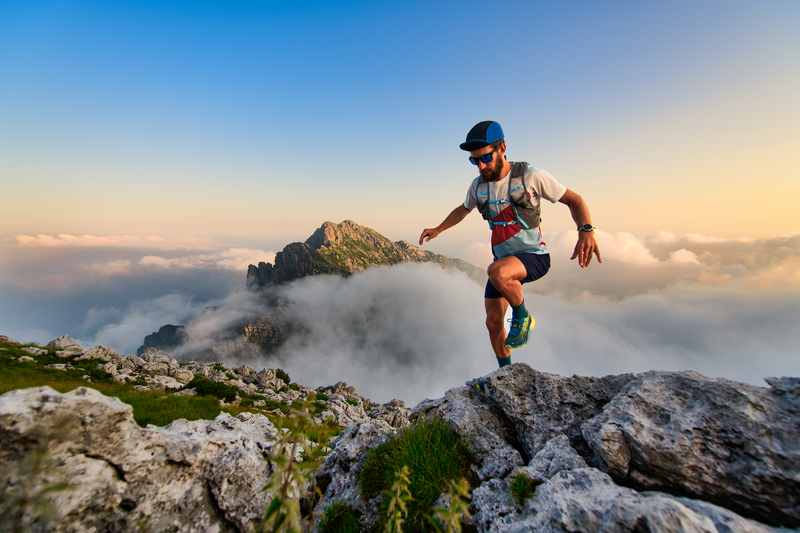
95% of researchers rate our articles as excellent or good
Learn more about the work of our research integrity team to safeguard the quality of each article we publish.
Find out more
ORIGINAL RESEARCH article
Front. Plant Sci. , 26 February 2020
Sec. Crop and Product Physiology
Volume 11 - 2020 | https://doi.org/10.3389/fpls.2020.00072
This article is part of the Research Topic Towards a Functional Characterization of Plant Biostimulants View all 20 articles
In order to reduce chemical fertilization and improve the sustainability of common wheat (Triticum aestivum L.) cultivation, maintaining at the same time high production and quality standards, this study investigated the effects of three commercial biofertilizers on rhizosphere bacterial biomass, biodiversity and enzymatic activity, and on plant growth and grain yield in a field trial. The wheat seeds were inoculated with the following aiding microrganisms: (i) a bacterial consortium (Azospirillum spp. + Azoarcus spp. + Azorhizobium spp.); and two mycorrhizal fungal-bacterial consortia, viz. (ii) Rhizophagus irregularis + Azotobacter vinelandii, and (iii) R. irregularis + Bacillus megaterium + Frateuria aurantia, and comparisons were made with noninoculated controls. We demonstrate that all the biofertilizers significantly enhanced plant growth and nitrogen accumulation during stem elongation and heading, but this was translated into only small grain yield gains (+1%–4% vs controls). The total gluten content of the flour was not affected, but in general biofertilization significantly upregulated two high-quality protein subunits, i.e., the 81 kDa high-molecular-weight glutenin subunit and the 43.6 kDa low-molecular-weight glutenin subunit. These effects were associated with increases in the rhizosphere microbial biomass and the activity of enzymes such as β-glucosidase, α-mannosidase, β-mannosidase, and xylosidase, which are involved in organic matter decomposition, particularly when Rhizophagus irregularis was included as inoculant. No changes in microbial biodiversity were observed. Our results suggest that seed-applied biofertilizers may be effectively exploited in sustainable wheat cultivation without altering the biodiversity of the resident microbiome, but attention should be paid to the composition of the microbial consortia in order to maximize their benefits in crop cultivation.
The use of microbial inoculants is of strategic interest for their potential to replace chemical fertilizers and pesticides in agricultural systems, and improve environmental sustainability.
Plant-aiding microorganisms, often referred to as plant growth-promoting rhizobacteria (PGPR) (Gupta et al., 2015) and arbuscular mycorrhizal fungi (AMF) (Igiehon and Babalola, 2017), interact with plants roots (Hayat et al., 2010) by enhancing growth, mineral nutrition, drought tolerance, and disease resistance (Nadeem et al., 2013).
Bacteria can beneficially contribute to plant growth via N2-fixation and solubilization of low mobile nutrients. Biological N2-fixation is carried out by various symbiotic and nonsymbiotic bacteria (Shridhar, 2012).
Symbiotic PGPR fix atmospheric N2 mainly within plant roots, with many genera involved, such as Rhizobium, Sinorhizobium, Bradyrhizobium, Mesorhizobium, and Azorhizobium. The latter can enter plant roots intercellularly and colonize the xylem of wheat, rice, corn, and other nonlegume crops without forming real symbiotic structures (Cocking, 2003), although Azorhizobium caulinodans is known to form both stem and root nodules in Sesbania rostrata (Robertson et al., 1995).
Nonsymbiotic N2 fixation is carried out by free-living diazotrophic bacteria, such as Azospirillum, Azoarcus, Azotobacter, Burkholderia, Gluconacetobacter, Clostridium, and Pseudomonas (Bhattacharyya and Jha, 2012; Singh, 2018). The absence of symbiosis with plants supports their common use in biofertilizers formulation. These bacteria can improve the uptake efficiency of nitrogen in many crops, thanks to the nitrogenase activity and soil N mineralization (Chauhan et al., 2015). In addition, Azotobacter and Azospirillum stimulate root hair formation, and lateral and adventitious root initiation through hormonal (auxins) exchange (Vejan et al., 2016; Zeffa et al., 2019).
Some PGPR are also known as phosphate- and potassium-solubilizing bacteria through rhizosphere acidification (Afzal and Bano, 2008; Meena et al., 2014). Among these, Bacillus megaterium and Frateuria aurantia were reported as efficient P- and K-mobilizing bacteria, respectively, thus being potentially exploitable in crop cultivation (Subhashini, 2014; Ghaffari et al., 2018).
AMF are nonpathogenic fungi living in symbioses with roots of a large number of spontaneous and cultivated plants, supplying them with mineral nutrients and water, particularly in natural environments (Solaiman and Mickan, 2014). Fostering AMF-plant symbiosis through inoculation, can significantly improve nutrients accumulation, the plant physiological processes and biomass accumulation (Mitra et al., 2019), besides root growth promotion and abiotic stresses mitigation (Begum et al., 2019).
However, many biotic and abiotic factors may affect the ability of plant-aiding microorganisms to successfully colonize the rhizosphere (Ahmad et al., 2011), and hence impact on these effects (Kloepper and Beauchamp, 1992; Castro-Sowinski et al., 2007).
In a context of environmental protection and increased demand for chemical-free food products, increasing numbers of commercial biofertilizers have come onto the market in recent years, containing either single or associated PGPR strains or mycorrhizal fungi (Owen et al., 2015).
There is considerable evidence for the positive effects of PGPR on plant growth under controlled conditions, and their often ineffectiveness in the open field (Dobbelaere et al., 2003; Dal Cortivo et al., 2017), possibly due to the use of strains unable to adequately colonize plant roots and/or to compete with the resident rhizobiome (Lugtenberg and Dekkers, 1999; Bloemberg and Lugtenberg, 2001; Kamilova et al., 2005). Aiding microorganisms need to be introduced into agroecosystems in sufficient quantities to efficiently colonize plant roots, as this step is crucial for their success (Rao, 1993; Bishnoi, 2015). Following biofertilization, it can be expected positive or negative competition with the indigenous bacteria population, or no interaction, depending on survival strategies (Schwieger and Tebbe, 2000; Bacilio-Jimenez et al., 2001; Brimecombe et al., 2007). In this regard, essential bacterial behaviors include tolerance to nutrient- or water-limited conditions, affinity for root exudates, and competition with the resident rhizobacteria through the secretion of antibiotics (Paul and Nair, 2008; Nadeem et al., 2013; Kaur et al., 2017). Little information is as yet available on the effects of PGPR-AMF consortia introduced into the resident bacterial community structure. Effective use of biofertilizers therefore rests on a better understanding of their effects on soil microbial communities, and hence of the role they play in soil biodiversity and plant health (Roesti et al., 2006; Hart et al., 2017).
Previous studies have shown the positive effects of PGPR on plant productivity, particularly under stress conditions, suggesting their potential role in a climate change scenario where extreme events, such as floods and droughts, occur with greater frequency in cultivated land (Egamberdieva and Adesemoye, 2016). However, little is so far known of their effects on the quality of cereal grains.
Our previous investigations in open fields documented the ability of a N-fixing bacteria consortium (i.e., Azospirillum + Azoarcus + Azorhizobium), when applied as foliar spraying inoculum during tillering, to improve root growth and N accumulation in common wheat (Dal Cortivo et al., 2017). Similarly, a AMF-bacteria consortium of Rhizophagus irregularis + Azotobacter vinelandii was found to enhance root growth and mineral uptake in this crop (Dal Cortivo et al., 2018).
In this framework, the current multidisciplinary study investigated a spectrum of effects of different commercial biofertilizers (consisting of PGPRs alone or in association with AMFs) on rhizosphere enzymatic processes, microbial biomass, and biodiversity (using high-throughput next-generation sequencing—NGS), and on growth, grain yield and quality (gluten content and composition, not previously investigated) in common wheat. The aim was to gather information on the potential advantages of their use as seed inoculums instead of postemergence foliar spraying on a widely-cultivated crop and on the mechanisms involved, and to assess safety issues with respect to their interaction with the resident rhizobiome.
The following three biofertilizers were applied to fungicide-free seeds of common wheat (Triticum aestivum L.) immediately before sowing: (i) TN: TripleN® (Mapleton Agri Biotec, Mapleton, Australia), at 0.02 g kg-1 of seeds, containing three PGPRs Azorhizobium spp., Azoarcus spp., and Azospirillum spp. (1×1010 CFU g-1); (ii) R-N: Rhizosum N® (Biosum Technology, Madrid, Spain), at 0.25 g kg-1 of seeds, containing the AMF Rhizophagus irregularis (previously known as Glomus intraradices) (2% w/w) and Azotobacter vinelandii (1×1010 CFU g-1); and (iii) R-PK: Rhizosum PK® (Biosum Technology, Madrid, Spain), at 0.375 g kg-1 of seeds, containing the AMF Rhizophagus irregularis (2% w/w) together with Bacillus megaterium (0.66 ×1010 CFU g-1) and Frateuria aurantia (0.33 ×1010 CFU g-1). The inoculum doses followed the manufacturers' recommendations. The freeze-dried inoculum was mixed with ultrapure water (10 ml kg-1 of seeds) in order to enhance its adherence to the seed surface, and 5 ml kg-1 of seeds of Delfan Plus (Tradecorp, Madrid, Spain), which contains amino acids for early bacterial activation.
The experiment was carried out in open field at the experimental farm of the University of Padua (Legnaro, Padua, NE Italy). The site has a deep, silty-loam soil (fulvi-calcaric-cambisol; USDA classification), pH 8.0, 1.7% organic matter, a CEC of 11.4 cmol(+) kg-1, and a total N content of 1.1 g kg-1 (arable layer, beginning of the experiment). As regards other soil nutrients, available P and K were moderately high: 15.48 and 97 mg kg-1, respectively; Mg was 247 mg kg-1 and Ca 2,619 mg kg-1.
The three biofertilizers were compared with untreated controls (CO) in a completely randomized block experimental design (n = 3). Each plot/replicate measured 30 m2 (10 × 3 m), and contained 24 rows of plants 12 cm apart. The previous crop was sugar beet. Soil tillage included 30-cm deep plowing followed by two harrowings. Presowing fertilization consisted in incorporating 32 kg ha-1 of N, 96 of P2O5 and 96 of K2O into the soil through harrowing. The total amount of N, supplied throughout the crop cycle was 160 kg ha-1 as ammonium nitrate. The wheat var. Bologna (SIS, Bologna, Italy) was sown on November 3, 2016, and harvested on June 22, 2017. The crop was protected against weeds, insects and fungal pathogens by specific treatments, following local recommendations. To preserve mycorrhizal fungi's survival, plants were protected from fungal pathogens at the heading stage using active ingredients (i.e., Cyproconazole, Azoxystrobin and Prochloraz) recognized as nonharmful to AMF (Plant Health Care Incorporation, 2009).
Leaf chlorophyll content was monitored twice during the growing cycle, at stem elongation (GS35) (Zadoks et al., 1974) and complete ear emergence (GS59), with a SPAD 502 chlorophyll meter (Konica-Minolta, Hong Kong) on the last fully developed leaf of the main culm (10 plants per plot/replicate). The monitored plants were then harvested to determine fresh and dry shoot biomass (after oven-drying for 36 h at 105°C). Shoot N content was also determined according to the Kjeldahl method, and Ca, K, P, Fe, Mg, and Zn concentrations by inductively coupled plasma-optical emission spectroscopy (ICP-OES) (SPECTRO CirOS Vision EOP, SPECTRO Analytical Instruments GmbH, Kleve, Germany) on 0.4-g microwave acid-digested (7 ml HNO3 65% v/v and 1 ml H2O2 30% v/v) samples (Mileston ETHOS 900, Bergamo, Italy) according to the EPA method 3052 (USEPA, 1995). Measurement accuracy was ensured with certified reference materials (ERM-CD281 and BRC-402; JRC-IRMM, Geel, Belgium).
Root colonization across a 1-m-deep soil profile was assessed at full flowering (GS65) on May 3, 2017 using the coring method (n = 3; one core per plot). Soil cores 70 mm in diameter were collected from a central row of the plot at least 1 m from the border. Each core was split into 0.1 m subsamples, which were frozen at –18°C until washing. After separation by a hydraulic sieving-centrifugation device on a 500-µm mesh sieve, the roots were stored in a 15% v/v ethanol solution at 4°C until digitalization. Root images were processed with the KS 300 Rel. 3.0 software (Karl Zeiss, Munich, Germany), using a minimum area of 40 pixels as the threshold for background noise. Root length was determined by the FbL (FiberLength) algorithm, and the mean root diameter as the area-to-length ratio of root objects in each sample (Vamerali et al., 2003). Root length density (RLD) was expressed as cm of root per cm3 of soil, and root surface density (RSD) as cm2 of root per cm3 of soil.
Wheat grain yield was measured at maturity in the central area of each plot (n = 3) by collecting the grains with a plot combine harvester. The harvest index (grain-to-total shoot weight ratio) was determined in a checking area of 1 m2 in each plot, together with the 1,000-kernel weight.
Gluten proteins were analyzed in 30-g seed samples (n = 3) gently milled by six pulses of 10 s each with a Knifetec 1095 (Foss, Hillerod, Denmark). Gliadins, high-molecular-weight glutenins (HMW-GS), and low-molecular-weight glutenin subunits (LWM-GS) were sequentially extracted from 30-mg subsamples, according to Visioli et al. (2018a) protocol. Relative quantification of HMW-GS, LMW-GS, and gliadins was performed spectrophotometrically by colorimetric Bradford assay (Bio-Rad, Hercules, CA, USA) at the 595 nm wavelength, with three technical replicates for each sample. Linear regression between absorbance and protein concentration was obtained through calibration with BSA (Bovine Serum Albumin) standards, and the results expressed as mg g-1 of wheat flour. Proteins belonging to the three gluten fractions were also separated by SDS-PAGE and subjected to densitometric analyses to evaluate possible variations in the amounts of single protein subunits, and the results expressed as mg/g-1 of flour (Visioli et al., 2018a).
Microbial biomass, enzymatic activity, and bacterial biodiversity were evaluated in the wheat rhizosphere (Rh) and in bulk soil (BS) collected on December 16, 2016. Soil monoliths 0.2 m deep containing the root system of ∼30 plants for each biological replicate were randomly taken from the central area of each plot. Plants were gently extracted from the ground, and after removing most of the soil by shaking, the remaining rhizosphere soil adhering to the roots was carefully collected with a small sterile brush. The rhizosphere soil samples from the 30 plants of each replicate were pooled to obtain one sample of >2 g, which was placed in a sterile Falcon tube and immediately stored at –80°C until analysis. The reference bulk soil sample, without wheat roots, was collected from a 0.2-m-deep profile in uncultivated zones between the plots.
Soil microbial biomass was determined as double-strand DNA (dsDNA) content (Bragato et al., 2016) in 300-mg DW soil samples through DNA extraction with a 0.12 M, pH 8 Na2HPO4 buffer using bead beating and quantification with PicoGreen reagent, as described by Fornasier et al. (2014). Biomass was expressed as µg dsDNA g-1 soil D. W.
Nineteen types of enzymatic activity representing the various nutrient cycles were then determined in treated wheat and compared with untreated controls and bulk soil (n = 3). The enzymes examined were: arylsulfatase (aryS), alpha-glucosidase (alfaG), beta-glucosidase (betaG), alpha-galactosidase (alfa GAL), beta-galactosidase (beta GAL), alpha-mannosidase (alfa_MAN), beta-mannosidase (beta_MAN), glucuronidase (uroni), cellobiohydrolase (cell), xilosidase (xilo), chitinase (chit), leucine-aminopeptidase (leu), tripsin- and papain-like protease (trip), acid phosphomonoesterase (acP), phosphodiesterase (bisP), pirophosphate-phosphodiesterase (piroP), alkaline phosphomonoesterase (alkP), inositol-phosphatase (phytase) (inosit), and nonanoate-esterase (nona). Their activity was measured by a heteromolecular exchange procedure (Cowie et al., 2013) using a solution of lysozyme (3%) and bead beating, as described in Bardelli et al. (2017). All activities were determined in 300-mg DW soil samples and expressed as nmol of MUF (4-methyl-umbelliferyl) min−1 g−1 soil DW.
DNA was extracted from 0.5-g samples of Rh and BS with the FastDNA® Spin Kit for Soil (MP Biomedicals, Santa Ana, CA, USA) according to the manufacturer's protocols, visualized by electrophoresis on 0.8% (w/v) agarose gels to test for DNA integrity, and quantified by Nanodrop ND1000. 16S rDNA amplification, sequencing and data analysis were performed at GenProbio srl's DNA sequencing facility (www.genprobio.com), according to the protocol described by Visioli et al. (2018b).
Differences between samples in the relative abundances of the taxonomic units were ascertained by a one-way Analysis of Variance (ANOVA). Bacterial taxa with P-values <0.05 were selected and identified as the phylotypes or bacterial families that were significantly influenced by the biofertilizers being tested. Pyrosequencing reads were sent to GenBank to obtain their under accession numbers. They are available as bioproject PRJNA388660.
An ANOVA was carried out on the dataset for all the parameters examined using the Statgraphics Centurion XI software (Adalta, Arezzo, Italy). Separation of means was set at P ≤ 0.05 with the Newman-Keuls test.
To facilitate interpretation of the whole dataset, a factorial discriminant analysis (MDA, Multigroup Discriminant Analysis with Wilks' lambda and Pillai's trace tests) and a principal component analysis (PCA) were carried out to describe the plant- and microbiologically-related variables. Multivariate data normality was first verified by the Shapiro test. Before analysis, the data were standardized by subtracting the mean and dividing by the standard deviation within each variable. All analyses were performed with MS Excel XLSTAT (Addinsoft, Paris, France).
The climatic parameters recorded by the local meteorological station (ARPAV, Teolo, Italy) during the field trial showed that the average monthly temperature was quite similar to the 10-year mean (2007–2017), but large differences were found for precipitation. Compared with the historical mean, temperatures were lower in December and January, while rainfall was higher in November but markedly lower for the rest of the cycle, particularly during the winter (Figure SI 1). From December to June, overall precipitation was only 170 mm.
As expected, the soil microbial biomass in cultivated soil was greater than in bulk soil. There was also greater microbial biomass in the rhizosphere of the inoculated plants than in that of the noninoculated controls, particularly with R-PK, although the difference was not statistically significant (Table 1).
Table 1 Total microbial biomass (ug dsDNA g-1 dry soil; n = 3 ± S.E.) and enzymatic activity (nmol of MUF min-1 g-1 dry soil; n = 3 ± S.E.) in bulk soil (BS), and in the rhizosphere of Triticum aestivum inoculated with biofertilizers (TN, TripleN; R-N, Rhizosum N; R-PK, Rhizosum PK) vs untreated controls (CO).
The levels of activity of many of the rhizosphere enzymes investigated here were higher in inoculated plants than in bulk soil, but they were also seldom higher than in the noninoculated controls. The soil microbial enzymatic activity response allowed us to clearly separate treatments by principal coordinate analysis (PCoA) (Figure 1), which showed that the R-N and R-PK treatments were more distant from the untreated controls (CO). The two dimensions/variables of db-RDA (distance-based redundancy analysis) explained an overall variability of 81%, mostly attributed to the first (db-RDA1 = 59%, P = 0.004) than to the second variable (db-RDA2 = 21%, P = 0.292).
Figure 1 Overall differences among rhizosphere hydrolytic enzyme activities determined by distance-based redundancy analysis (dbRDA) ordination among treatments (TN, TripleN; R-N, Rhizosum N; R-PK, Rhizosum PK) and noninoculated controls (CO). Percentages along the axes show the proportions of dissimilarity captured.
Comparison among the rhizospheres of the controls and treated plants showed that the enzymatic activity of beta-glucosidase, α-mannosidase, β;-mannosidase, and xylosidase was significantly higher with R-N and R-PK, i.e., the treatments including plant-aiding fungi, than in the biofertilizer containing only PGP rhizobacteria. The activity of the alkaline phosphomonoesterase was also significantly higher in treated plants, particularly with TN and R-N, and nonanoate-esterase activity was considerably enhanced with R-PK (Table 1).
Regarding bacterial biodiversity in the rhizosphere and the bulk soil, the total number of gene sequences (average of three replicates) ranged from 54,929 to 59,843. Bacterial diversity, measured as OTUs (Operational Taxonomic Units), and the calculated bacterial diversity indices, i.e., the Shannon diversity index and the Chao1 estimator of richness, revealed no significant differences among treatments. The rarefaction curves using the Chao and Shannon indices approached the plateau, indicating that further sequencing would not have resulted in additional OTUs (Figure SI 2). Differences in the microbial rhizosphere compositions were detected at both the phylum and family levels (Figure 2, Table SI 1). The major differences were between bulk soil and the rhizosphere, confirming the significant impact of soil root colonization on microbiota development. In particular, the Bacteroidetes, Cyanobacteria, Proteobacteria, and Fibrobacteres phyla were significantly more abundant in the rhizosphere, and Actinobacteria, Acidobacteria, Gemmatimonadetes, and Chloroflexi in the bulk soil (Figure 3). Some differences were also found between the rhizospheres of the controls and the inoculated plants: seed treatment with R-PK greatly stimulated the abundance of Cyanobacteria (+ 24% vs controls), TN the bacteria of the Flavobacteriaceae family, which reached 3.34% of the total community vs 1.99% of controls, and R-N the Planctomycetaceae family (Table SI 1). Compared with controls, R-PK reduced the abundance of the Verrucomicrobiaceae family, and R-N the Gaiellaceae, although these were present at <1%.
Figure 2 Microbial community composition (%; n = 3 ± S.E.) at the phylum level based on 16S rDNA reads in bulk soil (BS) and the rhizosphere of Triticum aestivum inoculated with biofertilizers (TN, TripleN; R-N, Rhizosum N; R-PK, Rhizosum PK) and noninoculated controls (CO) (Newman-Keuls test, P ≤ 0.05). Only taxa >0.6% are shown.
Figure 3 Shoot fresh (A) and dry (B) weight, leaf chlorophyll content (C), and shoot nitrogen content (D) (n = 3 ± S.E.) in Triticum aestivum plants inoculated with biofertilizers (TN, TripleN; R-N, Rhizosum N; R-PK, Rhizosum PK) vs untreated controls (CO). Letters indicate statistically significant differences among treatments (Newman-Keuls test, P ≤ 0.05).
Wheat plant growth was appreciably enhanced following seed inoculation with biofertilizers, as evidenced by the greater shoot biomass at both the stem elongation (GS35, fifth node detectable) and complete ear emergence (GS59) stages, although on the second observation the R-PK treatment was the only significant (P ≤ 0.05) (Figures 3A, B). Leaf chlorophyll content of the youngest developed leaf, which decreased with plant aging from stem elongation to heading, was not affected by seed inoculation, although it was slightly improved by R-N and R-PK (Figure 3C). However, inoculated plants accumulated a greater amount of nitrogen above ground as a result of both greater biomass and nitrogen concentration, with significant improvements for all treatments at stem elongation, and for R-PK at the heading stage (Figure 3D). At this stage, the shoot concentrations of other nutrients (i.e., K, P, Ca, Mg, Fe, and Zn) were generally lower with plant inoculation, but the overall content (uptake) of Ca, K and Zn improved significantly, particularly with R-PK, due to better plant growth (Table 2).
Table 2 Shoot element (Ca, Calcium; Fe, Iron; K, Potassium; Mg, Magnesium; P, Phosphorus; Zn, Zinc) concentrations and contents (mean ± S.E.; n = 3) at the heading stage of Triticum aestivum inoculated with biofertilizers (TN, TripleN; R-N, Rhizosum N; R-PK, Rhizosum PK) vs untreated controls (CO).
Wheat yield and its components, i.e., the harvest index (HI), and thousand kernel weight (TKW), were very stable across treatments, with small nonsignificant improvements in the inoculated wheat (P > 0.05), mainly with R-PK. The average values were: HI = 43%, TKW = 31 g, and yield = 756 g m-2.
Destructive root investigations at complete flowering revealed similar root patterns among the inoculated plants and controls in terms of the average whole root profile. However, significant benefits in root length density (RLD) and root area density (RAD) were found in the arable layer with the TN treatment (P > 0.05). On the other hand, the two biofertilizers containing AMF (i.e., R-N and R-PK) led to slight decreases in root length, area and diameter (Table 3).
Table 3 Root length density (RLD), root surface density (RSD), and diameter (D) (mean ± S.E.; n = 3) at the flowering stage as averages of different soil layers in Triticum aestivum inoculated with biofertilizers (TN, TripleN; R-N, Rhizosum N; R-PK, Rhizosum PK) vs noninoculated controls (CO).
Biofertilizers had no significant effects on flour gluten content, which was similar with noninoculated controls, although there was a general slight increase in gliadins and a reduction in glutenins, particularly the high-molecular-weight glutenin subunits (HMW-GS) (Table 4). Maximum variations were found with the R-PK treatment, which resulted in a 7% increase in gliadins, and an 8% reduction in glutenins. SDS-PAGE revealed that all the inocula led to a significant increase in the 81 kDa HMW-GS and the 43,6 kDa LMW-GS (Figures 4A, B), both playing a key role in technological quality. No significant changes in the composition of the gliadin subunits were observed (Figure 4C).
Table 4 Total gliadins, low-molecular-weight (LMW-GS) and high-molecular-weight glutenin subunits (HMW-GS), total glutenins (mg g-1; n = 3; ± S.E.) and glutenin/gliadin and HMW/LMW ratios in grains of Triticum aestivum inoculated with biofertilizers (TN, TripleN; R-N, Rhizosum N; R-PK, Rhizosum PK) vs noninoculated controls (CO).
Figure 4 High-molecular-weight glutenin (HMW-GS, A), low-molecular-weight glutenin subunits (LMW-GS, B), and gliadins (C) (mg g-1 D. W. flour; n = 3; mean ± S.E.) as represented by different kDa bands revealed with SDS-PAGE in Triticum aestivum plants inoculated with biofertilizers (TN, TripleN; R-N, Rhizosum N; R-PK, Rhizosum PK) vs noninoculated controls (CO). Letters indicate significant differences among treatments within the same band (Newman-Keuls test, P ≤ 0.05).
The PCA identified two synthetic components which explained an overall variability of 99.85%, mostly attributed to the first one (F1 = 95.16%; F2 = 4.69%) (Figure 5). Most of the relevant variables (loadings > |0.4|) i.e., gluten subunits, rhizosphere bacterial composition and shoot growth were assigned to F2, while gliadins and root parameters (both length and surface area density) were the most representative ones in F1.
Figure 5 Principal component analysis (PCA; top right) with variable loadings (values > |0.4| in bold; bottom) and discriminant analysis (DA; top left) for Triticum aestivum inoculated with biofertilizers (TN, TripleN; R-N, Rhizosum N; R-PK, Rhizosum PK) vs noninoculated controls (CO). Circles in the PCA include 75% of cases.
The direction of the vector of each variable indicated generally good correlations among the variables plotted very closely together, i.e., SPAD, shoot biomass, and shoot nitrogen uptake, which were negatively correlated with the abundances of the bacterial phyla Acidobacteria, Proteobacteria, and Actinobacteria. The abundance of Cyanobacteria was correlated with LMW-GS and gliadin contents, while HMW-GS content was correlated with root growth and the abundances of Proteobacteria and Actinobacteria.
The centroid position and cluster separation in the discriminant analysis (Figure 5) summarize wheat response to the three biofertilizers, and show that TN and R-N treatments promoted root growth, while R-PK mainly affected gluten composition. In this way, the PCA highlighted the effects on root parameters as the most relevant impact of bacterial inoculation.
Biofertilizers represent a sustainable tool for improving crop yield, as beneficial bacteria and fungi can exert several positive effects on plant nutrition and growth in many crops, including wheat (Basu et al., 2017; Igiehon and Babalola, 2017). Although this is generally clear under laboratory and controlled conditions, the application of plant-aiding microorganisms in open field may be constrained by poor agronomic response. Two of the biofertilizers studied here, i.e., TN composed of a bacteria consortium (Azospirillum spp. + Azoarcus spp. + Azorhizobium spp.), and R-N, a fungal-bacterial consortium (Rhizophagus irregularis + Azotobacter vinelandii), have already been successfully applied in open field by foliar spraying before stem elongation (Dal Cortivo et al., 2017; Dal Cortivo et al., 2018). In the present study, the biofertilizers were applied as seed inoculants, which is less costly than canopy spraying, with the aim to investigate their potential agronomic effects together with the environmental/ecological impact, which is related to the interaction with the resident microbial community, and the possible mechanisms of interaction with the wheat plants. Using this application method, we expect endophytic PGPR to colonize the root surface and intercellularly colonize the internal plant tissues of different plant organs, and AMF to colonize the plant roots starting with the first rootlet of the germinating seeds, thereby contributing to plant nutrition and growth (Lodewyckx et al., 2002; Smith and Read, 2008; Pagnani et al., 2020). Indeed, efficient colonization of root tissues by PGPR bacteria included in TN, as well as by Rhizophagus irregularis contained in R-N and R-PK, has been already documented by electron microscopy (ESEM) in our previous studies (Dal Cortivo et al., 2017; Dal Cortivo et al., 2018). This fits with the appreciable increases in shoot growth and accumulation of minerals, particularly nitrogen, detected across the growing season, although these did not translate into significant increases in wheat yield in our field experiment. The different plant responses may be attributed to the microbial composition of the biofertilizers, as TN mainly stimulated root growth, while R-N and R-PK enhanced the uptake of low-mobile nutrients, such as Ca, K, and Zn, mainly through improved plant growth.
The spectrum of effects of seed inoculation on wheat reported here are similar to previous literature dealing with various growing conditions (Turan et al., 2012; Piccinin et al., 2013; Nadeem et al., 2014). Growth enhancements may be attributed to the N-fixing and nutrient-solubilizing abilities of the applied microorganisms, and to the production of growth-promoting substances, such as IAA (indole-3-acetic acid). PGPR can also modify the level of phytohormones involved in plant senescence through the production of the enzyme ACC-deaminase, and toxins, like rhizobitoxine, which limits ethylene synthesis (Stamenković et al., 2018).
As a result of the associations among a mycorrhizal fungus and P- and K-solubilizing bacteria, such as Bacillus megaterium and Frateuria aurantia (Elkoca et al., 2010; Velázquez et al., 2016; Ezawa and Saito, 2018), the R-PK treatment was found to engender the best plant growth and nutrient uptake responses, but seldom together with a reduction in nutrient concentrations in the plant tissues, although this is expected where soil fertility is high (Richardson et al., 2009). Mycorrhizal fungi are known to form a highly-developed hyphal network that absorbs nutrients, particularly phosphates, up to several centimeters from the root adsorption zone, often leading to a decrease in fine root length (Liu, 2009; Ezawa and Saito, 2018), as in this trial.
We found a clear positive effect on root growth of inoculation with TN, resulting in greater volumetric root length density in the arable layer. In fact, Azospirillum bacteria, included in the formulation of TN, are recognized as stimulating root length and area through the release of auxins, thereby increasing nitrogen and low-mobile nutrients, and water uptake (Bhattacharyya and Jha, 2012). Several studies have reported root growth enhancements, particularly at the early plant stages, but this may be not a stable response in mature plants and across years, as the PGPR-plant association in open field is strongly affected by adverse environmental conditions (e.g., excessive precipitation) after soil/plant inoculation (Basaglia et al., 2003; Dal Cortivo et al., 2017). However, this experiment confirms that soil conditions and fertility were probably favorable to the onset of the plant-PGPR signals that precede colonization in competition with the resident microbiome (Videira e Castro et al., 2016), allowing wheat to benefit in terms of root growth.
Wheat seed inoculation also had a clear positive impact on the rhizosphere microbiome, at least relatively soon after sowing (about 6 weeks). There was a general increase in total microbial biomass and some soil enzymatic activities, demonstrating enhanced microbial metabolism, mainly when the inoculum contained both plant-aiding bacteria and AMF. The increased enzymatic activities included beta-glucosidase, which hydrolyzes cellobiose to free glucose (Zang et al., 2018), alfa-mannosidase, beta-mannosidase, and xylosidase, which is produced by both endophytic bacteria and fungi, and which hydrolyzes mannans and xylans as the main components of lignified organic materials together with cellulose and lignin (Nankai et al., 2002; Robl et al., 2013). Alkaline nonophosphoesterase, a key enzyme for organic P degradation into inorganic phosphate available for plant uptake, particularly under P-limiting conditions (Acuña et al., 2016), was also generally upregulated by inoculation. Nonanoate-esterase activity was increased significantly by the R-PK treatment, although other contributing enzymes act on ester bonds, including esterases and proteases.
An important issue to be considered in plant/soil inoculation is the possible impact on the resident microbiome. In our study, there was a considerable higher bacterial biodiversity in the rhizosphere compared with bulk soil, confirming the essential role played by root presence in the soil microbiome. On the other hand, we found small differences between the rhizospheres of the treated plants vs the noninoculated controls in terms of bacterial biodiversity, suggesting that all the inocula applications studied here are safe. Nevertheless, inoculation modified the abundances of specific rhizosphere bacteria phyla. Interestingly, the R-PK inoculum highly stimulated the Cyanobacteria, which are known to form associations with wheat roots to alleviate nitrogen deficiency and enhance the rhizosphere microbial biomass (Karthikeyan et al., 2007). As Cyanobacteria are also able to produce plant growth-promoting substances, it has been suggested they be included as inoculants for rice and maize (Prasanna et al., 2016). Bacteria belonging to the Flavobacteriaceae family, which were stimulated by the TN treatment, may also be beneficial for wheat in this experiment, as they proved to have important ecological functions contributing to organic matter turnover and pesticide decomposition (Wolińska et al., 2017), which in part helps explain the increase in some soil enzymatic activities (Table 1).
The main agronomic result obtained in this study was the greater nitrogen uptake in inoculated plants, although this did not translate into significant gains in grain yield, and no effects on grain quality in terms of gluten content were observed, in contrast with the findings of some authors which tested the effects of PGPR inoculation in ancient Triticum varieties (Pagnani et al., 2020). Accumulation of gluten proteins is a complex process involving spatial and temporal regulation. Environmental conditions, such as heat and drought stress, as well as the dose and application timing of nitrogen fertilizers can affect significant changes in gluten composition (Flagella et al., 2010; Visioli et al., 2018a). However, very little information is available on possible changes in gluten protein composition in response to biofertilizer application. Stępień and Wojtkowiak (2013) showed that gluten content, particularly of HMW-GS and LMW-GS, increased in spring and winter wheat cultivars with organic fertilizers, regardless of the addition of effective microorganisms. A novel finding in this study is the significant upregulation of certain LMW-GS and HMW-GS, the latter being polymeric proteins involved in dough strength and elasticity (Sissons, 2008). In particular, the 81 kDa HMW-GS is the Bx subunit codified by the locus Glu-B1 closely correlated with the technological quality of flour (Sissons, 2008), and the LMW-2 asset, in which the LMW-GS with the highest molecular weight (~44 kDa) is the most abundant subunit of this class (D'Ovidio and Masci, 2004) and is generally upregulated by N supply (Visioli et al., 2018a). Hence, the upregulation of both 81 kDa HMW-GS and 43.6 kDa LMW-GS with all the biofertilizers tested, particularly R-PK, may be attributed to the N-fixing and nutrient-solubilizing contribution of the applied microorganisms or the changes induced in the bacteria groups during grain filling.
This study has shown that bacteria or bacteria-AMF consortia tested can be safely applied as seed inocula, as they did not alter the bacterial taxa associated with wheat roots allowing the resident microbial biodiversity to be preserved. The benefits of seed inoculation included enhancement of the rhizosphere microbial biomass and of the activity of enzymes involved in organic matter decomposition and nutrient release, especially when the biofertilizer contains the AMF Rhizophagus irregularis. We confirmed the importance of diazotrophic bacteria (i.e., Azoarcus, Azospirillum, Azorhizobium) in enhancing plant nitrogen nutrition and root growth. Despite with moderate effects, the biofertilizers tested here are also expected to alleviate nutrient deficiency, particularly P and Fe, which is of great importance in the worldwide spread alkaline soils, like in our site. Seed inoculation is therefore suitable for aiding the fertilization of wheat, and possibly of other cereals in organic agriculture, and may also provide beneficial environmental effects in reducing N losses.
We did not find any improvements in grain yield, although significant agronomic benefits in poor soil, low-input agriculture or under abiotic stress conditions cannot be excluded. A new finding was the increase in gluten quality due to upregulation of specific gluten protein subunits, which can be exploited in the wheat food chain.
Currently, the most suitable option seems to be the association between bacteria and AMF, but future research is required to gain further specific insights into biofertilizer composition in order to exploit the synergistic action of various bacteria and mycorrhizal fungi, and understand their behavior under reduced soil resource availability.
The sequencing data generated by this study can be found in the NCBI under accession number bioproject PRJNA388660.
TV conceived and designed the experiment, and revised the MS. CC and MF carried out the experiment and analyzed the data. GV and ML carried out gluten protein analyses and metagenomics analyses, and FF performed soil enzymatic activities analysis. GB performed the statistical analysis of data. CC wrote the first draft of the paper. AP contributed to improve the Introduction and the Discussion sections. All authors contributed to interpreting and discussing the results, and read and approved the final version of the manuscript.
The authors declare that the research was conducted in the absence of any commercial or financial relationships that could be construed as a potential conflict of interest.
The authors wish to thank Adriano Massignan, Francesco Munari, and Simone Cassanego for helping with data collection, and Tessa Say for revising the English text. East Balt Italia Bakeries is gratefully acknowledged for the financial support.
The Supplementary Material for this article can be found online at: https://www.frontiersin.org/articles/10.3389/fpls.2020.00072/full#supplementary-material
Acuña, J. J., Durán, P., Lagos, L. M., Ogram, A., de la Luz Mora, M., Jorquera, M. A. (2016). Bacterial alkaline phosphomonoesterase in the rhizospheres of plants grown in Chilean extreme environments. Biol. Fertil. Soils 52, 763–773. doi: 10.1007/s00374-016-1113-9
Afzal, A., Bano, A. (2008). Rhizobium and phosphate solubilizing bacteria improve the yield and phosphorus uptake in wheat (Triticum aestivum). Int. J. Agric. Biol. 10 (1), 85–88. doi: 07-092/MFA/2008/10–1–85–88
Ahmad, F., Husain, F. M., Ahmad, I. (2011). “Rhizosphere and Root Colonization by Bacterial Inoculants and Their Monitoring Methods: A Critical Area in PGPR Research,” in Microbes and Microbial Technology. Eds. Ahmad, I., Ahmad, F., Pichtel, J. (New York, NY: Springer). doi: 10.1007/978-1-4419-7931-5_14
Bacilio-Jimenez, M., Aguilar-Flores, S., del Valle, M. V., Prez, A., Zepeda, A., Zenteno, E. (2001). Endophytic bacteria in rice seeds inhibit early colonization of roots by Azospirillum brasilense. Soil Biol. Biochem. 33, 167–172. doi: 10.1016/S0038-0717(00)00126-7
Bardelli, T., Gómez-Brandón, M., Ascher-Jenull, J., Fornasier, F., Arfaioli, P., Francioli, D., et al. (2017). Effects of slope exposure on soil physico-chemical and microbiologicalproperties along an altitudinal climosequence in the Italian Alps. Sci. Total Environ. 575, 1041–1055. doi: 10.1016/j.scitotenv.2016.09.176
Basaglia, M., Casella, S., Peruch, U., Poggiolini, S., Vamerali, T., Mosca, G., et al. (2003). Field release of genetically marked Azospirillum brasilense in association with Sorghum bicolor L. Plant Soil 256, 281–290. doi: 10.1023/A:1026198522123
Basu, S., Rabara, R., Negi, S. (2017). Towards a better greener future - an alternative strategy using biofertilizers. I: Plant growth promoting bacteria. Plant Gene 12, 43–49. doi: 10.1016/j.plgene.2017.07.004
Begum, N., Qin, C., Ahanger, M. A., Raza, S., Khan, M. I., Ashraf, M., et al. (2019). Role of Arbuscular Mycorrhizal fungi in plant growth regulation: implications in abiotic stress tolerance. Front. Plant Sci. 10, 1068. doi: 10.3389/fpls.2019.01068
Bhattacharyya, P. N., Jha, D. K. (2012). Plant growth-promoting rhizobacteria (PGPR): emergence in agriculture. World J. Microbiol. Biotechnol. 28, 1327–1350. doi: 10.1007/s11274-011-0979-9
Bishnoi, U. (2015). PGPR interaction: an ecofriendly approach promoting the sustainable agriculture system. Adv. Bot. Res. 75, 81–113. doi: 10.1016/bs.abr.2015.09.006
Bloemberg, G. V., Lugtenberg, B. J. J. (2001). Molecular basis of plant growth promotion and biocontrol by rhizobacteria. Curr. Opin. Plant Biol. 4, 343–350. doi: 10.1016/S1369-5266(00)00183-7
Bragato, G., Fornasier, F., Brus, D. (2016). Characterization of soil fertility and soil biodiversity with dsDNA as a covariate in a regression estimator for mean microbial biomass. Eur. J. Soil Sci. 67, 827–834. doi: 10.1111/ejss.12387
Brimecombe, M. J., De Leij, F. A. A. M., Lynch, J. M. (2007). “Rhizodeposition and microbial populations,” in The rhizosphere: Biochemistry and organic substances at the soil-plant interface. Eds. Pinton, R., Varanini, Z., Nannipieri, P. (Boca Raton, USA: CRC Press), 73–109. doi: 10.1201/9781420005585.ch3
Castro-Sowinski, S., Herschkovitz, Y., Okon, Y., Jurkevitch, E. (2007). Effects of inoculation with plant growth-promoting rhizobacteria on resident rhizosphere microorganisms. FEMS Microbiol. Lett. 276, 1–11. doi: 10.1111/j.1574-6968.2007.00878.x
Chauhan, H. S., Bagyaraj, D. J., Selvakumar, G., Sundaram, S. P. (2015). Novel plant growth promoting rhizobacteria-Prospects and potential. Appl. Soil Ecol. 95, 38–53. doi: 10.1016/j.apsoil.2015.05.011
Cocking, E. C. (2003). Endophytic colonization of plant roots by nitrogen-fixing bacteria. Plant Soil 252 (1), 169–175. doi: 10.1023/A:1024106605806
Cowie, A., Lonergan, V. E., Rabbi, F. S. M., Fornasier, F., Macdonald, C., Harden, S., et al. (2013). The impact of carbon farming practices on soil carbon in northern New South Wales. Soil Res. 51 (8), 707–718. doi: 10.1071/SR13043
D'Ovidio, R., Masci, S. (2004). The low-molecular-weight glutenin subunits of wheat gluten. J. Cereal Sci. 39, 321–339. doi: 10.1016/j.jcs.2003.12.002
Dal Cortivo, C., Barion, G., Visioli, G., Mattarozzi, M., Mosca, G., Vamerali, T. (2017). Increased root growth and nitrogen accumulation in common wheat following PGPR inoculation: assessment of plant-microbe interactions by ESEM. Agric. Ecosyst. Environ. 247, 396–408. doi: 10.1016/j.agee.2017.07.006
Dal Cortivo, C., Barion, G., Ferrari, M., Visioli, G., Dramis, L., Panozzo, A., et al. (2018). Effects of field inoculation with VAM and bacteria consortia on root growth and nutrients uptake in common wheat. Sustainability 10, 3286. doi: 10.3390/su10093286
Dobbelaere, S., Vanderleyden, J., Okon, Y. (2003). Plant growth promoting effects of diazotrophs in the rhizosphere. CRC Crit. Rev. Plant Sci. 22, 107–149. doi: 10.1080/713610853
Egamberdieva, D., Adesemoye, A. O. (2016). “Improvement of crop protection and yield in hostile agroecological conditions with PGPR-based biofertilizer formulations,” in Bioformulations: For Sustainable Agriculture. Eds. Arora, N. K., Mehnaz, S., Balestrini, R. (New Delhi, India: Springer), 199–211. doi: 10.1007/978-81-322-2779-3_11
Elkoca, E., Turan, M., Donmez, M. F. (2010). Effects of single, dual and triple inoculations with Bacillus subtilis, Bacillus megaterium and Rhizobium leguminosarum bv. phaseoli on nodulation, nutrient uptake, yield and yield parameters of common bean (Phaseolus vulgaris L. cv. ‘elkoca-05'). J. Plant Nutr. 33 (14), 2104–2119. doi: 10.1080/01904167.2010.519084
Ezawa, T., Saito, K. (2018). How do arbuscular mycorrhizal fungi handle phosphate? New insight into fine-tuning of phosphate metabolism. New Phytol. 220 (4), 1116–1121. doi: 10.1111/nph.15187
Flagella, Z., Giuliani, M. M., Giuzio, L., Volpi, C., Masci, S. (2010). Influence of water deficit on durum wheat storage protein composition and technological quality. Eur. J. Agron. 33, 197–207. doi: 10.1016/j.eja.2010.05.006
Fornasier, F., Ascher, J., Ceccherini, M. T., Tomat, E., Pietramellara, G. (2014). A simplified rapid, low-cost and versatile DNA-based assessment of soil microbial biomass. Ecol. Indic. 45, 75–82. doi: 10.1016/j.ecolind.2014.03.028
Ghaffari, H., Gholizadeh, A., Biabani, A., Fallah, A., Mohammadian, M. (2018). Plant Growth Promoting Rhizobacteria (PGPR) application with different nitrogen fertilizer levels in rice (Oryza sativa L.). Pertanika J. Trop. Agric. Sci. 41 (2), 715–728.
Gupta, G., Parihar, S. S., Ahirwar, N. K., Snehi, S. K., Singh, V. (2015). Plant growth promoting rhizobacteria (PGPR): current and future prospects for development of sustainable agriculture. J. Microb. Biochem. Technol. 7, 96–102. doi: 10.4172/1948-5948.1000188
Hart, M. M., Antunes, P. M., Abbott, L. K. (2017). Unknown risks to soil biodiversity from commercial fungal inoculants. Nat. Ecol. Evol. 1, 0115. doi: 10.1038/s41559-017-0115
Hayat, R., Ali, S., Amara, U., Khalid, R., Iftikhar, A. (2010). Soil beneficial bacteria and their role in plant growth promotion: a review. Ann. Microbiol. 60, 579. doi: 10.1007/s13213-010-0117-1
Igiehon, N. O., Babalola, O. O. (2017). Biofertilizers and sustainable agriculture: exploring arbuscular mycorrhizal fungi. Appl. Microbiol. Biotechnol. 101, 4871–4881. doi: 10.1007/s00253-017-8344-z
Kamilova, F., Validov, S., Azarova, T., Mulders, I., Lugtenberg, B. (2005). Enrichment for enhanced competitive plant root tip colonizers selects for a new class of biocontrol bacteria. Environ. Microbiol. 7, 1809–1817. doi: 10.1111/j.1462-2920.2005.00889.x
Karthikeyan, N., Prasanna, R., Nain, L., Kaushik, B. D. (2007). Evaluating the potential of plant growth promoting cyanobacteria as inoculants for wheat. Eur. J. Soil Biol. 43, 23–30. doi: 10.1016/j.ejsobi.2006.11.001
Kaur, C., Selvakumar, G., Ganeshamurthy, A. N. (2017). “Rhizocompetence of applied bioinoculants,” in Plant-Microbe Interactions in Agro-Ecological Perspectives. Eds. Dhananjaya, P. S., Harikesh, B. S., Ratna, P. (Singapore: Springer), 501–511. doi: 10.1007/978-981-10-5813-4_25
Kloepper, J. W., Beauchamp, C. J. (1992). A review of issues related to measuring colonization of plant roots by bacteria. Can. J. Microbiol. 12, 1219–1232. doi: 10.1139/m92-202
Liu, W. (2009). Correlation between specific fine root length and mycorrhizal colonization of maize in different soil types. Front. Agric. China 3 (1), 13–15. doi: 10.1007/s11703-009-0004-3
Lodewyckx, C., Vangronsveld, J., Porteous, F., Moore, E. R. B., Taghavi, S., Mezgeay, M., et al. (2002). Endophytic bacteria and their potential applications. Crit. Rev. Plant Sci. 21, 583–606. doi: 10.1080/0735-260291044377
Lugtenberg, B. J. J., Dekkers, L. C. (1999). What makes Pseudomonas bacteria rhizosphere competent. Environ. Microbiol. 1, 9–13. doi: 10.1046/j.1462-2920.1999.00005.x
Meena, V. S., Maurya, B. R., Verma, J. P. (2014). Does a rhizospheric microorganism enhance K+ availability in agricultural soils? Microbiol. Res. 169, 337–347. doi: 10.1016/j.micres.2013.09.003
Mitra, D., Navendra, U., Panneerselvam, U., Ansuman, S., Ganeshamurthy, A. N., Divya, J. (2019). Role of mycorrhiza and its associated bacteria on plant growth promotion and nutrient management in sustainable agriculture. Int. J. Life Sci. Appl. Sci. 1, 1–10.
Nadeem, S. M., Naveed, M., Zahir, Z. A., Asghar, H. M. (2013). “Plant-microbe interactions for sustainable agriculture: fundamentals and recent advances,” in Plant Microbe Symbiosis: Fundamentals and Advances. Eds. Arora, N. K. (New Delhi, India: Springer), 51–103. doi: 10.1007/978-81-322-1287-4_2
Nadeem, S. M., Ahmad, M., Zahir, Z. A., Javaid, A., Ashraf, M. (2014). The role of mycorrhizae and plant growth promoting rhizobacteria (PGPR) in improving crop productivity under stressful environments. Biotechnol. Adv. 32, 429–448. doi: 10.1016/j.biotechadv.2013.12.005
Nankai, H., Hashimoto, W., Murata, K. (2002). Molecular identification of family 38 α-mannosidase of Bacillus sp. strain GL1, responsible for complete depolymerization of xanthan. Appl. Environ. Microbiol. 68 (6), 2731–2736. doi: 10.1128/AEM.68.6.2731-2736.2002
Owen, D., Williams, A. P., Griffith, G. W., Withers, P. J. A. (2015). Use of commercial bio-inoculants to increase agricultural production through improved phosphorus acquisition. Appl. Soil Ecol. 86, 41–54. doi: 10.1016/j.apsoil.2014.09.012
Pagnani, G., Galieni, A., Stagnari, F., Pellegrini, M., Del Gallo, M., Pisante, M. (2020). Open field inoculation with PGPR as a strategy to manage fertilization of ancient Triticum genotypes. Biol. Fert. Soils. 56, 111–124. doi: 10.1007/s00374-019-01407-1
Paul, D., Nair, S. (2008). Stress adaptations in a plant growth promoting rhizobacterium (PGPR) with increasing salinity in the coastal agricultural soils. J. Basic Microbiol. 48, 378–384. doi: 10.1002/jobm.200700365
Piccinin, G. G., Braccini, A. L., Dan, L. G. M., Scapim, C. A., Ricci, T. T., Bazo, G. L. (2013). Efficiency of seed inoculation with Azospirillum brasilense on agronomic characteristics and yield of wheat. Ind. Crop Prod. 43, 393–397. doi: 10.1016/j.indcrop.2012.07.052
Plant Health Care Incorporation. (2009). Effects of Fungicides on Mycorrhizal Fungi and Root Colonization. Available online: www.planthealthcare.com (accessed on 25 January 2017).
Prasanna, R., Kanchan, A., Ramakrishnan, B., Ranjan, K., Venkatachalam, S., Hossain, F., et al. (2016). Cyanobacteria-based bioinoculants influence growth and yields by modulating the microbial communities favourably in the rhizospheres of maize hybrids. Eur. J. Soil Biol. 75, 15–23. doi: 10.1016/j.ejsobi.2016.04.001
Rao, N. S. S. (1993). Biofertilizers in agriculture and forestry (New York, USA: International Science Publishers).
Richardson, A. E., Barea, J.-M., McNeill, A. M., Prigent-Combaret, C. (2009). Acquisition of phosphorus and nitrogen in the rhizosphere and plant growth promotion by microorganisms. Plant Soil 321, 305–339. doi: 10.1007/s11104-009-9895-2
Robertson, B. K., Dreyfus, B., Alexander, M. (1995). Ecology of stem-nodulating Rhizobium and Azorhizobium in four vegetation zones of Senegal. Microb. Ecol. 29, 71–81. doi: 10.1007/BF00217424
Robl, D., da Silva, Delabona, P., Montanari Mergel, C., Rojas, J. D., dos Santos Costa, P., Chapaval Pimentel, I., et al. (2013). The capability of endophytic fungi for production of hemicellulases and related enzymes. BMC Biotechnol. 13, 94. doi: 10.1186/1472-6750-13-94
Roesti, D., Gaur, R., Johri, B. N., Imfeld, G., Sharma, S., Kawaljeet, K., et al. (2006). Plant growth stage, fertiliser management and bio-inoculation of arbuscular mycorrhizal fungi and plant growth promoting rhizobacteria affect the rhizobacterial community structure in rain-fed wheat fields. Soil Biol. Biochem. 38, 1111–1120. doi: 10.1016/j.soilbio.2005.09.010
Schwieger, F., Tebbe, C. C. (2000). Effect of field inoculation with Sinorhizobium meliloti L33 on the composition of bacterial communities in rhizospheres of a target plant (Medicago sativa) and a non-target plant (Chenopodium album) - Linking of 16S rRNA gene-based single-strand conformation polymorphism community profiles to the diversity of cultivated bacteria. Appl. Environ. Microbiol. 66, 3556–3565. doi: 10.1128/AEM.66.8.3556-3565.2000
Shridhar, B. S. (2012). Review: nitrogen fixing microorganisms. Int. J. Microbiol. Res. 3 (1), 46–52. doi: 10.5829/idosi.ijmr.2012.3.1.61103
Singh, I. (2018). Plant Growth Promoting Rhizobacteria (PGPR) and their various mechanisms for plant growth enhancement in stressful conditions: a review. Eur. J. Biol. Res. 8 (4), 191–213. doi: 10.5281/zenodo.1455995
Sissons, M. (2008). Role of durum wheat composition on the quality of pasta and bread. Food 2, 75–90.
Solaiman, Z. M., Mickan, B. (2014). “Use of mycorrhiza in sustainable agriculture and land restoration,” in Mycorrhizal Fungi: Use in Sustainable Agriculture and Land Restoration. Eds. Solaiman, Z. M., Abbott, L. K., Varma, A. (Heidelberg, Germany: Springer), 1–16. doi: 10.1007/978-3-662-45370-4_1
Stępień, A., Wojtkowiak, K. (2013). Composition of gluten proteins in spring and winter wheat grain cultivated under conditions of varied fertilization. Acta Agric. Scand. 63 (7), 588–594. doi: 10.1080/09064710.2013.829866
Stamenković, S., Beškoski, V., Karabegović, I., Lazić, M., Nikolić, N. (2018). Microbial fertilizers: a comprehensive review of current findings and future perspectives. Span. J. Agric. Res. 16 (1), e09R01. doi: 10.5424/sjar/2018161-12117
Subhashini, D. V. (2014). Growth promotion and increased potassium uptake of tobacco by potassium-mobilizing bacterium Frateuria aurantia grown at different potassium levels in vertisols. Commun. Soil Sci. Plan. Anal. 46 (2), 210–220. doi: 10.1080/00103624.2014.967860
Turan, M., Gulluce, M., Von Wiren, N., Sahin, F. (2012). Yield promotion and phosphorus solubilisation by plant promoting rhizobacteria in extensive wheat production. J. Plant Nutr. Soil Sc. 175, 818–826. doi: 10.1002/jpln.201200054
USEPA. (1995). “EPA method 3052: Microwave assisted acid digestion of siliceous and organically based matrices,” in Test Methods for Evaluating Solid Waste, 3rd ed. (Washington, USA: USEPA).
Vamerali, T., Guarise, M., Ganis, A., Bona, S., Mosca, G. (2003). Analysis of root images from auger sampling with a fast procedure: A case of application to sugar beet. Plant Soil 255, 387–397. doi: 10.1023/A:1026147607879
Vejan, P., Abdullah, R., Khadiran, T., Ismail, S., Nasrulhaq Boyce, A. (2016). Role of plant growth promoting rhizobacteria in agricultural sustainability - A review. Molecules 21, 573. doi: 10.3390/molecules21050573
Velázquez, E., Silva, L. R., Ramírez-Bahena, M. H., Peix, A. (2016). “Diversity of Potassium-Solubilizing Microorganisms and Their Interactions with Plants,” in Potassium Solubilizing Microorganisms for Sustainable Agriculture. Eds. Meena, V., Maurya, B., Verma, J., Meena, R. (New Delhi, India: Springer). doi: 10.1007/978-81-322-2776-2_7
Videira e Castro, I., Fareleira, P., Ferreira, E. (2016). “Nitrogen fixing symbiosis in a sustainable agriculture,” in Plant, Soil and Microbes. Eds. Hakeem, K., Akhtar, M., Abdullah, S. (Cham: Springer). doi: 10.1007/978-3-319-27455-3_4
Visioli, G., Bonas, U., Dal Cortivo, C., Pasini, G., Marmiroli, N., Mosca, G., et al. (2018a). Variations in yield and gluten proteins in durum wheat varieties under late-season foliar versus soil application of nitrose fertilizer in a northern Mediterranean environment. J. Sci. Food Agric. 98 (6), 2360–2369. doi: 10.1002/jsfa.8727
Visioli, G., Sanangelantoni, A. M., Vamerali, T., Dal Cortivo, C., Blandino, M. (2018b). 16S rDNA profiling to reveal the influence of seed-applied biostimulants on the rhizosphere of young maize plants. Molecules 23, 1461. doi: 10.3390/molecules23061461
Wolińska, A., Kuźniar, A., Zielenkiewicz, U., Izak, D., Szafranek-Nakonieczna, A., Banach, A., et al. (2017). Bacteroidetes as a sensitive biological indicator of agricultural soil usage revealed by a culture-independent approach. Appl. Soil Ecol. 119, 128–137. doi: 10.1016/j.apsoil.2017.06.009
Zadoks, J. C., Chang, T. T., Konzak, C. F. (1974). A decimal code for growth stages of cereals. Weed Res. 14, 415–421. doi: 10.1111/j.1365-3180.1974.tb01084.x
Zang, X., Liu, M., Fan, Y., Xu, J., Xu, X., Li, H. (2018). The structural and functional contributions of β-glucosidase-producing microbial communities to cellulose degradation in composting. Biotechnol. Biofuels 11, 51. doi: 10.1186/s13068-018-1045-8
Keywords: plant growth-promoting rhizobacteria, vesicular arbuscular mycorrhizae, soil bacterial biodiversity, 16S rDNA sequencing, gluten composition, sustainable agriculture
Citation: Dal Cortivo C, Ferrari M, Visioli G, Lauro M, Fornasier F, Barion G, Panozzo A and Vamerali T (2020) Effects of Seed-Applied Biofertilizers on Rhizosphere Biodiversity and Growth of Common Wheat (Triticum aestivum L.) in the Field. Front. Plant Sci. 11:72. doi: 10.3389/fpls.2020.00072
Received: 20 November 2019; Accepted: 17 January 2020;
Published: 26 February 2020.
Edited by:
Carlos Alberto Silva, Universidade Federal de Lavras, BrazilReviewed by:
Orlando Carlos Huertas Tavares, Universidade Federal Rural do Rio de Janeiro, BrazilCopyright © 2020 Dal Cortivo, Ferrari, Visioli, Lauro, Fornasier, Barion, Panozzo and Vamerali. This is an open-access article distributed under the terms of the Creative Commons Attribution License (CC BY). The use, distribution or reproduction in other forums is permitted, provided the original author(s) and the copyright owner(s) are credited and that the original publication in this journal is cited, in accordance with accepted academic practice. No use, distribution or reproduction is permitted which does not comply with these terms.
*Correspondence: Cristian Dal Cortivo, Y3Jpc3RpYW4uZGFsY29ydGl2b0B1bmlwZC5pdA==
Disclaimer: All claims expressed in this article are solely those of the authors and do not necessarily represent those of their affiliated organizations, or those of the publisher, the editors and the reviewers. Any product that may be evaluated in this article or claim that may be made by its manufacturer is not guaranteed or endorsed by the publisher.
Research integrity at Frontiers
Learn more about the work of our research integrity team to safeguard the quality of each article we publish.