- 1Department of Plant Pathology, ESALQ, University of São Paulo, Piracicaba, Brazil
- 2Department of Crop Sciences, Institute of Applied Plant Nutrition, University of Göttingen, Göttingen, Germany
- 3Department of Plant Biology, Institute of Biology, University of Campinas, Campinas, Brazil
- 4Department of Crop Sciences, Division of Plant Pathology and Crop Protection, University of Göttingen, Göttingen, Germany
Downy mildew caused by Plasmopara viticola is one of the most destructive diseases of Vitis vinifera worldwide. Grapevine breeding programs have introgressed P. viticola-resistant traits into cultivated V. vinifera genotypes and launched interspecific hybrids with resistance against downy mildew. In general, pathogen infection affects primary metabolism, reduces plant growth and development and modifies the secondary metabolism toward defense responses, which are costly in terms of carbon production and utilization. The objective of this work was to evaluate the photosynthesis impairment by inducible defenses at the leaf level in V. vinifera cultivars resistant to P. viticola. Photosynthetic limitations imposed by P. viticola in susceptible and resistant grapevine cultivars were evaluated. Histochemical localization of hydrogen peroxide and superoxide and the activity of ascorbate peroxidase were assessed. Measurements of leaf gas exchange, chlorophyll fluorescence and the response of leaf CO2 assimilation to increasing air CO2 concentrations were taken, and photosynthetic limitations determined in cultivars Solaris (resistant) and Riesling (susceptible). The net photosynthetic rates were reduced (−25%) in inoculated Solaris plants even before the appearance of cell death-like hypersensitive reactions (“HR”). One day after “HR” visualization, the net photosynthetic rate of Solaris was reduced by 57% compared with healthy plants. A similar pattern was noticed in resistant Cabernet Blanc and Phoenix plants. While the susceptible cultivars did not show any variation in leaf gas exchange before the appearance of visual symptoms, drastic reductions in net photosynthetic rate and stomatal conductance were found in diseased plants 12 days after inoculation. Decreases in the maximum Rubisco carboxylation rate and photochemical impairment were noticed in Riesling after inoculation with P. viticola, which were not found in Solaris. Damage to the photochemical reactions of photosynthesis was likely associated with the oxidative burst found in resistant cultivars within the first 24 h after inoculation. Both chlorophyll degradation and stomatal closure were also noticed in the incompatible interaction. Taken together, our data clearly revealed that the defense response against P. viticola causes a photosynthetic cost to grapevines, which is not reversible even 12 days after the pathogen infection.
Introduction
Downy mildew, caused by the biotrophic oomycete Plasmopara viticola, is one of the most devastating diseases of grapevine. P. viticola is native to North America and was considered to be of minor importance on Vitis aestivalis, in which it was first described in 1834 (Wilcox et al., 2015). However, this pathogen was inadvertently introduced into Europe in 1878 and now it is endemic in the main grapevine growing regions worldwide (Gessler et al., 2011). The European grapevine (Vitis vinifera) evolved in the absence of this disease and it is extremely susceptible to P. viticola. After P. viticola introduction the pathogen quickly spread throughout the European vineyards (Rossi et al., 2013). P. viticola affects grapevine leaves, inflorescences, berries and shoots with high disease severity causing defoliation and producing low-quality, unsightly or entirely damaged grapes. The pathogen can also cause weakening, dwarfing and death of young shoots (Agrios, 2005). When the weather is conducive to the disease and in the absence of effective disease control, downy mildew can easily reduce the grapevine yield up to 100% (Agrios, 2005; Wilcox et al., 2015). P. viticola zoospores penetrate the host through stomata and develop intercellular mycelium in the mesophyll of grapevine leaves, generating globose haustoria responsible for the oomycete nutrition. After infection and colonization periods, sporangiophores and sporangia emerge from the stomata (Ash, 2000; Agrios, 2005; Gessler et al., 2011; Kamoun et al., 2015; Armijo et al., 2016). Currently, downy mildew is of paramount importance in all humid parts of the world where grapevine is grown. In subtropical regions of South America, the occurrence of rainy periods at the time of budbreak and flowering can lead to severe epidemics of downy mildew. Consequently, high frequency of fungicide sprays is necessary to control downy mildew during the rainy season, i.e., from summer to autumn (Cappello et al., 2017).
Although European V. vinifera cultivars are highly susceptible to downy mildew, Muscadinia species and several American and Asian Vitis species exhibit varying levels of resistance to P. viticola. By the conventional breeding, V. vinifera has been crossed with grapevines showing resistance against P. viticola, and resistant interspecific hybrids have been subsequently found. In resistant accessions obtained by crosses with North American Vitis species, P. viticola completes its life cycle and releases less sporangia than on susceptible cultivars (Bellin et al., 2009). The genomic regions conferring resistance to downy mildew referred to as resistance to P. viticola (Rpv) are quantitatively inherited. To date, 27 quantitative trait loci (QTLs) with major effects on downy mildew resistance are known and described (Fischer et al., 2004; Welter et al., 2007; Bellin et al., 2009; Marguerit et al., 2009; Vezzulli et al., 2019). Rpv1 and Rpv2 are responsible for the resistance derived from Muscadinia rotundifolia (Peressotti et al., 2010) and map to chromosomes 12 and 18, respectively (Merdinoglu et al., 2003; Welter et al., 2007). The locus Rpv3, which was first described in cultivars Regent and Bianca, came from the American wild Vitis species and is located on chromosome 18 (Fischer et al., 2004; Welter et al., 2007; Bellin et al., 2009). The Asian resistance loci Rpv8, Rpv10, and Rpv12 originated from Vitis amurensis. Rpv8 and Rpv12 map to chromosome 14 (Venuti et al., 2013) and Rpv10 maps to chromosome 9 and is present in cultivar Solaris (Blasi et al., 2011; Schwander et al., 2012; Venuti et al., 2013). The table of loci traits in grapevine relevant for breeding and genetics with a complete description of associated markers, their chromosomal localization, and the donor genotype/species of P. viticola resistance is available online (www.vivc.de/loci).
The mechanisms by which grapevine cells reduce downy mildew intensity are complex and not fully elucidated (Kortekamp, 2006; Liu et al., 2015). These mechanisms comprise pre-existing chemical defenses and inducible structural and biochemical defenses. High constitutive levels of antimicrobial compounds, such as inositol and caffeic acid, are observed in healthy leaves of resistant cultivars (Figueiredo et al., 2008). The induced structural defenses include formation of callose (Kortekamp et al., 1997; Gindro et al., 2003) and lignification (Dai et al., 1995). The accumulation of stilbenes and pathogenesis-related proteins (Kortekamp, 2006; Richter et al., 2006; Slaughter et al., 2008), the generation of reactive oxygen species, the induction of peroxidases (Kortekamp and Zyprian, 2003), and finally, cell death-like hypersensitive reactions (“HR”) represent induced biochemical defenses found in grapevine resistant cultivars after inoculation with P. viticola (Díez-Navajas et al., 2008).
Pathogen infection in incompatible interactions may affect the plant primary and also the secondary metabolism due to the induction of defenses (Berger et al., 2007). In the incompatible interaction of Phytophthora nicotianae and Nicotiana tabacum, photosynthesis is switched off few hours after pathogen inoculation, and defense mechanisms are initiated. This metabolic shift is related to an early blockage of intercellular sugar transport by callose deposition and increases in apoplastic invertase activity (Scharte et al., 2005). Both constitutive and inducible defenses are costly in terms of carbohydrate production and utilization. However, inducible systems seem to be less costly than constitutive systems in the absence of pathogens because inducible defenses require allocation of both energy and resources away from growth and reproduction (Cipollini, 1998; DeWitt et al., 1998; Purrington, 2000; Heil and Baldwin, 2002). The cost of inducible defenses is a widely discussed topic among breeders and pathologists and there are few papers conveying a significant piece of novel information on the drawbacks of plant disease resistance. The development of new resistant varieties to P. viticola is a promising way to control downy mildew and the better understanding of the effect of P. viticola in the leaf carbon assimilation of resistant plants is an important issue for the scientific community interested in grapevine-pathogen interaction.
Plasmopara viticola affects the photosynthesis of susceptible grapevine cultivars by reducing the diffusion of CO2 in leaf mesophyll (Jermini et al., 2010; Nogueira Júnior et al., 2019). In diseased plants, the concentration of photosynthetic pigments in areas nearby lesions and the primary photochemistry are reduced (Moriondo et al., 2005). Reductions and abundance in Rubisco activity have also been reported in susceptible cultivars of V. vinifera and Vitis labrusca infected by P. viticola (Gamm et al., 2011; Nogueira Júnior et al., 2019). However, the effect of downy mildew on photosynthetic capacity and leaf gas-exchange for resistant cultivars has not been characterized. Here, alterations in gas exchange, chlorophyll fluorescence and production of oxygen reactive species of leaves from cultivars with different resistance levels to downy mildew were examined. The objective of this work was to estimate the impairment of photosynthesis caused by inducible responses to the infection of P. viticola on downy mildew resistant cultivars and to elucidate the underlying limitations imposed by P. viticola to the photosynthesis of resistant and susceptible cultivars. The oxidative burst induced by P. viticola was also evaluated and related to the photosynthetic limitations in resistant cultivars.
Materials and Methods
The characterization of photosynthetic cost associated with inducible defenses to P. viticola in grapevine was performed in three steps. The first step, which is described in section “Gas Exchange Variables in Cultivars Resistant and Susceptible to Downy Mildew,” aimed to evaluate gas exchange in grapevine leaves of different cultivars, resistant and susceptible to downy mildew. The resistant cultivars Solaris, Cabernet Blanc and Phoenix and the susceptible cultivars Riesling, Niagara Rosada, Merlot and Moscato were used in this step. For the second step two representative cultivars, one resistant (Solaris) and one susceptible (Riesling) to P. viticola were selected. This step aimed to evaluate the diffusive, photochemical and biochemical limitations imposed by P. viticola on grapevine photosynthesis. The experiment at the last step aimed to characterize some induced responses in the cultivar Solaris and to correlate these responses with the early decrease on photosynthesis of leaves challenged with P. viticola.
Plant Material and Inoculum Maintenance
All experiments were performed with potted grapevine plants using the downy mildew resistant cultivars of V. vinifera Solaris, Cabernet Blanc and Phoenix; the susceptible cultivars Riesling, Merlot, Moscato; and the susceptible cultivar Niagara Rosada (V. labrusca). The cuttings were grown in pots containing sterilized substrate (manure, clay soil and sand at a ratio of 3:3:1) under greenhouse conditions where the average air temperature was 25 ± 5°C (error SEM), the RH was 60% and the photosynthetic active radiation (PAR) was 500 μmol m–2 s–1 with a 12-h photoperiod. The substrate was fertilized with 3 g of Osmocote® per gram of soil. After budbreak, the plants were conducted in a single stem and top pruned when they presented 6–7 fully expanded leaves. The plants received 200 mL of water daily and were fertilized monthly with 10 g of Hakaphos® Blau.
Leaves with symptoms of downy mildew were collected in vineyards, and sporangia of P. viticola were harvested, dried at room temperature and maintained at −25°C in Eppendorf tubes. Sporangia were rehydrated in 50 mL of bidistilled water and suspensions of the inoculum at a concentration of 104 sporangia mL–1 were obtained using a Neubauer chamber. One month after budbreak, potted plants of cv. Merlot were inoculated by spraying inoculum suspension in the abaxial side of leaves up to runoff. Immediately after the inoculation, plants were kept in an incubation chamber at 25°C and covered with a black plastic bag to keep leaves in darkness and high air relative humidity (>90%) for 24 h. Three drops of 50 μL each of the sporangia suspension used for inoculation were placed in a polystyrene dish and kept in the same incubation chamber of inoculated plants to evaluate the viability of sporangia. Viability of sporangia was determined by the observation at light microscope of the opened sporangium 24 h after incubation. Afterward, the plastic bags were removed and plants were kept in the same incubation chamber with a 12-h photoperiod and PAR of 400 μmol m–2 s–1. The inoculum production was carried out by successive inoculations of P. viticola suspensions into new healthy potted plants every month.
Gas Exchange Variables in Cultivars Resistant and Susceptible to Downy Mildew
Seven experiments were performed, one per cultivar, with the three resistant and the four susceptible cultivars. Five plants of each cultivar were inoculated as described above and kept at humid chamber for 12 h. As a control, five plants were sprayed with distilled water. The experiments were performed in the full randomized design. Net photosynthetic rate (A), stomatal conductance (gs), intercellular CO2 concentration (Ci) and transpiration (E) were measured on the inoculation day (day 0) and 1, 4, and 12 days after the inoculation (dai) of P. viticola in all resistant cultivars, except for cv. Solaris in which the gas exchange was evaluated at 0, 1, 2, 5, and 12 dai. In the susceptible cultivars the gas exchange variables were measured at 0, 1, 2, 6, and 12 dai. The instantaneous carboxylation efficiency (k) was estimated as A/Ci (Machado et al., 2005) in each measurement of leaf gas exchange. The evaluations were performed with portable infrared gas analyzers (GFS3000, Heinz Walz GmbH, Germany; and Li-6400-XT, LI-COR Inc., United States) equipped with fluorometers (PAM 3055 and 6400-40, respectively). Cuvette temperature was set to 25°C and relative humidity was 60% at the inlet of the cuvette. The cuvette sizes were 2 and 4 cm2, respectively for Li-6400-XT and GFS3000. CO2 concentration was kept constant at 400 μmol mol−1. A LED array provided PAR of 1000 μmol m–2 s–1. A, gs, Ci and E were recorded after fluxes had stabilized. The leaf areas evaluated for gas exchange were photographed at 4 and 12 dai and digital images were processed with Quant software (Vale et al., 2001) to estimate downy mildew severity. All experiments, except for cv. Phoenix, were repeated once. The average values of photosynthetic variables measured in healthy and diseased plants were compared by Student’s t-test (p ≤ 0.05) using STATISTICA 6.0 software (StatSoft Inc., Tulsa, OK, United States). The normal distribution of data was verified by the Shapiro-Wilk’s test and transformations were performed when necessary (Supplementary Table S1).
Photosynthetic and Photochemical Evaluations in Solaris and Riesling Cultivars
The photosynthetic limitations caused by P. viticola were evaluated in the grapevine cultivars Solaris and Riesling, which are resistant and susceptible to downy mildew, respectively. Three plants of each cultivar were inoculated, and three plants were sprayed with water as described previously. Six evaluations were performed in each experiment from the 5th day after inoculation onward (twice a day) using the GFS3000 (Heinz Walz, GmbH, Germany) equipped with a fluorometer (PAM 3055). Leaf CO2 assimilation response to increasing air CO2 concentration (Ca) was initially evaluated at 400 μmol mol–1, which was gradually changed to 250, 200, 150, and 50 μmol mol–1 and then gradually increased to 400, 600, 800, 1400, and 2000 μmol mol–1. Day respiration (Rd) was obtained through a linear regression between A (response variable) and Ci (predictor variable) under Ca <400 μmol mol–1 and corresponds to A when Ci is zero. Mesophyll conductance (gm) was estimated as follows (Flexas et al., 2007):
where Γ∗ is the photosynthetic compensation point, i.e., the CO2 concentration at which the photorespiratory efflux of CO2 is equal to the CO2 photosynthetic assimilation rate; and J is the transport of electrons from chlorophyll fluorescence assessments. The calculated values of gm were used to convert A-Ci curves into A-Cc (Cc is CO2 concentration at carboxylation sites in chloroplasts) curves using the following equation (Flexas et al., 2007):
From A-Cc curves, the maximum Rubisco carboxylation rate (Vcmax) and maximum rate of electron transport driving regeneration of ribulose-1,5-bisphosphate (Jmax) were estimated as follows (Farquhar et al., 1980; Flexas et al., 2007):
where Kc and Ko are the Michaelis-Menten constants of Rubisco for carboxylation and oxygenation (Bernacchi et al., 2003), respectively, and O is the internal O2 concentration, which is considered equal to the external O2 concentration. Vcmax and Jmax were estimated by non-linear regressions using STATISTICA 6.0 software (StatSoft Inc., Tulsa, OK, United States).
The maximum leaf CO2 assimilation (Amax) and the maximum stomatal conductance (gsmax) were also obtained in each A-Cc curve. The experiment was performed twice and the average values of photosynthetic variables measured in healthy and diseased plants were compared using Student’s t-test (p ≤ 0.05).
Detection and Quantification of Chlorophyll a Fluorescence, H2O2, O2– and APX Activity
Chlorophyll a fluorescence was measured using an Imaging-PAM Maxi (Heinz Walz GmbH, Germany) in cv. Solaris. The measurements were performed 6, 12, 30, 60, 120, and 288 h after P. viticola inoculation (hai). After dark-adapting leaves for 20 min, a saturation light pulse of 2700 μmol m–2s–1 was applied and the maximum PSII quantum yield [Fv/Fm = (Fm−Fo)/Fm] was measured (Maxwell and Johnson, 2000). Fm and Fo denote the maximum and minimum fluorescence of dark-adapted samples, respectively.
The detection of H2O2 and O2– was performed in cvs. Solaris and Riesling and its quantification was only performed where H2O2 was detected. The samples were collected at 6, 12, 30, 60, 120, and 288 hai. For quantification of H2O2, five leaf discs of 0.46 cm2 were removed with a cork borer approximately 2 cm away from the central vein of healthy and inoculated leaves. All five discs were immediately transferred to 1 mL of acetone acidified with 25 mM H2SO4 and frozen in liquid nitrogen, where they remained until measurements. For measurements, 50 μL of thawed samples were added to 1 mL of ferrous ammonium sulfate xylenol orange (FOX) solution that contained 250 μM ferrous ammonium sulfate, 100 mM sorbitol, 100 μM xylenol orange and 25 mM H2SO4. The samples were then incubated at room temperature for 30 to 45 min. H2O2 concentration was determined at 550 nm (EPOCH, BioTec, United States) and 850 nm (8453 UV–vis Spectroscopy System, Agilent, United States) using a standard curve ranging from 0 to 100 μM H2O2 (Wolff, 1994).
Leaf samples (0.5 g) were harvested and immediately frozen in liquid nitrogen. To assess ascorbate peroxidase (APX) activity, samples were homogenized in 5 mL phosphate buffer (pH 7.6) including 1% polyvinylpyrrolidone (PVP) and 0.1 mM EDTA and centrifuged for 20 min at 16,000 g at 4°C. The supernatant was collected and used as crude extract in the reaction mixtures of the enzyme activity assays. For the APX assay, the 0.3-mL reaction mixture contained 0.5 mM ascorbic acid, 50 mM phosphate buffer, 1 mM EDTA, 0.5 mM H2O and 10–15 μL of the supernatant. The reaction was started by adding 10 μL of 15 mM of H2O2, and APX was assayed spectrometrically (EPOCH, BioTec, United States/8453 UV-VIS Spectroscopy System, Agilent, United States) following the decrease in absorbance at 290 nm (Nakano and Asada, 1981).
Histochemical detection of H2O2 and O2– was performed on leaf discs (3 cm2) removed from the same leaves used for the estimation of H2O2 concentration. The samples were collected at 6, 12, 30, 60, 120, and 288 hai from each plant of each treatment. The samples were placed in glass vials containing 3,3′−diaminobenzidine tetrahydrochloride (DAB), pH 5.5, 1%; Sigma−Aldrich, or nitroblue tetrazolium (NBT), pH 6, 0.1%; Sigma−Aldrich, and kept at 25°C for 2 h in darkness (Thordal-Christensen et al., 1997). After this period, the samples were cleared in 80% ethanol for 24 h, stored in glycerol solution (70%), and then observed with an optical microscope. The average values of H2O2 concentrations measured in healthy and diseased plants were compared by Student’s t-test (p ≤ 0.05).
Results
Differential Gas Exchange Responses of Resistant and Susceptible Grapevine Cultivars to Plasmopara viticola Infection
The death-like hypersensitive reactions “HR” were observed 4 dai with P. viticola on the resistant cultivars Solaris, Cabernet Blanc and Phoenix (Supplementary Figure S1). In the susceptible cultivars, the symptoms of downy mildew and P. viticola sporulation were observed 6 to 7 dai. The visual area affected by “HR” did not change for cvs. Cabernet Blanc and Phoenix after 4 dai, and a slow progression (from 5.9% at 4 dai to 10.2% at 12 dai) was exclusively noticed in cv. Solaris. After 12 dai, necrotic areas accounted for 2.3 and 3.3% (on average) of Cabernet Blanc and Phoenix, respectively (Supplementary Figures S1, S2). Typical symptoms of downy mildew, i.e., oil spots on the adaxial leaf surface and abundant sporulation of P. viticola on the abaxial leaf surface (Supplementary Figure S2), were observed on all susceptible cultivars. Disease severity reached 47, 53, 46, and 34% in Riesling, Niagara Rosada, Merlot and Moscato, respectively.
On the day of inoculation with P. viticola, photosynthetic rates were similar for inoculated and non-inoculated leaves of resistant cultivars Solaris, Cabernet Blanc and Phoenix (Figure 1). However, significant reductions of leaf photosynthesis (A) were found at 2 dai in Solaris (Figure 1B) and at 1 dai for Cabernet Blanc and Phoenix (Figures 1D,F). At the appearance of “HR” and compared with healthy leaves, A values were 54, 35, and 77% lower on diseased leaves of Solaris, Cabernet Blanc, and Phoenix, respectively (Figures 1B,D,F). Then, the A values for inoculated leaves did not change significantly until 12 dai.
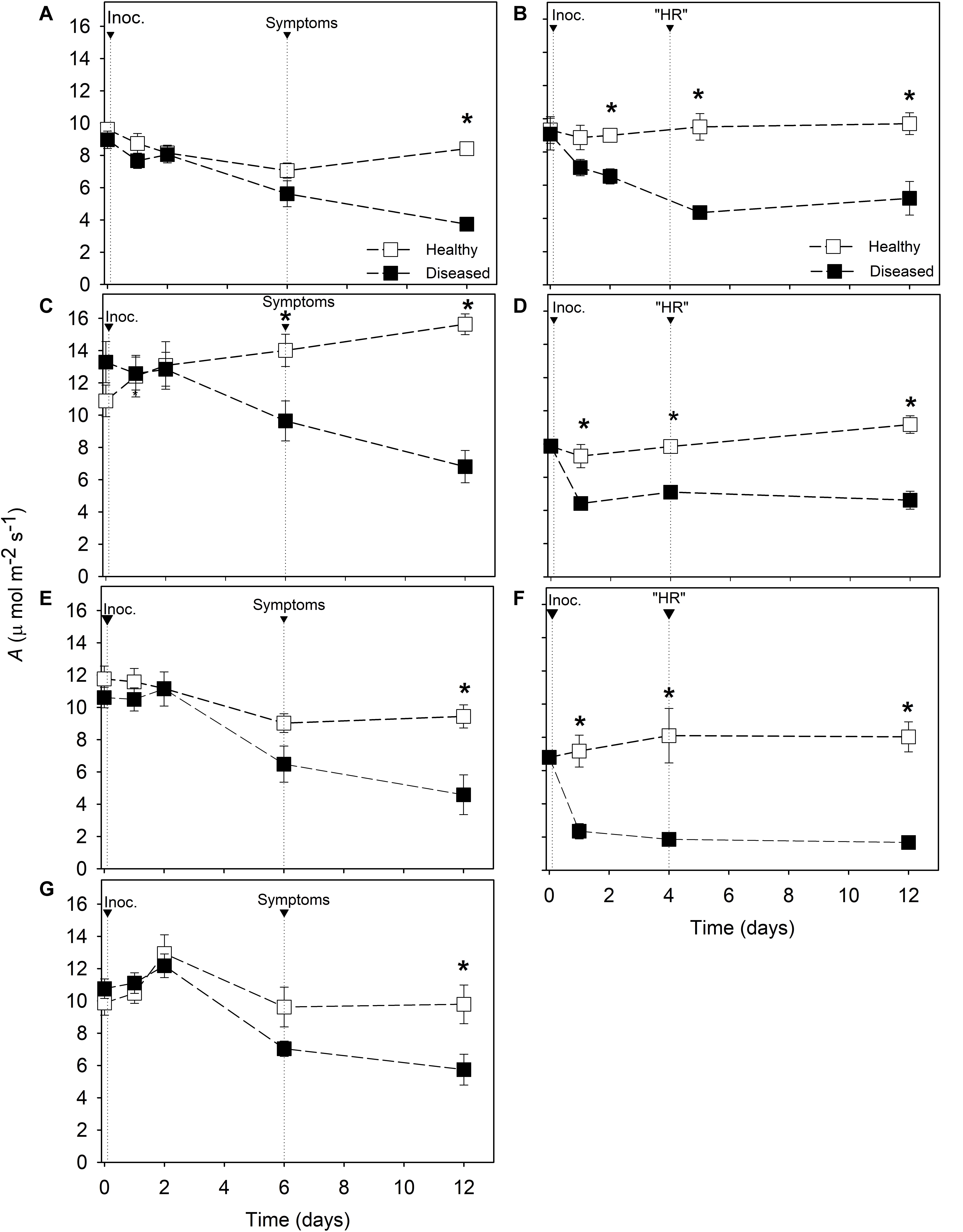
Figure 1. Net photosynthetic rate (A) in Vitis spp. cultivars. Downy mildew symptoms were observed in Riesling (A), Niagara Rosada (C), Merlot (E) and Moscato (G) 6 days after inoculation. Cell death-like hypersensitive reactions “HR” were observed in Solaris (B), Cabernet Blanc (D) and Phoenix (F) 4 days after inoculation (Inoc.). The average values of healthy and diseased leaves were compared using the Student’s t test for each cultivar (n = 6, ± SE), and * indicates significant differences (p < 0.05).
Photosynthetic rates ranged from 8.9 to 13.3 μmol m–2 s–1 on susceptible cultivars at the day of inoculation when no differences were found between inoculated and non-inoculated leaves (Figures 1A,C,E,G). When visual symptoms appeared, significant reduction of A was only observed in Niagara Rosada (Figure 1C). After 12 days of inoculation, A values on inoculated leaves were reduced by 56, 56, 51, and 41% compared with healthy leaves of Riesling, Niagara Rosada, Merlot, and Moscato, respectively.
No differences in Ci of inoculated and non-inoculated leaves were found before the appearance of “HR” in resistant cultivars (Figure 2), and this situation persisted until 12 dai for Cabernet Blanc and Phoenix. A slight increase (10.5%) was detected on Ci of inoculated leaves of Solaris 12 dai compared with non-inoculated leaves (Figure 2B). Similar Ci values were measured on inoculated and non-inoculated leaves of susceptible cultivars until symptoms appearance (Figures 2A,C,E,G). From this time, diseased leaves of Riesling and Niagara Rosada showed increases in Ci values of 14% compared with healthy leaves (Figures 2A,C). Similar to Cabernet Blanc and Phoenix (Figures 2D,F), Ci of Merlot and Moscato remained stable from 6 to 12 dai regardless of the treatment (Figures 2E,G).
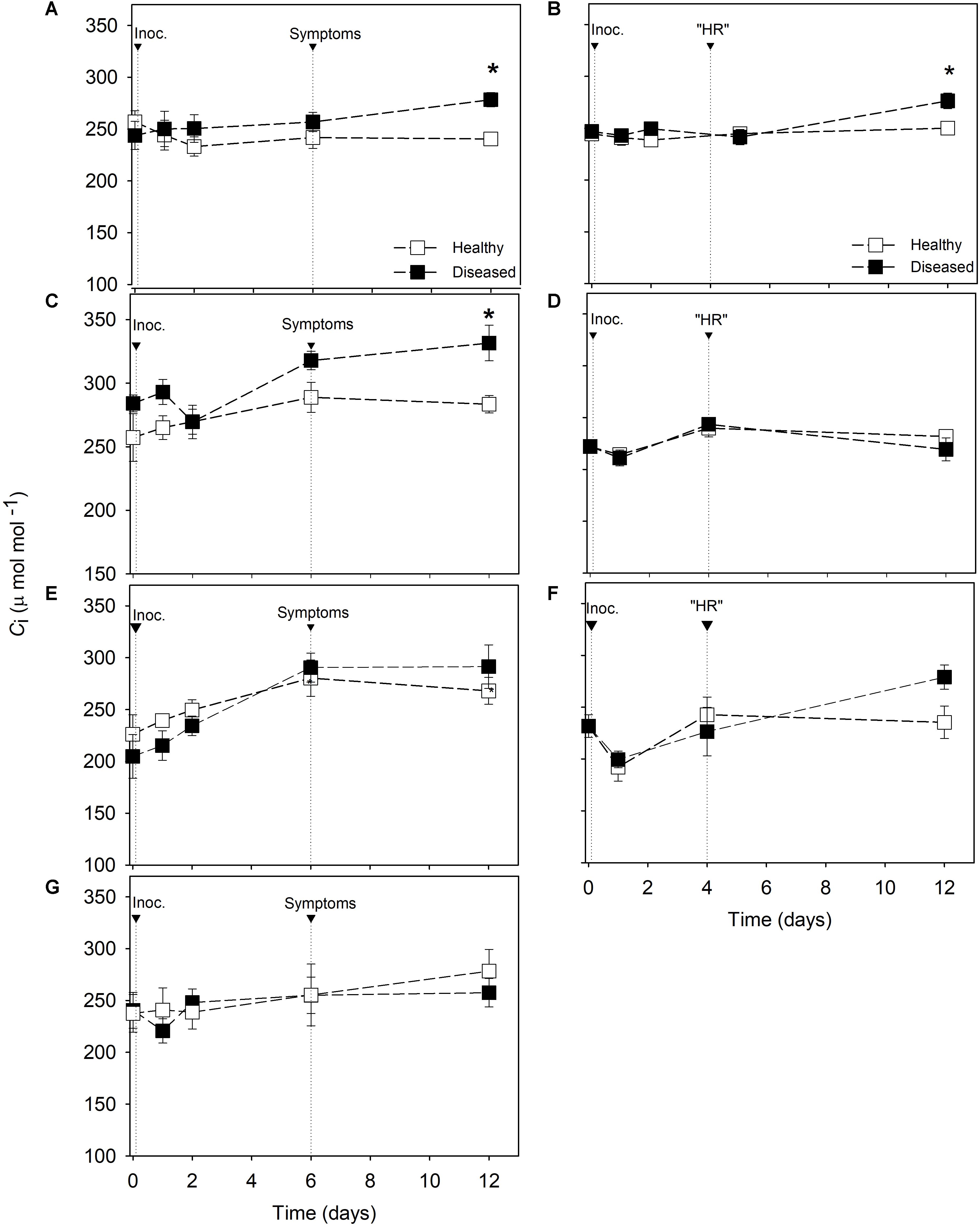
Figure 2. Intercellular CO2 concentration (Ci) in Vitis spp. cultivars. Downy mildew symptoms were observed in Riesling (A), Niagara Rosada (C), Merlot (E) and Moscato (G) 6 days after inoculation. Cell death-like hypersensitive reactions “HR” were observed in Solaris (B), Cabernet Blanc (D) and Phoenix (F) 4 days after inoculation (Inoc.). The average values of healthy and diseased leaves were compared using the Student’s t test for each cultivar (n = 6, ± SE), and * indicates significant differences (p < 0.05).
Considering downy mildew resistant cultivars, gs and E were always lower in diseased leaves than in healthy leaves from 4 dai (Figures 3, 4). Differences in gs between inoculated and non-inoculated leaves of susceptible cultivars were observed only at 12 dai in Riesling, Merlot, and Moscato (Figures 3A,E,G). Nevertheless, no reductions in E were found in susceptible cultivars with the exception of Riesling at 12 dai when the average E value of diseased leaves was reduced by 40% compared with healthy leaves (Figure 4A).
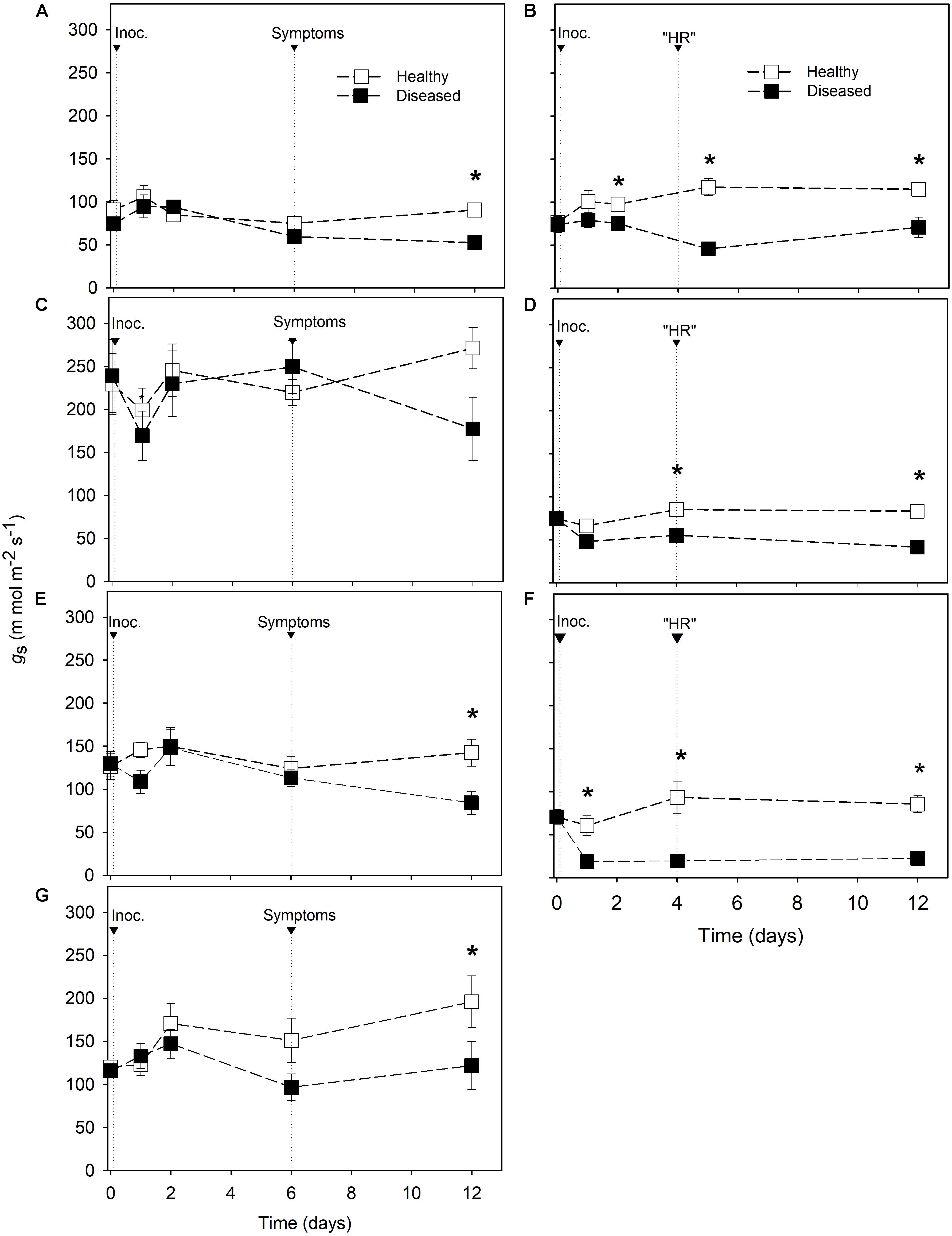
Figure 3. Stomatal conductance (gs) in Vitis spp. cultivars. Downy mildew symptoms were observed in Riesling (A), Niagara Rosada (C), Merlot (E) and Moscato (G) 6 days after inoculation. Cell death-like hypersensitive reactions “HR” were observed in Solaris (B), Cabernet Blanc (D) and Phoenix (F) 4 days after inoculation (Inoc.). The average values of healthy and diseased leaves were compared using the Student’s t test for each cultivar (n = 6, ± SE), and ∗ indicates significant differences (p < 0.05).
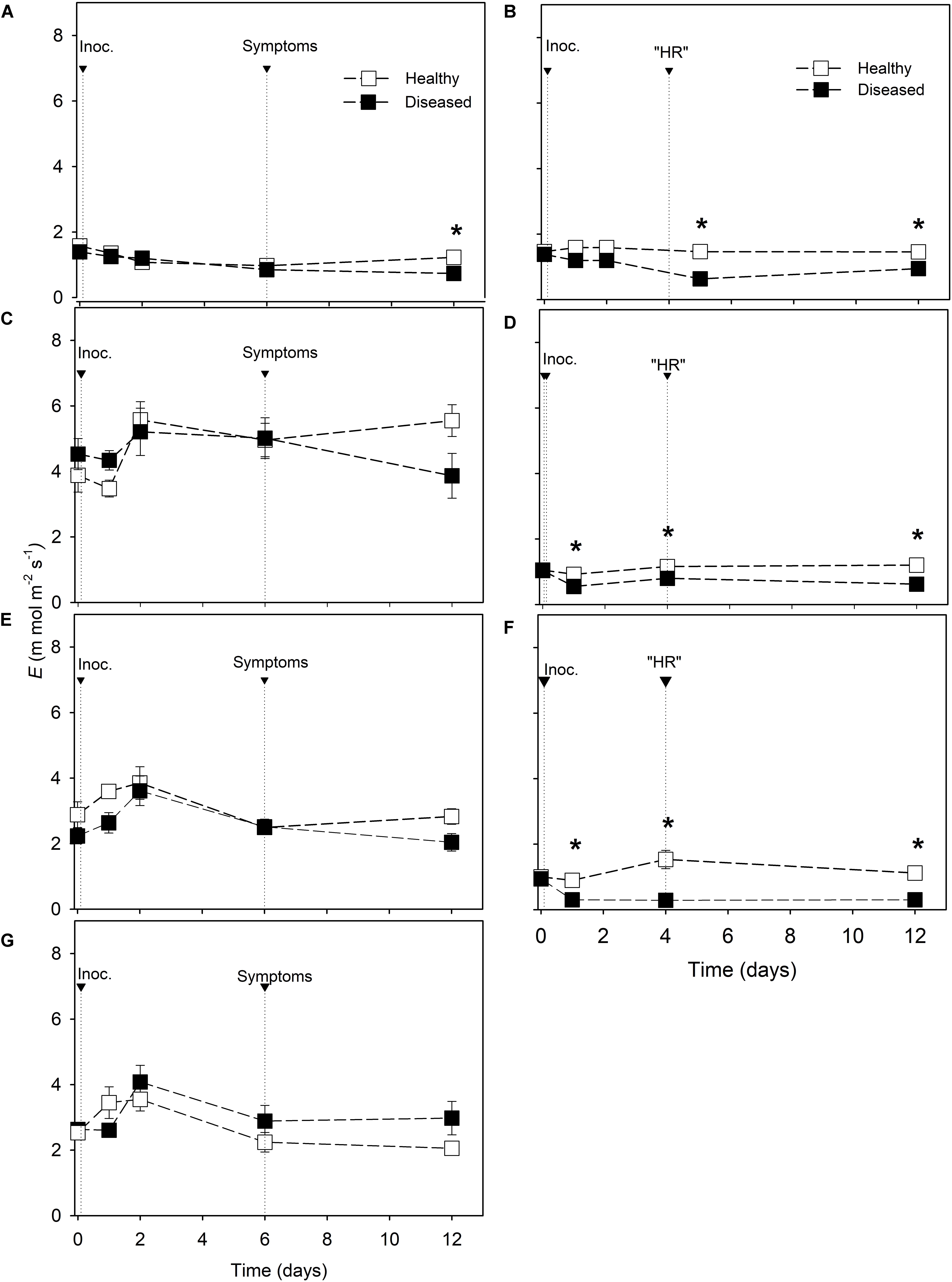
Figure 4. Transpiration rate (E) in Vitis spp. cultivars. Downy mildew symptoms were observed in Riesling (A), Niagara Rosada (C), Merlot (E) and Moscato (G) 6 days after inoculation. Cell death-like hypersensitive reactions “HR” were observed in Solaris (B), Cabernet Blanc (D) and Phoenix (F) 4 days after inoculation (Inoc.). The average values of healthy and diseased leaves were compared using the Student’s t test for each cultivar (n = 6, ± SE), and * indicates significant differences (p < 0.05).
Significant reductions in the instantaneous carboxylation efficiency (k) due to downy mildew were measured from 1 dai for resistant cultivars and at 12 dai for susceptible cultivars (Supplementary Figure S3). In Niagara Rosada, reduction in k was also observed in diseased leaves at 6 dai (Supplementary Figure S3C).
Photosynthetic and Photochemical Evaluations in Solaris and Riesling Cultivars
Healthy plants were more responsive to increasing chloroplastic CO2 concentrations when compared with P. viticola inoculated plants regardless of the cultivar (Figure 5). The maximum leaf CO2 assimilation (Amax) was 18.7 and 12.7 μmol m–2 s–1 in healthy and diseased leaves of Solaris, respectively, and a reduction of 23% was noticed due to P. viticola infection. Vcmax was similar in healthy and diseased leaves of Solaris; however, Jmax was reduced by 34% in diseased leaves (Table 1). The maximum stomatal conductance (gsmax) was reduced (−27%) in Solaris without any significant change in gm (Table 1). In Riesling, Amax was 22% higher in healthy leaves than in diseased leaves. Unlike Solaris, biochemical limitations were higher in diseased leaves of Riesling, with plants showing low Vcmax and Jmax (Table 1). In addition, no significant diffusive limitation was found in diseased leaves compared with healthy leaves of Riesling, as suggested by gm and gsmax (Table 1).
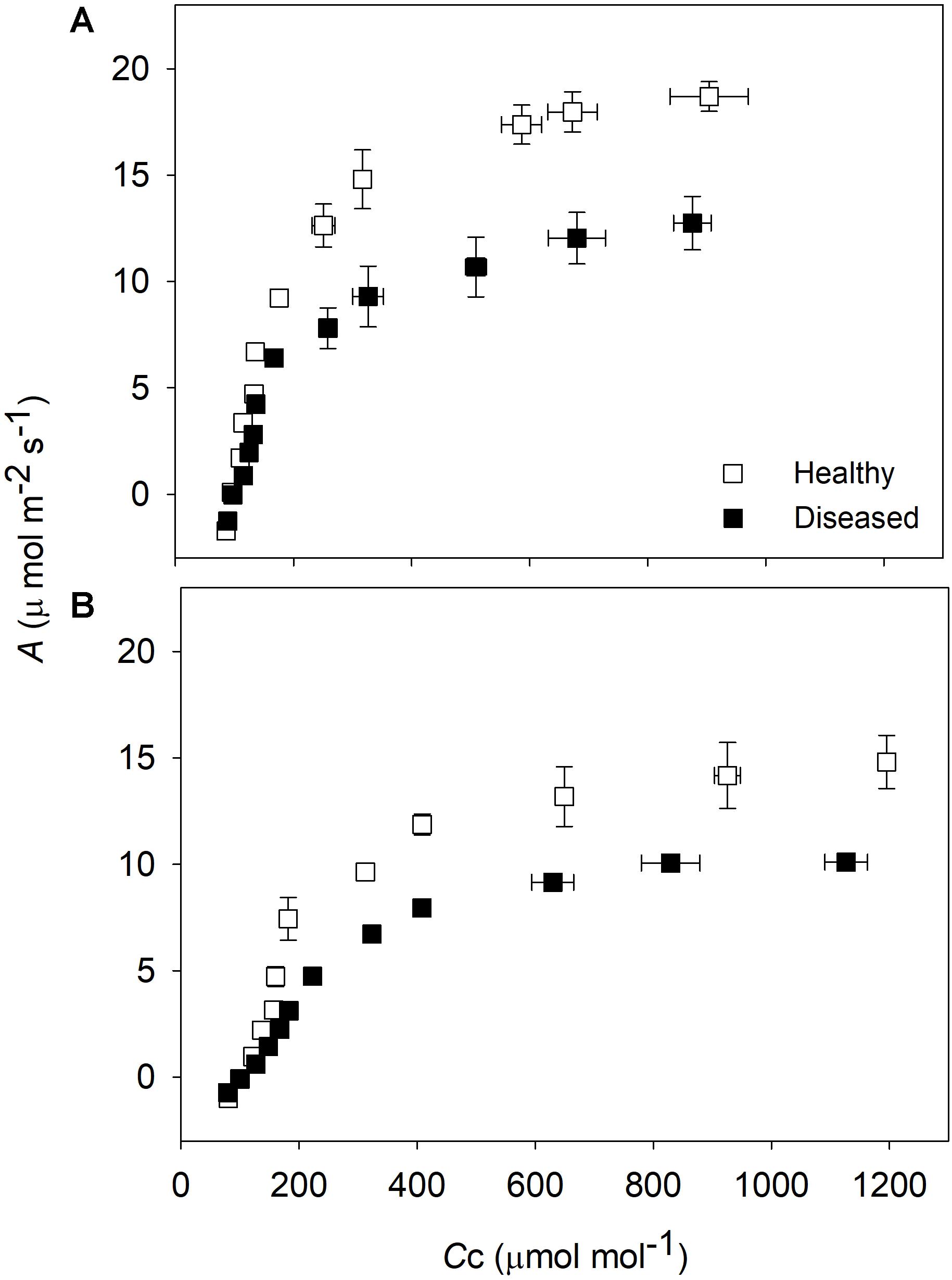
Figure 5. Response of leaf CO2 assimilation (A) to increasing CO2 concentration at carboxylation sites in chloroplasts (Cc) of Vitis vinifera cv. Solaris (A) and Riesling (B). Open symbols represent mean values (n = 3, ± SE) of healthy leaves, while filled symbols indicate diseased leaves.
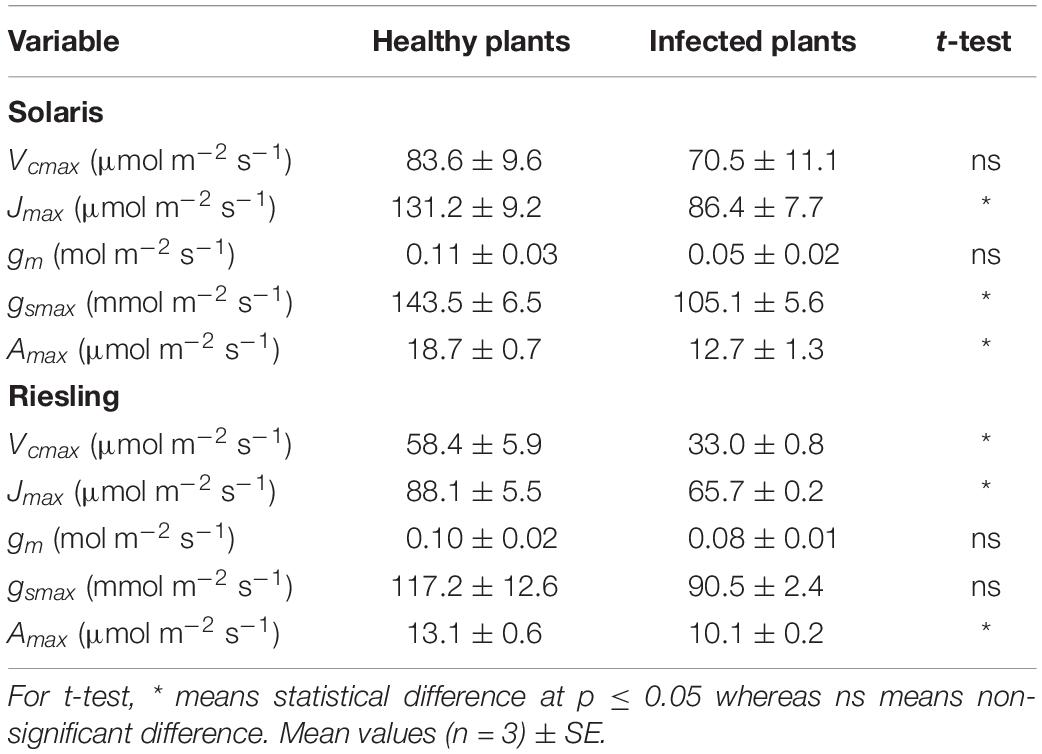
Table 1. Maximum Rubisco carboxylation rate (Vcmax), maximum rate of electron transport driving regeneration of ribulose-1,5-bisphosphate (Jmax), mesophyll conductance (gm), maximum stomatal conductance (gsmax), and maximum photosynthetic rate (Amax) in Solaris and Riesling cultivars as affected by downy mildew (Plasmopara viticola).
Chlorophyll fluorescence from healthy and inoculated leaves of Solaris indicated similar maximum PSII quantum efficiency (Fv/Fm) at 12 hai (0.770 and 0.766). Reduced Fv/Fm in inoculated leaves was found at 5 dai (0.735) when “HR” symptoms were visible (Figure 6). In non-inoculated leaves, Fv/Fm was 0.752. Although the effects of P. viticola infection are clearly visible by imaging of chlorophyll a fluorescence, no significant difference was detected on any day (Supplementary Figure S4).
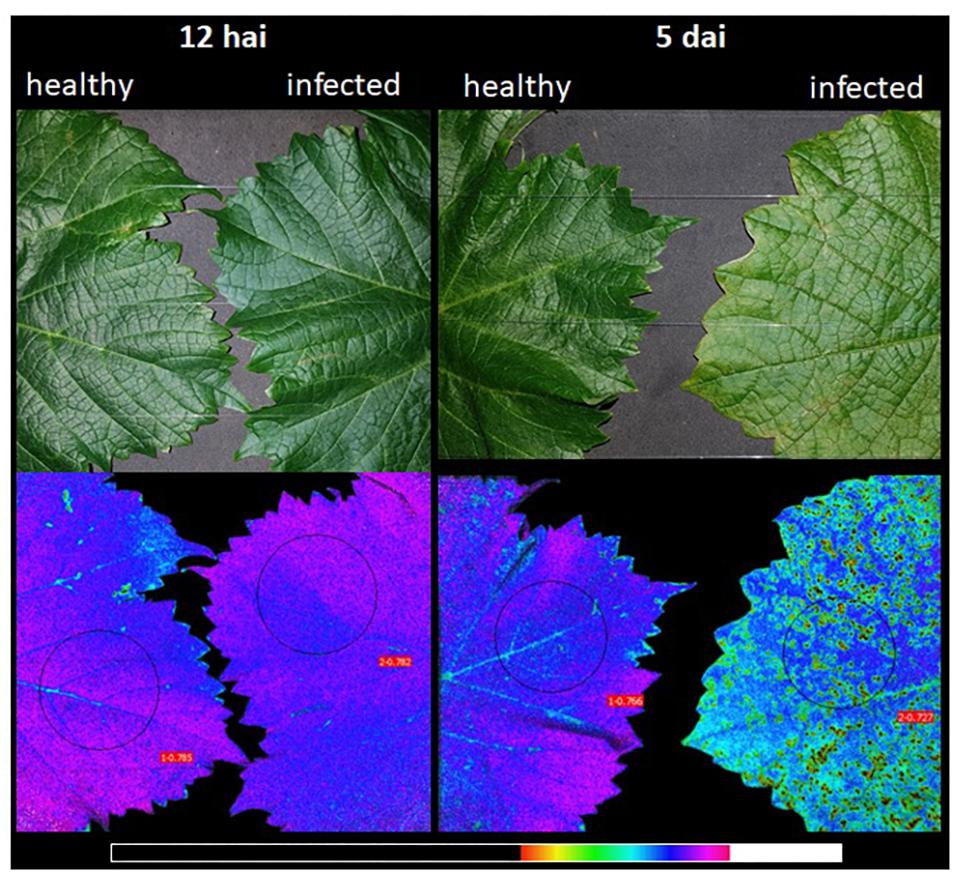
Figure 6. Digital and chlorophyll fluorescence images of grapevine leaves of cv. Solaris after 12 h and 5 days after infection (dai) with Plasmopara viticola. Chlorophyll fluorescence images show the maximum PSII quantum efficiency (Fv/Fm). Circles indicate the area used for calculation of Fv/Fm. Leaves were dark-adapted prior to measurements. The false color code depicted at the bottom of images represents the range of 0 (black) to 1 (white).
Oxidative Burst in the Resistant Cultivar Solaris
The presence of H2O2 as indicated by DAB staining was detected as brown spots close to the secondary veins of diseased leaves (Figure 7B). Brown spots were frequent and intense at 12 and 30 hai (Supplementary Figure S5). Necrotic tissue formed on diseased leaves from 120 hai, and no reaction to DAB staining was observed in diseased leaves at this time (data not shown). Brown spots were not detected in healthy leaves. H2O2 concentrations were lower than 0.5 μmol g–1 fresh mass (FM) in both healthy and diseased leaves of Solaris at 6 hai and from 120 hai. However, increases in leaf H2O2 concentration and data variability were noticed at 12 and 60 hai (Figure 7). O2– was detected as dark blue spots similar in shape to those spots observed in H2O2 detection and near secondary veins. Similar to H2O2, the frequency and intensity of blue spots were high at 12 hai (Figure 7C). No blue spots were observed at 30 hai in diseased leaves, and no O2– was detected in healthy leaves (data not shown). No significant difference was observed for APX activity between healthy and diseased leaves, although the highest APX activity (2.33 μmol H2O2 g–1 FM min–1) was noticed in diseased leaves at 288 hai (Supplementary Figure S6).
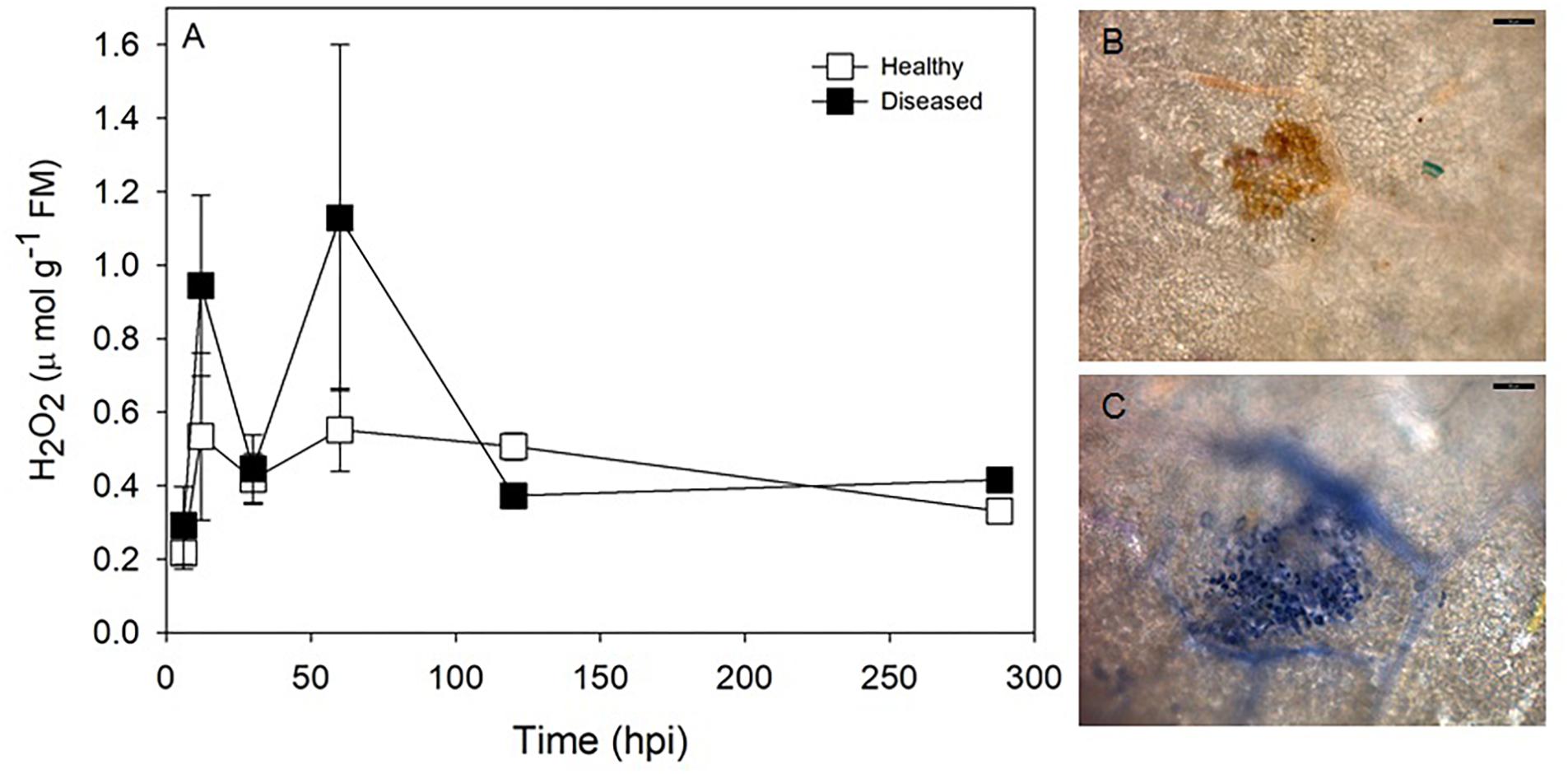
Figure 7. Time-course of leaf H2O2 concentration (A) in healthy and P. viticola infected leaves of cv. Solaris after inoculation and visual localization of H2O2 (B) and O2– (C) in P. viticola inoculated leaves, 12 h after inoculation. Scale bar = 50 μm.
Discussion
The results of this study indicate that P. viticola infection is associated with a cost in terms of CO2 assimilation per unit of leaf area, i.e., resistant cultivars reduced A earlier and more severely than susceptible cultivars. On average, 55% reduction in leaf CO2 assimilation was noticed few hours after pathogen contact with its host, and such a decrease remained up to 12 days after P. viticola inoculation in resistant cultivars Solaris, Cabernet Blanc and Phoenix. This behavior could result from the downregulation of proteins related to photosynthesis and carbohydrate metabolism as previously reported in resistant grapevine cultivars (Figueiredo et al., 2017; Nascimento-Gavioli et al., 2017). A fast and massive transcription of signaling genes affected by P. viticola has also been reported on Rpv-bearing in grapevine cultivars (Fröbel et al., 2019). This response presumably is initiated by a receptor gene that triggers the signal transduction cascade such as that noted in a pathogen-associated molecular pattern (PAMP, Fröbel et al., 2019). In contrast, no effect on leaf gas exchange was observed during the infection and colonization of P. viticola in susceptible cultivars. The net photosynthetic rate on susceptible cultivars was reduced only when symptoms appeared at approximately 6 days after pathogen inoculation. In the susceptible cultivar Trincadeira there was no reduction of photosynthetic pigments content 24 h after P. viticola inoculation (Nascimento et al., 2019). This suggests that the photosynthetic rate remains unaltered on the first stages of the interaction where there are no visible lesions or symptoms (Nascimento et al., 2019). Similarly to our results, in the biotroph interaction between barley and powdery mildew (Blumeria graminis), no reduction of photosynthesis was observed in compatible interactions up to 3 dai. However, the photosynthesis in those leaves was progressively reduced up to 60% compared with healthy leaves at 7 dai (Swarbrick et al., 2006).
Plasmopara viticola infection increased stomatal closure and decreased transpiration rates of resistant cultivars from 4 dai. Stomatal closure is a mechanism related to grapevine resistance to downy mildew, impairing pathogen penetration and sporulation (Allègre et al., 2009). As soon as P. viticola zoospores are released from sporangia, they move toward stomata by chemotaxis, encyst and rapidly penetrate leaf tissue through the stomata in susceptible cultivars. Partial stomatal closure contributes to avoiding P. viticola penetration in resistant grapevine cultivars, although it is insufficient to limit infection (Allègre et al., 2009). Stomatal conductance was reduced only 12 days after inoculation in most susceptible cultivars and was less affected when compared with photosynthesis. In cv. Marselan, a susceptible cultivar to downy mildew, P. viticola affects guard cell function and interferes with stomatal opening, as observed for other oomycetes (Allègre et al., 2007). For instance, Phytophthora infestans can cause abnormal stomata functioning in potato leaves to facilitate the emergence of sporangiophores and sporulation in dark conditions (Farrell et al., 1969).
Although drastic alterations were observed in A, gs and E of inoculated resistant grapevines, no variation was noticed in Ci of Cabernet Blanc and Phoenix leaves while it increased in inoculated leaves of Solaris at 12 dai. This was somehow unexpected since decreases in Ci are expected in leaves due to decreases in stomatal conductance. However, the low variation in Ci of resistant cultivars and the reduction in the instantaneous carboxylation efficiency after the infection (Supplementary Figure S3) in parallel to stomatal closure suggest that another physiological process controls intercellular CO2 concentrations of P. viticola-infected leaves. A rapid increase in the respiration rate of barley leaves resistant to Erysiphe graminis was reported after pathogen contact, while such increase in respiration was found only 3 days after inoculation in compatible interactions (Smedegaard-Petersen and Tolstrup, 1985). A late increase in Ci after the development of disease symptoms was observed in downy mildew-susceptible cultivars, which is in agreement with the linear increase in Ci with increasing rust severity observed in V. labrusca infected with Phakopsora euvitis (Nogueira Júnior et al., 2017).
The activation of signal transduction pathways following the recognition of P. viticola by resistant grapevines leads to the production of reactive oxygen species (Aziz et al., 2003), such as O2– and H2O2 (Kortekamp and Zyprian, 2003). H2O2 production is one of the earliest detectable cytological responses to downy mildew in resistant cultivars, as noticed in Solaris 12 h after inoculation (our data and Trouvelot et al., 2008). The production of reactive oxygen species is associated with localized cell death and is crucial for delimiting the growth of pathogen into leaf tissues (Aziz et al., 2003). Reactive oxygen species cause oxidative damage to a variety of macromolecular targets, such as DNA, lipids and proteins, including enzymes of the Calvin–Benson Cycle and proteins located in the thylakoid membrane, leading to irreversible damage and ultimately tissue necrosis (Halliwell, 2006). Oxidative stress blocks key steps in chlorophyll biosynthesis by directly or indirectly inhibiting the enzymes involved in chlorophyll synthesis (Aarti et al., 2006). Herein, necrotic areas were observed 4 days after inoculation and ranged from 1.8 to 6.0% of total leaf area in resistant cultivars Solaris, Cabernet Blanc and Phoenix. Solaris inoculated with P. viticola showed low maximum PSII quantum efficiency 5 days after inoculation and low regeneration of RuBP driven by electron transport (Figure 6 and Table 1). This photochemical limitation is presumably related to the production of H2O2 and the degradation of cell photosynthetic pigments. As shown in Figure 6, the occurrence of distinct spots indicative of low photosynthetic efficiency is clearly visible upon infection with P. viticola. These spots might contribute to overall reduction on photosynthetic performance. In grapevine leaves of cv. Marselan infected with P. viticola, chlorophyll concentrations were reduced by approximately 30% (Gamm et al., 2011). Measurements conducted in spots and adjacent areas without symptoms confirmed that Fv/Fm was reduced in the spots, but the asymptomatic adjacent areas had Fv/Fm values comparable to non-infected leaves (Gamm et al., 2011). In the present study, Fv/Fm tends to decrease at 5 dai (although not statistically significant). This might be due to the occurrence of necrotic spots that are present in the leaf area considered for calculation of Fv/Fm. Reduced Fv/Fm is an indicator for impaired PSII functionality due to damage to reaction center proteins by reactive oxygen species, and this finding is consistent with reduced assimilation rates. In susceptible cultivars, such as Riesling (this study), Sangiovese (Moriondo et al., 2005) and Niagara Rosada (Nogueira Júnior et al., 2019), the increase in photochemical limitations is also observed during downy mildew symptom expression. Low concentrations of photosynthetic pigments in the yellow halos surrounding P. viticola lesions are related with the reduction in photochemical reactions in these cultivars (Moriondo et al., 2005).
Expression of plant defenses is often assumed to be costly, requiring diversion of resources away from plant growth and development (Walters and Boyle, 2005), and P. viticola greatly enhances carbohydrate hydrolysis and represses photosynthesis-associated proteins in resistant cultivars (Nascimento-Gavioli et al., 2017). In the incompatible interaction of barley and Erysiphe graminis f. sp. hordei, pathogen recognition occurs during haustoria development into host cells. After recognition, several histochemical and biochemical alterations are induced in hosts (Smedegaard-Petersen and Tolstrup, 1985). The cost to the net photosynthetic rate in barley leaves challenged by E. graminis f. sp. hordei is also similar to the cost observed in downy mildew-resistant grapevines. However, in the incompatible interaction of Eucalyptus grandis and Puccinia psidii, the host reacts immediately during pathogen appressoria formation. Consequently, only small flecks are observed in the host before pathogen penetration and photosynthetic activity is unaffected (Alves et al., 2011).
Although resistant cultivars decrease the photosynthetic activity when infected by P. viticola, its use provides a substantial contribution to the sustainability of viticulture while reducing pesticide applications (Buonassisi et al., 2017). P. viticola can complete its life cycle in resistant cultivars. However, low oomycete sporulation is noted compared with the sporulation in susceptible cultivars, and sporangiophores are abnormal. The reduced sporulation affects the secondary spread of the pathogen and reduces the rate of disease progression in field (Parlevliet, 1979). In the last 20 years, newly bred grapevine cultivars with disease resistance, desirable agronomic attributes and good enological characteristics have been introduced to the market (Buonassisi et al., 2017). These cultivars bear different Rpv resistance loci to downy mildew and several studies have been developed to identify the resistance loci on these materials. Locus Rpv 3 is present in all resistant cultivars used in the present work. However, the cultivar Solaris has also the Rpv10 locus1. In general, the effect of P. viticola on photosynthesis was similar for cvs. Solaris, Cabernet Blanc and Phoenix.
Conclusion
In conclusion, P. viticola differently affects leaf gas exchange in resistant and susceptible cultivars, with resistant cultivars exhibiting faster responses. While net photosynthetic rate and stomatal conductance of resistant cultivars are rapidly and negatively affected by P. viticola, susceptible cultivars only exhibit reduced leaf gas exchange at late stages of disease development. These results would suggest that the recognition followed by induced resistance responses is costly for grapevine carbon assimilation. In the absence of recognition, the pathogen colonizes the leaf tissue without affecting photosynthesis until the appearance of visual symptoms. Although the induction of defenses in resistant grapevine cultivars is costly, the use of genetic resistance to control downy mildew as an alternative to chemical control is highly desirable and complementary for a sustainable viticulture.
Data Availability Statement
The raw data supporting the conclusions of this article will be made available by the authors, without undue reservation, to any qualified researcher.
Author Contributions
AN and LA devised the project and the main conceptual ideas. AN and MT performed the experiments. AN, LA, RR, and AT revised critically the project and the manuscript for important intellectual content. All authors discussed the results and contributed to the final manuscript.
Funding
This research was received financial support from FAPESP (2013/24003-9, 2017/02432-6, and 2018/00952-5).
Conflict of Interest
The authors declare that the research was conducted in the absence of any commercial or financial relationships that could be construed as a potential conflict of interest.
Acknowledgments
The authors acknowledge support by the Open Access Publication Funds of the Göttingen University. The authors gratefully acknowledge the financial support from FAPESP (2013/24003-9, 2017/02432-6, and 2018/00952-5), and are indebt with Barbara L. Navarro (Department of Crop Sciences, University of Göttingen) for helping in H2O2 and O2– localization analyses, and with Dr. Andreas Kortekamp (DLR Rheinpfalz, Institute of Plant Protection, Neustadt an der Weinstrasse, Germany) for supplying the strain of P. viticola. RR and LA also acknowledge the fellowships granted by the National Council for Scientific and Technological Development (CNPq, Brazil).
Supplementary Material
The Supplementary Material for this article can be found online at: https://www.frontiersin.org/articles/10.3389/fpls.2020.00235/full#supplementary-material
Footnotes
References
Aarti, P. D., Tanaka, R., and Tanaka, A. (2006). Effects of oxidative stress on chlorophyll biosynthesis in cucumber (Cucumis sativus) cotyledons. Physiol Plant. 128, 186–197. doi: 10.1111/j.1399-3054.2006.00720.x
Allègre, M., Daire, X., Héloir, M. C., Trouvelot, S., Mercier, L., Adrian, M., et al. (2007). Stomatal deregulation in Plasmopara viticola – Infected grapevines leaves. New Phytol. 173, 832–840. doi: 10.1111/j.1469-8137.2006.01959.x
Allègre, M., Héloir, M. C., Trouvelot, S., Daire, X., Pugin, A., Wendehenne, D., et al. (2009). Are grapevine stomata involved in the elicitor-induced protection against downy mildew? Mol. Plant Microbe Interact. 22, 977–986. doi: 10.1094/mpmi-22-8-0977
Alves, A. A., Guimarães, L. M. S., Chaves, A. R. M. C., DaMatta, F. M., and Alfenas, A. C. (2011). Leaf gas exchange and chlorophyll a fluorescence of Eucalyptus urophylla in response to Puccinia psidii infection. Acta Physiol. Plant. 33, 1831–1839. doi: 10.1007/s11738-011-0722-z
Armijo, G., Schlechter, R., Agurto, M., Muñoz, D., Nuñez, C., and Arce-Johnson, P. (2016). Grapevine pathogenic microorganisms: understanding infection strategies and host response scenarios. Front. Plant Sci. 7:382. doi: 10.3389/fpls.2016.00382
Ash, G. (2000). Downy mildew of grape. The Plant Health Instructor. Available online at: http://www.apsnet.org/edcenter/intropp/lessons/fungi/Oomycetes/Pages/DownyMildewGrape.aspx (accessed November 20, 2018).
Aziz, A., Poinssot, B., Daire, X., Adrian, M., Bézier, A., Lambert, B., et al. (2003). Laminarin elicits defense responses in grapevine and induces protection against Botrytis cinerea and Plasmopara viticola. Mol. Plant Microbe Interact. 16, 1118–1128. doi: 10.1094/MPMI.2003.16.12.1118
Bellin, D., Peressotti, E., Merdinoglu, D., Wiedemann-Merdinoglu, S., Adam-Blondon, A. F., Cipriani, G., et al. (2009). Resistance to Plasmopara viticola in grapevine ‘Bianca’ is controlled by a major dominant gene causing localised necrosis at the infection site. Theor. Appl. Genet. 120, 163–176. doi: 10.1007/s00122-009-1167-2
Berger, S., Sinha, A. K., and Roitsch, T. (2007). Plant physiology meets phytopathology: plant primary metabolism and plant-pathogen interactions. J. Exp. Bot. 58, 4019–4026. doi: 10.1093/jxb/erm298
Bernacchi, C. J., Pimentel, C., and Long, S. P. (2003). In vivo temperature response functions of parameters required to model RuBP-limited photosynthesis. Plant Cell Environ. 26, 1419–1430. doi: 10.1046/j.0016-8025.2003.01050.x
Blasi, P., Blanc, S., Wiedemann-Merdinoglu, S., Prado, E., Rühl, E. H., Mestre, P., et al. (2011). Construction of a reference linkage map of Vitis amurensis and genetic mapping of Rpv8, a locus conferring resistance to grapevine downy mildew. Theor. Appl. Genet. 123, 43–53. doi: 10.1007/s00122-011-1565-0
Buonassisi, D., Colombo, M., Migliaro, D., Dolzani, C., Peressotti, E., Mizzotti, C., et al. (2017). Breeding for grapevine downy mildew resistance: a review of “omics” approaches. Euphytica 213:103. doi: 10.1007/s10681-017-1882-8
Cappello, F. P., Spósito, M. B., and Osaki, M. (2017). Production costs and profitability of ‘Niagara Rosada’ table grape grown in different regions of São Paulo state. Rev. Bras. Frutic. 39, e–774. doi: 10.1590/0100-29452017774
Cipollini, D. (1998). Induced defenses and phenotypic plasticity. Trends Ecol. Evol. 13, 200. doi: 10.1016/S0169-5347(98)01366-4
Dai, G. H., Andary, C., Mondolot-Cosson, L., and Boubals, D. (1995). Histochemical studies on the interaction between three species of grapevine, Vitis vinifera, V. rupestris and V. rotundifolia and the downy mildew fungus, Plasmopara viticola. Physiol. Mol. Plant Pathol. 46, 177–188. doi: 10.1006/pmpp.1995.1014
DeWitt, T. J., Sih, A., and Wilson, S. D. (1998). Costs and limits of phenotypic plasticity. Trends Ecol. Evol. 13, 77–81. doi: 10.1016/S0169-5347(97)01274-3
Díez-Navajas, A. M., Wiedemann-Merdinoglu, S., Greif, C., and Merdinoglu, D. (2008). Nonhost versus host resistance to the grapevine downy mildew, Plasmopara viticola, studied at the tissue level. Phytopathology 98, 776–780. doi: 10.1094/PHYTO-98-7-0776
Farquhar, G. D., von Caemmerer, S., and Berry, J. A. (1980). A biochemical model of photosynthetic CO2 assimilation in leaves of C3 species. Planta 149, 78–90. doi: 10.1007/BF00386231
Farrell, G. M., Preece, T. F., and Wren, M. J. (1969). Effects of infection by Phytophthora infestans (Mont.) de Bary on the stomata of potato leaves. Ann. Appl. Biol. 63, 265–275. doi: 10.1111/j.1744-7348.1969.tb05488.x
Figueiredo, A., Fortes, A. M., Ferreira, S., Sebastiana, M., Choi, Y. H., Souza, L., et al. (2008). Transcriptional and metabolic profiling of grape (Vitis vinifera L.) leaves unravel possible innate resistance against pathogenic fungi. J. Exp. Bot. 59, 3371–3381. doi: 10.1093/jxb/ern187
Figueiredo, A., Martins, J., Sebastiana, M., Guerreiro, A., Silva, A., Matos, A. R., et al. (2017). Specific adjustments in grapevine leaf proteome discriminating resistant and susceptible grapevine genotypes to Plasmopara viticola. J. Proteomics 152, 48–57. doi: 10.1016/j.jprot.2016.10.012
Fischer, B. M., Salakhutdinov, I., Akkurt, M., Eibach, R., Edwards, K. J., Töpfer, R., et al. (2004). Quantitative trait locus analysis of fungal disease resistance factors on a molecular map of grapevine. Theor. Appl. Genet. 108, 501–515. doi: 10.1007/s00122-003-1445-3
Flexas, J., Diaz-Espejo, A., Galmés, J., Kaldenhoff, R., Medrano, H., and Ribas-Carbo, M. (2007). Rapid variations of mesophyll conductance in response to changes in CO2 concentration around leaves. Plant Cell Environ. 30, 1284–1298. doi: 10.1111/j.1365-3040.2007.01700.x
Fröbel, S., Dudenhöffer, J., Töpfer, R., and Zyprian, E. (2019). Transcriptome analysis of early downy mildew (Plasmopara viticola) defense in grapevine carrying the Asian resistance locus Rpv10. Euphytica 215:28. doi: 10.1007/s10681-019-2355-z
Gamm, M., Héloir, M. C., Bligny, R., Vaillant-Gaveau, N., Trouvelot, S., Alcaraz, G., et al. (2011). Changes in carbohydrate metabolism in Plasmopara viticola-infected grapevine leaves. Mol. Plant Microbe Interact. 24, 1061–1073. doi: 10.1094/MPMI-02-11-0040
Gessler, C., Pertot, I., and Perazzolli, M. (2011). Plasmopara viticola: a review of knowledge on downy mildew of grapevine and effective disease management. Phytopathol. Mediterr. 50, 3–44. doi: 10.14601/Phytopathol_Mediterr-9360
Gindro, K., Pezet, R., and Viret, O. (2003). Histological study of the responses of two Vitis vinifera cultivars (resistant and susceptible) to Plasmopara viticola infections. Plant Physiol. Biochem. 41, 846–853. doi: 10.1016/S0981-9428(03)00124-4
Halliwell, B. (2006). Reactive species and antioxidants. Redox biology is a fundamental theme of aerobic life. Plant Physiol. 141, 312–322. doi: 10.1104/pp.106.077073
Heil, M., and Baldwin, I. T. (2002). Fitness costs of induced resistance: emerging experimental support for a slippery concept. Trends Plant Sci. 7, 61–67. doi: 10.1016/S1360-1385(01)02186-0
Jermini, M., Blaise, P., and Gessler, C. (2010). Influence of Plasmopara viticola on gas exchange parameters on field-grown Vitis vinifera ‘Merlot’. Vitis 49, 87–93.
Kamoun, S., Furzer, O., Jones, J. D., Judelson, H. S., Ali, G. S., Dalio, R. J., et al. (2015). The Top 10 oomycete pathogens in molecular plant pathology. Mol. Plant Pathol. 16, 413–434. doi: 10.1111/mpp.12190
Kortekamp, A. (2006). Expression analysis of defence-related genes in grapevine leaves after inoculation with a host and a non-host pathogen. Plant Physiol. Biochem. 44, 58–67. doi: 10.1016/j.plaphy.2006.01.008
Kortekamp, A., Wind, R., and Zyprian, E. (1997). The role of callose deposits during infection of two downy mildew-tolerant and two -susceptible Vitis cultivars. Vitis 36, 103–104.
Kortekamp, A., and Zyprian, E. (2003). Characterization of Plasmopara-resistance in grapevine using in vitro plants. J. Plant Physiol. 160, 1393–1400. doi: 10.1078/0176-1617-01021
Liu, R., Wang, L., Zhu, J., Chen, T., Wang, Y., and Xu, Y. (2015). Histological responses to downy mildew in resistant and susceptible grapevines. Protoplasma 252, 259–270. doi: 10.1007/s00709-014-0677-1
Machado, E. C., Schmidt, P. T., Medina, C. L., and Ribeiro, R. V. (2005). Respostas da fotossíntese de três espécies de citros a fatores ambientais. Pesq. Agropec. Bras. 40, 1161–1170. doi: 10.1590/S0100-204X2005001200002
Marguerit, E., Boury, C., Manicki, A., Donnart, M., Butterlin, G., Némorin, A., et al. (2009). Genetic dissection of sex determinism, inflorescence morphology and downy mildew resistance in grapevine. Theor. Appl. Genet. 118, 1261–1278. doi: 10.1007/s00122-009-0979-4
Maxwell, K., and Johnson, G. N. (2000). Chlorophyll fluorescence – A practical guide. J. Exp. Bot. 51, 659–668. doi: 10.1093/jexbot/51.345.659
Merdinoglu, D., Wiedeman-Merdinoglu, S., Coste, P., Dumas, V., Haetty, S., Butterlin, G., et al. (2003). Genetic analysis of Downy Mildew resistance derived from Muscadinia rotundifolia. Acta Hortic. 603, 451–456. doi: 10.17660/ActaHortic.2003.603.57
Moriondo, M., Orlandini, S., Giuntoli, A., and Bindi, M. (2005). The effect of downy and powdery mildew on grapevine (Vitis vinifera L.) leaf gas exchange. J. Phytopathol. 153, 350–357. doi: 10.1111/j.1439-0434.2005.00984.x
Nakano, Y., and Asada, K. (1981). Hydrogen peroxide is scavenged by ascorbate-specific peroxidase in spinach chloroplasts. Plant Cell Physiol. 22, 867–880. doi: 10.1093/oxfordjournals.pcp.a076232
Nascimento, R., Maia, M., Ferreira, A. E., Silva, A. B., Freire, A. P., Cordeiro, C., et al. (2019). Early stage metabolic events associated with the establishment of Vitis vinifera – Plasmopara viticola compatible interaction. Plant Physiol. Biochem. 137, 1–13. doi: 10.1016/j.plaphy.2019.01.026
Nascimento-Gavioli, M. C. A., Agapito-Tenfen, S. Z., Nodari, R. O., Welter, L. J., Mora, F. D. S., Saifert, L., et al. (2017). Proteome of Plasmopara viticola-infected Vitis vinifera provides insights into grapevine Rpv1/Rpv3 pyramided resistance to downy mildew. J Proteomics 151, 264–274. doi: 10.1016/j.jprot.2016.05.024
Nogueira Júnior, A. F., Ribeiro, R. V., Appezzato-da-Glória, B., Soares, M. K., Rasera, J. B., and Amorim, L. (2017). Phakopsora euvitis causes unusual damage to leaves and modifies carbohydrate metabolism in grapevine. Front. Plant Sci. 8:1675. doi: 10.3389/fpls.2017.01675
Nogueira Júnior, A. F., Ribeiro, R. V., Marcos, F. C. C., and Amorim, L. (2019). Virtual lesions and photosynthetic damage caused by Plasmopara viticola in Vitis labrusca. Eur. J. Plant Pathol. 155, 545–555. doi: 10.1007/s10658-019-01791-2
Parlevliet, J. E. (1979). Components of resistance that reduce the rate of epidemic development. Ann. Rev. Phytopathol. 17, 203–222. doi: 10.1146/annurev.py.17.090179.001223
Peressotti, E., Wiedemann-Merdinoglu, S., Delmotte, F., Bellin, D., Di Gaspero, G., Testolin, R., et al. (2010). Breakdown of resistance to grapevine downy mildew upon limited deployment of a resistant variety. BMC Plant Biol. 10:147. doi: 10.1186/1471-2229-10-147
Purrington, C. B. (2000). Costs of resistance. Curr. Opin. Plant Biol. 3, 305–308. doi: 10.1016/S1369-5266(00)00085-6
Richter, H., Pezet, R., Viret, O., and Gindro, K. (2006). Characterization of 3 new partial stilbene synthase genes out of over 20 expressed in Vitis vinifera during the interaction with Plasmopara viticola. Physiol. Mol. Plant Pathol. 67, 248–260. doi: 10.1016/j.pmpp.2006.03.001
Rossi, V., Caffi, T., and Gobbin, D. (2013). Contribution of molecular studies to botanical epidemiology and disease modelling: grapevine downy mildew as a case-study. Eur. J. Plant Pathol. 135, 641–654. doi: 10.1007/s10658-012-0114-2
Scharte, J., Schön, H., and Weis, E. (2005). Photosynthesis and carbohydrate metabolism in tobacco leaves during an incompatible interaction with Phytophthora nicotianae. Plant Cell Environ. 28, 1421–1435. doi: 10.1111/j.1365-3040.2005.01380.x
Schwander, F., Eibach, R., Fechter, I., Hausmann, L., Zyprian, E., and Töpfer, R. (2012). Rpv10: a new locus from the Asian Vitis gene pool for pyramiding downy mildew resistance loci in grapevine. Theor. Appl. Genet. 124, 163–176. doi: 10.1007/s00122-011-1695-4
Slaughter, A. R., Hamiduzzaman, M. M., Gindro, K., Neuhaus, J. M., and Mauch-Mani, B. (2008). Beta-aminobutyric acid-induced resistance in grapevine against downy mildew: involvement of pterostilbene. Eur. J. Plant Pathol. 122, 185–195. doi: 10.1007/s10658-008-9285-2
Smedegaard-Petersen, V., and Tolstrup, K. (1985). The limiting effect of disease resistance on yield. Ann. Rev. Phytopathol. 23, 475–490. doi: 10.1146/annurev.py.23.090185.002355
Swarbrick, P. J., Schulze-Lefert, P., and Scholes, J. D. (2006). Metabolic consequences of susceptibility and resistance (race-specific and broad-spectrum) in barley leaves challenged with powdery mildew. Plant Cell Environ. 29, 1061–1076. doi: 10.1111/j.1365-3040.2005.01472.x
Thordal-Christensen, H., Zhang, Z., Wei, Y., and Collinge, D. B. (1997). Subcellular localization of H2O2 in plants. H2O2 accumulation in papillae and hypersensitive response during the barley-powdery mildew interaction. Plant J. 11, 1187–1194. doi: 10.1046/j.1365-313X.1997.11061187.x
Trouvelot, S., Varnier, A.-L., Allègre, M., Mercier, L., Baillieul, F., Arnould, C., et al. (2008). A β-1,3 glucan sulfate induces resistance in grapevine against Plasmopara viticola through priming of defense responses, including HR-like cell death. Mol. Plant-Microbe Interact. 21, 232–243. doi: 10.1094/MPMI-21-2-0232
Vale, F. X. R., Fernandes Filho, E. I. F., and Liberato, J. R. (2001). “QUANT – a software for plant disease severity assessment,” in Proceedings of the 8th International Congress of Plant Pathology. Christchurch, NZ, 105. doi: 10.1094/mpmi-21-2-0232
Venuti, S., Copetti, D., Foria, S., Falginella, L., Hoffmann, S., Bellin, D., et al. (2013). Historical introgression of the downy mildew resistance gene Rpv12 from the Asian species Vitis amurensis into grapevine varieties. PLoS ONE 8:e61228. doi: 10.1371/journal.pone.0061228
Vezzulli, S., Malacarne, G., Masuero, D., Vecchione, A., Dolzani, C., Goremykin, V., et al. (2019). The Rpv3-3 haplotype and stilbenoid induction mediate downy mildew resistance in a grapevine interspecific population. Front. Plant Sci. 10:234. doi: 10.3389/fpls.2019.00234
Walters, D. R., and Boyle, C. (2005). Induced resistance and allocation cost: what is the impact of pathogen challenge? Physiol. Mol. Plant Pathol. 66, 40–44. doi: 10.1016/j.pmpp.2005.04.002
Welter, L., Göktürk-Baydar, N., Akkurt, M., Maul, E., Eibach, R., Töpfer, R., et al. (2007). Genetic mapping and localization of quantitative trait loci affecting fungal disease resistance and leaf morphology in grapevine (Vitis vinifera L). Mol. Breed. 20, 359–374. doi: 10.1007/s11032-007-9097-7
Wilcox, W. F., Gubler, W. D., and Uyemoto, J. K. (2015). Compendium of Grape Diseases, Disorders and Pests. Saint Paul, MN: APS Press.
Keywords: gas exchange, downy mildew, Vitis vinifera, photosynthesis limitations, biotic stress
Citation: Nogueira Júnior AF, Tränkner M, Ribeiro RV, von Tiedemann A and Amorim L (2020) Photosynthetic Cost Associated With Induced Defense to Plasmopara viticola in Grapevine. Front. Plant Sci. 11:235. doi: 10.3389/fpls.2020.00235
Received: 19 April 2019; Accepted: 14 February 2020;
Published: 19 March 2020.
Edited by:
Eva Maria Zyprian, Julius Kühn-Institut, GermanyReviewed by:
Marisa Maia, University of Lisbon, PortugalClaudio Moser, Fondazione Edmund Mach, Italy
Copyright © 2020 Nogueira Júnior, Tränkner, Ribeiro, von Tiedemann and Amorim. This is an open-access article distributed under the terms of the Creative Commons Attribution License (CC BY). The use, distribution or reproduction in other forums is permitted, provided the original author(s) and the copyright owner(s) are credited and that the original publication in this journal is cited, in accordance with accepted academic practice. No use, distribution or reproduction is permitted which does not comply with these terms.
*Correspondence: Lilian Amorim, lilian.amorim@usp.br; Merle Tränkner, merle.traenkner@ agr.uni-goettingen.de