- 1Plant Molecular Biology Laboratory, M.S. Swaminathan Research Foundation, Chennai, India
- 2National Centre for Biological Sciences, Tata Institute of Fundamental Research, Bengaluru, India
- 3Tasmanian Institute of Agriculture, College of Science and Engineering, University of Tasmania, Hobart, TAS, Australia
- 4School of Science and Health, Hawkesbury Institute for the Environment, Western Sydney University, Penrith, NSW, Australia
The genome of Asian cultivated rice (Oryza sativa L.) shows the presence of six organelle-specific and one plasma membrane (OsNHX1-7) NHX-type cation proton antiporters. Of these, vacuolar-localized OsNHX1 is extensively characterized. The genus Oryza consists of 27 species and 11 genome-types, with cultivated rice, diploid O. sativa, having an AA-type genome. Oryza NHX1 orthologous regions (gene organization, 5′ upstream cis elements, amino acid residues/motifs) from closely related Oryza AA genomes cluster distinctly from NHX1 regions from more ancestral Oryza BB, FF and KKLL genomes. These sequence-specific distinctions also extend to two separate intron retention (IR) events involving Oryza NHX1 transcripts that occur at the 5′ and 3′ ends of the NHX1 transcripts. We demonstrate that the IR event involving the 5′ UTR is present only in more recently evolved Oryza AA genomes while the IR event governing retention of the 13th intron of Oryza NHX1 (terminal intron) is more ancient in origin, also occurring in halophytic wild rice, Oryza coarctata (KKLL). We also report presence of a retro-copy of the OcNHX1 cDNA in the genome of O. coarctata (rOcNHX1). Preferential species and tissue specific up- or down-regulation of the correctly spliced NHX1 transcript/5′ UTR/13th intron-retaining splice variants under salinity was observed. The implications of IR on NHX1 mRNA stability and ORF diversity in Oryza spp. is discussed.
Introduction
Plant growth, development and abiotic stress responses crucially depend on the regulation of intracellular ion homeostasis in different cellular endomembrane compartments (Bassil and Blumwald, 2014). To a large extent, cellular ion homeostasis is driven by compartment-specific activity of H+-translocating enzymes (H+-pumping ATPases or pyrophosphatases) that establish a H+ gradient utilized by other membrane transporters to couple the passive transport of H+ with an active uptake of ions (K+, Na+) against their electrochemical gradient (Martinoia et al., 2007; Amtmann and Leigh, 2009). This active uptake of ions provides the driving force vital for regulation of internal pH and cell volume expansion related processes (Bassil and Blumwald, 2014) as well as supporting metabolic activities of the cell. Na+/H+ exchangers (NHX) belong to monovalent cation/proton antiporter (CPA1) group of transporters (Maser et al., 2001; Sze and Chanroj, 2018). In plants, NHXs are ubiquitous, exist as multigene families and catalyze the electroneutral exchange of H+ for Na+ or K+. NHXs are present in both the plasma membrane (Shi et al., 2002) and in intracellular membranous compartments (IC), with the latter being localized to both vacuolar and endosomal membranes (Bassil and Blumwald, 2014). Previous research involving plant NHXs has primarily focused on their role in conferring salinity tolerance by Na+ sequestration in vacuoles under salinity. More recent studies suggest that, in addition to compartmentalizing Na+ in vacuoles in under salinity, they also have a role in maintaining K+ homeostasis (Bassil and Blumwald, 2014; Reguera et al., 2015; Bassil et al., 2019) and thus confer tissue salinity tolerance.
The genome of Asian cultivated rice, Oryza sativa L., shows the presence of one plasma membrane-based (OsNHX7/OsSOS1) and six organelle-specific (OsNHX1-6) antiporters. Of these, OsNHX1 has been extensively characterized. The OsNHX1 cDNA encodes a vacuolar antiporter (Fukuda et al., 1999), with expression of the OsNHX1 transcript being induced by NaCl/KCl treatments in both roots and shoots (Fukuda et al., 2004). OsNHX1 suppresses Na+, Li+ and hygromycin sensitivity of yeast nhx1 mutants and sensitivity to a high K+ concentration while overexpression of OsNHX1 in rice imparts salinity tolerance at seedling and reproductive stages (Fukuda et al., 2004; Islam and Seraj, 2009; Biswas et al., 2015; Amin et al., 2016).
Alternative splicing (AS) is an important post-transcriptional gene regulatory mechanism contributing to transcript diversity from the same locus, resulting in the production of two or more transcript isoforms from a given pre-mRNA (Filichkin et al., 2015). Five basic modes of AS are recognized: exon skipping, mutually exclusive exons, intron-retention (IR), alternative donor sites, and alternative acceptor sites (Zhang et al., 2015). Of these, IR events are the most common form of AS observed in plants (Ner-Gaon et al., 2004; Mei et al., 2017). IR in the 5′ UTR or 3′ UTR can affect transcript stability and/or translatability. However, IR between coding exons can lead to loss of ORF integrity, introduction of premature termination codons (PTCs) that can mark transcripts for nonsense mediated decay (NMD; Nicholson et al., 2010). The biological rationale for increased IR during AS of transcripts within coding regions of transcripts in plants is now being increasingly understood. Stimulus-specific reversible sequestration of IR transcripts with subsequent splicing may be a mechanism to prevent targeting by the NMD pathway and rapid protein synthesis in the absence of transcription (Boothby et al., 2013; Gohring et al., 2014; Brown et al., 2015; Filichkin et al., 2015). In O. sativa, three splice variants have been reported for OsNHX1. Two AS transcripts are generated by splicing out/retention of an intron in the OsNHX1 5′ UTR while the third transcript retains the terminal 13th intron, leading to a truncated ORF (Jung et al., 2009).
The genus Oryza spans ∼15 million years of evolution, has 27 species and consists of 11 genome types, six of which are diploid (AA, BB, CC, EE, FF, GG) and four that are polyploid (BBCC, CCDD, HHJJ, KKLL; Stein et al., 2018). With a view to understanding conservation of the mentioned AS events in NHX1 orthologs in the genus Oryza, we first examined gene structure, conserved motifs/amino acid residues and promoter motifs in 11 Oryza species. Using RT-PCR and RACE analysis we demonstrate that the spliceosomal machinery, governing retention of the 13th intron, appears to have a more ancient origin while that controlling IR involving the 5′ UTR is of more recent origin, and is present only in Oryza AA genomes. The latter can be attributed to substantial differences in the sequence of 5′ UTRs of NHX1 in species belonging to Oryza AA genomes vis-à-vis the Oryza BB, FF and KKLL genomes. Preferential tissue specific up- or downregulation of the differentially spliced intron retaining/excluding NHX1 transcripts under salinity is observed in Oryza spp.
Materials and Methods
Bioinformatic Analysis
Oryza spp. NHX1 sequences were retrieved from Ensembl Plants1 using O. coarctata NHX1 genomic sequence as query (Accession No. JQ796375.1; Kizhakkedath et al., 2015) and searched against Oryza sativa japonica (IRGSP-1.0; Supplementary Table S1). The Na+/H+ exchanger domain was identified in corresponding NHX1 protein sequences using HMMER v 2.28.02, and domain boundaries were manually curated based on multiple sequence alignment (MSA) generated using CLUSTALW23. Phylogenetic relationships were inferred using neighbor joining with Setaria italica NHX1 (XP_004958612.1) as the outgroup. Bootstrap values were derived from 1,000 replicate runs and the phylogenetic tree was constructed using MEGA 7 (Kumar et al., 2016).
5′ upstream sequences of Oryza NHX1s were retrieved by querying the NCBI whole genome shotgun (WGS) database (Oryza subset) using the O. coarctata NHX1 5′ upstream region (JQ796375.1). Transcription factor binding sites (TFBS) were predicted using the algorithm Stress-Responsive Transcription Factor DataBase2 (STIF DB2; Naika et al., 2013), with a z-score threshold cut off of ≥ 1.5. A database of Hidden Markov Models of known stress involved TFBS was employed in the STIF algorithm to predict binding sites in 5′ upstream region of genes. TFBS were graphically represented using GSDS 2.0 (Hu et al., 2015).
Plant Treatments
Isolation of Splice Variants From O. coarctata and O. sativa
O. coarctata used in the present study was collected from Pichavaram, Tamil Nadu, India and maintained in a greenhouse (temperature: 25 ± 2°C; 12 h light/12 h dark photoperiod). Two-month-old tillers were transferred to half strength MS medium (Murashige and Skoog, 1962). Following acclimatization, 1-month-old tillers were transferred to MS medium containing 150 mM NaCl. Seeds of O. sativa ssp. japonica cv. Dinorado (AC41038), O. sativa ssp. indica cv.IR20 (AC41066), O. barthii (AC100498), O. nivara (AC100010), and O. rufipogon (AC100028) were obtained from CRRI, Cuttack, India while O. sativa ssp. indica cv. Pokkali (IC324582) was obtained from NBPGR, Thrissur, India. Following dormancy breaking, seeds were sown in vermiculite and irrigated with Yoshida medium (25 ± 1°C; 16 h light/8 h dark; Yoshida et al., 1976) for 2 weeks. The seedlings were then incrementally stressed with NaCl (0, 25, and 50 mM in Yoshida medium) every second day. In both cases, leaf tissues were collected and used for RNA isolation.
Plant Treatments for qRT-PCR Analysis
For expression studies, O. coarctata (3-month-old) and Oryza spp. (25 day-old seedlings) were subjected to incremental salinity stress in 0, 25, 50 and 75 mM NaCl (in Yoshida medium at 3-day intervals) and finally in 100 mM NaCl (4 days). Leaf and root tissues were frozen in liquid nitrogen and used for RNA isolation.
Isolation of rOcNHX1 From O. coarctata Genomic DNA
Overlapping rOcNHX1 genomic fragments were assembled using a combination of (i) genomic PCR (OcNHX1 cDNA-specific primers; single-step PCR as well as nested PCRs; Supplementary Table S2 and Supplementary Figure S1) and (ii) inverse PCR strategies. For the former, O. coarctata genomic DNA (50 ng) was used as template for PCR amplification with OcNHX1 specific primers (Supplementary Table S2). Amplified rOcNHX1 fragments corresponding to the OcNHX1 cDNA size were gel eluted. The amplified product was subjected to electrophoresis in agarose gel from which it was then extracted and purified. Purified fragments were cloned in a T/A vector (pTZ57R/T; Fermentas, United States) and sequenced with universal M13 F/R primers. For nested genomic PCRs, the primary PCR reaction was performed in a 20 μL volume with Pfu polymerase (Fermentas, United States; 1.25 U). The primary PCR product was diluted (1:40) and 2 μL of template was used for secondary PCR. The 1:20 diluted secondary PCR sample was used for tertiary PCR. PCR products were cloned and sequenced as described before.
For inverse PCR, 100 ng of O. coarctata genomic DNA (XhoI digestion) was ligated overnight at 16°C with T4 DNA ligase (4U; Fermentas, United States) and precipitated using ethanol. Ligated DNA was used for primary PCR [20 μL reaction volume; 5 μL ligated genomic DNA, 250 nM each dNTP, 200 nM of each oligonucleotide primer; 1.25 U Pfu polymerase (Fermentas, United States)]. To increase specificity, secondary and tertiary PCRs were carried out with OcNHX1-specific (cDNA junction primers) nested primers. The amplified product was purified, cloned in a T/A vector and sequenced as described above.
RNA Isolation and RT-PCR
For samples mentioned in Plant Treatments for qRT-PCR (Materials and Methods), total RNA was isolated using RNAiso Plus (Takara) as per the manufacturer’s protocol. For isolation of the OcNHX1i13 splice variant (O. coarctata 13th intron retaining splice variant), cDNA was synthesized from 1 μg of DNase1 treated O. coarctata leaf total RNA. Primer pairs used to amplify the 13th intron retention splice variants from O. coarctata cDNA are listed in Supplementary Table S2. A “minus RT” (–RT-PCR) reaction was included to eliminate genomic DNA contamination in all cases. The fragments were cloned in T/A vector and sequenced. Oryza spp. 13th intron retention RT-PCR products were also amplified from O. sativa ssp. japonica cv. Dinorado, O. sativa ssp. indica cv. Pokkali, O. barthii, O. glaberrima, O. rufipogon, O. nivara, cloned in T/A vector and the fragments from O. sativa ssp. japonica cv. Dinorado and O. barthii sequenced. 5′ UTR splice variants from Oryza spp. were amplified from their respective cDNAs using OsNHX1 Fwd1/Rev1 primers (Supplementary Table S2). Specific fragments (O. sativa ssp. indica cv. IR20, O. sativa ssp. japonica cv. Dinorado, O. barthii, O. nivara) were eluted, cloned in T/A vector and sequenced.
3′ RACE Amplification of OcNHX1i13
3′ RACE was performed with the SMARTer RACE 5′/3′ cDNA amplification kit (BD Biosciences, Takara). Template RNA was extracted as described above. 3′ RACE was performed according to the manufacturer’s instructions using nested primers listed in Supplementary Table S2. The primers used for nested PCR were designed against the exon-intron junction [GSP1 (13th exon/13th intron)] and in the 13th intron (GSP2) respectively]. The RACE-PCR product was gel eluted and sequenced.
Real-Time Quantitative PCR Analysis (O. coarctata; Oryza spp.)
First strand cDNA synthesis was performed in a 20 μL reaction volume with 1 μg of total RNA and Superscript III (Invitrogen, United States) at 42°C for 60 min, followed by heat inactivation at 70oC for 10 min. The primer pairs listed in Supplementary Table S2 were used to amplify fragments of indicated sizes for each splice variant (Oc-β-Actin; housekeeping gene; internal control). Real time PCR (Quant Studio 6; Thermo Fisher Scientific, United States) was carried out in a reaction volume of 10 μL [1 μL of cDNA, 5 μL of Takara TB GreenTM Primix Ex TaqTM II (2X), 0.5 μL each of a given primer pair (final concentration 250 nM each) under the following cycling conditions: 95°C (30 s), 40 cycles of denaturation at 95°C (5 s), annealing and extension at 60°C (30 s) in a 96-well optical reaction plate (Thermo Fisher Scientific, United States). Each real time PCR reaction was performed in triplicate, in order to evaluate data reproducibility for three biological replicates. Amplicon specificity was verified by melt curve analysis (60–95°C at 40 cycles) and subsequent agarose gel electrophoresis. Gene expression was quantified using the comparative CT (2–Δ Δ CT) quantitation method for all splice variants (O. coarctata and Oryza spp.) in leaf and root tissues, with values representing “n”-fold difference relative to the housekeeping control gene.
Results
The NHX1 Gene Shows Conservation of Exon Number and Key Amino Acid Residues Within the Encoded NHX Na+/H+ Exchanger Domain Across Oryza Species
There is substantial variation in the lengths of the genomic and coding regions of the eleven Oryza spp. NHX1 sequences examined (Supplementary Figure S2 and Supplementary Table S1). A maximum genomic length of 4,535 bp was observed for O. brachyantha, followed by 4,472 bp for O. longistaminata and the shortest sequence length of 4,103 bp was observed for O. coarctata. Of all the Oryza NHX1 amino acid sequences examined, a maximum of length 540 amino acid residues was observed for O. coarctata and the shortest of 505 amino acids for O. longistaminata (Supplementary Table S1). All Oryza NHX1 genes examined showed a conserved exon-intron organization: viz.14 exons and 13 introns (Supplementary Figure S2), with the second and the thirteenth (last) introns being the longest.
A single Na+/H+ exchanger domain belonging to the sodium/hydrogen exchanger family is identifiable in all the Oryza species examined using HMMER (Supplementary Figure S3). Domain boundaries were manually curated by multiple alignment; nine of the Oryza spp. NHX1 have similar domain lengths (434 amino acid residues), with O. longistaminata, and O. glumaepatula showing shorter domain lengths of 392 and 412 amino acid residues, respectively (Figure 1). Both O. longistaminata and O. glumaepatula sequences show deletions (42 and 22 amino acid residues, respectively) in the region centered around and inclusive of the Na+/H+ inhibitory amiloride binding domain “LFFIYLLPPI.” Two of three identified putative glycosylation sites are absolutely conserved (“NES”; “NVT”) across all Oryza species while the third site shows variation at the third amino acid residue (VLI/V/L). In addition, motifs “A1T2D3S4,” “E5---N6D7,” serine and arginine (R8) residues (marked ∗) are also absolutely conserved across the Oryza species. These motifs and residues do not occur sequentially but appear on different transmembrane helices that form part of the protein core containing the substrate binding site and have been implicated in antiporter function (with the exception of serine marked with an asterisk; Masrati et al., 2018).
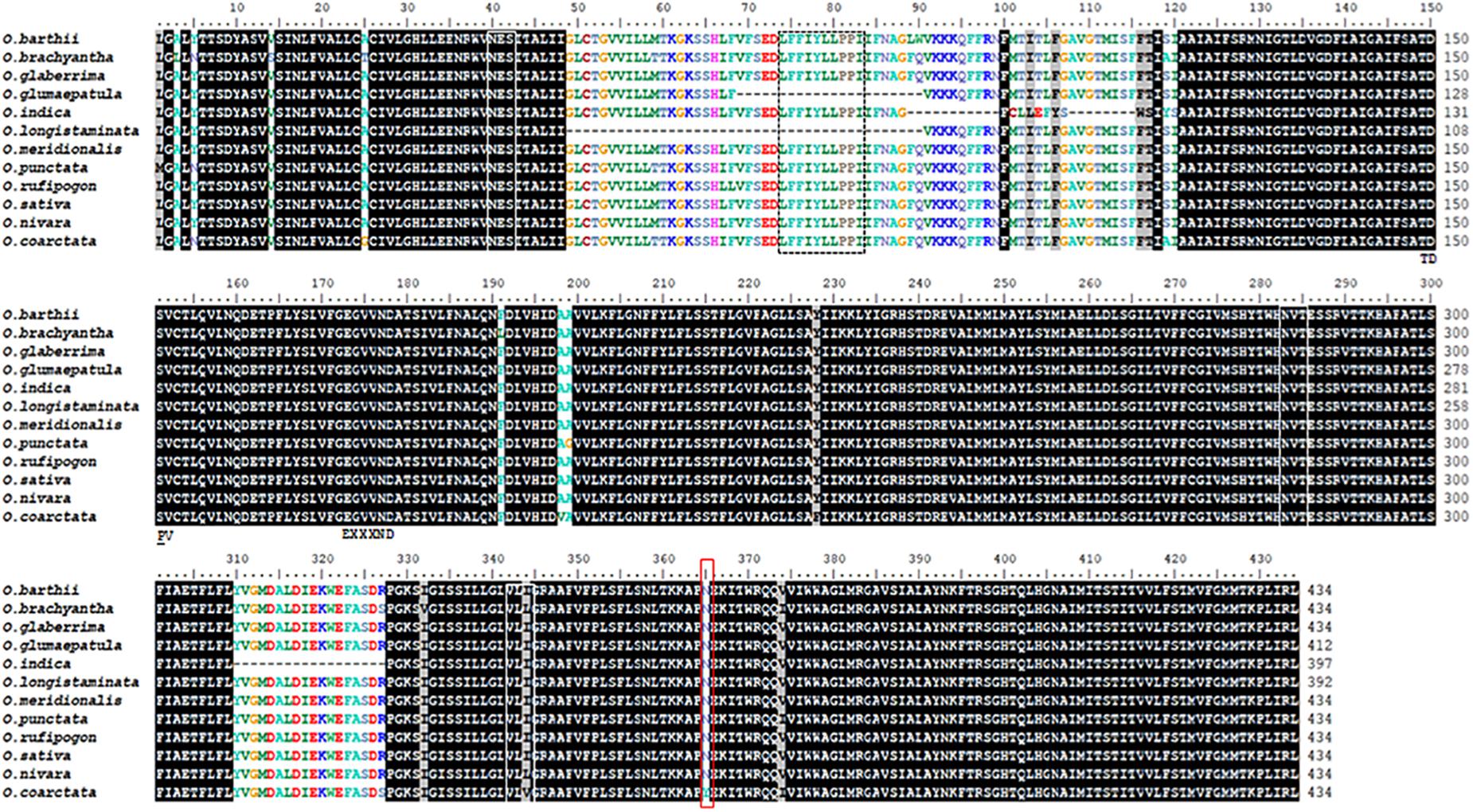
Figure 1. Alignment of the Na+/H+ exchanger domain found in NHX1 protein sequences (Oryza species). The Na+/H+ exchanger domain length is approximately 443-446 amino acid residues (O. longistaminata and O. glumaepatula are exceptions). The Na+/H+ inhibitory amiloride binding domain “LFFIYLLPPI” is boxed (dotted line). Putative glycosylation sites are conserved and indicated by white boxes (“NES,” “NVT,” and “VLI/V/L”). Motifs implicated in antiporter function: “A1T2D3S4” (marked TDPV), “E5---N6D7” (marked EXXND), serine and arginine (R8) residues (marked *) are also absolutely conserved across Oryza species. These motifs and residues occur on different transmembrane helices that form part of the protein core containing the substrate binding site (Masrati et al., 2018). The amino acid residues boxed in red are conserved across Oryza species except in O. coarctata.
Phylogenetic analysis of the NHX1 domain protein sequences recognizes two main clades in which all the Oryza spp. NHX1 sequences belonging to AA genomes (expect O. nivara) cluster together while O. coarctata (KKLL), O. brachyantha (FF), and O. punctata (BB) NHX1 sequences cluster separately and are more closely related (Supplementary Figure S4).
Analysis of the 5′ Upstream Sequences of Oryza NHX1 Identifies a Conserved Order of cis-Elements That Are Implicated in Abiotic Stress Responsiveness
Aligned 5′ upstream sequences of Oryza spp. NHX1 (Supplementary Figure S5) showed variation in length, with a maximum size of 1,590 bp (O. brachyantha) and a minimum size of 1,367 bp (O. barthii). In eight of the eleven sequences examined, two conserved regions were identified: (i) the proximal conserved region (PCRe) encompassing bases -1 to -275 bp upstream of NHX1 “ATG” start codon (O. coarctata promoter sequence used as reference for numbering; inclusive of 5′ UTR) and (ii) the distal conserved region (DCRe), starting -276 bp upstream of the NHX1 gene to end of the analyzed sequence (upstream) examined (Supplementary Figure S5 and Figure 2A). Transcription factor (TF) binding cis-motifs were predicted using the STIF algorithm (Naika et al., 2013) and 16 cis-elements each were identified in O. glumaepatula and O. meridionalis while 12 cis-elements each were identified in O. coarctata and O. punctata.
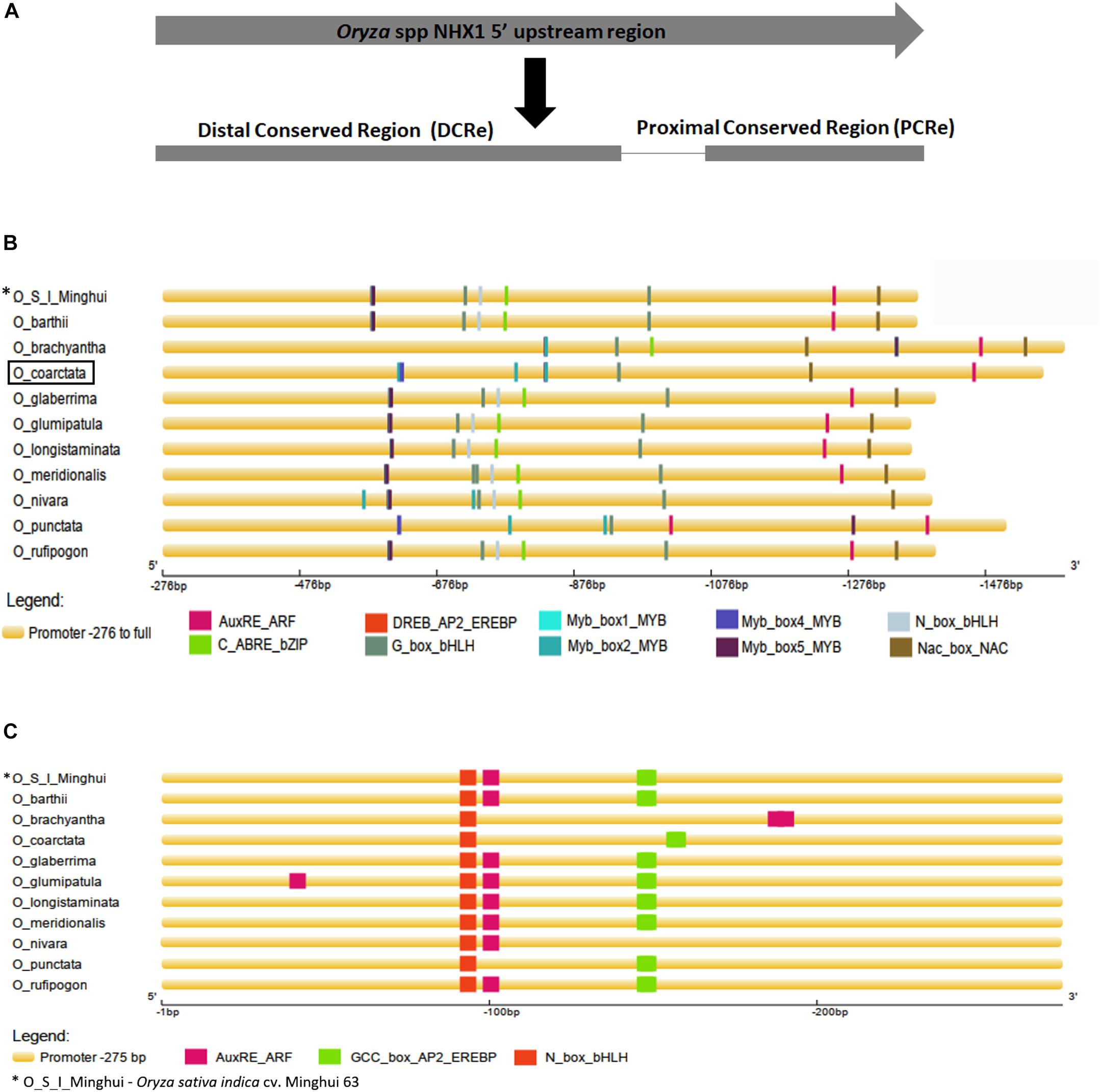
Figure 2. Analysis of 5′ upstream sequences from Oryza species. (A) Alignment of NHX1 5′ upstream sequences from Oryza species shows two conserved stretches (i) Region 1, starting -276 bp upstream of the NHX1 5′ UTR to the end of examined sequence (O. coarctata NHX1 promoter sequence used as reference for numbering) and (ii) Region 2 encompassing bases -1 to -275 bp, upstream of the NHX1 5′ UTR. (B,C) Prediction of transcription factor binding cis-elements using STIF DB2 (Naika et al., 2013) in the 5′ upstream sequences of NHX1 (Oryza species), Region 1 and Region 2 respectively, aligned NHX1.
Multiple cis-elements were clustered within the DCRe of the Oryza NHX1 gene 5′ upstream sequences analyzed (Figure 2B). Based on the cis-elements and their relative positions/order within the DCRe, sequences from Oryza AA genomes cluster into one group while O. coarctata, O. brachyantha and O. punctata share a similar organization of cis-elements in their 5′ upstream sequences. In the Oryza AA genome, NHX1 5′ upstream sequences, cis-elements were found in the order (-275 to end): (i) overlapping Myb/DREB binding element; (ii) Gbox-HLH; (iii) Nbox-HLH binding site; (iv) ABRE/bZIP; (v) Gbox-HLH binding site; (vi) AuxRE; and (viii) NAC binding site. O. coarctata, O. brachyantha and O. punctata NHX1 5′ upstream sequences (-275 to end) show: (i) one NAC binding site; (ii) one AuxRE; (iii) one Gbox-HLH; and (iv) numerous Myb TF binding sites. All eleven sequences show the presence of a highly conserved distal Gbox-HLH motif, ten have NHX1 5- upstream sequences and, in addition, show conservation of the AuXRE (O. nivara is an exception) and nine sequences show conservation of a distal NAC TF binding region in the DCRe.
Within the PCRe, seven Oryza NHX1 gene upstream sequences (Figure 2C) show the presence of a conserved N_box_bHLH cis-element, the AuxRE and the GCC_box_AP2_EREBP cis-element. With the exception of O. nivara, sequences from Oryza AA genomes cluster together, based on the cis-elements and their relative positions/order within the PCRe.
Alternative Splicing of an Intron in the 5′ UTR of the NHX1 Gene in Oryza AA Genomes Leads to Two Distinct Transcripts
The 5′ UTR of NHX1 is highly conserved in Oryza species containing the AA genome (Supplementary Figure S6) and includes a conserved intron that is either retained or spliced out to give two distinct O. sativa OsNHX1 transcripts (Jung et al., 2009). We examined if the alternative splicing events observed for the 5′ UTR of NHX1 are conserved in other Oryza species containing AA genomes. RT-PCR analysis of O. barthii and O. nivara, O. rufipogon, O. sativa (cvv. IR20 and Pokkali) using leaf tissues and NHX1 5′ UTR-specific primers (OsNHX1 Fwd/OsNHX1 Rev1) amplified one fragment showing IR (labeled Frag-G) and a second transcript, termed Frag-cD, in which the first intron was spliced out (Figure 3A; marked + RT). DNase I treatment was used to eliminate genomic DNA contamination prior to RT-PCR (Figure 3A; marked -RT). The two cDNA fragments (Frag-G/Frag-cD) obtained for O. sativa (cv. japonica and cv. IR20), O. nivara, and O. barthii are shown in Figure 3B. RT-PCR products corresponding to Frag-G and Frag-cD were cloned for O. sativa cv. IR20 and O. barthii (Figure 3C). BLAST analysis confirmed in all cases that the cloned fragments corresponded with OsNHX1. The 5′ UTR sequence of NHX1 examined in the Oryza AA species shows a high degree of conservation including the intron. The O. sativa cDNA fragments (cv. japonica and IR20) group together and show two distinct differences from O. nivara and O. barthii: (i) length of a “C” repeat region in the intron differs and (ii) the presence of a “GATCTTT” insertion in the second exon (also part of the 5′ UTR; underlined).
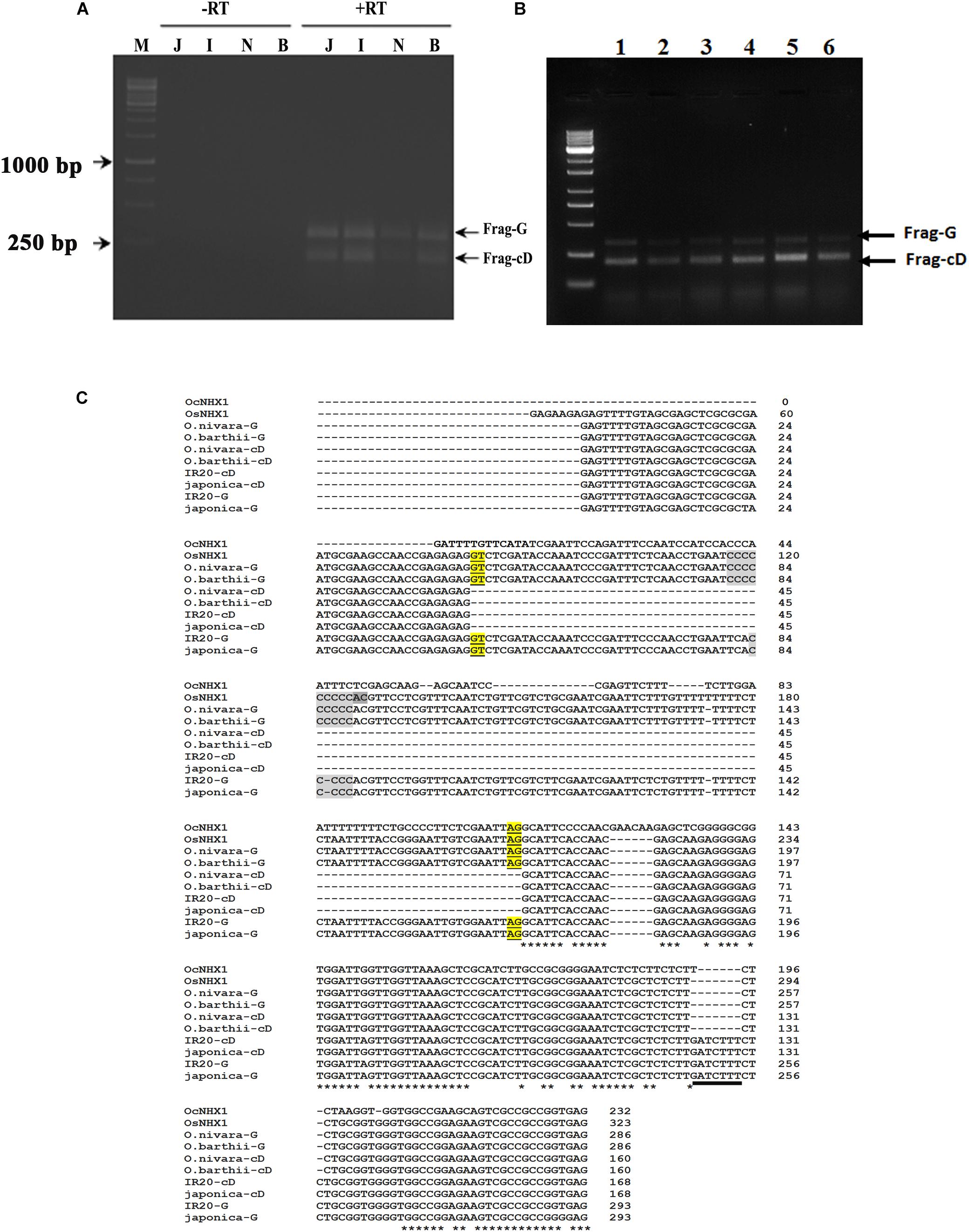
Figure 3. Cloning of 5′ UTR NHX1 splice variants from Oryza AA genome types. (A) RT-PCR analysis of NHX1 5′ UTR splice variants from O. sativa [ssp. japonica (J), cv. IR20 (I), O. nivara (N), and O. barthii (B) that either retain the first intron (Frag-G) or splice it out (Frag-cD). M- Marker, Letter on top of each image indicate the rice spices; J-Japonica, I-indica IR20, N-O. nivara, and B-O. barthii; -RT: PCR using DNase treated RNA, + RT: PCR using cDNA. (B) RT-PCR analysis of NHX1 5′ UTR splice variants from O. barthii (1), O. nivara (2), O. glaberrima (3), O. sativa [ssp. japonica (4)], O. sativa [ssp. indica cv. Pokkali (5)], O. rufipogon (6); (Frag-G), and (Frag-cD) are indicated. (C) RT-PCR fragments shown in (A) were cloned and sequenced. The O. sativa NHX1 cDNA fragments (cv. japonica and IR20) group together and show two distinct differences from O. nivara and O. barthii cDNA fragments: (i) length of a “C” repeat region in the intron and (ii) the presence of a “GATCTTT” insertion in the second exon that is underlined (5′ UTR); the “GATCTTT” insertion also occurs in O. rufipogon and O. longistaminata (Supplementary Figure S6).
The OcNHX1i13 Transcript From Wild Rice O. coarctata Shows Retention of the 13th Intron
Of the three OsNHX1 transcripts reported, Os07g47100.3 (OsNHX1; MSU annotation; japonica) shows retention of the 13th intron. RT-PCR analysis of salinity-treated O. coarctata leaf tissues (150 mM NaCl) confirmed that the IR splicing event observed for OsNHX1 (13th intron) is also conserved in O. coarctata (OcNHX1i13; 680 bp); this was further confirmed by RT-PCR amplification of fragments of varying sizes using primers flanking the 13th intron (209, 680, 593, and 152 bp; Figures 4A–E). The 680 bp fragment showed 100% identity with the OcNHX1 genomic sequence: Accession no: JQ796375.1 (Kizhakkedath et al., 2015; Figure 4F). The 3′ RACE PCR OcNHX1i13 product shows complete retention of the 13th intron and 14th exon (Supplementary Figure S7).
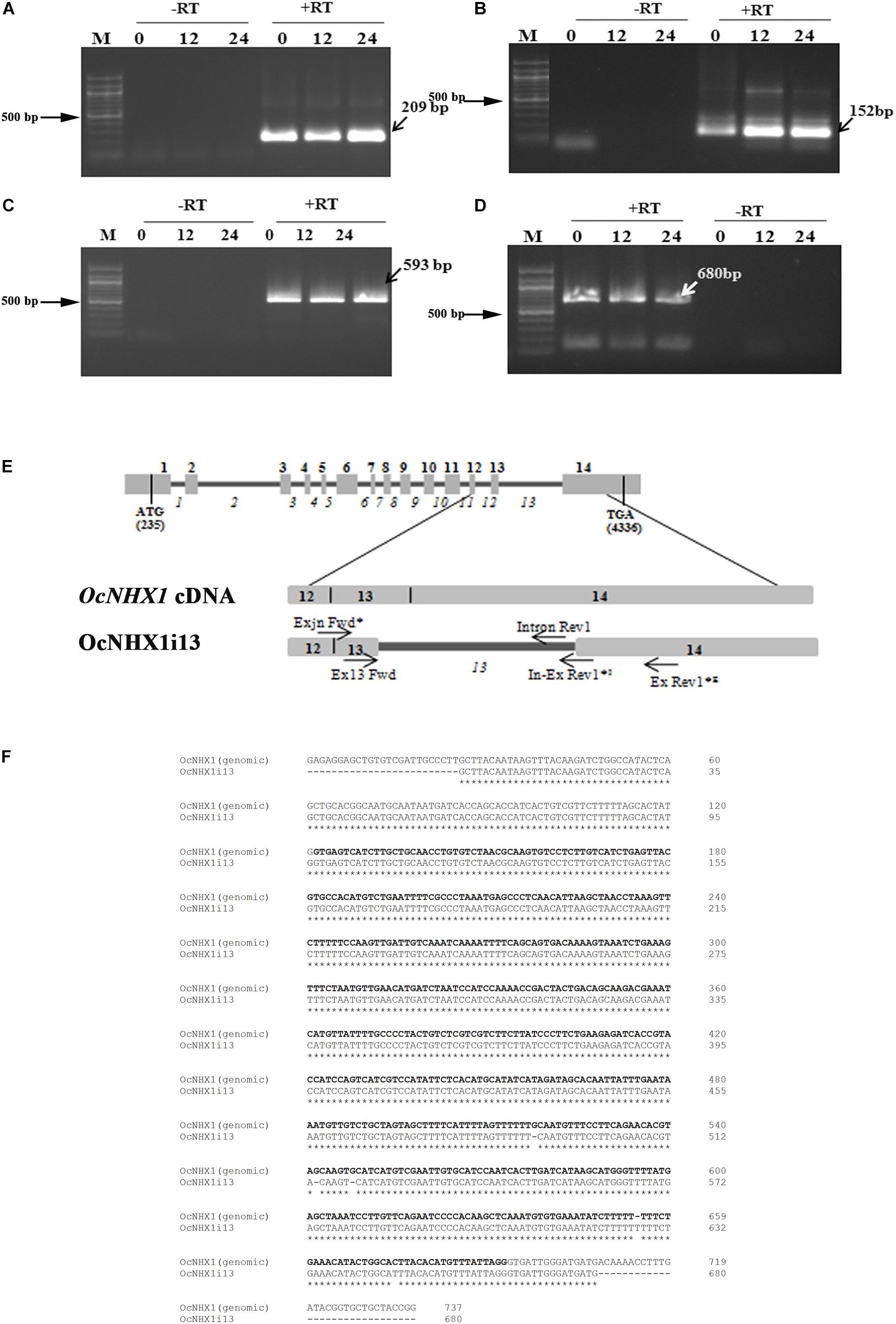
Figure 4. Cloning of the 13th intron retaining splice variant from O. coarctata. Complete retention of the 13th intron in O. coarctata is confirmed by RT-PCR amplification using primer pairs listed in Supplementary Table S2, generating fragments of sizes (A) 209 bp; (B) 152 bp; (C) 593 bp, and (D) 680 bp (OcNHX1il3), respectively. In all cases a “RT-PCR” reaction was included to rule out genomic DNA contamination. (E) Schematic representation showing alignment of the OcNHX1il3 RT-PCR product with the corresponding OcNHX1 genomic region. Primer pairs used to isolate OcNHX1il3 by RT-PCR are indicated by an asterisk (*). (F) Alignment of OcNHX1il3 and corresponding OcNHX1 genomic sequence. The 680 bp fragment showed near 100% identity with the corresponding OcNHX1 genomic sequence (Accession no: JQ796375.1). The 13th intron is highlighted in bold.
Retention of the 13th Intron in NHX1 Transcripts Is Also Observed in Oryza Species AA Genomes
RT-PCR products from the Oryza species containing the AA genome, O. barthii, O. glaberrima and O. nivara NHX1 (amplified by primers flanking the 13th intron), also show retention of the 13th intron (based on a larger amplified fragment size obtained; expected size based on OsNHX1: 534 bases). In addition, retention of the 13th intron was also confirmed in O. sativa subspp. japonica and O. sativa subspp. indica cv. Pokkali by RT-PCR (Figure 5A). Subsequent cloning and sequencing of O. barthii and Oryza sativa subspp. japonica RT-PCR products confirmed complete retention of the 13th intron (Figure 5B).
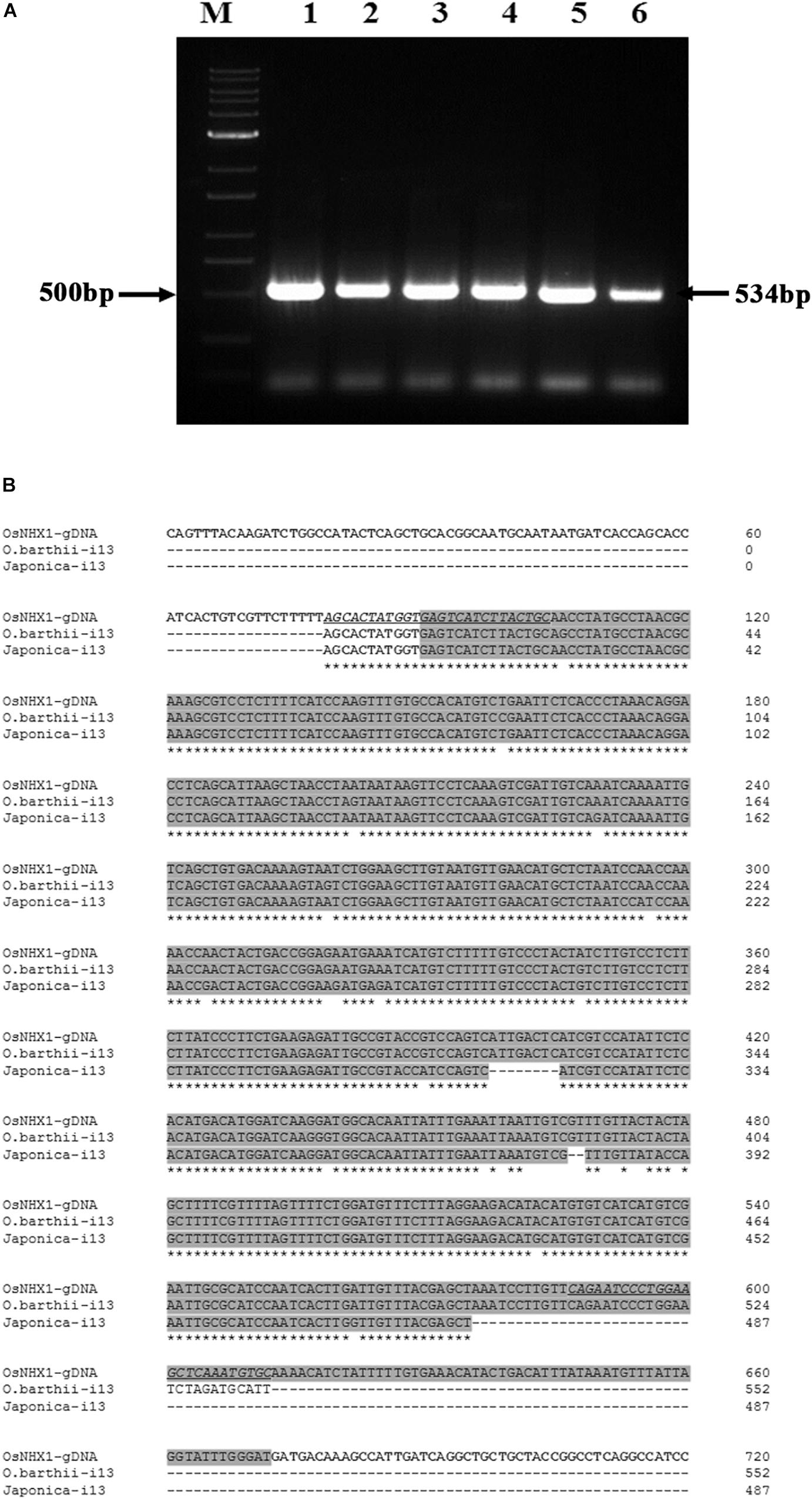
Figure 5. Cloning of NHX1 13th intron retaining splice variants from Oryza AA genome types. (A) RT-PCR (DNAse I treated RNA) amplification of 13th intron retaining NHX1 fragments from O. barthii (1), O. nivara (2), O. glaberrima (3), O. sativa [ssp. japonica (4)], O. sativa [ssp. indica cv. Pokkali (5)], O. rufipogon (6); Expected fragment size of 534 bp O. sativa [ssp. japonica] is indicated. Primers were designed to target the 13th intron of NHX1 in all cases (12th exon-13th intron junction forward and 13th intron reverse primer. (B) Sequence alignment OsNHX1 genomic DNA and RT-PCR fragments from O. sativa [ssp. japonica] and O. barthii from (A) were sequenced to show retention of the 13th intron. Primer sequence is underlined and the intron region is shaded gray.
The O. coarctata Genome Shows the Presence of a Retrocopy (rOcNHX1) of the OcNHX1 cDNA
While performing a routine PCR with O. coarctata genomic DNA, multiple fragments were amplified using primer pair Seq Fwd and Seq Rev (Supplementary Table S2). Of these, one corresponded exactly in size with OcNHX1 cDNA. This fragment was cloned and sequenced and was found to be identical to the OcNHX1 cDNA except for a 11 bp sequence insertion, corresponding to the 5′ end of the 11th intron of the OcNHX1 genomic sequence. Subsequent PCR amplifications using combinations of exon-exon junction primers suggested that a copy of the OcNHX1 cDNA may be inserted in the O. coarctata genome (Supplementary Figure S1). Using a combination of genome walking techniques, a 2,032 bp sequence corresponding to the OcNHX1 retrogene (rOcNHX1) was isolated from O. coarctata genomic DNA (Figures 6A–E and Supplementary Figure S8). rOcNHX1 differs from the OcNHX1 cDNA in having an 11 bp “GGCGAGTATTT” insertion identical to a region flanking the 5′ splice site of the 11th intron in the OcNHX1 genomic sequence (Figure 6G) and two single base pair changes. Analysis of the sequence read archive (SRA; O. coarctata transcriptome; Garg et al., 2013) confirmed the expression of rOcNHX1 in salinity stress treatments (450 and 700 mM NaCl, Submergence, Submergence + 450 mM NaCl; Figure 6F).
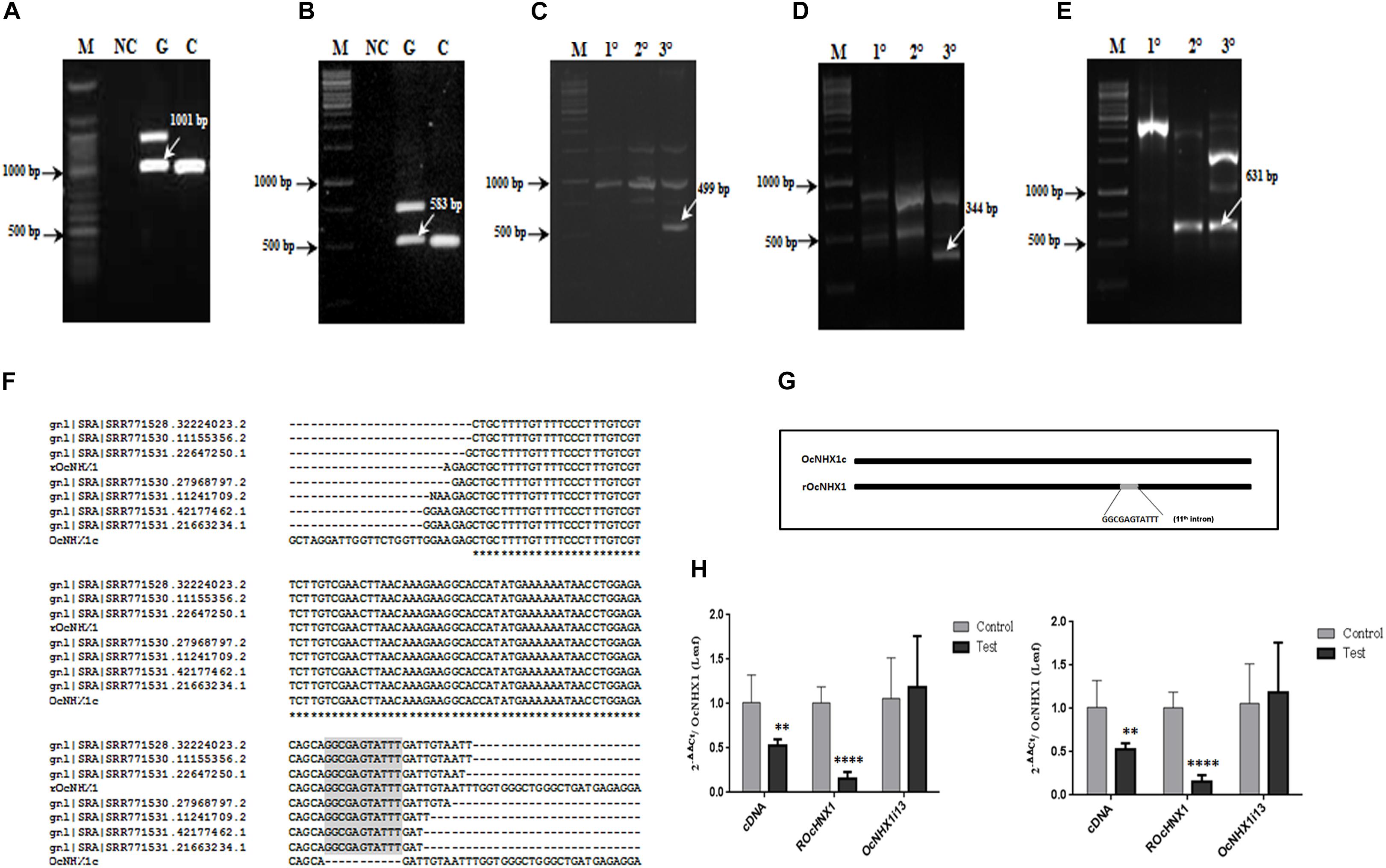
Figure 6. Isolation of rOcNHX1 from O. coarctata. (A–E) A 2,032 bp size OcNHX1 retrogene (rOcNHX1) was isolated from O. coarctata genomic DNA using a combination of genome walking techniques (also Supplementary Figure S1). (A,B) PCR with OcNHX1 specific primers and exon-exon junction primers used for gene isolation (1001 and 583 bp) (C) 499 bp fragment was isolated by Inverse PCR (XhoI enzyme) using nested primers. (D,E) 344and 602 bp amplicons were isolated by nested PCR. M- DNA ladder; NC-Negative control; G- O. coarctata genomic DNA; C- O. coarctata cDNA; 10 – Primary PCR; 20 –Secondary PCR; 30 – Tertiary PCR. (F) rOcNHX1 differs from the OcNHX1 cDNA only in having (i) 11-bp insertion corresponding to the part of the 5′ splice site of the 11th intron in the OcNHX1 genomic sequence (ii) 2 single nucleotide polymorphisms. (G) Analysis of the sequence read archive (SRA; O. coarctata transcriptome; Garg et al., 2013) confirmed the rOcNHX1 expression across stress treatments (450 and 700 mM NaCl, Submergence, Submergence + 450 mM NaCl). (H) qRT-PCR expression analysis of OcNHX1 splice variants in O. coarctata leaf and root tissues respectively under salinity. cDNA-correctly spliced OcNHX1 cDNA; rOcNHX1: OcNHX1 retrocopy; OcNHX1i13- OcNHX1 splice variant with retention of 13th intron. Data presented is the mean of three biological replicates (n = 3; with each replicate analyzed twice). Significance was calculated using one-way ANOVA (Student’s T-test). Statistical significance (p-value) : *P < 0.05; **P < 0.01, ***P < 0.001, ****P < 0.0001.
Alternatively Spliced O. coarctata and Oryza spp. NHX1 Transcripts Show Tissue Specific Switches in Isoform Expression Under Salinity
In O. coarctata leaf tissues, expression of the correctly spliced OcNHX1 cDNA and its retrocopy, rOcNHX1 is significantly reduced (P < 0.001 and P < 0.0001) under salinity stress while the 13th intron retaining transcript, OcNHX1i13, shows increased expression (Figure 6H). In contrast, in root tissues under salinity stress, expression of the correctly spliced OcNHX1 cDNA and rOcNHX1 (P < 0.0001) is upregulated and the 13th intron retaining transcript, OcNHX1i13 is downregulated (P < 0.001; Figure 6H).
We also examined expression of the correctly spliced NHX1 transcript, the 13th intron retaining transcript (NHXi13), the 5′ UTR intron retaining NHX1 transcript (R-NHX1) and the 5′ UTR intron splicing NHX1 transcript (Sp-NHX1) in leaf and root tissues (Figures 7, 8 and Supplementary Figures S9, S10) of four Oryza AA genome-type species (Oryza sativa subspp. japonica, Oryza sativa subspp. indica cv. Pokkali, O. rufipogon, O. barthii, O. glaberrima, and O. nivara) under salinity, the salient features for which are summarized as:
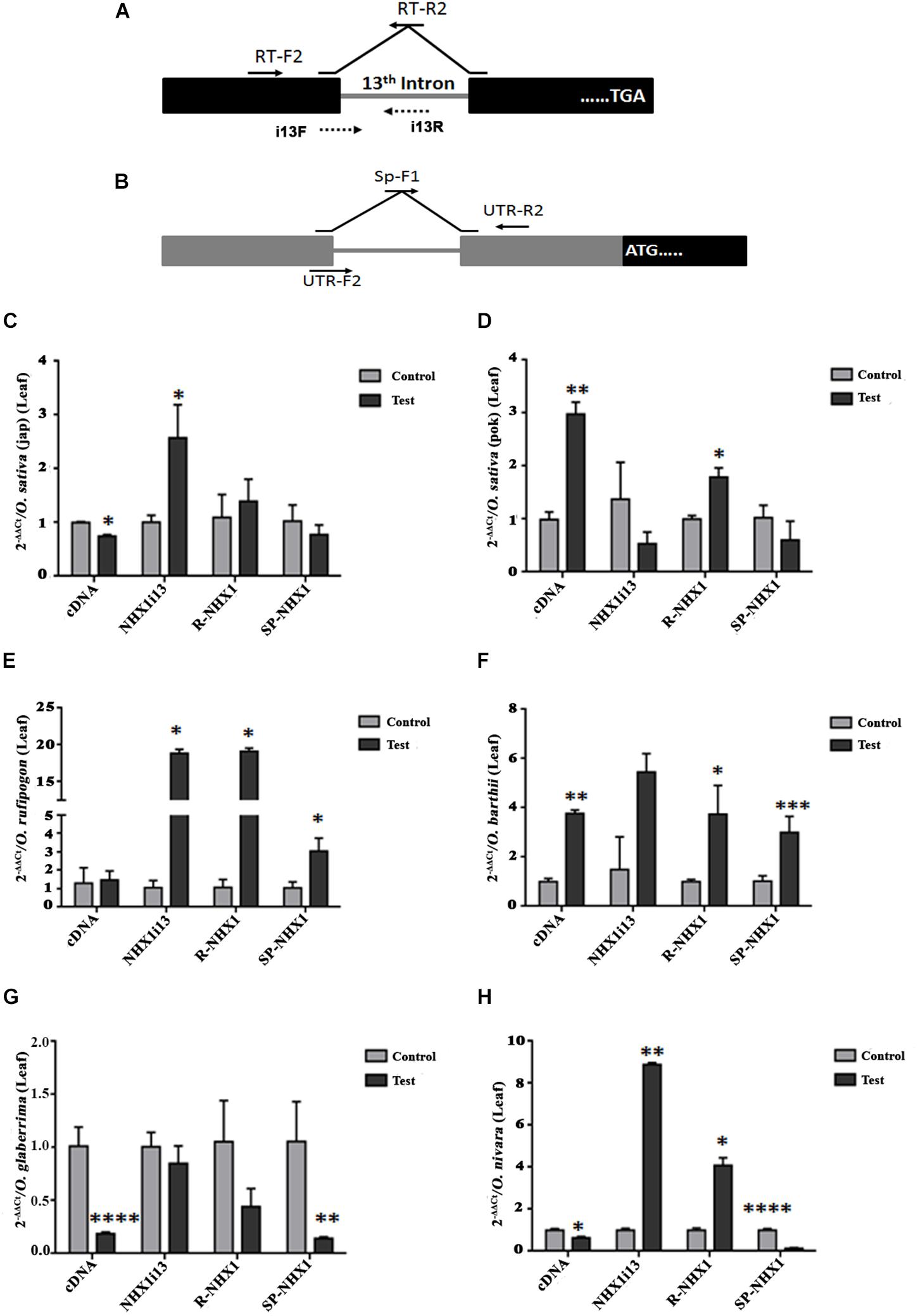
Figure 7. qRT-PCR expression analysis of NHX1 splice variants in Oryza spp. leaf tissues under salinity. The following splice variants were examined: correctly spliced transcript (cDNA), 13th intron retaining transcript (NHXi13), the 5′ UTR intron retaining NHX1 transcript (R-NHX1) and the 5′ UTR intron splicing NHX1 transcript (Sp-NHX1). (A) For the correctly spliced NHX1 transcript 12th exon forward primer (RT-F2) and 13th–12th exon reverse (RT-R2) primers were used (Kizhakkedath et al., 2015). For the 13th intron retention transcript i13F (12th exon-13th intron junction) and i13R (13th intron) primers were used. (B) For the 5′ UTR intron retaining/splicing transcripts primer pairs (UTR-F2 and UTR-R2; Sp-F1 and UTR-R2 respectively) were use (Supplementary Table S2). (C–H) Oryza spp. examined: O. sativa [ssp. japonica (C)], O. sativa [ssp. indica cv. Pokkali (D)], O. rufipogon (E), O. barthii (F), O. glaberrima (G), O. nivara (H). All qRT-PCRs for a given sample (splice variants) were analyzed on the same plate under identical reaction conditions from the same cDNA batch. Data presented is the mean of three biological replicates (n = 3; with each replicate analyzed twice; each replicate had 5 pooled plants). Significance was calculated using one-way ANOVA (Student’s T-test). *P < 0.05; **P < 0.01, ***P < 0.001, ****P < 0.0001.
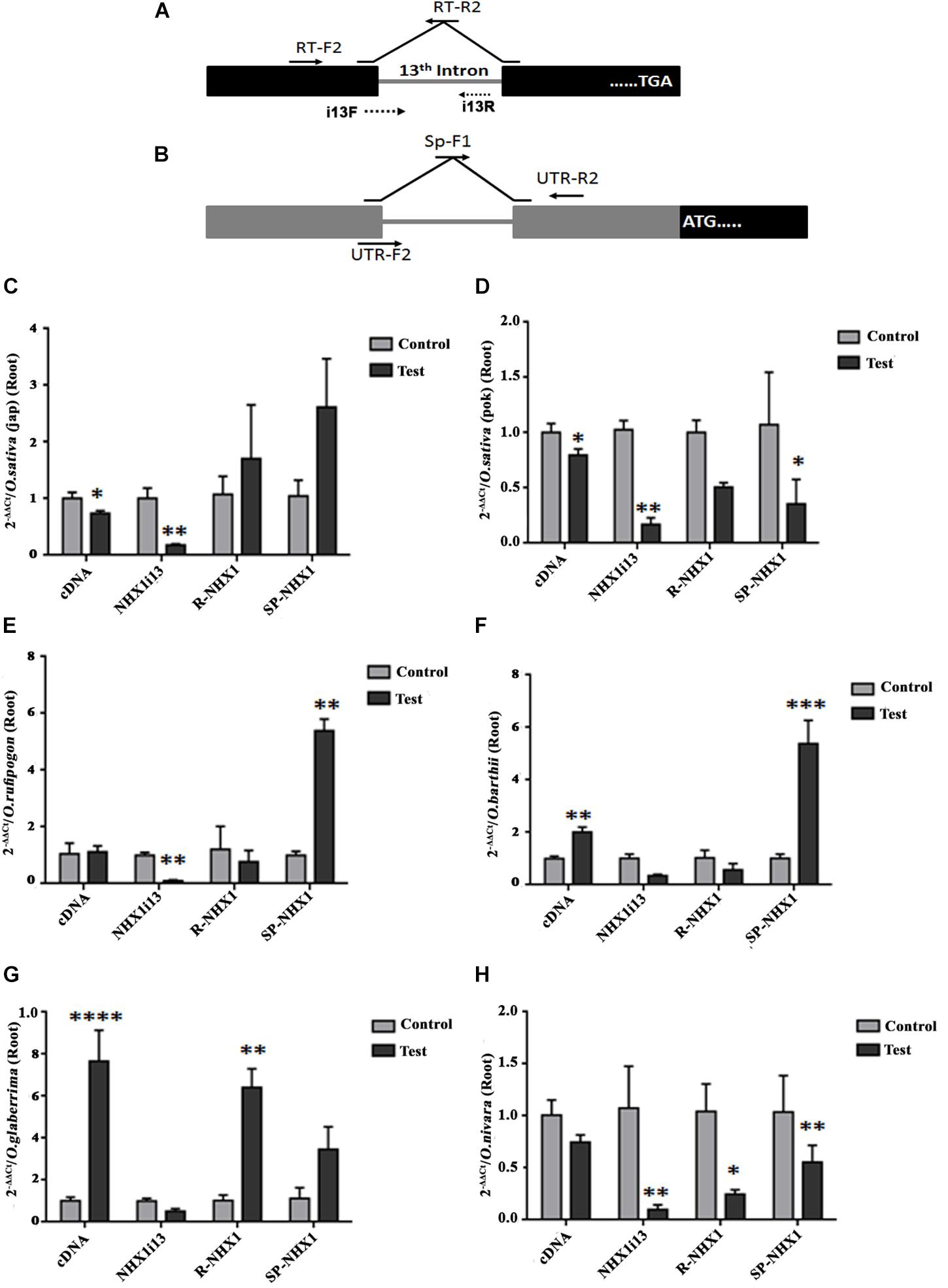
Figure 8. qRT-PCR expression analysis of NHX1 splice variants in Oryza spp. root tissues under salinity. (A,B) Same as in Figures 7A,B. (C–H) Oryza spp. examined: O. sativa [ssp. japonica (C)], O. sativa [ssp. indica cv. Pokkali (D)], O. rufipogon (E), O. barthii (F), O. glaberrima (G), O. nivara (H). All qRT-PCRs for a given sample (splice variants) were analyzed on the same plate under identical reaction conditions from the same cDNA batch. Data presented is the mean of three biological replicates (n = 3; with each replicate analyzed twice; each replicate had 5 pooled plants). Significance was calculated using one-way ANOVA (Student’s T-test). *P < 0.05; **P < 0.01, ***P < 0.001, ****P < 0.0001.
(1) O. sativa varieties show variability in tissue specific expression of differentially spliced NHX1 isoforms under salinity (japonica: downregulation and Pokkali: upregulation in leaf of the correctly spliced isoform; downregulation in roots of both varieties under salinity).
(2) The 13th intron retaining NHX1 isoform (i13) is upregulated in Oryza spp. leaf tissues (except Oryza sativa spp. indica cv. Pokkali and O. glaberrima) and downregulated in root tissues of Oryza spp.
(3) The 5′ UTR intron retaining NHX1 (R-NHX1) is upregulated in leaf tissues of Oryza spp. (except for O. glaberrima) and downregulated in root tissues (except in Oryza sativa spp. japonica and O. glaberrima).
(4) The 5′ UTR intron splicing NHX1 transcript (Sp-NHX1) shows downregulation in Oryza spp. under salinity in leaf tissues (exceptions are O. rufipogon and O. barthii and upregulation in root tissues (exceptions include Oryza sativa spp. indica cv. Pokkali, and O. nivara).
Discussion
Plant NHXs occur as multigene families, and recent data from Arabidopsis triple and quadruple vacuolar NHX knockouts suggests they have a central role in cellular cation homeostasis that impacts growth and development (Bassil et al., 2019). Cultivated rice, O. sativa, has a multiplicity of NHX transporters (Fukuda et al., 2011). Of these, OsNHX1 has been studied in more detail (Fukuda et al., 2004; Islam and Seraj, 2009; Biswas et al., 2015; Amin et al., 2016).
Oryza NHX1 Orthologs Show Conservation of Critical Amino Acid Residues That Are Vital for Antiporter Function and Characteristics of Type I Cation/Proton Antiporters (CPAs)
Orthologs of NHX1 in Oryza species show conservation of the Na+/H+ exchanger domain, with NHX1 sequences from AA Oryza genomes forming a distinct cluster. Eight critical residues governing the function of EcNhaA antiporter in Escherichia coli, in the “NhaA fold” (X1X2X3X4----E5XXN6D7------R8), occur clustered in the protein core. They reside on different transmembrane (TM) helices and do not occur necessarily in a continuous manner in the protein sequence (Padan and Michel, 2015; Masrati et al., 2018). These residues are highly conserved in Oryza NHX1s. The amino acid residues “ATDS” in Oryza NHX1 species form the first four positions that occur continuously (TM4 of EcNhaA) and are spaced at a distance of 20 amino acid residues from the crucial “E5XXN6D7” motif (TM5 of EcNhaA). In fungal antiporters (Zygosaccharomyces rouxii ZrSod2-22p; Saccharomyces pombe SpSod2p; S. cerevisiae ScNha1p; Candida albicans CaCnh1p; C. tropicalis CtNha1p; Pichia sorbithophila PsNha1p), the motif “ATDP” is found instead of “ATDS” seen in Oryza species; replacement of proline by serine/threonine abolishes Na+ specificity and confers a broader cation transport ability on ZrSod2-22p (Kinclova-Zimmermannova et al., 2006). Similarly, a single amino acid change (Asp) in the second pore loop domain of Thellungiella salsuginea TsHKT1;2, a class 1 HKT protein, confers strong selectivity for K+ transport over Na+; the corresponding residue in other class 1 HKT transporters is Asn (Ali et al., 2012). Based on presence of the “ATDS” motif, a broader cation substrate specificity for Oryza NHX1 proteins is predicted. Thus, OsNHX1 shows broad substrate preference and suppresses Na+, Li+, and K+ sensitivity of the yeast nhx1 mutant while O. coarctata (OcNHX1) suppresses Na+, but enhances Li+ sensitivity of a yeast nhx1 mutant (Fukuda et al., 2004; Jegadeeson et al., 2019). Within the E5XXN6D7 motif, E5 is highly conserved in type I CPAs, with N6 and D7 being vital for ion binding and translocation. The eighth residue, R8 (marked ∗) is 167 amino acid residues downstream from the E5XXN6D7 motif. It corresponds to K300 of EcNhaA (TM10), and it has been implicated in maintenance of the NhaA fold (Rimon et al., 2018). The crucial N6D7 residues have been experimentally verified as being important for transporter function in poplar NHX3 (PeNHX3) and are also conserved in AtNHX1 (Wang et al., 2014; Sze and Chanroj, 2018).
5′ Upstream Regions of Oryza NHX1 Show a Conserved Order of cis-Elements
Multiple, conserved cis-elements were predicted using the STIF algorithm in the 5′ upstream region (in DCRE and PCRe regions) of Oryza NHX1 sequences. A nucleotide sequence characteristic of a Gbox HLH binding site (DCRe) and a Nbox HLH binding motif (proximal end) is conserved in all sequences. In addition, ten NHX1 5′ upstream sequences show conservation of a distal AuxRE binding site, while nine sequences show conservation of a distal NAC TF binding region. In the PCRe, an AuxRE and a GCC_box_AP2_EREBP cis-motif are also conserved in seven Oryza species. HLH, NAC and AP2/EREBP transcription factors have well-documented roles under salinity stress (Zhang and Shi, 2013; Chen et al., 2016), while AuxRE bind Auxin Response Factors (ARFs) which are known to be induced in response to salinity (Qi et al., 2012; Bouzroud et al., 2018). The cladogram derived from the order of predicted cis-elements reveals that Oryza species with AA genomes have a distinct pattern of cis-elements that are distinct from BB, FF and KKLL genome types. In O. sativa, OsNHX1 expression is induced in response to hyper-ionic stress (NaCl, KCl) stress in both shoots and roots (Fukuda et al., 2004). Our data too suggests responsiveness to NaCl application in O. sativa and O. coarctata. However, NHXs have broader roles in plant function including cell growth, expansion, cellular trafficking in relation to environmental cues. It is worth examining this clustering of cis elements vis-à-vis tissue specific/stimulus specific gene expression.
Sequence Divergence of the 5′ UTR in Oryza Species Determines Splicing Outcomes
For the first time, in this work, we demonstrate experimentally the existence of 5′ UTR splice variants for OsNHX1 and conservation of the 5′ UTR splicing event in the NHX1 gene in three cultivated Oryza progenitor genomes (O. nivara, O. rufipogon, and O. barthii all AA genomes). Diversity in the genus Oryza arose as a consequence of two rapid speciation events that occurred approximately 13–15 million years ago (MYA), wherein the present G, F, and extant H/J/K/L Oryza genome types separated from an ancestral species that gave rise to the Oryza A, B, C genome types (Zou et al., 2013; Stein et al., 2018). The AA genomes are thought to have developed in a second burst of speciation about 2.41 MYA (Stein et al., 2018). Divergence of African cultivated rice, O. glaberrima (progenitor species, O. barthii) from Asian cultivated rice O. sativa (progenitor species, O. rufipogon and O. nivara for O. sativa ssp. indica and O. sativa ssp. japonica, respectively), occurred about 0.86 MYA, from an extinct common Oryza ancestor (Zhu et al., 2014). The presence of the 5′ UTR intron containing/splicing out variants in the African rice progenitor, O. barthii, and O. nivara and O. rufipogon (O. sativa progenitor species) suggests that the transcriptional machinery governing these splicing events has a conserved, ancient origin, dating back to at least 0.86 MYA and was probably inherited from a shared common ancestor. Further, alternative splicing events involving the 5′ UTRs of NHX1 transcripts are conserved in Oryza AA species.
The NHX1 5′ UTR regions of Oryza BB, CC and FF genome types (O. punctate, BB genome type, accession No: KC610950.1; O. officinalis, CC genome type, accession No: KC610951.1; O. brachyantha, FF genome type, accession No.:XM_006658016.2); and O. coarctata (KKLL) (accession no: JQ796375.1; Kizhakkedath et al., 2015; Supplementary Figure S11) show substantial sequence differences from the NHX1 5′ UTR regions of Oryza AA genome sequences. This may account for why we were able to detect only one transcript for OcNHX1 by RT-PCR (using 5′ UTR specific primers). Based on sequence comparisons, we predict that this splicing event is likely absent in NHX1 transcripts of Oryza BB and FF genome types and possibly other Oryza genome types as well.
Alternative Splicing Involving Retention of the 13th Intron in Oryza NHX1 Transcripts Is More Ancient in Origin Compared to the 5′ UTR Splicing Events
MSU annotation of the Os07g47100.3 (OsNHX1) transcript suggests partial retention of the 13th intron. However, the O. coarctata OcNHX1i13 transcript not only shows complete retention of the 13th intron but also the entire last is exon (confirmed by 3′ RACE-PCR; Supplementary Figure S7). Analysis of the Transcriptome Shotgun Assembly (TSA) database at NCBI suggests that transcripts showing complete retention of the 13th intron may also be present in O. sativa and O. meyeriana (GG genome type) transcriptomes [GFYC01000051.1; GFMS01046554.1 (O. sativa); GBYS01092482.1; GBYS01172993.1 (O. meyeriana)]. The 3′ splice site in 13th intron of the OcNHX1 genomic sequence is “GG” instead of the canonical “AG” seen in OsNHX1 genomic DNA (canonical AG present in the remaining NHX1 sequences). The 13th intron is the last intron seen in both OcNHX1 and OsNHX1 genomic sequences. IR appears to constitute a significant proportion of alternatively spliced transcripts observed in plants and in numerous cases is induced under abiotic stress conditions (Ner-Gaon et al., 2004; Filichkin et al., 2018). Further, in a large majority of transcripts, it is the last intron that is usually retained, similar to Os07g47100.3 and OcNHX1i13 (Ner-Gaon et al., 2004). The presence of transcripts retaining the 13th intron in Oryza AA, KKLL, and GG genome types suggests that this splicing event may have a more ancient origin that pre-dates the alternative splicing event involving the NHX1 5′ UTR in Oryza AA species.
NHX1 ORF and Transcript Diversity Generated by Alternative Splicing Across Oryza Species
Splicing usually occurs co-transcriptionally and preferential or position-specific intron retention during alternative splicing is influenced by DNA methylation in rice (Wei et al., 2018) as well as chromatin remodeling in rice and Arabidopsis (Ullah et al., 2018). Our data shows preferential tissue specific up- or downregulation of the correctly spliced NHX1 transcript as well as splice variants under salinity in Oryza AA species as well as in O. coarctata. This suggests the spliceosome machinery governing alternative splicing events involving NHX1 has tissue specific effects under salinity in Oryza species.
The splicing event involving the 5′ UTR in Oryza AA genomes does not affect ORF length but may influence transcript stability or translatability. Thus, intron inclusion in promoters of maize Adh1, Sh1, Bx1, or Act or 5′ UTRs (naturally occurring) of A. thaliana polyubiquitin and EF1-a genes enhances reporter gene expression several fold (Liu et al., 2001). 5′ UTR variants of AtZIF2 and AtPSY (phytoene synthase) resulting from alternative splicing show distinct translation efficiencies that result in differential zinc tolerance and carotogenesis respectively (Remy et al., 2014; Álvarez et al., 2016).
In plants, stress-induced retention of the last intron is a widely recognized phenomenon (Ner-Gaon et al., 2004). The intron retaining NHX1 (i13) transcript seems to be highly expressed in leaf tissues of most Oryza species (Supplementary Figure S9). Retention of the 13th intron would result in PTC of Oryza NHX1 and potentially target the transcript for NMD. However, increasing evidence suggests that intron-retaining intermediate transcripts in plants may be preferentially sequestered to escape NMD. This sequestration is reversed under specific environmental cues, triggering intron removal by the spliceosomal machinery and allowing for productive translation and is a mechanism to allow rapid protein synthesis in the absence of transcription (Boothby et al., 2013; Gohring et al., 2014; Brown et al., 2015; Filichkin et al., 2015). In poplar, there appears to exist an inverse relationship between the fully spliced and differential intron retention-harboring mRNA isoforms under abiotic stress conditions (Filichkin et al., 2018). Thus, the OcNHX1 correctly spliced cDNA and the 13th intron retaining isoform OcNHX1i13, show opposite regulation in tissues under salinity and also in leaf tissues of most Oryza species under salinity, suggesting a similar mechanism may also be operative in Oryza NHX1 transcripts.
The 11-bp insertion in the rOcNHX1 retrocopy sequence leads to premature ORF truncation. rOcNHX1 has the potential to code for a long non-coding RNA (lncRNA). Retrogenes have been reported from rice, Populus trichocarpa and Arabidopsis (Zhang et al., 2005; Zhu et al., 2009; Sakai et al., 2011). Tissue specificity of retrogenes in rice is similar to their source genes with expression between retrocopies and source genes correlating across tissues (Sakai et al., 2011). rOcNHX1 too, appears to show a tissue specific expression profile that is similar to the correctly spliced transcript under salinity in O. coarctata. rOcNHX1 adds one more layer of complexity in the post-transcriptional regulation of NHX1 in O. coarctata.
Data Availability Statement
The raw data supporting the conclusions of this article will be made available by the authors, without undue reservation, to any qualified researcher.
Author Contributions
GS and VJ carried out the bulk of this study. GS carried out the qRT-PCR. PP and RR contributed to RT-PCR analysis, cloning, and sequencing. RSS carried out bioinformatic analysis. RS, LS, KR, Z-HC, MZ, SS, and GV contributed to overall planning and design. GV and GS wrote the manuscript. All authors have read and edited the final manuscript.
Funding
This research work was carried out with funded grants provided by the Department of Biotechnology, Government of India to GV [BT/PR11396/NDB/52/118/2008; Indo-Australian Biotechnology Fund (BT/Indo-Aus/09/03/2015)].
Conflict of Interest
The authors declare that the research was conducted in the absence of any commercial or financial relationships that could be construed as a potential conflict of interest.
Acknowledgments
We thank Dr. V. Selvam of the Coastal System Research Group, MSSRF, as well as Director, CRRI, Cuttack, India and Director, NBPGR, India for the plant material used in this study and Professor Paul Holford of Western Sydney University for editing the manuscript.
Supplementary Material
The Supplementary Material for this article can be found online at: https://www.frontiersin.org/articles/10.3389/fpls.2020.00267/full#supplementary-material
Abbreviations
AS, alternative splicing; CPA, cation/proton antiporters; DCR, distal conserved region; IR, intron-retention; MSU, Michigan State University; NHX, Na+(K+)/H+ exchanger; NMD, nonsense mediated decay; ORF, Open reading Frame; PTC, premature termination codons; UTR, Un-translated Region.
Footnotes
- ^ http://plants.ensembl.org/index.html
- ^ https://www.ebi.ac.uk/Tools/hmmer/
- ^ https://www.ebi.ac.uk/Tools/msa/clustalw2/
References
Ali, Z., Park, H. C., Ali, A., Oh, D.-H., Aman, R., Kropornicka, A., et al. (2012). TsHKT1;2, a HKT1 homolog from the extremophile Arabidopsis relative Thellungiella salsuginea, shows K+ specificity in the presence of NaCl. Plant Physiol. 158, 1463–1474. doi: 10.1104/pp.111.193110
Álvarez, D., Voß, B., Maass, D., Wüst, F., Schaub, P., Beyer, P., et al. (2016). Carotenogenesis is regulated by 5′UTR-mediated translation of phytoene synthase splice variants. Plant Physiol. 172, 2314–2326. doi: 10.1104/pp.16.01262
Amin, U. S., Biswas, S., Elias, S. M., Razzaque, S., Haque, T., Malo, R., et al. (2016). Enhanced salt tolerance conferred by the complete 2.3 kb cDNA of the rice Vacuolar Na+/H+ antiporter gene compared to 1.9 kb coding region with 5. UTR in transgenic lines of rice. Front. Plant Sci. 7:14. doi: 10.3389/fpls.2016.00014
Amtmann, A., and Leigh, R. (2009). “Ion homeostasis,” in Abiotic Stress Adaptation in Plants, eds A. Pareek, S. Sopory, and H. Bohnert (Dordrecht: Springer).
Bassil, E., and Blumwald, E. (2014). The ins and outs of intracellular ion homeostasis: NHX type cation/H+ transporters. Curr. Opin. Plant Biol. 22, 1–6. doi: 10.1016/j.pbi.2014.08.002
Bassil, E., Zhang, S., Gong, H., Tajima, H., and Blumwald, E. (2019). Cation Specificity of Vacuolar NHX-Type Cation/H(+) Antiporters. Plant Physiol. 179, 616–629. doi: 10.1104/pp.18.01103
Biswas, S., Razzaque, S., Elias, S. M., Amin, U. S. M., Haque, T., Islam, S. M. T., et al. (2015). Effect of the vacuolar Na+/H+ antiporter transgene in a rice landrace and a commercial rice cultivar after its insertion by crossing. Acta Physiol. Plant. 37, 1–10.
Boothby, T. C., Richard, S. Z., van der Weele, C. M., and Stephen, M. W. (2013). Removal of retained introns regulates translation in the rapidly developing gametophyte of Marsilea vestita. Dev. Cell 24, 517–529. doi: 10.1016/j.devcel.2013.01.015
Bouzroud, S., Gouiaa, S., Hu, N., Bernadac, A., Mila, I., Bendaou, N., et al. (2018). Auxin Response Factors (ARFs) are potential mediators of auxin action in tomato response to biotic and abiotic stress (Solanum lycopersicum). PLoS One 13:e0193517. doi: 10.1371/journal.pone.0193517
Brown, J. W., Simpson, C. G., Marquez, Y., Gadd, G. M., Barta, A., and Kalyna, M. (2015). Lost in translation: pitfalls in deciphering plant alternative splicing transcripts. Plant Cell 27, 2083–2087. doi: 10.1105/tpc.15.00572
Chen, L. H., Han, J. P., Deng, X. M., Tan, S. L., Li, L. L., Li, L., et al. (2016). Expansion and stress responses of AP2/EREBP superfamily in Brachypodium distachyon. Sci. Rep. 6:21623. doi: 10.1038/srep21623
Filichkin, S., Priest, H. D., Megraw, M., and Mockler, T. C. (2015). Alternative splicing in plants: directing traffic at the crossroads of adaptation and environmental stress. Curr. Opin. Plant Biol. 24, 125–135. doi: 10.1016/j.pbi.2015.02.008
Filichkin, S. A., Hamilton, M., Dharmawardhana, P. D., Singh, S. K., Sullivan, C., Ben-Hur, A., et al. (2018). Abiotic stresses modulate landscape of poplar transcriptome via alternative splicing, differential intron retention, and isoform ratio switching. Front. Plant Sci. 9:5. doi: 10.3389/fpls.2018.00005
Fukuda, A., Nakamura, A., Hara, N., Toki, S., and Tanaka, Y. (2011). Molecular and functional analyses of rice NHX-type Na+/H+ antiporter genes. Planta 233, 175–188. doi: 10.1007/s00425-010-1289-4
Fukuda, A., Nakamura, A., Tagiri, A., Tanaka, H., Miyao, A., Hirochika, H., et al. (2004). Function, intracellular localization and the importance in salt tolerance of a vacuolar Na+/H+ antiporter from rice. Plant Cell Physiol. 45, 146–159. doi: 10.1093/pcp/pch014
Fukuda, A., Nakamura, A., and Tanaka, Y. (1999). Molecular cloning and expression of the Na+/H+ exchanger gene in Oryza sativa. Biochim. Biophys. Acta. Gene Struct. Expr. 1446, 149–155. doi: 10.1016/s0167-4781(99)00065-2
Garg, R., Verma, M., Agrawal, S., Shankar, R., Majee, M., and Jain, M. (2013). Deep transcriptome sequencing of wild halophyte rice, Porteresia coarctata, provides novel insights into the salinity and submergence tolerance factors. DNA Res. 21, 69–84. doi: 10.1093/dnares/dst042
Gohring, J., Jacak, J., and Barta, A. (2014). Imaging of endogenous messenger RNA splice variants in living cells reveals nuclear retention of transcripts inaccessible to nonsense-mediated decay in Arabidopsis. Plant Cell 26, 754–764. doi: 10.1105/tpc.113.118075
Hu, B., Jin, J., Guo, A. Y., Zhang, H., Luo, J., and Gao, G. (2015). GSDS 2.0: an upgraded gene features visualization server. Bioinformatics 31, 1296–1297. doi: 10.1093/bioinformatics/btu817
Islam, S. M. T., and Seraj, Z. I. (2009). Vacuolar Na+/H+ antiporter expression and salt tolerance conferred by actin1D and CaMV35S are similar in transgenic Binnatoa rice. Plant Tissue Cult. Biotech. 19, 257–262. doi: 10.3329/ptcb.v19i2.5444
Jegadeeson, V., Kumari, K., Pulipati, S., Parida, A., and Venkataraman, G. (2019). Expression of wild rice Porteresia coarctata PcNHX1 antiporter gene (PcNHX1) in tobacco controlled by PcNHX1 promoter (PcNHX1p) confers Na+-specific hypocotyl elongation and stem-specific Na+ accumulation in transgenic tobacco. Plant Physiol. Biochem. 39, 161–170. doi: 10.1016/j.plaphy.2019.03.014
Jung, K. H., Bartley, L. E., Cao, P., Canlas, P. E., and Ronald, P. C. (2009). Analysis of alternatively spliced rice transcripts using microarray data. Rice 2, 44–55. doi: 10.14348/molcells.2017.2297
Kinclova-Zimmermannova, O., Zavrel, M., and Sychrova, H. (2006). Importance of the seryl and threonyl residues of the fifth transmembrane domain to the substrate specificity of yeast plasma membrane Na+/H+ antiporters. Mol. Membr. Biol. 23, 349–361. doi: 10.1080/09687860600738908
Kizhakkedath, P., Jegadeeson, V., Venkataraman, G., and Parida, A. (2015). A vacuolar antiporter is differentially regulated in leaves and roots of the halophytic wild rice Porteresia coarctata (Roxb.) Tateoka. Mol. Biol. Rep. 42, 1091–1105. doi: 10.1007/s11033-014-3848-4
Kumar, S., Stecher, G., and Tamura, K. (2016). MEGA7: molecular evolutionary genetics analysis version 7.0 for bigger datasets. Mol. Biol. Evo. 33, 1870–1874. doi: 10.1093/molbev/msw054
Liu, Q., Brubaker, C. L., Green, A. G., Marshall, D. R., Sharp, P. J., and Singh, S. P. (2001). Evolution of the FAD2-1 fatty acid desaturase 5′ UTR intron and the molecular systematics of Gossypium (Malvaceae). Am. J. Bot. 88, 92–102.
Martinoia, E., Maeshima, M., and Neuhaus, H. E. (2007). Vacuolar transporters and their essential role in plant metabolism. J. Exp. Bot. 58, 83–102. doi: 10.1093/jxb/erl183
Maser, P., Thomine, S., Schroeder, J. I., Ward, J. M., Hirschi, K., Sze, H., et al. (2001). Phylogenetic relationships within cation transporter families of Arabidopsis. Plant Physiol. 126, 1646–1667. doi: 10.1104/pp.126.4.1646
Masrati, G., Dwivedi, M., Rimon, A., Gluck-Margolin, Y., Kessel, A., Ashkenazy, H., et al. (2018). Broad phylogenetic analysis of cation/proton antiporters reveals transport determinants. Nat. Commun. 9:4205. doi: 10.1038/s41467-018-06770-5
Mei, W., Liu, S., Schnable, J. C., Yeh, C. T., Springer, N. M., Schnable, P. S., et al. (2017). A comprehensive analysis of alternative splicing in paleopolyploid maize. Front. Plant Sci. 8:694. doi: 10.3389/fpls.2017.00694
Murashige, T., and Skoog, F. (1962). A revised medium for rapid growth and bioassays with tobacco tissue cultures. Physiol. Plant 15, 473–497. doi: 10.1111/j.1399-3054.1962.tb08052.x
Naika, M., Shameer, K., Mathew, O. K., Gowda, R., and Sowdhamini, R. (2013). STIFDB2: an updated version of plant stress-responsive transcription factor database with additional stress signals, stress-responsive transcription factor binding sites and stress-responsive genes in Arabidopsis and rice. Plant Cell Physiol. 54:e8. doi: 10.1093/pcp/pcs185
Ner-Gaon, H., Halachmi, R., Savaldi-Goldstein, S., Rubin, E., Ophir, R., and Fluhr, R. (2004). Intron retention is a major phenomenon in alternative splicing in Arabidopsis. Plant J. 39, 877–885. doi: 10.1111/j.1365-313x.2004.02172.x
Nicholson, P., Yepiskoposyan, H., Metze, S., Zamudio Orozco, R., Kleinschmidt, N., and Mühlemann, O. (2010). Nonsense-mediated mRNA decay in human cells: mechanistic insights, functions beyond quality control and the double-life of NMD factors. Cell Mol. Life Sci. 67, 677–700. doi: 10.1007/s00018-009-0177-1
Padan, E., and Michel, H. (2015). NhaA: a unique structural fold of secondary active transporters. Isr. J. Chem. 55, 1233–1239. doi: 10.1002/ijch.201500044
Qi, Y., Wang, S., Shen, C., Zhang, S., Chen, Y., Xu, Y., et al. (2012). OsARF12, a transcription activator on auxin response gene, regulates root elongation and affects iron accumulation in rice (Oryza sativa). New Phytol. 193, 109–120. doi: 10.1111/j.1469-8137.2011.03910.x
Reguera, M., Bassil, E., Tajima, H., Wimmer, M., Chanoca, A., Otegui, M. S., et al. (2015). pH regulation by NHX-type antiporters is required for receptor-mediated protein trafficking to the vacuole in Arabidopsis. Plant Cell 27, 1200–1217. doi: 10.1105/tpc.114.135699
Remy, E., Cabrito, T. R., Batista, R. A., Hussein, M. A., Teixeira, M. C., Athanasiadis, A., et al. (2014). Intron retention in the 5′ UTR of the novel ZIF2 transporter enhances translation to promote zinc tolerance in Arabidopsis. PLoS Genet. 10:e1004375. doi: 10.1371/journal.pgen.1004375
Rimon, A., Dwivedi, M., Friedler, A., and Padan, E. (2018). Asp133 Residue in NhaA Na(+)/H(+) antiporter is required for stability cation binding and transport. J. Mol. Biol. 430, 867–880. doi: 10.1016/j.jmb.2018.01.014
Sakai, H., Mizuno, H., Kawahara, Y., Wakimoto, H., Ikawa, H., Kawahigashi, H., et al. (2011). Retrogenes in rice (Oryza sativa L. ssp. japonica) exhibit correlated expression with their source genes. Genome Biol. Evol. 3, 1357–1368. doi: 10.1093/gbe/evr111
Shi, H., Quintero, F. J., Pardo, J. M., and Zhu, J. K. (2002). The putative plasma membrane Na+/H+ antiporter SOS1 controls long-distance Na+ transport in plants. Plant Cell 14, 465–477. doi: 10.1105/tpc.010371
Stein, J. C., Yu, Y., Copetti, D., Zwickl, D. J., Zhang, L., Zhang, C., et al. (2018). Genomes of 13 domesticated and wild rice relatives highlight genetic conservation, turnover and innovation across the genus Oryza. Nat. Genet. 50, 285–296. doi: 10.1038/s41588-018-0040-0
Sze, H., and Chanroj, S. (2018). Plant endomembrane dynamics: studies of K(+)/H(+) antiporters provide insights on the effects of pH and ion homeostasis. Plant Physiol. 177, 875–895. doi: 10.1104/pp.18.00142
Ullah, F., Hamilton, M., Reddy, A. S. N., and Ben-Hur, A. (2018). Exploring the relationship between intron retention and chromatin accessibility in plants. BMC Genomics 19:21. doi: 10.1186/s12864-017-4393-z
Wang, L., Feng, X., Zhao, H., Wang, L., An, L., and Qiu, Q.-S. (2014). Functional analysis of the Na+, K+/H+ Antiporter PeNHX3 from the tree halophyte Populus euphratica in yeast by model-guided mutagenesis. PLoS ONE 9:e104147. doi: 10.1371/journal.pone.0104147
Wei, G., Liu, K., Shen, T., Shi, J., Liu, B., Han, M., et al. (2018). Position-specific intron retention is mediated by the histone methyltransferase SDG725. BMC Biol. 16:44. doi: 10.1186/s12915-018-0513-8
Yoshida, S., Forno, D. A., Cock, J. H., and Gomez, K. A. (1976). Laboratory Manual for Physiological Studies of Rice. Los Baños: Philippines IRRI, 83.
Zhang, C., Yang, H., and Yang, H. (2015). Evolutionary character of alternative splicing in plants. Bioinform Biol Insights. 9, 47–52. doi: 10.4137/BBI.S33716
Zhang, J. L., and Shi, H. (2013). Physiological and molecular mechanisms of plant salt tolerance. Photosynth. Res. 115, 1–22. doi: 10.1007/s11120-013-9813-6
Zhang, Y., Wu, Y., Liu, Y., and Han, B. (2005). Computational identification of 69 retroposons in Arabidopsis. Plant Physiol. 138, 935–948. doi: 10.1104/pp.105.060244
Zhu, T., Xu, P. Z., Liu, J. P., Peng, S., Mo, X. C., and Gao, L. Z. (2014). Phylogenetic relationships and genome divergence among the AA- genome species of the genus Oryza as revealed by 53 nuclear genes and 16 intergenic regions. Mol. Phylogenet Evol. 70, 348–361. doi: 10.1016/j.ympev.2013.10.008
Zhu, Z., Zhang, Y., and Long, M. (2009). Extensive structural renovation of retrogenes in the evolution of the Populus genome. Plant Physiol. 151, 1943–1951. doi: 10.1104/pp.109.142984
Keywords: Oryza, NHX1, alternative splicing, intron retention, evolutionary origin
Citation: Sellamuthu G, Jegadeeson V, Sajeevan RS, Rajakani R, Parthasarathy P, Raju K, Shabala L, Chen Z-H, Zhou M, Sowdhamini R, Shabala S and Venkataraman G (2020) Distinct Evolutionary Origins of Intron Retention Splicing Events in NHX1 Antiporter Transcripts Relate to Sequence Specific Distinctions in Oryza Species. Front. Plant Sci. 11:267. doi: 10.3389/fpls.2020.00267
Received: 23 September 2019; Accepted: 20 February 2020;
Published: 11 March 2020.
Edited by:
Sonia Negrao, University College Dublin, IrelandReviewed by:
Mingsheng Chen, Institute of Genetics and Developmental Biology, Chinese Academy of Sciences, ChinaArun Lahiri Majumder, Bose Institute, India
Sabrina Moriom Elias, University of Dhaka, Bangladesh
Copyright © 2020 Sellamuthu, Jegadeeson, Sajeevan, Rajakani, Parthasarathy, Raju, Shabala, Chen, Zhou, Sowdhamini, Shabala and Venkataraman. This is an open-access article distributed under the terms of the Creative Commons Attribution License (CC BY). The use, distribution or reproduction in other forums is permitted, provided the original author(s) and the copyright owner(s) are credited and that the original publication in this journal is cited, in accordance with accepted academic practice. No use, distribution or reproduction is permitted which does not comply with these terms.
*Correspondence: Gayatri Venkataraman, Z2F5YXRyaUBtc3NyZi5yZXMuaW4=; Z2F5YXRyaS5wYXRhY2FrZS52ZW5rYXRhcmFtYW5AZ21haWwuY29t
†These authors have contributed equally to this work