- 1Laboratorio Nacional de Identificación y Caracterización Vegetal (LaniVeg-CONACYT), Departamento de Botánica y Zoología, Centro Universitario de Ciencias Biológicas y Agropecuarias, Universidad de Guadalajara, Zapopan, Mexico
- 2Maestría en Ciencias en Recursos Naturales y Desarrollo Rural, Colegio de la Frontera Sur, Tapachula, Mexico
- 3Cátedras CONACYT-Universidad de Guadalajara, Laboratorio Nacional de Identificación y Caracterización Vegetal (LaniVeg), Departamento de Botánica y Zoología, Centro Universitario de Ciencias Biológicas y Agropecuarias, Universidad de Guadalajara, Zapopan, Mexico
- 4Maestría en Biosistemática y Manejo de Recursos Forestales y Agrícolas, Centro Universitario de Ciencias Biológicas y Agropecuarias, Universidad de Guadalajara, Zapopan, Mexico
Forestry systems in Mexico are examples of traditional management of land and biodiversity that integrates the use, conservation and restoration of forest elements. Current in situ management practices of Agave maximiliana in western Mexico include the tolerance of many forest elements, reintroduction of young Agave plants and germination of seeds. More intense forms of management include monocultures, which are agroindustrialized systems developed in more recent times and characterized by the establishment of high densities of A. maximiliana plants in deforested areas and abandoned agricultural lands. We compared monocultures, forestry systems and wild populations (i.e., non/slightly-exploited forests) in order to evaluate whether these practices have had an effect on intraspecific morphological and genetic variation and divergence. We also tested whether divergence has a positive relationship with environmental and geographic distance. We analyzed 16 phenotypic traits in 17 populations of A. maximiliana, and 14 populations were further examined by amplifying 9 SSR loci. We employed multivariate methods and analyses of variance in phenotypic and genetic traits to test whether clusters and the percentage of variation contained in the managed and wild categories can be identified. Tests of isolation by environment (IBE) and distance (IBD) were performed to detect the magnitude of divergence explained by climatic and geographic variables. We found that forestry systems are effective as reservoirs of morphological and genetic diversity, since they maintain levels similar to those of wild populations. Moreover, the monocultures showed similar levels, reflecting their recent emergence. While the species showed high morphological diversity (IMD = 0.638, SE ± 0.07), it had low to intermediate genetic diversity (A = 2.37, HE = 0.418). Similar morphological and genetic divergences were found among populations, but these were not correlated with each other in population pairs. Non-significant morphological differentiation was found among categories. Only IBE was significant in the genetic structure (β = 0.32, p = 0.007), while neither IBE nor IBD was detected in the morphological differentiation. We discuss the implications of these results in the context of the weaknesses and strengths of A. maximiliana in the face of the socio-ecological changes predicted for the study area in the short term.
Introduction
Agaves have considerable cultural, economic and ecological importance in North America (Gentry, 1982). Around fifty three species of Agave L. are used for mescal production (Colunga-GarcíaMarín and Zizumbo-Villarreal, 2007; Torres et al., 2015b); however, most of the plants utilized are not cultivated but rather extracted from natural populations by the simple gathering of plants (ca. 37 spp., Torres et al., 2015b). Of these, 53 species used for mescal production, around 12 species of Agave are managed in situ (Torres et al., 2015b), i.e., a range of practices other than simple gathering is conducted. These populations managed in situ constitute valuable Forestry (FS) and Agroforestry (AFS) Systems maintained in Mexico. The terms FS and AFS refer to a land utilization type with several criteria of classification (Nair, 1985). In Mexico, these criteria are based on a traditional management of land and biodiversity that integrates the use, conservation and restoration of multiple useful species of domesticated and wild plants and animals for different purposes (Moreno-Calles et al., 2013). While AFS represent alternatives to the current problems relating to the management and conservation of biodiversity (Torres-García et al., 2019), Agave monocultures have had an impact on traditional ecological knowledge and agrobiodiversity, while also depending heavily on the use of toxic agrochemicals (Bowen and Gerritsen, 2007). These agroindustrial management forms are characterized by the establishment of high densities of a single species for one simple purpose in deforested areas and/or abandoned agricultural lands (Torres-García et al., 2019). Less than ten Agave species are maintained in monocultures (Torres et al., 2015b). Monocultures of Agave tequilana F.A.C. Weber Azul, used in the spirits industry for the production of tequila, have spread rapidly in western Mexico, particularly from the end of the 20th century up to the present day (Leclert et al., 2010).
Analyses based on genetic and phenotypic markers have revealed that differences between wild and managed plant populations depend on life history traits, geographic distribution and cultivation history (Lindsay et al., 2018), as well as on the forms and intensities of management. These constitute an extraordinary broad spectrum of expressions of management types (Casas et al., 1999, 2017; Otero-Arnaiz et al., 2005; Illsey et al., 2007; Blancas et al., 2009, 2013; Parra et al., 2010, 2012; Guillén et al., 2011; Cruse-Sanders et al., 2013; Contreras-Negrete et al., 2015; Torres et al., 2015a). On one hand, agave species with a dominance of vegetative reproduction, form the primary gene pools from which a high diversity of traditional landraces have been artificially selected (A. angustifolia Haw. and A. rhodacantha Trel., Vargas-Ponce et al., 2007, 2009; Rivera-Lugo et al., 2018; Trejo et al., 2018). Vegetative reproduction can also become dominant in managed populations, although it is not a common reproductive system in wild populations (A. parryi Engelm., Parker et al., 2010) or in their wild relatives (A. hookeri Jacobi, Figueredo-Urbina et al., 2017). This can, in turn, produce faster morphological divergences between wild and managed populations (Parker et al., 2010, 2014; Figueredo et al., 2014; Figueredo-Urbina et al., 2017). The constant reintroduction of wild germplasm to traditional landraces and high exchange of germplasm between farmers can maintain high levels of genetic diversity (A. hookeri, Figueredo-Urbina et al., 2017; A. angustifolia, A. rhodacantha, Vargas-Ponce et al., 2009), compared to the wild populations.
On the other hand, species that present sexual reproduction, found in wild populations or managed in FS and monocultures, have been studied to a lesser extent. While morphological divergences can be detected between wild and managed species (A. inaequidens K. Koch, A. cupreata Trel. & A. Berger, Figueredo et al., 2014; Figueredo-Urbina et al., 2017), the genetic divergences explained by management have been reported as null (A. inaequidens, A. cupreata, Figueredo-Urbina et al., 2017) or incipient (A. potatorum Zucc., Félix-Valdez et al., 2016). This reflects the fact that the human activities practiced in these systems are still governed by natural gene flow.
Wild and domesticated Agave spp. used for the production of spirits have paniculate inflorescences, a frequent nocturnal anther dehiscence and, in some cases, occur in geographic areas that overlap with those of the long-nosed bats (Arita, 1991). These aspects suggest the occurrence of chiropterophily (Molina-Freaner and Eguiarte, 2003; Rocha et al., 2006; Estrella-Ruiz, 2008; León, 2013). Indeed, their strong relationship with the bats could have driven the radiation of the genera and their close linages (Flores-Abreu et al., 2019). These bats present a foraging behavior over a wide geographical range (e.g., Leptonycteris curasoae Miller, 1900, L. yerbabuenae, Martinez and Villa, 1940, of distances of up to 100 km, Horner et al., 1998; Medellín et al., 2018) which is congruent with the low genetic differentiation presented by some Agave species used for the production of spirits, and presenting sexual reproduction, (e.g., A. inaequidens, A. palmeri Engelm., A. cupreata, Table 1). Since this dynamic maintains highly diverse gene pools, monocultures of sexually reproduced plants lose genetic diversity at a slower rate (see A. inaquidens, A. cupreata, wild vs. monocultures, Table 1) compared to those of vegetative propagation (see A. angustifolia vs. A. tequilana; A. inaequidens vs. A. hookeri, Table 1).
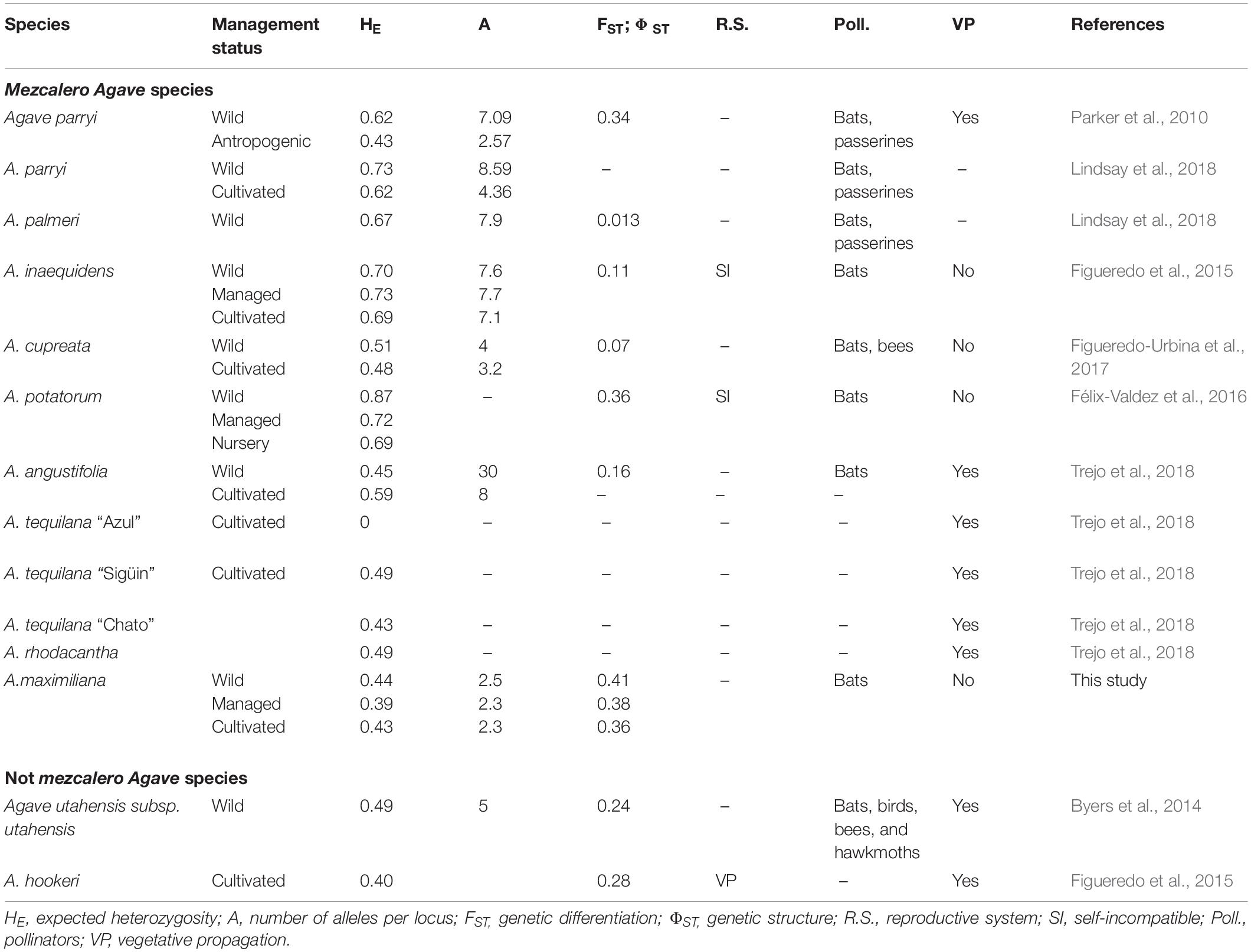
Table 1. Genetic diversity (SSRs) and population differentiation parameters, pollination vectors and reproductive system of Agave species.
In this study, we explored the case of Agave maximiliana Baker growing in pine-oak forest in western Mexico. This is one of the Agave species used in Jalisco for the production of spirits other than tequila. The spirit known as “raicilla” forms part of the history in this region thanks to mining, an important activity during the colonial period, when raicilla was in high demand but also prohibited by the Spanish crown, along with all agave spirits at that time (Valenzuela-Zapata, 2015). The spirit is known as “raicilla de la sierra” (hereafter, “raicilla”) corresponding to a highland region, and distinguishable from “raicilla de la costa,” which is produced using other Agave species (A. rhodacantha and A. angustifolia) in the coastal region of Jalisco. The historical impact of this activity on A. maximiliana populations has never been evaluated, and no landraces or ecotypes have been documented.
Recently, our research group carried out studies through structured interviews. The study showed that most of the people who produce raicilla have actually learned the tradition transversally; i.e., not as a result of inherited knowledge. It appears that there has been a resurgence of interest in this activity and that the management practices of this species are therefore probably recent (Huerta-Galván, 2018). In addition, the study showed that nearly 90% of people interviewed conduct “promotion,” a management practice that conserves many forest elements, such as pines and oaks that are maintained for the protection of the Agave plants against frost and pests. This action is a common element of Agave management in Mexico, especially on species managed at elevations of 800–2,500 m.a.s.l (e.g., A. inaequidens, A. cupreata), where frosts, are light and fleeting, but where some species such as A. maximiliana are frequently damaged (Gentry, 1982). The management of agave plants and native trees, both elements of the forest, are therefore combined in areas known as “forestry systems (FS)” which differ from AFS by the absence of domesticated crop elements (Nair, 1985; Torres-García et al., 2019). “Promotion” also includes the reintroduction of young plants (3 years of age) to the forest, by collecting seeds from multiple wild plants, the origin of which is located at a linear distance of less than 10 km, or buying plants from other producers. Most people also germinate seeds, the resulting seedlings of which are grown in their backyards and remove weeds from their FS or monocultures, either manually with tools or through the use of herbicides. Some people conduct simple gathering, where they establish closed seasons to allow population regeneration, and some few (nearly 30%) grow their plants in monocultures, which is a much more recent development over the last 15–30 years since the current boom in mezcal began (Huerta-Galván, 2018). The current FS therefore provide most of the raw material that sustains raicilla production in this temperate region. Monocultures are characterized by a high transformation of the habitat, with no associated native trees within these systems, and greater dependence on the use of agrochemicals. It is notable that there is no clear criteria regarding what traits are selected, except for one producer who selects large plants with the highest sugar content in the leaves. Management practice details and associated native tree species, where applicable, are presented in Supplementary Material SM1 for monocultures, forestry systems and wild populations. It is also important to mention that, while FS tolerate several tree elements naturally occurring in these habitats, a qualitative observation is that the density of shrub elements may be lower compared to that of wild populations. In contrast, the density of agave plants is generally higher in FS than in wild populations (Figure 1). While human actions are more evident in FS than in wild populations, it is important to mention that the latter are not pristine: they are found in accessible sites and present signs of light exploitation. Most of these populations present a small number of plants with the inflorescence removed, as these are occasionally sold as food.
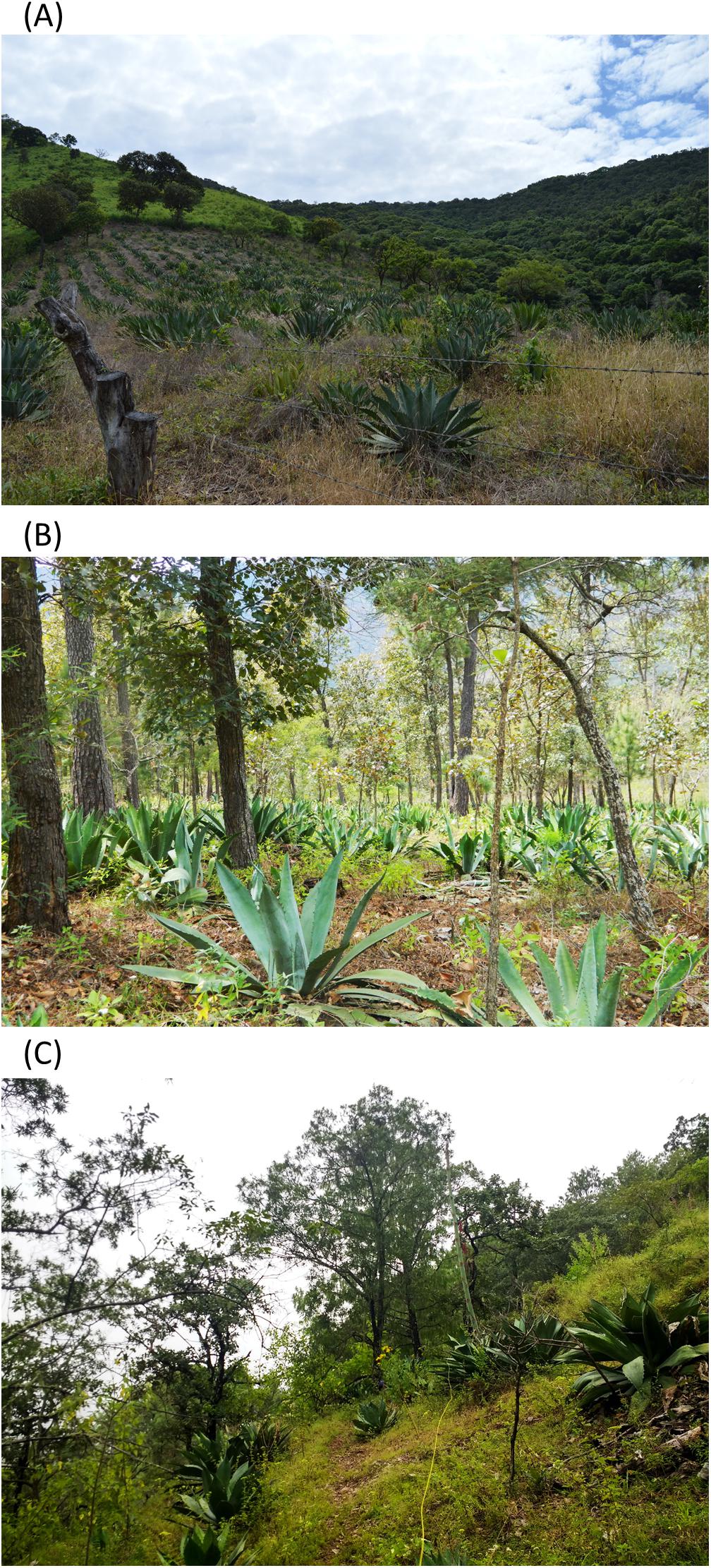
Figure 1. Categories of studied populations of Agave maximiliana. (A) Monoculture “El Mosco,” (B) Forestry System “Rincón de Mirandillas,” (C) Wild population “El Palmito.” Photos by: SA-R, DC-T and OH-G, respectively.
A common perception among raicilla producers is that a drastic decrease (60–80%) has occurred in wild populations in the region in the last 30 years (stated by 60% of the informants in Huerta-Galván, 2018). If these perceptions reflect a true decrease, genetic bottlenecks and demographic stochasticity should be expected (Luikart and Cornuet, 1998; Frankham et al., 2006) in A. maximiliana populations. The plants are harvested prior to flowering, thus precluding the incorporation a high proportion of new individuals from one generation to the next (cf. Aguirre-Dugua and Eguiarte, 2013; Torres et al., 2015b). Moreover, the density of flowering plants is an important attractant for pollinators of Agave (Estrella-Ruiz, 2008) such that, even when some plants are allowed to flower, these may still be insufficient in number to maintain an effective pollination dynamic. Thus, overexploitation promotes population decline, leading to some cases of local extinction (e.g., Agave potatorum, Delgado-Lemus et al., 2014). There are no studies addressing the pollination system in A. maximiliana but, given the general aspects described above, i.e., paniculate inflorescences, probable nocturnal anthesis dehiscence and geographic area overlapping with that of Leptonycterys yerbabuenae, L. nivalis Saussure, 1860 (Arita, 1991) and Choeronycteris mexicana Tschudi, 1844 (Arroyo-Cabrales et al., 1982; Ortega-García et al., 2017), we infer a chiropterophilous syndrome, with these bat species as likely pollinators.
Finally, we envision social changes for the management of A. maximiliana in the short term, given that raicilla recently obtained its denomination of origin (DOR, Diario Oficial de la Federación, 2019). Considering the case of tequila production in the state of Jalisco, where an increased demand for plants to produce this spirit occurred following its DOR (Bowen and Valenzuela-Zapata, 2009; Leclert et al., 2010), we consider that a similar increase in the demand of plants for raicilla production is highly probable.
In summary, we consider that a recent but dynamic management exists in A. maximiliana, where increased population density of this species is a priority, along with the conservation of dominant forest elements, the benefits of which are still recognized by most of the producers; seeds and plants from multiple sites in the region are constantly reintroduced to forestry systems and monocultures and a high exchange of these germplasm also takes place among producers. These actions have acted to maintain, to date, the forestry systems that in turn support most raicilla production. Such systems conserve many elements of the original habitat and they are still governed, to some extent, by the environmental and geographical location effects of the natural landscapes. This is in contrast to the agricultural systems, i.e., monocultures, that present higher levels of habitat modification and more artificially controlled natural processes (e.g., pests, germplasm origin, density and reproduction of plants, etc.). Finally, we assume that the sexual reproductive system and probably chiropterophilous pollination system of A. maximiliana are biological strengths that have acted to maintain the connectivity of populations through gene flow.
In the context of these observations and assumptions, we aim to determine: (1) whether the methods and intensity of the management practices realized in A. maximiliana populations have had an effect on their intraspecific diversity; and (2) whether isolation by environment (IBE, Lichstein, 2007; Wang, 2013) and by distance (IBD) are significant in this species. We hypothesized that: (1) the recent management context will favor morphological and genetic diversity in this species, where divergence among categories is incipient, and thus we expect high morphological and genetic diversity for the species, with equal or higher levels in forestry systems compared to monocultures and wild populations and low differentiation among management categories; (2) while an assumed wide geographical range of the foraging behavior of the candidate pollinators of A. maximiliana will be reflected in a high genetic connectivity, the influence of geographical and environmental factors will have a positive relationship with the morphological and genetic divergences; therefore, we expect low genetic differentiation among populations and significant isolation by distance (IBD) and by environment (IBE). This is to say, the greater the geographical distance and the environmental differentiation, the greater the genetic and morphological divergence. Our purpose was to delimit and define the state of diversity and intraspecific differentiation of A. maximiliana growing in western Mexico in order to identify biological, ecological and management weaknesses and strengths in the face of imminent socioecological changes in the region.
Materials and Methods
Study Species
Agave maximiliana, known locally as “Lechuguilla,” is endemic to Mexico. It commonly inhabits rocky slopes in the states of Jalisco, Sinaloa, Durango, Nayarit, Colima and Zacatecas, at 900–2,000 m.a.s.l, and requires an annual precipitation of 750–1,000 mm (Gentry, 1982). It is frequent in pine-oak forests on calcareous soils or on those derived from igneous rock (Gentry, 1982; González-Elizondo et al., 2009). It is a perennial single rosette, monocarpic and medium size plant, balled or open, that habitually lacks asexual reproduction. Its leaves are broadly lanceolate, curved or straight, soft fleshy, with heteromorphic teeth of greater size toward the middle part of the leaf. The inflorescence is paniculate, of 5–8 m in height, branched, and with 15–30 small umbels. The greenish-yellow flowers are 52–65 mm in length. The fruits are small capsules of 3.5–5 × 1.7–2 cm, and are short oblong, stipitate, with rounded apex. The seeds measure 5.5–6 × 4.5–5 mm, the testa wavy, finely punctate, with marginal wing abruptly raised (Gentry, 1982; Vázquez-García et al., 2007). Gentry (1982) recognized two varieties with an overlapped distribution (Figure 2): A. maximiliana var. maximiliana and A. maximiliana var. katherinae (A. Berger) Gentry. The latter differs from the typical variety by its larger rosettes, greener leaves with more undulate repand margins with numerous variable interstitial teeth, and a deeper flower tube. However, McVaugh (1989) treated A. maximiliana var. katherinae as synonymous of A. maximiliana sensu stricto. Recently, Scheinvar (2018) defined the current potential distribution maps of both varieties and indicated that A. maximiliana var. katherinae could occupy the northern windward zone of the Sierra Madre Occidental (SMO), while A. maximiliana var. maximiliana could be found in the southern leeward zone of the SMO, but also in the Sierra Madre del Sur (SMS) and Transmexican Volcanic Belt (TVB) (Morrone et al., 2017). We perceived a wide morphological variation within populations in all sites sampled and decided to use A. maximiliana s.l. as recognized by McVaugh (1989).
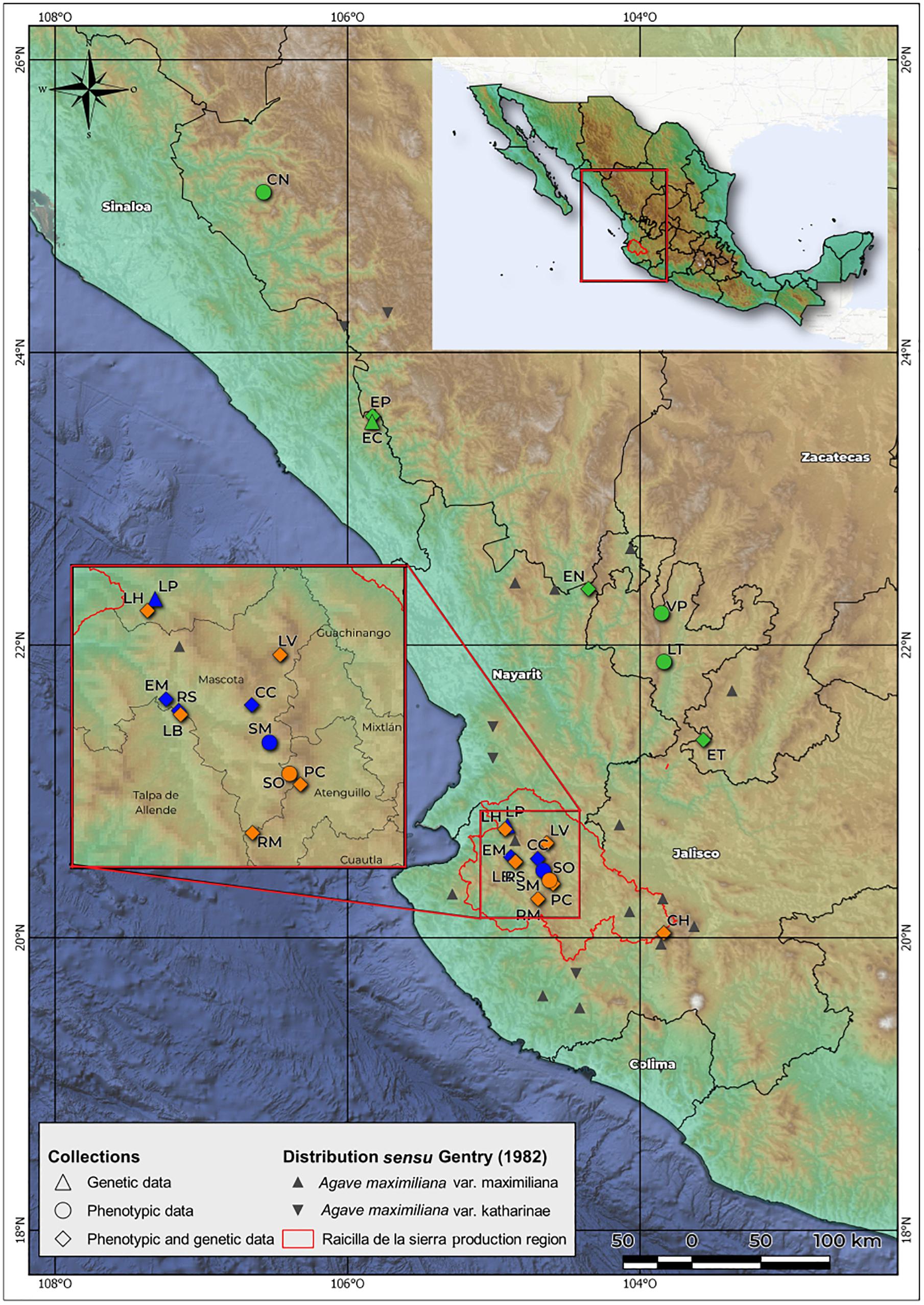
Figure 2. Geographical location of sampled populations. Green: wild populations. Orange: managed populations. Blue: cultivated populations. Raicilla de la sierra production region is the area in which raicilla is distilled and the A. maximiliana populations are apparently overexploited.
Study Area, Populations Sampled and Categorization
In accordance with our hypothesis, core populations of our study area were collected in western Jalisco in municipalities that produce raicilla (Figure 2). Populations sampled outside this area, in the states of Zacatecas, Nayarit, Sinaloa, and Durango, were considered wild populations (hereafter, “wild”) with little or no evidence of exploitation; these were used in the study as an external reference and for comparison of management gradients. Forestry systems (hereafter, “managed”) are those in which one or several of the practices described above are carried out (sensu Huerta-Galván, 2018). The monocultures (hereafter, “cultivated”) were established very recently, from around 30 or less years ago. The farmers use land in open areas that were previously used for other monocultures, usually maize. Agave rows are planted, almost always in lines following the slope. Agrochemicals are added to fertilize the plants or to eliminate weeds. Some farmers also apply chemicals on the leaves to combat pests. Monocultures have a high density of plants compared to plantations managed under the forest, and are characterized by a drastic change in land use. The seeds used for monocultures are collected from plants in the forest, domestic cultivation and in vitro propagation. This latter propagation type is currently carried out by a single producer only, who obtains 2–10 seedlings from each seed. This strategy is a response to the scarce availability of seeds and plants in recent years (Huerta-Galván, 2018).
Morphological data were obtained from 17 populations and the genetic analyses included microsatellite markers from 14 populations. For some populations, it was not possible either to obtain successful amplifications or to collect morphological data or leaf tissue (see Table 2). Finally, 12 populations were analyzed for environmental and geographic analyses using both genetic and morphological data.
Data Analyses
To answer our research questions, the following general strategy was adopted: For testing the contribution of management to the diversity and differentiation of A. maximiliana populations, we first calculated levels of diversity in the morphological traits and tested for differences among categories. We then employed multivariate methods and permutational analysis of variance to test whether clusters and the percentage of variation contained among cultivated, managed and wild categories can be identified. Analogous methods were conducted for genetic traits where differentiation among populations was also important in terms of detecting the contribution of gene flow. Finally, to identify the contribution of geographical distribution and environmental factors to the morphological and genetic divergences, we determined the IBD and IBE.
Diversity and Differentiation of Morphological Traits
Ten adult individuals were selected per population. Only plants with reproductive structures or visible indications of imminent reproductive maturity were selected, following the criteria of the producers. In total, 16 quantitative morphological traits were evaluated, as proposed by Vargas-Ponce et al. (2007) and Figueredo-Urbina et al. (2017) for agaves (Table 3). These traits are vegetative characters, since the presence of inflorescences is rare in cultivated and managed populations, and vegetative rather than floral characters are the targets of artificial selection. In each individual, three leaves of the mid verticil were measured in order to record variation and obtain mean values for these traits. Measurements were taken with a flexometer (resolution ± 0.001 m) and a ruler of 30 cm in length, and the data were used to construct a matrix for subsequent analysis. To discriminate the correlated morphological traits, a Pearson correlation test (r ≥ 0.9) was used following the criteria of Valdivia-Mares et al. (2016). Four traits (i.e., minimum diameter (MinD), leaf length (LL), leaf width at middle (LWm) and number of teeth in 10 cm2 (NT10) were excluded from the analysis because they presented multicollinearity (Table 3 and Supplementary Material SM2).
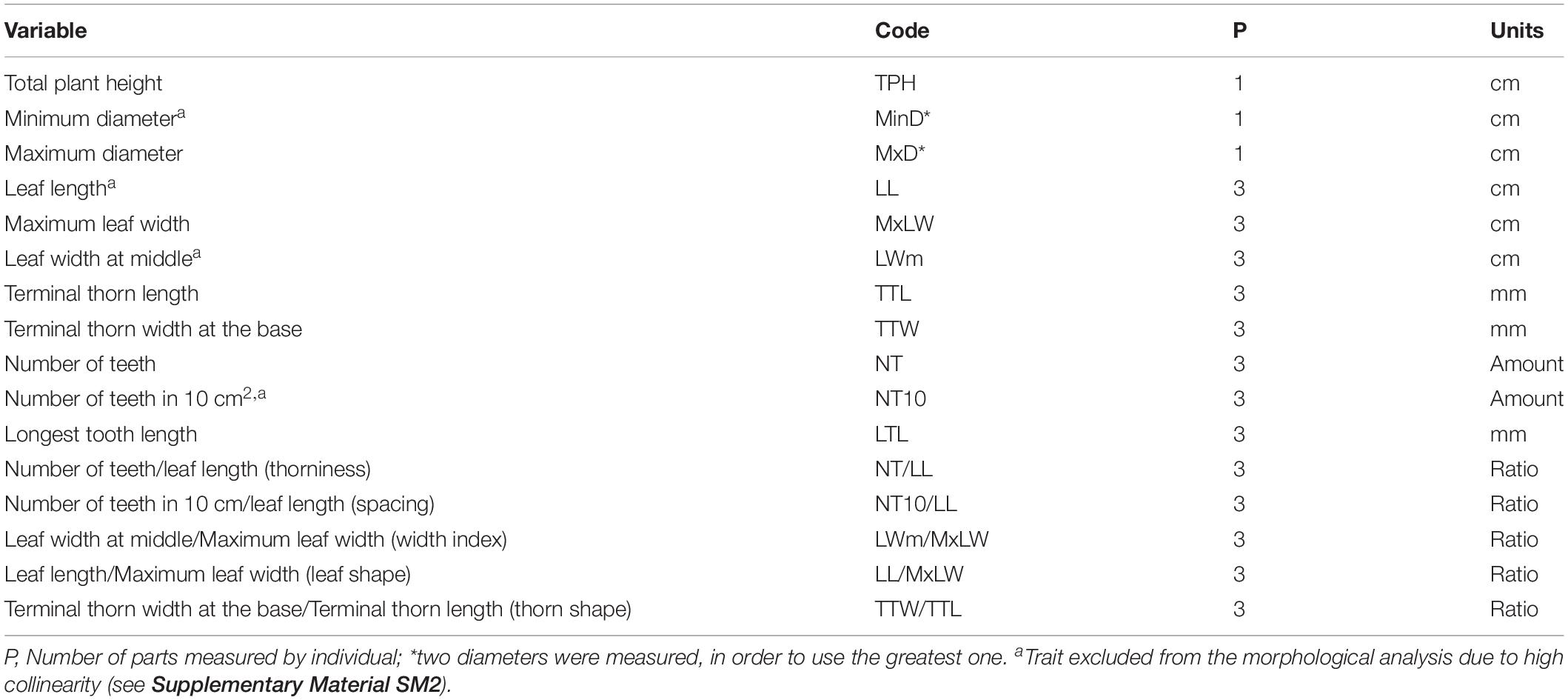
Table 3. Vegetative characters measured for wild, managed and cultivated populations of Agave maximiliana.
To analyze the morphological diversity per population and predicted management category, the Index of Morphological Diversity (IMD) was estimated. For this, the traits with the highest eigenvalues in the LDA (explained below) were selected in order to utilize those that better explained morphological diversity. Individual plant values of each trait from all populations were assigned to different morphological states according to the Sturges rule (Daniel, 2006). This procedure produced a matrix of discrete values and the IMD was estimated based on the Simpson Diversity Index (SDI). This index was defined as IMD = 1−Σ1−s(pi)2, in which pi is the proportion of the total number of individual plants sampled in a population showing the ith state of a morphological trait (categorized with the Sturges rule) and s is the number of states of that character (Casas et al., 2006). In this way, a unique estimator of the mean morphological diversity was obtained for all traits per population and category (cf. Figueredo-Urbina et al., 2017). The existence of significant differences among management categories by IMD was evaluated using a Kruskal Wallis test in Primer v6.1.11 (Anderson, 2017).
To estimate the multivariate differentiation among groups and determine which morphological traits have greater influence on the dispersion of the variation, a Linear Discriminant Analysis (LDA) was performed. For this, the database was standardized with the expression Y0 = (Y–a)/b); where Y0 is the standardized value, Y is the observed value of the state of a character, a is its mean and b is its standard deviation (Figueredo-Urbina et al., 2017). From the LDA, the centroids were obtained for each population, paired Euclidean distances were calculated and random resampling conducted to construct the null model (with 10,000 replicates) as a test of significance. To corroborate the identity of the cultivated plants, on the assumption that cultivated plants came mainly from managed rather than wild populations (if either the farmers collected seeds or bought the whole plant), we cross-validated the population assignation of each individual, from which we obtained a confidence value (i.e., the probability of correctly classifying an individual with respect to its population of origin). With these values and the predict function obtained from the LDA, we estimated the probable population of origin for each cultivated individual in monocultures (Legendre and Legendre, 1998; Medina-Villarreal and González-Astorga, 2016). All analyses were performed in R (R Development Core Team, 2012).
In order to determine whether the existing set of phenotypes differs significantly among management categories, a nested two-way permutational multivariate analysis of variance (PERMANOVA) was conducted with a type III (mixed effects) model: a fixed factor (condition) with three levels (wild, managed and cultivated) and a random factor (populations) with 17 levels. A PERMANOVA was performed with the records obtained from twelve non-correlated traits in Primer v6.1.11/PERMANOVA+ v1.0.1 (Anderson et al., 2008). First, a pretreatment was conducted in order to standardize the data (z-values) of the 12 traits analyzed, in order to transform the variables of different nature and measurement units to the same scale. A matrix of Euclidean distances was then constructed and the PERMANOVA performed. The statistical significance of the analysis was tested with 10,000 permutations based on a type III sum of squares. Likewise, the general patterns of morphological variation of the studied populations in each management category were analyzed using a Principal Coordinates Analysis (PCoA, Legendre and Legendre, 1998). This PCoA was performed based on the mean values per population of the 12 morphological traits used in the PERMANOVA. Therefore, the same pretreatment and Euclidian distance considered in the PERMANOVA design were used.
Diversity and Differentiation of Genetic Traits
From each population, samples of plant tissue were collected from 15 individuals, transported at 4°C and stored at −45°C. From this tissue, DNA was extracted following Doyle (1991), modified with sodium chloride-tris-EDTA (STE). The DNA was quantified with a spectrophotometer and its quality evaluated by absorbance at 260/280 ng/μL. Dilutions at 40 ng/μL of all samples were prepared. A total of 16 microsatellites were tested; seven described by Lindsay et al. (2012), four by Parker et al. (2010) and five designed by Cabrera-Toledo (unpublished data) from the genome of A. tequilana. Only nine microsatellites were reproducible and polymorphic (Supplementary Material SM3). Amplification by PCR was conducted using the Multiplex PCR kit with TaqMan® (Qiagen) in a final reaction volume of 11 μL. Each reaction contained 120 ng of DNA, 4 μL of Multiplex, 0.8 μL of each primer (10 mM) and 2.4 μL of molecular grade water (MiliQ). The amplification conditions were: 15 min at 95°C, followed by 40 cycles of 30 s at 94 °C, 30 s at the annealing temperature of each primer (Supplementary Material SM3) and an extension step of 2 min at 72°C, followed by a final extension step of 30 min at 60°C. The amplification was corroborated in 1.5% agarose gels with 1X TBE buffer. The PCR products of each primer per population were separated by vertical electrophoresis in 8% polyacrylamide gels (1–1.5 mm thickness) without urea. Each gel was left to run for 5 h. A 100 bp marker (Invitrogen) was used as a reference, and silver staining was used to visualize the bands (Sanguinetti et al., 1994). The gels were photo-documented with the software Gel Logic 100, Kodak and the fragment size in base pairs of the amplified bands was determined in Phoretix 1D v10 (Total lab Ltd.).
To analyze the genetic diversity, five parameters were evaluated: percentage of polymorphic loci (P), mean number of alleles per locus (A), mean number of effective alleles per locus (AE), observed heterozygosity (HO), expected heterozygosity (HE) under Hardy-Weinberg equilibrium (HWE). Also, we performed HWE analysis based on the X2 test (GenAlex 6.5, Peakall and Smouse, 2006). A Kruskal-Wallis test was conducted (SigmaPlot, v 13.0) to evaluate the significance of the differences in genetic diversity among management categories. The fixation indices FIS and FIT of Wright (1965) were used to evaluate inbreeding. To evaluate the possible effect of null alleles on the levels of inbreeding, a Bayesian Individual Inbreeding Model (IIM) analysis was performed in INest 2.2 (Chybicki and Burczyk, 2009). This permits non-biased calculation of the coefficient of inbreeding for multilocus data in the presence of null alleles (Fi). This analysis evaluates the effect of null alleles (n), inbreeding (f) and genotyping errors (b) on the values of homozygosity using the complete model (nfb) and its comparison with the model excluding the effect of the null alleles (fb), through the Deviance Information Criterion (DIC) between the two models. The analysis was run with 500,000 MCMC iterations and 50,000 burn-in cycles. Where the nfb model obtains the lowest DIC, this indicates that the null alleles have an important weight in the calculation of inbreeding. The null alleles also have a potential effect on genetic differentiation (FST). To evaluate this, the FreeNA software was used to perform this estimation by detecting the frequency of null alleles for each locus and population based on the Expectation-Maximization (EM) algorithm (Dempster et al., 1977; Chapuis and Estoup, 2007). The population differentiation (FST) was evaluated excluding null alleles, i.e., the ENA correction method (Chapuis and Estoup, 2007). A total of 50,000 bootstrap replicates were used to calculate the confidence intervals of the corrected global FST.
Multivariate analyses were used to identify the genetic structure. First, analysis of molecular variance (AMOVA) was performed (GenAlex 6.5, Peakall and Smouse, 2012) to quantify the proportion of diversity within populations, among populations and among categories of management. This analysis uses the ΦST index, which is analogous to Wright’s index of differentiation FST (Hedrick, 2005). In addition, a Discriminant Principal Components Analysis (DPCA) (Adegenet, R Development Core Team, 2012) was executed to visualize the genetic structure in a graphic form. DPCA retains the virtues of a discriminant analysis, defining a model in which components of the genetic variation are maximized among groups and minimized within groups. The DPCA is supported by a transformation using a Principal Components Analysis (PCA) as a first step, guaranteeing that the subjected variables are not correlated and are lower in number than those of the individuals analyzed (Jombart et al., 2010). Finally, to visualize the degree of differentiation between pairs of populations, a paired matrix of genetic distances of Nei and Chakraborty (1973) was generated in the program GenAlex version 6.5 (Peakall and Smouse, 2012), with which a dendogram was constructed using the UPGMA method.
Isolation by Distance/Isolation by Environment
Genetic/Morphological Distances – Geographic+Environmental Distances Matrix
To identify whether similar processes are molding morphological and genetic distances among populations, a simple regression analysis between these two distance matrices was conducted using the Multiple Regression Method (MRM) (Legendre et al., 1994; Lichstein, 2007) in R platform (ecodist v2.0.1, Goslee and Urban, 2007). The significance of the coefficient of regression was estimated with 1,000 permutations.
Separately, to test for IBD and IBE, we analyzed the relationship of genetic distances with geographic and environmental conditions, respectively, using the MRM method. The significance of the coefficient of regression was estimated with 1,000 permutations. The genetic distances were calculated by using the pairwise comparisons corrected for the presence of null alleles (Chapuis and Estoup, 2007) and linearized according to the equation FST/1−FST. The geographic distance matrix was generated using the geosphere package version 1.5.10 (Hijmans, 2015). To construct an environmental distance matrix, the 19 environmental variables of WorldClim Global Climate data version 21, with a resolution of 30 arcsec (1 km2), were obtained. To reduce multicollinearity among environmental variables, those that presented a correlation ≥0.8 (JMP version 9, SAS Institute) were excluded. Separately, for each environmental variable, a simple regression analysis between the environmental distance matrix and the genetic distances was performed using the MRM method. In order to capture the most informative variables, those that exhibited a significant association with genetic distances were selected. Finally, Principal Component Analysis (PCA) was used to reduce the variation of these data, and the two first Principal Components (explaining 97% of the total variation) were used to calculate the Euclidean similarity distance matrix (PAST version 3.24, Hammer et al., 2001).
To test IBD and IBE in morphological distances, we performed the MRM method (1,000 permutations) using the geographical and environmental matrices generated with the procedure explained above. The morphological distance matrix was obtained based on the 12 morphological traits and the same pretreatment and Euclidian distance used in the PCoA and PERMANOVA (Primer v6.1.11.).
Results
Morphological and Genetic Diversity
The Index of Morphological Diversity (IMD) reflected a general mean of 0.638 ± 0.07 (interval 0.48–0.75, Supplementary Material SM4). In the cultivated populations, a narrow interval of 0.620–0.673 was observed. In the managed populations, this interval broadened to 0.493–0.747 and in the wild populations it was 0.460–0.700. However, no significant differences were found in mean IMD among categories or populations (H = 16, p = 0.453), suggesting that the plants were morphologically diverse regardless of management condition or the population to which they belong. However, variation tended to be lower among cultivated populations (SD ± 0.03), compared to the managed (SD ± 0.09) and wild populations (SD ± 0.1) (Supplementary Material SM4).
The mean percentage of polymorphic loci for all of the populations was 94.75%, the mean number of alleles per locus was 2.37 and the mean number of effective alleles was 1.87. There was an observed heterozygosity of 0.295 and an expected heterozygosity of 0.418 (Supplementary Material SM4). Mean values per management category (Table 4) did not present significant differences in any of the parameters of genetic diversity (P, A, AE, HO and HE). On average, close to half of the loci (49.2%) were found to be in Hardy-Weinberg equilibrium, with slight variation presented in each category (cultivated 41.67%, managed 51.85% and wild 52.78%). Another 38.09% of the loci, on average, were found to present a deficit of heterozygotes, also with slight variation among categories (cultivated 38.89%, managed 35.18% and wild 41.67%). A total of 7.14% of the loci presented greater heterozygosity than expected in equilibrium: the highest average (13.89%) was found in the cultivated plants, followed by the managed (5.56%) and wild (2.78%) plants. The inbreeding coefficient was FIS = 0.273 ± 0.134, only five of the nine loci were significant (estimated X2 > critical value, p = 0.001). The loci that did not present local inbreeding corresponded to the primers 12, 1763, 28 and 20. A frequency of null alleles was found in a range of 0–0.392. Inbreeding corrected for null alleles was of Avg (Fi) = 0.1529, with a density interval after 95% of 0.0024–0.3179. The model with the lowest DIC value was nfb (DIC: 10216), compared to the model fb (DIC: 10424), indicating that the null alleles had an important effect on the calculation of the inbreeding.
Morphological and Genetic Structure
The first two functions of the Linear Discriminant Analysis (LDA) explained 70% of the morphological variation. The maximum leaf width (MxLW) had the highest value in the LDA1 and traits related to plant thorniness (NT/LL; NT) and plant size (MxD) had the highest values in the LDA2. This analysis did not define a congruent grouping with the management categories established a priori (Figure 3 and Supplementary Material SM5). The cross validation test showed confidence values of between 30 and 100%, which corresponded to the proportion of individuals correctly identified in their population of origin based on morphological similarity. Prediction functions reclassified monocultures by their morphological similarity within mainly managed populations (Figure 4). Moreover, the PERMANOVA evidenced that 36% of the variation was presented among the populations (p < 0.05) with a non-significant 19.8% variation found among a priori categories (Table 5). Likewise, the PCoA showed a wide pattern of variation similar to that identified by the LDA and PERMANOVA. In PCoA (Figures 5A,B) three groups of populations and two isolated entities were distinguished. Group I comprised LH, EP, RS and CN, which are plants with a tendency to present high numbers of teeth (NT ≥ 73), large terminal thorns (TTL ≥ 32 mm) and more elliptic leaves (LWm/MxLW = 0.9) compared to the rest of the populations, which tend to have spathulate leaves (LWm/MxLW = 0.8). Group II comprised CH, CC, EM and LB, which include the largest and robust plants, of greatest height (TPH ≥ 116 cm), maximum diameter (MxD ≥ 176 cm), and with the widest leaves (LWm ≥ 19.5 cm). Group III comprised VP, EN, RM, SM, PC, LV and LT, which present plants of smaller dimensions (compared to group II) and most populations with a high thorniness index (NT10/LL = 0.2), as well as shorter and stouter terminal spines (TTW/TTL = 0.2). Finally, the ET and SO populations are isolated entities: ET (group IV) had the smallest plants (TPH = 58 cm; MxD = 101 cm; LWm = 10.8 cm) with the highest thorniness (NT10/LL = 0.4; NT/LL = 1.5) and, finally, SO (group V), much like group II, had large plants but presented the lowest thorniness index of all (NT10/LL = 0; NT/LL = 0.5) (Figures 5A,B). Even though such grouping tendencies can be observed, the values of the variation coefficient are wide in all traits (Supplementary Material SM6), constituting another expression of the high morphological variation of the species.

Table 5. Permutational analysis of variance (PERMANOVA) for twelve morphological traits in wild, cultivated, and managed populations of A. maximiliana.
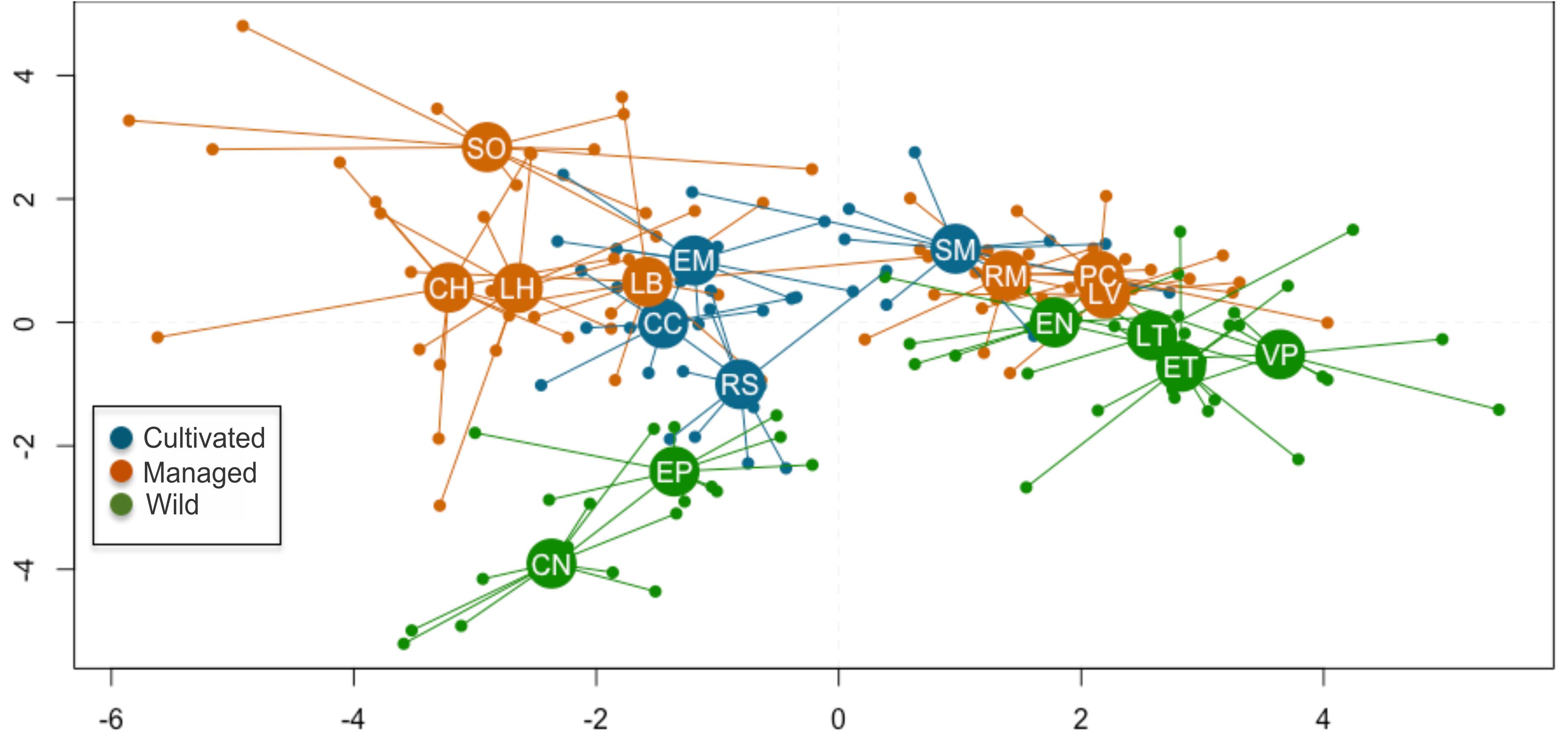
Figure 3. Scatterplot of discriminant analysis, explaining 70% of the total morphological variation. Small circles represent the 170 adult individuals evaluated, while larger circles are the centroids in each population. See Table 2 for definition of codes.
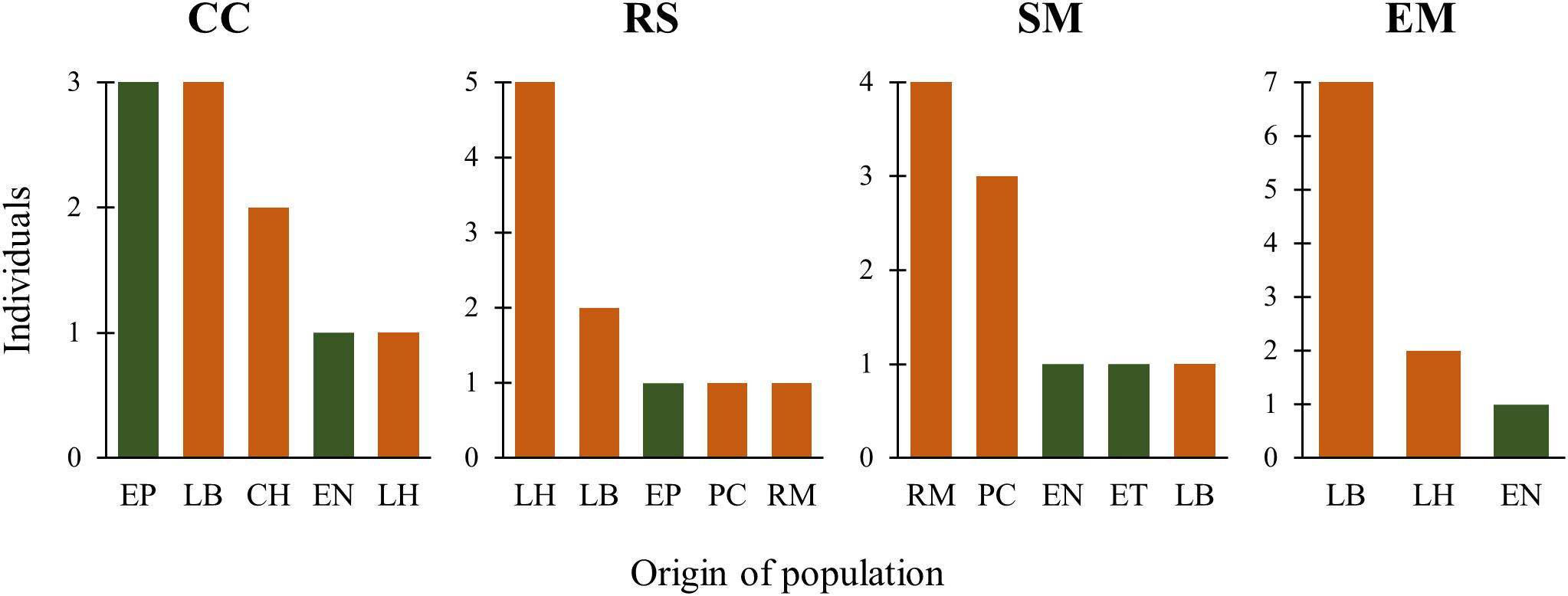
Figure 4. Histograms of probable origin of cultivated plants according to their morphological similarity. Green: wild populations, orange: managed populations. Black letters are monoculture codes. CC = Cimarrón Chico, RS = Rincón Seco, SM = San Miguel, EM = El Mosco. The names of the population of probable origin follow those in Table 2.
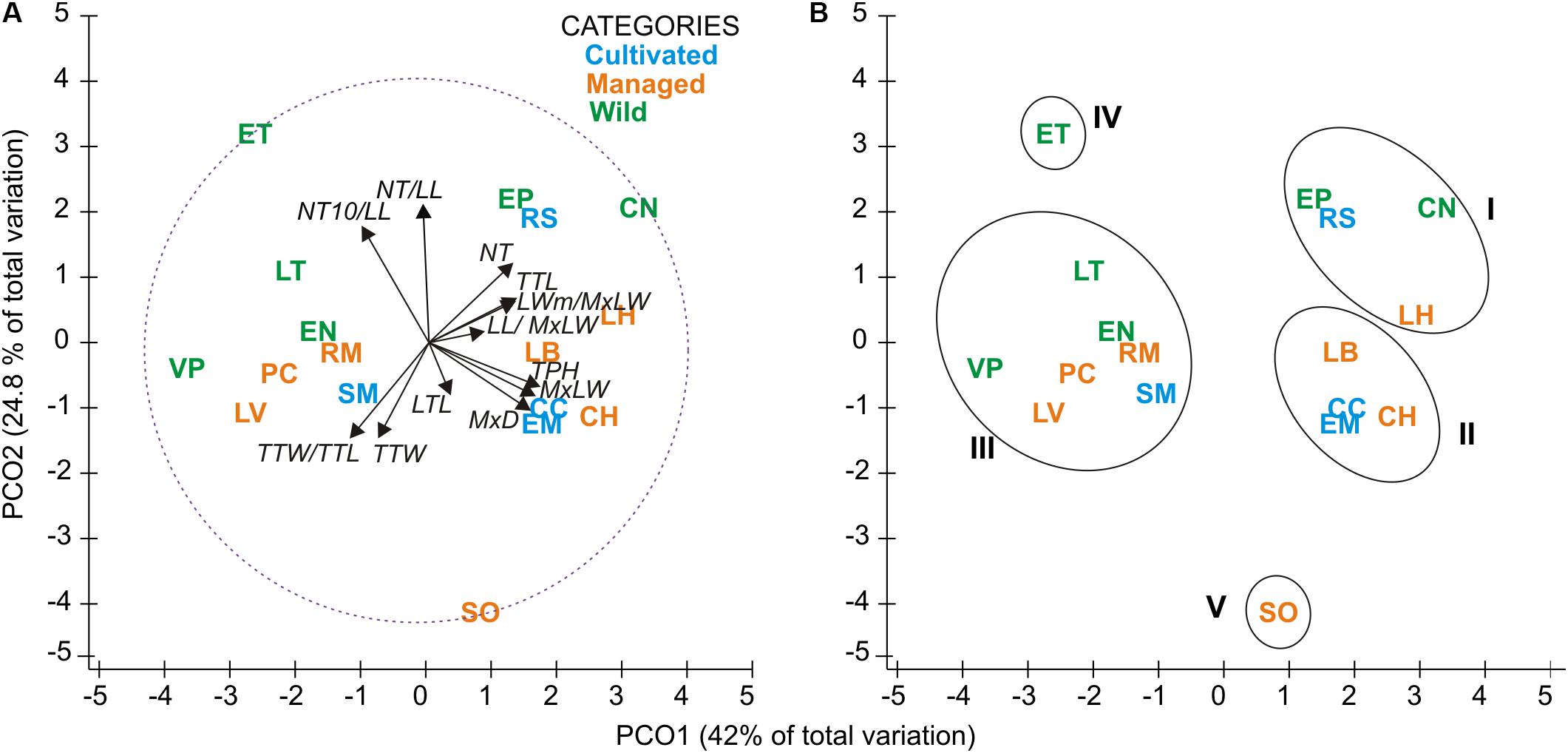
Figure 5. Principal coordinates analysis (PCoA) ordination; (A) Relationships among populations and morphological traits. Longitude of vectors corresponds to the magnitude of the contribution (correlation) of each trait, and the circle with dotted line represents the Pearson correlation ranges from -1 to 1. (B) Ellipsoids correspond to population groups (I to III) with similar traits, and two isolated entities (IV and V) with divergent traits. See Tables 2 and 3 for definition of codes.
With respect to the genetic data, the AMOVA (Table 6) revealed that 61% of the variation was found within populations and 38% was found among populations (ΦCT = 0.38, p = 0.001), which is similar to that found with the morphological data, while 1% corresponded to the variation among management categories (ΦPC = 0.01, p = 0.003). Population differentiation, corrected for null alleles, was FST = 0.43 with ± 0.34–0.51 confidence intervals. Finally, the DAPC showed no genetic structure congruent with the pre-established categories (Figure 6). Most of the populations overlapped in the multidimensional space, apart from three: two wild (EN and EP), which were the most geographically distant, being located in Nayarit and Sinaloa, and one managed (RM). The population EC is geographically very close to EP (Figure 2); however, it overlapped genetically with managed and cultivated populations (Figure 6). The UPGMA showed a pattern of genetic distances that is not congruent with geography, but which groups together almost all of the cultivated and managed populations (except for EM and LV) (Figure 7). The wild EP is the most distant population of a second cluster group comprising a cultivated (EM) and a managed population (LV) from Jalisco, and two wild populations (EN) from Nayarit and (EC) from Sinaloa.

Table 6. Molecular analysis of variance based on 9 SSR loci, in wild, cultivated and managed populations of A. maximiliana.
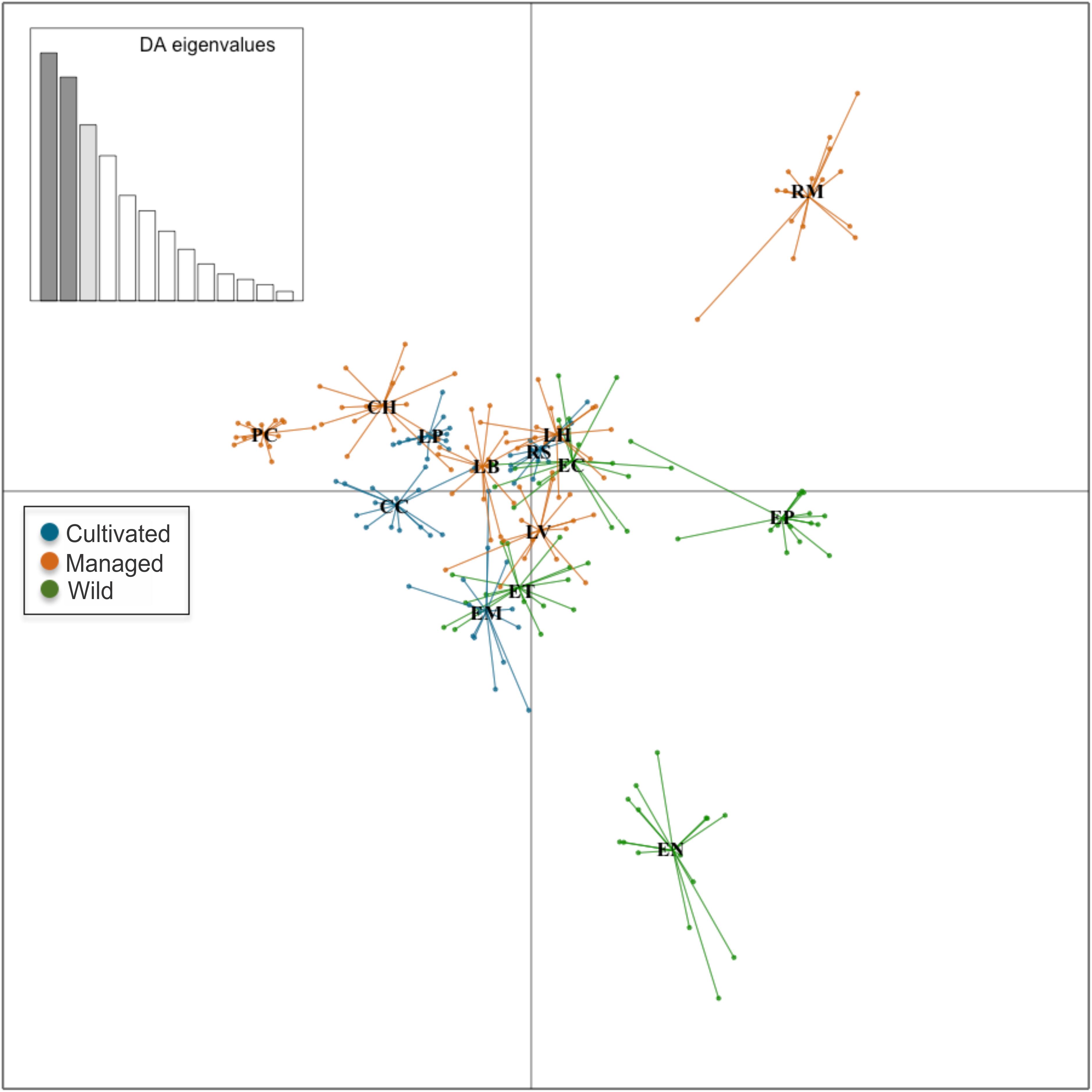
Figure 6. Discriminant analysis of principal components in nine loci and 14 populations of A. maximiliana. Small circles represent the 229 genetic samples. Lines represent the genetic variation within populations.
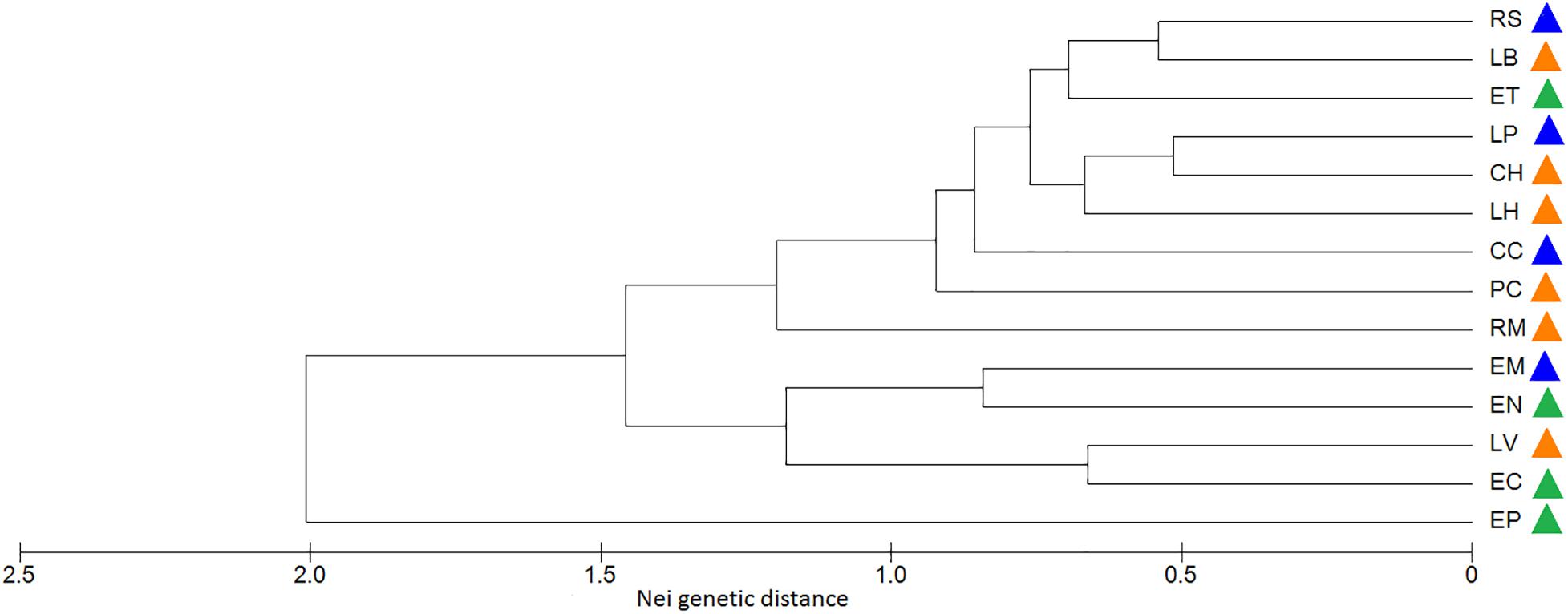
Figure 7. Dendrogram of genetic similarities in A. maximiliana populations based on Nei genetic distances. Genetically closer populations show the origin of clusters skewed to the right by reference of the Nei genetic distance scale. Green: wild; orange: managed; blue: cultivated. See Table 2 for definition of codes.
Isolation by Distance/Isolation by Environment for Genetic and Morphological Distance Matrices
Morphological and genetic distances were not related to each other (R2 = 0.02, p = 0.22). The general model for IBD and IBE in terms of genetic data was not significant (R2 = 0.20, p = 0.06); however, we detected a significant effect of environmental variables on the genetic distance matrix (β = 0.32, p = 0.007). These variables were annual precipitation (BIO12; 785–1312 mm), precipitation of wettest month (BIO13; 187–333 mm), precipitation of driest month (BIO14; 2–11 mm), precipitation seasonality (BIO15; 87–115 mm), precipitation of warmest quarter (BIO18; 239–795 mm), precipitation of coldest quarter (BIO19; 45–156 mm). This result supports an IBE process, while the geographical distances matrix did not present a significant association with the genetic distances matrix (β = −0.13, p = 0.28) and therefore does not support an IBD process.
On the other hand, the MRM general model used to explore the influence of geographical and environmental variables on the morphological distance matrix was not statistically significant (R2 = 0.20, p = 0.06), and neither were the regression coefficients for the geographic and environmental distance matrices (β = 0.03, p = 0.74 and β = 0.13, p = 0.12, respectively).
Discussion
This research showed that forestry systems of Agave maximiliana are effective as reservoirs of morphological and genetic diversity, since their levels are similar to those presented in wild populations. This result is congruent with other Agave species with similar management practices (A. inaequidens, A. cupreata, A. potatorum, Table 1). However, despite having similar reproductive and pollination systems to these species, A. maximiliana showed high levels of morphological diversity while, contrary to our hypothesis, the species presented low levels of genetic variation and high genetic differentiation among populations. Morphological divergences are not congruent with management categorization, although a slight genetic differentiation is. Morphological and genetic distances are not correlated, which suggests that different ecological-evolutionary processes are governing these traits. Alternatively, the number of loci evaluated might be not enough to test an association of genetic and morphological traits. Thus, further studies, including more genetic markers, are needed to analyze this relationship. Precipitation variables influence the environmental heterogeneity that in turn partly governs genetic structure in A. maximiliana. At the same time, no isolation by distance or by environment was detected in terms of the morphological differentiation in population pairs.
Morphological and Genetic Diversity
Levels of morphological diversity in A. maximiliana are high (IMD = 0.628 on average, with an interval of 0.460–0.747), with values that are comparable to those reported for columnar cacti under in situ management categories, with mainly sexual (Myrtillocactus schenckii (J.A. Purpuss) Britton & Rose, IMD 0.652–0.703, Blancas et al., 2009) and, to a lesser extent, asexual (Stenocereus pruinosus (Otto ex Pfeiff.) Buxb. IMD 0.647–0.677, Parra et al., 2012) reproduction, but higher than Stenocereus stellatus (Pfeiff.) Riccob. with asexual reproduction (IMD 0.408–0.479, Casas et al., 2006). With respect to other agaves, the levels of morphological diversity are higher than those reported for species propagated from seeds (IMD = 0.413 Agave inaequidens; 0.489 A. cupreata) and from shoots (0.481 A. hookeri, Figueredo-Urbina et al., 2017). In all of the cases mentioned, managed populations present morphological diversity that is greater than or similar to that of wild populations. This provides evidence that traditional in situ management maintains the levels of diversity found in the wild state, and is in contrast to the forms of modern plant improvement responsible for the reduction of genetic and morphological diversity in global agriculture (Vellvé, 1992; Clunies-Ross, 1995). The evidence provided here confers A. maximiliana with the highest morphological diversity reported for a species of Agave to date.
In contrast, the low genetic diversity found in A. maximiliana, with similar values across the management categories (Table 4), suggests that there is a general biological and/or ecological process underway that is independent of the management context pre-established in this study. This result is incongruent with its life history characteristics, particularly the fact that it propagates from seed. The genetic diversity of A. maximiliana is lower than that of other long-living perennial cross-pollinating species (HE = 0.61–0.68, Nybom, 2004), as well as that of its congeners A. inaequidens and A. potatorum (Table 1). It is barely comparable with other agaves of vegetative propagation (e.g., cultivated plants of A. parryi, A. angustifolia) and even equal or inferior to some landraces of domesticated species such as A. angustifolia, A. rhodacantha and A. tequilana (Table 1). Moreover, the levels of inbreeding found are significant at population level (FIS = 0.273), although it should be noted that the inbreeding corrected for null alleles was lower (Fi = 0.153). This value must therefore be treated with some caution. Assuming that A. maximiliana has an outcrossing reproductive system, this would depend to a large extent on the pollinators. The low genetic diversity and presence of inbreeding in this species could be the consequence of low visit rates or inefficient pollinator behavior.
The Crenatae group, to which A. maximiliana (Gentry, 1982) belongs, includes species with strong inbreeding depression and a xenogamic pollination system that are pollinated by bats. In general, agaves with paniculate flowers are often pollinated by bats (Gentry, 1982; Rocha et al., 2006). However, the specific reproductive and pollination system of A. maximiliana has not been examined in detail. Arizaga et al. (2000) demonstrated in A. macroacantha Zucc. that, without pollination by bats, the plants produce only approximately 50% of the number of seeds. The size of the population of chiropterans that visit the plants influences the proportion of viable seeds (Howell and Roth, 1981; Arizaga et al., 2000). González (2005) found that visits by a low density of bats in A. garciae-mendozae Galván and Hernández produce low dispersion of pollen, since these bats visit flowers of a given plant at different stages, thus causing inbreeding.
Seed scarcity is frequently mentioned by various producers in the raicilla de la sierra region, and has led to the implementation of an in vitro reproduction technique from seeds in the local technical high school (pers. obs.). In some of the sample populations, there is also a high incidence of plants with poorly developed inflorescences; i.e., of small size and with few flowers, and with evidence of infection by fungi and bacteria, (pers. obs.). Low levels of genetic diversity are generally associated with inbreeding and this, in turn, can trigger a reduction in the average adaptation of the population, in a process known as inbreeding depression (Charlesworth and Charlesworth, 1987; Reed and Frankham, 2003; Hedrick, 2005). This association between genetic diversity and low seed production has been reported in congeners such as Agave murphyi Gibson (Gentry, 1982; Parker et al., 2007) and Agave schottii Engelm (Trame et al., 1995). Considering these arguments, inbreeding depression in A. maximiliana is an issue that merits further exploration in future studies.
It is notable that management has not eroded the genetic diversity of cultivated and managed populations and that these remain similar to that of the wild populations. This could reflect very recent processes of artificial selection, an active exchange of seeds and plants among farmers or a low intensity of exploitation that still does not genetically impact the populations (Casas et al., 2006; Parra et al., 2010). The maintenance and increase in local diversity of in situ managed and cultivated populations is relatively common in perennial plants or those with wide generation times in other regions of Mexico and America (Stenocereus pruinosus, Parra et al., 2010; S. stellatus, Cruse-Sanders et al., 2013; Myrtillocactus schenckii, Blancas et al., 2009; Spondias tuberosa Arruda, Neto et al., 2013; Pouteria sapota (Jacq.) H. E. Moore and Stearn, Arias et al., 2015). In Mexico, this is due to the broad spectrum of activities of in situ management that not only include the extraction of plants, but also manual dispersion of seeds, tolerance of some individuals and the protection and promotion of plants with characteristics that are desired by humans, among others. These are practices that have been widely documented for agaves in central (Torres et al., 2015a) and western Mexico (Vargas-Ponce et al., 2007; Huerta-Galván, 2018) and for other useful plants in the Tehuacan Valley region (Blancas et al., 2010).
Morphological and Genetic Structure
Divergences in morphological and genetic characters evaluated for Agave maximiliana in the studied area respond mainly to ecological-evolutionary processes related to the habitat and, to a lesser extent, to management practices. This is evidenced by the wide dispersion of variation in morphological and genetic traits that does not correspond to the management categories established a priori and contrasts with that reported for congeners such as A. parryi (Parker et al., 2014), A. inaequidens and A. cupreata (Figueredo-Urbina et al., 2017), as well as other species in more advanced stages of domestication, such as A. angustifolia and A. rhodacantha (Vargas-Ponce et al., 2007, 2009). In these domesticated species, the phenotypic traits are adjusted to the assigned categories or the cultivars differ morphologically among themselves. Our results validate the information recorded by Huerta-Galván (2018), that the interchange of plants or seeds in monocultures of A. maximiliana came mainly from the forestry systems within the raicilla de la sierra region and that the monocultures themselves are very recent systems. This can be inferred from their morphological similarity, where 60% (CC) to 90% (EM, RS) of individuals in monocultures can be morphologically identified as belonging to other in situ managed populations (Figure 4). This can also be inferred by genetic similarity, where most of the monocultures and managed populations (except for EM and LV) tended to form a cluster (Figure 7). However, morphological and genetic variation is wider among populations (36 and 38%, respectively) than among categories (19.8 and 1%, respectively). Consequently, our study did not find significant divergence related to management.
Different factors are involved in morphological divergences compared to genetic structure, since these were not related to each other. A correlation between phenotypic variation and neutral genetic markers may occur if the same neutral processes (e.g., gene flow and genetic drift) have historically affected the spatial structure of both types of variation (e.g., Hipp et al., 2018; Rodríguez-Gómez et al., 2018). Another aspect is that populations usually exhibit contrasting spatial patterns for different genetic markers because the mutation rates, combined with the evolutionary forces acting on them, are not the same (Habel et al., 2015). It is therefore necessary to include more genetic markers to support these results. On the other hand, isolation by environment (six variables related to precipitation; β = 0.32, p = 0.007) and not geographic distance is one of the mechanisms that structure the populations of A. maximiliana. This means that, to some extent, populations are genetically isolated as a result of different precipitation conditions (e.g., annual precipitation varies from 785 to1312 mm, precipitation of warmest quarter varies from 239 to 795 mm; cf. Trejo et al., 2016). Local precipitation is related to the regeneration and colonization of plant populations (Jiang et al., 2019). Thus, the influence of precipitation on demographic dynamics may explain its effect on the population genetic structure as revealed by neutral markers (Jiang et al., 2019), where demographic parameters mediate ecological–evolutionary interactions via adaptive and non-adaptive mechanisms (Lowe et al., 2017). Gene flow could be reduced by the poor establishment success of immigrant seeds. Moreover, a decreased chance of outcrossing conditions may compound these gene flow reductions and accelerate the genetic fixation rate in populations (Jiang et al., 2019). This phenomenon not only appears in adaptive loci but could also extend to the whole genome via genetic drift caused by selective sweeps (Nosil et al., 2009). Since morphological variation is not explained by any of these isolation processes, this could be a sign to discard phenotypic plasticity (cf. Rodríguez-Gómez et al., 2018) in the evaluated traits. However, other approaches, such as wide genome scans or/and common garden experiments, are necessary to confirm these interpretations (de Villemereuil et al., 2018). Finally, it is important to mention that Scheinvar et al. (2017) modeled environmental niche changes in A. maximiliana and other congeners, and found that A. maximiliana could have undergone an extension of its environmental niche during the Last Interglacial period (LIG, 130 Kyr), followed by a reduction in the Last Glacial Maximum period (LGM, 31Kyr). Thus, past extension-contraction cycles in the demographic history of A. maximiliana could result in decreases in the levels of genetic diversity and increases in genetic structure (e.g., A. lechuguilla Torr., Scheinvar et al., 2017). This hypothesis should also be tested using phylogeographic approaches and with other types of molecular markers in order to support the main findings of this study.
The hypothetical presence of two varieties of A. maximiliana was not clearly reflected in our data. The two northern populations collected for this study (CN and EP, Figure 2) occupy a region in which A. maximiliana var. katherinae is probably distributed (Gentry, 1982; Scheinvar, 2018). Both populations are morphologically similar or close in the multivariate space (Figures 5A,B) to LH and RS, which are not characterized by larger rosettes or greater thorniness, compared to A. maximiliana var. maximiliana as Gentry (1982) described it. The population EP is differentiated genetically (Figures 6, 7) but we did not obtain a sufficient number of microsatellite amplifications from CN, and therefore do not know its genetic components and cannot determine its location in the genetic multivariate space. The population EC, which is geographically close to EP and also within the hypothetical distribution area of A. maximiliana var. katherinae, is grouped as a genetic entity similar to managed and cultivated populations of the raicilla de la sierra region. This suggests that an important gene flow is still perceptible between the two hypothetical varieties. We consider that further research with a sample design focused on this taxonomic approach is necessary to test this hypothesis.
Finally, A. maximiliana maintains the highest genetic structure reported to date among species of Agave (ΦST = 0.4, Table 1; Eguiarte et al., 2013). This is perhaps a reflection of the type and extension of the distribution areas of their populations; this can be perceived in other congeners, in which a discontinuous distribution is associated with high genetic structure, in contrast to those of continuous distribution (e.g., A. parryi vs. A. palmeri, Lindsay et al., 2018). Furthermore, together with the results of genetic diversity and inbreeding, this reinforces the need to investigate the reproductive and pollination system of this species. The higher the genetic structure, the greater the probability that populations will reflect changes as independent evolutionary units (Slatkin, 1994), whether through genetic drift or selection.
Perspectives and Recommendations
Based on the evidence presented in this study, we consider that traditional management represented by forestry systems of A. maximiliana has been a sustainable option in terms of genetic germplasm conservation. However, the current threatening scenario of the use of Agave maximiliana as a raw material for agroindustrial production of raicilla is disheartening. Of particular concern are the low genetic diversity, high genetic structure and presence of inbreeding in this species. This fact should be taken into account in management when translocating seeds or plants from one site to another, due to possible processes of local adaptation that could preclude the optimum development of plants outside of their populations of origin (Habel et al., 2015). We perceive this as a weakness of the species, if the forms of management move to more intensive practices, which is highly probable in the light of the recent denomination of origin of raicilla (Diario Oficial de la Federación, 2019). However, recovery of this situation is possible based on promotion of these forestry systems that still maintain A. maximiliana.
Our research approach had restrictions related to the identification of local adaptation processes and phenotypic plasticity. As a result, we cannot make direct conclusions about the extent to which the wild and managed populations (either in situ or monocultures) of A. maximiliana are locally adapted. In addition, adoption of new approaches regarding developmental plasticity, variation in quantitative trait loci related to natural and artificial selection or niche construction theory (Chen et al., 2017; Piperno, 2017) could complete the understanding of diversification versus domestication contexts (Pickersgill, 2018). Despite this, we consider that the general patterns of intraspecific diversity in in situ contexts of management practices are still poorly documented, and that this study constitutes a valuable base on which to establish more specific research questions to further the understanding of the origin of domestication processes.
Finally, we recognize in this case study that, beyond the improvement of technical options to multiply the number of plants available per year, it would be more meaningful to consider broader aspects of sustainability and conservation. This would generate benefits not only for the raicilla production chain, but also for the range of environmental goods and services their habitat provides.
Data Availability Statement
The datasets generated and analyzed for this study can be found in the Mendeley Data, http://dx.doi.org/10.17632/3379nnccmy.4.
Author Contributions
DC-T and OV-P conceived and designed the study, partially organized fieldwork and carried out some final statistical analysis. SA-R and LV-S carried out the collection and first analysis of phenotypic and genetic data, respectively, as part of their bachelor theses. JP-A gave input on the study design and carried out some final statistical analysis and design of figures. JM-S led laboratory work. OH-G described the management practices which were the basis of the hypothesis of this study, he also partially organized fieldwork. All authors reviewed and approved the final manuscript.
Funding
The research was funded by Consejo Nacional de Ciencia y Tecnología (CONACyT) through the Laboratorio Nacional de Identificación y Caracterización Vegetal (LaniVeg) research grants (Nos. 261099 and 272045).
Conflict of Interest
The authors declare that the research was conducted in the absence of any commercial or financial relationships that could be construed as a potential conflict of interest.
Acknowledgments
We thank P. Carrillo-Reyes, A. Dávalos-Martínez, D. Figueroa-Martínez, A. Zabalgoitia, J. Rendón, Z. López-Dellamary, K. Rostro, P. Velázquez-Ríos, J. González-Gallegos, and A. Castro-Castro for field assistance, and P. Zamora for laboratory assistance. P. Carrillo-Reyes, A. Castro-Castro, D. Figueroa-Martínez, and A. Zabalgoitia for their contributions in floristic information. We also thank C. J. Figueredo-Urbina and Anwar Medina-Villareal for their help and advice in the analysis of morphological data. Thanks also go to the raicilla de la sierra producers, Gerardo Peña, Juan Lomelí, Aristeo Macedo, Manuel Rodríguez, Benito Salcedo, Manuel Salcedo, Gelo Aguirre, Don Delfino, the families Arrizon, and Segura for their kindness and permission to work on their properties, and to the communities of El Palmito, Sinaloa, and Canelas, Durango for their kind welcome.
Supplementary Material
The Supplementary Material for this article can be found online at: https://www.frontiersin.org/articles/10.3389/fpls.2020.00817/full#supplementary-material
Footnotes
References
Aguirre-Dugua, X., and Eguiarte, L. E. (2013). Genetic diversity, conservation and sustainable use of wild Agave cupreata and Agave potatorum extracted for mezcal production in Mexico. J. Arid Environ. 90, 36–44. doi: 10.1016/j.jaridenv.2012.10.018
Anderson, M. J. (ed.). (2017). “Permutational multivariate analysis of variance (PERMANOVA),” in Wiley StatsRef: Statistics Reference Online, (Hoboken, NJ: Wiley), 1–15. doi: 10.1002/9781118445112.stat07841
Anderson, M. J., Gorley, R., and Clarke, R. (2008). PERMANOVA+para PRIMER: Guía Para el Programa y Métodos Estadísticos. PRIMER-E. Plymouth: Massey University Press.
Arias, R., Martínez-Castillo, J., Sobolev, V., Blancarte-Jasso, N., Simpson, S., Ballard, L., et al. (2015). Development of a large set of microsatellite markers in zapote mamey (Pouteria sapota (Jacq.) HE Moore & Stearn) and their potential use in the study of the species. Molecules 20, 11400–11417. doi: 10.3390/molecules200611400
Arita, H. (1991). Spatial segregation in long-nosed bats, Leptonycteris nivalis and Leptonycteris curasoe, in Mexico. Am. Soc. Mammal. 72, 706–714. doi: 10.2307/1381831
Arizaga, S., Ezcurra, E., Peters, E., de Arellano, F. R., and Vega, E. (2000). Pollination ecology of Agave macroacantha (Agavaceae) in a Mexican tropical desert. II. The role of pollinators. Am. J. Bot. 87, 1011–1017. doi: 10.2307/2657001
Arroyo-Cabrales, J., Hollander, R. R., and Jones, J. K. J. (1982). Choeronycteris mexicana. Mammal. Species 291, 1–5.
Blancas, J., Casas, A., Lira, R., and Caballero, J. (2009). Traditional management and morphological patterns of Myrtillocactus schenckii (Cactaceae) in the Tehuacán Valley, Central Mexico. Econ. Bot. 63, 375–387. doi: 10.1007/s12231-009-9095-2
Blancas, J., Casas, A., Pérez-Salicrup, D., Caballero, J., and Vega, E. (2013). Ecological and socio-cultural factors influencing plant management in Náhuatl communities of the Tehuacán Valley, Mexico. J. Ethnobiol. Ethnomed. 9:39. doi: 10.1186/1746-4269-9-39
Blancas, J., Casas, A., Rangel-Landa, S., Moreno-Calles, A., Torres, I., Pérez-Negrón, E., et al. (2010). Plant management in the Tehuacán-Cuicatlán Valley, Mexico1. Econ. Bot. 64, 287–302. doi: 10.1007/s12231-010-9133-0
Bowen, S., and Gerritsen, P. R. W. (2007). Reverse leasing and power dynamics among blue Agave farmers in western Mexico. Agric. Hum. Values 24, 473–488. doi: 10.1007/s10460-007-9088-7
Bowen, S., and Valenzuela-Zapata, A. G. (2009). Geographical indications, terroir, and socioeconomic and ecological sustainability: the case of tequila. J. Rural Stud. 25, 108–119. doi: 10.1016/j.jrurstud.2008.07.003
Byers, C., Maughan, P. J., Clouse, J., and Stewart, J. R. (2014). Microsatellite primers in Agave utahensis (Asparagaceae), a keystone species in the Mojave Desert and Colorado Plateau. Appl. Plant Sci. 2:1400047. doi: 10.3732/apps.1400047
Casas, A., Caballero, J., Valiente-Banuet, A., Soriano, J. A., and Dávila, P. (1999). Morphological variation and the process of domestication of Stenocereus stellatus (Cactaceae) in Central Mexico. Am. J. Bot. 86, 522–533. doi: 10.2307/2656813
Casas, A., Cruse-Sanders, J., Morales, E., Otero-Arnaiz, A., and Valiente-Banuet, A. (2006). Maintenance of phenotypic and genotypic diversity in managed populations of Stenocereus stellatus (Cactaceae) by indigenous peoples in Central Mexico. Biodivers. Conserv. 15, 879–898. doi: 10.1007/s10531-004-2934-7
Casas, A., Parra-Rondinel, F., Aguirre-Dugua, X., Rangel-Landa, S., Blancas, J., Vallejo, M., et al. (2017). “Manejo y domesticación de plantas en mesoamérica,” in Domesticación en el Continente Americano, eds A. Casas, J. Torres-Guevara, and F. Parra (México: UNAM), 69–102.
Chapuis, M. P., and Estoup, A. (2007). Microsatellite null alleles and estimation of population differentiation. Mol. Biol. Evol. 24, 621–631. doi: 10.1093/molbev/msl191
Charlesworth, D., and Charlesworth, B. (1987). Inbreeding depression and its evolutionary consequences. Annu. Rev. Ecol. Syst. 18, 237–268. doi: 10.1146/annurev.es.18.110187.001321
Chen, Y. H., Shapiro, L. R., Benrey, B., and Cibrián-jaramillo, A. (2017). Back to the origin: in situ studies are needed to understand selection during crop diversification. Front. Ecol. Evol. 5:125. doi: 10.3389/fevo.2017.00125
Chybicki, I. J., and Burczyk, J. (2009). Simultaneous estimation of null alleles and inbreeding coefficients. J. Hered. 100, 106–113. doi: 10.1093/jhered/esn088
Clunies-Ross, T. (1995). Mangolds, manure and mixtures. The importance of crop diversity on British farms. Ecologist 25, 181–187.
Colunga-GarcíaMarín, P., and Zizumbo-Villarreal, D. (2007). Tequila and other Agave spirits from west-central Mexico: current germplasm diversity, conservation and origin. Biodivers. Conserv. 16, 1653–1667. doi: 10.1007/s10531-006-9031-z
Contreras-Negrete, G., Ruíz-Durán, M. E., Cabrera-Toledo, D., Casas, A., Vargas, O., and Parra, F. (2015). Genetic diversity and structure of wild and managed populations of Polaskia chende (Cactaceae) in the Tehuacán-Cuicatlán Valley, Central Mexico: insights from SSR and allozyme markers. Genet. Resour. Crop Evol. 62, 85–101. doi: 10.1007/s10722-014-0137-y
Cruse-Sanders, J. M., Parker, K. C., Friar, E. A., Huang, D. I., Mashayekhi, S., Prince, L. M., et al. (2013). Managing diversity: domestication and gene flow in Stenocereus stellatus Riccob.(Cactaceae) in Mexico. Ecol. Evol. 3, 1340–1355. doi: 10.1002/ece3.524
de Villemereuil, P., Mouterde, M., Gaggiotti, O. E., and Till-bottraud, I. (2018). Patterns of phenotypic plasticity and local adaptation in the wide elevation range of the alpine plant Arabis alpina. J. Ecol. 106, 1952–1971. doi: 10.1111/1365-2745.12955
Delgado-Lemus, A., Casas, A., and Téllez, O. (2014). Distribution, abundance and traditional management of Agave potatorum in the Tehuacán Valley, Mexico: bases for sustainable use of non-timber forest products. J. Ethnobiol. Ethnomed. 10, 1–12. doi: 10.1186/1746-4269-10-63
Dempster, A. P., Laird, N. M., and Rubin, D. B. (1977). Maximum likelihood from incomplete data via the EM algorithm. J. R. Stat. Soc. Ser. B 39, 1–22.
Diario Oficial de la Federación (2019). Declaración General de Protección de la Denominación de Origen Raicilla. Available online at: https://dof.gob.mx/nota_detalle.php?codigo=5564454&fecha=28/06/2019&print=true (accessed June 1, 2020).
Doyle, J. (1991). “DNA protocols for plants,” in Molecular Techniques in Taxonomy, eds G. Hewitt, A. Jhonston, and J. Young (Brussels: NATO), 283–293. doi: 10.1007/978-3-642-83962-7_18
Eguiarte, L. E., Aguirre-Planter, E., Aguirre, X., Colíni, R., González, A., Rocha, M., et al. (2013). From isozymes to genomics: population genetics and conservation of Agave in México. Bot. Rev. 79, 483–506. doi: 10.1007/s12229-013-9123-x
Estrella-Ruiz, P. (2008). Efecto de la Explotación Humana en la Biología de la Polinización de Agave salmiana y Agave potatorum en el Valle de Tehuacán-Cuicatlán. Bachelor thesis, Universidad Nacional Autónoma de México, Mexico.
Félix-Valdez, L. I., Vargas-Ponce, O., Cabrera-Toledo, D., Casas, A., Cibrian-Jaramillo, A., and de la Cruz-Larios, L. (2016). Effects of traditional management for mescal production on the diversity and genetic structure of Agave potatorum (Asparagaceae) in central Mexico. Genet. Resour. Crop Evol. 63, 1255–1271. doi: 10.1007/s10722-015-0315-6
Figueredo, C. J., Casas, A., Colunga-GarcíaMarín, P., Nassar, J. M., and González-Rodríguez, A. (2014). Morphological variation, management and domestication of “maguey alto” (Agave inaequidens) and “maguey manso” (A. hookeri) in Michoacán, México. J. Ethnobiol. Ethnomed. 10, 1–12. doi: 10.1186/1746-4269-10-66
Figueredo, C. J., Casas, A., González-Rodríguez, A., Nassar, J. M., Colunga-GarcíaMarín, P., and Rocha-Ramírez, V. (2015). Genetic structure of coexisting wild and managed Agave populations: implications for the evolution of plants under domestication. AoB Plants 7:lv114. doi: 10.1093/aobpla/plv114
Figueredo-Urbina, C. J., Casas, A., and Torres-García, I. (2017). Morphological and genetic divergence between Agave inaequidens, A. cupreata and the domesticated A. hookeri. Analysis of their evolutionary relationships. PLoS One 12:e0187260. doi: 10.1371/journal.pone.0187260
Flores-Abreu, I. N., Trejo-Salazar, R. E., Sánchez-Reyes, L. L., Good, S. V., Magallón, S., García-Mendoza, A., et al. (2019). Tempo and mode in coevolution of Agave sensu lato (Agavoideae, Asparagaceae) and its bat pollinators, Glossophaginae (Phyllostomidae). Mol. Phylogenet. Evol. 133, 176–188. doi: 10.1016/j.ympev.2019.01.004
Frankham, R., Ballou, J., and Briscoe, D. (2006). Introduction to Conservation Genetics, 6th Edn. Cambridge: Cambridge University press.
Gentry, H. S. (1982). Agaves of Continental North America, 1st Edn. Tucson, AZ: University of Arizona Press.
González, A. (2005). Biología Reproductiva y Genética de Poblaciones de Agave garciaemendozae. Bachelor thesis, Universidad Nacional Auntónoma de México, México.
González-Elizondo, M., Galván-Villanueva, R., López-Enríquez, I., Reséndiz-Rojas, L., and Gonzalez-Elizondo, M. S. (2009). Agaves -Magueyes, Lechuguillas y Noas- del Estado de Durango y sus Alrededores. México: CONABIO-Instituto Politécnico Nacional.
Goslee, S. C., and Urban, D. L. (2007). The ecodist package for dissimilarity-based analysis of ecological data. J. Stat. Softw. 22, 1–19.
Guillén, S., Terrazas, T., De la Barrera, E., and Casas, A. (2011). Germination differentiation patterns of wild and domesticated columnar cacti in a gradient of artificial selection intensity. Genet. Resour. Crop Evol. 58, 409–423. doi: 10.1007/s10722-010-9586-0
Habel, J. A. N. C., Zachos, F. E., Dapporto, L., Rödder, D., Radespiel, U. T. E., Tellier, A., et al. (2015). Population genetics revisited – towards a multidisciplinary research field. Biol. J. Linn. Soc. 115, 1–12. doi: 10.1111/bij.12481
Hammer, Ø., Harper, D., and Ryan, P. (2001). PAST: paleontological statistics software package for education and data analysis. Palaeontol. Electronica 4, 1–9.
Hipp, A. L., Manos, P. S., Gonz, A., Hahn, M., Kaproth, M., Mcvay, J. D., et al. (2018). Sympatric parallel diversification of major oak clades in the Americas and the origins of Mexican species diversity. New Phytol. 217, 439–452. doi: 10.1111/nph.14773
Horner, M. A., Fleming, T. H., and Sahley, C. T. (1998). Foraging behaviour and energetics of a nectar-feeding bat, Leptonycteris curasoae (Chiroptera: Phyllostomidae). Zool. Soc. Lond. 244, 575–586. doi: 10.1111/j.1469-7998.1998.tb00062.x
Howell, D. J., and Roth, B. S. (1981). Sexual reproduction in agaves: the benefits of bats; the cost of semelparous advertising. Ecology 62, 1–7. doi: 10.2307/1936660
Huerta-Galván, O. (2018). Uso y Manejo de Agave maximiliana: Producción de Raicilla en el Municipio de Mascota, Jalisco. Bachelor thesis, Universidad de Guadalajara, Mexico.
Illsey, C., Vega, E., Pisanty, I., Tlacotempa, A., García, P., Morales, P., et al. (2007). “Maguey papalote: hacia el manejo campesino sustentable de un recurso colectivo en el trópico seco de Guerrero, México,” in En lo Ancestral hay Futuro: Del Tequila, Los Mezcales y Otros Agaves, eds P. Colunga-GarcíaMarín, A. Larqué, L. Eguiarte, and D. Zizumbo-Villareal (México: CICY, CONACYT, CONABIO, SEMARNAT).
Jiang, S., Luo, M., Gao, R., Zhang, W., Yang, Y., and Li, Y. (2019). Isolation-by-environment as a driver of genetic differentiation among populations of the only broad-leaved evergreen shrub Ammopiptanthus mongolicus in Asian temperate deserts. Sci. Rep. 9:12008. doi: 10.1038/s41598-019-48472-y
Jombart, T., Devillard, S., and Balloux, F. (2010). Discriminant analysis of principal components: a new method for the analysis of genetically structured populations. BMC Genet. 11:94. doi: 10.1186/1471-2156-11-94
Leclert, L., Gerritsen, P. R. W., and van der Meulen, H. (2010). “Agave azul: crisis cíclicas y las posibilidades para la planeación del cultivo en el estado de Jalisco,” in Agave azul, Sociedad y Medio Ambiente, eds P. R. W. Gerritsen and L. M. Martínez-Rivera (México: DERN-IMECBIO, CUCSUR-UDG), 19–35.
Legendre, P., Lapointe, F., and Casgrain, P. (1994). Modeling brain evolution from behavior: a permutational regression approach. Evolution 48, 1487–1499. doi: 10.1111/j.1558-5646.1994.tb02191.x
León, A. (2013). Aspectos de la Fenología, Visitantes Florales y Polinización de Agave inaequidens Koch ssp. inaequidens (Agavaceae) en el Estado de Michoacán. Bachelor Thesis, Universidad Michoacana de San Nicolás de Hidalgo, Morelia.
Lichstein, J. (2007). Multiple regression on distance matrices: a multivariate spatial analysis tool. Plant Ecol. 188, 117–131. doi: 10.1007/s11258-006-9126-3
Lindsay, D., Edwards, C., Jung, M., Bailey, P., and Lance, R. (2012). Novel microsatellite loci for Agave parryi and cross-amplification in Agave palmeri (Agavaceae). J. Bot. 99, 295–297. doi: 10.3732/ajb.1200033
Lindsay, D. L., Swift, J. F., Lance, R. F., and Edwards, C. E. (2018). A comparison of patterns of genetic structure in two co-occurring Agave species (Asparagaceae) that differ in the patchiness of their geographical distributions and cultivation histories. Bot. J. Linn. Soc. 186, 361–373. doi: 10.1093/botlinnean/box099
Lowe, W., Kovach, R., and Allendorf, F. W. (2017). Population genetics and demography unite ecology and evolution. Trends Ecol. Evol. 32, 141–152. doi: 10.1016/j.tree.2016.12.002
Luikart, G., and Cornuet, J.-M. (1998). Empirical evaluation of a test for identifying recently bottlenecked populations from allele frequency data. Conserv. Biol. 12, 228–237. doi: 10.1111/j.1523-1739.1998.96388.x
McVaugh, R. (1989). Flora Novo-Galiciana: Bromeliaceae to Dioscoreaceae. Ann Arbor, MI: University of Michigan Herbarium.
Medellín, R., Rivero, M., Ibarra, A., De la Torre, J., Gonzalez-Terrazas, T. P., Torres-Knoop, L., et al. (2018). Follow me: foraging distances of Leptonycteris yerbabuenae (Chiroptera: Phyllostomidae) in Sonora determined by fluorescent powder. J. Mammal. 99, 306–311. doi: 10.1093/jmammal/gyy016
Medina-Villarreal, A., and González-Astorga, J. (2016). Morphometric and geographical variation in the Ceratozamia mexicana Brongn. (Zamiaceae) complex: evolutionary and taxonomic implications. Biol. J. Linn. Soc. 119, 213–233. doi: 10.1111/bij.12806
Molina-Freaner, F., and Eguiarte, L. E. (2003). The pollination biology of two paniculate agaves (Agavaceae) from northwestern Mexico: contrasting roles of bats as pollinators. Am. J. Bot. 90, 1016–1024. doi: 10.3732/ajb.90.7.1016
Moreno-Calles, A. I., Toledo, V. M., and Casas, A. (2013). Los sistemas agroforestales tradicionales de México: una aproximación biocultural. Bot. Sci. 91, 375–398. doi: 10.17129/botsci.419
Morrone, J. J., Escalante, T., and Rodríguez-Tapia, G. (2017). Mexican biogeographic provinces: map and shapefiles. Zootaxa 4277, 8–11. doi: 10.11646/zootaxa.4277.2.8
Nair, P. K. R. (1985). Classification of agroforestry systems. Agroforestry Syst. 3, 97–128. doi: 10.1007/bf00122638
Nei, M., and Chakraborty, R. (1973). Genetic distance and electrophoretic identity of proteins between taxa. J. Mol. Evol. 2, 323–328. doi: 10.1007/bf01654100
Neto, E. M. F. L., de Oliveira, I. F., Britto, F. B., and de Albuquerque, U. P. (2013). Traditional knowledge, genetic and morphological diversity in populations of Spondias tuberosa Arruda (Anacardiaceae). Genet. Resour. Crop Evol. 60, 1389–1406. doi: 10.1007/s10722-012-9928-1
Nosil, P., Funk, D., and Ortiz-Barrientos, D. (2009). Divergent selection and heterogeneous genomic divergence. Mol. Ecol. 18, 375–402. doi: 10.1111/j.1365-294X.2008.03946.x
Nybom, H. (2004). Comparison of different nuclear DNA markers for estimating intraspecific genetic diversity in plants. Mol. Ecol. 13, 1143–1155. doi: 10.1111/j.1365-294X.2004.02141.x
Ortega-García, S., Arroyo-Cabrales, J., Guevara, L., Lindig-Cisneros, R., Martínez-Meyer, E., Vega, E., et al. (2017). The thermal niche of Neotropical nectar feeding bats: its evolution and application to predict responses to global warming. Ecol. Evol. 7, 6691–6701. doi: 10.1002/ece3.3171
Otero-Arnaiz, A., Casas, A., Hamrick, J., and Cruse-Sanders, J. (2005). Genetic variation and evolution of Polaskia chichipe (Cactaceae) under domestication in the Tehuacán Valley, central Mexico. Mol. Ecol. 14, 1603–1611. doi: 10.1111/j.1365-294X.2005.02494.x
Parker, K. C., Hamrick, J. L., Hodgson, W. C., Trapnell, D. W., Parker, A. J., and Kuzoff, R. K. (2007). Genetic consequences of pre-Columbian cultivation for Agave murpheyi and A. delamateri (Agavaceae). Am. J. Bot. 94, 1479–1490. doi: 10.3732/ajb.94.9.1479
Parker, K. C., Trapnell, D. W., Hamrick, J. L., and Hodgson, W. C. (2014). Genetic and morphological contrasts between wild and anthropogenic populations of Agave parryi var. huachucensis in south-eastern Arizona. Ann. Bot. 113, 939–952. doi: 10.1093/aob/mcu016
Parker, K. C., Trapnell, D. W., Hamrick, J. L., Hodgson, W. C., and Parker, A. J. (2010). Inferring ancient Agave cultivation practices from contemporary genetic patterns. Mol. Ecol. 19, 1622–1637. doi: 10.1111/j.1365-294X.2010.04593.x
Parra, F., Blancas, J. J., and Casas, A. (2012). Landscape management and domestication of Stenocereus pruinosus (Cactaceae) in the Tehuacán Valley: human guided selection and gene flow. J. Ethnobiol. Ethnomed. 8:32. doi: 10.1186/1746-4269-8-32
Parra, F., Casas, A., Peñaloza-Ramírez, J. M., Cortés-Palomec, A. C., Rocha-Ramírez, V., and González-Rodríguez, A. (2010). Evolution under domestication: ongoing artificial selection and divergence of wild and managed Stenocereus pruinosus (Cactaceae) populations in the Tehuacán Valley, Mexico. Ann. Bot. 106, 483–496. doi: 10.1093/aob/mcq143
Peakall, R., and Smouse, P. E. (2012). GenAlEx tutorials-part 2: genetic distance and analysis of molecular variance (AMOVA). Bioinformatics 28, 2537– 2539.
Peakall, R. O. D., and Smouse, P. E. (2006). GENALEX 6: genetic analysis in Excel. Population genetic software for teaching and research. Mol. Ecol. Notes 6, 288–295.
Pickersgill, B. (2018). Parallel vs. convergent evolution in domestication and diversification of crops in the Americas. Front. Ecol. Evol. 6:56. doi: 10.3389/fevo.2018.00056
Piperno, D. R. (2017). Assessing elements of an extended evolutionary synthesis for plant domestication and agricultural origin research. Proc. Natl. Acad. Sci. U.S.A. 114, 6429–6437. doi: 10.1073/pnas.1703658114
R Development Core Team (2012). R: a Language and Environment for Statistical Computing. Vienna: R foundation for Statistical Computing.
Reed, D. H., and Frankham, R. (2003). Correlation between fitness and genetic diversity. Conserv. Biol. 17, 230–237. doi: 10.1046/j.1523-1739.2003.01236.x
Rivera-Lugo, M., García-Mendoza, A., Simpson, J., Solano, E., and Gil-Vega, K. (2018). Taxonomic implications of the morphological and genetic variation of cultivated and domesticated populations of the Agave angustifolia complex (Agavoideae, Asparagaceae) in Oaxaca, Mexico. Plant Syst. Evol. 304, 969–979. doi: 10.1007/s00606-018-1525-0
Rocha, M., Good-Ávila, S., Molina-Freaner, F., Arita, H., Castillo, A., García-Mendoza, A., et al. (2006). Pollination biology and adaptive radiation of Agavaceae, with special emphasis on the genus Agave. Aliso 22, 329–344. doi: 10.5642/aliso.20062201.27
Rodríguez-Gómez, F., Oyama, K., Ochoa-Orozco, M., Mendoza-Cuenca, L., Gaytán-Legaria, R., and González-Rodríguez, A. (2018). Phylogeography and climate-associated morphological variation in the endemic white oak Quercus deserticola (Fagaceae) along the Trans-Mexican Volcanic Belt. Botany 133, 121–133. doi: 10.1139/cjb-2017-0116
Sanguinetti, C. J., Neto, E. D., and Simpson, A. J. G. (1994). Rapid silver staining and recovery of PCR products separated on polyacrylamide gels. Biotechniques 17, 915–918.
Scheinvar, E. (2018). Filogeografía de Agave lechuguilla y Patrones de Distribución de Agave en México. Ph.D. thesis Universidad Nacional Autónoma de México, México.
Scheinvar, E., Gámez, N., Castellanos-Morales, G., Aguirre-Planter, E., and Eguiarte, L. E. (2017). Neogene and pleistocene history of Agave lechuguilla in the Chihuahuan Desert. J. Biogeogr. 44, 322–334. doi: 10.1111/jbi.12851
Slatkin, M. (1994). “Gene flow and population structure,” in Ecological Genetics, ed. L. Real (Princeton, NJ: Princeton University Press), 3–17.
Torres, I., Blancas, J., León, A., and Casas, A. (2015a). TEK, local perceptions of risk, and diversity of management practices of Agave inaequidens in Michoacán, Mexico. J. Ethnobiol. Ethnomed. 11:61. doi: 10.1186/s13002-015-0043-1
Torres, I., Casas, A., Vega, E., Martínez-Ramos, M., and Delgado-Lemus, A. (2015b). Population dynamics and sustainable management of mescal agaves in central Mexico: Agave potatorum in the Tehuacán-Cuicatlán Valley. Econ. Bot. 69, 26–41. doi: 10.1007/s12231-014-9295-2
Torres-García, I., Rendón-Sandoval, F. J., Blancas, J., Casas, A., and Moreno-Calles, I. (2019). The genus Agave in agroforestry systems of Mexico. El género Agave en los sistemas agroforestales de México. Bot. Sci. 97, 261–288. doi: 10.17129/botsci.2202
Trame, A.-M., Coddington, A. J., and Paige, K. N. (1995). Field and genetic studies testing optimal outcrossing in Agave schottii, a long-lived clonal plant. Oecologia 104, 93–100.
Trejo, L., Alvarado-Cárdenas, L. O., Scheinvar, E., and Eguiarte, L. E. (2016). Population genetic analysis and bioclimatic modeling in Agave striata in the Chihuahuan Desert indicate higher genetic variation and lower differentiation in drier and more variable environments. Am. J. Bot. 103, 1020–1029.
Trejo, L., Limones, V., Peña, G., Scheinvar, E., Vargas-Ponce, O., Zizumbo-Villarreal, D., et al. (2018). Genetic variation and relationships among agaves related to the production of Tequila and Mezcal in Jalisco. Ind. Crops Prod. 125, 140–149. doi: 10.1016/j.indcrop.2018.08.072
Valdivia-Mares, L., Rodríguez Zaragoza, F. A., Sánchez-González, J., and Vargas-Ponce, O. (2016). Phenology, agronomic and nutritional potential of three wild husk tomato species (Physalis, Solanaceae) from Mexico. Sci. Hortic. 200, 83–94. doi: 10.1016/j.scienta.2016.01.005
Valenzuela-Zapata, A. G. (2015). “Raicillas, mezcales artesanales de Jalisco,” in Agua de las Verdes Matas, Tequila y Mezcal, eds J. L. Vera-Cortés and R. Fernández (México: CONACULTA, INA, ARTES DE MÉXICO), 131–146.
Vargas-Ponce, O., Zizumbo-Villarreal, D., and Colunga-GarcíaMarin, P. (2007). In situ diversity and maintenance of traditional Agave landraces used in spirits production in west-central Mexico. Econ. Bot. 61, 362–375.
Vargas-Ponce, O., Zizumbo-Villarreal, D., Martínez-Castillo, J., Coello-Coello, J., and Colunga-GarcíaMarín, P. (2009). Diversity and structure of landraces of Agave grown for spirits under traditional agriculture: a comparison with wild populations of A. angustifolia (Agavaceae) and commercial plantations of A. tequilana. Am. J. Bot. 96, 448–457. doi: 10.3732/ajb.0800176
Vázquez-García, J., Cházaro-Basañez, M., Hernández-Vera, G., Flores, E., and Vargas-Rodriguez, Y. (2007). Agaves del Occidente de México, 1st Edn. México: Universidad de Guadalajara.
Vellvé, R. (1992). Saving the Seed: Genetic Diversity and European Agriculture. London: Earthscan. doi: 10.4324/9781315066837
Wang, I. (2013). Examining the full effects of landscape heterogeneity on spatial genetic variation: a multiple matrix regression approach for quantifying geographic and ecological isolation. Evolution 67, 3403–3411. doi: 10.1111/evo.12134
Keywords: forestry systems, population genetics, morphological diversity, distilled beverages, raicilla
Citation: Cabrera-Toledo D, Vargas-Ponce O, Ascencio-Ramírez S, Valadez-Sandoval LM, Pérez-Alquicira J, Morales-Saavedra J and Huerta-Galván OF (2020) Morphological and Genetic Variation in Monocultures, Forestry Systems and Wild Populations of Agave maximiliana of Western Mexico: Implications for Its Conservation. Front. Plant Sci. 11:817. doi: 10.3389/fpls.2020.00817
Received: 14 December 2019; Accepted: 20 May 2020;
Published: 17 June 2020.
Edited by:
Luis Enrique Eguiarte, National Autonomous University of Mexico, MexicoReviewed by:
Angelica Cibrian-Jaramillo, Instituto Politécnico Nacional de México (CINVESTAV), MexicoAlejandro Casas, National Autonomous University of Mexico, Mexico
Antonio Gonzalez-Rodriguez, National Autonomous University of Mexico, Mexico
Copyright © 2020 Cabrera-Toledo, Vargas-Ponce, Ascencio-Ramírez, Valadez-Sandoval, Pérez-Alquicira, Morales-Saavedra and Huerta-Galván. This is an open-access article distributed under the terms of the Creative Commons Attribution License (CC BY). The use, distribution or reproduction in other forums is permitted, provided the original author(s) and the copyright owner(s) are credited and that the original publication in this journal is cited, in accordance with accepted academic practice. No use, distribution or reproduction is permitted which does not comply with these terms.
*Correspondence: Dánae Cabrera-Toledo, ZGFuYWV0b2xlZG9AZ21haWwuY29t