a decrease in Y(II) was not accompanied by an increase in Y(NPQ) but by a substantial increase in Y(NO). Similar phenomena were observed in severely osmotic-stressed rice plants under high temperature (Wada et al., 2019). In addition, the values of 1−qL increased in the PEG-treated RBCS-antisense plants and were higher than those in other genotypes treated with the same (PEG), suggesting that reduction of the plastoquinone pool and/or lumenal acidification was further enhanced.
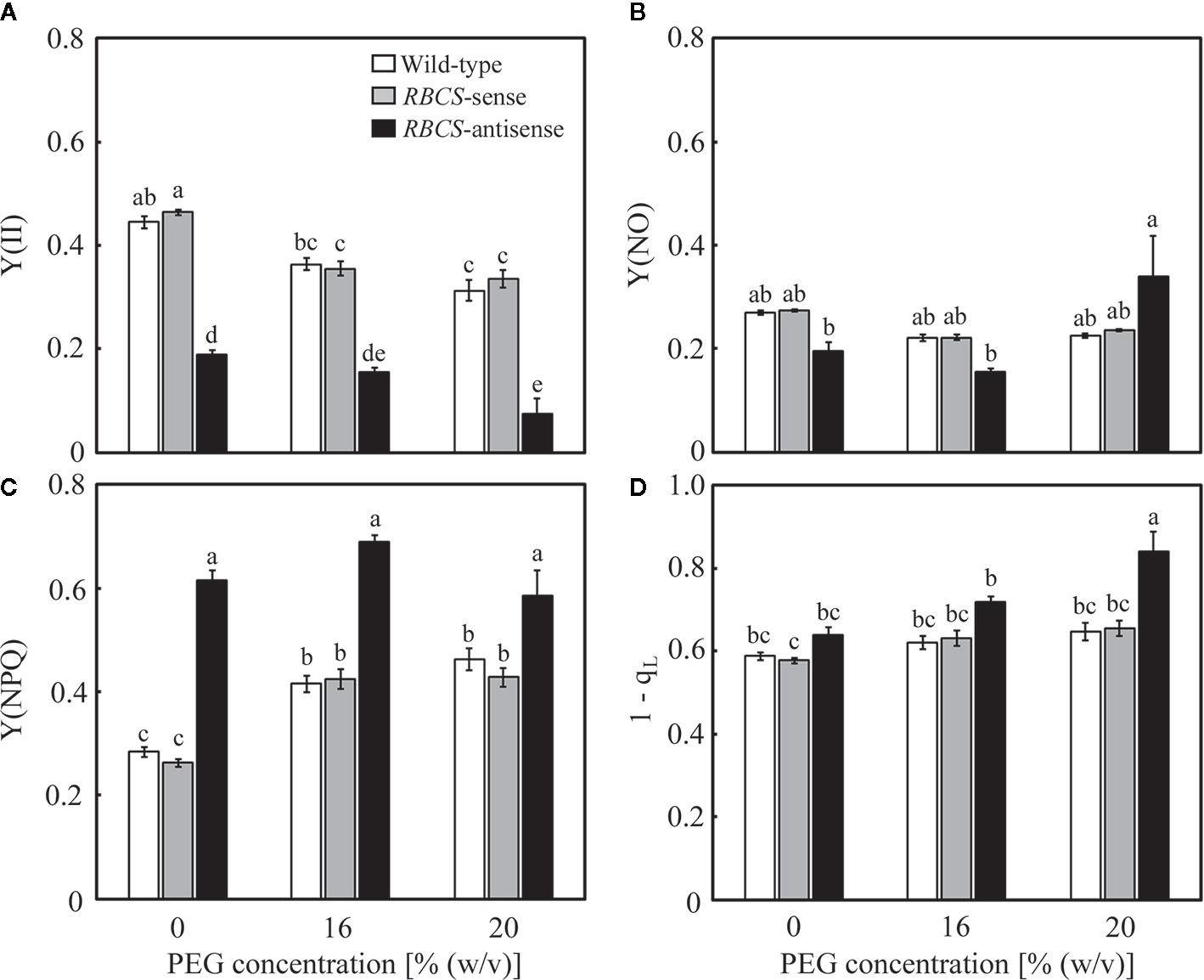
Figure 5 Chlorophyll fluorescence parameters after water stress treatment in transgenic rice plants with an increased (RBCS-sense) or decreased (RBCS-antisense) Rubisco content. Wild-type plants were used as a control. Sixty days after germination, hydroponically grown plants were water-stressed using culture solutions containing PEG at 0, 16, and 20% (w/v) for 2 d under an irradiance of 400–500 μmol photon m−2 s−1 and day/night air-temperatures of 27/22°C. Y(II) (A), Y(NPQ) (B), Y(NO) (C), and 1−qL (D) were measured under the conditions of an actinic light intensity of 1,200 μmol photon m−2 s−1, an ambient CO2 partial pressure of 40 Pa, leaf temperature of 27°C, and relative humidity of 60–70%. Data are presented as means ± SE (n = 4–5). Statistical analysis was carried out using ANOVA followed by the Tukey–Kramer’s test. Columns with the same letter are not significantly different (p < 0.05).
Changes in the photochemistry of PSI were examined simultaneously with those of PSII. In wild-type plants, Y(I) tended to marginally decrease in the PEG-treated plants, while slight decreases were also observed in Y(NA) (Figures 6A, C). These changes were reflected in increases in Y(ND) (Figure 6B), showing that the oxidation of P700 was stimulated by the PEG treatments. In RBCS-sense plants, the values of these parameters and their responses to the PEG treatments were similar to those in wild-type plants (Figures 6A–C). In RBCS-antisense plants, Y(I) and Y(NA) were lower than those in wild-type plants when not treated with PEG (Figures 6A, C). These changes were reflected in increases in Y(ND), being 2.0-fold higher than the level in wild-type plants (Figure 6B). Thus, the oxidation of P700 was stimulated in RBCS-antisense plants without the PEG treatments in the present study, although such a phenomenon was not observed in the previous study (Wada et al., 2018). Y(ND) gradually increased as the (PEG) in the culture solution increased, whereas Y(I) and Y(NA) gradually decreased (Figures 6A–C). Y(ND) in RBCS antisense plants was higher than that in other genotypes when treated with PEG, showing that P700 in RBCS-antisense plants was also in a more oxidized state by the PEG treatments.
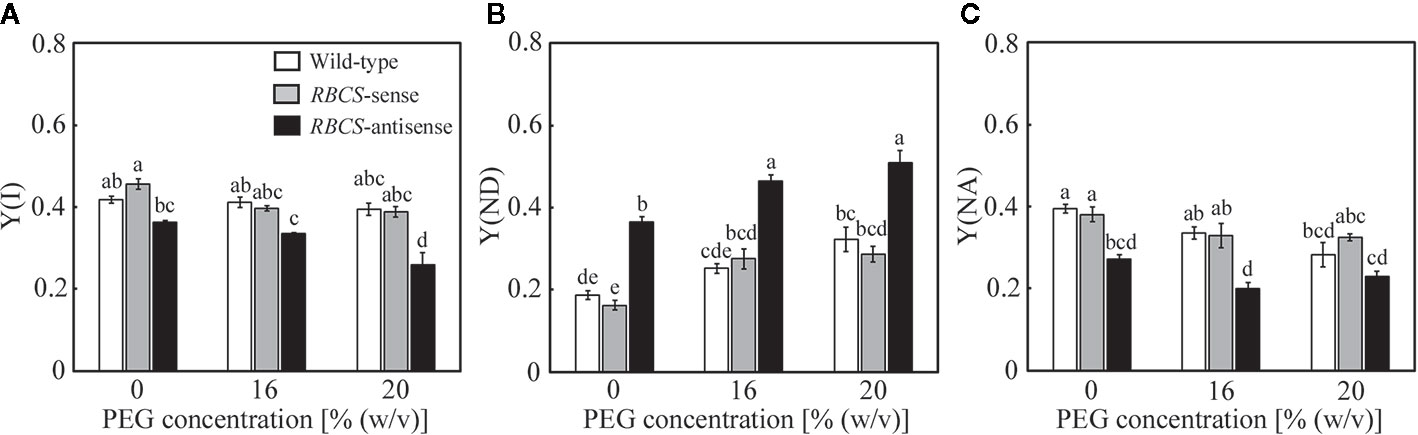
Figure 6 Redox state of P700 after water stress treatment in transgenic rice plants with an increased (RBCS-sense) or decreased (RBCS-antisense) Rubisco content. Wild-type plants were used as a control. Sixty days after germination, hydroponically grown plants were water-stressed using culture solutions containing PEG at 0, 16, and 20% (w/v) for 2 d under an irradiance of 400–500 μmol photon m−2 s−1 and day/night air-temperatures of 27/22°C. Y(I) (A), Y(ND) (B), and Y(NA) (C) were measured under the conditions of an actinic light intensity of 1200 μmol photon m−2 s−1, an ambient CO2 partial pressure of 40 Pa, leaf temperature of 27°C, and relative humidity of 60–70%. Data are presented as means ± SE (n = 4–5). Statistical analysis was carried out using ANOVA followed by the Tukey–Kramer’s test. Columns with the same letter are not significantly different (p < 0.05).
Relationships between the parameters of the PET reactions were analyzed (Table 2). Data obtained with different genotypes were analyzed together. The mutual relationships between the successive PET reactions were as follows: Y(II) was strongly, negatively correlated with 1−qL and Y(NPQ); 1−qL was strongly, negatively correlated with Y(I), which in turn was strongly, negatively correlated with Y(ND). 1−qL was strongly correlated with these parameters. These results are consistent with those in osmotic-stressed rice plants under normal and high temperatures (Wada et al., 2019). However, some differences were observed compared to the results reported in Wada et al. (2019). In the present study, strong, negative correlations were observed between Y(II) and Y(NPQ) and between Y(ND) and Y(NA).
The properties in leaf gas-exchange and the photochemistry of PSII and PSI in the PEG-untreated RBCS-sense and RBCS-antisense plants, and the PEG-treatment response of wild-type plants were basically consistent with those observed in our previous studies (Makino et al., 2000; Hirotsu et al., 2004; Suzuki et al., 2007; Suzuki et al., 2009; Sudo et al., 2014; Wada et al., 2018; Wada et al., 2019).
Discussion
Photorespiration Coupled With CO2 Assimilation Plays a Crucial Role in the Protection of PSI From Photoinhibition Under PEG-Induced Moderate Osmotic Stress
In the present study, we examined the role of photorespiration coupled with CO2 assimilation in the protection of PSI from PEG-induced osmotic stress using Rubisco-transgenic rice plants. The PEG treatments did not significantly affect the relative water content of leaves in all genotypes (Figure 1), but substantially decreased gs (Figure 3B). Stomatal closure is the earliest drought-stress response and was reported to be observed even when water status of plants was unaffected by withdrawal of water (Davies and Zhang, 1991; Chaves et al., 2003). Therefore, moderate osmotic stress was thought to be imposed to the plants by the PEG-treatments used in the present study. In RBCS-antisense plants, decreases in the activities of photorespiration and CO2 assimilation led to photoinhibition of PSI and PSII under moderate osmotic stress that did not lead to photoinhibition of both photosystems in wild-type plants (Figures 2, 3A, and 4A). These results clearly indicate that photorespiration coupled with CO2 assimilation plays a crucial role in the protection of PSI from photoinhibition under moderate osmotic stress conditions. It has been reported that PSI was more sensitive to drought or osmotic stress than PSII (Huang et al., 2013; Wada et al., 2019). Similar trends were observed in RBCS-antisense plants, as PSI suffered from photoinhibition in the 16% PEG-treated plants, whereas PSII did not (Figure 2). These results suggest that the weakness of PSI to osmotic stress can be compensated for by the operation of photorespiration coupled with CO2 assimilation to some extent.
To examine whether photorespiration contributes the consumption of excess light energy under osmotic stress conditions, elevated CO2 condition might be useful as it suppresses photorespiration. However, in the case of RBCS-antisense plants, decrease in Rubisco content affect both CO2 assimilation and photorespiration. A was shown to be limited by Rubisco under elevated CO2 conditions where A is not limited by Rubisco in wild-type plants (Makino et al., 2000; Suzuki et al., 2009). If osmotic-stress response in RBCS-antisense plants was altered under elevated (CO2) conditions, it is very difficult to distinguish whether it sorely depended on the suppression of photorespiration.
In contrast, there were no large differences between wild-type and RBCS-sense plants in terms of the activities of photorespiration and CO2 assimilation, osmotic-stress tolerance, and the photochemistry of PSII and PSI (Figures 2–6). These results are consistent with those in our previous study, in which these genotypes were exposed to the combination of high irradiance and CO2-compensated conditions (Wada et al., 2018). We have suggested that Rubisco was not fully functional in RBCS-sense plants The rate of CO2 assimilation was not increased proportionally with an increase in Rubisco content in RBCS-sense plants, as Rubisco was partially deactivated probably owing to imbalance between the processes of CO2 assimilation (Makino and Sage, 2007; Suzuki et al., 2007; Suzuki et al., 2009). The same problem probably arose in the present study.
Photorespiration Is Possibly Inhibited in RBCS-Antisense Plants Under PEG-Induced Osmotic Stress
It has previously been observed that the absolute and/or relative rates of energy consumption by photorespiration increased under drought or osmotic stress in a number of plant species, including rice (Cornic and Briantais, 1991; Haupt-Herting and Fock, 2002; Galmés et al., 2007; Zivcak et al., 2013; Chastain et al., 2014; Wada et al., 2019), accounting for the substantial part of light energy absorbed by leaves. Therefore, it has been suggested that photorespiration plays a role in the consumption of excess light energy, at least in part, under drought or osmotic stress condition. Similar trends were observed in wild-type plants in the present study. Moderate osmotic stress by the PEG treatments tended to increase JPR/ETRII simultaneously with decreases in pCi (Figures 3C and 4B), although the magnitude of the increases were relatively small. However, such trends were not observed in RBCS-antisense plants even when pCi decreased in the PEG-treated plants. These results could mean that photorespiration was inhibited in RBCS-antisense plants under osmotic stress. Our previous report also suggested the inhibition of photorespiration when rice plants were severely osmotic-stressed under high temperature conditions (Wada et al., 2019). These results imply that some processes of the photorespiratory pathway were damaged when excess light energy caused by osmotic stress was far beyond the capacity of photorespiration. As it was reported that the amounts of some photorespiratory enzymes were not affected even under severe drought stress (Wingler et al., 1999), further study is necessary to reveal whether and how photorespiration was inhibited under these osmotic stress conditions. In addition, although pCi was higher in RBCS-antisense plants than in wild-type plants when not treated with PEG (Figure 3C), there were no differences in JPR/ETRII (Figure 4B), which is expected to be higher in wild-type plants owing to the nature of the carboxylase and oxygenase reactions of Rubisco. The method for the evaluation of photorespiration might also need to be improved.
P700 Oxidation Is Stimulated in Response to PEG-Induced Osmotic Stress Even When the Activities of Photorespiration and CO2 Assimilation Are Restricted
It has been reported that the PET reactions responded to drought or osmotic stress in a manner that limits the electron flow toward PSI, leading to P700 oxidation (Golding and Johnson, 2003; Huang et al., 2012; Zivcak et al., 2013; Zivcak et al., 2014; Wada et al., 2019). Consistent with these studies, decreases in Y(II), increases in 1−qL, slight decreases in Y(I), and increases in Y(ND) were observed in the present study irrespective of genotype (Figures 5A, D and 6A, B). Decreases in Y(II) in response to osmotic stress were accompanied by induction of NPQ in wild-type plants, RBCS-sense plants, and the 16% PEG-treated RBCS-antisense plants (Figure 5C), as observed in previous studies (Golding and Johnson, 2003; Zhou et al., 2007; Lawlor and Tezara, 2009; Huang et al., 2012; Zivcak et al., 2013; Zivcak et al., 2014; Wada et al., 2019). In the 20% PEG-treated RBCS-antisense plants, Y(NO) increased instead of Y(NPQ) (Figures 5B, C), as was observed in wild-type rice plants severely osmotic-stressed under high temperature conditions (Wada et al., 2019). These results indicate that the osmotic-stress responses of the PET reactions were normally operative even when the energy consumption by photorespiration and CO2 assimilation were largely restricted. PSII photoinhibition occurred in the 20%-PEG treated RBCS-antisense plants as Fv/Fm substantially decreased (Figure 2A). This might have restricted the electron flow to PSI and led to P700 oxidation. However, Y(II) was well correlated with Y(I) and Y(ND) when data of the 20%-PEG treated RBCS-antisense plants were included in the correlation analysis (Table 2). Decreases in Fv/Fm did also not disturb the relationships among these parameters in osmotic-stressed rice plants, including severely damaged ones under high temperature conditions (Wada et al., 2019). Therefore, electron flow to PSI were likely to be limited by Y(II), not by PSII photoinhibition.
Lumenal acidification is thought to be one of the regulatory factors for the drought-stress responses of the PET reactions as it induces NPQ at PSII (Li et al., 2000; Müller et al., 2001; Huang et al., 2012) and slows down the oxidation of plastoquinol by the cytochrome b6/f complex (Kohzuma et al., 2009; Rott et al., 2011; Tikhonov, 2013; Zivcak et al., 2014; Takagi et al., 2017b). It has also been suggested that over-reduction of the plastoquinone pool suppresses the Q cycle and electron flow at the cytochrome b6/f complex in cyanobacteria (Shaku et al., 2016; Shimakawa et al., 2018). This system was suspected to be operative in osmotic-stressed rice plants (Wada et al., 2019). The PET reactions are thought to be regulated by such processes in response to osmotic stress even in RBCS-antisense plants, as 1−qL was strongly correlated with Y(II), Y(NPQ), Y(I), and Y(ND) among the genotypes (Table 2).
In the present study, some results were different from those observed in our previous studies. P700 oxidation was not stimulated in RBCS-antisense plants in the absence of osmotic stress (Wada et al., 2018). P700 was over-reduced when RBCS-antisense plants were exposed to the combination of high irradiance and CO2-compensated conditions (Wada et al., 2018), whereas such phenomena as indicated by increases in Y(NA) were not observed in the PEG-treated RBCS-antisense plants (Figure 6C). The latter can be accounted for, at least partly, by substantial decreases in pCi that led to large decreases in energy consumption by photorespiration coupled with CO2 assimilation (Wada et al., 2018), whereas the magnitude of the decreases in pCi was not as much in the present study (Figure 3C). In addition, correlations between Y(II) and Y(NPQ) and between Y(ND) and Y(NA) were not apparent in osmotic-stressed rice plants at normal and high temperatures (Wada et al., 2019). The reason for this discrepancy is unclear. For example, growth conditions were different between these studies as different types of growth chambers were used. Such differences could lead to differences in the responses of the PET reactions, as it was shown that differences in growth irradiance affected the levels of Y(ND) in wheat (Takagi et al., 2019). Recently, Kadota et al. (2019) suggested that excess electron is dissipated by charge recombination within PSI, leading to P700 oxidation. As increases in Y(ND) was observed along with decreases in Y(NA) (Figures 6B, C and Table 2) when the activity of photorespiration coupled with CO2 assimilation was limited (Figures 3A and 4A), charge recombination in PSI might have functioned in P700 oxidation in the RBCS-antisense plants used in the present study.
P700 Oxidation Is Not Sufficient for the Protection of P700 in RBCS-Antisense Plants
We have previously reported that PSI suffered from photoinhibition even when P700 was highly oxidized under osmotic stress in rice (Wada et al., 2019). Similar trends were observed in osmotic-stressed RBCS-antisense plants (Figures 2B and 6B). These results indicate that P700 oxidation cannot fully protect PSI from photoinhibition under osmotic stress. The reason PSI underwent photoinhibition under conditions of highly oxidized P700 still remains unknown. As PSI-specific photoinhibition has been observed under drought or osmotic stress (Huang et al., 2013; Wada et al., 2019; Figure 2B), it is speculated that ROS unavoidably generated within and/or near PSI led to PSI photoinhibition. In addition, P700 oxidation was shown to be gradually stimulated while PSI photoinhibition was induced in RBCS-antisense plants by repetitive saturated pulse-illumination under the combination of high irradiance and CO2-compensated conditions (Wada et al., 2018), suggesting the possibility that P700 oxidation and ROS generation occurred at the same time.
Conclusion
In the present study, it is shown that antisense suppression of Rubisco content led to decreases in energy consumption by photorespiration coupled with CO2 assimilation under PEG-induced osmotic stress in rice plants, leading to the photoinhibition of PSI and PSII. These results clearly indicate that photorespiration coupled with CO2 assimilation plays a crucial role in the protection of PSI from photoinhibition caused by osmotic stress. As PSI was shown to be more sensitive to osmotic stress, photorespiration might compensate for such weakness in PSI. The PET reactions responded to osmotic stress and oxidized P700 in RBCS-antisense plants and in the other genotypes. Lumenal acidification and/or the redox state of the plastoquinone pool might primarily regulate the PET reactions under osmotic stress even if the activities of photorespiration and CO2 assimilation were restricted. It is shown again that P700 oxidation was not sufficient for the protection of P700 against osmotic stress. ROS unavoidably generated in PSI might damage PSI even if P700 oxidation was stimulated. Overproduction of Rubisco, in contrast, did not alter the activities of photorespiration and CO2 assimilation under osmotic stress. As a result, the photochemistry of PSII and PSI were not altered. These results suggest that further modifications of the metabolism of photorespiration and CO2 assimilation is necessary to improve drought or osmotic stress tolerance and photosynthesis.
Data Availability Statement
All datasets generated for this study are included in the article/supplementary material.
Author Contributions
YS conceived the experimental design. SW performed the experiments. SW and YS analyzed the data. SW and YS wrote the manuscript. SW, CM, AM, and YS edited the manuscript.
Funding
This study was supported by the Core Research for Environmental Science and Technology (Scientific Research Grant No. AL65D21010 to CM) and Grants-in-Aid for Scientific Research from the Japan Society for the Promotion of Science (No. 18H02111 to YS and No. 16H06379 to AM).
Conflict of Interest
The authors declare that this research was conducted in the absence of any commercial or financial relationships that could be construed as a potential conflict of interest.
Acknowledgments
We would like to thank Editage (www.editage.com) for English language editing.
Abbreviations
A, the rate of CO2 assimilation; ETRII, the rate of electron trasnport; gs, stomatal conductance; JPR, the rate of electron flow donated for photorespiration; NPQ, non-photochemical quenching; P700, the reaction center chlorophyll of photosystem I; pCi, an intercellular CO2 partial pressure PEG, poly (ethylene glycol); PET, photosynthetic electron transport; PSII, photosystem II; PSI, photosystem I; QA, the primary quinone electron acceptor of photosystem II; 1–qL, the fraction of photosystem II centers in closed states; Rd, the rate of respiration under illumination; ROS, reactive oxygen species; Y(II), the quantum efficiency of photosystem II; Y(NO), the quantum yield of non-regulated and non-photochemical energy dissipation at photosystem II; Y(NPQ), the quantum yield of non-photochemical quenching at photosystem II; Y(I), the quantum efficiency of photosystem I; Y(NA), the quantum yield of the acceptor side limitation of photosystem I; Y(ND), the quantum yield of the donor side limitation of photosystem I.
References
Arnon, D. I. (1949). Copper enzymes in isolated chloroplasts. Polyphenoloxidase in Beta vulgaris. Plant Physiol. 24, 1–15. doi: 10.1104/pp.24.1.1
Asada, K. (1999). The water-water cycle in chloroplasts: scavenging of active oxygens and dissipation of excess photons. Annu. Rev. Plant Mol. Biol. 50, 601–639. doi: 10.1146/annurev.arplant.50.1.601
Baker, N. R. (2008). Chlorophyll fluorescence: a probe of photosynthesis in vivo. Annu. Rev. Plant Biol. 59, 89–113. doi: 10.1146/annurev.arplant.59.032607.092759
Chastain, D. R., Snider, J. L., Collins, G. D., Perry, C. D., Whitaker, J., Byrd, S. A. (2014). Water deficit in field-grown Gossypium hirsutum primarily limits net photosynthesis by decreasing stomatal conductance, increasing photorespiration, and increasing the ratio of dark respiration to gross photosynthesis. J. Plant Physiol. 171, 1576–1585. doi: 10.1016/j.jplph.2014.07.014
Chaves, M. M., Maroco, J. P., Pereira, J. S. (2003). Understanding plant responses to drought—from genes to the whole plant. Func. Plant Biol. 30, 239–264. doi: 10.1071/FP02076
Cornic, G., Briantais, J.-M. (1991). Partitioning of photosynthetic electron flow between CO2 and O2 reduction in a C3 leaf (Phaseolus vulgaris L.) at different CO2 concentrations and during drought stress. Planta 183, 178–184. doi: 10.1007/BF00197786
Cruz de Carvalho, M. H. (2008). Drought stress and reactive oxygen species. Plant Signal. Behav. 3, 156–165. doi: 10.4161/psb.3.3.5536
Davies, W. J., Zhang, J. (1991). Root signals and the regulation of growth and development of plants in drying soil. Annu. Rev. Plant Physiol. Plant Mol. Biol. 42, 55–76. doi: 10.1146/annurev.pp.42.060191.000415
Demmig-Adams, B., Adams, W. W., II (1992). Photoprotection and other responses of plants to high light stress. Annu. Rev. Plant Physiol. Plant Mol. Biol. 43, 599–626. doi: 10.1146/annurev.pp.43.060192.003123
Farquhar, G. D., von Caemmerer, S., Berry, J. A. (1980). A biochemical model of photosynthetic CO2 assimilation in leaves of C3 species. Planta 149, 78–90. doi: 10.1007/BF00386231
Galmés, J., Abadía, A., Cifre, J., Medrano, H., Flexas, J. (2007). Photoprotection processes under water stress and recovery in Mediterranean plants with different growth forms and leaf habits. Physiol. Plant 130, 495–510. doi: 10.1111/j.1399-3054.2007.00919.x
Golding, A. J., Johnson, G. N. (2003). Down-regulation of linear and activation of cyclic electron transport during drought. Planta 218, 107–114. doi: 10.1007/s00425-003-1077-5
Hanawa, H., Ishizaki, K., Nohira, K., Takagi, D., Shimakawa, G., Sejima, T., et al. (2017). Land plants drive photorespiration as higher electron-sink: comparative study of post-illumination transient O2-uptake rates from liverworts to angiosperms through ferns and gymnosperms. Physiol. Plant 161, 138–149. doi: 10.1111/ppl.12580
Haupt-Herting, S., Fock, H. P. (2002). Oxygen exchange in relation to carbon assimilation in water-stressed leaves during photosynthesis. Ann. Bot. 89, 851–859. doi: 10.1093/aob/mcf023
Hirotsu, N., Makino, A., Ushio, A., Mae, T. (2004). Changes in the thermal dissipation and the electron flow in the water-water cycle in rice grown under conditions of physiologically low temperature. Plant Cell Physiol. 45, 635–644. doi: 10.1093/pcp/pch075
Huang, W., Yang, S. J., Zhang, S. B., Zhang, J. L., Cao, K. F. (2012). Cyclic electron flow plays an important role in photoprotection for the resurrection plant Paraboea rufescens under drought stress. Planta 235, 819–828. doi: 10.1007/s00425-011-1544-3
Huang, W., Fu, P. L., Jiang, Y. J., Zhang, J. L., Zhang, S. B., Hu, H., et al. (2013). Differences in the responses of photosystem I and photosystem II of three tree species Cleistanthus sumatranus, Celtis philippensis and Pistacia weinmannifolia exposed to a prolonged drought in a tropical limestone forest. Tree Physiol. 33, 211–220. doi: 10.1093/treephys/tps132
Kadota, K., Furutani, R., Makino, A., Suzuki, Y., Wada, S., Miyake, C. (2019). Oxidation of P700 induces alternative electron flow in photosystem I in wheat leaves. Plants 8:152. doi: 10.3390/plants8060152
Klughammer, C., Schreiber, U. (1994). An improved method, using saturating light pulses, for the determination of photosystem I quantum yield via P700+-absorbance changes at 830 nm. Planta 192, 261–268. doi: 10.1007/BF01089043
Kohzuma, K., Cruz, J. A., Akashi, K., Hoshiyasu, S., Munekage, Y. N., Yokota, A., et al. (2009). The long-term responses of the photosynthetic proton circuit to drought. Plant Cell Environ. 32, 209–219. doi: 10.1111/j.1365-3040.2008.01912.x
Kramer, D. M., Johnson, G., Kiirats, O., Edwards, G. E. (2004). New fluorescence parameters for the determination of QA redox state and excitation energy fluxes. Photosynth. Res. 79, 209–218. doi: 10.1023/B:PRES.0000015391.99477.0
Krieger-Liszkay, A. (2005). Singlet oxygen production in photosynthesis. J. Exp. Bot. 56, 337–346. doi: 10.1093/jxb/erh237
Kudoh, H., Sonoike, K. (2002). Irreversible damage to photosystem I by chilling in the light: cause of the degradation of chlorophyll after returning to normal growth temperature. Planta 215, 541–548. doi: 10.1007/s00425-002-0790-9
Lawlor, D. W., Tezara, W. (2009). Causes of decreased photosynthetic rate and metabolic capacity in water-deficient leaf cells: a critical evaluation of mechanisms and integration of processes. Ann. Bot. 103, 561–579. doi: 10.1093/aob/mcn244
Li, X. P., Björkman, O., Shih, C., Grossman, A. R., Rosenquist, M., Jansson, S., et al. (2000). A pigment-binding protein essential for regulation of photosynthetic light harvesting. Nature 403, 391–395. doi: 10.1038/35000131
Makino, A., Sage, R. F. (2007). Temperature response of photosynthesis in transgenic rice transformed with ‘sense’ or ‘antisense’ rbcS. Plant Cell Physiol. 48, 1472–1483. doi: 10.1093/pcp/pcm118
Makino, A., Mae, T., Ohira, K. (1985). Enzymic properties of ribulose-1,5-bisphosphate carboxylase/oxygenase purified from rice leaves. Plant Physiol. 79, 57–61. doi: 10.1104/pp.79.1.57
Makino, A., Mae, T., Ohira, K. (1988). Differences between wheat and rice in the enzyme properties of ribulose-1,5-bisphosphate carboxylase/oxygenase and their relationship to photosynthetic gas exchange. Planta 174, 30–38. doi: 10.1007/BF00394870
Makino, A., Shimada, T., Takumi, S., Kaneko, K., Matsuoka, M., Shimamoto, K., et al. (1997). Does decrease in ribulose-1,5-bisphosphate carboxylase by antisense rbcS lead to a higher N-use efficiency of photosynthesis under conditions of saturating CO2 and light in rice plants? Plant Physiol. 114, 483–491. doi: 10.1104/pp.114.2.483
Makino, A., Nakano, H., Mae, T., Shimada, T., Yamamoto, N. (2000). Photosynthesis, plant growth and N allocation in transgenic rice plants with decreased Rubisco under CO2 enrichment. J. Exp. Bot. 51, 383–389. doi: 10.1093/jexbot/51.suppl_1.383
Miyake, C., Amako, K., Shiraishi, N., Sugimoto, T. (2009). Acclimation of tobacco leaves to high light intensity drives the plastoquinone oxidation system - relationship among the fraction of open PSII centers, non-photochemical quenching of Chl fluorescence and the maximum quantum yield of PSII in the dark. Plant Cell. Physiol. 50, 730–743. doi: 10.1093/pcp/pcp032
Müller, P., Li, X., Niyogi, K. K. (2001). Non-photochemical quenching. A response to excess light energy. Plant Physiol. 125, 1558–1566. doi: 10.1104/pp.125.4.1558
Murata, N., Takahashi, S., Nishiyama, Y., Allakhverdiev, S. I. (2007). Photoinhibition of photosystem II under environmental stress. Biochim. Biophys. Acta 1767, 414–421. doi: 10.1016/j.bbabio.2006.11.019
Ogren, W. L. (1984). Photorespiration: pathways, regulation, and modification. Annu. Rev. Plant Physiol. 35, 415–442. doi: 10.1146/annurev.pp.35.060184.002215
Rott, M., Martins, N. F., Thiele, W., Lein, W., Bock, R., Kramer, D. M., et al. (2011). ATP synthase repression in tobacco restricts photosynthetic electron transport, CO2 assimilation, and plant growth by overacidification of the thylakoid lumen. Plant Cell 23, 304–321. doi: 10.1105/tpc.110.079111
Schreiber, U., Klughammer, C. (2008). Saturation Pulse method for assessment of energy conversion in PS I. PAM App. Notes 1, 11–14.
Sejima, T., Takagi, D., Fukayama, H., Makino, A., Miyake, C. (2014). Repetitive short-pulse light mainly inactivates photosystem I in sunflower leaves. Plant Cell Physiol. 55, 1184–1193. doi: 10.1093/pcp/pcu061
Sejima, T., Hanawa, H., Shimakawa, G., Takagi, D., Suzuki, Y., Fukayama, H., et al. (2016). Post-illumination transient O2-uptake is driven by photorespiration in tobacco leaves. Physiol. Plant 156, 227–238. doi: 10.1111/ppl.12388
Shaku, K., Shimakawa, G., Hashiguchi, M., Miyake, C. (2016). Reduction-induced suppression of electron flow (RISE) in the photosynthetic electron transport system of Synechococcus elongatus PCC 7942. Plant Cell Physiol. 57, 1443–1453. doi: 10.1093/pcp/pcv198
Shimakawa, G., Shaku, K., Miyake, C. (2018). Reduction-induced suppression of electron flow (RISE) is relieved by non-ATP-consuming electron flow in Synechococcus elongatus PCC 7942. Front. Microbiol. 9:886:886. doi: 10.3389/fmicb.2018.00886
Sonoike, K. (2011). Photoinhibition of photosystem I. Physiol. Plant 142, 56–64. doi: 10.1111/j.1399-3054.2010.01437.x
Sudo, E., Suzuki, Y., Makino, A. (2014). Whole-plant growth and N utilization in transgenic rice plants with increased or decreased Rubisco content under different CO2 partial pressures. Plant Cell Physiol. 55, 1905–1911. doi: 10.1093/pcp/pcu119
Suganami, M., Suzuki, Y., Sato, T., Makino, A. (2018). Relationship between Rubisco activase and Rubisco contents in transgenic rice plants with overproduced or decreased Rubisco content. Soil Sci. Plant Nutr. 64, 352–359. doi: 10.1080/00380768.2018.1433455
Suzuki, Y., Ohkubo, M., Hatakeyama, H., Ohashi, K., Yoshizawa, R., Kojima, S., et al. (2007). Increased Rubisco content in transgenic rice transformed with the ‘sense’ rbcS gene. Plant Cell Physiol. 48, 626–637. doi: 10.1093/pcp/pcm035
Suzuki, Y., Miyamoto, T., Yoshizawa, R., Mae, T., Makino, A. (2009). Rubisco content and photosynthesis of leaves at different positions in transgenic rice with an overexpression of RBCS. Plant Cell Environ. 32, 417–427. doi: 10.1111/j.1365-3040.2009.01937.x
Takagi, D., Ishizaki, K., Hanawa, H., Mabuchi, T., Shimakawa, G., Yamamoto, H., et al. (2017a). Diversity of strategies for escaping reactive oxygen species production within photosystem I among land plants. Physiol. Plant 161, 56–74. doi: 10.1111/ppl.12562
Takagi, D., Amako, K., Hashiguchi, M., Fukaki, H., Ishizaki, K., Goh, T., et al. (2017b). Chloroplastic ATP synthase builds up a proton motive force preventing production of reactive oxygen species in photosystem I. Plant J. 91, 306–324. doi: 10.1111/tpj.13566
Takagi, D., Ihara, H., Takumi, S., Miyake, C. (2019). Growth light environment changes the sensitivity of photosystem I photoinhibition depending on common wheat cultivars. Front. Plant Sci. 10:686:686. doi: 10.3389/fpls.2019.00686
Tikhonov, A. N. (2013). pH-Dependent regulation of electron transport and ATP synthesis in chloroplasts. Photosynth. Res. 116, 511–534. doi: 10.1007/s11120-013-9845-y
Valentini, R., Epron, D., De Angelis, P., Matteucci, G., Dreyer, E. (1995). In situ estimation of net CO2 assimilation, photosynthetic electron flow and photorespiration in Turkey oak (Q.cerris L.) leaves: diurnal cycles under different levels of water supply. Plant Cell Environ. 18, 631–640. doi: 10.1111/j.1365-3040.1995.tb00564.x
Wada, S., Suzuki, Y., Takagi, D., Miyake, C., Makino, A. (2018). Effects of genetic manipulation of the activity of photorespiration on the redox state of photosystem I and its robustness against excess light stress under CO2-limited conditions in rice. Photosynth. Res. 137, 431–441. doi: 10.1007/s11120-018-0515-y
Wada, S., Takagi, D., Miyake, C., Makino, A., Suzuki, Y. (2019). Responses of the photosynthetic electron transport reactions stimulate the oxidation of the reaction center chlorophyll of photosystem I, P700, under drought and high temperatures in rice. Int. J. Mol. Sci. 20, 2068. doi: 10.3390/ijms20092068
Wingler, A., Quick, W. P., Bungard, R. A., Bailey, K. J., Lea, P. J., Leegood, R. C. (1999). The role of photorespiration during drought stress: an analysis utilizing barley mutants with reduced activities of photorespiratory enzymes. Plant Cell Environ. 22, 361–373. doi: 10.1046/j.1365-3040.1999.00410.x
Xu, Z., Zhou, G., Shimizu, H. (2010). Plant responses to drought and rewatering. Plant Signal. Behav. 5, 649–654. doi: 10.4161/psb.5.6.11398
Zhou, Y., Lam, H. M., Zhang, J. (2007). Inhibition of photosynthesis and energy dissipation induced by water and high light stresses in rice. J. Exp. Bot. 58, 1207–1217. doi: 10.1093/jxb/erl291
Zivcak, M., Brestic, M., Balatova, Z., Drevenakova, P., Olsovska, K., Kalaji, H. M., et al. (2013). Photosynthetic electron transport and specific photoprotective responses in wheat leaves under drought stress. Photosynth. Res. 117, 529–546. doi: 10.1007/s11120-013-9885-3
Zivcak, M., Kalaji, H. M., Shao, H. B., Olsovska, K., Brestic, M. (2014). Photosynthetic proton and electron transport in wheat leaves under prolonged moderate drought stress. J. Photochem. Photobiol. B. 137, 107–115. doi: 10.1016/j.jphotobiol.2014.01.007
Keywords: osmotic stress, Rubisco, photorespiration, CO2 assimilation, photosystem I, photosystem II, rice
Citation: Wada S, Miyake C, Makino A and Suzuki Y (2020) Photorespiration Coupled With CO2 Assimilation Protects Photosystem I From Photoinhibition Under Moderate Poly(Ethylene Glycol)-Induced Osmotic Stress in Rice. Front. Plant Sci. 11:1121. doi: 10.3389/fpls.2020.01121
Received: 01 October 2019; Accepted: 07 July 2020;
Published: 24 July 2020.
Edited by:
Adriano Nunes-Nesi, Universidade Federal de Viçosa, BrazilReviewed by:
Xenie Johnson, Commissariat à l'Energie Atomique et aux Energies Alternatives (CEA), FranceXinguang Zhu, Chinese Academy of Sciences (CAS), China
Copyright © 2020 Wada, Miyake, Makino and Suzuki. This is an open-access article distributed under the terms of the Creative Commons Attribution License (CC BY). The use, distribution or reproduction in other forums is permitted, provided the original author(s) and the copyright owner(s) are credited and that the original publication in this journal is cited, in accordance with accepted academic practice. No use, distribution or reproduction is permitted which does not comply with these terms.
*Correspondence: Yuji Suzuki, eXN1enVraUBpd2F0ZS11LmFjLmpw