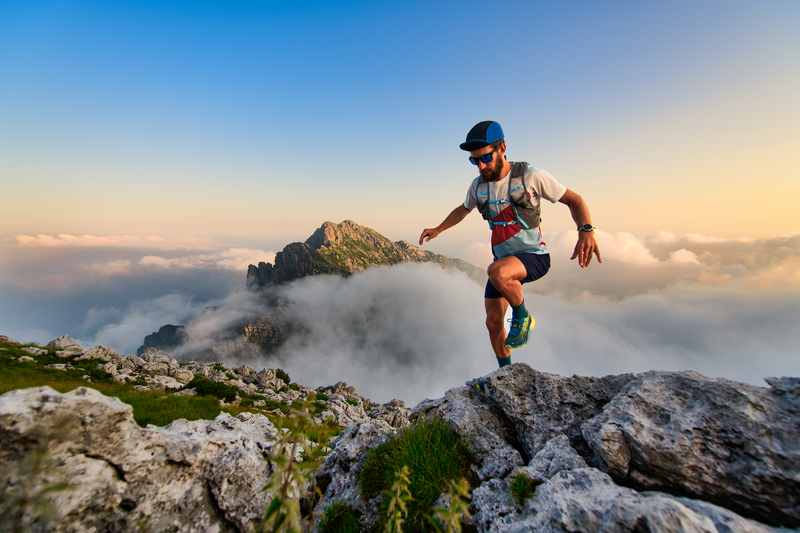
95% of researchers rate our articles as excellent or good
Learn more about the work of our research integrity team to safeguard the quality of each article we publish.
Find out more
MINI REVIEW article
Front. Plant Sci. , 28 May 2020
Sec. Plant Cell Biology
Volume 11 - 2020 | https://doi.org/10.3389/fpls.2020.00565
This article is part of the Research Topic Plant Organelle Interaction Network and Membrane Contact Sites View all 7 articles
Autophagy is an intracellular degradation process, which is highly conserved in eukaryotes. During this process, unwanted cytosolic constituents are sequestered and delivered into the vacuole/lysosome by a double-membrane organelle known as an autophagosome. The autophagosome initiates from a membrane sac named the phagophore, and after phagophore expansion and closure, the outer membrane fuses with the vacuole/lysosome to release the autophagic body into the vacuole. Membrane sources derived from the endomembrane system (e.g., Endoplasmic Reticulum, Golgi and endosome) have been implicated to contribute to autophagosome in different steps (initiation, expansion or maturation). Therefore, coordination between the autophagy-related (ATG) proteins and membrane tethers from the endomembrane system is required during autophagosome biogenesis. In this review, we will update recent findings with a focus on comparing the selected core ATG complexes and the endomembrane tethering machineries for shaping the autophagosome membrane in yeast, mammal, and plant systems.
Macroautophagy (hereafter referred to as autophagy) is an evolutionarily conserved degradative process required for maintaining cellular homeostasis. In this pathway, detrimental or unwanted cellular materials are engulfed by a de novo formed double-membrane autophagosome and delivered into the vacuole (in yeast and plants) or lysosome (in animals) for degradation/recycling (Mizushima et al., 2011; Liu and Bassham, 2012). The autophagosome biogenesis process can be divided into four major stages: initiation of a cup-shaped membrane sac (phagophore/isolation membrane), expansion of the phagophore, autophagosome closure, and autophagosome-vacuole/lysosome fusion (Soto-Burgos et al., 2018; Zhuang et al., 2018). To form a mature autophagosome, a number of autophagy-related (ATG) proteins are employed to orchestrate the membrane shaping of the autophagosome in the different steps of autophagy (Soto-Burgos et al., 2018; Zhuang et al., 2018). In addition, membrane tethers from the endomembrane system have also been implicated to participate in membrane remodeling processes during autophagosome biogenesis. Exciting findings have shed new light on the non-ATG regulators in shaping the autophagosome membrane in plants, such as cytoskeleton, phospholipids, and membrane tethering machineries (e.g., the exocyst complex), which have been reviewed in several recent excellent publications (Tzfadia and Galili, 2013; Pecenkova et al., 2017; Wang et al., 2017; Soto-Burgos et al., 2018; Chung, 2019) and therefore will not be covered here. In this mini review, we will discuss the recent advances of the core ATG machineries as well as the related endomembrane tethering machineries in shaping the autophagosome membrane (Table 1).
In yeast, autophagy is initiated as a pre-autophagosomal structure (PAS) by the hierarchical recruitment of a number of Atg proteins, in particular the Atg1 complex and Atg9 (Yamamoto et al., 2016). The PAS in yeast is often detected in close proximity to both the ER and the vacuole, and a vesicle delivery model has been proposed, contributed by Atg9 vesicles and COPII vesicles (Figure 1A, left; Otomo et al., 2018; Hollenstein et al., 2019). Key players like Ypt1 and the transport protein particle (TRAPP) GEF (guanine-nucleotide exchange factors) complex are both reported to participate in this process (Lipatova et al., 2016). In yeast, 4 types of TRAPP complex (TRAPPI-IV) have been identified, and most homologs of TRAPP subunits are also found in mammal and plants (Lipatova et al., 2016). Upon autophagic induction, the TRAPPIII complex consisting Trs85 is recruited to the PAS for the activation of Ypt1 (Lynch-Day et al., 2010). Of note, it is shown that Trs33, which was originally considered to be a subunit exists in all TRAPP complexes, may assemble into a distinct TRAPP complex in the absence of Trs85 for Ypt1-mediated autophagy as well (Lipatova et al., 2016). Subsequently, Ypt1 triggers the assembly of Atg1-Atg13-Atg17-Atg31-Atg29 complex on the PAS for the clustering of Atg9 vesicles and COPII vesicles to produce a membrane sac (Figure 1A, left). Structural studies have revealed that the Atg17-Atg31-Atg29 subcomplex forms an S-shaped scaffold to bridge two Atg9 vesicles together, while such a crescent-shape structure perfectly matches the size of Atg9 vesicles (∼20–30 nm) (Ragusa et al., 2012). Additionally, COPII subunits also bind to Ypt1 and Atg9, as well as the Atg17-Atg31-Atg29 tethering complex for phagophore initiation (Wang et al., 2014).
Figure 1. Core tethering machineries in autophagosome biogenesis in yeast, mammals, and plants. (A) The phagophore initiation is mediated by the recruitment of Atg1 complex to the pre-autophagosomal structure (PAS) in yeast. Atg9 vesicles can interact with Atg1 complex and provide a membrane source by nucleation to form a cup-shape phagophore. In mammals, trafficking of ATG9 to PAS is mediated by the TRAPPIII complex, and the S-shaped ULK1 complex is recruited to the ATG9-positive ER membrane for phagophore initiation. Another membrane source, COPII vesicles, are involved in the initiation process in both yeast and mammals. In plants, the ER is one of the assembly sites for phagophore initiation, which requires the ATG1 complex and ATG9 vesicles, but the roles of the TRAPPIII complex and COPII vesicles remain unknown (dashed and question mark). (B) In yeast, Atg9 directs the Atg2-Atg18 complex onto the phagophore, which mediates the formation of IM-ER contacts for phagophore expansion. In mammals, WIPI2 interacts with ULK1/FIP200-VAPs complex assembled on the ER membrane to mediate the IM contact with the ER. ATG2-WIPI4 complex interacts with mitochondrial proteins to establish the IM-MAM contact and to recruit ATG9 vesicles to MAM. In plants, ATG18 transiently associates with ATG9 but the function of ATG18-ATG2 in phagophore extension remains unknown (dashed and question mark). In addition, COPII vesicles have been implicated to contribute to phagophore expansion, although their role in plants is unclear (dashed and question mark). (C) Atg8 and ESCRT complex are potential candidates in regulating autophagosome closure. Atg8-PE is likely to promote hemifusion by tethering lipid membrane or another Atg8-PE for the closure process in both yeast and mammals. But it is also suggested that specific LC3 isoforms interact with ATG2 which are anchored to the ER to close the autophagosome in mammals. ESCRT subunits might also participate in the final fission process to seal the autophagosome. (D) In yeast and mammals, distinct pairs of SNAREs are recruited to the autophagosomal membrane and the vacuole/lysosome, respectively. Upon the activation of GTPase, the HOPS complex is recruited to assist in SNARE complex assembly and tethering events for autophagosome-vacuole/lysosome fusion. In Arabidopsis, how Rab GTPase, SNARE complex and HOPS complex are coordinated to regulate autophagosome-vacuole fusion remains elusive (dashed and question mark).
Unlike yeasts which contain a single PAS, multiple PAS have been detected in mammalian cells. The current model for phagophore nucleation involves an intimate membrane association among an omega-shape structure called the omegasome, which emerges from the ER, as well as ATG9 vesicles and COPII vesicles (Figure 1A, middle; Axe et al., 2008). It appears that ATG9 vesicles are more likely to supply proteins/lipids, instead of being directly incorporated into the phagophore membrane. This is supported by a recent study showing that ATG9 vesicles deliver the PI4-kinase, PI4KIIIβ, to the autophagosome initiation site for the recruitment of the ULK1/2 complex (counterpart of yeast ATG1 complex) (Judith et al., 2019). In addition, the TRAPPIII complex in mammal is required for the relocalization of ATG9 vesicles from peripheral recycling endosomes to the early Golgi during autophagy (Lamb et al., 2016). Using high-resolution imaging analysis, it has been observed that ULK and FIP200 are recruited onto the ER membrane prior to their association with the ATG9 vesicles (Karanasios et al., 2016). After that, the ULK1 complex translocates to the ATG9A-positive autophagosome precursors in a PI3P-dependent manner, while ATG9 is phosphorylated by ULK1, thus further promoting ATG9 trafficking under starvation conditions (Zhou et al., 2017). Interestingly, recently it is showed that FIP200 dimerizes as a C-shaped hub for the assembly of the ULK1 complex, but whether the C-shape would fit the ATG9 vesicles to promote phagophore nucleation remains an open question (Shi et al., 2019).
In plant cells, omegasome-like structures have also been observed during autophagy (Zhuang et al., 2013) and conserved ATG1 complex subunits have been identified in the plant genome (Huang et al., 2019; Figure 1A, right). It has been reported that mutation of all ATG1 isoforms significantly compromised autophagosome formation (Huang et al., 2019). Conversely, depletion of plant ATG9 results in abnormal ATG8-positive tubules connecting to the PI3P-enriched ER subdomain, and similar defects are also observed in atg11 mutant, indicating that they both act downstream of ATG1 to organize phagophore assembly (Zhuang et al., 2017; Huang et al., 2019). Interestingly, structural analysis showed that ATG9 may form a trimer, suggesting that ATG9 may recruit additional ATG9-containing vesicles via self-tethering during phagophore nucleation (Lai et al., 2019). However, to get a better understanding of how the ATG1 complex and ATG9 vesicles are assembled at the initiation site, future investigations are needed to examine their hierarchical order (Figure 1A, right).
Later the phagophore will elongate to expand its membrane size for cargo sequestration, and this also requires a subset of membrane remodeling proteins to transport lipids/proteins from the membrane donors. Membrane donors including the ER, ATG9 vesicles, COPII vesicles, as well as other endosomes have also been implicated as contributing to phagophore elongation (Gomez-Sanchez et al., 2018; Osawa et al., 2019; Shima et al., 2019; Figure 1B, left). During phagophore expansion, the PI3K complex mediates the production of PI3P, which further recruits PI3P effectors (e.g., ATG18), as well as the ATG12-ATG5-ATG16 conjugate (Simonsen and Tooze, 2009; Romanov et al., 2012). Afterward, cytosolic ATG8 is conjugated to phosphatidylethanolamine (PE) to form ATG8-PE (LC3-II in mammal) that decorates the phagophore, which may contribute to cargo recognition and phagophore closure (discuss later) (Fujita et al., 2008). In yeast, Atg8 may function in autophagosome expansion, as mutation of atg8 leads to reduced-sized or even no autophagosome production (Xie et al., 2008).
Recent studies have demonstrated that the isolation membrane (IM) expands via lipid transfer from the ER at the IM-ER contact (Gomez-Sanchez et al., 2018). In yeast, Atg2-Atg18 interacts with Atg9 vesicles to mediate Atg9 vesicle recycling, while ATG9 directs Atg2 to the IM-ER contact sites (Figure 1B, left; Gomez-Sanchez et al., 2018). Loss of Atg2-Atg9 interaction compromises the formation of the IM-ER contact site (Gomez-Sanchez et al., 2018). Interestingly, Atg2 also recognizes PI3P, and Atg18 alone cannot bind to PI3P on IM unless Atg2 is present (Gomez-Sanchez et al., 2018; Kotani et al., 2018). Mutation of the Atg2 lipid-binding site leads to shorter IM (Osawa et al., 2019). Moreover, fluorescence-based liposome binding assays have showed that Atg2 has a stronger membrane tethering ability to small liposomes (with high curvature) that are consistent with the size of Atg9 vesicles, suggesting that Atg9 vesicles might be the membrane donor in phagophore expansion (Osawa et al., 2019). Similar to the lipid transport protein vacuolar protein sorting 13 (Vps13), Atg2 also contains a hydrophobic cavity in the conserved N-terminal region, which allows Atg2 to solubilize lipid for lipid transfer (Kumar N. et al., 2018; Osawa et al., 2019).
The ability of lipid transfer is conserved in mammalian ATG2. Structural analysis has revealed that ATG2 is a rod-like protein that forms a complex with WIPI1 (WD repeat domain phosphoinositides-interacting protein 1), WIPI2 and WIPI4 (mammalian homologs of Atg18) to bridge IM-ER contact (Chowdhury et al., 2018; Maeda et al., 2019). Unidirectional lipid transfer from the ER to the PI3P-enriched IM is facilitated by ATG2 with the assistance of WIPI proteins (Figure 1B, middle; Chowdhury et al., 2018; Maeda et al., 2019). In addition, ER receptor vesicle-associated membrane protein-associated proteins (VAPs) have also been reported to mediate the IM-ER contact formation via interaction with FIP200 and ULK1 (Zhao et al., 2018). Then, WIPI2 tethers the ER with the IM via binding to PI3P on IM and the ULK1/FIP200-VAPs on the ER (Figure 1B, middle). VAPs probably stabilize the complex for membrane contacts and loss of VAPs results in impairment of phagophore growth (Zhao et al., 2018). Of note, a recent study also reported accumulation of ATG2 at mitochondria-associated membrane (MAM) (Tang et al., 2019). It has been suggested that after having been directed to MAM by TOM40 on mitochondria, ATG2 will recruit ATG9 vesicles to mediate lipid transfer from ATG9 vesicles to the IM (Figure 1B, middle; Tang et al., 2019). This is supported by the observation that loss of ATG2-ATG9 interaction resulted in a failure of ATG9 vesicle delivery and defects in phagophore expansion (Tang et al., 2019).
Arabidopsis atg2, atg9, and atg18 mutants all show defects in autophagosome formation and display an early senescence phenotype, implying that a conserved plant ATG2-ATG18-ATG9 complex might function in autophagosome expansion as well (Figure 1B, right) (Hanaoka et al., 2002; Zhuang et al., 2017; Kang et al., 2018). Particularly, the levels of both the unlipidated and lipidated ATG8 are significantly increased in all atg2, atg9, and atg18 mutants, suggesting a failure in autophagosome formation (Kang et al., 2018). It is worth noting that in the atg2 mutant, numerous tiny autophagic structures are detected in the cytosol and their delivery into the vacuole are blocked (Kang et al., 2018). Intriguingly, extending tubules positive with both ATG8 and ATG18, which are sensitive to PI3P inhibitor, are accumulated in atg9 mutant (Zhuang et al., 2017). One possible scenario is that ATG9 vesicles may regulate the retrieval of ATG18 and ATG2 from the PAS to prevent excessive expansion of the phagophore.
The closure of the phagophore into an autophagosome is a process involving outer membrane fusion along a “rim of a cup,” while an inner autophagic vesicle is separated from the outer membrane, which is topologically identical to the membrane fission process (Zhou et al., 2019).
In yeast, Atg8-PE itself has been shown to facilitate membrane tethering and hemifusion (Figure 1C, left) (Nakatogawa et al., 2007). In mammalian cells, a similar function for the LC3/GABARAP protein family (the homolog of Atg8) in autophagosome closure has been reported. Knockdown of all six LC3/GABARAP isoforms results in the accumulation of open IMs (Nguyen et al., 2016). Moreover, inhibiting LC3 lipidation also induced the accumulation of open autophagic structures (Fujita et al., 2008). Structural analysis showed that the unique N-terminal region of LC3/GABARAP can bind to both lipid and protein (e.g., another LC3/GABARAP) (Wu et al., 2015), suggesting a multimerization ability of the LC3/GABARAP family for hemifusion. Interestingly, another study revealed a distinct role of ATG2-GABARAP interaction in autophagosome closure (Bozic et al., 2019). Both ATG2A and ATG2B contain LC3 interacting region (LIR) motifs for interaction with LC3/GABARAP. In atg2a/atg2b double knock out cells, or even expressing mutated ATG2A lacking the interaction region to GABARAP, the number of unsealed autophagosomes is significantly increased. However, such defects cannot be complemented in atg2a/atg2b double knock out cells expressing ATG2 which abolishes WIPI4 interaction. Therefore, the ATG2-GABARAP interaction might represent a novel mechanism in autophagosome closure, which is independent of the association of ATG2-WIPI in phagophore expansion. In plants, the great expansion of ATG8 family, which comprises 9 isoforms, hinders the characterization of the specific roles of different ATG8 isoforms in autophagosome formation (Liu and Bassham, 2012). However, future efforts are needed to utilize different tools like in vitro assays and structural analysis to obtain more detailed information on the ATG8 family in plants.
The ESCRT machinery has been well characterized to function in reverse topology scission of membrane, particularly for the formation of the internal vesicles in the multivesicular body (MVB) (Gao et al., 2017). In regard to the similar topology for the invagination of the internal vesicles from the outer membrane, the ESCRT complex would be a good membrane scission candidate for sealing the autophagosome membrane (Figure 1C). Indeed, in most ESCRT mutants, abnormal autophagosome structures are often detected in the cytosol (Takahashi et al., 2018, 2019; Zhou et al., 2019). Recently, using a novel method to distinguish the phagophore and nascent autophagosome by labeling LC3 with permeable and impermeable dyes, it was shown that an ESCRT-III component CHMP2A and an AAA-ATPase vacuolar protein sorting-associated 4 (VPS4) translocate to the phagophore during autophagy (Takahashi et al., 2018). Furthermore, more unsealed phagophore structures and protease-unprotected GFP-LC3-II are detected in chmp2a and vps4 cells. Recently, another ESCRT-I subunit VPS37A, has been identified to recruit the ESCRT machinery onto the phagophore to mediate its closure (Takahashi et al., 2019). Another strong piece of evidence from yeast study is that Atg17 binds to Snf7 for its recruitment to the phagophore in a Rab5-dependent manner (Zhou et al., 2019). Depletion of Snf7, Vps4 and Rab5 GTPase Vps21 all result in an accumulation of immature autophagosomes. Particularly, with an artificially forced Atg17–Snf7 interaction, no defects are displayed in a vps21 mutant, suggesting that Rab5 GTPase catalyzes the Atg17–Snf7 interaction for autophagosome closure.
In plants, the essential roles of ESCRT machinery in plant MVB-mediated pathways have been well documented and abnormal autophagosomes are accumulated in several ESCRT-related mutants as well (Gao et al., 2017). For instance, in the chmp1 mutant, delayed autophagosome closure as well as abnormal pattern in plastid division is observed, indicating that CHMP1 may function in closure of autophagosome for sequestering plastid cargo (Spitzer et al., 2015). Autophagosome maturation and delivery into the vacuole are also severely suppressed when another plant unique ESCRT subunit, FREE1 is mutated. Further evidence demonstrates a direct link between the ESCRT machinery and autophagic machinery via a FREE1-SH3P2 interaction (Gao et al., 2015), whereby SH3P2 has been shown to interact with ATG8 and to translocate onto the phagophore upon autophagic induction (Zhuang et al., 2013). However, the regulatory mechanism of plant ESCRT in autophagosome closure awaits further investigations (Figure 1C, right).
In the endomembrane system, membrane tethering machineries, including the soluble N-ethylmaleimide-sensitive factor attachment receptor (SNARE) complex and homotypic fusion and vacuole protein sorting (HOPS) complex, have been well characterized to mediate the fusion between the vacuole/lysosome and other vesicles (Itakura et al., 2012; Jiang et al., 2014; Bas et al., 2018; Matsui et al., 2018).
The SNARE complex can change its conformation to shorten the distance between two compartments and facilitate membrane insertion into the target membrane (Jahn and Scheller, 2006). Two types of SNAREs, Q-SNAREs and R-SNARE, which are localized on the membrane acceptor and the membrane donor, respectively, assemble into a four-helix SNARE complex and recruit the HOPS complex (Jahn and Scheller, 2006). In yeast, the Q-SNARE on the vacuole, comprised of Vti1, Vam3, Vam7, cooperates with the R-SNARE on autophagosome, Ykt6, to mediate the autophagosome/vacuole fusion (Bas et al., 2018). The HOPS complex, which is highly conserved in yeast, mammals, and plants, is characterized as an elongated seahorse-like tether (Brocker et al., 2012). To activate the HOPS complex, Rab7-like GTPase Ypt7 is recruited onto the autophagosome membrane via the Mon1-CcZ1 (monensin sensitivity protein 1 -caffeine, calcium, and zinc 1) GEF complex, which is conserved in yeast, mammals and plants (Cui et al., 2014; Gao et al., 2018). Importantly, Ccz1 consists of an LC3-interacting region (LIR) motif which interacts with Atg8 on the autophagosome, activating Ypt7 for its recruitment via binding to PI3P on the autophagosome (Gao et al., 2018). However, mutation of the PI3K subunits Atg14 and Vps34, which facilitate the PI3P synthesis, strongly inhibits autophagosome fusion with the vacuole, suggesting PI3P serves as a prerequisite for the assembly of this tethering complex (Bas et al., 2018). Ypt7 subsequently binds to the two Ypt7-binding sites at the two ends of the HOPS complex to catalyze its flexible bending for driving the autophagosome-vacuole fusion (Figure 1D, left) (Balderhaar and Ungermann, 2013).
In mammalian cells, autophagosome-lysosome fusion is mediated by a SNARE complex consisting STX17-SNAP29-VAMP7/8 (Figure 1D, middle; Itakura et al., 2012). STX17 is recruited to autophagosomes via binding to the immunity-related GTPase M (IRGM) and LC3 on the autophagosomes (Kumar S. et al., 2018). Interestingly, STX17 interacts with all the HOPS subunits, and STX17 knockdown leads to improper autolysosome formation (Jiang et al., 2014). Another SNARE complex STX7-SNAP29-YKT6 also contributes to the fusion process, and the HOPS complex is likely to be recruited by STX7 (Jiang et al., 2014; Matsui et al., 2018). Different to yeast, it is reported that RAB7 and RAB2, which localize to the vacuole and autophagosomes, respectively, bind to the HOPS complex to trigger the autophagosome-lysosome fusion (Fujita et al., 2017; Figure 1D, middle).
Multiple SNAREs have been identified in Arabidopsis, but the involvement and underlying mechanisms of SNAREs in autophagy are largely unexplored (Fujiwara et al., 2014). In Arabidopsis, the VTI family (VTI11/12/13), the homologs of yeast Vti1, functions as Qb-SNARE and other associated Q-SNAREs have been identified via an interactomic approach (Surpin et al., 2003; Fujiwara et al., 2014). VTI11 interacts with syntaxin of plants 2 (SYP2), SYP5 and VAMP7 to form a Q-SNARE complex, while VTI12 assembles with SYP4, SYP6, and YKT6 (Figure 1D, right; Surpin et al., 2003; Fujiwara et al., 2014). These two Q-SNAREs participate in trans-Golgi networking and vacuole trafficking, as well as vacuole biogenesis (Ebine et al., 2008; Zouhar et al., 2009). The possible involvement of these SNARE proteins in plant autophagy is supported by the observation that both vti11 and vti12 mutants exhibit autophagy-associated phenotypes and abnormal autophagosomes are accumulated in vti1 (Surpin et al., 2003). On the other hand, one Q-SNARE subunit, VAMP713 (vesicle-associated membrane protein 713), has been shown to interact with the HOPS complex to mediate vacuole fusion together with RAB7 (RabG3f) in plants (Takemoto et al., 2018). In addition, another Q-SNARE SYP22 binds the core subunit VPS33 of HOPS complex to mediate vacuolar fusion (Brillada et al., 2018). Importantly, proper localization of both VPS33 and the unique subunit VPS41 of HOPS complex to the vacuole is VTI11-dependent (Brillada et al., 2018). Therefore, it is very likely that a coordination between the HOPS complex and SNARE complex also operates in plant cells for heterotypic fusion with the vacuole membrane. Further studies are needed to investigate how they assemble and coordinate to tether the autophagosome and vacuole membrane (Figure 1D, right).
The de novo formation of autophagosomes needs drastic lipid synthesis, transfer, exchange, and fusion to accomplish its fate into the vacuole for cargo sequestration and degradation. Sophisticated regulation mechanisms and machineries are utilized to shape the nascent membrane into a mature autophagosome timely and spatially, particularly with the coordination between the core ATG proteins and conserved membrane tethering complexes derived from the endomembrane system, thereby fulfilling the needs of different cell types under specific developmental processes or unfavorable conditions. A recent study also highlighted a role of the endocytic machinery in mediating autophagosome initiation at the ER-PM contact sites (Wang et al., 2019). Nevertheless, the diversity and conservation of regulatory mechanisms, models and novel tools learnt from yeast and mammalian systems, provides useful future insights for plant autophagy research. Autophagy has been implicated to function in unique plant cell types for plant development and growth. Therefore, future investigations in the autophagosome biogenesis in plant cells should provide valuable information for crop engineering to improve nutrient utilization and yield.
C-LW, YQ, and XZ designed the concept and the organization of the manuscript, and wrote and edited the manuscript.
This work was supported by grants from the Research Grants Council of Hong Kong (G-CUHK404/18, C4002-17G, R4005-18F, AoE/M-05/12, and AoE/M-403/16) and CUHK Research Committee.
The authors declare that the research was conducted in the absence of any commercial or financial relationships that could be construed as a potential conflict of interest.
We apologize to researchers whose work has not been included in this manuscript owing to a limit in space.
Axe, E. L., Walker, S. A., Manifava, M., Chandra, P., Roderick, H. L., Habermann, A., et al. (2008). Autophagosome formation from membrane compartments enriched in phosphatidylinositol 3-phosphate and dynamically connected to the endoplasmic reticulum. J. Cell Biol. 182, 685–701. doi: 10.1083/jcb.200803137
Balderhaar, H. J., and Ungermann, C. (2013). CORVET and HOPS tethering complexes - coordinators of endosome and lysosome fusion. J. Cell Sci. 126, 1307–1316. doi: 10.1242/jcs.107805
Bas, L., Papinshi, D., Licheva, M., Torggler, R., Rohringer, S., Schuschnig, M., et al. (2018). Reconstitution reveals Ykt6 as the autophagosomal SNARE in autophagosome–vacuole fusion. J. Cell Biol. 217, 3656–3669. doi: 10.1083/jcb.201804028
Bozic, M., van den Bekerom, L., Milne, B. A., Goodman, N., Roberston, L., Prescott, A. R., et al. (2019). A conserved ATG2-GABARAP interaction is critical for phagophore closure. bioRxiv [Preprint]. doi: 10.1101/624627
Brillada, C., Zheng, J., Kruger, F., Rovira-Diaz, E., Askani, J. C., Schumacher, K., et al. (2018). Phosphoinositides control the localization of HOPS subunit VPS41, which together with VPS33 mediates vacuole fusion in plants. Proc. Natl. Acad. Sci. U.S.A. 115, E8305–E8314. doi: 10.1073/pnas.1807763115
Brocker, C., Kuhlee, A., Gatsogiannis, C., Balderhaar, H. J., Honscher, C., Engelbrecht-Vandre, S., et al. (2012). Molecular architecture of the multisubunit homotypic fusion and vacuole protein sorting (HOPS) tethering complex. Proc. Natl. Acad. Sci. U.S.A. 109, 1991–1996. doi: 10.1073/pnas.1117797109
Chowdhury, S., Otomo, C., Leitner, A., Ohashi, K., Aebersold, R., Lander, G. C., et al. (2018). Insights into autophagosome biogenesis from structural and biochemical analyses of the ATG2A-WIPI4 complex. Proc. Natl. Acad. Sci. U.S.A. 115, E9792–E9801. doi: 10.1073/pnas.1811874115
Chung, T. (2019). How phosphoinositides shape autophagy in plant cells. Plant Sci. 281, 146–158. doi: 10.1016/j.plantsci.2019.01.017
Cui, Y., Zhao, Q., Gao, C., Ding, Y., Zeng, Y., Ueda, T., et al. (2014). Activation of the Rab7 GTPase by the MON1-CCZ1 complex is essential for PVC-to-vacuole trafficking and plant growth in Arabidopsis. Plant Cell 26, 2080–2097. doi: 10.1105/tpc.114.123141
Ebine, K., Okatani, Y., Uemura, T., Goh, T., Shoda, K., Niihama, M., et al. (2008). A SNARE complex unique to seed plants is required for protein storage vacuole biogenesis and seed development of Arabidopsis thaliana. Plant Cell 20, 3006–3021. doi: 10.1105/tpc.107.057711
Fujita, N., Hayashi-Nishino, M., Fukumoto, H., Omori, H., Yamamoto, A., Noda, T., et al. (2008). An Atg4B mutant hampers the lipidation of LC3 paralogues and causes defects in autophagosome closure. Mol. Biol. Cell 19, 4651–4659. doi: 10.1091/mbc.E08-03-0312
Fujita, N., Huang, W., Lin, T. H., Groulx, J. F., Jean, S., Nguyen, J., et al. (2017). Genetic screen in Drosophila muscle identifies autophagy-mediated T-tubule remodeling and a Rab2 role in autophagy. eLife 6:e23367. doi: 10.7554/eLife.23367
Fujiwara, M., Uemura, T., Ebine, K., Nishimori, Y., Ueda, T., Nakano, A., et al. (2014). Interactomics of Qa-SNARE in Arabidopsis thaliana. Plant Cell Physiol. 55, 781–789. doi: 10.1093/pcp/pcu038
Gao, C., Zhuang, X., Cui, Y., Fu, X., He, Y., Zhao, Q., et al. (2015). Dual roles of an Arabidopsis ESCRT component FREE1 in regulating vacuolar protein transport and autophagic degradation. Proc. Natl. Acad. Sci. U.S.A. 112, 1886–1891. doi: 10.1073/pnas.1421271112
Gao, C., Zhuang, X., Shen, J., and Jiang, L. (2017). Plant ESCRT complexes: moving beyond endosomal sorting. Trends Plant Sci. 22, 986–998. doi: 10.1016/j.tplants.2017.08.003
Gao, J., Langemeyer, L., Kummel, D., Reggiori, F., and Ungermann, C. (2018). Molecular mechanism to target the endosomal Mon1-Ccz1 GEF complex to the pre-autophagosomal structure. eLife 7:e31145. doi: 10.7554/eLife.31145
Gomez-Sanchez, R., Rose, J., Guimaraes, R., Mari, M., Papinski, D., Rieter, E., et al. (2018). Atg9 establishes Atg2-dependent contact sites between the endoplasmic reticulum and phagophores. J. Cell Biol. 217, 2743–2763. doi: 10.1083/jcb.201710116
Hanaoka, H., Noda, T., Shirano, Y., Kato, T., Hayashi, H., Shibata, D., et al. (2002). Leaf senescence and starvation-induced chlorosis are accelerated by the disruption of an Arabidopsis autophagy gene. Plant Physiol. 129, 1181–1193. doi: 10.1104/pp.011024
Hollenstein, D. M., Gomez-Sanchez, R., Ciftci, A., Kriegenburg, F., Mari, M., Torggler, R., et al. (2019). Vac8 spatially confines autophagosome formation at the vacuole in S. cerevisiae. J. Cell Sci. 132:jcs235002. doi: 10.1242/jcs.235002
Huang, X., Zheng, C., Liu, F., Yang, C., Zheng, P., Lu, X., et al. (2019). Genetic analyses of the Arabidopsis ATG1 kinase complex reveal both kinase-dependent and independent autophagic routes during fixed-carbon starvation. Plant Cell 31, 2973–2995. doi: 10.1105/tpc.19.00066
Itakura, E., Kishi-Itakura, C., and Mizushima, N. (2012). The hairpin-type tail-anchored SNARE syntaxin 17 targets to autophagosomes for fusion with endosomes/lysosomes. Cell 151, 1256–1269. doi: 10.1016/j.cell.2012.11.001
Jahn, R., and Scheller, R. H. (2006). SNAREs–engines for membrane fusion. Nat. Rev. Mol. Cell Biol. 7, 631–643. doi: 10.1038/nrm2002
Jiang, P., Nishimura, T., Sakamaki, Y., Itakura, E., Hatta, T., Natsume, T., et al. (2014). The HOPS complex mediates autophagosome-lysosome fusion through interaction with syntaxin 17. Mol. Biol. Cell 25, 1327–1337. doi: 10.1091/mbc.E13-08-0447
Judith, D., Jefferies, H. B. J., Boeing, S., Frith, D., Snijders, A. P., and Tooze, S. A. (2019). ATG9A shapes the forming autophagosome through Arfaptin 2 and phosphatidylinositol 4-kinase IIIbeta. J. Cell Biol. 218, 1634–1652. doi: 10.1083/jcb.201901115
Kang, S., Shin, K. D., Kim, J. H., and Chung, T. (2018). Autophagy-related (ATG) 11, ATG9 and the phosphatidylinositol 3-kinase control ATG2-mediated formation of autophagosomes in Arabidopsis. Plant Cell Rep. 37, 653–664. doi: 10.1007/s00299-018-2258-9
Karanasios, E., Walker, S. A., Okkenhaug, H., Manifava, M., Hummel, E., Zimmermann, H., et al. (2016). Autophagy initiation by ULK complex assembly on ER tubulovesicular regions marked by ATG9 vesicles. Nat. Commun. 7:12420. doi: 10.1038/ncomms12420
Kotani, T., Kirisako, H., Koizumi, M., Ohsumi, Y., and Nakatogawa, H. (2018). The Atg2-Atg18 complex tethers pre-autophagosomal membranes to the endoplasmic reticulum for autophagosome formation. Proc. Natl. Acad. Sci. U.S.A. 115, 10363–10368. doi: 10.1073/pnas.1806727115
Kumar, N., Leonzino, M., Hancock-Cerutti, W., Horenkamp, F. A., Li, P., Lees, J. A., et al. (2018). VPS13A and VPS13C are lipid transport proteins differentially localized at ER contact sites. J. Cell Biol. 217, 3625–3639. doi: 10.1083/jcb.201807019
Kumar, S., Jain, A., Farzam, F., Jia, J., Gu, Y., Choi, S. W., et al. (2018). Mechanism of Stx17 recruitment to autophagosomes via IRGM and mammalian Atg8 proteins. J. Cell Biol. 217, 997–1013. doi: 10.1083/jcb.201708039
Lai, L. T. F., Yu, C., Wong, J. S. K., Lo, H. S., Benlekbir, S., Jiang, L., et al. (2019). Subnanometer resolution cryo-EM structure of Arabidopsis thaliana ATG9. Autophagy 2019, 1–9. doi: 10.1080/15548627.2019.1639300
Lamb, C. A., Nuhlen, S., Judith, D., Frith, D., Snijders, A. P., Behrends, C., et al. (2016). TBC1D14 regulates autophagy via the TRAPP complex and ATG9 traffic. EMBO J. 35, 281–301. doi: 10.15252/embj.201592695
Lipatova, Z., Majumdar, U., and Segev, N. (2016). Trs33-containing TRAPP IV: a novel autophagy-specific Ypt1 GEF. Genetics 204, 1117–1128. doi: 10.1534/genetics.116.194910
Liu, Y., and Bassham, D. C. (2012). Autophagy: pathways for self-eating in plant cells. Annu. Rev. Plant Biol. 63, 215–237. doi: 10.1146/annurev-arplant-042811-105441
Lynch-Day, M. A., Bhandari, D., Menon, S., Huang, J., Cai, H., Bartholomew, C. R., et al. (2010). Trs85 directs a Ypt1 GEF, TRAPPIII, to the phagophore to promote autophagy. Proc. Natl. Acad. Sci. U.S.A. 107, 7811–7816. doi: 10.1073/pnas.1000063107
Maeda, S., Otomo, C., and Otomo, T. (2019). The autophagic membrane tether ATG2A transfers lipids between membranes. eLife 8:e45777. doi: 10.7554/eLife.45777
Matsui, T., Jiang, P., Nakano, S., Sakamaki, Y., Yamamoto, H., and Mizushima, N. (2018). Autophagosomal YKT6 is required for fusion with lysosomes independently of syntaxin 17. J. Cell Biol. 217, 2633–2645. doi: 10.1083/jcb.201712058
Mizushima, N., Yoshimori, T., and Ohsumi, Y. (2011). The role of Atg proteins in autophagosome formation. Annu. Rev. Cell Dev. Biol. 27, 107–132. doi: 10.1146/annurev-cellbio-092910-154005
Nakatogawa, H., Ichimura, Y., and Ohsumi, Y. (2007). Atg8, a ubiquitin-like protein required for autophagosome formation, mediates membrane tethering and hemifusion. Cell 130, 165–178. doi: 10.1016/j.cell.2007.05.021
Nguyen, T. N., Padman, B. S., Usher, J., Oorschot, V., Ramm, G., and Lazarou, M. (2016). Atg8 family LC3/GABARAP proteins are crucial for autophagosome-lysosome fusion but not autophagosome formation during PINK1/Parkin mitophagy and starvation. J. Cell Biol. 215, 857–874. doi: 10.1083/jcb.201607039
Osawa, T., Kotani, T., Kawaoka, T., Hirata, E., Suzuki, K., Nakatogawa, H., et al. (2019). Atg2 mediates direct lipid transfer between membranes for autophagosome formation. Nat. Struct. Mol. Biol. 26, 281–288. doi: 10.1038/s41594-019-0203-4
Otomo, T., Chowdhury, S., and Lander, G. C. (2018). The rod-shaped ATG2A-WIPI4 complex tethers membranes in vitro. Contact 1:2515256418819936. doi: 10.1177/2515256418819936
Pecenkova, T., Markovic, V., Sabol, P., Kulich, I., and Zarsky, V. (2017). Exocyst and autophagy-related membrane trafficking in plants. J. Exp. Bot. 69, 47–57. doi: 10.1093/jxb/erx363
Ragusa, M. J., Stanley, R. E., and Hurley, J. H. (2012). Architecture of the Atg17 complex as a scaffold for autophagosome biogenesis. Cell 151, 1501–1512. doi: 10.1016/j.cell.2012.11.028
Romanov, J., Walczak, M., Ibiricu, I., Schuchner, S., Ogris, E., Kraft, C., et al. (2012). Mechanism and functions of membrane binding by the Atg5-Atg12/Atg16 complex during autophagosome formation. EMBO J. 31, 4304–4317. doi: 10.1038/emboj.2012.278
Shi, X., Yokom, A. L., Wang, C., Young, L. N., Youle, R. J., and Hurley, H. (2019). The FIP200 N-terminal domain orchestrates assembly of the autophagy-inducing ULK complex. biorxiv [Preprint]. doi: 10.1101/840009
Shima, T., Kirisako, H., and Nakatogawa, H. (2019). COPII vesicles contribute to autophagosomal membranes. J. Cell Biol. 218, 1503–1510. doi: 10.1083/jcb.201809032
Simonsen, A., and Tooze, S. A. (2009). Coordination of membrane events during autophagy by multiple class III PI3-kinase complexes. J. Cell Biol. 186, 773–782. doi: 10.1083/jcb.200907014
Soto-Burgos, J., Zhuang, X., Jiang, L., and Bassham, D. C. (2018). Dynamics of autophagosome formation. Plant Physiol. 176, 219–229. doi: 10.1104/pp.17.01236
Spitzer, C., Li, F., Buono, R., Roschzttardtz, H., Chung, T., Zhang, M., et al. (2015). The endosomal protein CHARGED MULTIVESICULAR BODY PROTEIN1 regulates the autophagic turnover of plastids in Arabidopsis. Plant Cell 27, 391–402. doi: 10.1105/tpc.114.135939
Surpin, M., Zheng, H., Morita, M. T., Saito, C., Avila, E., Blakeslee, J. J., et al. (2003). The VTI family of SNARE proteins is necessary for plant viability and mediates different protein transport pathways. Plant Cell 15, 2885–2899. doi: 10.1105/tpc.016121
Takahashi, Y., He, H., Tang, Z., Hattori, T., Liu, Y., Young, M. M., et al. (2018). An autophagy assay reveals the ESCRT-III component CHMP2A as a regulator of phagophore closure. Nat. Commun. 9:2855. doi: 10.1038/s41467-018-05254-w
Takahashi, Y., Liang, X., Hattori, T., Tang, Z., He, H., Chen, H., et al. (2019). VPS37A directs ESCRT recruitment for phagophore closure. J. Cell Biol. 218, 3336–3354. doi: 10.1083/jcb.201902170
Takemoto, K., Ebine, K., Askani, J. C., Kruger, F., Gonzalez, Z. A., Ito, E., et al. (2018). Distinct sets of tethering complexes, SNARE complexes, and Rab GTPases mediate membrane fusion at the vacuole in Arabidopsis. Proc. Natl. Acad. Sci. U.S.A. 115, E2457–E2466. doi: 10.1073/pnas.1717839115
Tang, Z., Takahashi, Y., He, H., Hattori, T., Chen, C., Liang, X., et al. (2019). TOM40 targets Atg2 to mitochondria-associated ER membranes for phagophore expansion. Cell Rep. 28, 1744–1757.e5. doi: 10.1016/j.celrep.2019.07.036
Tzfadia, O., and Galili, G. (2013). The Arabidopsis exocyst subcomplex subunits involved in a golgi-independent transport into the vacuole possess consensus autophagy-associated atg8 interacting motifs. Plant Signal. Behav. 8:e26732. doi: 10.4161/psb.26732
Wang, J., Tan, D., Cai, Y., Reinisch, K. M., Walz, T., and Ferro-Novick, S. (2014). A requirement for ER-derived COPII vesicles in phagophore initiation. Autophagy 10, 708–709. doi: 10.4161/auto.28103
Wang, P., Hawkins, T. J., and Hussey, P. J. (2017). Connecting membranes to the actin cytoskeleton. Curr. Opin. Plant Biol. 40, 71–76. doi: 10.1016/j.pbi.2017.07.008
Wang, P., Pleskot, R., Zang, J., Winkler, J., Wang, J., Yperman, K., et al. (2019). Plant AtEH/Pan1 proteins drive autophagosome formation at ER-PM contact sites with actin and endocytic machinery. Nat. Commun. 10:5132. doi: 10.1038/s41467-019-12782-6
Wu, F., Watanabe, Y., Guo, X. Y., Qi, X., Wang, P., Zhao, H. Y., et al. (2015). Structural basis of the differential function of the two C. elegans Atg8 homologs, LGG-1 and LGG-2, in autophagy. Mol. Cell 60, 914–929. doi: 10.1016/j.molcel.2015.11.019
Xie, Z., Nair, U., and Klionsky, D. J. (2008). Atg8 controls phagophore expansion during autophagosome formation. Mol. Biol. Cell 19, 3290–3298. doi: 10.1091/mbc.E07-12-1292
Yamamoto, H., Fujioka, Y., Suzuki, S. W., Noshiro, D., Suzuki, H., Kondo-Kakuta, C., et al. (2016). The intrinsically disordered protein Atg13 mediates supramolecular assembly of autophagy initiation complexes. Dev. Cell 38, 86–99. doi: 10.1016/j.devcel.2016.06.015
Zhao, Y. G., Liu, N., Miao, G., Chen, Y., Zhao, H., and Zhang, H. (2018). The ER contact proteins VAPA/B interact with multiple autophagy proteins to modulate autophagosome biogenesis. Curr. Biol. 28, 1234–1245.e4. doi: 10.1016/j.cub.2018.03.002
Zhou, C., Ma, K., Gao, R., Mu, C., Chen, L., Liu, Q., et al. (2017). Regulation of mATG9 trafficking by Src- and ULK1-mediated phosphorylation in basal and starvation-induced autophagy. Cell Res. 27, 184–201. doi: 10.1038/cr.2016.146
Zhou, F., Wu, Z., Zhao, M., Murtazina, R., Cai, J., Zhang, A., et al. (2019). Rab5-dependent autophagosome closure by ESCRT. J. Cell Biol. 218, 1908–1927. doi: 10.1083/jcb.201811173
Zhuang, X., Chung, K. P., Cui, Y., Lin, W., Gao, C., Kang, B. H., et al. (2017). ATG9 regulates autophagosome progression from the endoplasmic reticulum in Arabidopsis. Proc. Natl. Acad. Sci. U.S.A. 114, E426–E435. doi: 10.1073/pnas.1616299114
Zhuang, X., Chung, K. P., Luo, M., and Jiang, L. (2018). Autophagosome biogenesis and the endoplasmic reticulum: a plant perspective. Trends Plant Sci. 23, 677–692. doi: 10.1016/j.tplants.2018.05.002
Zhuang, X., Wang, H., Lam, S. K., Gao, C., Wang, X., Cai, Y., et al. (2013). A BAR-domain protein SH3P2, which binds to phosphatidylinositol 3-phosphate and ATG8, regulates autophagosome formation in Arabidopsis. Plant Cell 25, 4596–4615. doi: 10.1105/tpc.113.118307
Keywords: autophagosome, membrane tethering, lipid transfer, vesicle fusion, membrane shaping
Citation: Wun C-L, Quan Y and Zhuang X (2020) Recent Advances in Membrane Shaping for Plant Autophagosome Biogenesis. Front. Plant Sci. 11:565. doi: 10.3389/fpls.2020.00565
Received: 06 January 2020; Accepted: 15 April 2020;
Published: 28 May 2020.
Edited by:
Patrick J. Hussey, Durham University, United KingdomReviewed by:
Tamara Pecenkova, Institute of Experimental Botany (CAS), CzechiaCopyright © 2020 Wun, Quan and Zhuang. This is an open-access article distributed under the terms of the Creative Commons Attribution License (CC BY). The use, distribution or reproduction in other forums is permitted, provided the original author(s) and the copyright owner(s) are credited and that the original publication in this journal is cited, in accordance with accepted academic practice. No use, distribution or reproduction is permitted which does not comply with these terms.
*Correspondence: Xiaohong Zhuang, eGh6aHVhbmdAY3Voay5lZHUuaGs=
Disclaimer: All claims expressed in this article are solely those of the authors and do not necessarily represent those of their affiliated organizations, or those of the publisher, the editors and the reviewers. Any product that may be evaluated in this article or claim that may be made by its manufacturer is not guaranteed or endorsed by the publisher.
Research integrity at Frontiers
Learn more about the work of our research integrity team to safeguard the quality of each article we publish.