- DynaMo Center, Department of Plant and Environmental Sciences, University of Copenhagen, Frederiksberg, Denmark
Dynamically changing environmental conditions promote a complex regulation of plant metabolism and balanced resource investments to development and defense. Plants of the Brassicales order constitutively allocate carbon, nitrogen, and sulfur to synthesize glucosinolates as their primary defense metabolites. Previous findings support a model in which steady-state levels of glucosinolates in intact tissues are determined by biosynthesis and turnover through a yet uncharacterized turnover pathway. To investigate glucosinolate turnover in the absence of tissue damage, we quantified exogenously applied allyl glucosinolate and endogenous glucosinolates under different nutrient conditions. Our data shows that, in seedlings of Arabidopsis thaliana accession Columbia-0, glucosinolate biosynthesis and turnover are coordinated according to nutrient availability. Whereas exogenous carbon sources had general quantitative effects on glucosinolate accumulation, sulfur or nitrogen limitation resulted in distinct changes in glucosinolate profiles, indicating that these macronutrients provide different regulatory inputs. Raphanusamic acid, a breakdown product that can potentially be formed from all glucosinolate structures appears not to reflect in planta turnover rates, but instead correlates with increased accumulation of endogenous glucosinolates. Thus, raphanusamic acid could represent a metabolic checkpoint that allows glucosinolate-producing plants to measure the flux through the biosynthetic and/or turnover pathways and thereby to dynamically adjust glucosinolate accumulation in response to internal and external signals.
Introduction
Plants rely on a multitude of constitutive and inducible specialized metabolites that mediate interactions with the environment. These range from deterring pathogen and herbivores, over attracting beneficial organisms and enemies, to providing protection from, for example, UV radiation or drought. The chemodiversity of specialized metabolites and their regulation in terms of quantity and composition across tissues and developmental stages is beyond comparison (Moore et al., 2014). Arabidopsis thaliana, a small herbaceous plant of the Brassicaceae family, is a widely used and well-studied model organism for plant specialized metabolism, particularly the metabolism of glucosinolates (D’Auria and Gershenzon, 2005; Jensen et al., 2014; Burow and Halkier, 2017). Glucosinolates are chemically diverse amino acid-derived, sulfur- and nitrogen-containing thioglucosides almost solely found in plants of the order Brassicales with more than 130 identified structures to date (Blažević et al., 2019). The activation of glucosinolates has been intensively studied, both for their defensive roles against pathogens and herbivores and for their health beneficial roles for humans (Burow et al., 2010; Pastorczyk and Bednarek, 2016; Traka, 2016; Wittstock et al., 2016a). In recent years, more and more studies uncovering additional roles of glucosinolates and glucosinolate-derived metabolites in feedback regulation of plant metabolism, growth and defense emerged (Zhao et al., 2008; Kerwin et al., 2011; Burow et al., 2015; Jensen et al., 2015; Katz et al., 2015; Francisco et al., 2016a; Malinovsky et al., 2017; Urbancsok et al., 2017; Urbancsok et al., 2018). These studies have connected primary and specialized metabolism via glucosinolate-mediated signaling networks, and provided first insights into the regulatory interplay between glucosinolate metabolism and plant development.
As precursors of defensive metabolites, glucosinolates are constitutively biosynthesized in almost all tissues of A. thaliana throughout all developmental stages of the plant. Sufficient amounts of glucosinolates must be present in a given tissue for the glucosinolate-myrosinase system to function as defense. In the flower stalk, S-cells store glucosinolates accounting for 40% of the total sulfur in that tissue and these have also been identified in petioles (Koroleva et al., 2000; Koroleva et al., 2010). A considerable proportion of glucosinolates thus seems to be stored in these idioblasts. Nevertheless, glucosinolate quantity and composition vary dynamically depending on the age of the plant, the tissue studied and the environmental conditions (Petersen et al., 2002; Brown et al., 2003; Kliebenstein et al., 2005; Mewis et al., 2005; Gigolashvili et al., 2009; Textor and Gershenzon, 2009; Mewis et al., 2012; Züst et al., 2012; Huseby et al., 2013; Augustine and Bisht, 2016; Burow, 2016). Furthermore, a growing number of studies have identified additional functions of glucosinolates; often of distinct of glucosinolate structures, that are not directly linked to plant defense, but instead to hormone signaling, stomatal aperture, the circadian clock, root growth, biomass, and the onset of flowering (Zhao et al., 2008; Kerwin et al., 2011; Burow et al., 2015; Jensen et al., 2015; Katz et al., 2015; Francisco et al., 2016a; Francisco et al., 2016b; Malinovsky et al., 2017; Urbancsok et al., 2017; Urbancsok et al., 2018). These observations illustrate how intricately glucosinolate metabolism connects to a multitude of metabolic and developmental processes; and they may explain the dynamic nature of glucosinolate profiles, also in the absence of a biotic attacker.
Glucosinolate content reaches levels of up to 3% of the dry weight (Brown et al., 2003; Falk et al., 2007) making it a potentially costly investment for the plant (Bekaert et al., 2012; Züst and Agrawal, 2017). Because glucosinolates contain sulfur and nitrogen, their metabolism is tightly linked with sulfur and nitrogen assimilation and metabolism (Kopriva and Gigolashvili, 2016; Marino et al., 2016). Changes in abundance and composition depending on sulfur and nitrogen availability has been intensely studied in A. thaliana and glucosinolate-producing vegetables. Generally, sulfur availability and assimilation is positively correlated with aliphatic glucosinolate levels, while nitrogen availability is positively correlated with indolic glucosinolate levels [for example: (Booth et al., 1991; Withers and O’Donnell, 1994; Koprivova et al., 2000; Krumbein et al., 2001; Kopsell et al., 2007; Omirou et al., 2009; Frerigmann and Gigolashvili, 2014)]. However, increasing nitrogen concentrations have also been shown to have negative effects on total and aliphatic glucosinolate levels depending on the proportion of other macronutrients present such as sulfur, potassium and phosphorous (Li et al., 2007; Schonhof et al., 2007; Chun et al., 2017). Further, the type of nitrogen source (nitrate versus ammonium) can affect glucosinolate levels (Marino et al., 2016). Besides aforementioned inorganic macronutrients, different sugars have been shown to have a positive effect on glucosinolate levels in broccoli sprouts (Guo et al., 2011). Up to 30% of the total sulfur pool are bound in glucosinolates, which were therefore discussed as potential sulfur storage that can be remobilized under conditions of sulfur deficiency or starvation (Falk et al., 2007). The general ability of A. thaliana to metabolize glucosinolates has been directly demonstrated. When p-hydroxybenzyl glucosinolate (sinalbin) was provided as the only sulfur source, seedlings accumulated higher levels of sulfur-containing metabolites, including glucosinolates, than seedlings on glucosinolate-free, sulfur-deficient medium (Zhang et al., 2011). However, endogenous glucosinolates were shown not to be a sulfur source in Brassica seedlings when other sources of organic sulfur were present (Aghajanzadeh et al., 2014). This suggests that seedlings are capable of utilizing glucosinolates as a nutrient under conditions of deficiency, but glucosinolates are not a preferred source for sulfur remobilization. Unlike cyanogenic glycosides (Pičmanová et al., 2015), glucosinolates have not been investigated for a potential function as storage form of nitrogen.
Studies on glucosinolate breakdown have traditionally focused on the enzymatic activation of glucosinolates to bioactive compounds initiated by myrosinases upon tissue damage, i.e. caused by feeding insects (Matile, 1980; Kissen et al., 2009; Wittstock and Burow, 2010). Turnover in intact tissue (i.e. their in planta metabolization in absence of tissue-damage induced breakdown) is less well understood and the pathways and their regulation are yet to be uncovered (Figure 1). Earlier studies that investigated the dynamics of glucosinolates in A. thaliana during plant development or in adult rosettes at different times of day suggested turnover processes in concurrence with de novo biosynthesis (Petersen et al., 2002; Brown et al., 2003; Huseby et al., 2013). For example, relative and absolute amounts of glucosinolates change considerably during the transitions from seeds to seedlings and from seedlings to adult rosette (Brown et al., 2003). Furthermore, in A. thaliana Col-0, some methionine-derived glucosinolates (embryo-synthesized glucosinolates, Supplementary Figure 1) are only present in seeds and seedlings but not in vegetative rosette tissue of young plants indicative of in planta turnover of glucosinolates in seedlings (Petersen et al., 2002; Brown et al., 2003). Indeed, labeled [14C]p-hydroxybenzyl glucosinolate fed to rosette leaves of flowering plants accumulated in the seeds and subsequently showed a decline to 70% within the first weeks of plant development in the next generation (Petersen et al., 2002).
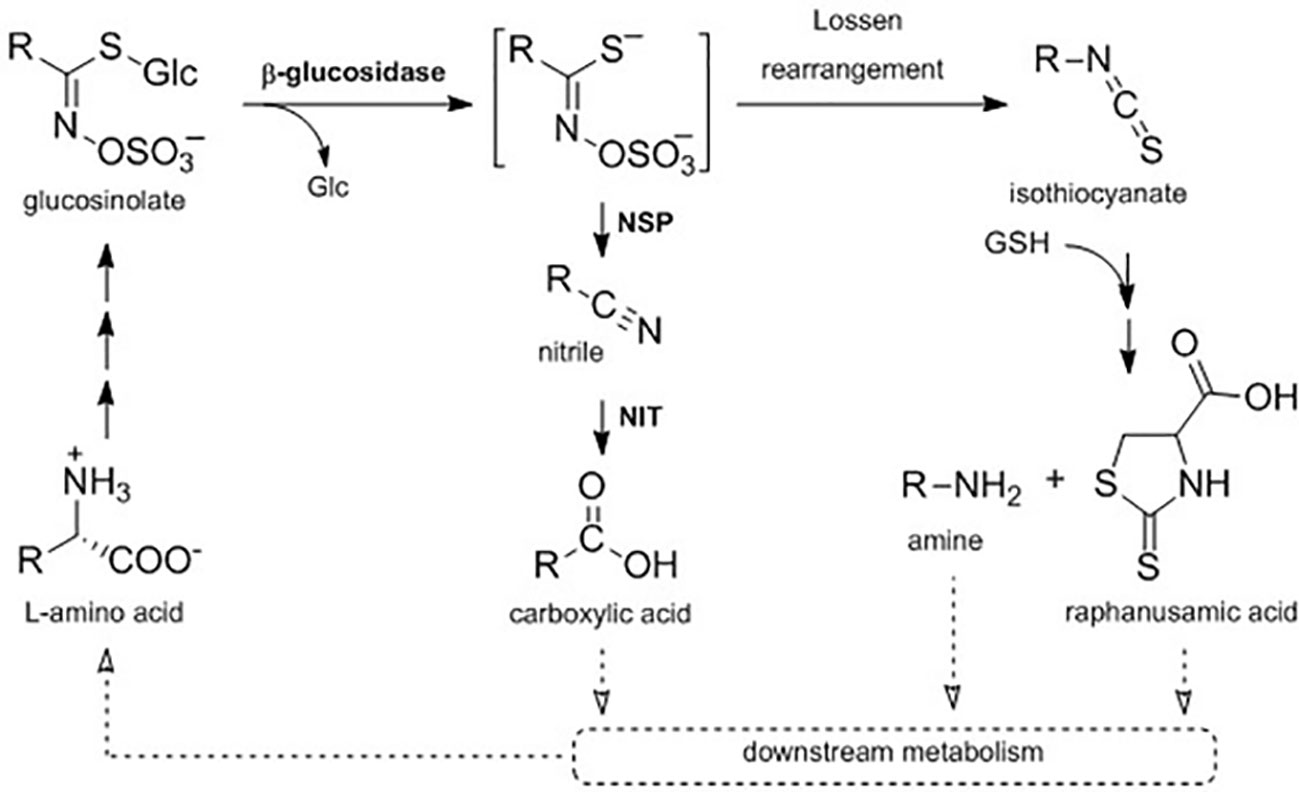
Figure 1 A proposed metabolic pathway connecting primary and specialized metabolism in intact A. thaliana Col-0 seedlings. In planta turnover of glucosinolates may proceed via hydrolytic cleavage of the glucose (Glc) moiety by a ß-glucosidase to form an aglucone, which is either metabolized to the corresponding nitrile in dependency of nitrile-specifier proteins (NSPs) or rearranged to the isothiocyanate. Nitriles can be further metabolized by nitrilases (NIT) to carboxylic acids, while isothiocyanates can be conjugated to glutathione (GSH) and metabolized to the corresponding amine and raphanusamic acid. Further downstream metabolization of those metabolites is not yet elucidated (dotted arrows).
It is currently unknown which enzymes and pathways are involved in in planta turnover of glucosinolates. Hydrolysis of the thioglucosidic bond by a β-glucosidase has been proposed as the first step (Figure 1). Experimental evidence suggests that the classical myrosinases TGG1 and TGG2 are not involved in glucosinolate turnover in the absence of tissue damage (Barth and Jander, 2006), but in total, 40 family 1 β-glucosidases are encoded in the A. thaliana Col-0 genome (Xu et al., 2004). For three of these enzymes besides classical myrosinases, it has been shown that they can hydrolyze glucosinolates under certain conditions and in certain tissues (Bednarek et al., 2009; Nakano et al., 2017; Nakazaki et al., 2019). In A. thaliana Col-0, damage-induced glucosinolate activation by myrosinases results in the formation of aglucones that can either arrange to isothiocyanates or be converted into simple nitriles (Figure 1) (Wittstock and Burow, 2007; Wittstock and Burow, 2010; Wittstock et al., 2016a). Nitrile-formation is dependent on action of nitrile-specifier proteins (NSPs), which are expressed in seeds, seedlings, leaves, and roots with an organ-specific regulation in A. thaliana Col-0 (Burow et al., 2009; Wittstock et al., 2016b). Glucosinolate-derived nitriles were suggested to be further converted to the corresponding carboxylic acids by nitrilases (NITs), yet these conversions were not considered to contribute to growth regulation via auxin signaling (Piotrowski, 2008; Janowitz et al., 2009). More recently, in silico modelling did, however, not hint to a major impact of nitrilase activity on auxin signaling (Vik et al., 2018). Spontaneous rearrangement of the aglucones yields isothiocyanates, which are highly reactive electrophiles (Brown and Hampton, 2011). Upon activation of 4-methoxyindol-3-ylmethyl glucosinolate (4MOI3M) by the atypical myrosinase PEN2, the resulting isothiocyanate is conjugated with glutathione and undergoes further metabolization to the corresponding amine bearing the indolic side-chain, a reaction that also yields the sulfur-containing raphanusamic acid (Figure 1) (Bednarek et al., 2009; Piślewska-Bednarek et al., 2018). Raphanusamic acid has been reported to have inhibitory properties on plant growth (Inamori et al., 1992) and to be involved in plant immunity (Bednarek, 2012), but the in planta mode of action remained elusive.
Here, to investigate the dynamics of glucosinolate biosynthesis and turnover in seedlings of A. thaliana Col-0 in the absence of tissue damage, we quantitatively analyzed the profiles of endogenous glucosinolates and the dynamics of the exogenous allyl glucosinolate under different nutrient conditions, specifically sulfur and nitrogen limitation as well as availability of exogenous sugars. Our data shows that glucosinolate biosynthesis and its downstream in planta turnover are coordinated according to nutrient availability. Under our experimental conditions, raphanusamic acid accumulation correlated with glucosinolate accumulation, suggesting that this turnover pathway intermediate can provide the plant with information on total glucosinolate levels.
Materials and Methods
Plant Treatments
A. thaliana seedling Col-0 (NASC, N1093) were sterilized with chlorine gas (generated by mixing 100 ml 14% sodium hypochlorite and 3 ml 37% HCL) for 3h. Growth medium was prepared as described in a published protocol (dx.doi.org/10.17504/protocols.io.5q6g5ze). Total nitrogen concentrations were 3 mM (+N) and 0.3 mM (−N) and total sulfur concentrations 1.68 mM (+S) and 0.015 mM (−S). ½ MS medium (Figure 3B) was purchased from Duchefa Biochemie (product no. M0222) and had a total sulfur concentration of 0.82 mM and total nitrogen concentration of 30 mM. Sugars were added to the growth medium solution prior to autoclaving. The concentrations of sugars used correspond to 1% (w/v, 29.2 mM) and 2% (w/v, 58.4 mM) for the monosaccharides glucose and fructose and the sugar alcohols mannitol and sorbitol, and 1.9% (w/v, 29.2 mM) and 3.8% (w/v, 58.4 mM) for the disaccharide sucrose. To study allyl glucosinolate accumulation and turnover, 100 mM allyl glucosinolate (Sinigrin, Sigma-Aldrich, No. S1647) stock solution in water was added to 50 ml of the autoclaved hand warm medium to the final concentrations indicated in the figures and carefully mixed by inversion. For all media treatments, 50 ml medium was poured into a square plate (120 ×120 × 16 mm, Frisenette, Denmark) and the medium was allowed to cool. After plating of the seeds, plates were placed for four days in the dark at 4°C for stratification and subsequently moved to a growth chamber (16 h light/8 h dark, 22/21°C, average light intensity 160 µE), in a vertical orientation. Germination was scored daily as the emergence of the radicle. Only seedlings that germinated one day after stratification were considered for further analysis. To investigate turnover of glucosinolates under different nutrient conditions, seedlings were carefully transferred after phase 1 (accumulation of exogenously applied allyl glucosinolate) using soft forceps onto plates containing different media compositions but no allyl glucosinolate (phase 2) (Supplementary Figure 3). Seedling weight was recorded for a single seedling using a fine-balance prior to metabolite sampling if indicated.
Metabolite Analyses
For glucosinolate and raphanusamic acid analysis, seedlings were harvested into 300 µl 85% (v/v) methanol (HPLC grade) containing 10 µM p-hydroxybenzyl glucosinolate (pOHb; PhytoLab, cat. No. 89793) as internal standard and subsequently homogenized in a bead mill (3 mm bearing balls, 2 × 30 s at 30 Hz). After centrifugation (5 min, 4,700×g, 4°C), 20 µl of the supernatant was diluted with 180 µL MilliQ-grade water (1:10) and filtered (Durapore® 0.22 µm PVDF filters, Merck Millipore, Tullagreen, Ireland) for the analysis of raphanusamic acid. For glucosinolate analysis, samples were prepared as desulfo-glucosinolates as previously described (alternate protocol 2, Crocoll et al., 2016) and analysed with LC-MS/MS. LC-MS/MS analysis was carried out on an Advance UHPLC system (Bruker, Bremen, Germany) equipped with a Kinetex® XB-C18 column (100 × 2.1 mm, 1.7 µm, 100 Å, Phenomenex, USA) coupled to an EVOQ Elite TripleQuad mass spectrometer (Bruker, Bremen, Germany) equipped with an electrospray ionization source (ESI). The injection volume was 1 µL. Separation was achieved with a gradient of water/0.05% (v/v) formic acid (solvent A)–acetonitrile (solvent B) at a flow rate of 0.4 ml/min at 40°C (formic acid, Sigma-Aldrich, cat. no. F0507, reagent grade; acetonitrile, HPLC grade). The elution profile was: 0–0.5 min 2% B; 0.5–1.2 min 2-30% B; 1.2–2.0 min 30–100% B; 2.0–2.5 min 100% B; 2.5–2.6 min 100–2% B; 2.6–4.0 min 2% B. For raphanusamic acid the instrument parameters were optimized by infusion with the pure standard (Sigma-Aldrich, No. 273449). The mass spectrometer parameters were as follows: ionspray voltage was maintained at 3,500 V, cone temperature was set to 300°C and heated probe temperature to 400°C, cone gas flow was set to 20 psi, probe gas flow to 40 psi, nebulizer gas 60 psi, and collision gas to 1.5 mTorr. Nitrogen was used as cone and nebulizer gas, and argon as collision gas. Multiple reaction monitoring (MRM) was used to monitor analyte parent ion > product ion transitions [collision energy]: (pos) 164 > 118 [15 V] (quantifier) and 164 > 59 [15 V] (qualifier). Both Q1 and Q3 quadrupoles were maintained at unit resolution. Glucosinolates were quantified relative pOHb using experimentally determined response factors with commercially available standards in a representative plant matrix. Raphanusamic acid was quantified based on external calibration using a dilution series of an authentic standard.
Statistical Analysis
Statistical analyses (parametric and non-parametric standard tests) were performed using the software R (version 3.3.2, R Core Team, 2017) after checking the suitability of the model for scedasticity and distribution of the data. Models used for analysis are indicated in the figure and supplementary table legends. Figures were generated using the package "ggplot2." MFA (multiple factor analysis) was performed and visualized using the packages "FactoMineR" and "factoextra."
Results
Exogenous Carbon Sources Affect Total Amounts of Glucosinolates During Seedling Development
Glucosinolate profiles differ markedly between seeds and seedlings of A. thaliana (Haughn et al., 1991; Brown et al., 2003). In the accession Col-0, glucosinolates with hydroxyalkyl and benzoyloxyalkyl side chains are synthesized in the developing seeds and still present in seedlings but not at later developmental stages (Supplementary Figure 2) (Kliebenstein et al., 2001; Brown et al., 2003; Kliebenstein et al., 2007). We refer here to these structures as embryo-synthesized glucosinolates. During the transition from seed to seedling, the total glucosinolate content transiently increases, but then drops again to seed level within two to six days after planting. Interestingly, the content of embryo-synthesized glucosinolates is stable during germination (Brown et al., 2003), suggesting that they are not yet catabolized at this early stage of seed-to-seedling transition. To study glucosinolate turnover kinetics, we therefore focused on seedling development after germination.
We analyzed glucosinolates in individual seedlings, starting three days after germination. Overall glucosinolate content continuously increased by 4-fold within six days (P < 0.001, Figure 2A) and more than 30-fold within 20 days after germination (P < 0.001, Figure 3A). This increase affects all three main glucosinolate classes of short- and long-chain aliphatic and indolic glucosinolates but is initially mainly driven by indolic glucosinolates (most dominantly NMOI3M; Supplementary Tables 1 and 2) and then later also by long-chain aliphatic glucosinolates (Supplementary Table 3). The group of embryo-synthesized glucosinolates (Supplementary Figure 1), herein 3bzo and 4bzo glucosinolates, slightly decrease with seedling development (P = 0.062) until they are not detected at nine days after germination (Figure 2B). Interestingly, we detected relatively high levels of embryo-synthesized glucosinolates decreasing at later developmental stages in a different developmental setup (see below).
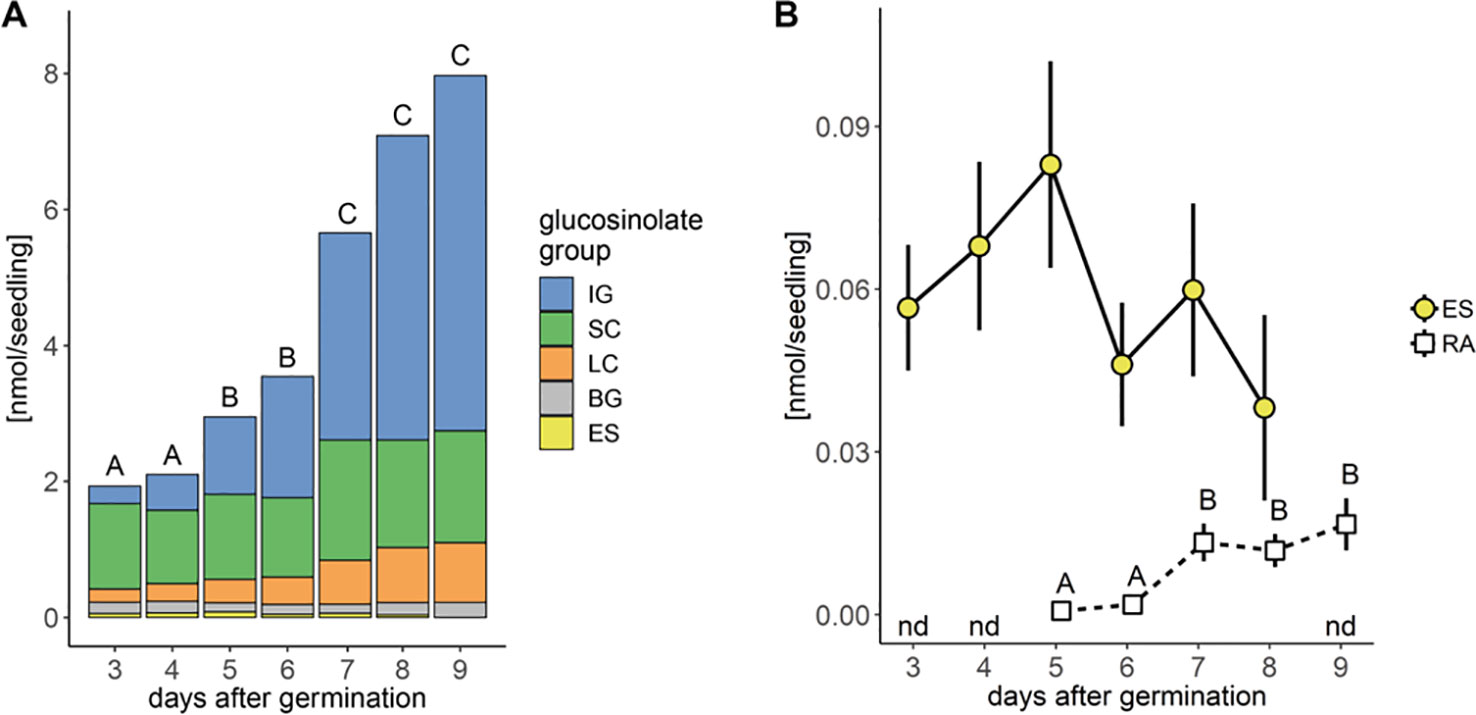
Figure 2 Glucosinolate accumulation during A. thaliana Col-0 seedling development. (A) Plotted are means (N = 6–18, one experimental round) of glucosinolate classes: IG, indolic glucosinolates; SC, short-chain aliphatic glucosinolates; LC, long-chain aliphatic glucosinolates; BG, benzenic glucosinolates; ES, embryo-synthesized glucosinolates. Letters denote significant differences at the level on total glucosinolate levels (P < 0.05). (B) Levels of embryo-synthesized glucosinolates (ES; P = 0.62) and raphanusamic acid (RA; P < 0.001). Embryo-synthesized glucosinolates were not detected (nd) in nine-day-old seedlings and raphanusamic acid was not detected in three- and four-day-old seedlings. Depicted are means ± SE, N = 6–18. Statistical testing was performed with Kruskal–Wallis rank sum test followed by pairwise comparisons using Wilcoxon rank sum test (P-value adjustment method: Benjamini & Hochberg). Details on the statistical analysis including means and standard deviations are provided in Supplementary Table 1 for glucosinolate groups and Supplementary Table 2 for individual glucosinolates.
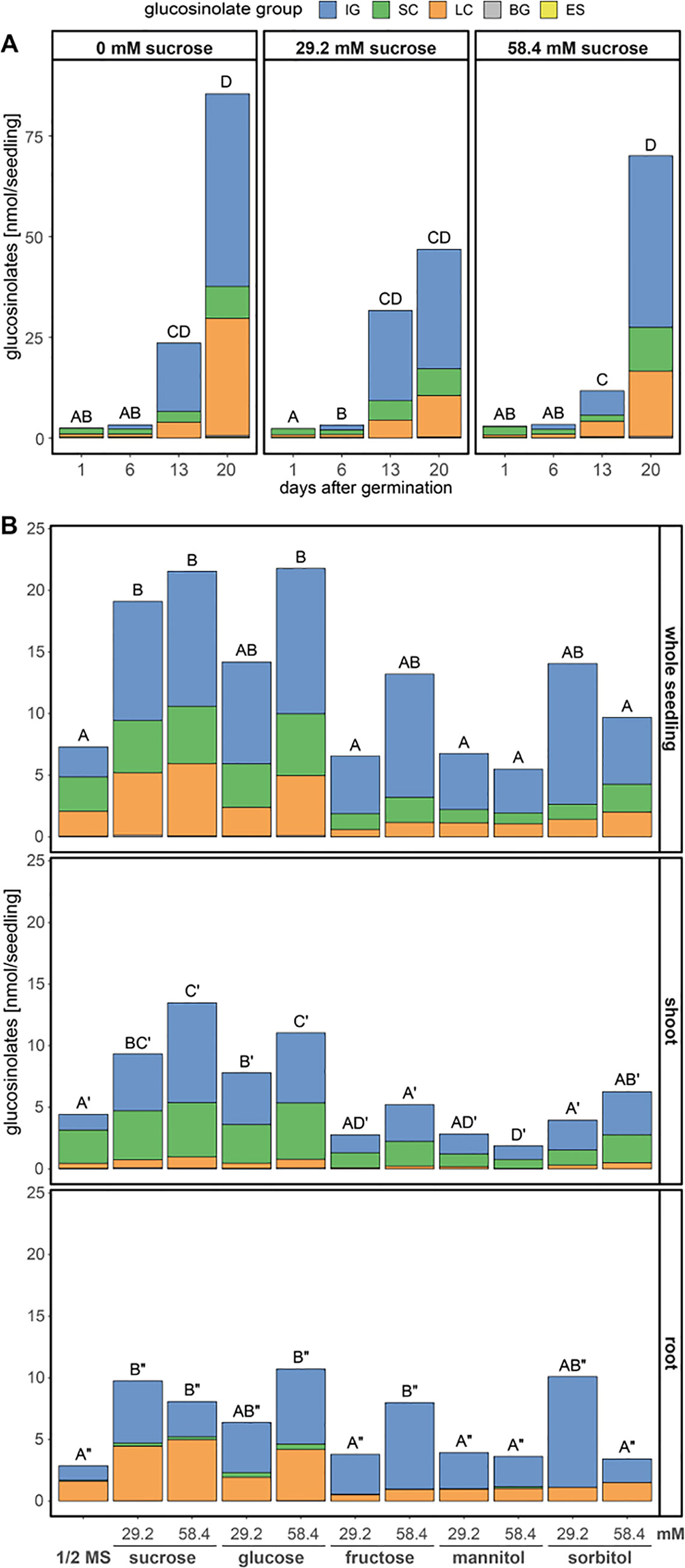
Figure 3 Glucosinolate accumulation in A. thaliana Col-0 seedlings depending on the presence and concentration of different sugars in the growth media. Plotted are the means of glucosinolate classes: IG, indolic glucosinolates; SC, short-chain aliphatic glucosinolates; LC, long-chain aliphatic glucosinolates; BG, benzenic glucosinolates; ES, embryo-synthesized glucosinolates. Letters denote significant differences at the 0.05 level on total glucosinolate levels. (A) Seedlings were grown on medium (1.68 mM S, 3 mM N) with three different sucrose concentrations and harvested 1–20 days after germination, (N = 6–8, one experimental round). Different letters indicate statistical differences across all treatments and days. Statistical details including means and standard deviations are in Supplementary Table 3 for glucosinolate groups and Supplementary Table 4 for individual glucosinolates. (B) Seedlings grew for twelve days after germination on ½ MS medium (0.82 mM S, 30 mM N) supplemented with different sugars, before shoot and root tissue were separately harvested per individual seedling. Letters denote significant differences of total glucosinolates levels within a tissue determined by pairwise comparison using Wilcoxon rank sum test (P-value adjustment method: Benjamini & Hochberg) (N = 12, one experimental round). Details on the statistical analysis including means and standard deviations are provided in Supplementary Table 5 for glucosinolate groups and Supplementary Table 6 for individual glucosinolates. Growth phenotypes are depicted in Supplementary Figure 4. ½ MS, half strength Murashige & Skoog medium.
Noticeable increases of glucosinolate content between five and seven days after germination correlated with the development of the first lateral roots and the expansion of the first true leaves, respectively. Concurrent with these physiological changes in seedling development and the pattern of glucosinolate accumulation, levels of raphanusamic acid show increases at five and seven days after germination (P < 0.001, Figure 2B).
The addition of exogenous carbon sources to the growth medium, as frequently done in studies on A. thaliana seedlings, had several effects on the dynamics of glucosinolate accumulation (Figure 3) and seedling development (Supplementary Figure 4). Exogenously supplied sucrose affected the rate and timing of the accumulation of all individual glucosinolates in whole seedlings (Figures 3A, B, top panel, Supplementary Table 3). Most dominantly affected by 29.2 mM sucrose treatment were the dynamics of long-chain aliphatic and indolic glucosinolate accumulation while the changes in short-chain aliphatic glucosinolates over time seemed unaffected (Supplementary Table 3). Compared to sucrose treatment, the effects of glucose treatment on glucosinolate levels was similar, while that of fructose was weaker (Figure 3B). Twelve-day-old seedlings showed a clear distribution of short-chain aliphatic glucosinolates predominantly in shoots and long-chain aliphatic glucosinolates mostly in roots. This tissue-specific distribution remained unaffected by all sugar treatments, albeit the absolute levels and ratios between all glucosinolate groups changed depending on the sugar applied (Figure 3B and Supplementary Table 5). The tested sugars additionally had considerable growth and developmental effects (Supplementary Figure 4). While seedlings grown in the presence of sucrose or glucose show an increase in lateral root formation and shoot size, fructose and mannitol caused an overall stunted growth phenotype. This suggests that the increase in glucosinolates in sucrose and glucose treated seedlings relates to the increased seedling size, while fructose treated seedlings accumulate considerably lower glucosinolate contents, possibly due to their smaller size (Figure 3B and Supplementary Figure 4).
Sulfur and Nitrogen Limitation Differentially Affects Total and Relative Glucosinolate Levels
Next, we tested the effect of sulfur and nitrogen limitation, alone and in combination, on glucosinolate accumulation and growth of A. thaliana Col-0 seedlings for several days. Before the seedlings were exposed to different nutrient regimes, seeds were germinated and grown for six days on control medium containing sufficient amounts of sulfur and nitrogen. Seedlings were then transferred to different media limited in sulfur and/or nitrogen (Supplementary Figure 3) and their development and glucosinolate profile was followed for several days. Nitrogen limitation led to reduced seedling weight (Supplementary Figure 5) and significantly affected accumulation of all de novo synthesized glucosinolate classes (indolic, short- and long-chain aliphatic glucosinolates) within ten to 13 days after transfer (16 to 19 day-old seedlings) and caused stagnation in glucosinolate accumulation (Figure 4A and Supplementary Table 7). After 13 days, nitrogen limitation alone (+S/−N medium) reduces short-chain aliphatic glucosinolate levels to 8%, long-chain aliphatic glucosinolate levels to 20% and indolic glucosinolate levels to 15% compared to the nutrient-sufficient control group (+S/+N medium). Within the long-chain aliphatic glucosinolates, this reduction was more pronounced for methylthioalkyl glucosinolates (7MTH, 8MTO) than for methylsulfinylalkyl glucosinolates (7MSH, 8MSO) (Supplementary Table 7).
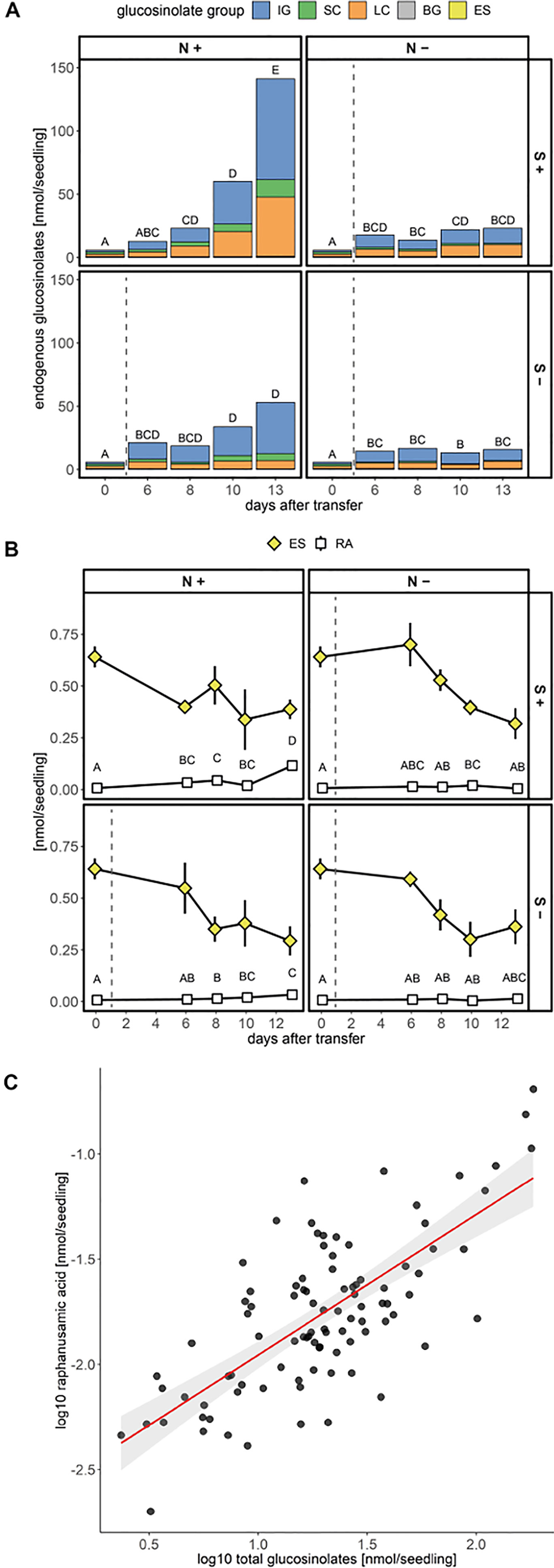
Figure 4 Accumulation of glucosinolates and raphanusamic acid in A. thaliana Col-0 seedlings under different sulfur (S) and nitrogen (N) regimes. Seeds were germinated on control medium containing sufficient amounts of sulfur (+S) and nitrogen (+N) and seedlings were grown for six days (phase 1) before they were transferred to media with varying sulfur and nitrogen nutrition (phase 2, Supplementary Figure 3). Day 0 describes the day of transfer and reflects the metabolite levels of six-day-old seedlings grown on +S/+N medium. The dashed grey line indicates that seedlings had a change to a limiting medium condition. (A) Total glucosinolate levels and (B) levels of embryo-synthesized glucosinolates (ES) and accumulation of raphanusamic acid (RA) under sufficient and limiting sulfur and nitrogen nutrition. Plotted are (A) means of the glucosinolate classes: IG, indolic glucosinolates; SC, short-chain aliphatic glucosinolates; LC, long-chain aliphatic glucosinolates; BG, benzenic glucosinolates; ES, embryo-synthesized glucosinolates, and (B) means ± SE (N = 5–16, two independent experiments pooled). Letters denote significant difference at the 0.05 level determined by pairwise comparison using Wilcoxon rank sum test (P-value adjustment method: Benjamini & Hochberg). Details on the statistical analysis including means and standard deviations are provided in Supplementary Table 7 for glucosinolate groups and Supplementary Table 8 for individual glucosinolates. Seedling fresh weight in nitrogen and/or sulfur sufficient and limiting conditions is plotted in Supplementary Figure 5. (C) Correlation between total levels of glucosinolates and raphanusamic acid for seedlings grown on sulfur and nitrogen sufficient medium harvested daily three to nine days after germination. Slope = 0.667 ± 0.066, P < 0.001 (F = 103.30), adj. R2 = 0.521 of the linear model lm(logRA∼logGLS). Correlations of raphanusamic acid to levels of indolic, short-chain aliphatic and long-chain aliphatic glucosinolates for seedlings grown on glucosinolate-free medium are depicted in Supplementary Figures 5A–C, correlations of raphanusamic acid to total glucosinolate levels and accumulated allyl glucosinolate for seedlings grown on medium supplemented with 50 μM allyl glucosinolate are depicted in Supplementary Figures 5D–E.
Sulfur limitation resulted in an overall reduced accumulation of glucosinolates, but when looking at the three main classes, only the reduced levels of aliphatic glucosinolates were statistically significant. Long-chain aliphatic glucosinolate levels were reduced to 14% and short-chain aliphatic glucosinolate levels to 40% of the nutrient-sufficient control group with similar effects on the individual structures within these classes. Although the levels of total indolic glucosinolates and the levels of NMOI3M were not affected by sulfur limitation, the levels of I3M and 4MOI3M were significantly reduced (Supplementary Tables 7 and 8). Almost exclusively for long-chain aliphatic glucosinolates, we observed synergistic effects of sulfur and nitrogen limitation (Supplementary Table 8).
Levels of embryo-synthesized glucosinolates decreased over time after transfer, but their turnover rate was not affected by nitrogen or sulfur limitation or the combination thereof (Figure 4B and Supplementary Table 7). Accumulation of the general turnover metabolite raphanusamic acid was highest under nitrogen sufficient conditions and thus did not follow the turnover of embryo-synthesized glucosinolates (Figure 4B). Instead, we found the levels of raphanusamic acid to be positively correlated with total glucosinolate accumulation (Figure 4C and Supplementary Figure 5). Availability of nitrogen and sulfur significantly affected seedling growth (Supplementary Figure 6). Nitrogen limitation showed the strongest negative effect on seedling growth (P < 0.001), resulting in stagnation of growth after eight days. The seedling weight was reduced to 13% compared to the nutrient-sufficient group after 13 days under limiting conditions irrespective of the availability of sulfur, which had a smaller negative effect on seedling weight (P = 0.047; Supplementary Table 9). Thus, total endogenous glucosinolate accumulation followed the pattern of seedling growth under nutrient limiting conditions.
Allyl Glucosinolate Accumulation Is Time- and Dose-Dependent and Promotes the Accumulation of Raphanusamic Acid
A. thaliana Col-0 does not biosynthesize allyl glucosinolate at any developmental stage (Kliebenstein et al., 2001; Li and Quiros, 2003; Wentzell et al., 2007; Burow et al., 2015). Accordingly, we did not detect allyl glucosinolate in this study unless it had been applied exogenously. Feeding this glucosinolate to Col-0 seedlings therefore makes it possible to experimentally uncouple biosynthesis and turnover (Francisco et al., 2016a). Seedlings grown on medium containing 50 µM allyl glucosinolate steadily accumulated increasing amounts of allyl glucosinolate (Figure 5A) and reached levels corresponding to approx. 5% of the total endogenous glucosinolates (Figure 5B and Supplementary Tables 10 and 11). Notably, raphanusamic acid levels were considerably higher in allyl glucosinolate-treated seedlings. Nine days after germination, seedlings that had grown on allyl glucosinolate-containing medium accumulated 1.3 nmol raphanusamic acid, whereas the control seedlings only accumulated less than 0.02 nmol (Figures 2B and 5C). Feeding of allyl glucosinolate moreover coincided with accumulation of 0.56 nmol allyl glucosinolate per seedling and increased levels of total endogenous glucosinolates (11 nmol/seedling compared to 8 nmol/seedling grown on control medium) nine days after germination, indicating that the turnover of endogenous glucosinolates might contribute to the accumulation of raphanusamic acid at this developmental stage.
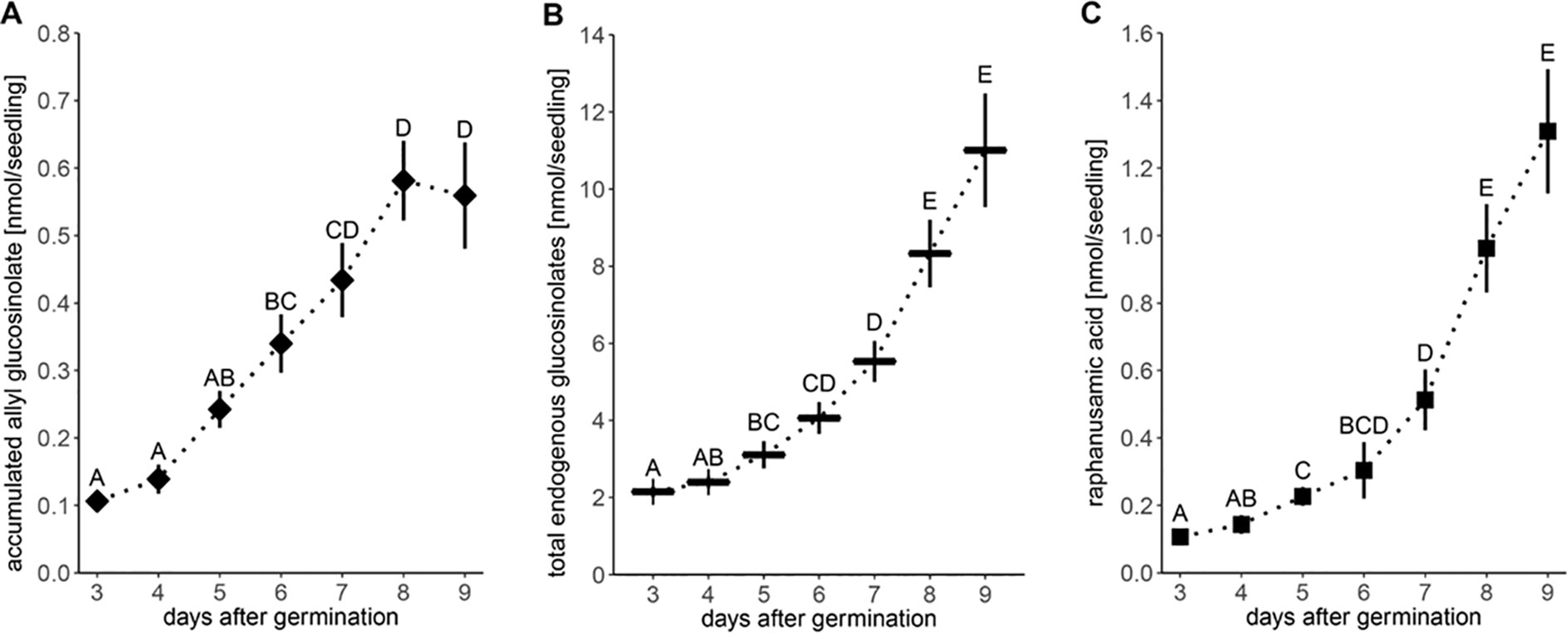
Figure 5 Accumulation of allyl glucosinolate (A), total endogenous glucosinolates (B) and raphanusamic acid, (C) in A. thaliana Col-0 seedlings grown continuously on media containing 50 µM allyl glucosinolate. Depicted are means ± SE (N = 17–19, one experimental round) and letters denote significant difference at the 0.05 level. Details on the statistical analysis including means and standard deviation are provided in Supplementary Table 10 for allyl glucosinolate, glucosinolate groups and raphanusamic acid.
Furthermore, allyl glucosinolate accumulated to higher levels with increasing concentrations of allyl glucosinolate in sucrose-free growth medium (Figure 6). The presence of 29.2 mM sucrose in the growth medium decreased the accumulation in seedlings grown on high relative to low allyl glucosinolate concentrations six days after germination (white diamonds, Figure 6) and allyl glucosinolate accumulation remained sucrose-dependent over time (Supplementary Figure 7 and Supplementary Table 12). To investigate the turnover of allyl glucosinolate, allyl glucosinolate-containing seedlings were transferred to glucosinolate-free medium with the same sucrose concentration and grown for another seven days (Figure 6). Allyl glucosinolate levels were reduced by 54%, 58% and 72% for seedlings treated with 10 µM, 50 µM and 200 µM allyl glucosinolate, respectively, after growing on 0 mM sucrose compared to the levels on the day of transfer (black diamonds, Figure 6). In comparison, allyl glucosinolate levels were reduced only by up to 20% for seedlings grown on 29.2 mM sucrose (Figure 6) suggesting a reduced rate of allyl glucosinolate turnover. We further investigated the effect of nitrogen and sulfur limitation on allyl glucosinolate turnover, which had only a minor effect within our time frame of investigation depending on the initial allyl glucosinolate concentration applied in the medium (Supplementary Figure 8 and Supplementary Table 12). Seedling weight, however, was significantly affected by both, the concentration of allyl glucosinolate supplied in the accumulation phase (Phase 1, P < 0.001) and nitrogen limitation in the turnover phase (Phase 2, P < 0.001) for 0 mM sucrose treatment (Supplementary Figure 9 and Supplementary Table 13).
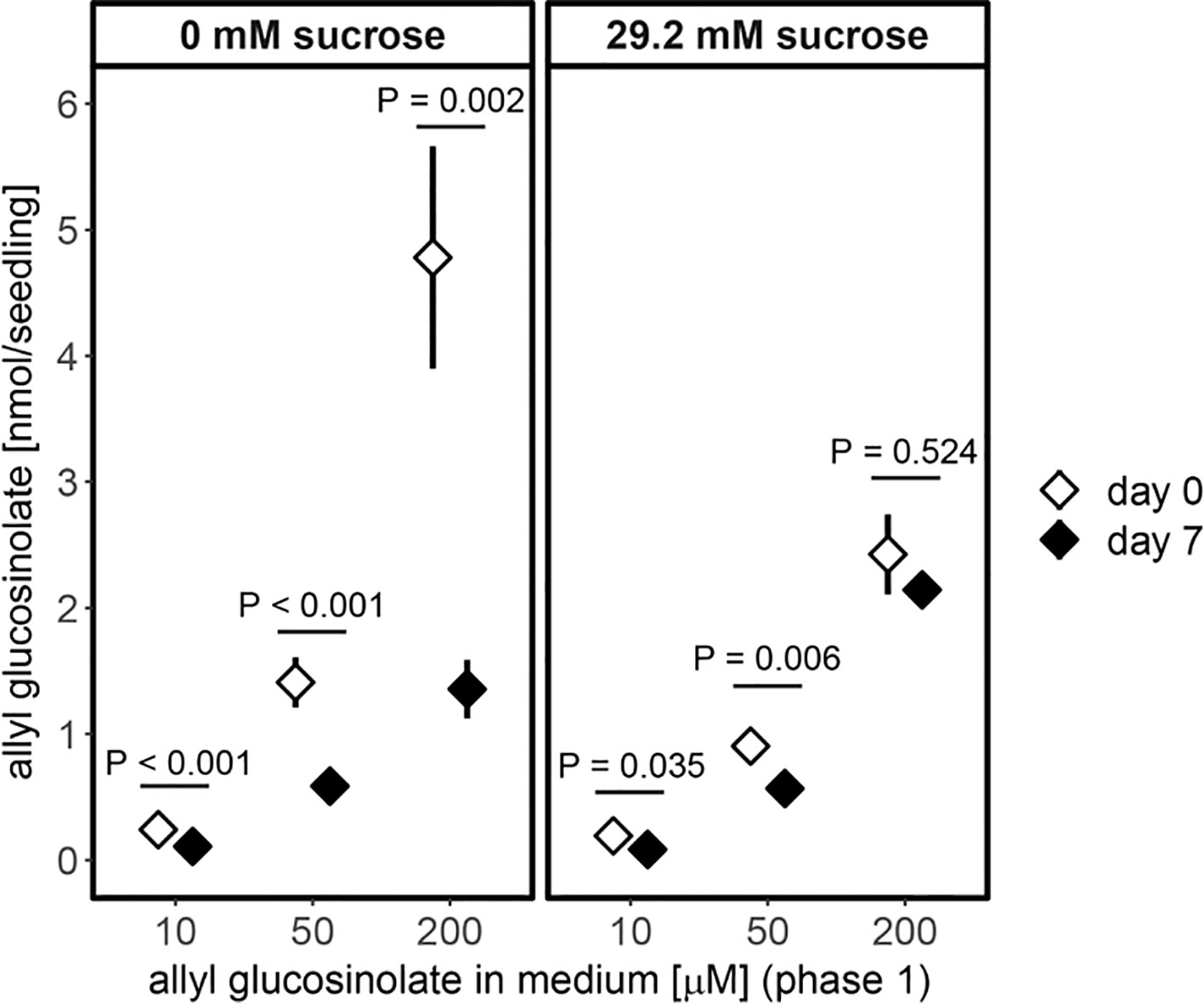
Figure 6 Accumulation of allyl glucosinolates in six-day-old seedlings (white diamond, transfer day) and its turnover after seven days on allyl glucosinolate-free medium (black diamond, end of phase 2) is dependent on the concentration of allyl glucosinolate present in the growth medium (phase 1) and the absence (left panel) and presence of 29.2 mM sucrose (right panel). Depicted are means ± SE, N = 15–18 for 0 mM sucrose (data pooled from two independent experiments) and N = 5–8 for 29.2 mM sucrose treatment (one experiment). Pairwise comparison of day 0 to day 7 within an allyl glucosinolate and sucrose treatment group was performed using Wilcoxon rank sum test (P-value adjustment method: Benjamini & Hochberg). Details on the statistical analysis are provide in Supplementary Table 11. For details on the experimental set-up refer to Supplementary Figure 3. For the effect of varying nitrogen and sulfur content on the growth medium on allyl glucosinolate turnover refer to Supplementary Figure 8. Fresh weight of seedlings grown on nitrogen and/or sulfur sufficient and limiting conditions (phase 2) depending on the allyl glucosinolate concentration in phase 1 on sucrose-free medium is plotted in Supplementary Figure 9.
The accumulation of raphanusamic acid was positively correlated with increased allyl glucosinolate accumulation (P(concentration) < 0.001) and nitrogen availability (P(N) < 0.001; Figure 7). This effect was stronger for seedlings that had initially grown on high allyl glucosinolate concentrations (P(N * concentration) < 0.001). Although allyl glucosinolate levels were similar across all four media seven days after transfer depending on the initial allyl glucosinolate concentration in Phase 1 (Supplementary Figure 8), raphanusamic acid levels varied among media (Figure 7). Raphanusamic acid accumulated to amounts expected based on turnover of allyl glucosinolate under nitrogen limiting conditions (−N) if 1 nmol allyl glucosinolate is detected as 1 nmol raphanusamic acid. For nitrogen sufficient conditions (+N), appr. 2-fold higher amounts were detected as could be expected from allyl glucosinolate turnover alone (comparing the difference of days 0 and 7, Figure 6, to the levels in Figure 7), suggesting that raphanusamic acid also stems from turnover of endogenous glucosinolates in intact plant tissue.
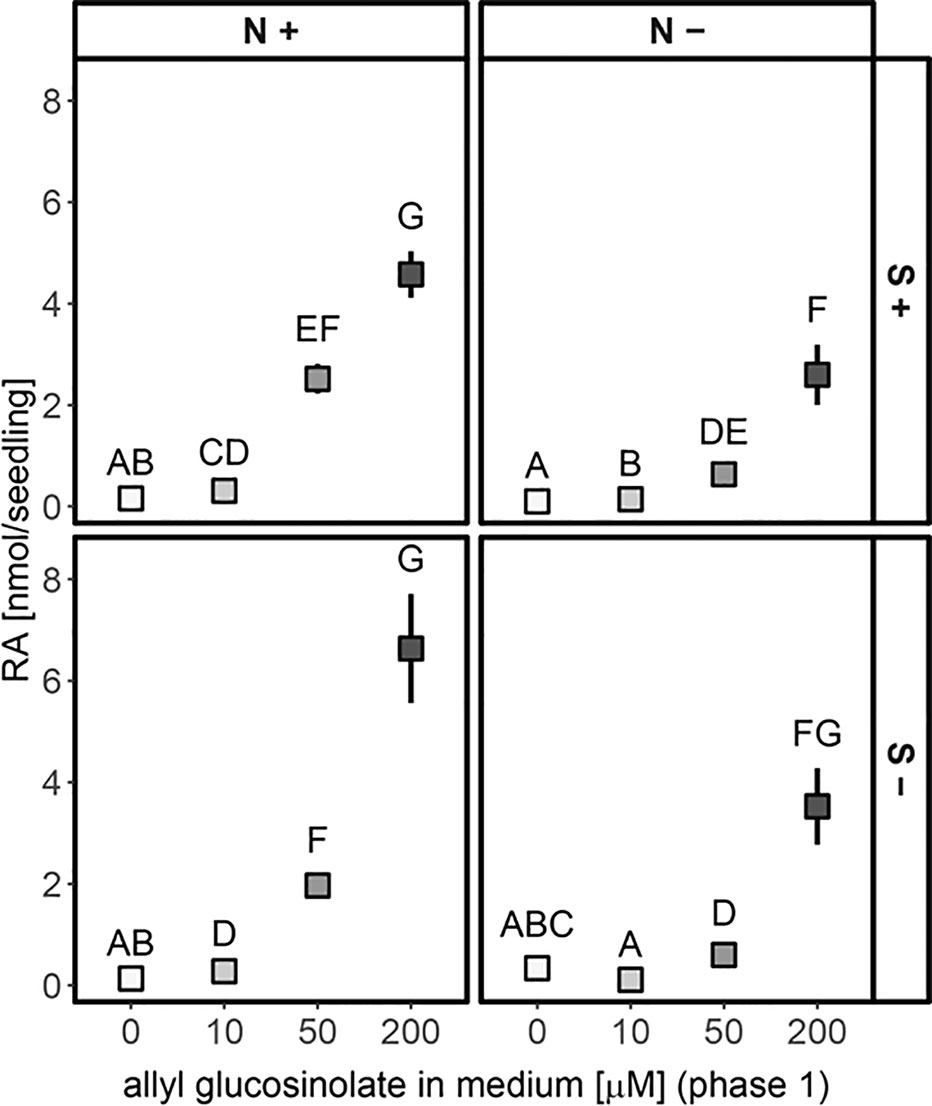
Figure 7 Accumulation of raphanusamic acid in 13-day-old seedlings dependent on the concentration of allyl glucosinolate supplied in the growth medium in the first six days of seedling development (phase 1, grey scale) and the sulfur (S) and nitrogen (N) availability for additional seven days (phase 2) on 0 mM sucrose medium. Depicted are means ± SE, N = 15–18, data pooled from two independent experiments. Letters denote significant differences at the 0.05 level with pairwise comparison using Wilcoxon rank sum test (P-value adjustment method: Benjamini & Hochberg). Details on the statistical analysis are provided in Supplementary Table 14. For details on the experimental set-up refer to Supplementary Figure 3.
Allyl Glucosinolate Treatment Alters the Turnover of Embryo-Synthesized Glucosinolates
In seedlings grown on 50 µM allyl glucosinolate-containing medium, short-chain aliphatic glucosinolates showed the same pronounced increase seven days after germination (Figures 2A and 8), but accumulated to higher levels compared to those in seedlings grown on allyl glucosinolate-free medium (P = 0.024, Supplementary Table 10). This was driven by a 30% higher accumulation of 4mtb glucosinolate compared to the control group (Supplementary Table 15). Indolic glucosinolates, showing the strongest increase rate at this developmental stage, increased continuously over time and reached 43% higher levels in allyl glucosinolate-treated seedlings than in control seedlings nine days after germination. In contrast, neither total long-chain aliphatic glucosinolates nor any individual glucosinolate in the group was affected by allyl glucosinolate feeding Figures 2A, 8, and Supplementary Table 15). Until eight days after germination, allyl glucosinolate-treated seedlings also maintained significantly higher levels of embryo-synthesized glucosinolates compared to the control group (P = 0.008) (Figure 8). In this experimental setup, the levels of embryo-synthesized glucosinolates decreased over time until they were not detectable nine days after germination irrespective of the presence of allyl glucosinolate in the medium.
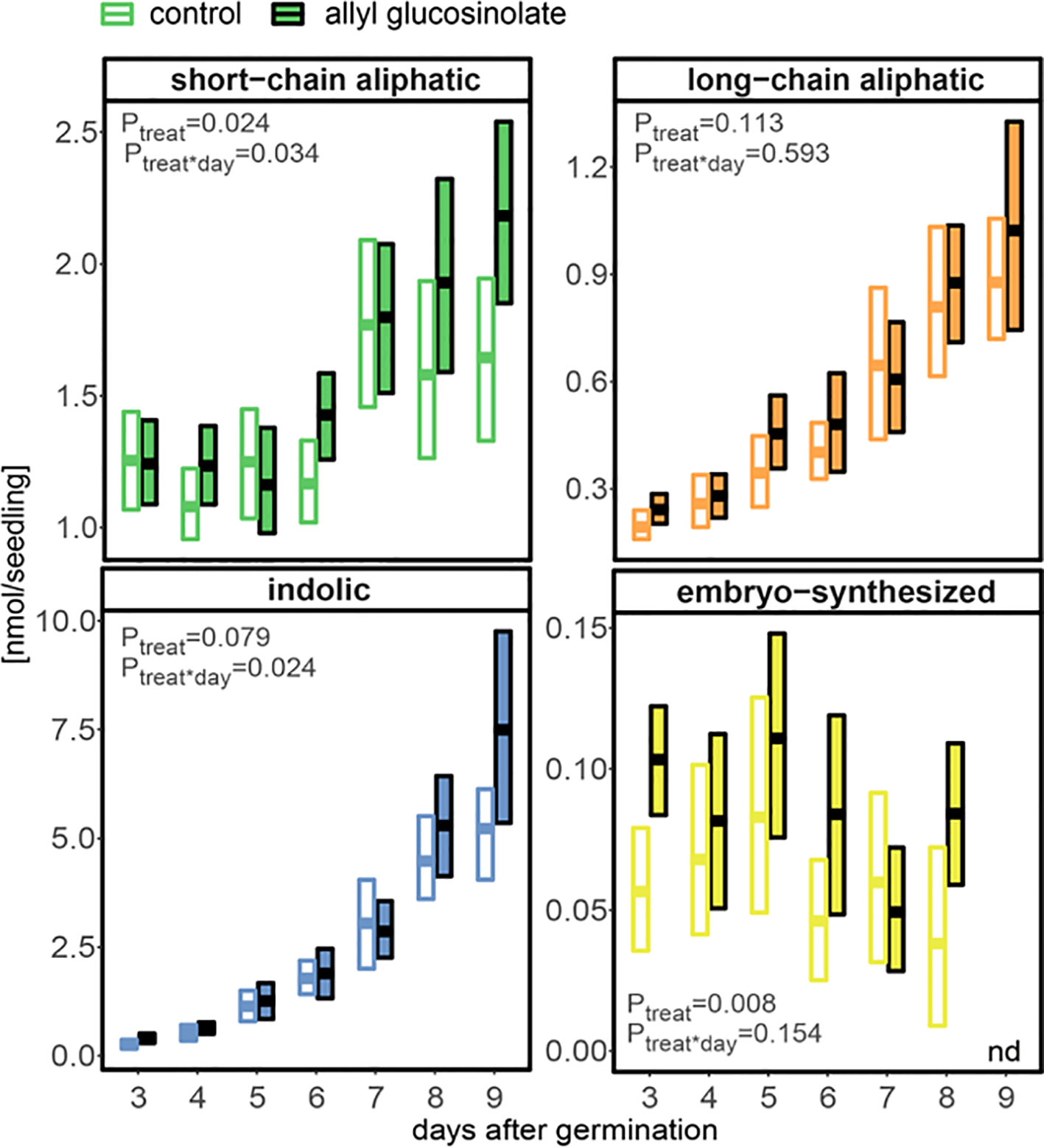
Figure 8 The influence of exogenously applied allyl glucosinolate on endogenous glucosinolate profiles in A. thaliana Col-0 seedlings. Seedlings were grown on control (glucosinolate-free) medium (white fill) and medium containing 50 µM allyl glucosinolate (colored fill) for nine days after germination. Plotted are means (bold bar) and 95% confidence interval (box), N = 6–19 (one experimental round). Embryo-synthesized glucosinolates were not detected (nd) in nine-day-old seedlings. Statistical comparison of the control and allyl glucosinolate treatment was performed with a linear model of the following function: aov(analyte ∼ treat*day), with treat = treatment. Details on the statistical analysis including means, standard error and post-hoc tests are in Supplementary Table 1 for the control treatment and Supplementary Table 15 for allyl glucosinolate treatment.
Nine days after germination, seedlings grown on 50 µM allyl glucosinolate-containing medium had accumulated >35% higher total levels of glucosinolates than seedlings grown on control medium (Figures 2A and 8). To compare the effects of allyl glucosinolate feeding to those of nitrogen and sulfur availability, and sucrose on endogenous glucosinolate profiles, we performed a multiple factor analysis (MFA) on a principle component analysis (PCA) on the entirety of the individual glucosinolates detected with our method (for all values and treatment combinations, Supplementary Table 17). The analysis was performed on single seedlings that were first grown on various concentrations of allyl glucosinolate with or without the addition of 29.2 mM sucrose (phase 1). Six days after germination, the seedlings were grown for another seven days (in sum, 13-day-old seedlings) on glucosinolate-free media that were sufficient or limiting in nitrogen and/or sulfur and either contained 29.2mM sucrose, or not (phase 2, Supplementary Figure 3). These four variations in medium composition (allyl glucosinolate concentration in Phase 1; nitrogen content, sulfur content and sucrose content in Phase 2) were considered as categorical variables in the principal component analysis, and the individual glucosinolates were further divided into groups (as in Supplementary Table 17) as continuous variables for the MFA-PCA analysis. Of the treatments, the absence or presence of sucrose (Figure 9, bottom right panel) is the dominant factor describing the separation of the data in the first dimension.
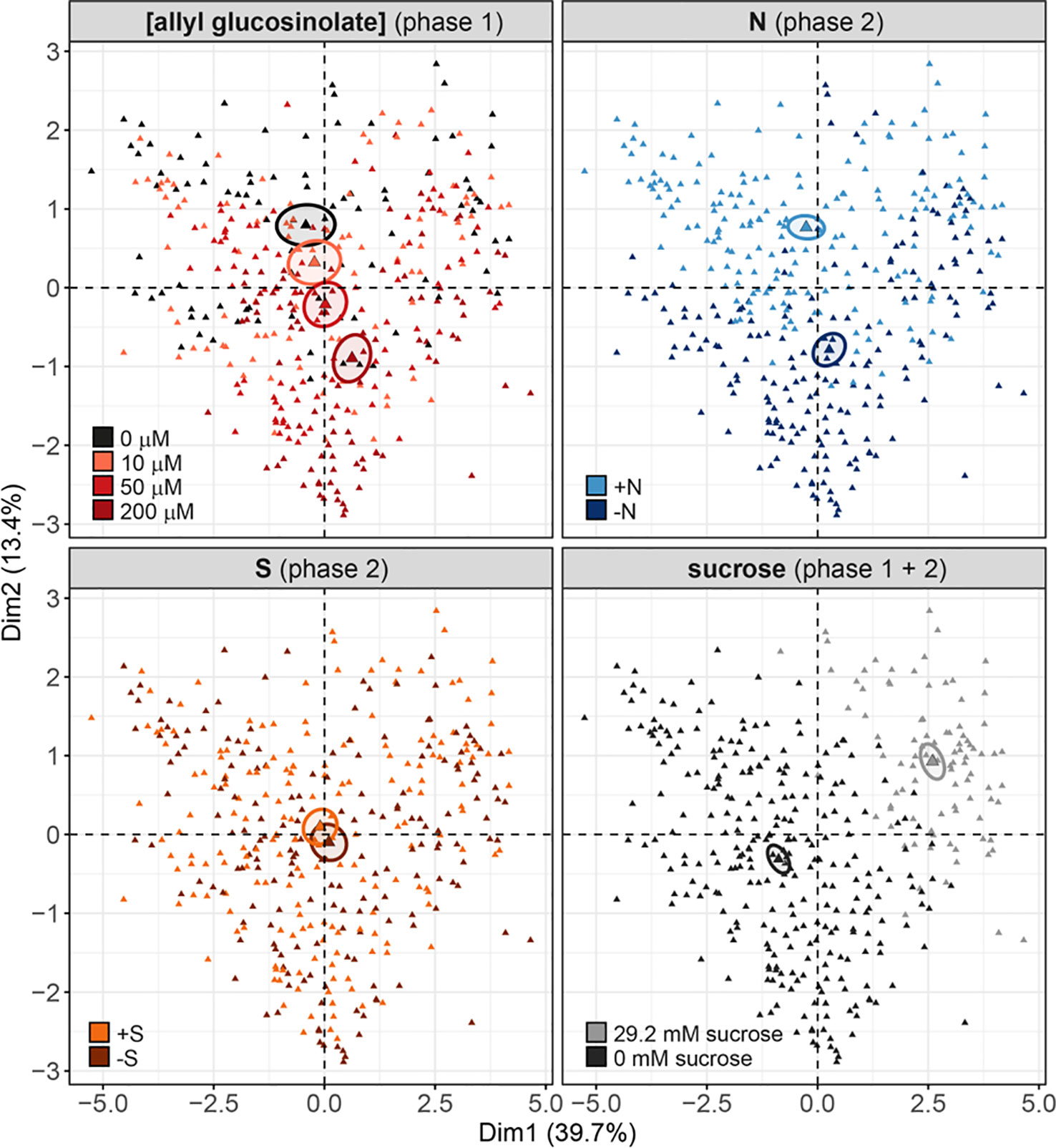
Figure 9 Multiple factor analysis (MFA) of the effects of exogenously feeding allyl glucosinolate (in 0, 10, 50 and 200 μM), varying nitrogen in the growth medium (0.3 mM (−N) and 3 mM (+N)), varying sulfur in the growth medium (0.015 mM (−S) and 1.68 mM (+S) and the effects of the presence (29.2 mM) and absence and sucrose on endogenous glucosinolate levels. Seedlings were grown on medium containing different concentrations of allyl glucosinolate for six days (phase 1) and transferred to glucosinolate-free medium varying in their nitrogen and sulfur content for seven days (phase 2, for details on the experimental set-up refer to Supplementary Figure 3). Sucrose treatment was constant over phase 1 and phase 2. Each triangle represents the sum of all individual glucosinolate concentrations of one single seedling, data pooled from two independent experiments. For a list of the glucosinolate concentrations and the statistical analysis by treatment group refer to Supplementary Tables 16 and 17. For a list of the contribution of the treatment and glucosinolate classes, and of the contributions of the individual glucosinolates to the separation of dimensions 1 and 2, refer to Supplementary Figure 10.
Overall, the presence of sucrose in the growth medium correlates with lowered total glucosinolate levels (Supplementary Tables 16 and 17) independent of other variable factors in this experiment. The glucosinolates most strongly contributing to this dimension (thus correlating with the effect of sucrose treatment) are 2PE and NMOI3M, followed by C8 aliphatic glucosinolates, embryo-synthesized glucosinolates and short-chain aliphatic glucosinolate to a lesser extent (Supplementary Figure 10, bottom left panel). Dimension 2 best describes the effect of nitrogen variation, followed by allyl glucosinolate feeding (Figure 9, top panels; Supplementary Figure 10, top right panel). In the 0 mM sucrose treatment group, we find that total glucosinolate levels are negatively correlated with allyl glucosinolate concentration affecting aliphatic and indolic glucosinolates (Supplementary Table 16). Nitrogen limitation negatively impacts short-chain aliphatic glucosinolate levels dependent on the allyl glucosinolate concentration fed, particularly negatively affecting 4mtb levels (but not raising 4msb levels) (Supplementary Figure 10, bottom right panel; Supplementary Table 17). Variation in sulfur availability has a negligible effect on the overall changes in in glucosinolate profiles, and this effect could not be resolved in any other dimension described in this model (dimensions 3 and upwards not shown).
Discussion
Glucosinolate Biosynthesis and Turnover Are Coordinated According to Nutrient Availability
Steady-state levels of metabolites are determined by the rates of biosynthesis and turnover. Whereas the regulation of the biosynthetic pathways and their regulators has been intensely studied, especially at the transcriptional level, glucosinolate turnover pathways remained largely elusive and their impact on steady-state levels of glucosinolates awaits to be determined. To this end, we investigated the nutrient-driven dynamics of glucosinolate levels during seedling development in A. thaliana, i.e. at a developmental stage at which biosynthesis and turnover of glucosinolates coincide. Between three and nine days after germination, the average rate of total glucosinolate accumulation was ca. 1 nmol/day (Figure 2A), indicating that the rate of biosynthesis exceeds the rate of turnover at this stage. Embryo-synthesized glucosinolates were detectable at least nine days after germination under our experimental conditions (Figure 2B) and generally found to decrease during seedling development (Figures 2B, 4B, and Supplementary Table 7) demonstrating turnover of these compounds. Similarly, the levels of allyl glucosinolate exogenously applied during the first days of seedling development decreased after the seedlings had been moved to allyl glucosinolate-free medium (Figure 6). Although, we cannot determine the relative impact of biosynthesis and turnover on those glucosinolate structures that are de novo synthesized in the seedlings, our data confirm previous findings (Petersen et al., 2002; Brown et al., 2003) that seedlings possess the enzymatic machinery to metabolize glucosinolates.
Metabolic processes, growth and development in A. thaliana seedlings are regulated by nutrient availability, for example a balanced carbon to nitrogen ratio (Martin et al., 2002). The addition of 29.2 mM (1 %) sucrose to the growth medium with sufficient concentrations of sulfur and nitrogen alters glucosinolate profiles (Figure 3) (Gigolashvili et al., 2007; Francisco et al., 2016a). Under our experimental conditions, external sucrose had a stronger effect on the dynamics of long-chain aliphatic and indolic glucosinolate accumulation than on short-chain aliphatic glucosinolates, without changes in glucosinolate composition within these three groups (Figure 3A and Supplementary Table 3). In twelve-day-old seedlings, sucrose and glucose led to higher glucosinolate accumulation in roots and shoots, whereas fructose treatment did not result in significant changes in glucosinolate content (Figure 3B). Although the expression of MYB28, a positive regulator of aliphatic glucosinolate biosynthesis, is short-term inducible by glucose (Gigolashvili et al., 2007), long term exposure to external carbon sources appears to affect overall glucosinolate content by regulating seedling growth (Supplementary Figure 4) without fine-tuning glucosinolate composition.
Sucrose treatment further affected accumulation of the exogenous allyl glucosinolate, which could reflect a negative effect of sucrose on allyl glucosinolate uptake or a positive effect on turnover (Figure 6). When we subsequently followed the turnover of allyl glucosinolate after transfer of the seedlings from plates containing 200 µM allyl glucosinolate to allyl glucosinolate-free media, lower amounts were metabolized in seedlings kept on sucrose-containing plates. This observation suggests a lower turnover rate of allyl glucosinolate in the presence of sucrose and shows that sucrose treatment regulates not only glucosinolate biosynthesis but also turnover.
Also sulfur and nitrogen limitation negatively affected the accumulation of all de novo synthesized glucosinolate classes (indolic, short- and long-chain aliphatic glucosinolates). Under nitrogen limiting conditions, the strongest fold-reduction was detected for short-chain aliphatic glucosinolates, which are, however, less abundant than indolic and long-chain aliphatic glucosinolates at this developmental stage (Figure 4A). In contrast, sulfur limitation had the strongest negative impact on long-chain aliphatic glucosinolates. Although nitrogen has the more pronounced quantitative effect on glucosinolate levels, both nitrogen and sulfur negatively affected de novo synthesis of all three groups under conditions of long-term nutrient limitation. In addition to the overall reduction in glucosinolates, sulfur or nitrogen limitation resulted in distinct changes in the levels of individual glucosinolates (Supplementary Table 7), indicating that these macronutrients provide a regulatory input different from sucrose.
Because glucosinolates can represent up to 30 % of the total sulfur in a given tissue, they have been discussed as potential source for sulfur under sulfur limiting conditions (Falk et al., 2007). However, in seedlings of Brassica juncea and B. napus challenged by sulfate deprivation, sulfur is not mobilized from glucosinolates (Aghajanzadeh et al., 2014). Similarly, neither sulfur nor nitrogen limitation had a significant effect on the turnover of embryo-synthesized glucosinolates under our experimental conditions (Figure 4) or on the turnover of exogenously fed allyl glucosinolate (Supplementary Figure 8). Although sulfur and nitrogen availability do not seem to change glucosinolate turnover rates in Brassicaceae seedlings, this may nevertheless happen at later developmental stages.
Exogenously Applied Allyl Glucosinolate Is Metabolized and Fine-Tunes Glucosinolate Biosynthesis and Turnover
When grown on medium containing allyl glucosinolate, A. thaliana seedlings gradually accumulated allyl glucosinolate until eight days after germination in a dose-dependent manner (Figures 5A and 6). Although exogenously applied glucosinolates have previously been shown to be transported between root and shoot in the same way as endogenous glucosinolates and to undergo further side chain modification by the glucosinolate biosynthetic machinery (Li and Quiros, 2003; Andersen et al., 2013; Burow et al., 2015; Francisco et al., 2016a; Malinovsky et al., 2017), it cannot be ruled out that an unknown proportion of allyl glucosinolate provided in the medium will encounter myrosinase-catalyzed breakdown. Nevertheless, the addition of sucrose to the growth medium quantitatively affects allyl glucosinolate accumulation depending on seedling development (Figure 6 and Supplementary Figure 7) (Francisco et al., 2016a). Exogenously applied allyl glucosinolate further affects the endogenous glucosinolate profile (Figures 5 and 8) (Francisco et al., 2016a). Short -chain aliphatic and indolic glucosinolates accumulated to higher levels in seedlings grown on allyl glucosinolate compared to seedlings grown on allyl glucosinolate-free medium, although this trend was not significant for long-chain aliphatic glucosinolates (Figure 8). This further supports the positive regulatory effect of allyl glucosinolate on the biosynthesis of glucosinolates from methionine and tryptophan (Francisco et al., 2016a).
The presence of sucrose resulted in decreased accumulation of allyl glucosinolate depending on the initial allyl glucosinolate concentration in the growth medium (Figure 6), which is likely due to a lower uptake rate of allyl glucosinolate as discussed above. In the absence of external sucrose, seedlings grown on allyl glucosinolate-containing medium maintained higher levels of the embryo-synthesized glucosinolates (Figure 8). Likewise, allyl glucosinolate feeding did not lead to altered levels of the short-chain aliphatic 4-methylsulfinylbutyl glucosinolate predominantly present in adult plants, but resulted in altered levels of 4mtb glucosinolate depending on seedling age, illustrating the fine-tuning effect of allyl glucosinolate on the glucosinolate profile in seedlings. Because a substantial proportion of 4mtb but not 4msb glucosinolate in seedlings is derived from the seed, we cannot conclude whether allyl glucosinolate affects biosynthesis and/or turnover at this developmental stage.
Structure-specific effects on growth and development have also been shown for the structurally unrelated 3ohp glucosinolate, which belongs to the embryo-synthesized glucosinolates in A. thaliana Col-0. 3ohp glucosinolate was recently shown to inhibit seedling root growth due to its signaling function via genes in the sugar sensing Target Of Rapamycin (TOR) pathway (Malinovsky et al., 2017). Dose-dependent effects on root growth have further been shown for the glucosinolate breakdown products indole-3-carbinol and allyl isothiocyanate, although at much higher concentrations and in case of indole-3-carbinol through auxin signaling (Katz et al., 2015; Urbancsok et al., 2017). Based on these observations, we propose a model in which the glucosinolate composition and the metabolic status coordinately feedback regulate glucosinolate profiles and seedling development.
Raphanusamic Acid Levels Reflect the Levels of Endogenous Glucosinolates
Turnover of glucosinolates via the formation of isothiocyanates and their conjugation with glutathione yields raphanusamic acid (Bednarek et al., 2009; Piślewska-Bednarek et al., 2018). Although glucosinolates are predominantly metabolized to simple nitriles in homogenates of A. thaliana seedlings (Wittstock et al., 2016b), we monitored the levels of raphanusamic acid as potential indicator of glucosinolate turnover during seedling development and indeed detected increasing amounts from five days after germination (Figure 2B). Over time, the kinetics of raphanusamic acid accumulation did, however, not follow the turnover of the embryo-synthesized glucosinolates (Figures 2 and 4B). Raphanusamic acid levels were instead correlated with the accumulation of total glucosinolate levels (Figure 4C). Considering that one week after germination, the indolic glucosinolate NMOI3M constitutes >40% of total glucosinolates and accumulates at high rate (Supplementary Table 1), raphanusamic acid may at least partially reflect the biosynthetic rate of indolic or possibly of total glucosinolates.
In support of this hypothesis, higher levels of raphanusamic acid repeatedly coincided with high flux through the glucosinolate biosynthetic pathways. The levels of raphanusamic acid and glucosinolates were reduced under nitrogen and sulfur limiting conditions (Figure 4). Further, the accumulation of allyl glucosinolate over time positively correlated with raphanusamic acid, an effect that persisted seven days after the seedlings had been transferred to allyl glucosinolate-free media (Figure 7). These increased levels of raphanusamic acid upon and after allyl glucosinolate feeding may stem from allyl glucosinolate itself but may also reflect an allyl glucosinolate-mediated increased biosynthetic rate. Because raphanusamic acid can possibly be formed form all glucosinolates irrespective of their side chain chemistry, it cannot carry glucosinolate structure-specific regulatory information. Nevertheless, this intermediate of glucosinolate metabolism could serve as checkpoint allowing the plant to measure the flux through the glucosinolate biosynthetic and turnover pathways and thereby to dynamically adjust glucosinolate levels to internal and external signals.
Data Availability Statement
All datasets generated for this study are included in the article/Supplementary Material.
Author Contributions
VJ and MB design the experiments. KW and SM conducted the sugar dependency experiments. VJ carried out all other experimental work, the statistical analyses and made the figures. VJ and MB wrote the manuscript.
Funding
This work was supported by The Danish National Research Foundation, DNRF grant 99 (VJ, KW, MB) and Villumfonden, project no. 13169 (VJ, MB).
Conflict of Interest
The authors declare that the research was conducted in the absence of any commercial or financial relationships that could be construed as a potential conflict of interest.
Acknowledgments
We would like to thank Christoph Crocoll and the DynaMo Metabolomics Facility for help with Data Acquisition.
Supplementary Material
The Supplementary Material for this article can be found online at: https://www.frontiersin.org/articles/10.3389/fpls.2019.01560/full#supplementary-material
References
Aghajanzadeh, T., Hawkesford, M. J., De Kok, L. J. (2014). The significance of glucosinolates for sulfur storage in Brassicaceae seedlings. Front. Plant Sci. 5, 704. doi: 10.3389/fpls.2014.00704
Andersen, T. G., Nour-Eldin, H. H., Fuller, V. L., Olsen, C. E., Burow, M., Halkier, B. A. (2013). Integration of biosynthesis and long-distance transport establish organ-specific glucosinolate profiles in vegetative Arabidopsis. Plant Cell 25, 3133–3145. doi: 10.1105/tpc.113.110890
Augustine, R., Bisht, N. C. (2016). “Glucosinolates,” in Regulation of Glucosinolate Metabolism: From Model Plant Arabidopsis thaliana to Brassica Crops. Eds. Mérillon, J.-M., Ramawat, K. G. (Cham: Springer International Publishing), 1–37. doi: 10.1007/978-3-319-26479-0_3-1
Barth, C., Jander, G. (2006). Arabidopsis myrosinases TGG1 and TGG2 have redundant function in glucosinolate breakdown and insect defense. Plant J. 46, 549–562. doi: 10.1111/j.1365-313X.2006.02716.x
Bednarek, P., Pislewska-Bednarek, M., Svatos, A., Schneider, B., Doubsky, J., Mansurova, M., et al. (2009). A glucosinolate metabolism pathway in living plant cells mediates broad-spectrum antifungal defense. Science 323, 101–106. doi: 10.1126/science.1163732
Bednarek, P. (2012). Sulfur-containing secondary metabolites from Arabidopsis thaliana and other Brassicaceae with function in plant immunity. Chembiochem 13, 1846–1859. doi: 10.1002/cbic.201200086
Bekaert, M., Edger, P. P., Hudson, C. M., Pires, J. C., Conant, G. C. (2012). Metabolic and evolutionary costs of herbivory defense: systems biology of glucosinolate synthesis. New Phytol. 196, 596–605. doi: 10.1111/j.1469-8137.2012.04302.x
Blažević, I., Montaut, S., Burcul, F., Olsen, C. E., Burow, M., Rollin, P., et al. (2019). Glucosinolate structural diversity, identification, chemical synthesis and metabolism in plants. Phytochemistry. doi: 10.1016/j.phytochem.2019.112100
Booth, E. J., Walker, K. C., Griffiths, D. W. (1991). A time-course study of the effect of sulphur on glucosinolates in oilseed rape (Brassica napus) from the vegetative stage to maturity. J. Sci. Food Agric. 56, 479–493. doi: 10.1002/jsfa.2740560408
Brown, K. K., Hampton, M. B. (2011). Biological targets of isothiocyanates. Biochim. Biophys. Acta 1810, 888–894. doi: 10.1016/j.bbagen.2011.06.004
Brown, P. D., Tokuhisa, J. G., Reichelt, M., Gershenzon, J. (2003). Variation of glucosinolate accumulation among different organs and developmental stages of Arabidopsis thaliana. Phytochemistry 62, 471–481. doi: 10.1016/S0031-9422(02)00549-6
Burow, M., Halkier, B. A. (2017). How does a plant orchestrate defense in time and space? Using glucosinolates in Arabidopsis as case study. Curr. Opin. Plant Biol. 38, 142–147. doi: 10.1016/j.pbi.2017.04.009
Burow, M., Losansky, A., Müller, R., Plock, A., Kliebenstein, D. J., Wittstock, U. (2009). The genetic basis of constitutive and herbivore-induced ESP-independent nitrile formation in Arabidopsis. Plant Physiol. 149, 561–574. doi: 10.1104/pp.108.130732
Burow, M., Halkier, B. A., Kliebenstein, D. J. (2010). Regulatory networks of glucosinolates shape Arabidopsis thaliana fitness. Curr. Opin. Plant Biol. 13, 348–353. doi: 10.1016/j.pbi.2010.02.002
Burow, M., Atwell, S., Francisco, M., Kerwin, R. E., Halkier, B. A., Kliebenstein, D. J. (2015). The glucosinolate biosynthetic gene aop2 mediates feed-back regulation of jasmonic acid signaling in Arabidopsis. Mol. Plant 8, 1201–1212. doi: 10.1016/j.molp.2015.03.001
Burow, M. (2016). “Gucosinolates Advances in Botanical Research,” in Complex Environments Interact With Plant Development to Shape Glucosinolate Profiles. Ed. Kopriva, S. (Elsevier Ltd.), 15–30. doi: 10.1016/bs.abr.2016.06.001
Chun, J.-H., Kim, S., Arasu, M. V., Al-Dhabi, N. A., Chung, D. Y., Kim, S.-J. (2017). Combined effect of Nitrogen, Phosphorus and Potassium fertilizers on the contents of glucosinolates in rocket salad (Eruca sativa Mill.). Saudi J. Biol. Sci. 24, 436–443. doi: 10.1016/j.sjbs.2015.08.012
Crocoll, C., Halkier, B. A., Burow, M. (2016). Analysis and quantification of glucosinolates. Curr. Protoc. Plant Biol. 1 (2), 385–409. doi: 10.1002/cppb.20027
D’Auria, J. C., Gershenzon, J. (2005). The secondary metabolism of Arabidopsis thaliana: growing like a weed. Curr. Opin. Plant Biol. 8, 308–316. doi: 10.1016/j.pbi.2005.03.012
Falk, K. L., Tokuhisa, J. G., Gershenzon, J. (2007). The effect of sulfur nutrition on plant glucosinolate content: physiology and molecular mechanisms. Plant Biol. (Stuttg.) 9, 573–581. doi: 10.1055/s-2007-965431
Francisco, M., Joseph, B., Caligagan, H., Li, B., Corwin, J. A., Lin, C., et al. (2016a). The defense metabolite, allyl glucosinolate, modulates Arabidopsis thaliana biomass dependent upon the endogenous glucosinolate pathway. Front. Plant Sci. 7, 774. doi: 10.3389/fpls.2016.00774
Francisco, M., Joseph, B., Caligagan, H., Li, B., Corwin, J. A., Lin, C., et al. (2016b). Genome wide association mapping in Arabidopsis thaliana identifies novel genes involved in linking allyl glucosinolate to altered biomass and defense. Front. Plant Sci. 7, 1010. doi: 10.3389/fpls.2016.01010
Frerigmann, H., Gigolashvili, T. (2014). Update on the role of R2R3-MYBs in the regulation of glucosinolates upon sulfur deficiency. Front. Plant Sci. 5, 626. doi: 10.3389/fpls.2014.00626
Gigolashvili, T., Yatusevich, R., Berger, B., Müller, C., Flügge, U.-I. (2007). The R2R3-MYB transcription factor HAG1/MYB28 is a regulator of methionine-derived glucosinolate biosynthesis in Arabidopsis thaliana. Plant J. 51, 247–261. doi: 10.1111/j.1365-313X.2007.03133.x
Gigolashvili, T., Berger, B., Flügge, U.-I. (2009). Specific and coordinated control of indolic and aliphatic glucosinolate biosynthesis by R2R3-MYB transcription factors in Arabidopsis thaliana. Phytochem. Rev. 8, 3–13. doi: 10.1007/s11101-008-9112-6
Guo, R., Yuan, G., Wang, Q. (2011). Sucrose enhances the accumulation of anthocyanins and glucosinolates in broccoli sprouts. Food Chem. 129, 1080–1087. doi: 10.1016/j.foodchem.2011.05.078
Haughn, G. W., Davin, L., Giblin, M., Underhill, E. W. (1991). Biochemical genetics of plant secondary metabolites in Arabidopsis thaliana: the glucosinolates. Plant Physiol. 97, 217–226. doi: 10.1104/pp.97.1.217
Huseby, S., Koprivova, A., Lee, B.-R., Saha, S., Mithen, R., Wold, A.-B., et al. (2013). Diurnal and light regulation of sulphur assimilation and glucosinolate biosynthesis in Arabidopsis. J. Exp. Bot. 64, 1039–1048. doi: 10.1093/jxb/ers378
Inamori, Y., Muro, C., Tanaka, R., Adachi, A., Miyamoto, K., Tsujibo, H. (1992). Phytogrowth-inhibitory activity of sulphur-containing compounds. I. Inhibitory activities of thiazolidine derivatives on plant growth. Chem. Pharm. Bull. 40, 2854–2856. doi: 10.1248/cpb.40.2854
Janowitz, T., Trompetter, I., Piotrowski, M. (2009). Evolution of nitrilases in glucosinolate-containing plants. Phytochemistry 70, 1680–1686. doi: 10.1016/j.phytochem.2009.07.028
Jensen, L. M., Halkier, B. A., Burow, M. (2014). How to discover a metabolic pathway? An update on gene identification in aliphatic glucosinolate biosynthesis, regulation and transport. Biol. Chem. 395, 529–543. doi: 10.1515/hsz-2013-0286
Jensen, L. M., Jepsen, H. S. K., Halkier, B. A., Kliebenstein, D. J., Burow, M. (2015). Natural variation in cross-talk between glucosinolates and onset of flowering in Arabidopsis. Front. Plant Sci. 6, 697. doi: 10.3389/fpls.2015.00697
Katz, E., Nisani, S., Yadav, B. S., Woldemariam, M. G., Shai, B., Obolski, U., et al. (2015). The glucosinolate breakdown product indole-3-carbinol acts as an auxin antagonist in roots of Arabidopsis thaliana. Plant J. 82, 547–555. doi: 10.1111/tpj.12824
Kerwin, R. E., Jimenez-Gomez, J. M., Fulop, D., Harmer, S. L., Maloof, J. N., Kliebenstein, D. J. (2011). Network quantitative trait loci mapping of circadian clock outputs identifies metabolic pathway-to-clock linkages in Arabidopsis. Plant Cell 23, 471–485. doi: 10.1105/tpc.110.082065
Kissen, R., Rossiter, J. T., Bones, A. M. (2009). The "mustard oil bomb": not so easy to assemble?! Localization, expression and distribution of the components of the myrosinase enzyme system. Phytochem. Rev. 8, 69–86. doi: 10.1007/s11101-008-9109-1
Kliebenstein, D. J., Lambrix, V. M., Reichelt, M., Gershenzon, J., Mitchell-Olds, T. (2001). Gene duplication in the diversification of secondary metabolism: tandem 2-oxoglutarate-dependent dioxygenases control glucosinolate biosynthesis in Arabidopsis. Plant Cell 13, 681–693. doi: 10.1105/tpc.13.3.681
Kliebenstein, D. J., Rowe, H. C., Denby, K. J. (2005). Secondary metabolites influence Arabidopsis/Botrytis interactions: variation in host production and pathogen sensitivity. Plant J. 44, 25–36. doi: 10.1111/j.1365-313X.2005.02508.x
Kliebenstein, D. J., D’Auria, J. C., Behere, A. S., Kim, J. H., Gunderson, K. L., Breen, J. N., et al. (2007). Characterization of seed-specific benzoyloxyglucosinolate mutations in Arabidopsis thaliana. Plant J. 51, 1062–1076. doi: 10.1111/j.1365-313X.2007.03205.x
Kopriva, S., Gigolashvili, T. (2016). Chapter five - glucosinolate synthesis in the context of plant metabolism. Adv. In Botanical Res. 80, 99–124. doi: 10.1016/bs.abr.2016.07.002
Koprivova, A., Suter, M., Camp, R. O., Brunold, C., Kopriva, S. (2000). Regulation of sulfate assimilation by nitrogen in Arabidopsis. Plant Physiol. 122, 737–746. doi: 10.1104/pp.122.3.737
Kopsell, D. A., Barickman, T. C., Sams, C. E., McElroy, J. S. (2007). Influence of nitrogen and sulfur on biomass production and carotenoid and glucosinolate concentrations in watercress (Nasturtium officinale R. Br.). J. Agric. Food Chem. 55, 10628–10634. doi: 10.1021/jf072793f
Koroleva, O. A., Davies, A., Deeken, R., Thorpe, M. R., Tomos, A. D., Hedrich, R. (2000). Identification of a new glucosinolate-rich cell type in Arabidopsis flower stalk. Plant Physiol. 124, 599–608. doi: 10.1104/pp.124.2.599
Koroleva, O. A., Gibson, T. M., Cramer, R., Stain, C. (2010). Glucosinolate-accumulating S-cells in Arabidopsis leaves and flower stalks undergo programmed cell death at early stages of differentiation. Plant J. 64, 456–469. doi: 10.1111/j.1365-313X.2010.04339.x
Krumbein, A., Schonhof, I., Rühlmann, J., Widell, S. (2001). “Influence of sulphur and nitrogen supply on flavour and health-affecting compounds in Brassicaceae,” in Plant Nutrition. Eds. Horst, W. J., Schenk, M. K., Bürkert, N., Claassen, A., Flessa, H., Frommer, W. B., Goldbach, H., Olfs, H. W., Römheld, V., Sattelmacher, B. (Dordrecht: Springer Netherlands), 294–295. doi: 10.1007/0-306-47624-X_141
Li, G., Quiros, C. F. (2003). In planta side-chain glucosinolate modification in Arabidopsis by introduction of dioxygenase Brassica homolog BoGSL-ALK. Theor. Appl. Genet. 106, 1116–1121. doi: 10.1007/s00122-002-1161-4
Li, S., Schonhof, I., Krumbein, A., Li, L., Stützel, H., Schreiner, M. (2007). Glucosinolate concentration in turnip (Brassica rapa ssp. rapifera L.) roots as affected by nitrogen and sulfur supply. J. Agric. Food Chem. 55, 8452–8457. doi: 10.1021/jf070816k
Malinovsky, F. G., Thomsen, M. F., Nintemann, S. J., Jagd, L. M., Bourgine, B., Burow, M., et al. (2017). An evolutionarily young defense metabolite influences the root growth of plants via the ancient TOR signaling pathway. Elife 6. doi: 10.7554/eLife.29353
Marino, D., Ariz, I., Lasa, B., Santamaría, E., Fernández-Irigoyen, J., González-Murua, C., et al. (2016). Quantitative proteomics reveals the importance of nitrogen source to control glucosinolate metabolism in Arabidopsis thaliana and Brassica oleracea. J. Exp. Bot. 67, 3313–3323. doi: 10.1093/jxb/erw147
Martin, T., Oswald, O., Graham, I. A. (2002). Arabidopsis seedling growth, storage lipid mobilization, and photosynthetic gene expression are regulated by carbon:nitrogen availability. Plant Physiol. 128, 472–481. doi: 10.1104/pp.010475
Matile, P. H. (1980). The mustard oil bomb-compartmentation of the myrosinase system. Biochemie und Physiol. der. Pflanzen. 175 (8–9), 722–731. doi: 10.1016/S0015-3796(80)80059-X
Mewis, I., Appel, H. M., Hom, A., Raina, R., Schultz, J. C. (2005). Major signaling pathways modulate Arabidopsis glucosinolate accumulation and response to both phloem-feeding and chewing insects. Plant Physiol. 138, 1149–1162. doi: 10.1104/pp.104.053389
Mewis, I., Khan, M. A. M., Glawischnig, E., Schreiner, M., Ulrichs, C. (2012). Water stress and aphid feeding differentially influence metabolite composition in Arabidopsis thaliana (L.). PloS One 7, e48661. doi: 10.1371/journal.pone.0048661
Moore, B. D., Andrew, R. L., Külheim, C., Foley, W. J. (2014). Explaining intraspecific diversity in plant secondary metabolites in an ecological context. New Phytol. 201, 733–750. doi: 10.1111/nph.12526
Nakano, R. T., Piślewska-Bednarek, M., Yamada, K., Edger, P. P., Miyahara, M., Kondo, M., et al. (2017). PYK10 myrosinase reveals a functional coordination between endoplasmic reticulum bodies and glucosinolates in Arabidopsis thaliana. Plant J. 89, 204–220. doi: 10.1111/tpj.13377
Nakazaki, A., Yamada, K., Kunieda, T., Sugiyama, R., Hirai, M. Y., Tamura, K., et al. (2019). Leaf endoplasmic reticulum bodies identified in Arabidopsis rosette leaves are involved in defense against herbivory. Plant Physiol. 179, 1515–1524. doi: 10.1104/pp.18.00984
Omirou, M. D., Papadopoulou, K. K., Papastylianou, I., Constantinou, M., Karpouzas, D. G., Asimakopoulos, I., et al. (2009). Impact of nitrogen and sulfur fertilization on the composition of glucosinolates in relation to sulfur assimilation in different plant organs of broccoli. J. Agric. Food Chem. 57, 9408–9417. doi: 10.1021/jf901440n
Pastorczyk, M., Bednarek, P. (2016). Chapter seven - the function of glucosinolates and related metabolites in plant innate immunity. Adv. In Botanical Res. 80, 171–198. doi: 10.1016/bs.abr.2016.06.007
Petersen, B. L., Chen, S., Hansen, C. H., Olsen, C. E., Halkier, B. A. (2002). Composition and content of glucosinolates in developing Arabidopsis thaliana. Planta 214, 562–571. doi: 10.1007/s004250100659
Pičmanová, M., Neilson, E. H., Motawia, M. S., Olsen, C. E., Agerbirk, N., Gray, C. J., et al. (2015). A recycling pathway for cyanogenic glycosides evidenced by the comparative metabolic profiling in three cyanogenic plant species. Biochem. J. 469, 375–389. doi: 10.1042/BJ20150390
Piślewska-Bednarek, M., Nakano, R. T., Hiruma, K., Pastorczyk, M., Sanchez-Vallet, A., Singkaravanit-Ogawa, S., et al. (2018). Glutathione transferase U13 functions in pathogen-triggered glucosinolate metabolism. Plant Physiol. 176, 538–551. doi: 10.1104/pp.17.01455
Piotrowski, M. (2008). Primary or secondary? Versatile nitrilases in plant metabolism. Phytochemistry 69, 2655–2667. doi: 10.1016/j.phytochem.2008.08.020
R Core Team (2017). R: A language and environment for statistical computing. R Foundation for Statistical Computing, Vienna, Austria. https://www.R-project.org/
Schonhof, I., Blankenburg, D., Müller, S., Krumbein, A. (2007). Sulfur and nitrogen supply influence growth, product appearance, and glucosinolate concentration of broccoli. Z. Pflanzenernähr. Bodenk. 170, 65–72. doi: 10.1002/jpln.200620639
Textor, S., Gershenzon, J. (2009). Herbivore induction of the glucosinolate–myrosinase defense system: major trends, biochemical bases and ecological significance. Phytochem. Rev. 8, 149–170. doi: 10.1007/s11101-008-9117-1
Traka, M. H. (2016). Chapter Nine - Health Benefits of Glucosinolates. Adv. In Botanical Res. 80, 247–279. doi: 10.1016/bs.abr.2016.06.004
Urbancsok, J., Bones, A. M., Kissen, R. (2017). Glucosinolate-derived isothiocyanates inhibit Arabidopsis growth and the potency depends on their side chain structure. Int. J. Mol. Sci. 18 (11), 2372. doi: 10.3390/ijms18112372
Urbancsok, J., Bones, A. M., Kissen, R. (2018). Benzyl Cyanide Leads to auxin-like effects through the action of nitrilases in Arabidopsis thaliana. Front. Plant Sci. 9, 1240. doi: 10.3389/fpls.2018.01240
Vik, D., Mitarai, N., Wulff, N., Halkier, B. A., Burow, M. (2018). Dynamic modeling of indole glucosinolate hydrolysis and its impact on auxin signaling. Front. Plant Sci. 9, 550. doi: 10.3389/fpls.2018.00550
Wentzell, A. M., Rowe, H. C., Hansen, B. G., Ticconi, C., Halkier, B. A., Kliebenstein, D. J. (2007). Linking metabolic QTLs with network and cis-eQTLs controlling biosynthetic pathways. PLoS Genet. 3, 1687–1701. doi: 10.1371/journal.pgen.0030162
Withers, P. J. A., O’Donnell, F. M. (1994). The response of double-low winter oilseed rape to fertiliser sulphur. J. Sci. Food Agric. 66, 93–101. doi: 10.1002/jsfa.2740660114
Wittstock, U., Burow, M. (2007). Tipping the scales–specifier proteins in glucosinolate hydrolysis. IUBMB Life 59, 744–751. doi: 10.1080/15216540701736277
Wittstock, U., Burow, M. (2010). Glucosinolate breakdown in Arabidopsis: mechanism, regulation and biological significance. Arabidopsis Book 8, e0134. doi: 10.1199/tab.0134
Wittstock, U., Kurzbach, E., Herfurth, A. M., Stauber, E. J. (2016a). Chapter six - glucosinolate breakdown. Adv. In Botanical Res. 80, 125–169. doi: 10.1016/bs.abr.2016.06.006
Wittstock, U., Meier, K., Dörr, F., Ravindran, B. M. (2016b). NSP-dependent simple nitrile formation dominates upon breakdown of major aliphatic glucosinolates in roots, seeds, and seedlings of Arabidopsis thaliana Columbia-0. Front. Plant Sci. 7, 1821. doi: 10.3389/fpls.2016.01821
Xu, Z., Escamilla-Treviño, L., Zeng, L., Lalgondar, M., Bevan, D., Winkel, B., et al. (2004). Functional genomic analysis of Arabidopsis thaliana glycoside hydrolase family 1. Plant Mol. Biol. 55, 343–367. doi: 10.1007/s11103-004-0790-1
Züst, T., Agrawal, A. A. (2017). Trade-offs between plant growth and defense against insect herbivory: an emerging mechanistic synthesis. Annu. Rev. Plant Biol. 68, 513–534. doi: 10.1146/annurev-arplant-042916-040856
Züst, T., Heichinger, C., Grossniklaus, U., Harrington, R., Kliebenstein, D. J., Turnbull, L. A. (2012). Natural enemies drive geographic variation in plant defenses. Science 338, 116–119. doi: 10.1126/science.1226397
Zhang, J., Sun, X., Zhang, Z., Ni, Y., Zhang, Q., Liang, X., et al. (2011). Metabolite profiling of Arabidopsis seedlings in response to exogenous sinalbin and sulfur deficiency. Phytochemistry 72, 1767–1778. doi: 10.1016/j.phytochem.2011.06.002
Keywords: glucosinolate metabolism, nutrient conditions, seedling development, nitrogen limitation, sulphur limitation, metabolic regulation
Citation: Jeschke V, Weber K, Moore SS and Burow M (2019) Coordination of Glucosinolate Biosynthesis and Turnover Under Different Nutrient Conditions. Front. Plant Sci. 10:1560. doi: 10.3389/fpls.2019.01560
Received: 29 July 2019; Accepted: 07 November 2019;
Published: 06 December 2019.
Edited by:
Tamara Gigolashvili, University of Cologne, GermanyReviewed by:
Kei Hiruma, Nara Institute of Science and Technology (NAIST), JapanPaweł Bednarek, Institute of Bioorganic Chemistry (PAS), Poland
Ute Wittstock, Technische Universitat Braunschweig, Germany
Copyright © 2019 Jeschke, Weber, Moore and Burow. This is an open-access article distributed under the terms of the Creative Commons Attribution License (CC BY). The use, distribution or reproduction in other forums is permitted, provided the original author(s) and the copyright owner(s) are credited and that the original publication in this journal is cited, in accordance with accepted academic practice. No use, distribution or reproduction is permitted which does not comply with these terms.
*Correspondence: Meike Burow, bWJ1QHBsZW4ua3UuZGs=