- 1Department of Biochemistry and Molecular Biology, Graduate School of Science and Engineering, Saitama University, Saitama, Japan
- 2Graduate School of Art and Sciences, The University of Tokyo, Tokyo, Japan
- 3Department of Molecular Microbiology, Faculty of Life Sciences, Tokyo University of Agriculture, Tokyo, Japan
The tolerance of photosynthesis to strong light increases in photosynthetic organisms during acclimation to strong light. We investigated the role of carotenoids in the protection of photosystem II (PSII) from photoinhibition after acclimation to strong light in the cyanobacterium Synechocystis sp. PCC 6803. In cells that had been grown under strong light at 1,000 μmol photons m−2 s−1 (SL), specific carotenoids, namely, zeaxanthin, echinenone, and myxoxanthophyll, accumulated at high levels, and the photoinhibition of PSII was less marked than in cells that had been grown under standard growth light at 70 μmol photons m−2 s−1 (GL). The rate of photodamage to PSII, as monitored in the presence of lincomycin, did not differ between cells grown under SL and GL, suggesting that the mitigation of photoinhibition after acclimation to SL might be attributable to the enhanced ability to repair PSII. When cells grown under GL were transferred to SL, the mitigation of photoinhibition of PSII occurred in two distinct stages: a first stage that lasted 4 h and the second stage that occurred after 8 h. During the second stage, the accumulation of specific carotenoids was detected, together with enhanced synthesis de novo of proteins that are required for the repair of PSII, such as the D1 protein, and suppression of the production of singlet oxygen (1O2). In the ΔcrtRΔcrtO mutant of Synechocystis, which lacks zeaxanthin, echinenone, and myxoxanthophyll, the mitigation of photoinhibition of PSII, the enhancement of protein synthesis, and the suppression of production of 1O2 were significantly impaired during the second stage of acclimation. Thus, elevated levels of the specific carotenoids during acclimation to strong light appeared to protect protein synthesis from 1O2, with the resultant mitigation of photoinhibition of PSII.
Introduction
Light is necessary for photosynthesis but excess light impairs photosynthesis. Photosystem II (PSII), which is a protein-pigment complex that converts light energy to chemical energy, is known to be particularly sensitive to strong light. Exposure of photosynthetic organisms to strong light results in the specific inactivation of PSII, and this phenomenon is referred to as photoinhibition of PSII (Aro et al., 1993b; Vass, 2012). Photoinhibition of PSII becomes apparent under strong light when the rate of photodamage to PSII exceeds the rate of repair of PSII (Murata and Nishiyama, 2018). Photodamaged PSII is repaired via an efficient repair system that involves proteolytic degradation of damaged D1 protein; synthesis de novo of the precursor to the D1 protein (pre-D1); insertion of the pre-D1 into PSII; processing of pre-D1 at the carboxy-terminal extension; and reactivation of PSII (Theis and Schroda, 2016; Li et al., 2018).
Photodamage to PSII depends on the intensity of incident light (Allakhverdiev and Murata, 2004), whereas the repair of PSII is adversely affected by various types of environmental stress and, in particular, by oxidative stress due to reactive oxygen species (ROS), such as the superoxide anion radical, hydrogen peroxide, the hydroxyl radical, and singlet oxygen (1O2: Nishiyama et al., 2001; Nishiyama et al., 2004; Allakhverdiev and Murata, 2004). These ROS are produced in abundance in the photosynthetic machinery under strong light, as a result of the transport of electrons and the transfer of excitation energy (Asada, 1999). In the cyanobacterium Synechocystis sp. PCC 6803 (hereafter, Synechocystis), the ROS-induced inhibition of the repair of PSII has been attributed to the inhibition of synthesis of pre-D1 during translational elongation (Nishiyama et al., 2001; Nishiyama et al., 2004). Biochemical studies revealed that two translation factors, EF-Tu and EF-G, key proteins that support translational elongation, are inactivated via oxidation by ROS of specific cysteine residues (Kojima et al., 2007; Yutthanasirikul et al., 2016). Expression, in Synechocystis, of mutated EF-Tu or mutated EF-G in which one of the ROS-sensitive cysteine residues had been replaced by a serine residue mitigated the photoinhibition of PSII via the acceleration of synthesis de novo of proteins, including the D1 protein, with the enhancement of the repair of PSII under strong light (Ejima et al., 2012; Jimbo et al., 2018). Thus, the sensitivity of the repair system to ROS appears to be a critical factor that determines the extent of photoinhibition of PSII.
To minimize levels of ROS, photosynthetic organisms have evolved various anti-oxidative systems, which incorporate ROS-scavenging enzymes and antioxidants. Defects in the anti-oxidative systems exacerbate the photoinhibition of PSII. For example, in mutants of Synechocystis that were deficient in catalase and thioredoxin peroxidase, in zeaxanthin and echinenone, and in α-tocopherol, respectively, the photoinhibition of PSII was accelerated as a consequence of the decelerated repair of PSII (Nishiyama et al., 2001; Inoue et al., 2011; Kusama et al., 2015). By contrast, in a mutant of Synechocystis that overexpressed superoxide dismutase and catalase, the photoinhibition of PSII was mitigated, with the accelerated repair of PSII (Sae-Tang et al., 2016). In addition, overexpression in Synechocystis of orange carotenoid protein, which dissipates excitation energy and depresses the production of 1O2, protected the repair of PSII under strong light, with the resultant mitigation of photoinhibition of PSII (Takahashi et al., 2019). Thus, the capacity to minimize levels of ROS appears to be essential for the efficient repair of PSII under strong light.
Photosynthetic organisms exhibit enhanced tolerance of PSII to photoinhibition when they acclimate to strong light (Adams et al., 1987). This ability has been associated with the enhanced ability to repair PSII in plants (Aro et al., 1993a), in algae (Erickson et al., 2015), and in cyanobacteria (Samuelsson et al., 1987; Jimbo et al., 2019). During acclimation to strong light, various physiological changes occur (Muramatsu and Hihara, 2012). In cyanobacteria, such changes include a reduction in the size of the antenna phycobilisomes (Grossman et al., 1993), stimulation of the state transition and thermal dissipation of excitation energy (Fujimori et al., 2005), and activation of the Calvin-Benson cycle (Hihara et al., 1998) and anti-oxidative systems (Latifi et al., 2009). With respect to the repair of PSII, it seems likely that activation of anti-oxidative systems might contribute significantly to the enhanced ability to repair PSII during acclimation to strong light. It has been reported that cyanobacteria accumulate specific carotenoids, namely, zeaxanthin, echinenone, and myxoxanthophyll, at high levels when they are grown under strong light, such as 1,300 µmol photons m−2 s−1 (Masamoto and Furukawa, 1997; Steiger et al., 1999), but the roles of such carotenoids in acclimation to strong light remain to be elucidated.
In the present study, we investigated the roles of carotenoids in the protection of PSII from photoinhibition during acclimation to strong light in the ΔcrtRΔcrtO mutant of Synechocystis. In this mutant, zeaxanthin, echinenone, and myxoxanthophyll are deficient as a result of inactivation of genes for β-carotene hydroxylase CrtR, which converts β-carotene and deoxymyxoxanthophyll to zeaxanthin and myxoxanthophyll, respectively, and β-carotene ketolase CrtO, which converts β-carotene to echinenone (Fernández-González et al., 1997; Masamoto et al., 1998; Domonkos et al., 2013). When cells were transferred from growth light to strong light, the photoinhibition of PSII was mitigated in two stages: the first stage occurred within 4 h and the second stage occurred after 8 h. The second stage of mitigation was associated with the accumulation of zeaxanthin, echinenone, and myxoxanthophyll, which contributed to the enhanced repair of PSII via suppression of the production of 1O2 and acceleration of the synthesis de novo of proteins that are required for the repair of PSII, such as the D1 protein.
Materials and Methods
Cell and Culture Conditions
Cells of a glucose-tolerant strain (hereafter referred to as wild-type) and of the ΔcrtRΔcrtO mutant strain of Synechocystis sp. PCC 6803 (Kusama et al., 2015) were grown photoautotrophically at 32°C in liquid BG11 medium under standard growth light at 70 μmol photons m−2 s−1 (GL), moderately strong light at 200 μmol photons m−2 s−1 (ML), or strong light at 1,000 μmol photons m−2 s−1 (SL), with aeration by sterile air that contained 1% (v/v) CO2. Cells in cultures with an optical density at 730 nm of 1.0 ± 0.1 were used for assays unless otherwise noted.
Analysis of Carotenoids
Pigments were extracted from cells with a mixture of acetone and methanol (7:2, v/v) and were analyzed by HPLC on a system equipped with a μBondapak C18 column (8 mm × 100 mm; RCM type; Waters, Milford, MA, U.S.A.), as described previously (Kusama et al., 2015), with slight modifications. Carotenoids were eluted with a linear gradient from a mixture of methanol and water (9:1, v/v) to 100% methanol for 20 min and then with isocratic 100% methanol, at a rate of 1.8 ml min−1. Zeaxanthin and β-carotene were detected at 450 nm, while echinenone, myxoxanthophyll, and synechoxanthin were detected at 470 nm. Levels of individual carotenoids were normalized by the content of chlorophyll a.
Assay of Photoinhibition of PSII
For standard assay of photoinhibition, 30-ml aliquots of cell cultures were exposed to light at 2,000 μmol photons m−2 s−1 at 32°C for designated periods of time to induce the photoinhibition of PSII. For assays of photodamage, lincomycin was added to suspensions of cells at a final concentration of 200 μg ml−1 just before the onset of illumination. The activity of PSII was measured at 32°C in the terms of the evolution of oxygen in the presence of 1 mM 1,4-benzoquinone and 1 mM K3Fe(CN)6 with a Clark-type oxygen electrode (Hansatech Instruments, Norfolk, U.K.). For time-course assays after the shift from GL to SL, 30-mL suspensions of cells grown under GL with an optical density at 730 nm of 0.4 ± 0.1 were incubated under SL and aliquots of 1 mL were withdrawn at designated times for measurements of the activity of PSII. Aliquots of 1 mL were illuminated with strong light at 1,500 µmol photons m−2 s−1 for 30 min at 32°C within the chamber of the oxygen electrode and the activity of PSII was measured. The ratio, as a percentage, of the residual activity of PSII to the initial activity of PSII at each designated time point was defined as the strong-light tolerance of PSII.
Quantitation of Chlorophyll and Carotenoids
Chlorophyll a and carotenoids were extracted from cells with 100% methanol and the concentrations of these pigments were determined spectroscopically, as described previously (Wellburn, 1994; Ritchie, 2006).
Detection of 1O2
The production of 1O2 in cells was detected by measuring the rate of the light-induced uptake of oxygen in the presence of histidine, as described previously (Rehman et al., 2013; Kusama et al., 2015). Cells in cultures with an optical density at 730 nm of 0.5 ± 0.1 were exposed to light at 2,500 μmol photons m−2 s−1 at 32°C in the presence of 5 mM histidine and in its absence, and the evolution of oxygen was measured in the absence of electron acceptors. The generation of 1O2 was quantitated by subtracting the rate of the evolution of oxygen in the absence of histidine from the rate in its presence. Assays were also performed in the presence of either 10 µM DCMU or 10 mM NaN3.
Labeling of Proteins In Vivo
For pulse labeling of proteins, 15-ml aliquots of cell cultures were incubated at 32°C in light at 1,500 µmol photons m-2 s-1 for 15 min in the presence of 240 kBq ml−1 35S-labeled methionine plus cysteine (EasyTag™ EXPRE35S35S; PerkinElmer, Waltham, MA, U.S.A.), as described previously (Nishiyama et al., 2004). Labeling was terminated by the addition of non-radioactive methionine and cysteine to a final concentration of 2 mM each, with immediate cooling of samples on ice. Thylakoid membranes were isolated from cells as described previously (Nishiyama et al., 2004), and membrane proteins were separated by SDS-PAGE on a 12.5% polyacrylamide gel that contained 6 M urea. Labeled proteins on the gel were visualized with an imaging analyzer (FLA-7000; Fujifilm, Tokyo, Japan) and levels of the D1 protein were determined densitometrically, as described previously (Kojima et al., 2007).
Results
Effects of Specific Carotenoids on Growth Under Strong Light
We grew cells of the wild-type strain of Synechocystis and the derivative ΔcrtRΔcrtO strain, which is deficient in zeaxanthin, echinenone, and myxoxanthophyll (Kusama et al., 2015), under standard growth light (GL; 70 µmol photons m−2 s−1), moderately strong light (ML; 200 µmol photons m−2 s−1), and strong light (SL; 1,000 µmol photons m−2 s−1). Wild-type cells grew faster under ML than under GL (Figure 1A). Under SL, wild-type cells grew at the almost same rate as under ML during the first 4 d and then the proliferation of cells ceased (Figure 1A). The growth of ΔcrtRΔcrtO cells was slower than that of wild-type cells under light at the three different intensities and exhibited light dependency similar to that of wild-type cells (Figure 1B). Under SL, suspensions of both types of cell looked yellower in color than under GL and ML (Figure 1C). Spectroscopic analyses showed that under SL, the contents of chlorophyll a and phycocyanin decreased in both types of cell, while the contents of carotenoids increased (Supplementary Figure S1, Supplementary Table S1). The most striking difference between the two strains was that wild-type cells remained blue-green in color for 10 d under SL, whereas ΔcrtRΔcrtO cells started to bleach within 8 to 10 d under SL, indicating that the mutant was sensitive to strong light.
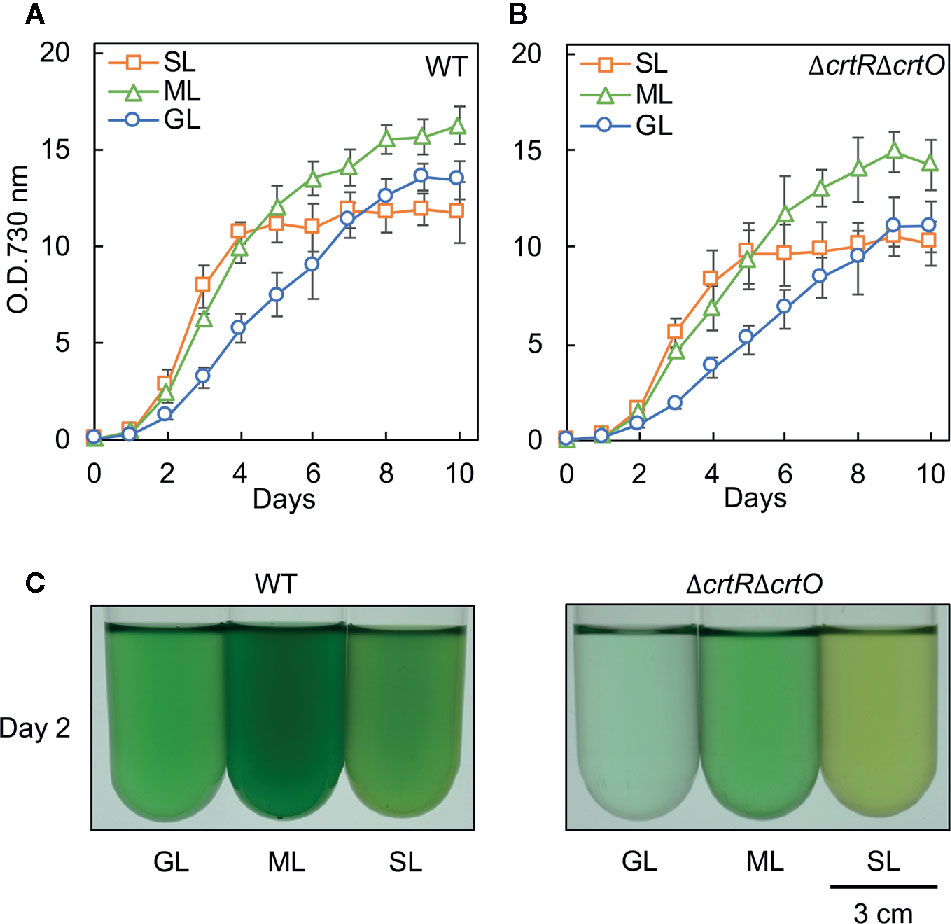
Figure 1 Growth of wild-type and ΔcrtRΔcrtO cells under light at three different intensities. Cells were grown photoautotrophically at 32°C under standard growth light (GL) at 70 μmol photons m−2 s−1, under moderately strong light (ML) at 200 μmol photons m−2 s−1, and under strong light (SL) at 1,000 μmol photons m−2 s−1. Growth curves of wild-type (A) and ΔcrtRΔcrtO (B) cells as monitored in terms of optical density at 730 nm. (C) Representative images of cell suspensions after culture for 2 days. Values are means ± SD (bars) of results from three independent experiments.
Levels of Carotenoids in Cells Grown Under Strong Light
The major carotenoids in Synechocystis are β-carotene, zeaxanthin, echinenone, and myxoxanthophyll (Takaichi and Mochimaru, 2007; Kusama et al., 2015). We analyzed the levels of these carotenoids in wild-type and ΔcrtRΔcrtO cells that had been grown under GL, ML, and SL for 24 h (Figure 2). In wild-type cells grown under SL, levels of β-carotene, zeaxanthin, echinenone, and myxoxanthophyll were much higher than those in cells grown under GL and ML. There was no detectable zeaxanthin, echinenone or myxoxanthophyll in ΔcrtRΔcrtO cells. However, in ΔcrtRΔcrtO cells grown under SL, levels of deoxymyxoxanthophyll and β-carotene were higher than those in cells grown under GL and ML. Since myxoxanthophyll is synthesized from deoxymyxoxanthophyll via a reaction catalyzed by β-carotene hydroxylase CrtR (Takaichi et al., 2001), it is reasonable that deoxymyxoxanthophyll accumulated in ΔcrtRΔcrtO cells. The level of β-carotene in ΔcrtRΔcrtO cells was about twice that in wild-type cells. Synechoxanthin, a carotenoid found specifically in cyanobacteria (Graham and Bryant, 2008), accumulated at very low levels in both types of cell under all light conditions tested.
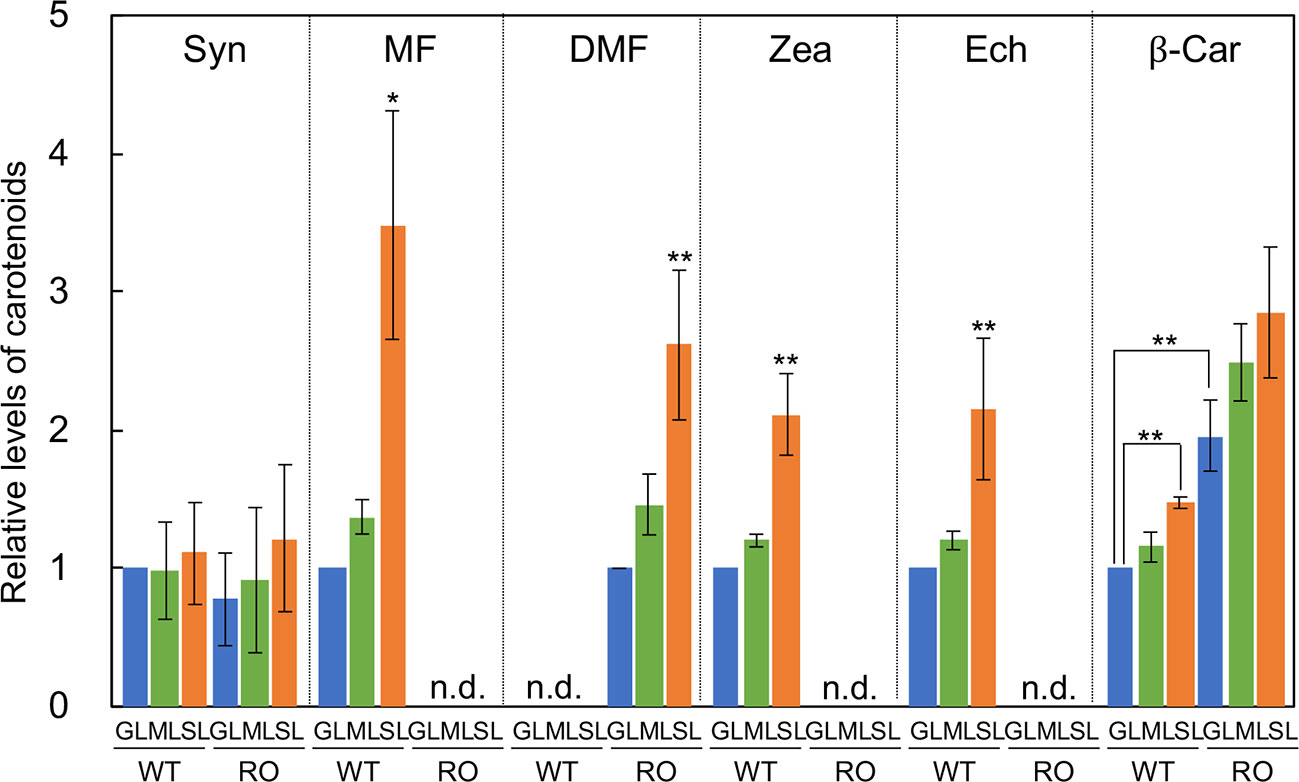
Figure 2 Levels of carotenoids in wild-type (WT) and ΔcrtRΔcrtO (RO) cells grown under GL, ML and SL (see legend to Figure 1). Syn, synechoxanthin; MF, myxoxanthophyll; DMF, deoxymyxoxanthophyll; Zea, zeaxanthin; Ech, echinenone; β-Car, β-carotene; n.d., not detected. Levels of Syn, MF, Zea, Ech, and β-Car are relative to those in wild-type cells grown under GL, respectively, while levels of DMF are relative to that in ΔcrtRΔcrtO cells grown under GL. Values are means ± SD (bars) of results from three independent experiments. Asterisks indicate statistically significant differences (*P < 0.05; **P < 0.01; Student's t test).
Specific Carotenoids Protect the Repair of PSII During Acclimation to Strong Light
We examined the photoinhibition of PSII in wild-type and ΔcrtRΔcrtO cells that had been grown under GL, ML, and SL to an optical density at 730 nm of 0.8 ± 0.1. When wild-type cells grown under GL were exposed to light at 2,000 µmol photons m−2 s−1, the activity of PSII fell to 51% of the initial level in 120 min (Figure 3A). By contrast, the activity of PSII in cells grown under ML remained at 65% of the initial level after 120 min, and the activity of PSII in cells grown under SL remained at 88% of the initial level. However, when cells were exposed to light at 2,000 µmol photons m−2 s−1 in the presence of lincomycin, which blocks the repair of PSII, the activity of PSII in cells grown under GL, under ML, and under SL fell at similar rates (Figure 3B). When cells were exposed to a weaker light at 700 µmol photons m−2 s−1 in the presence of lincomycin, the activity of PSII in cells grown under GL, under ML and under SL also fell at similar rates (Figure 3C), suggesting that increasing the intensity of the growth light did not affect photodamage but enhanced the repair of PSII. When ΔcrtRΔcrtO cells that had been grown under GL were exposed to light at 2,000 µmol photons m−2 s−1, the activity of PSII fell to 26% of the initial level within 120 min (Figure 3D). The activity of PSII in ΔcrtRΔcrtO cells that had been grown under ML remained at 45% of initial level after 120 min, and the activity of PSII in cells in ΔcrtRΔcrtO cells that had been grown under SL remained at 53% of the initial level. There were no differences in the extent of photodamage to PSII under light at 2,000 or 700 µmol photons m−2 s−1 among cells grown under GL, under ML and under SL (Figures 3E, F). Thus, it appeared that ΔcrtRΔcrtO cells were more susceptible than wild-type cells to photoinhibition of PSII, as a consequence of the decreased ability to repair PSII. Nevertheless, the ability to enhance the repair of PSII after growth under ML and SL was retained to some extent in ΔcrtRΔcrtO cells.
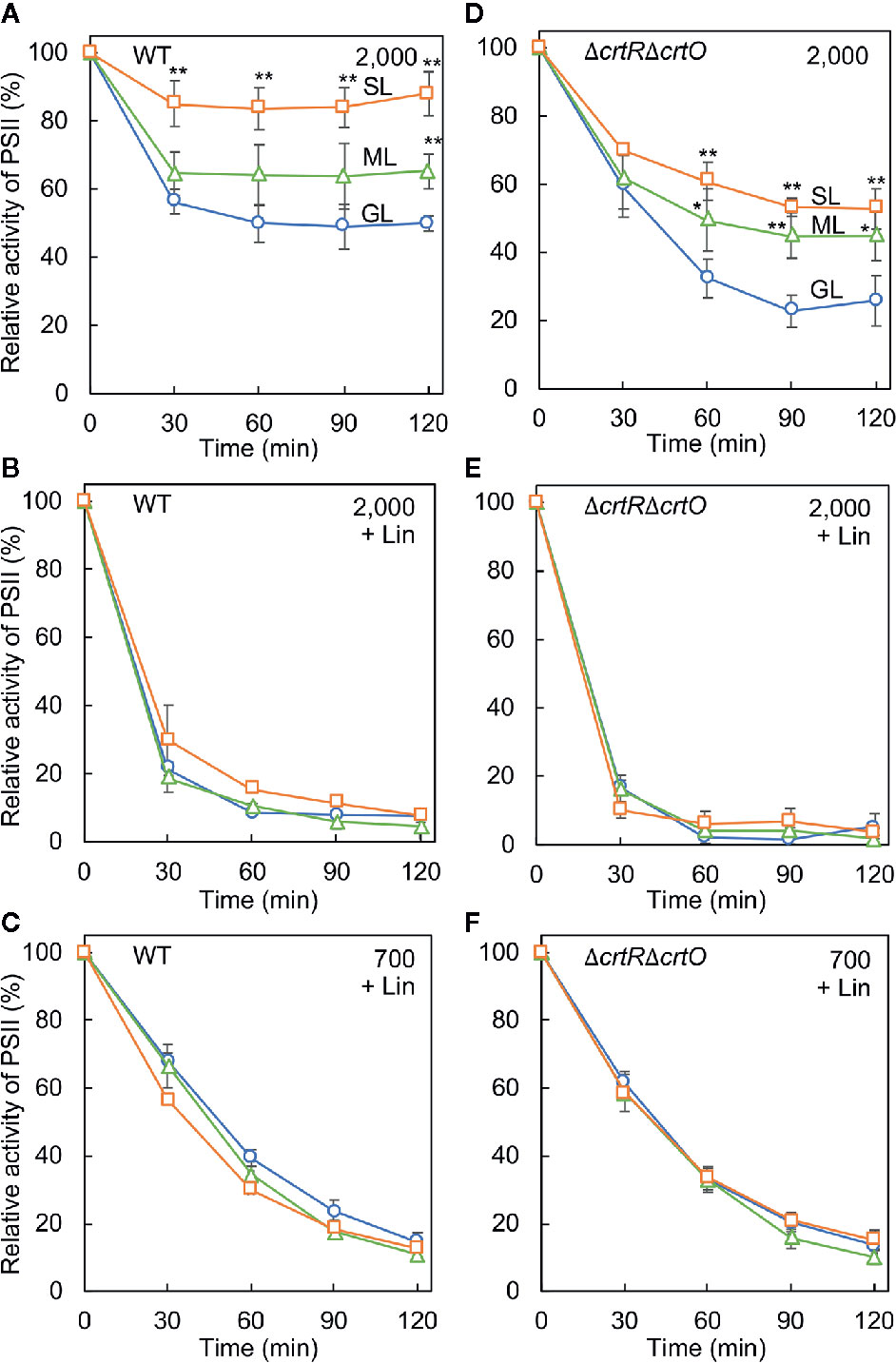
Figure 3 Effects of growth light on the photoinhibition of PSII in wild-type (A–C) and ΔcrtRΔcrtO (D–F) cells. Cells that had been grown under GL, ML, and SL (see legend to Figure 1) for 24 h were exposed to strong light at 2,000 µmol photons m−2 s−1, with aeration by ambient air, in the absence of lincomycin (Lin; A, D) and in its presence (B, E). Cells were also exposed to strong light at 700 µmol photons m−2 s−1 in the presence of lincomycin (C, F). The activity of PSII was monitored in terms of the evolution of oxygen in the presence 1 mM 1,4-benzoquinone as the electron acceptor. The activities taken as 100% for wild-type cells grown under GL, ML, and SL were 1,075 ± 118, 1,060 ± 144, 1,006 ± 200 µmol O2 mg−1 Chl h−1, respectively. The activities taken as 100% for ΔcrtRΔcrtO cells grown under GL, ML, and SL were 561 ± 167, 501 ± 71, 482 ± 99 µmol O2 mg−1 Chl h−1, respectively. Values are means ± SD (bars) of results from five independent experiments. Asterisks indicate statistically significant differences compared to cells grown under GL (*P < 0.05; **P < 0.01; Student's t-test).
Specific Carotenoids Mitigate the Photoinhibition of PSII During the Second Stage of Acclimation to Strong Light
We monitored the time course of changes in the activity of PSII after cells that had been grown under GL were transferred to SL. In wild-type cells, the activity of PSII dropped by 15% in 2 h and then increased to above the initial activity in 4 h (Figure 4A, 0 min). We also withdrew aliquots of cell suspensions at designated times during incubation under SL and exposed them to light at 1,500 µmol photons m−2 s−1 for 30 min to induce the photoinhibition of PSII. The residual activity of PSII increased during incubation under SL (Figure 4A, 30 min). The ratio (as a percentage) of the residual activity of PSII to the initial activity of PSII at each designated time point was defined as the strong-light tolerance of PSII. Under SL, the strong-light tolerance of PSII increased from 14% to 36% in 4 h and reached a plateau (Figure 4B, −Lin). Then the strong-light tolerance started to increase again after 8 h and reached 45% within 12 h. Thus, it appeared that the mitigation of photoinhibition of PSII occurred in two stages: the first stage occurred during the first 4 h and the second stage occurred after 8 h. However, when the strong-light tolerance of PSII was monitored in the presence of lincomycin, it failed to increase (Figure 4B, +Lin), suggesting that the repair of PSII was enhanced during transfer of cells from GL to SL. We also monitored changes in levels of chlorophyll a and carotenoids after the transfer of cells to SL. Levels of chlorophyll a fell rapidly under SL, whereas levels of carotenoids started to rise within 8 h under SL (Figure 4C).
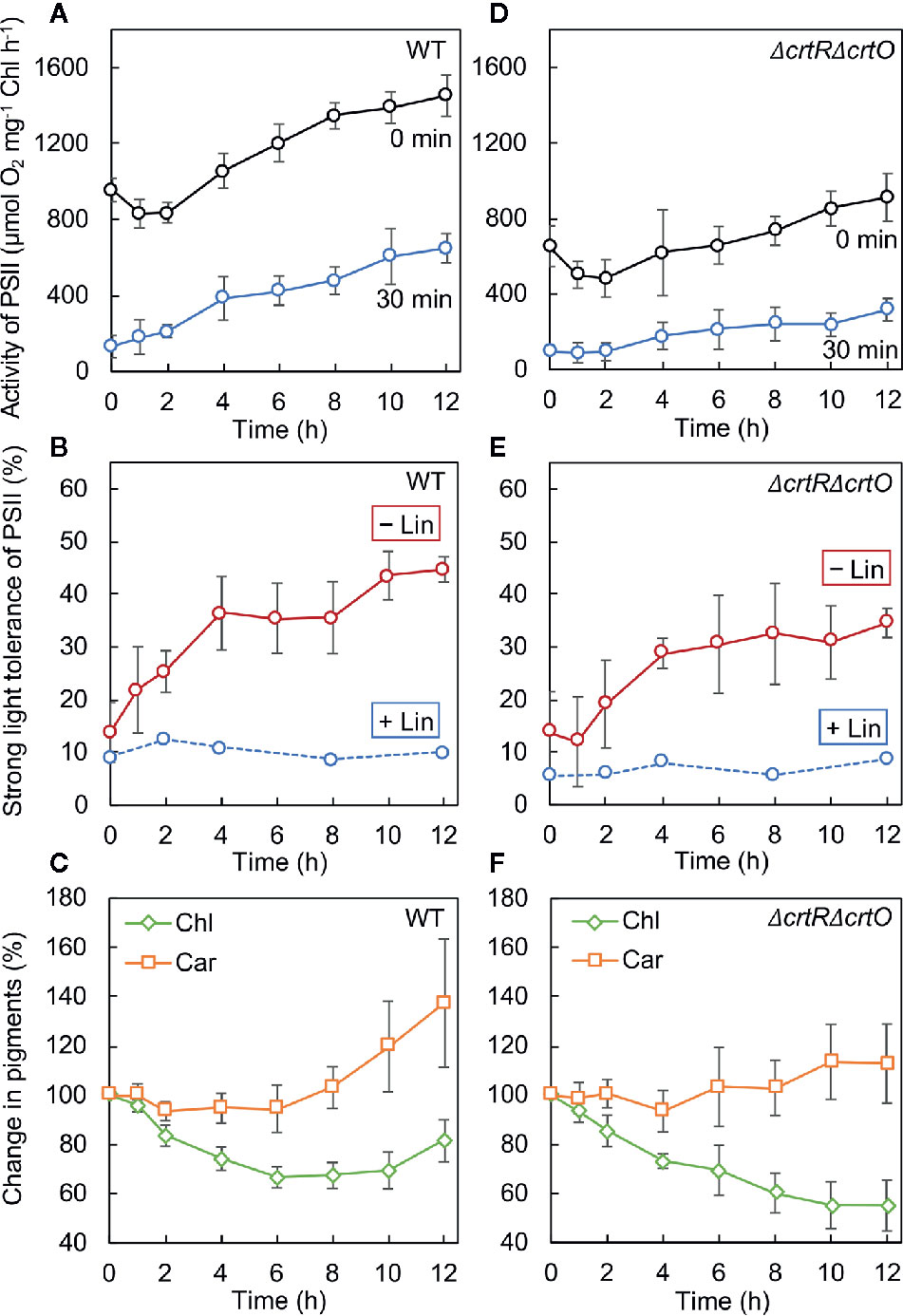
Figure 4 Changes in the activity of PSII, the strong-light tolerance of PSII, and levels of pigments after transfer of wild-type (A–C) and ΔcrtRΔcrtO (D–F) cells from GL to SL (see legend to Figure 1). (A, D) Changes in the activity of PSII at the indicated times (0 min) and the residual activity of PSII after cells at the indicated times had been exposed to light at 1,500 µmol photons m−2 s−1 for 30 min (30 min). (B, E) The strong-light tolerance of PSII, defined as the ratio (as a percentage) of the residual activity of PSII (30 min) to the initial activity of PSII (0 min) at the indicated times, as mentioned above. Photodamage to PSII was also monitored by addition of lincomycin (Lin) prior to exposure of cells to light at 1,500 µmol photons m−2 s−1 for 30 min. (C, F) Changes in levels of chlorophyll a (Chl) and carotenoids (Car). Values are means ± SD (bars) of results from five independent experiments.
In ΔcrtRΔcrtO cells, the activity of PSII dropped by 21% in 2 h and then increased, albeit more slowly than that in wild-type cells, under SL (Figure 4D, 0 min). The residual activity of PSII after exposure of cells to light at 1,500 µmol photons m−2 s−1 for 30 min was much lower than that in wild-type cells and also increased under SL, but again more slowly than in wild-type cells (Figure 4D, 30 min). Under SL, the strong-light tolerance of PSII increased from 14% to 35% and then ceased to increase significantly (Figure 4E, −Lin). Moreover, it did not change in the presence of lincomycin (Figure 4E, +Lin). These results indicate that the enhancement of the repair of PSII during the second stage might have been impaired in the mutant cells. Levels of chlorophyll a continued to decline under SL, while levels of carotenoids remained almost unchanged (Figure 4F). Comparison of wild-type cells to ΔcrtRΔcrtO cells suggested that the accumulation of zeaxanthin, echinenone, and myxoxanthophyll might be associated with the enhanced repair of PSII during the second stage.
Specific Carotenoids Enhance the Synthesis of the D1 Protein During Acclimation to Strong Light
The synthesis de novo of the D1 protein plays a vital role in the repair of PSII (Aro et al., 1993b). To examine the effects of elevated levels of carotenoids on the synthesis de novo of the D1 protein, we monitored the incorporation of 35S-labeled methionine plus cysteine into proteins during the exposure of cells to strong light at 1,500 µmol photons m−2 s−1 for 15 min. Figure 5A shows the patterns of pulse-labeled proteins from thylakoid membranes of wild-type and ΔcrtRΔcrtO cells after cells had been transferred from GL to SL and incubated for designated times. In wild-type cells, the rate of synthesis of the D1 protein dropped by 11% in 2 h and then increased 1.1- and 1.4-fold by 4 and 12 h, respectively, under SL (Figure 5B). In ΔcrtRΔcrtO cells, the rate of synthesis of the D1 protein under SL was 20% lower than in wild-type cells (Figure 5C). The rate of synthesis dropped by 20% within 2 h, returned to the initial level at 4 h, and had increased 1.2-fold by 12 h (Figure 5C). In particular, the acceleration of synthesis of the D1 protein, as determined after 12 h, was much lower in ΔcrtRΔcrtO cells than in wild-type cells. Note that the patterns of synthesis of almost all the thylakoid proteins were similar to that of the D1 protein, suggesting that the absence of zeaxanthin, echinenone, and myxoxanthophyll had an adverse effect on overall protein synthesis during incubation under SL (Figure 5A).
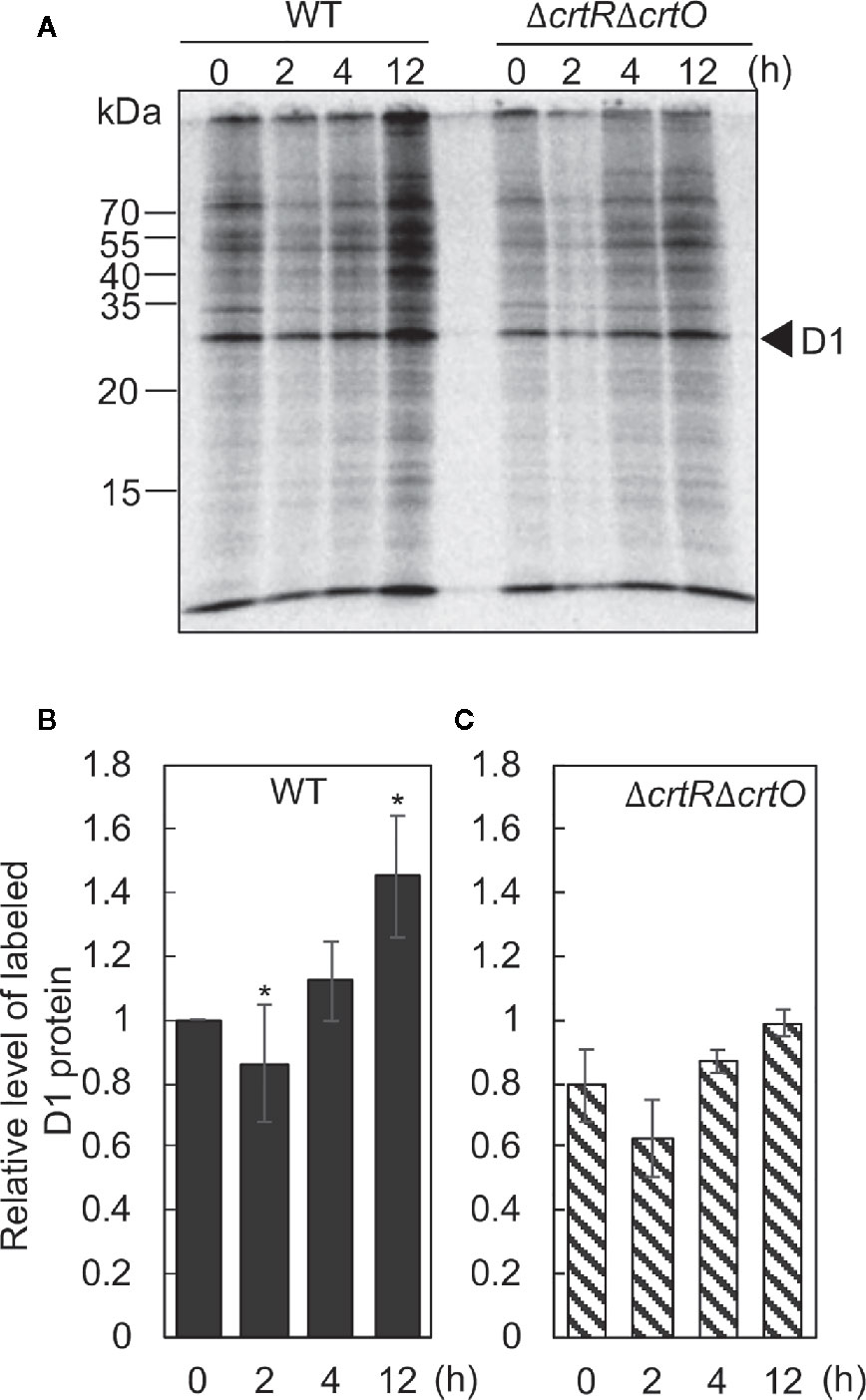
Figure 5 Changes in the synthesis de novo of proteins in thylakoid membranes after transfer of wild-type and ΔcrtRΔcrtO cells from GL to SL (see legend to Figure 1). Proteins from cells that had been incubated under SL for the indicated times were pulse-labeled by incubation of cells at 32°C, for 15 min, under strong light at 1,500 µmol photons m−2 s−1 in the presence of 35S-labeled methionine plus cysteine. (A) A representative radiogram of pulse-labeled proteins from thylakoid membranes. (B, C) Quantitation of levels of labeled D1 protein in wild-type (B) and ΔcrtRΔcrtO (C) cells. Levels of labeled D1 protein were normalized by reference to those in wild-type cells before transfer of cells to SL, namely, at zero time. Values are means ± SD (bars) of results from three independent experiments. Asterisks indicate statistically significant differences compared to the levels at zero time (*P < 0.05; Student's t-test).
Specific Carotenoids Depress the Production of 1O2 During Acclimation to Strong Light
Zeaxanthin, echinenone, and myxoxanthophyll are effective scavengers of 1O2 (Young and Frank, 1996; Sandmann, 2019), and the scavenging abilities of these carotenoids are associated with the repair of PSII (Kusama et al., 2015). To monitor changes in levels of 1O2 during acclimation to strong light, we measured the rates of production of 1O2 by cells under strong illumination at 2,500 µmol photons m−2 s−1 in terms of the light-induced uptake of O2 in the presence of histidine (Kusama et al., 2015). When wild-type cells were transferred to SL, the rate of production of 1O2 increased 2.3-fold within 2 h; it returned to the initial level within 4 h; and it had fallen to 37% of the initial level by 12 h (Figure 6A). The production of 1O2 was unaffected by 3-(3,4-dichlorophenyl)-1,1-dimethylurea (DCMU), which blocks the photosynthetic transport of electrons, while it was abolished in the presence of NaN3, a quencher of 1O2, confirming the accurate detection of 1O2, as reported previously (Kusama et al., 2015). In ΔcrtRΔcrtO cells, the rate of production of 1O2 was 2.4 times higher than that in wild-type cells before the transfer of cells to SL (Figure 6B). After the transfer of cells from GL to SL, the rate of production of 1O2 increased 1.2-fold within 2 h, returned to the initial level within 4 h, and decreased to 67% of the initial level within 12 h (Figure 6B). Thus, it appeared that the presence of zeaxanthin, echinenone, and myxoxanthophyll might depress the production of 1O2 under strong light and, also, that the accumulation of these carotenoids during acclimation to strong light might suppress the production of 1O2 to an even greater extent.
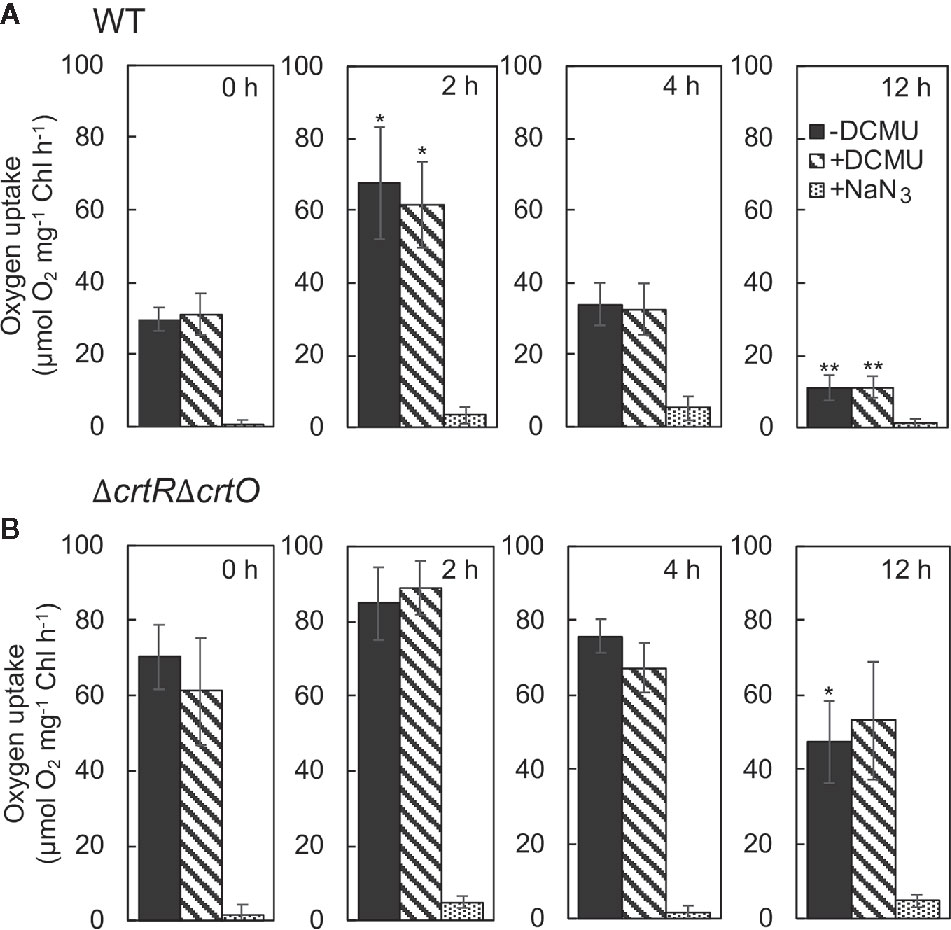
Figure 6 Changes in the rate of production of 1O2 in wild-type (A) and ΔcrtRΔcrtO (B) cells after transfer of cells from GL to SL. The production of 1O2 was monitored in terms of the difference between uptake of O2 in the presence of histidine and in its absence under strong light at 2,500 µmol photons m−2 s−1. The uptake of O2 was measured in the absence of DCMU, in the presence of 10 µM DCMU, and in the presence of 10 µM DCMU plus 10 mM NaN3. Values are means ± SD of results from three independent experiments. Asterisks indicate statistically significant differences compared to the levels at zero time (*P < 0.05; **P < 0.01; Student's t test).
Discussion
Involvement of Specific Carotenoids in the Protection of the Repair of PSII During Acclimation to Strong Light
Earlier studies found that mitigation of the photoinhibition of PSII during acclimation to strong light is associated with the enhanced repair of PSII in plants (Aro et al., 1993a), algae (Erickson et al., 2015), and cyanobacteria (Samuelsson et al., 1987; Jimbo et al., 2019). It was also reported that carotenoids are required for the assembly and photoprotection of PSII (Sozer et al., 2010; Hakkila et al., 2013). The present study revealed that the accumulation of specific carotenoids, namely, zeaxanthin, echinenone, and myxoxanthophyll, during acclimation to strong light is involved in the enhanced repair of PSII, with the resultant mitigation of photoinhibition of PSII in Synechocystis. However, even in the absence of such carotenoids, ΔcrtRΔcrtO cells were able to enhance the repair of PSII to some extent when grown under strong light (Figure 3). These results indicate that not only the accumulation of the specific carotenoids but also some other mechanism(s) might be responsible for the optimally enhanced repair of PSII during acclimation to strong light. Nevertheless, the significant decrease in the capacity for repair of PSII in ΔcrtRΔcrtO cells suggests that these specific carotenoids might play a crucial role in protection of the repair of PSII from inhibition under strong light, thereby allowing cells to survive under such conditions (Figure 1).
Mechanism of the Mitigation of Photoinhibition of PSII During Acclimation to Strong Light
The mitigation of photoinhibition of PSII, namely, the enhancement of repair of PSII, occurred in two distinct stages: the first stage occurred during the first 4 h, and the second stage occurred after 8 h (Figure 4). During the second stage, specific carotenoids, namely, zeaxanthin, echinenone, and myxoxanthophyll, accumulated at high levels, while the synthesis de novo of proteins, including the D1 protein, was activated, and the production of 1O2 was suppressed. Conversely, in the absence of these carotenoids, the second stage of mitigation of photoinhibition of PSII, the activation of protein synthesis, and the suppression of production of 1O2 were significantly impaired. These observations together suggest a mechanism by which carotenoids might protect the repair of PSII during acclimation to strong light as follows. High levels of accumulation of the specific carotenoids during the second stage of acclimation might protect protein synthesis by depressing the production of 1O2, with the resultant enhanced repair of PSII.
By contrast, we detected no obvious increase in levels of carotenoids during the first stage of mitigation of photoinhibition of PSII (Figure 4). What mechanism might be involved in this first stage of mitigation? Within the first 2 h after cells had been transferred from growth light to strong light, both the activity of PSII and the rate of synthesis of the D1 protein decreased, while the production of 1O2 rose. These features are typical of photoinhibition of PSII. During the next 2 h under strong light, by contrast, the activity of PSII and the rate of synthesis of the D1 protein rose, while the production of 1O2 decreased. These changes might involve other mechanisms that protect protein synthesis from photo-oxidative stress. Recent studies of the acclimation of Synechocystis to strong light revealed that levels of the translation factor EF-Tu, which is sensitive to oxidation by ROS, rise during acclimation to strong light and that the elevated levels of EF-Tu help to accelerate protein synthesis and enhance the repair of PSII under strong light (Jimbo et al., 2019). It seems likely that, during the first 4 h after transfer of cells from growth light to strong light, levels of EF-Tu might increase and contribute to enhanced repair of PSII. In addition, transfer of cells to strong light should stimulate the production of ATP and reducing power, which in turn enhances the repair of PSII via the acceleration of the synthesis of the D1 protein at both transcriptional and translational levels (Murata and Nishiyama, 2018). Thermal dissipation of excitation energy, which is a major component of non-photochemical quenching (NPQ), might also contribute to the enhanced repair of PSII during the first 4 h of acclimation. In Synechocystis, exposure of cells to strong light converts orange carotenoid protein (OCP) from its inactive form to its active form, stimulating the thermal dissipation of excitation energy (Wilson et al., 2006) and enhancing the repair of PSII (Takahashi et al., 2019). The impaired mitigation of photoinhibition of PSII in crtRΔcrtO cells might also be due, in part, to the lack of 3′-hydroxyechinenone, the cofactor of OCP, and the consequent loss of active OCP. Reduction in the size of the antenna complex, the phycobilisomes, might also minimize oxidative stress and enhance the repair of PSII via reduction of the transfer of excitation energy to the reaction center (Grossman et al., 1993; Kopečná et al., 2012). All these mechanisms might work together to enhance the repair of PSII not only during the first stage but also during the second stage of acclimation.
The Physiological Roles of Specific Carotenoids During Acclimation to Strong Light
Zeaxanthin, echinenone, and myxoxanthophyll are effective scavengers of 1O2 and free radicals (Sandmann, 2019). Their abilities to scavenge 1O2 in organic solvents are higher than that of β-carotene because of the presence of hydroxyl and glycosyl groups (Sandmann, 2019). These features might explain why these three specific carotenoids accumulate in abundance during acclimation to strong light. In ΔcrtRΔcrtO cells, the level of β-carotene was about twice that in wild-type cells and increased 1.5-fold after acclimation to strong light (Figure 2). Nonetheless, the impaired ability to repair PSII in ΔcrtRΔcrtO cells suggests that β-carotene cannot substitute for these three specific carotenoids in terms of the protection of the repair of PSII under strong light.
Most carotenoids are located in thylakoid and cytoplasmic membranes, although their precise localization within these membranes remains to be elucidated (Masamoto et al., 1999; Zhang et al., 2015). It seems likely that carotenoids that are localized within and in close vicinity to the reaction center of PSII quench the triplet state of chlorophyll to prevent the formation of 1O2, while other carotenoids scavenge 1O2 directly. Zeaxanthin, echinenone, and myxoxanthophyll at elevated levels are likely to act in this way to depress intracellular levels of 1O2, which is produced in abundance from PSII during the transfer of excitation energy under strong light.
As mentioned above, ΔcrtRΔcrtO cells did retain some ability to enhance the repair of PSII. We found that, in this mutant, deoxymyxoxanthophyll, a precursor to myxoxanthophyll, accumulated, with its level increasing 2.5-fold after the transfer of cells from growth light to strong light (Figure 2). The accumulation of deoxymyxoxanthophyll might, in part, contribute to the enhanced repair of PSII in the absence of zeaxanthin, echinenone, and myxoxanthophyll since this carotenoid also has a glycosyl group. The roles of myxoxanthophyll and deoxymyxoxanthophyll in the protection of the repair of PSII and their localization require further clarification.
Conclusion
During the acclimation of Synechocystis to strong light, specific carotenoids, namely, zeaxanthin, echinenone, and myxoxanthophyll, accumulate in abundance and enhance the repair of PSII, with the resultant mitigation of photoinhibition of PSII. The accumulation of these carotenoids, which occurs at the late stage of acclimation, depresses the production of 1O2 and thereby protects the synthesis de novo of proteins that are required for the repair of PSII, such as the D1 protein, under strong light.
Data Availability Statement
The datasets generated for this study are available on request to the corresponding author.
Author Contributions
TI performed most of the experiments. IK examined photoinhibition. HJ supervised the experiments. ST analyzed carotenoids. TI and YN conceived the project and wrote the article, with contributions from all the other authors.
Funding
This work was supported, in part, by a grant from the MIRAI Program of the Japan Science and Technology Agency (to YN); by a grant from the Japan Society for the Promotion of Science, KAKENHI (grant number JP18K06276 to YN); and by a grant from the Research Program of the “Dynamic Alliance for Open Innovation, Bridging Humans, the Environment and Materials” at the Network Joint Research Center for Materials and Devices (to YN).
Conflict of Interest
The authors declare that the research was conducted in the absence of any commercial or financial relationships that could be construed as a potential conflict of interest.
Acknowledgments
The authors thank Mr. Tomohisa Niimi (Saitama University) for the radioisotopic analysis of proteins.
Supplementary Material
The Supplementary Material for this article can be found online at: https://www.frontiersin.org/articles/10.3389/fpls.2020.01030/full#supplementary-material
References
Adams, W. W., Osmond, C. B., Sharkey, T. D. (1987). Responses of two CAM species to different irradiances during growth and susceptibility to photoinhibition by high light. Plant Physiol. 83, 213–218. doi: 10.1104/pp.83.1.213
Allakhverdiev, S. I., Murata, N. (2004). Environmental stress inhibits the synthesis de novo of proteins involved in the photodamage-repair cycle of photosystem II in Synechocystis sp. PCC 6803. Biochim. Biophys. Acta Bioenerg. 1657, 23–32. doi: 10.1016/j.bbabio.2004.03.003
Aro, E. M., McCaffery, S., Anderson, J. M. (1993a). Photoinhibition and D1 protein degradation in peas acclimated to different growth irradiances. Plant Physiol. 103, 835–843. doi: 10.1104/pp.103.3.835
Aro, E. M., Virgin, I., Andersson, B. (1993b). Photoinhibition of photosystem II. Inactivation, protein damage and turnover. Biochim. Biophys. Acta Bioenerg. 1143, 113–134. doi: 10.1016/0005-2728(93)90134-2
Asada, K. (1999). The water-water cycle in chloroplasts: scavenging of active oxygens and dissipation of excess photons. Ann. Rev. Plant Physiol. Plant Mol. Biol. 50, 601–639. doi: 10.1146/annurev.arplant.50.1.601
Domonkos, I., Kis, M., Gombos, Z., Ughy, B. (2013). Carotenoids, versatile components of oxygenic photosynthesis. Prog. Lipid Res. 52, 539–561. doi: 10.1016/j.plipres.2013.07.001
Ejima, K., Kawaharada, T., Inoue, S., Kojima, K., Nishiyama, Y. (2012). A change in the sensitivity of elongation factor G to oxidation protects photosystem II from photoinhibition in Synechocystis sp. PCC 6803. FEBS Lett. 586, 778–783. doi: 10.1016/j.febslet.2012.01.042
Erickson, E., Wakao, S., Niyogi, K. K. (2015). Light stress and photoprotection in Chlamydomonas reinhardtii. Plant J. 82, 449–465. doi: 10.1111/tpj.12825
Fernández-González, B., Sandmann, G., Vioque, A. (1997). A new type of asymmetrically acting β-carotene ketolase is required for the synthesis of echinenone in the cyanobacteriumSynechocystis sp. PCC 6803. J. Biol. Chem. 272, 9728–9733. doi: 10.1074/jbc.272.15.9728
Fujimori, T., Hihara, Y., Sonoike, K. (2005). PsaK2 subunit in photosystem I is involved in state transition under high light condition in the cyanobacterium Synechocystis sp. PCC 6803. J. Biol. Chem. 280, 22191–22197. doi: 10.1074/jbc.M500369200
Graham, J. E., Bryant, D. A. (2008). The biosynthetic pathway for synechoxanthin, an aromatic carotenoid synthesized by the euryhaline, unicellular cyanobacterium Synechococcus sp. strain PCC 7002. J. Bacteriol. 190, 7966–7974. doi: 10.1128/JB.00985-08
Grossman, A. R., Schaefer, M. R., Chiang, G. G., Collier, J. L. (1993). The phycobilisome, a light-harvesting complex responsive to environmental conditions. Microbiol. Rev. 57, 725–749. doi: 10.1128/MMBR.57.3.725-749.1993
Hakkila, K., Antal, T., Gunnelius, L., Kurkela, J., Matthijs, H. C. P., Tyystjärvi, E., et al. (2013). Group 2 sigma factor mutant ΔsigCDE of the cyanobacterium Synechocystis sp. PCC 6803 reveals functionality of both carotenoids and flavodiiron proteins in photoprotection of photosystem II. Plant Cell Physiol. 54, 1780–1790. doi: 10.1093/pcp/pct123
Hihara, Y., Sonoike, K., Ikeuchi, M. (1998). A novel gene, pmgA, specifically regulates photosystem stoichiometry in the cyanobacterium Synechocystis species PCC 6803 in response to high light. Plant Physiol. 117, 1205–1216. doi: 10.1104/pp.117.4.1205
Inoue, S., Ejima, K., Iwai, E., Hayashi, H., Appel, J., Tyystjärvi, E., et al. (2011). Protection by α-tocopherol of the repair of photosystem II during photoinhibition in Synechocystis sp. PCC 6803. Biochim. Biophys. Acta 1807, 236–241. doi: 10.1016/j.bbabio.2010.11.003
Jimbo, H., Yutthanasirikul, R., Nagano, T., Hisabori, T., Hihara, Y., Nishiyama, Y. (2018). Oxidation of translation factor EF-Tu inhibits the repair of photosystem II. Plant Physiol. 176, 2691–2699. doi: 10.1104/pp.18.00037
Jimbo, H., Izuhara, T., Hihara, Y., Hisabori, T., Nishiyama, Y. (2019). Light-inducible expression of translation factor EF-Tu during acclimation to strong light enhances the repair of photosystem II. Proc. Natl. Acad. Sci. U. S. A. 116, 21268–21273. doi: 10.1073/pnas.1909520116
Kojima, K., Oshita, M., Nanjo, Y., Kasai, K., Tozawa, Y., Hayashi, H., et al. (2007). Oxidation of elongation factor G inhibits the synthesis of the D1 protein of photosystem II. Mol. Microbiol. 65, 936–947. doi: 10.1111/j.1365-2958.2007.05836.x
Kopečná, J., Komenda, J., Bučinská, L., Sobotka, R. (2012). Long-term acclimation of the cyanobacteriumSynechocystis sp. PCC 6803 to high light is accompanied by an enhanced production of chlorophyll that is preferentially channeled to trimeric photosystem I. Plant Physiol. 160, 2239–2250. doi: 10.1104/pp.112.207274
Kusama, Y., Inoue, S., Jimbo, H., Takaichi, S., Sonoike, K., Hihara, Y., et al. (2015). Zeaxanthin and echinenone protect the repair of photosystem II from inhibition by singlet oxygen in Synechocystis sp. PCC 6803. Plant Cell Physiol. 56, 906–916. doi: 10.1093/pcp/pcv018
Latifi, A., Ruiz, M., Zhang, C. C. (2009). Oxidative stress in cyanobacteria. FEMS Microbiol. Rev. 33, 258–278. doi: 10.1111/j.1574-6976.2008.00134.x
Li, L., Aro, E. M., Millar, A. H. (2018). Mechanisms of photodamage and protein turnover in photoinhibition. Trends Plant Sci. 23, 667–676. doi: 10.1016/j.tplants.2018.05.004
Masamoto, K., Furukawa, K. I. (1997). Accumulation of zeaxanthin in cells of the cyanobacterium Synechococcus sp. strain PCC 7942 grown under high irradiance. J. Plant Physiol. 151, 257–261. doi: 10.1016/S0176-1617(97)80250-7
Masamoto, K., Misawa, N., Kaneko, T., Kikuno, R., Toh, H. (1998). β-carotene hydroxylase gene from the cyanobacterium Synechocystis sp. PCC 6803. Plant Cell Physiol. 39, 560–564. doi: 10.1093/oxfordjournals.pcp.a029405
Masamoto, K., Zsiros, O., Gombos, Z. (1999). Accumulation of zeaxanthin in cytoplasmic membranes of the cyanobacterium Synechococcus sp. strain PCC 7942 grown under high light condition. J. Plant Physiol. 155, 136–138. doi: 10.1016/S0176-1617(99)80155-2
Muramatsu, M., Hihara, Y. (2012). Acclimation to high-light conditions in cyanobacteria: from gene expression to physiological responses. J. Plant Res. 125, 11–39. doi: 10.1007/s10265-011-0454-6
Murata, N., Nishiyama, Y. (2018). ATP is a driving force in the repair of photosystem II during photoinhibition. Plant Cell Environ. 41, 285–299. doi: 10.1111/pce.13108
Nishiyama, Y., Yamamoto, H., Allakhverdiev, S. I., Inaba, M., Yokota, A., Murata, N. (2001). Oxidative stress inhibits the repair of photodamage to the photosynthetic machinery. EMBO J. 20, 5587–5594. doi: 10.1093/emboj/20.20.5587
Nishiyama, Y., Allakhverdiev, S. I., Yamamoto, H., Hayashi, H., Murata, N. (2004). Singlet oxygen inhibits the repair of photosystem II by suppressing the translation elongation of the D1 protein in Synechocystis sp. PCC 6803. Biochemistry 43, 11321–11330. doi: 10.1021/bi036178q
Rehman, A. U., Cser, K., Sass, L., Vass, I. (2013). Characterization of singlet oxygen production and its involvement in photodamage of photosystem II in the cyanobacterium Synechocystis PCC 6803 by histidine-mediated chemical trapping. Biochim. Biophys. Acta Bioenerg. 1827, 689–698. doi: 10.1016/j.bbabio.2013.02.016
Ritchie, R. J. (2006). Consistent sets of spectrophotometric chlorophyll equations for acetone, methanol and ethanol solvents. Photosynth. Res. 89, 27–41. doi: 10.1007/s11120-006-9065-9
Sae-Tang, P., Hihara, Y., Yumoto, I., Orikasa, Y., Okuyama, H., Nishiyama, Y. (2016). Overexpressed superoxide dismutase and catalase act synergistically to protect the repair of PSII during photoinhibition in Synechococcus elongatus PCC 7942. Plant Cell Physiol. 57, 1899–1907. doi: 10.1093/pcp/pcw110
Samuelsson, G., Lönneborg, A., Gustafsson, P., Öquist, G. (1987). The susceptibility of photosynthesis to photoinhibition and the capacity of recovery in high and low light grown cyanobacterium Anacystis nidulans. Plant Physiol. 83, 438–441. doi: 10.1104/pp.83.2.438
Sandmann, G. (2019). Antioxidant protection from UV- and light-stress related to carotenoid structures. Antioxidants 8, 219. doi: 10.3390/antiox8070219
Sozer, O., Komenda, J., Ughy, B., Domonkos, I., Laczkó-Dobos, H., Malec, P., et al. (2010). Involvement of carotenoids in the synthesis and assembly of protein subunits of photosynthetic reaction centers of Synechocystis sp. PCC 6803. Plant Cell Physiol. 51, 823–835. doi: 10.1093/pcp/pcq031
Steiger, S., Schäfer, L., Sandmann, G. (1999). High-light-dependent upregulation of carotenoids and their antioxidative properties in the cyanobacterium Synechocystis PCC 6803. J. Photochem. Photobiol. B. Biol. 52, 14–18. doi: 10.1016/S1011-1344(99)00094-9
Takahashi, H., Kusama, Y., Li, X., Takaichi, S., Nishiyama, Y. (2019). Overexpression of orange carotenoid protein protects the repair of PSII under strong light in Synechocystis sp. PCC 6803. Plant Cell Physiol. 60, 367–375. doi: 10.1093/pcp/pcy218
Takaichi, S., Mochimaru, M. (2007). Carotenoids and carotenogenesis in cyanobacteria: unique ketocarotenoids and carotenoid glycosides. Cell. Mol. Life Sci. 64, 2607–2619. doi: 10.1007/s00018-007-7190-z
Takaichi, S., Maoka, T., Masamoto, K. (2001). Myxoxanthophyll in Synechocystis sp. PCC 6803 is myxol 2′-dimethyl-fucoside, (3R,2'S)-myxol 2′-(2,4-di-O-methyl-alpha-L-fucoside), not rhamnoside. Plant Cell Physiol. 42, 756–762. doi: 10.1093/pcp/pce098
Theis, J., Schroda, M. (2016). Revisiting the photosystem II repair cycle. Plant Signal. Behav. 11, e1218587. doi: 10.1080/15592324.2016.1218587
Vass, I. (2012). Molecular mechanisms of photodamage in the photosystem II complex. Biochim. Biophys. Acta Bioenerg. 1817, 209–217. doi: 10.1016/j.bbabio.2011.04.014
Wellburn, A. R. (1994). The spectral determination of chlorophylls a and b, as well as total carotenoids, using various solvents with spectrophotometers of different resolution. J. Plant Physiol. 144, 307–313. doi: 10.1016/S0176-1617(11)81192-2
Wilson, A., Ajlani, G., Verbavatz, J. M., Vass, I., Kerfeld, C. A., Kirilovsky, D. (2006). A soluble carotenoid protein involved in phycobilisome-related energy dissipation in cyanobacteria. Plant Cell 18, 992–1007. doi: 10.1105/tpc.105.040121
Young, A. J., Frank, H. A. (1996). Energy transfer reactions involving carotenoids: quenching of chlorophyll fluorescence. J. Photochem. Photobiol. B. Biol. 36, 3–15. doi: 10.1016/S1011-1344(96)07397-6
Yutthanasirikul, R., Nagano, T., Jimbo, H., Hihara, Y., Kanamori, T., Ueda, T., et al. (2016). Oxidation of a cysteine residue in elongation factor EF-Tu reversibly inhibits translation in the cyanobacterium Synechocystis sp. PCC 6803. J. Biol. Chem. 291, 5860–5870. doi: 10.1074/jbc.M115.706424
Keywords: acclimation, carotenoids, singlet oxygen, photoinhibition, photosystem II
Citation: Izuhara T, Kaihatsu I, Jimbo H, Takaichi S and Nishiyama Y (2020) Elevated Levels of Specific Carotenoids During Acclimation to Strong Light Protect the Repair of Photosystem II in Synechocystis sp. PCC 6803. Front. Plant Sci. 11:1030. doi: 10.3389/fpls.2020.01030
Received: 18 March 2020; Accepted: 23 June 2020;
Published: 07 July 2020.
Edited by:
Cornelia Spetea, University of Gothenburg, SwedenReviewed by:
Josef Komenda, Academy of Sciences of the Czech Republic, CzechiaTaina Tyystjärvi, University of Turku, Finland
Copyright © 2020 Izuhara, Kaihatsu, Jimbo, Takaichi and Nishiyama. This is an open-access article distributed under the terms of the Creative Commons Attribution License (CC BY). The use, distribution or reproduction in other forums is permitted, provided the original author(s) and the copyright owner(s) are credited and that the original publication in this journal is cited, in accordance with accepted academic practice. No use, distribution or reproduction is permitted which does not comply with these terms.
*Correspondence: Yoshitaka Nishiyama, bmlzaGl5YW1hQG1haWwuc2FpdGFtYS11LmFjLmpw