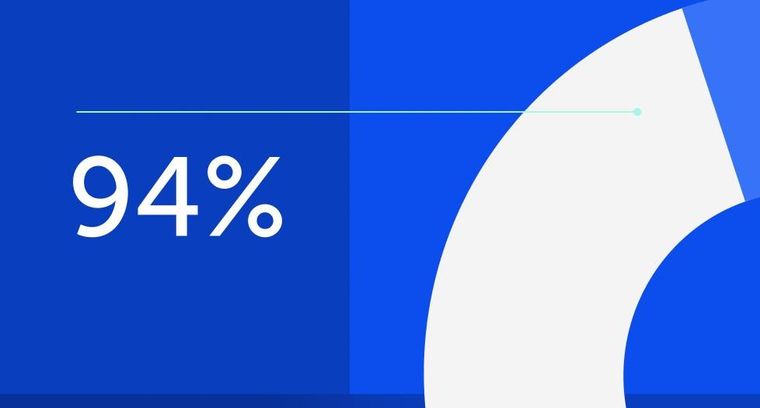
94% of researchers rate our articles as excellent or good
Learn more about the work of our research integrity team to safeguard the quality of each article we publish.
Find out more
ORIGINAL RESEARCH article
Front. Plant Sci., 04 February 2020
Sec. Plant Biotechnology
Volume 11 - 2020 | https://doi.org/10.3389/fpls.2020.00010
This article is part of the Research TopicCRISPR-Cas in Agriculture: Opportunities and ChallengesView all 12 articles
The CRISPR/Cas9 system is a powerful tool for targeted gene editing in many organisms including plants. However, most of the reported uses of CRISPR/Cas9 in plants have focused on modifying one or a few genes, and thus the overall specificity, types of mutations, and heritability of gene alterations remain unclear. Here, we describe the molecular characterization of 361 T0 transgenic tomato plants that were generated using CRISPR/Cas9 to induce mutations in 63 immunity-associated genes. Among the T0 transformed plants, 245 carried mutations (68%), with 20% of those plants being homozygous for the mutation, 30% being heterozygous, 32% having two different mutations (biallelic), and 18% having multiple mutations (chimeric). The mutations were predominantly short insertions or deletions, with 87% of the affected sequences being smaller than 10 bp. The majority of 1 bp insertions were A (50%) or T (29%). The mutations from the T0 generation were stably transmitted to later generations, although new mutations were detected in some T1 plants. No mutations were detected in 18 potential off-target sites among 144 plants. Our study provides a broad and detailed view into the effectiveness of CRISPR/Cas9 for genome editing in an economically important plant species.
Derived from a native adaptive immune system in eubacteria and archaea, the CRISPR/Cas system enables the alteration of DNA sequences in many organisms to achieve precise gene modifications (Jaganathan et al., 2018). The most widely used Streptococcus pyogenes Cas9 (SpCas9) requires the 20-bp spacer sequence of a guide RNA (gRNA) to recognize a complementary target DNA site upstream of a protospacer adjacent motif (PAM) and generates a double-stranded break (DSB) near the target region (Xie and Yang, 2013). DSBs are repaired through either non-homologous end joining (NHEJ) or homology-directed recombination (HDR) resulting in small insertions/deletions (indels) or substitutions at the target region, respectively (Jinek et al., 2012). Compared to other genome editing tools such as zinc finger nucleases (ZFNs; Kim et al., 1996) and transcription activator-like effector nucleases (TALENs; Bogdanove and Voytas, 2011), CRISPR/Cas is more robust in that the Cas protein can theoretically bind to any genomic region preceding a PAM site and, importantly, target multiple sites simultaneously. However, the possibility of off-target mutations caused by CRISPR/Cas is a potential concern in both basic and applied research in plants, although it has been reported that off-target effects of CRISPR/Cas occur at a much lower frequency in plants than in mammals (Fu et al., 2013; Kuscu et al., 2014). The most effective way to minimize off-target mutations is to select a gRNA target with little or no homology to other genomic regions (Baltes and Voytas, 2015). Other methods to reduce off-target mutations include using paired Cas9 nickases (Ran et al., 2013) or paired fusions of a catalytically dead Cas9 nuclease to the FokI cleavage domain (Guilinger et al., 2014; Tsai et al., 2014).
As one of the most important vegetable crops in the world (Kimura and Sinha, 2008), tomato (Solanum lycopersicum) is an important source of health-promoting nutrients including vitamin C and E, minerals, and carotenes such as ß-carotene and lycopene (Canene-Adams et al., 2005). However, tomato production is threatened by many infectious diseases, including bacterial speck disease caused by Pseudomonas syringae pv. tomato (Pst), which can result in severe economic losses due to reduced yield and quality (Xin and He, 2013). A large number of candidate immunity-associated genes have been identified in tomato, but validation of the functional importance of these genes had been technically challenging before the emergence of CRISPR/Cas technology (Pedley and Martin, 2003; Oh and Martin, 2011; Rosli et al., 2013; Pombo et al., 2014). Although CRISPR/Cas has been used to modify genes with key roles in growth, development, and biotic and abiotic stresses in plants (Ito et al., 2015; Jacobs et al., 2017; Nekrasov et al., 2017; Rodriguez-Leal et al., 2017; Shimatani et al., 2017; Yu et al., 2017; D’Ambrosio et al., 2018; Hashimoto et al., 2018; Li et al., 2018a; Li et al., 2018b; Tashkandi et al., 2018; Zsogon et al., 2018; Ortigosa et al., 2019; Wang et al., 2019; Xu et al., 2019), all of the reported studies have focused on one or a few genes and could not provide broad insights into the specificity, types of mutations and heritability of genome editing by CRISPR/Cas9 in tomato.
Recently, we developed the Plant Genome Editing Database (PGED; http://plantcrispr.org/cgi-bin/crispr/index.cgi) which provides information about a collection of tomato lines with CRISPR/Cas9-induced mutations in immunity-associated genes (Zheng et al., 2019). In the present study, we molecularly characterized 361 T0 transgenic tomato plants that were generated using CRISPR/Cas9 to induce mutations in 63 candidate immunity-associated genes. To enhance the mutation efficiency and reduce the number of transformations needed, we evaluated gRNA efficiency by transient expression in tomato leaves and conducted tomato transformation with Agrobacterium pools containing 2–4 Cas9/gRNA constructs. This initial evaluation of gRNAs allowed us to select the most efficient ones for tomato stable transformation while using “agrobacterium pools” with various Cas9/gRNAs constructs shortened the time for generating multiple tomato mutant lines. We established an efficient CRISPR/Cas9 system to generate a large number of primary transgenic lines and we report for the first time a systematic investigation of the specificity of targeting, the types of mutations generated and the heritability of the mutations through multiple generations of tomato. Our CRISPR/Cas9-induced tomato mutant plants provide a powerful resource for better understanding the molecular mechanisms of plant-microbe interactions in the future.
All 20-nt gRNAs specific for the target genes were designed using the software Geneious R11 as described previously (Jacobs et al., 2017). The tomato (Solanum lycopersicum) reference genome sequence (SL2.5 or SL3.0) was used as an off-target database to score each gRNA (GN19 or gN19; “g” represents a manually added “G” to accommodate the transcription initiation requirement of the U6 promoter if the first nucleotide is not a G at target sites) preceding a PAM (NGG) sequence. For each gene, 2–3 gRNA targets with minimum off-target scores were designed. Single or multiple gRNA cassettes were cloned into a binary vector p201N:Cas9 by Gibson assembly as described previously (Jacobs and Martin, 2016). Colonies containing correct gRNA sequences were confirmed by PCR and Sanger sequencing.
Each Cas9/gRNA vector was transformed into the Agrobacterium tumefaciens strain 1D1249 (Wroblewski et al., 2005) by electroporation. For agroinfiltration into tomato leaves, the bacterial cells containing different gRNA plasmids were grown in liquid YEP medium with 150 mg/L kanamycin overnight at 30°C. The bacterial pellet was collected and resuspended in an induction buffer containing 10 mM MgCl2, 10 mM MES (pH 5.7), and 200 μM acetosyringone (Sigma-Aldrich). Bacterial suspensions were adjusted to OD600 = 0.3 and incubated at room temperature for 2–5 h. The third and fourth leaves of 4-week-old tomato plants were infiltrated with needle-less syringes and the whole plant was then placed in a growth chamber with a temperature of 22°C–24°C, 16 h light/8 h dark photoperiod and 65% relative humidity. Three days later, a pool of six leaf discs were collected from three individual plants (two leaf discs from each of three plants) that had been infiltrated with the tested Cas9/gRNA vector, and used for genomic DNA extraction, PCR and sequencing. The web-based tool TIDE (https://tide.deskgen.com) was used to determine the mutation frequency induced by corresponding Cas9/gRNA vectors.
Tomato transformation was performed either at the plant transformation facility at the Boyce Thompson Institute (BTI) or North Carolina State University (NCSU) (Gupta and Van Eck, 2016; Van Eck et al., 2019). Modifications of the transformation methods were made for Rio Grande (RG), including using 100 mg/L kanamycin for selection, and adding 0.1 mg/L indole-3-acetic acid (IAA) to the plant regeneration media (2Z, 1Z) and 1 mg/L IAA to the rooting medium. Each Cas9/gRNA vector was first electrotransformed into Agrobacterium tumefaciens LBA4404 (BTI), AGL1 (BTI), or GV3101(pMP90) (NCSU). In most cases, 2–4 Agrobacterium culture preparations (of the same Agrobacterium strain), each carrying a different Cas9/gRNA construct, were pooled together and used for transformation to minimize the number of experiments. Tomato genotypes RG-PtoR or RG-prf3 were used for transformation if not specifically labeled (Table 1).
Table 1 Mutation rates and mutation types in T0 transgenic plants. See also Table S3.
Genomic DNA was extracted from tomato cotyledons or young leaves using a modified CTAB method (Murray and Thompson, 1980). The existence of T-DNA was confirmed by PCR using primers listed in Table S6. To determine the mutation specificity, genomic regions flanking the target site of each gene were amplified with specific primers (see PGED; http://plantcrispr.org/) and sequenced by Sanger sequencing. TIDE was used to rapidly evaluate the mutated allelic sequences using the sequencing files (.ab1 format), especially for PCR amplicons of biallelic, heterozygous, or chimeric mutants whose mutation length and frequency cannot be determined due to superimposed sequencing chromatograms.
To evaluate potential off-target mutations caused by gRNAs in CRISPR-induced mutant plants, twelve gRNAs were selected and used as queries to search for potential off-target sites across the tomato genome with up to 4-nt mismatches and 1-nt indel by the software Geneious R11 or with up to 3-nt mismatches by a web tool Cas-OFFinder. Each off-target site was given a score based on how similar it was to the spacer sequence of gRNAs. A higher score for an off-target site indicated a higher similarity to the original target site and a higher likelihood to cause off-target mutations. A shortlist of potential off-target sites of each gRNA queried was generated by selecting their relatively high-scoring off-target sites predicted by either Geneious R11 or Cas-OFFinder (Table 3). Similar to mutation genotyping described above, genomic regions flanking the putative off-target sites were amplified with specific primers (Table S7) and PCR amplicons were sequenced to detect if off-target mutations were induced in those regions.
To study the efficiency and specificity of genome editing in tomato by CRISPR/Cas9 and to better understand plant-pathogen interactions, we generated a collection of tomato lines with targeted CRISPR/Cas9-induced mutations in genes that have been implicated in the immune response. Candidate genes were selected based on previous studies involving RNA-Seq, biochemical approaches, virus-induced gene silencing (VIGS), or yeast two-hybrid (Y2H) screens (Zeng et al., 2012; Rosli et al., 2013; Pombo et al., 2014; Giska and Martin, 2019); orthologs of immunity-associated genes reported in other plant species such as Arabidopsis and rice were also included (Shimizu et al., 2010; Hutin et al., 2015; Xin et al., 2016; Yamada et al., 2016; Stegmann et al., 2017).
For each candidate gene, at least two gRNAs targeting different DNA sites were designed and separately cloned into a Cas9-expressing binary vector p201N:Cas9 (Jacobs and Martin, 2016). The gRNAs were designed to be highly specific at target sites and their predicted off-target sites contained at least one nucleotide mismatch in the seed sequence (the last 12 nucleotides preceding the PAM) or two nucleotide mismatches against the full 20-bp protospacer (although some gRNAs were designed to intentionally modify multiple homologs simultaneously). Most Cas9/gRNA constructs in this study had only one gRNA expression cassette per plasmid, except one construct that contained three gRNA cassettes targeting three Aquaporin transporter genes (Table 1).
To initially evaluate the effectiveness of gRNAs and subsequently enhance the mutation rate in stably transformed tomato plants, 195 gRNAs were tested for their ability to cause mutations using Agrobacterium infiltration (agroinfiltration) in tomato leaves (Figure 1A; Table S1). After agroinfiltration, DNA was isolated from the leaf tissue and the genomic region spanning each target site was PCR amplified, sequenced, and analyzed with a web-based tool called Tracking of Indels by Decomposition (TIDE; https://tide.deskgen.com; Brinkman et al., 2014) to calculate the mutation frequency. gRNAs with mutation frequency >0 (p < 0.0001) were considered to be effective in inducing mutations in this assay while those with mutation rate = 0 were considered ineffective. A total of 61.5% of the tested gRNAs were effective in inducing transient mutations in tomato leaves (Figure 1B). Among these, 96% had a mutation rate greater than 0% but less than 10% in this assay, while only five gRNAs (4%) had a mutation frequency over 10% (Figure 1C).
Figure 1 Evaluation of gRNA-mediated mutation efficiency by agroinfiltration in tomato leaves. See also Figure S1 and Table S1. (A) Schematic showing the workflow of guide RNA (gRNA) evaluation by agroinfiltration. (B) Summary of gRNA efficiency tested by agroinfiltration. (C) The distribution of mutation efficiencies of the 195 gRNAs. Inset on the top right shows the number of gRNAs in each mutation efficiency range. TIDE (https://tide.deskgen.com) was used to calculate mutation efficiency by identifying the predominant types of insertions and deletions (indels) in the DNA of a targeted cell pool.
Agroinfiltration of tomato leaves is not very efficient and to test whether this affected our estimate of gRNA mutation efficacy, we evaluated the mutation efficiency of two of the gRNAs (Bti9ab-1 and Drm3-1) which each have identical target sites in both tomato and Nicotiana benthamiana (Figure S1). The mutation frequency induced by these two gRNAs in tomato was much lower than in N. benthamiana (Figure S1A). In addition to these two gRNAs, we also tested another four gRNAs that each target two of the four homologs of the Mai1 gene in N. benthamiana (Roberts et al., 2019; Figure S1B). In N. benthamiana, the majority of the gRNA targets showed a mutation frequency of 10%–40%, while a small number had a mutation frequency less than 5% (Figure S1B, C). These observations suggest that inefficiency of agroinfiltration in tomato leaves probably leads to an underestimate of the true efficacy of gRNAs for generating mutations. This is supported by later observations in which some low-efficient gRNAs were very effective in inducing mutations in stably-transformed tomato plants (Table S2). Factors that contribute to this lower activity of gRNAs by agroinfiltration of tomato leaves are unknown but might include possible specific immune responses of tomato against Agrobacterium or less efficient infiltration due to leaf morphology differences with N. benthamiana.
In most cases, we selected the most efficient gRNAs for subsequent stable transformation in tomato, however, some low-efficiency gRNAs were also used if limited gRNAs could be designed for a particular target gene. Most gRNAs that were effective in inducing mutations in the agroinfiltration transient assay also induced mutations in stable transgenic seedlings, with one exception where a gRNA that had a 4.4% mutation frequency in the transient assay did not edit target genes in two stably-transformed plants (Table S2). It was not possible, however, to directly compare gRNA efficiency in the transient assay with that in stable transformation, as other factors such as the bias of gRNA transformation into plants using “Agrobacterium pools” and the total number of regenerated transgenic seedlings varied from gene to gene in stable transformation.
A total of 361 putative primary (T0) transgenic tomato plants were regenerated by Agrobacterium tumefaciens-mediated stable transformation. To confirm the mutated sequence(s) in each plant, genomic regions spanning the target sites were PCR amplified and sequenced. All five possible genotypes, that is, wild-type, homozygous for the mutation, biallelic (a different mutation in each allele), heterozygous for the mutation, or multiple mutations (chimeric), were detected in our stably transformed tomato plants (Table 1). Direct sequencing of PCR amplicons containing biallelic, heterozygous, or chimeric mutations resulted in superimposed sequencing chromatograms, which made it difficult to determine specific mutation types and mutation frequency in those plants. To resolve this problem, TIDE was used to rapidly determine the mutated allelic sequences using the sequencing file (.ab1 format) with superimposed chromatograms (Brinkman et al., 2014), thus avoiding tedious and expensive cloning and multi-clone sequencing for mutation analysis.
Of the 361 T0 plants, 245 were found to have modifications at the target site(s) within 63 genes (Table 1; Table S3). Most of the lines had only one CRISPR-induced mutation in one gene per plant, while a few had mutations in two or even three genes (the latter cases occurred when using Agrobacterium pooling – see below). We identified only one mutant event for some of the targeted genes while for others up to 20 independent mutant events were generated (Table 1). Overall, the average editing efficiency (the number of edited plants/the number of transgenic plants) by CRISPR/Cas9 in tomato in our experiments was 68% (Figure 2A), although the mutation rate varied over a wide range from 14% to 100% from target gene to target gene in different mutant lines (Table 1). All four mutation types (homozygous, biallelic, heterozygous, or chimeric) were observed in several mutant lines that had sufficient independent mutant events, while there was a bias of mutation types in some mutant lines, probably due to the limited number of transgenic events generated (Table 1).
Figure 2 CRISPR/Cas9-induced gene mutations in T0 transgenic plants. See also Table S3. (A) The average mutation rate induced by CRISPR/Cas9 in T0 plants. (B) Summary of CRISPR-induced mutation types and their frequency in T0 plants. Left: Number of genes modified with the corresponding mutation type; Right: Percentage of genes harboring the corresponding mutation type. a Some plants have multiple target genes in one plant. (C) Frequency of each insertion or deletion mutation. x-axis: number of base pairs (bp) deleted (−) or inserted (+) into target sites. Inset on the left top shows the percentage of mutations ≤10 bp or >10 bp. bAll “possible > ± 50 bp” in the figure are included in >10 bp. TIDE only calculates mutation length ≤50 bp. (D) Percentage of different bases in the 1-bp insertion mutations. Inset at the right top shows the number of mutations with each type of inserted base.
We analyzed the distribution of the four mutation types in all the 245 T0 plants at the 267 mutated target sites (some plants had more than one target site) and found that the percentage of homozygous, biallelic, heterozygous, or chimeric mutation was 20%, 32%, 30%, and 18%, respectively (Figure 2B). In particular, plants having homozygous or biallelic mutations accounted for 52% of the total. These mutants and their progenies can be used directly for phenotype screening because no wild-type alleles are present, thus speeding the research process by saving time for further genotyping in the next generation. The most common mutation alterations induced by CRISPR/Cas9 were deletions or insertions, with 87% of these modifications at the target sites being less than 10 bp (Figure 2C). The proportion of deletion mutations was 77%, and the deletion length spanned a wide range from 1 bp to over 400 bp. Of all the mutations, the most abundant modification was 1-bp deletion or insertion (Figure 2C). For these, A- and T-insertions accounted for 79.5%, while G-insertions accounted for only 4.5% (Figure 2D). Base substitutions in combination with indels were also detected, but at a much lower frequency. Only three independent mutant events (two were the same mutation type) harbored a nucleotide substitution in one copy of the target genes at the positions 5-bp preceding the PAM, along with a short insertion or deletion at the target site (Figure S2).
Multiplex editing of three Aquaporin transporter (AquaT) genes by using one Cas9/gRNA construct was also tested. Three individual promoter-gRNA expression cassettes (in the order Aqua1-Aqua2-Aqua3) were assembled into the p201N:Cas9 vector (Figure S3A) as previously reported (Jacobs et al., 2017). Ten of the 12 regenerated transgenic plants were edited, including three single mutants, four double mutants, and three triple mutants (having mutations in all three genes). Interestingly, all the three single mutants knocked out AquaT1, while three double mutants modified AquaT1 and AquaT2 and one edited AquaT1 and AquaT3 simultaneously. Three plants had mutations in all the three AquaT genes together (Figure S3B). These data suggested the position of the gRNA cassette in the vector may affect its mutation rate, considering that all gRNAs were efficient enough to induce mutations once transformed into plants.
To evaluate the heritability of mutations in T0 plants, a large number of T1 and some T2 plants were generated and examined for their genotypes at the target sites. Most of the same genotypes from T0 plants were transmitted to plants in later generations, although we did not record segregation ratios in the progenies. Of note, no new mutations or reversions to wild-type were found in the progeny of any homozygous T0 plants, indicating all the homozygous mutations occurred in the transformed embryogenic cells before the first division. However, we did observe novel genotypes in a small percentage of T1 or T2 plants whose progenitor (T0 plants) harbored biallelic, heterozygous or chimeric mutations (Table 2). In particular, a homozygous mutation (−265 bp) was detected in the progeny of a NRC2 primary transgenic plant with a “+1 bp/+2 bp” biallelic mutation. It is possible that the new −265 bp modification at the target site was created by further modification of the existing +1 bp or +2 bp mutations in the progenitor as has been reported in rice and carrot (Klimek-Chodacka et al., 2018; Tang et al., 2018). It is also possible that the biallelic T0 plant (+1 bp/+2 bp) was a chimera and the new mutation derives from chimeric tissue of the T0 plant (Zhang et al., 2014).
We also observed new genotypes in the progeny of some T0 plants harboring heterozygous or chimeric mutations (Table 2) even though most of the progeny still possessed the same genotypes as the progenitor line. In some of these cases, CRISPR/Cas9 continued to modify the wild-type allele of the target gene in the progeny if the parent plants still contained a wild-type allele and the Cas9/gRNA expression cassette (Table 2). In other cases, unexpected genotypes were detected in some mutant lines including Mai1-E10, CathepsinB2-E8, Min7-E6, and ADE-E2 (Table 2). For instance, although the ADE-E2 T0 plant was chimeric (−4 bp/−5 bp/−13 bp) without a wild-type allele, we identified one T1 plant that was azygous (two copies of wild-type allele) and another one with a novel biallelic mutation (−4bp/−9 bp). Another example is the CathepsinB2-E8 T0 plant which had a heterozygous (−4 bp/WT) mutation. However, −2 bp homozygous and −1 bp/WT heterozygous mutations were detected in later generations. The unexpected new genotypes discussed above revealed that the one leaf/cotyledon sample may not reveal all the genotypes in the whole plant if the mutant is chimeric. Therefore, for T0 edited plants without any wild-type allele, it will still be useful to perform genotyping in subsequent generations to obtain homozygous mutants without the presence of Cas9/gRNA.
Mutations in unintended sequences (off-target mutations) is a possible concern in both functional genomics studies and plant breeding. To evaluate potential off-target effects by CRISPR/Cas9 in our tomato lines, we first evaluated the specificity of 12 gRNAs of Cas9 by Geneious R11 (https://www.geneious.com; Kearse et al., 2012) and Cas-OFFinder (Bae et al., 2014). The putative off-target sites predicted by Cas-OFFinder were then manually checked using JBrowse (https://solgenomics.net/jbrowse_solgenomics/) to confirm their locations in the tomato genome. The presence of a PAM was required for the site to be considered a candidate site. These gRNAs were selected for off-target analysis because morphological defects were observed in one or more mutant lines induced by these gRNAs (Figure S4; Table S4). A total of 18 possible off-target sites of the 12 gRNAs were identified and off-target mutations were examined in 12 T0 plants, 68 T1 plants and 44 T2 plants by PCR and Sanger sequencing (Table 3). No off-target modifications were discovered in the tested plants with or without Cas9, indicating our gRNAs and CRISPR-mediated mutations are highly specific.
Table 3 Examination of possible off-target mutations caused by 12 selected gRNAs in multiple generations. See also Tables S4 and S7.
Another way to evaluate the specificity of CRISPR/Cas9 is to test the efficiency of gRNAs with a few mismatches against the target sequence in the protospacer. One of our gRNAs targeted two tomato homologs, Mai5 and PBL-T1 (bothMai5/PBL-T1; 5’-gTAGATCGTAATGGATTGCA-3’; the first nucleotide “C” was converted to “G” to accommodate the transcription initiation requirement of the U6 promoter). The designed 20-bp protospacer sequence exactly matched the target site in Mai5 but had one mismatch in PBL-T1 at the third nucleotide from the 5’ end (5’-gTCGATCGTAATGGATTGCA-3’). We generated five T0 plants that contained the bothMai5/PBL-T1 gRNA construct, all of which had edits in Mai5 but not in PBL-T1, indicating the one mismatch (along with the first nucleotide at the 5’ end) in PBL-T1 appeared to significantly affect Cas9 binding and cleavage activity at the target site. Another gRNA, targeting the tomato FLS2.2 gene, did not induce targeted modifications in any of the 10 transgenic plants, possibly due to a 1-bp mismatch in the seed region of the gRNA in the Heinz 1706 reference genome (GTCATCAACATCTCGCTTGT) as compared to Rio Grande-PtoR (GTCATCAACATTTCGCTTGT). The reference genome was used for gRNA design and RG-PtoR was used for tomato transformation as it contains the resistance gene Pto for investigating Pto-mediated immunity in our mutants. This further indicates that CRISPR/Cas9 is highly specific, with even one mismatch in the gRNA rendering the site uncleavable to the Cas9/gRNA complex.
Tomato transformation is a lengthy and labor-intensive process. In an approach to minimize the number of transformation experiments needed, three to four Agrobacterium culture preparations each carrying a different gRNA construct were pooled and used for a single transformation experiment (Figure 3A). T0 plants were genotyped for both the presence of T-DNA carrying the gRNAs and for possible mutations in all the targeted genes in the pool. Of the 79 T0 plants generated, 58 plants (73%) contained precise modifications in one or more of the target genes. In terms of the number of target sites edited by CRISPR/Cas9 with pooled gRNAs, 48 (82.8%) of the T0 plants had mutations in just one gene, while 9 plants (15.5%) had mutations in two and 1 (1.7%) had mutations in three genes (Figure 3A). Among these T0 plants, 83.5% contained one gRNA cassette and 15.2% contained two different gRNA cassettes, while no plants recovered contained more than two gRNA cassettes integrated into the genome (Figure 3B). Interestingly, one mutant plant did not show detectable integration of the T-DNA sequence (expressing Cas9 and gRNA) but had a mutated gene, suggesting that transient expression of the Cas9/gRNA occurred in this plant. Additionally, we found another type of transient mutation in 8 T0 plants at 10 different target sites (Table S5). In these plants, a Cas9/gRNA expression cassette was integrated into the plant genome, as confirmed by PCR and Sanger sequencing, but the gRNA detected was not the one that induced the mutation in the plant (Table S5), suggesting that the mutation was caused by another transiently expressed Cas9/gRNA.
Figure 3 Tomato transformation with “Agrobacterium pools.” See also Table S5. (A) An example of a tomato transformation experiment designed to target 2–4 genes by using 3–4 pooled Agrobacterium cultures with each culture carrying a different gRNA. a Number of plants with mutations in the target gene is shown in parentheses; some plants had mutations in multiple genes. b Number of genes modified in the plants. “na” not applicable, since less than 3 genes were targeted in the experiment. (B) Left: Number of gRNAs detected in a single mutant plant by PCR and Sanger sequencing. Right: Percentage of T0 plants harboring no, one or two gRNAs.
Our effort to generate a large number of CRISPR/Cas9-induced tomato mutants targeting immunity-associated genes demonstrates that this mutation approach is efficient and robust for gene editing in tomato. Importantly, gene modifications mostly occurred in germline cells and were stably inherited in subsequent generations, similar to those in rice (Zhang et al., 2014) but not as in Arabidopsis, in which most mutations in T0 plants were somatically modified if a strong constitutive promoter was used to regulate the Cas9 expression (Feng et al., 2014; Feng et al., 2018). However, we detected a greater range of deletion and insertion lengths than observed in rice (Zhang et al., 2014), in which only 1-bp insertions and fewer deletion lengths were found, possibly due to different intrinsic DNA repair mechanisms between these two species. These differences could also be due to other factors including different transformation methods or culture conditions, and different sets of target genes that tolerate different degrees of mutations.
Base substitutions induced by the CRISPR/Cas9 system in tomato were very rare in our study. We frequently observed single nucleotide polymorphisms (SNPs) between Rio Grande (used for transformation) and the tomato reference genome (Heinz 1706), but these SNPs were due to natural variation, not mutagenesis, as confirmed by sequencing of the gene regions from untransformed plants. Most of these SNPs were located outside of the protospacer sequence of the gRNA targets, and to date we have only found one gRNA (targeting Fls2.2) which had a mismatch in the seed region that inhibited the Cas9 binding and cleavage at the target site. Therefore, it is reasonable to use the tomato reference genome as the template for gRNA design and subsequent mutation genotyping in transgenic Rio Grande and likely other tomato cultivars.
Various morphological phenotypes were detected in some mutants compared to wild-type plants. Some of these abnormal phenotypes were associated with all the mutation events occurring in a specific gene, strongly supporting that the mutation itself is responsible for the altered plant growth or development. However, some mutant lines showed unusual morphology associated with certain mutation events but not all, possibly indicating that another off-target mutation occurred in these plants. We therefore investigated a large number of these plants but did not find any evidence of off-target mutations, suggesting other mutations, if they exist, were either caused by tissue culture or Agrobacterium transformation, or spontaneous mutations during seed propagation (Tang et al., 2018). Our observations are consistent with previous reports that CRISPR/Cas9 causes few off-target mutations in plants including Arabidopsis (Feng et al., 2014), rice (Zhang et al., 2014; Tang et al., 2018), tomato (Nekrasov et al., 2017), cotton (Li et al., 2019), and maize (Young et al., 2019). True off-targets reported previously in plants showed high sequence homology to the original spacer sequence of gRNAs (Tang et al., 2018), which can be easily avoided by designing highly specific gRNAs using tools such as Geneious and Cas-OFFinder. Based on our data we devised a rule to avoid off-target effects of CRISPR/Cas9 by designing gRNAs whose highest scored potential off-target sites have at least a 1-nt mismatch in the seed sequence or 2-nt mismatches in the full protospacer sequence.
Surprisingly, we found new mutations in the progeny of some T0 plants that did not contain any wild-type allele. These new mutations did not appear to be derived from existing mutations in the T0 plants, as the Cas9-induced modifications were located within the seed sequence of the gRNA protospacer and as little as 1-bp mismatch in the seed sequence can dramatically impair the Cas9 binding and cleavage activity (Jinek et al., 2012). Therefore, we believe the new mutations were derived from chimeric tissue from the T0 plant that was not detected with the one cotyledon/leaf sample we used for mutation genotyping. We are currently advancing lines that have biallelic or heterozygous mutations, or that were chimeric to develop homozygous plants without the presence of Cas9/gRNA sequence. These plants will be used to investigate whether the mutations affect the plant immune response, especially to P. syringae pv. tomato.
The datasets generated for this study can be found in the bioRxiv: https://www.biorxiv.org/content/10.1101/835108v1. Primers used to amplify targeted region, the intervening genomic sequence, guide-RNA used, and the mutations found can be found in PGED (http://plantcrispr.org) by searching the relevant gene accession number.
GM and NZ conceived and designed the experiments. NZ designed gRNAs, constructed vectors, performed genotyping in T0 plants and other generations, and analyzed the data. HR performed genotyping of mutants in some T1 and T2 plants. JV optimized the plant transformation protocol and guided the transformation experiments. NZ and GM interpreted the data and wrote the manuscript
Funding was provided by National Science Foundation grants IOS-1732253 (JV) and IOS-1546625 (GM).
The authors declare that the research was conducted in the absence of any commercial or financial relationships that could be construed as a potential conflict of interest.
We thank Brian Bell, Jay Miller, and Nick Vail for plant care; Patricia Keen, Michelle Tjahjadi, and Sergei Krasnyanski for tomato transformation; and Colleen Conger and Elizabeth Trost for assistance with mutation genotyping. The authors declare no conflicts of interest. This manuscript has been released as a pre-print at bioRxiv (Zhang et al., 2019).
The Supplementary Material for this article can be found online at: https://www.frontiersin.org/articles/10.3389/fpls.2020.00010/full#supplementary-material
Bae, S., Park, J., Kim, J. S. (2014). Cas-OFFinder: a fast and versatile algorithm that searches for potential off-target sites of Cas9 RNA-guided endonucleases. Bioinformatics 30, 1473–1475. doi: 10.1093/bioinformatics/btu048
Baltes, N. J., Voytas, D. F. (2015). Enabling plant synthetic biology through genome engineering. Trends Biotechnol. 33, 120–131. doi: 10.1016/j.tibtech.2014.11.008
Bogdanove, A. J., Voytas, D. F. (2011). TAL effectors: customizable proteins for DNA targeting. Science 333, 1843–1846. doi: 10.1126/science.1204094
Brinkman, E. K., Chen, T., Amendola, M., van Steensel, B. (2014). Easy quantitative assessment of genome editing by sequence trace decomposition. Nucleic Acids Res. 42, e168. doi: 10.1093/nar/gku936
Canene-Adams, K., Campbell, J. K., Zaripheh, S., Jeffery, E. H., Erdman, J. W., Jr. (2005). The tomato as a functional food. J. Nutr. 135, 1226–1230. doi: 10.1093/jn/135.5.1226
D’Ambrosio, C., Stigliani, A. L., Giorio, G. (2018). CRISPR/Cas9 editing of carotenoid genes in tomato. Transgenic Res. 27, 367–378. doi: 10.1007/s11248-018-0079-9
Feng, Z., Mao, Y., Xu, N., Zhang, B., Wei, P., Yang, D. L., et al. (2014). Multigeneration analysis reveals the inheritance, specificity, and patterns of CRISPR/Cas-induced gene modifications in Arabidopsis. Proc. Natl. Acad. Sci. U. S. A 111, 4632–4637. doi: 10.1073/pnas.1400822111
Feng, Z., Zhang, Z., Hua, K., Gao, X., Mao, Y., Botella, J. R., et al. (2018). A Highly Efficient Cell Division-Specific CRISPR/Cas9 System Generates Homozygous Mutants for Multiple Genes in Arabidopsis. Int. J. Mol. Sci. 19, 3925. doi: 10.3390/ijms19123925
Fu, Y., Foden, J. A., Khayter, C., Maeder, M. L., Reyon, D., Joung, J. K., et al. (2013). High-frequency off-target mutagenesis induced by CRISPR-Cas nucleases in human cells. Nat. Biotechnol. 31, 822–826. doi: 10.1038/nbt.2623
Giska, F., Martin, G. B. (2019). PP2C phosphatase Pic1 negatively regulates the phosphorylation status of Pti1b kinase, a regulator of flagellin-triggered immunity in tomato. Biochem. J. 476, 1621–1635. doi: 10.1042/BCJ20190299
Guilinger, J. P., Thompson, D. B., Liu, D. R. (2014). Fusion of catalytically inactive Cas9 to FokI nuclease improves the specificity of genome modification. Nat. Biotechnol. 32, 577–582. doi: 10.1038/nbt.2909
Gupta, S., Van Eck, J. (2016). Modification of plant regeneration medium decreases the time for recovery of Solanum lycopersicum cultivar M82 stable transgenic lines. Plant Cell Tissue Organ Cult. (PCTOC) 127, 417–423. doi: 10.1007/s11240-016-1063-9
Hashimoto, R., Ueta, R., Abe, C., Osakabe, Y., Osakabe, K. (2018). Efficient multiplex genome editing induces precise, and self-ligated type mutations in tomato plants. Front. Plant Sci. 9, 916. doi: 10.3389/fpls.2018.00916
Hutin, M., Sabot, F., Ghesquiere, A., Koebnik, R., Szurek, B. (2015). A knowledge-based molecular screen uncovers a broad-spectrum OsSWEET14 resistance allele to bacterial blight from wild rice. Plant J. 84, 694–703. doi: 10.1111/tpj.13042
Ito, Y., Nishizawa-Yokoi, A., Endo, M., Mikami, M., Toki, S. (2015). CRISPR/Cas9-mediated mutagenesis of the RIN locus that regulates tomato fruit ripening. Biochem. Biophys. Res. Commun. 467, 76–82. doi: 10.1016/j.bbrc.2015.09.117
Jacobs, T. B., Martin, G. B. (2016). High-throughput CRISPR vector construction and generation of tomato hairy roots for validation of DNA modifications and experimental use. J. Vis. Exp. 110, e53843. doi: 10.3791/53843
Jacobs, T. B., Zhang, N., Patel, D., Martin, G. B. (2017). Generation of a collection of mutant tomato lines using pooled CRISPR libraries. Plant Physiol. 174, 2023–2037. doi: 10.1104/pp.17.00489
Jaganathan, D., Ramasamy, K., Sellamuthu, G., Jayabalan, S., Venkataraman, G. (2018). CRISPR for Crop Improvement: An Update Review. Front. Plant Sci. 9, 985. doi: 10.3389/fpls.2018.00985
Jinek, M., Chylinski, K., Fonfara, I., Hauer, M., Doudna, J. A., Charpentier, E. (2012). A programmable dual-RNA-guided DNA endonuclease in adaptive bacterial immunity. Science 337, 816–821. doi: 10.1126/science.1225829
Kearse, M., Moir, R., Wilson, A., Stones-Havas, S., Cheung, M., Sturrock, S., et al. (2012). Geneious Basic: an integrated and extendable desktop software platform for the organization and analysis of sequence data. Bioinformatics 28, 1647–1649. doi: 10.1093/bioinformatics/bts199
Kim, Y. G., Cha, J., Chandrasegaran, S. (1996). Hybrid restriction enzymes: zinc finger fusions to Fok I cleavage domain. Proc. Natl. Acad. Sci. U. S. A 93, 1156–1160. doi: 10.1073/pnas.93.3.1156
Kimura, S., Sinha, N. (2008). Tomato (Solanum lycopersicum): A model fruit-bearing crop. CSH Protoc. 2008, emo105. doi: 10.1101/pdb.emo105
Klimek-Chodacka, M., Oleszkiewicz, T., Lowder, L. G., Qi, Y., Baranski, R. (2018). Efficient CRISPR/Cas9-based genome editing in carrot cells. Plant Cell Rep. 37, 575–586. doi: 10.1007/s00299-018-2252-2
Kuscu, C., Arslan, S., Singh, R., Thorpe, J., Adli, M. (2014). Genome-wide analysis reveals characteristics of off-target sites bound by the Cas9 endonuclease. Nat. Biotechnol. 32, 677–683. doi: 10.1038/nbt.2916
Li, R., Fu, D., Zhu, B., Luo, Y., Zhu, H.(2018a). CRISPR/Cas9-mediated mutagenesis of lncRNA1459 alters tomato fruit ripening. Plant J. 94,513–524. doi: 10.1111/tpj.13872
Li, T., Yang, X., Yu, Y., Si, X., Zhai, X., Zhang, H., et al. (2018b). Domestication of wild tomato is accelerated by genome editing. Nat. Biotechnol. 36, 1160–1163. doi: 10.1038/nbt.4273
Li, J., Manghwar, H., Sun, L., Wang, P., Wang, G., Sheng, H., et al. (2019). Whole genome sequencing reveals rare off-target mutations and considerable inherent genetic or/and somaclonal variations in CRISPR/Cas9-edited cotton plants. Plant Biotechnol. J. 17, 858–868. doi: 10.1111/pbi.13020
Murray, M. G., Thompson, W. F. (1980). Rapid isolation of high molecular weight plant DNA. Nucleic Acids Res. 8, 4321–4325. doi: 10.1093/nar/8.19.4321
Nekrasov, V., Wang, C., Win, J., Lanz, C., Weigel, D., Kamoun, S. (2017). Rapid generation of a transgene-free powdery mildew resistant tomato by genome deletion. Sci. Rep. 7, 482. doi: 10.1038/s41598-017-00578-x
Oh, C.-S., Martin, G. B. (2011). Effector-triggered immunity mediated by the Pto kinase. Trends In Plant Sci. 16, 132–140. doi: 10.1016/j.tplants.2010.11.001
Ortigosa, A., Gimenez-Ibanez, S., Leonhardt, N., Solano, R. (2019). Design of a bacterial speck resistant tomato by CRISPR/Cas9-mediated editing of SlJAZ2. Plant Biotechnol. J. 17, 665–673. doi: 10.1111/pbi.13006
Pedley, K. F., Martin, G. B. (2003). Molecular basis of Pto-mediated resistance to bacterial speck disease in tomato. Ann. Rev. Phytopathol. 41, 215–243. doi: 10.1146/annurev.phyto.41.121602.143032
Pombo, M. A., Zheng, Y., Fernandez-Pozo, N., Dunham, D. M., Fei, Z., Martin, G. B. (2014). Transcriptomic analysis reveals tomato genes whose expression is induced specifically during effector-triggered immunity and identifies the Epk1 protein kinase which is required for the host response to three bacterial effector proteins. Genome Biol. 15, 492. doi: 10.1186/s13059-014-0492-1
Ran, F. A., Hsu, P. D., Lin, C. Y., Gootenberg, J. S., Konermann, S., Trevino, A. E., et al. (2013). Double nicking by RNA-guided CRISPR Cas9 for enhanced genome editing specificity. Cell 154, 1380–1389. doi: 10.1016/j.cell.2013.08.021
Roberts, R., Hind, S. R., Pedley, K. F., Diner, B. A., Szarzanowicz, M. J., Luciano-Rosario, D., et al. (2019). Mai1 protein acts between Host recognition of pathogen effectors and mitogen-activated protein kinase signaling. Mol. Plant Microbe Interact. 32, 1496–1507. doi: 10.1094/MPMI-05-19-0121-R
Rodriguez-Leal, D., Lemmon, Z. H., Man, J., Bartlett, M. E., Lippman, Z. B. (2017). Engineering quantitative trait variation for crop improvement by genome editing. Cell 171, 470–480 e478. doi: 10.1016/j.cell.2017.08.030
Rosli, H. G., Zheng, Y., Pombo, M. A., Zhong, S., Bombarely, A., Fei, Z., et al. (2013). Transcriptomics-based screen for genes induced by flagellin and repressed by pathogen effectors identifies a cell wall-associated kinase involved in plant immunity. Genome Biol. 14, R139. doi: 10.1186/gb-2013-14-12-r139
Shimatani, Z., Kashojiya, S., Takayama, M., Terada, R., Arazoe, T., Ishii, H., et al. (2017). Targeted base editing in rice and tomato using a CRISPR-Cas9 cytidine deaminase fusion. Nat. Biotechnol. 35, 441–443. doi: 10.1038/nbt.3833
Shimizu, T., Nakano, T., Takamizawa, D., Desaki, Y., Ishii-Minami, N., Nishizawa, Y., et al. (2010). Two LysM receptor molecules, CEBiP and OsCERK1, cooperatively regulate chitin elicitor signaling in rice. Plant J. 64, 204–214. doi: 10.1111/j.1365-313X.2010.04324.x
Stegmann, M., Monaghan, J., Smakowska-Luzan, E., Rovenich, H., Lehner, A., Holton, N., et al. (2017). The receptor kinase FER is a RALF-regulated scaffold controlling plant immune signaling. Science 355, 287–289. doi: 10.1126/science.aal2541
Tang, X., Liu, G., Zhou, J., Ren, Q., You, Q., Tian, L., et al. (2018). A large-scale whole-genome sequencing analysis reveals highly specific genome editing by both Cas9 and Cpf1 (Cas12a) nucleases in rice. Genome Biol. 19, 84. doi: 10.1186/s13059-018-1458-5
Tashkandi, M., Ali, Z., Aljedaani, F., Shami, A., Mahfouz, M. M. (2018). Engineering resistance against Tomato yellow leaf curl virus via the CRISPR/Cas9 system in tomato. Plant Signal Behav. 13, e1525996. doi: 10.1080/15592324.2018.1525996
Tsai, S. Q., Wyvekens, N., Khayter, C., Foden, J. A., Thapar, V., Reyon, D., et al. (2014). Dimeric CRISPR RNA-guided FokI nucleases for highly specific genome editing. Nat. Biotechnol. 32, 569–576. doi: 10.1038/nbt.2908
Van Eck, J., Keen, P., Tjahjadi, M. (2019). “Agrobacterium tumefaciens mediated transformation of tomato,” in Transgenic Plants (Springer), (New York, NY: Humana Press) 225–234. doi: 10.1007/978-1-4939-8778-8_16
Wang, T., Zhang, H., Zhu, H. (2019). CRISPR technology is revolutionizing the improvement of tomato and other fruit crops. Hortic. Res. 6, 77. doi: 10.1038/s41438-019-0159-x
Wroblewski, T., Tomczak, A., Michelmore, R. (2005). Optimization of Agrobacterium-mediated transient assays of gene expression in lettuce, tomato and Arabidopsis. Plant Biotechnol. J. 3, 259–273. doi: 10.1111/j.1467-7652.2005.00123.x
Xie, K., Yang, Y. (2013). RNA-guided genome editing in plants using a CRISPR-Cas system. Mol. Plant 6, 1975–1983. doi: 10.1093/mp/sst119
Xin, X. F., He, S. Y. (2013). Pseudomonas syringae pv. tomato DC3000: a model pathogen for probing disease susceptibility and hormone signaling in plants. Annu. Rev. Phytopathol. 51, 473–498. doi: 10.1146/annurev-phyto-082712-102321
Xin, X. F., Nomura, K., Aung, K., Velasquez, A. C., Yao, J., Boutrot, F., et al. (2016). Bacteria establish an aqueous living space in plants crucial for virulence. Nature 539, 524–529. doi: 10.1038/nature20166
Xu, J., Hua, K., Lang, Z. (2019). Genome editing for horticultural crop improvement. Horticult. Res. 6, 113. doi: 10.1038/s41438-019-0196-5
Yamada, K., Saijo, Y., Nakagami, H., Takano, Y. (2016). Regulation of sugar transporter activity for antibacterial defense in Arabidopsis. Science 354, 1427–1430. doi: 10.1126/science.aah5692
Young, J., Zastrow-Hayes, G., Deschamps, S., Svitashev, S., Zaremba, M., Acharya, A., et al. (2019). CRISPR-Cas9 editing in maize: systematic evaluation of off-target activity and its relevance in crop improvement. Sci. Rep. 9, 6729. doi: 10.1038/s41598-019-43141-6
Yu, Q. H., Wang, B., Li, N., Tang, Y., Yang, S., Yang, T., et al. (2017). CRISPR/Cas9-induced targeted mutagenesis and gene replacement to generate long-shelf life tomato lines. Sci. Rep. 7, 11874. doi: 10.1038/s41598-017-12262-1
Zeng, L., Velasquez, A. C., Munkvold, K. R., Zhang, J., Martin, G. B. (2012). A tomato LysM receptor-like kinase promotes immunity and its kinase activity is inhibited by AvrPtoB. Plant J. 69, 92–103. doi: 10.1111/j.1365-313X.2011.04773.x
Zhang, H., Zhang, J., Wei, P., Zhang, B., Gou, F., Feng, Z., et al. (2014). The CRISPR/Cas9 system produces specific and homozygous targeted gene editing in rice in one generation. Plant Biotechnol. J. 12, 797–807. doi: 10.1111/pbi.12200
Zhang, N., Roberts, M. H., Van Eck, J., Martin, G. B. (2019). Generation and molecular characterization of CRISPR/Cas9-induced mutations in 63 immunity-associated genes in tomato reveals specificity and a range of gene modifications. bioRxiv. doi: 10.1101/835108
Zheng, Y., Zhang, N., Martin, G. B., Fei, Z. (2019). Plant Genome Editing Database (PGED): A call for submission of information about genome-edited plant mutants. Mol. Plant 12, 127–129. doi: 10.1016/j.molp.2019.01.001
Keywords: CRISPR/Cas9, genome editing, immunity-associated genes, tomato, Off-target mutation
Citation: Zhang N, Roberts HM, Van Eck J and Martin GB (2020) Generation and Molecular Characterization of CRISPR/Cas9-Induced Mutations in 63 Immunity-Associated Genes in Tomato Reveals Specificity and a Range of Gene Modifications. Front. Plant Sci. 11:10. doi: 10.3389/fpls.2020.00010
Received: 08 November 2019; Accepted: 07 January 2020;
Published: 04 February 2020.
Edited by:
Hiroshi Ezura, University of Tsukuba, JapanReviewed by:
Yiping Qi, University of Maryland, College Park, United StatesCopyright © 2020 Zhang, Roberts, Van Eck and Martin. This is an open-access article distributed under the terms of the Creative Commons Attribution License (CC BY). The use, distribution or reproduction in other forums is permitted, provided the original author(s) and the copyright owner(s) are credited and that the original publication in this journal is cited, in accordance with accepted academic practice. No use, distribution or reproduction is permitted which does not comply with these terms.
*Correspondence: Gregory B. Martin, Z2JtN0Bjb3JuZWxsLmVkdQ==
†ORCID: Ning Zhang, orcid.org/0000-0003-2775-1755
Holly M. Roberts, orcid.org/0000-0002-8640-9469
Joyce Van Eck, orcid.org/0000-0002-8005-365X
Gregory B. Martin, orcid.org/0000-0003-0044-6830
Disclaimer: All claims expressed in this article are solely those of the authors and do not necessarily represent those of their affiliated organizations, or those of the publisher, the editors and the reviewers. Any product that may be evaluated in this article or claim that may be made by its manufacturer is not guaranteed or endorsed by the publisher.
Research integrity at Frontiers
Learn more about the work of our research integrity team to safeguard the quality of each article we publish.