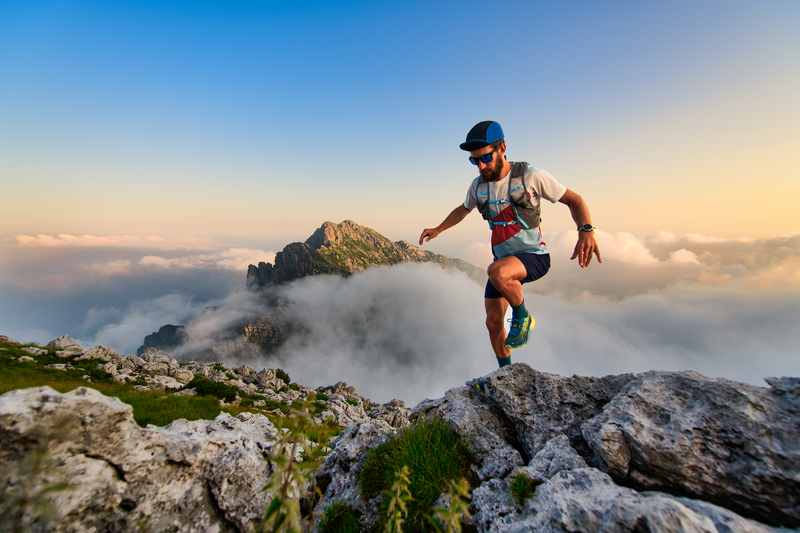
95% of researchers rate our articles as excellent or good
Learn more about the work of our research integrity team to safeguard the quality of each article we publish.
Find out more
ORIGINAL RESEARCH article
Front. Plant Sci. , 29 April 2020
Sec. Plant Pathogen Interactions
Volume 11 - 2020 | https://doi.org/10.3389/fpls.2020.00476
This article is part of the Research Topic RNAi Based Pesticides View all 15 articles
Over the last decade, several studies have revealed the enormous potential of RNA-silencing strategies as a potential alternative to conventional pesticides for plant protection. We have previously shown that targeted gene silencing mediated by an in planta expression of non-coding inhibitory double-stranded RNAs (dsRNAs) can protect host plants against various diseases with unprecedented efficiency. In addition to the generation of RNA-silencing (RNAi) signals in planta, plants can be protected from pathogens, and pests by spray-applied RNA-based biopesticides. Despite the striking efficiency of RNA-silencing-based technologies holds for agriculture, the molecular mechanisms underlying spray-induced gene silencing (SIGS) strategies are virtually unresolved, a requirement for successful future application in the field. Based on our previous work, we predict that the molecular mechanism of SIGS is controlled by the fungal-silencing machinery. In this study, we used SIGS to compare the silencing efficiencies of computationally-designed vs. manually-designed dsRNA constructs targeting ARGONAUTE and DICER genes of Fusarium graminearum (Fg). We found that targeting key components of the fungal RNAi machinery via SIGS could protect barley leaves from Fg infection and that the manual design of dsRNAs resulted in higher gene-silencing efficiencies than the tool-based design. Moreover, our results indicate the possibility of cross-kingdom RNA silencing in the Fg-barley interaction, a phenomenon in which sRNAs operate as effector molecules to induce gene silencing between species from different kingdoms, such as a plant host and their interacting pathogens.
Diseases of cereal crops, such as Fusarium head blight caused by phytopathogenic fungi of the genus Fusarium and primarily by the ascomycete Fusarium graminearum (Fg), exert great economic and agronomic impacts on global grain production and the grain industry (Goswami and Kistler, 2004; Kazan et al., 2012; McMullen et al., 2012). In addition to significant yield losses, food quality is adversely affected by grain contamination with mycotoxins, representing a serious threat to human and animal health (Ismaiel and Papenbrock, 2015). Plant-protection and toxin-reduction strategies are presently mediated by chemical treatments. Currently, the application of systemic fungicides, such as sterol demethylation inhibitors (DMIs), is essential for controlling Fusarium diseases and to assist in reaching the maximum attainable production level of high-yield cultivars. DMI fungicides act as ergosterol biosynthesis inhibitors because of cytochrome P450 lanosterol C-14α-demethylase (CYP51) binding, which subsequently disturbs fungal membrane integrity (Kuck et al., 2012). Because of a shortage of alternative chemicals, DMIs have been used extensively in the field since their discovery in the 1970s. Therefore, it is hardly surprising that reduced sensitivity, or even resistance to DMI fungicides, has begun to develop in many plant pathogenic fungi (Yin et al., 2009; Spolti et al., 2014). These alarming developments demonstrate that novel strategies in pathogen and pest control are urgently needed.
RNAi is known as a conserved and integral part of the gene regulation processes present in all eukaryotes and is mediated by small RNAs (sRNAs) that direct gene silencing at the post-transcriptional level. Post-transcriptional gene silencing (PTGS) starts with the initial processing or cleavage of a precursor double-stranded (ds)RNA into short 21–24 nucleotide (nt) small-interfering RNA (siRNA) duplexes by an RNaseIII-like enzyme called Dicer (Baulcombe, 2004; Ketting, 2011). Double-stranded siRNAs are incorporated into an RNA-induced silencing complex (RISC) that initially unwinds the siRNA, thereby generating an antisense (or guide) strand which base-pairs with complementary mRNA target sequences. Subsequent degradation of the targeted mRNA mediated by an RNase protein called Argonaute (AGO) prevents translation of the target transcript (Vaucheret et al., 2004; Borges and Martienssen, 2015) ideally resulting in a loss of function phenotype. Therefore, RNAi has emerged as a powerful genetic tool not only in fundamental research for the assessment of gene function but also in various fields of applied research, such as agriculture. In plants, RNAi strategies have the potential to protect host plants against infection by pathogens or predation by pests mediated by lethal RNAi signals generated in planta, a strategy known as ‘host-induced gene silencing’ (HIGS; Nowara et al., 2010) (for review, see Koch and Kogel, 2014; Yin and Hulbert, 2015; Guo et al., 2016; Zhang et al., 2017; Gaffar and Koch, 2019; Qi et al., 2019). In addition to the generation of RNA-silencing signals in planta, plants can be protected from pathogens and pests by spray-applied RNA biopesticides designated as spray-induced gene silencing (SIGS) (Koch et al., 2016; Wang et al., 2016; Konakalla et al., 2016; Mitter et al., 2017a; Kaldis et al., 2018; Koch et al., 2019). Regardless of how target-specific inhibitory RNAs are applied (i.e., endogenously or exogenously), the use of HIGS and SIGS technologies to control Fusarium species have been shown to be a potential alternative to conventional pesticides (Koch et al., 2013; Ghag et al., 2014; Cheng et al., 2015; Hu et al., 2015; Chen et al., 2016; Pareek and Rajam, 2017; Bharti et al., 2017; Baldwin et al., 2018; Koch et al., 2018, 2019) supporting the notion that RNAi strategies may improve food safety by controlling the growth of phytopathogenic, mycotoxin-producing fungi (reviewed by Majumdar et al., 2017; Machado et al., 2018).
Despite the notable efficiency the RNAi-based technology holds for agriculture, the mechanisms underlying HIGS and SIGS technologies are inadequately understood. There is little information regarding the contribution of either plant- or fungal-silencing machinery in cross-species RNA silencing (i.e., plant and fungus) or how inhibitory RNAs translocate from the plant to the fungus after its transgenic expression or spray application. Whereas HIGS is virtually based on the plant’s ability to produce mobile siRNAs (through plant Dicers [DCLs]), the mechanism of gene silencing by exogenously delivered dsRNA depends primarily on the fungal RNAi machinery, mainly fungal DCLs (Koch et al., 2016; Gaffar et al., 2019). Interestingly, recent studies revealed that AGO and DCL proteins of Fg contribute to fungal vegetative and generative growth, mycotoxin production, antiviral response, sensitivity to environmental RNAi, and plant disease development (Kim et al., 2015; Son et al., 2017; Yu et al., 2018; Gaffar et al., 2019). In Fg, two Dicer proteins (FgDCL1 and FgDCL2) and two AGO proteins (FgAGO1 and FgAGO2) were identified (Chen et al., 2015). Characterization of those RNAi core components revealed functional diversification, as FgAGO1 and FgDCL2 were shown to play important role in hairpin-RNA-induced gene silencing (Chen et al., 2015). In addition, we recently demonstrated that FgAGO2 and FgDCL1 are required for sex-specific RNAi (Gaffar et al., 2019). Moreover, FgAGO2 and FgDCL1 participate in the biogenesis of perithecium-specific microRNAs (Zeng et al., 2018).
Notably, we previously demonstrated that FgDCL1 is required for SIGS-mediated Fg disease resistance (Koch et al., 2016). However, further analysis of Fg RNAi KO mutants revealed that all tested mutants were slightly or strongly compromised in SIGS, whereas FgCYP51 target gene expression was completely abolished in Δdcl2 and Δqip1 mutants (Gaffar et al., 2019).
Together, these studies indicate a central role of RNAi pathways in regulating Fg development, pathogenicity, and immunity. Consistent with this notion, we assume that Fg RNAi components represent suitable targets for RNA spray-mediated disease control. To determine this, we generated different dsRNA constructs targeting FgAGO and FgDCL genes that were sprayed onto barley leaves. We also compared two different dsRNA design strategies; in particular, we used a tool-based prediction of suitable dsRNA construct sequences vs. a manual construct design related to current dsRNA design principles and experiences. The tool-designed dsRNA molecules, which target specific and easily accessible regions are shorter, while the manually-designed dsRNA molecules are longer and target non-overlapping regions.
Primers were designed to generate PCR amplicons of 658–912 bp in length for the manually-designed construct or of 173–193 bp in length for the tool-designed construct (Zhao Bioinformatics Laboratory tool)1, corresponding to exons of selected target genes, in which Fg represents Fusarium graminearum: FgAGO1 (FGSG_08752), FgAGO2 (FGSG_00348), FgDCL1 (FGSG_09025), and FgDCL2 (FGSG_04408) (Supplementary Figures S1–S4). The target gene sequences were amplified from Fg wt strain IFA65 cDNA using target-specific primers (Supplementary Table S1). The length of manually selected sequences were 658 bp for FgAGO1, 871 bp for FgAGO2, 912 bp for FgDCL1, and 870 bp for FgDCL2, while the respective tool-designed sequences were 173, 192, 182, and 193 bp in length, respectively. The respective sequences of tool- and manually-designed constructs did not overlap.
The construction of pGEMT plasmids comprised of the tool- and manually-designed target sequences was performed using restriction enzyme-cloning strategies. The first step in constructing pGEMT plasmids containing manually-designed double targets was to amplify target sequences of AGO1, AGO2, DCL1, and DCL2 from the confirmed plasmids with primers containing restriction sites (Supplementary Table S1). The manually-designed dsRNA targeting FgAGO1 and FgAGO2 had a length of 1,529 bp and was therefore named ago1/ago2_1529nt. According to this scheme the other manually-designed dsRNAs were named ago1/dcl1_1570nt, ago1/dcl2_1528nt, ago2/dcl1_1783nt, ago2/dcl2_1741nt, and dcl1/dcl2_1782nt. Briefly, an AGO2 PCR fragment was inserted between NotI and NdeI restriction sites of pGEMT plasmids containing AGO1 or DCL1 target sequences to generate ago1/ago2_1529nt and ago2/dcl1_1583nt constructs. The PCR fragment of AGO1 was inserted between NotI and NdeI restriction sites of pGEMT plasmids containing the DCL1 target sequence to construct ago1/dcl1_1570nt target plasmid. The other manually designed constructs (ago1/dcl2_1528nt, ago2/dcl2_1741nt and dcl1/dcl2_1782nt) were generated following the same procedure as described above: DCL2 PCR fragments were inserted in the AGO1 background (using NotI and NdeI), in AGO2 (using NotI and BstXI) and in DCL1 (using NotI and SalI). To construct pGEMT plasmids containing tool-designed target sequences (ago1/ago2_365nt, ago1/dcl1_355nt, ago2/dcl1_374nt, ago1/dcl2_366nt), the single targets were amplified using primers containing a restriction site (Supplementary Table S1), as described above. A tool-designed sequence of DCL1 was inserted between NotI and SalI restriction sites of the pGEMT plasmid containing AGO1 and AGO2 targets to generate ago1/dcl1_355nt and ago2/dcl1_374nt constructs, respectively. The DCL2 fragment was inserted between the NotI and SalI restriction sites of the pGEMT plasmid containing the AGO1 sequence to construct ago1/dcl2_366nt. Finally, AGO2 was inserted between the NotI and SalI restriction sites of the pGEMT plasmid containing the AGO1 target sequence to generate an ago1/ago2_365nt construct. As a negative control a previously described dsRNA corresponding to a 720 nt long fragment of the jellyfish green fluorescent protein (GFP) was used (Koch et al., 2016).
MEGAscript Kit High Yield Transcription Kit (Ambion) was used for dsRNA synthesis by following the manufacturers’ instructions using primers containing a T7 promoter sequence at the 5’ end of both forward and reverse primers (Supplementary Table S1).
The second leaves of 2- to 3 week old barley cultivar (cv.) Golden Promise were detached and transferred to square Petri plates containing 1% water-agar. The dsRNA was diluted in 500 μl of water to a final concentration of 20 ng μl–1. For the Tris-EDTA (TE) control, TE buffer was diluted in 500 μl of water, corresponding to the amount used for dilution of the dsRNA. The typical dsRNA concentration after elution was 500 ng μl–1, representing a buffer concentration of 400 μM of Tris-HCL and 40 μM of EDTA in the final dilution. Leaves were sprayed using a spray flask as described earlier (Koch et al., 2016). The upper half of each plate containing ten detached leaves was evenly sprayed (3–4 puffs) with the different tool- and manually-designed dsRNAs or TE buffer and subsequently kept at room temperature. Forty-eight hours after spraying, leaves were drop-inoculated with three 20 μl drops of Fg suspension containing 5 × 104 conidia ml–1 water. After inoculation, plates were closed and incubated for 5 days at room temperature. The relative infection of the leaves was recorded as the infection area (Supplementary Figure S5) (by determining the size of the chlorotic lesions) relative to the total leaf area using ImageJ software (Schneider et al., 2012). We produced four biological replicates for independent sample collection. Each treatment group was compared to the TE-Buffer control using students t-test.
To assess the silencing of the FgAGO and FgDCL genes, mRNA expression analysis was performed using quantitative reverse-transcription PCR (qRT-PCR). RNA extraction from the diseased leaves was performed with TRIzol (Invitrogen) following the manufacturer’s instructions. Freshly extracted mRNA was used for cDNA synthesis using a qScriptTM cDNA kit (Quantabio). For qRT-PCR, 10 ng of cDNA was used as a template with the reactions run in a QuantStudio 5 Real-Time PCR system (Applied Biosystems). Amplifications were performed in 7.5 μl of SYBR® Green JumpStart Taq ReadyMix (Sigma-Aldrich) with 5 pmol of oligonucleotides. Each sample had three technical repetitions. Primers were used for studying expressions of FgAGO and FgDCL genes with reference to the Elongation factor 1-alpha (EF1-a) gene (FGSG_08811) and ß-tubulin (Supplementary Table S1). After an initial activation step at 95°C for 5 min, 40 cycles (95°C for 30 s, 57°C for 30 s, 72°C for 30 s) were performed. Cycle threshold (Ct) values were determined using the 7,500 Fast software supplied with the instrument. Levels of FgAGO and FgDCL transcripts were determined via the 2–ΔΔCt method (Livak and Schmittgen, 2001) by normalizing the amount of target transcript to the amount of the reference transcripts of the EF1-a (translation elongation-factor 1α) and ß-tubulin.
Sequences of the single manually- and tool-designed dsRNA constructs for each gene, FgAGO1, FgAGO2, FgDCL1, and FgDCL2, were split into k-mers of 21 bases and mapped to the coding sequences of the four FgAGO and FgDCL genes. The efficient siRNAs were calculated on the basis of the thermodynamic properties of the siRNA-duplex, the 5’-nucleotide of the guide strand and the target site accessibility based on the default parameters of the SI-FI software tool2. These parameters were: no mismatches to the target sequence, a 5’-A or -U on the potential guide strand, a higher minimum free energy (MFE) on the 5’-end of the guide strand compared to the passenger strand and good target site accessibility; the default parameters were used.
We assessed whether FgAGO and FgDCL genes are suitable targets for SIGS-mediated plant protection strategies. Detached barley leaves were sprayed with 20 ng μl–1 dsRNA and drop-inoculated 48 h later with a suspension of Fg conidia. After 5 dpi, necrotic lesions were visible at the inoculation sites of leaves sprayed with TE buffer or non-homologous GFP-dsRNA as negative controls. All homologous dsRNAs reduced the Fg-induced symptoms, as revealed by significantly smaller lesions in detached barley leaves (Figure 1). Infected areas were reduced on the average by 50% compared to the control (Figure 1). The highest infection reduction of 60% was reached with dsRNAs targeting ago1/ago2_365nt and ago1/dcl1_1570nt (Figure 1). The lowest disease resistance efficiencies of 31% were shown for the ago2/dcl1_1783nt dsRNA construct (Figure 1).
Figure 1. Quantification of infection symptoms of Fg on barley leaves sprayed with AGO/DCL-targeting dsRNAs. Detached leaves of 3 week-old barley plants were sprayed with AGO/DCL-targeting dsRNAs or TE buffer. After 48 h, leaves were drop inoculated with 5 × 104 ml–1 of macroconidia and evaluated for infection symptoms at 5 dpi. Infection area, shown as the percent of the total leaf area for 10 leaves for each dsRNA and the TE control relative to the infected leaf area. Bars represent mean values ± SDs of three independent experiments. Asterisks indicate statistical significance (*p < 0.05, **p< 0.01, ***p< 0.001, students t-test).
DCL-dsRNAs Exhibited Higher Target Gene Silencing Than AGO-dsRNAs
To analyze whether the observed resistance phenotypes were provoked by target gene silencing, we measured the transcript levels of FgAGO and FgDCL genes of Fg grown in the infected leaf tissue by qRT-PCR. As anticipated, the relative transcript levels of targeted genes FgAGO1, FgAGO2, FgDCL1, and FgDCL2 were reduced after the inoculation of leaves sprayed with the respective dsRNA constructs (Figures 2A,B), except for FgAGO1, if targeted with tool-designed constructs ago1/dcl1_355nt, ago1/dcl2_366nt, and ago1/ago2_365nt (Figure 2A). However, regarding those three constructs, we detected silencing effects for the second target gene, as the FgDCL1 expression was reduced by 47%, FgDCL2 by 44%, and FgAGO2 by 52% (Figure 2A). The most efficient construct in terms of overall target gene silencing was ago2/dcl1_374nt, which reduced the transcripts of FgAGO2 and FgDCL1 by 40 and 74%, respectively, compared to the TE control (Figures 2A,B).
Figure 2. Relative expression of the respective fungal DCLs and AGOs 5 dpi on (A) tool- and (B) manually-designed-dsRNA-sprayed leaves. The expression was measured via the 2–ΔΔCt method in which the expression of the respective AGOs and DCLs was normalized against the fungal reference genes EF1α (translation elongation-factor 1 α) and ß-tubulin, and this Δ-Ct value was then normalized against the Δ-Ct of the GFP control. Error bars represent the SE of the four independent experiments, each using 10 leaves of 10 different plants for each transgenic line. Asterisks indicate statistical significance (*p < 0.05, **p< 0.01, ***p < 0.001, students t-test).
Notably, if we compared the results for the tool-designed dsRNA constructs with the manually-designed dsRNAs we observed similar results for the FgAGO1 target-silencing (Figures 2A,B). The constructs ago1/dcl1_1570nt and ago1/dcl2_1528nt reduced FgAGO1 transcripts by only 17 and 29%, respectively (Figure 2B). Analyzing the transcript levels of FgAGO2 revealed that: (a) the silencing efficiencies of ago2/dcl1_1783nt and ago2/dcl2_1741nt were higher than FgAGO1 target silencing and (b) targeting both FgAGO genes with the ago1/ago2_1529nt construct resulted in 50% reduction for FgAGO1 and 62% for FgAGO2. This, therefore, showed the highest overall FgAGOs gene silencing (Figure 2B).
Interestingly and consistent with the tool-designed target gene silencing results, we detected the strongest reduction of >70% for FgDCL1 (Figure 2B). For example, ago2/dcl1_1783nt-dsRNA provoked a 79% reduction of FgDCL1 transcripts. Target gene silencing for FgDCL2 was also highly efficient, as use of all three constructs, ago1/dcl2_1528nt, ago2/dcl2_1741nt and dcl1/dcl2_1782nt, resulted in an Ħ60% silencing efficiency (Figure 2B). The most efficient construct in terms of overall target gene silencing was dcl1/dcl2_1782nt, which reduced the transcripts of FgDCL1 and FgDCL2 by 78 and 58%, respectively, compared to control. Overall, these results suggest that silencing conferred by AGO- and DCL-dsRNAs exhibited the highest efficiency for silencing of FgDCL1 (AVE: 70%), followed by FgDCL2 (AVE: 58%), FgAGO2 (AVE: 48%) and FgAGO1 (AVE: 26%) (Table 1).
Table 1. Overview of target gene-silencing efficiencies of different tested AGO- and DCL-dsRNA constructs.
To assess whether tool-designed dsRNA is more efficient than manually designed constructs, we directly compared target gene-silencing efficiencies of both design approaches (Figure 3). We observed that target gene silencing of manually-designed constructs was superior to tool-designed dsRNA (Figure 3), except for FgAGO2, for which we found no differences between tool- or manually-designed dsRNA. Based on these findings and considering previous results, we anticipated that larger dsRNA constructs resulted in higher numbers of efficient siRNAs (Höfle et al., 2019; Koch et al., 2019). As the tool-designed constructs were <200 nt in length compared to >650 nt for the manually-designed dsRNA (Table 2 and Supplementary Figures S1–S4), we calculated bioinformatically the possible siRNA hits in the FgAGO and FgDCL target genes for all tested dsRNA constructs (Table 2).
Figure 3. Direct comparison of long (manual) and short (tool) constructs. Relative expression of the respective fungal DCLs and AGOs 5 dpi on dsRNA-sprayed leaves is grouped by the target gene. The expression was measured via the ΔΔ-Ct method in which the expression of the respective AGOs and DCLs was normalized against the fungal reference gene EF1α (translation elongation-factor 1 α) and β-tubulin, and this Δ-Ct value was then normalized against the Δ-Ct of the GFP control. The asterisks indicate a significant expression of the sprayed leaves in comparison to the mock-treated TE controls. Bars represent mean values ± SE of the four independent experiments.
For the manually-designed dsRNA, which target different regions of the respective genes, we calculated siRNAs that were 4- to 10-fold more efficient compared to the tool-designed constructs (Table 2), confirming that the dsRNA precursor length probably plays a role in determining the number of derived siRNAs. For example, we predicted 49 efficient siRNAs deriving from the 912 nt manually-designed dsRNA, which targets FgDCL1, which is 10-fold >5 siRNA hits derived from the 182 nt tool-designed FgDCL1-dsRNA (Table 2). Notably, these differences resulted in only an overall 10% silencing efficiency decrease of the tool-designed dsRNA compared to the manually-designed constructs targeting FgDCL1 (Table 2). Together, these data suggest that longer dsRNAs result in a higher number of efficient siRNAs, but there is no stringent correlation between the number of efficient siRNAs and the increase in target gene silencing (Table 2).
Microbial pathogens and pests, unlike mammals, are amenable to environmental sRNAs, meaning that they can take up non-coding RNAs from the environment, and these RNAs maintain their RNAi activity (Winston et al., 2007; Whangbo and Hunter, 2008; McEwan et al., 2012). This knowledge raises the possibility that plants can be protected from pathogens/pests by exogenously supplied RNA biopesticides (for review, see Mitter et al., 2017b; Cai et al., 2018b; Dubrovina and Kiselev, 2019; Gaffar and Koch, 2019; Dalakouras et al., 2020). Possible agronomic application of environmental RNA is affirmed by the high sensitivity of Fg to dsRNAs and siRNAs (Koch et al., 2016). Here, we demonstrated that targeting, via SIGS, key components of the Fg RNAi machinery, such as AGO and DCL genes, could protect barley leaves from Fg infection. Our findings, together with other reports, underline that Fg RNAi pathways play a crucial role in regulating fungal development, growth, reproduction, mycotoxin production and pathogenicity (Kim et al., 2015; Son et al., 2017; Gaffar et al., 2019). However, the mechanistic role of Fg RNAi components in these processes are inadequately understood. Nevertheless, existing data suggest that there is a functional diversification of FgAGO1/FgDCL2- and FgAGO2/FgDCL1-regulated pathways (Chen et al., 2015; Son et al., 2017; Zeng et al., 2018; Gaffar et al., 2019).
Based on these findings, the dsRNAs tested in this study were designed to target FgAGO and FgDCL genes pairwise. Thus, we generated six different dsRNA constructs covering all possible AGO-DCL combinations (Figure 4). Spraying the different dsRNAs onto barley leaves resulted in ∼50% inhibition of fungal infection for all constructs (Figure 1). By analyzing the silencing efficiencies of the different dsRNA constructs, we found that the expression of FgDCLs genes was more suppressed than FgAGOs genes (Table 1). More importantly, the expression of FgAGO1 was completely unaffected, regardless of which dsRNA was sprayed. Based on this result, we could speculate that FgAGO1 is required for binding of SIGS-associated siRNAs; thus, loss of function mediated by SIGS will not work. Of note, ΔAGO1 mutants of Fg were only slightly compromised in SIGS and less sensitive to dsRNA treatments, indicating redundant functions of FgAGO1 and FgAGO2 in the binding of SIGS-derived siRNAs (Gaffar et al., 2019). However, further studies must explore the mechanistic role of FgAGO1 in SIGS.
While our data showed that SIGS-mediated downregulation of FgDCLs gene expression resulted in inhibition of Fg infection, we cannot exclude the possibility of sprayed dsRNAs being processed by plant DCLs, which would explain the effective silencing even with silenced fungal DCLs. Consistent with this finding, previous studies demonstrated that spraying of siRNAs led to the induction of local and systemic RNAi in plants (e.g., Dalakouras et al., 2016; Koch et al., 2016). These findings are significant contributions to our mechanistic understanding of RNAi spray technology, as our previous data indicate that effective SIGS requires the processing of dsRNAs by the fungal RNAi machinery (Koch et al., 2016; Gaffar et al., 2019). Whereas HIGS mainly relies on the host plant’s ability to produce mobile siRNAs (generated from transgene-derived dsRNAs), the mechanism of gene silencing by exogenously delivered dsRNA constitutes a more complex situation; for instance, the possible involvement of the silencing machinery of the host and/or pathogen (Figure 5). Our previous finding that unprocessed long dsRNA is absorbed from leaf tissue (Koch et al., 2016) has important implications for future disease control strategies based on dsRNA. It is very likely that the application of longer dsRNAs might be more efficient than the application of siRNAs, given their dsRNAs more efficient translocation (Koch et al., 2016). Moreover, in contrast to using only one specific siRNA, processing of long dsRNA into many different inhibitory siRNAs by the fungus may reduce the chance of pathogen resistance under field test conditions. However, RNAi-based plant protection technologies are limited by the uptake of RNAi-inducing trigger molecules, either siRNAs and/or dsRNAs; both RNA types have been shown to confer plant disease resistance independent of how they were applied/delivered (i.e., endogenously or exogenously).
Figure 4. Representation of dsRNAs and the complementary region in the corresponding genes. (A) Graphic representation of all four targeted mRNAs and their respective accessions with target regions marked in colors. Manually selected regions are marked in dark colors and regions selected by the pssRNAit tool1 for better target accessibility are marked with light colors. All (B) manually and (C) tool designed dsRNAs triggers are shown. RNAs are represented correctly scaled to each other.
Figure 5. The molecular mechanism of SIGS is controlled by the fungal silencing machinery. In summary, our findings support the model that SIGS involves: (1) uptake of sprayed dsRNA by the plant (via stomata); (2) transfer of apoplastic dsRNAs into the symplast (DCL processing into siRNAs); (3) systemic translocation of siRNA or unprocessed dsRNA via the vascular system (phloem/xylem); (4) uptake of apoplastic dsRNA (a) or symplastic dsRNA/siRNA by the fungus (b) or siRNAs from multivesicular body (MVBs) derived extracellular vesicles (EVs) (c); (5) processing into siRNA by fungal DCL.
Previously, we discovered that longer dsRNAs of 400–800 nt exhibited a higher gene-silencing efficiency and a stronger disease resistance than 200 nt dsRNAs (Koch et al., 2019) indicating that the quantity of siRNAs derived from a longer dsRNA precursor is simply higher. To test whether the length and/or the selected target gene sequence influences silencing efficiencies, we constructed 10 different dsRNA constructs targeting FgAGO/FgDCL pairs (Figure 4). For the design of the dsRNA constructs we used a dsRNA design tool3 that generates dsRNAs of shorter lengths (173–197 nt), compared them to manually selected sequences (658–912 nt) and calculated the number of efficient siRNAs for each construct using si-Fi 2.14 in silico prediction tool (Table 2). These differences in length are inherent in the design methods and represent therefore the different design approaches. While the tool-designed RNA-trigger are designed to target a specific and well accessible region of the target mRNA the manual design approach pays little attention to these factors and is based on a more or less random selection of regions. Notably, we found that the number of efficient siRNAs derived from the longer, manually-designed dsRNAs was 4- to 5-fold higher for the constructs that target FgAGO1 and FgAGO2. Moreover, the manually-designed constructs targeting FgDCL1 and FgDCL2 resulted in 10-fold more efficient siRNAs than the tool-designed versions (Table 2). However, such a correlation was only observed when we compared tool- vs. manually-designed dsRNA (<200 vs. >650 nt constructs). If we attempt to predict the number of efficient siRNAs of all the manually-designed dsRNAs, based on the length of their precursors, we obtained contrasting results. For example, the 912 nt precursor dsRNA that targets FgDCL1 resulted in 49 efficient siRNA hits, which is approximately half of the 92 siRNA hits for the 870-nt dsRNA designed to target FgDCL2 (Table 2). Importantly, the tested dsRNAs that target FgDCL1, which showed the lowest number of siRNAs, revealed the highest efficiencies compared to all other constructs (Table 2). Together, our data support the notion that longer dsRNAs tend to result in higher numbers of siRNA, although this can differ in particular cases. However, these data were obtained from in silico predictions; therefore, their accuracies remain unknown. Small RNA-sequencing must be performed to quantify, analyze and map the SIGS-derived siRNAs to their target genes as well as their dsRNA precursors. Besides siRNA concentration, the siRNA sequence represents a crucial determinant affecting silencing efficiency of its complementary target genes (Ossowski et al., 2008). In addition, mapping of siRNAs to their target sequence revealed processing patterns that might help to define principles for RNAi trigger design, producing effective siRNAs (Yang et al., 2013; Koch et al., 2016; Baldwin et al., 2018). Importantly, to construct our manually-designed dsRNAs, we performed a random selection of sequences complementary to the specific target genes. Moreover, to guarantee optimal silencing, we chose longer dsRNA sequences compared to the tool-designed dsRNAs. Thus, a random selection of longer target sequences, which are more effective in target silencing, tends to increase off-target effects per se, due to the increase in the number of different potential siRNAs (Roberts et al., 2015). Shorter target sequences, which are also specifically selected to produce potential siRNAs with a minimal potential to silence unintended targets, could greatly reduce these off-target effects. Therefore, based on our results obtained with the tool-designed dsRNAs and the work of others, we suggest using minimal-length dsRNA sequences carefully selected based on known design criteria requirements. Another possible way to achieve high silencing efficiencies while retaining high target specificity (less off-target effect) could be the construction of dsRNAs repeating a shorter tool- designed sequence several times.
Nevertheless, the number of efficient siRNAs that reach the fungus depends on the uptake efficiency of sprayed dsRNA molecules and that can differ depending on the parameters which determine the uptake efficiency, such as the stomata opening (Koch et al., 2016). Additionally, as we previously found in SIGS, the concentration of siRNAs in the target organism (i.e., fungus) can vary and mainly rely on the uptake of unprocessed dsRNA from the plant’s apoplast and their processing by fungal DCLs (Koch et al., 2016; Gaffar et al., 2019). Finally, and even more important than quantities of target-specific siRNAs in determining silencing efficacy, is the target accessibility of a siRNA (Reynolds et al., 2004; Shao et al., 2007). Therefore, the design of RNAi triggers that likely mediate the efficient uptake of dsRNAs and/or siRNAs by the target pathogen is crucial in the success of SIGS as well as HIGS technologies.
Together, our results indicate that silencing fungal RNAi pathway genes, especially DCL genes, using SIGS efficiently increases plant disease resistance toward necrotrophic fungal pathogens, such as Fg. Moreover, our results support the notion that fungal RNAi-related genes in Fg play an essential, direct or indirect role in pathogenicity and/or virulence (Gaffar et al., 2019). These findings are consistent with other reports demonstrating that the two DCL proteins (DCL1 and DCL2) of the necrotrophic fungal pathogen Botrytis cinerea (Bc) play a central role in disease development (Wang et al., 2016). These authors showed that the application of sRNAs or dsRNAs on fruits, vegetables and flowers targeting BcDCL1 and BcDCL2 genes significantly inhibited gray mold disease. Of note, the same group previously discovered that Bc delivers sRNAs into plant cells to silence host immunity genes, a phenomenon called ‘cross-kingdom RNAi (ckRNAi)’ (Weiberg et al., 2013). Emerging data further suggest that some sRNA effectors can target multiple host defense genes to enhance Bc pathogenicity. For example, Bc-siR37 suppresses host immunity by targeting at least 15 Arabidopsis genes, including WRKY transcription factors, receptor-like kinases and cell wall-modifying enzymes (Wang B. et al., 2017). Moreover, one of the most destructive biotrophic pathogens of wheat Puccinia striiformis also delivers fungal sRNAs, such as microRNA-like RNA1 (milR1), into host cells and suppresses wheat Pathogenesis-related 2 (PR-2) in the defense pathway (Wang M. et al., 2017). Notably, such ckRNAi-related sRNA effectors are produced by fungal DCL proteins, and thus SIGS of fungal DCLs abolishes sRNA production and attenuates fungal pathogenicity and growth. However, whether our findings suggest that Fg utilizes ckRNAi-related sRNAs to suppress host immunity needs further exploration.
More importantly, while several studies have demonstrated bidirectional ckRNAi and sRNA trafficking between plant hosts and their interacting fungal pathogens (Zhang et al., 2012, 2016; Weiberg et al., 2015; Wang B. et al., 2017; Wang M. et al., 2017; Zhu et al., 2017; Cai et al., 2018a; Dubey et al., 2019; Zanini et al., 2019) the mechanisms underlying the transfer and uptake of transgene-derived artificial sRNAs (HIGS) as well as exogenously applied dsRNA (SIGS) remain elusive. Further research is needed to determine, for example: (a) how plant and fungal-silencing machinery contributes to HIGS and SIGS; (b) the nature of the inhibitory RNA that translocates from the plant to the fungus after its transgenic expression or spray application; (c) how that RNA crosses the plant-fungal interface; and (d) how dsRNA is transported at the apoplast-symplast interface. Therefore, addressing these questions is key for making RNAi-based strategies a realistic and sustainable approach in agriculture.
All datasets generated for this study are included in the article/Supplementary Material.
AK designed the study and wrote the manuscript. BW, FG, DB, and JS conducted the experiments. AK and BW analyzed all the data and drafted the figures. JS and DB conducted the RNA spray experiments. BW performed the bioinformatics analysis. All authors reviewed the final manuscript.
This work was supported by the Deutsche Forschungsgemein- schaft, Research Training Group (RTG) 2355 (project number 325443116) to AK.
The authors declare that the research was conducted in the absence of any commercial or financial relationships that could be construed as a potential conflict of interest.
This work was supported by Deutsche Forschungsgemeinschaft to AK (RTG:2355) and Deutscher Akademischer Austauschdienst to FG.
The Supplementary Material for this article can be found online at: https://www.frontiersin.org/articles/10.3389/fpls.2020.00476/full#supplementary-material
FIGURES S1–S4 | Coding sequences (CDS) of the respective Fg target gene with the sequences of the dsRNA marked (blue, tool-designed; red, manually designed).
FIGURE S5 | Representative pictures of barley (golden promise) leaves sprayed with 10 μg (20 ng/μl) of respective dsRNA in TE-Buffer and the control without dsRNA. DsRNA was applied on the upper half of 10 leaves and 2 days after spraying the leaves were inoculated with three 20 μl droplets of Fg (50,000 spores/ml). The pictures were taken 5 dpi.
TABLE S1 | Primers used in this study.
Baldwin, T., Islamovic, E., Klos, K., Schwartz, P., Gillespie, J., Hunter, S., et al. (2018). Silencing efficiency of dsRNA fragments targeting Fusarium graminearum TRI6 and patterns of small interfering RNA associated with reduced virulence and mycotoxin production. PLoS One 13:e0202798. doi: 10.1371/journal.pone.0202798
Bharti, P., Jyoti, P., Kapoor, P., Sharma, V., Shanmugam, V., and Yadav, S. K. (2017). Host-induced silencing of pathogenicity genes enhances resistance to Fusarium oxysporum wilt in tomato. Mol. Biotechnol. 59, 343–352. doi: 10.1007/s12033-017-0022-y
Borges, F., and Martienssen, R. A. (2015). The expanding world of small RNAs in plants. Nat. Rev. Mol. Cell Biol. 16, 727–741. doi: 10.1038/nrm4085
Cai, Q., He, B., Kogel, K. H., and Jin, H. (2018a). Cross-kingdom RNA trafficking and environmental RNAi nature’s blueprint for modern crop protection strategies. Curr. Opin. Microbiol. 46, 58–64. doi: 10.1016/j.mib.2018.02.003
Cai, Q., Qiaom, L., Wang, M., He, B., Lin, F. M., Palmquist, J., et al. (2018b). Plants send small RNAs in extracellular vesicles to fungal pathogen to silence virulence genes. Science 360,, 1126–1129. doi: 10.1126/science.aar4142
Chen, W., Kastner, C., Nowara, D., Oliveira-Garcia, E., Rutten, T., Zhao, Y., et al. (2016). Host-induced silencing of Fusarium culmorum genes protects wheat from infection. J. Exp. Bot. 67, 4979–4991. doi: 10.1093/jxb/erw263
Chen, Y., Gao, Q., Huang, M., Liu, Y., Liu, Z., Liu, X., et al. (2015). Characterization of RNA silencing components in the plant pathogenic fungus Fusarium graminearum. Sci. Rep. 5:12500. doi: 10.1038/srep12500
Cheng, W., Song, X. S., Li, H. P., Cao, L. H., Sun, K., Qiu, X. L., et al. (2015). Host-induced gene silencing of an essential chitin synthase gene confers durable resistance to Fusarium head blight and seedling blight in wheat. Plant Biotechnol. J. 13, 1335–1345. doi: 10.1111/pbi.12352
Dalakouras, A., Wassenegger, M., Dadami, E., Ganopoulos, I., Pappas, M. L., and Papadopoulou, K. (2020). Genetically modified organism-free RNA interference: exogenous. Plant Physiol. 182,, 38–50.
Dalakouras, A., Wassenegger, M., McMillan, J. N., Cardoza, V., Maegele, I., and Dadami, E. (2016). Induction of silencing in plants by high-pressure spraying of in vitro-synthesized small RNAs. Front. Plant Sci. 7:1327. doi: 10.3389/fpls.2016.01327
Dubey, H., Kiran, K., Jaswal, R., Jain, P., Kayastha, A. M., and Bhardwaj, S. C. (2019). Discovery and profiling of small RNAs from Puccinia triticina by deep sequencing and identification of their potential targets in wheat. Funct. Integr. Genomics 19,, 391–407.
Dubrovina, A. S., and Kiselev, K. V. (2019). Exogenous RNAs for gene regulation and plant resistance. Int. J. Mol. Sci. 20:2282. doi: 10.3390/ijms20092282
Gaffar, F. Y., Imani, J., Karlovsky, J. P., Koch, A., and Kogel, K. H. (2019). Various components of the RNAi pathway are required for conidiation, ascosporogenesis, virulence, DON production and SIGS-mediated fungal inhibition by exogenous dsRNA in the Head Blight pathogen Fusarium graminearum. Front. Microbiol. 10:1662. doi: 10.3389/fmicb.2019.01662
Gaffar, F. Y., and Koch, A. (2019). Catch me if you can! RNA silencing-based improvement of antiviral plant immunity. Viruses 11:673.
Ghag, S. B., Shekhawat, U. K., and Ganapathi, T. R. (2014). Host-induced post-transcriptional hairpin RNA-mediated gene silencing of vital fungal genes confers efficient resistance against Fusarium wilt in banana. Plant Biotechnol. J. 12, 541–553. doi: 10.1111/pbi.12158
Goswami, R. S., and Kistler, H. C. (2004). Heading for disaster: Fusarium graminearum on cereal crops. Mol. Plant Pathol. 5, 515–525. doi: 10.1111/j.1364-3703.2004.00252.x
Guo, Q., Liu, Q., Smith, N. A., Liang, G., and Wang, M. B. (2016). RNA silencing in plants: mechanisms, technologies and applications in horticultural crops. Curr. Genomics 17, 476–489. doi: 10.2174/1389202917666160520103117
Höfle, L., Shrestha, A., Jelonek, L., and Koch, A. (2019). Study on the efficiency of dsRNAs with increasing length in RNA-based silencing of the Fusarium CYP51 genes. RNA Biol. 17,, 463–473.
Hu, Z., Parekh, U., Maruta, N., Trusov, Y., and Botella, J. R. (2015). Down-regulation of Fusarium oxysporum endogenous genes by host-delivered RNA interference enhances disease resistance. Front. Chem. 3:1. doi: 10.3389/fchem.2015.00001
Ismaiel, A., and Papenbrock, J. (2015). Mycotoxins: producing fungi and mechanisms of phytotoxicity. Agriculture 5, 492–537. doi: 10.3390/agriculture5030492
Kaldis, A., Berbati, M., Melita, O., Reppa, C., Holeva, M., Otten, P., et al. (2018). Exogenously applied dsRNA molecules deriving from the Zucchini yellow mosaic virus (ZYMV) genome move systemically and protect cucurbits against ZYMV. Mol. Plant Pathol. 19,, 883–895. doi: 10.1111/mpp.12572
Kazan, K., Gardiner, D. M., and Manners, J. M. (2012). On the trail of a cereal killer: recent advances in Fusarium graminearum pathogenomics and host resistance. Mol. Plant Pathol. 13, 399–413. doi: 10.1111/j.1364-3703.2011.00762.x
Ketting, R. F. (2011). The many faces of RNAi. Dev. Cell 20, 148–161. doi: 10.1016/j.devcel.2011.01.012
Kim, H. K., Jo, S. M., Kim, G. Y., Kim, D. W., Kim, Y. K., and Yun, S. H. (2015). A large-scale functional analysis of putative target genes of mating-type loci provides insight into the regulation of sexual development of the cereal pathogen Fusarium graminearum. PLoS Genet. 11:e1005486. doi: 10.1371/journal.pgen.1005486
Koch, A., Biedenkopf, B., Furch, A. C. U., Abdellatef, E., Weber, L., Linicus, L., et al. (2016). An RNAi-based control of Fusarium graminearum infections through spraying of long dsRNAs involves a plant passage and is controlled by the fungal silencing machinery. PLoS Pathog. 12:e1005901.
Koch, A., and Kogel, K.-H. (2014). New wind in the sails: improving the agronomic value of crop plants through RNAi-mediated gene silencing. Plant Biotechnol. J. 12,, 821–831. doi: 10.1111/pbi.12226
Koch, A., Kumar, N., Weber, L., Keller, H., Imani, J., and Kogel, K. H. (2013). Host-induced gene silencing of cytochrome P450 lanosterol C14α-demethylase-encoding genes confers strong resistance to Fusarium spec. Proc. Natl. Acad. Sci. U.S.A. 110,, 19324–19329.
Koch, A., Stein, E., and Kogel, K. H. (2018). RNA-based disease control as a complementary measure to fight Fusarium fungi through silencing of the azole target cytochrome P450 lanosterol C-14 α-demethylase. Eur. J. Plant Pathol. 152, 1003–1010. doi: 10.1007/s10658-018-1518-4
Koch, A., Höfle, L., Werner, B. T., Imani, J., Schmidt, A., Jelonek, L., et al. (2019). SIGS vs HIGS: a study on the efficacy of two dsRNA delivery strategies to silence Fusarium FgCYP51 genes in infected host and non-host plants. Mol. Plant Pathol. 20, 1636–1644. doi: 10.1111/mpp.12866
Konakalla, N. C., Kaldis, A., Berbati, M., Masarapu, H., and Voloudakis, A. E. (2016). Exogenous application of double-stranded RNA molecules from TMV p126 and CP genes confers resistance against TMV in tobacco. Planta 244,, 961–969.
Kuck, K. H., Stenzel, K., and Vors, J. P. (2012). Sterol biosynthesis inhibitors. Mod. Crop Prot. Compd. 1, 761–805.
Livak, K. J., and Schmittgen, T. D. (2001). Analysis of relative gene expression data using real-time quantitative PCR and the 2- ΔΔCT method. Methods 25, 402–408. doi: 10.1006/meth.2001.1262
Machado, A. K., Brown, N. A., Urban, M., Kanyuka, K., and Hammond-Kosack, K. E. (2018). RNAi as an emerging approach to control Fusarium head blight disease and mycotoxin contamination in cereals. Pest Manag. Sci. 74, 790–799. doi: 10.1002/ps.4748
Majumdar, R., Rajasekaran, K., and Cary, J. W. (2017). RNA interference (RNAi) as a potential tool for control of mycotoxin contamination in crop plants: concepts and considerations. Front. Plant Sci. 8:200. doi: 10.3389/fpls.2017.00200
McEwan, D. L., Weisman, A. S., and Hunter, C. P. (2012). Uptake of extracellular double-stranded RNA by SID-2. Mol. Cell 47, 746–754. doi: 10.1016/j.molcel.2012.07.014
McMullen, M., Bergstrom, G., De Wolf, E., Dill-Macky, R., Hershman, D., Shaner, G., et al. (2012). A unified effort to fight an enemy of wheat and barley: Fusarium head blight. Plant Dis. 96, 1712–1728. doi: 10.1094/PDIS-03-12-0291-FE
Mitter, N., Worrall, E. A., Robinson, K. E., Li, P., Jain, R. G., Taochy, C., et al. (2017a). Clay nanosheets for topical delivery of RNAi for sustained protection against plant viruses. Nat. Plants 3:16207. doi: 10.1038/nplants.2016.207
Mitter, N., Worrall, E. A., Robinson, K. E., Xu, Z. P., and Carroll, B. J. (2017b). Induction of virus resistance by exogenous application of double-stranded RNA. Curr. Opin. Virol. 26, 49–55. doi: 10.1016/j.coviro.2017.07.009
Nowara, D., Gay, A., Lacomme, C., Shaw, J., Ridout, C., Douchkov, D., et al. (2010). HIGS: host-induced gene silencing in the obligate biotrophic fungal pathogen Blumeria graminis. Plant Cell 22,, 3130–3141. doi: 10.1105/tpc.110.077040
Ossowski, S., Schwab, R., and Weigel, D. (2008). Gene silencing in plants using artificial microRNAs and other small RNAs. Plant J. 53, 674–690. doi: 10.1111/j.1365-313x.2007.03328.x
Pareek, M., and Rajam, M. V. (2017). RNAi-mediated silencing of MAP kinase signalling genes (Fmk1, Hog1, and Pbs2) in Fusarium oxysporum reduces pathogenesis on tomato plants. Fungal Biol. 121, 775–784. doi: 10.1016/j.funbio.2017.05.005
Qi, T., Guo, J., Peng, H., Liu, P., Kang, Z., and Guo, J. (2019). Host-induced gene silencing: a powerful strategy to control diseases of wheat and barley. Int. J. Mol. Sci. 20:206.
Reynolds, A., Leake, D., Boese, Q., Scaringe, S., Marshall, W. S., and Khvorova, A. (2004). Rational siRNA design for RNA interference. Nat. Biotechnol. 22, 326–330. doi: 10.1038/nbt936
Roberts, A. F., Devos, Y., Lemgo, G. N., and Zhou, X. (2015). Biosafety research for non-target organism risk assessment of RNAi-based GE plants. Front. Plant Sci. 6:958. doi: 10.3389/fpls.2015.00958
Schneider, C. A., Rasband, W. S., and Eliceiri, K. W. (2012). NIH image to ImageJ: 25 years of image analysis. Nat. Methods 9, 671–675. doi: 10.1038/nmeth.2089
Shao, Y., Chan, C. Y., Maliyekkel, A., Lawrence, C. E., Roninson, I. B., and Ding, Y. (2007). Effect of target secondary structure on RNAi efficiency. RNA 13, 1631–1640. doi: 10.1261/rna.546207
Son, H., Park, A. R., Lim, J. Y., Shin, C., and Lee, Y. W. (2017). Genome-wide exonic small interference RNA-mediated gene silencing regulates sexual reproduction in the homothallic fungus Fusarium graminearum. PLoS Genet. 13:e1006595. doi: 10.1371/journal.pgen.1006595
Spolti, P., Del Ponte, E. M., Dong, Y., Cummings, J. A., and Bergstrom, G. C. (2014). Triazole sensitivity in a contemporary population of Fusarium graminearum from New York wheat and competitiveness of a tebuconazole-resistant isolate. Plant Dis. 98, 607–613. doi: 10.1094/PDIS-10-13-1051-RE
Vaucheret, H., Vazquez, F., Crété, P., and Bartel, D. P. (2004). The action of ARGONAUTE1 in the miRNA pathway and its regulation by the miRNA pathway are crucial for plant development. Genes Dev. 18, 1187–1197. doi: 10.1101/gad.1201404
Wang, B., Sun, Y. F., Song, N., Zhao, M. X., Liu, R., Feng, H., et al. (2017). Puccinia striiformis f. sp tritici microRNA-like RNA 1 (Pst-milR1), an important pathogenicity factor of Pst, impairs wheat resistance to Pst by suppressing the wheat pathogenesis-related 2 gene. New Phytol. 215,, 338–350. doi: 10.1111/nph.14577
Wang, M., Weiberg, A., Dellota, E. Jr., Yamane, D., and Jin, H. (2017). Botrytis small RNA Bc-siR37 suppresses plant defense genes by cross-kingdom RNAi. RNA Biol. 14,, 421–428. doi: 10.1080/15476286.2017
Wang, M., Weiberg, A., Lin, F.-M., Thomma, B. P. H. J., Huang, H. D., and Jin, H. (2016). Bidirectional cross-kingdom RNAi and fungal uptake of external RNAs confer plant protection. Nat. Plants 2:16151. doi: 10.1038/nplants.2016.151
Weiberg, A., Bellinger, M., and Jin, H. L. (2015). Conversations between kingdoms: small RNAs. Curr. Opin. Biotechnol. 32,, 207–215. doi: 10.1016/j.copbio.2014
Weiberg, A., Wang, M., Lin, F. M., Zhao, H., Zhang, Z., Kaloshian, I., et al. (2013). Fungal small RNAs suppress plant immunity by hijacking host RNA interference pathways. Science 342,, 118–123. doi: 10.1126/science.1239705
Whangbo, J. S., and Hunter, C. P. (2008). Environmental RNA interference. Trends Genet. 24, 297–305. doi: 10.1016/j.tig.2008.03.007
Winston, W. M., Sutherlin, M., Wright, A. J., Feinberg, E. H., and Hunter, C. P. (2007). Caenorhabditis elegans SID-2 is required for environmental RNA interference. Proc. Natl. Acad. Sci. U.S.A. 104, 10565–10570. doi: 10.1073/pnas.0611282104
Yang, Y., Jittayasothorn, Y., Chronis, D., Wang, X., Cousins, P., and Zhong, G. Y. (2013). Molecular characteristics and efficacy of 16D10 siRNAs in inhibiting root-knot nematode infection in transgenic grape hairy roots. PLoS One 8:e69463. doi: 10.1371/journal.pone.0069463
Yin, C., and Hulbert, S. (2015). Host induced gene silencing (HIGS), a promising strategy for developing disease resistant crops. Gene Technol. 4:130. doi: 10.4172/2329-6682.1000130
Yin, Y., Liu, X., Li, B., and Ma, Z. (2009). Characterization of sterol demethylation inhibitor-resistant isolates of Fusarium asiaticum and F. graminearum collected from wheat in China. Phytopathology 99, 487–497. doi: 10.1094/PHYTO-99-5-0487
Yu, J., Lee, K. M., Cho, W. K., Park, J. Y., and Kim, K. H. (2018). Differential contribution of RNA interference components in response to distinct Fusarium graminearum virus infections. J. Virol. 92:e01756-17. doi: 10.1128/JVI.01756-17
Zanini, S., Šeèiæ, E., Busche, T., Kalinowski, J., and Kogel, K. H. (2019). Discovery of interaction-related sRNAs and their targets in the Brachypodium distachyon and Magnaporthe oryzae pathosystem. BioRxiv [Preprint]
Zeng, W., Wang, J., Wang, Y., Lin, J., Fu, Y., Xie, J., et al. (2018). Dicer-like proteins regulate sexual development via the biogenesis of perithecium-specific microRNAs in a plant pathogenic fungus Fusarium graminearum. Front. Microbiol. 9:818. doi: 10.3389/fmicb.2018.00818
Zhang, J., Khan, S. A., Heckel, D. G., and Bock, R. (2017). Next-generation insect-resistant plants: RNAi-mediated crop protection. Trends Biotechnol. 35,, 871–882.
Zhang, L., Hu, D., Chen, X., Li, D., Zhu, L., and Zhang, Y. (2012). Exogenous plant MIR168a specifically targets mammalian LDLRAP1: evidence of cross-kingdom regulation by microRNA. Cell Res. 22,, 107–126. doi: 10.1038/cr.2011.158
Zhang, T., Zhao, Y. L., Zhao, J. H., Wang, S., Jin, Y., Chen, Z. Q., et al. (2016). Cotton plants export microRNAs to inhibit virulence gene expression in a fungal pathogen Nat. Plants 2:16153. doi: 10.1038/nplants.2016.153
Keywords: RNA spraying, RNA silencing, spray-induced gene silencing, Fusarium graminearium, AGO and DCL
Citation: Werner BT, Gaffar FY, Schuemann J, Biedenkopf D and Koch AM (2020) RNA-Spray-Mediated Silencing of Fusarium graminearum AGO and DCL Genes Improve Barley Disease Resistance. Front. Plant Sci. 11:476. doi: 10.3389/fpls.2020.00476
Received: 09 July 2019; Accepted: 30 March 2020;
Published: 29 April 2020.
Edited by:
Azeddine Si Ammour, Fondazione Edmund Mach, ItalyReviewed by:
Andrew Leigh Eamens, University of Newcastle, AustraliaCopyright © 2020 Werner, Gaffar, Schuemann, Biedenkopf and Koch. This is an open-access article distributed under the terms of the Creative Commons Attribution License (CC BY). The use, distribution or reproduction in other forums is permitted, provided the original author(s) and the copyright owner(s) are credited and that the original publication in this journal is cited, in accordance with accepted academic practice. No use, distribution or reproduction is permitted which does not comply with these terms.
*Correspondence: Aline Michaela Koch, YWxpbmUua29jaEBhZ3Jhci51bmktZ2llc3Nlbi5kZQ==
†These authors have contributed equally to this work
Disclaimer: All claims expressed in this article are solely those of the authors and do not necessarily represent those of their affiliated organizations, or those of the publisher, the editors and the reviewers. Any product that may be evaluated in this article or claim that may be made by its manufacturer is not guaranteed or endorsed by the publisher.
Research integrity at Frontiers
Learn more about the work of our research integrity team to safeguard the quality of each article we publish.