- 1Department of Biotechnology, Faculty of Bioscience Engineering, Ghent University, Ghent, Belgium
- 2Laboratory of Phytopathology, Department of Plants and Crops, Faculty of Bioscience Engineering, Ghent University, Ghent, Belgium
- 3Plant Genome Research Unit, Agrogenomics Research Center, National Institute of Agrobiological Sciences, Tsukuba, Japan
- 4Research Group EnVOC, Department of Sustainable Organic Chemistry and Technology, Ghent University, Ghent, Belgium
Next to their essential roles in plant growth and development, phytohormones play a central role in plant immunity against pathogens. In this study we studied the previously reported antagonism between the plant-pathogenic oomycete Pythium arrhenomanes and the root-knot nematode Meloidogyne graminicola, two root pathogens that co-occur in aerobic rice fields. In this manuscript, we investigated if the antagonism is related to imbalances in plant hormone levels, which could be involved in activation of plant defense. Hormone measurements and gene expression analyses showed that the jasmonate (JA) pathway is induced early upon P. arrhenomanes infection. Exogenous application of methyl-jasmonate (MeJA) on the plant confirmed that JA is needed for basal defense against both P. arrhenomanes and M. graminicola in rice. Whereas M. graminicola suppresses root JA levels to increase host susceptibility, Pythium inoculation boosts JA in a manner that prohibits JA repression by the nematode in double-inoculated plants. Exogenous MeJA supply phenocopied the defense-inducing capacity of Pythium against the root-knot nematode, whereas the antagonism was weakened in JA-insensitive mutants. Transcriptome analysis confirmed upregulation of JA biosynthesis and signaling genes upon P. arrhenomanes infection, and additionally revealed induction of genes involved in biosynthesis of diterpenoid phytoalexins, consistent with strong activation of the gene encoding the JA-inducible transcriptional regulator DITERPENOID PHYTOALEXIN FACTOR. Altogether, the here-reported data indicate an important role for JA-induced defense mechanisms in this antagonistic interaction. Next to that, our results provide evidence for induced expression of genes encoding ERF83, and related PR proteins, as well as auxin depletion in P. arrhenomanes infected rice roots, which potentially further contribute to the reduced nematode susceptibility seen in double-infected plants.
Introduction
Rice (Oryza sativa) is an important staple crop for a large part of the world population. Moreover, researchers use this plant as model organism for monocots. Sessile organisms, such as rice and other plants, have evolved sophisticated strategies to fend off pathogens. Phytohormones play a central role in the orchestration of plant immunity against pathogens and jasmonic acid (JA), salicylic acid (SA), and ethylene (ET) are considered the backbone of this network. In addition, abscisic acid (ABA), auxins (AUX), gibberellins, cytokinins, and brassinosteroids have also emerged as players in plant defense (Pieterse et al., 2012; De Vleesschauwer et al., 2013).
Jasmonates are lipid-derived signal molecules that play vital roles in diverse physiological processes, but have mostly been studied for their involvement in wounding responses, secondary metabolite biosynthesis, and defense. JA is synthesized through the oxylipin biosynthesis pathway starting from α-linolenic acid from membrane lipids. JA can further be metabolized to the volatile methyl-jasmonate (MeJA), or conjugated with amino acids such as Ile to the biologically active JA-Ile compound through the enzyme JA-Ile synthase (JAR1) (Pieterse et al., 2012; Lyons et al., 2013). JA-Ile plays a vital role in jasmonate signaling by activating genes that are suppressed by transcriptional repressor proteins with a Jasmonate ZIM (JAZ) domain. In the absence of JA, JAZ proteins bind to transcription factors (TFs) of JA-responsive genes and prevent their expression. The F-box protein Coronatine-Insensitive1 (COI1), a key regulator in the activation of JA-responsive genes, functions as the receptor of JA-Ile as part of the E3 ubiquitin-ligase SKP1-Cullin-F-box SCFCOI1complex. Upon binding to JA-Ile, this complex directs ubiquitination and subsequent degradation of JAZ proteins via the proteasome (Pauwels and Goossens, 2011; Pieterse et al., 2012), leading to activation of JA-response genes. In Arabidopsis, the JA-responsive genes can be divided in two branches, where the MYC genes are associated with wound response and defense against insect herbivores, while other genes known as APETALA2/Ethylene Response Factor (AP2/ERF) genes are controlled by both JA and ET and are associated with enhanced resistance to necrotrophic pathogens (Zhu et al., 2011).
Although very relevant for agronomy, pathogenic interactions between plants and multiple damage-causing organisms are largely understudied, certainly when considering the role of plant hormones and plant defense. Two such soil-borne pathogenic organisms that co-occur in aerobic rice fields are Pythium arrhenomanes and Meloidogyne graminicola (Kreye et al., 2009a; Verbeek et al., 2016). The soil-borne oomycete P. arrhenomanes infects several monocots such as rice (Kreye et al., 2009a), sugarcane (Bond et al., 2004), and wheat (Mojdehi et al., 1991). In a field study, P. arrhenomanes was found to be the most virulent species isolated from aerobic rice fields (Van Buyten et al., 2013). P. arrhenomanes can cause seed mortality, damping off, and stunting of young seedlings (Kreye et al., 2009b). The role of phytohormones in plant defense against P. arrhenomanes is poorly understood. However, in the Pythium graminicola-rice interaction, the oomycete has been reported to hijack the brassinosteroid pathway to antagonize gibberellin and SA-dependent defense pathways (De Vleesschauwer et al., 2012). ET signaling plays a positive role in pea immunity against Pythium irregulare (Blake et al., 2015), since ET insensitive ein2-mutant pea plants were shown to be highly susceptible. In the moss Physcomitrella patens, infection by P. irregulare and Pythium debaryanum activates expression of the defense genes chalcone synthase, lipoxygenase, and phenylalanine ammonia lysase, as well as accumulation of reactive oxygen species, JA, and its precursor 12-oxo-phytodienoic acid (OPDA) (Oliver et al., 2009). In a similar manner, Pythium ultimum induces expression of ET and JA biosynthesis and response genes in apple (Shin et al., 2014). In Arabidopsis, Staswick et al. (1998) showed that JA signaling is needed for defense against P. irregulare.
M. graminicola is one of the most damaging pathogens in aerobic rice (Mantelin et al., 2017). This root-knot nematode induces the formation of giant cells inside root vascular tissue of its host plant, leading to the visual symptoms of root galling and aboveground chlorosis (Mantelin et al., 2017). The M. graminicola-rice interaction has been studied thoroughly on a molecular level, revealing a major transcriptional repression of host defense pathways, in contrast to activation of cytokinesis and primary metabolic pathways (Kyndt et al., 2012; Ji et al., 2013). JA plays a pivotal role in the basal defense against M. graminicola in rice, while exogenous supply of benzothiadiazole (BTH)—a SA-analogue—only has minor defense-inducing capacity (Nahar et al., 2011). Exogenous application of ethephon (an ET-releaser) results in enhanced defense against this nematode, but works in a JA-dependent manner. Hormones which are traditionally known to be important for plant growth and development or abiotic stress responses rather promote host susceptibility to this nematode. For example, recent data show an accumulation of ABA in galls at 3 and 7 days post M. graminicola inoculation in rice, and exogenous application of ABA overrules JA-induced defense (Kyndt et al., 2017). In a similar manner, activation of gibberellins, which accumulate in nematode feeding sites, promotes rice root susceptibility while it suppresses the JA pathway (Yimer et al., 2018). AUX accumulation is also of crucial importance for M. graminicola-induced feeding site formation in rice (Yimer et al., 2018), as well as in dicotyledonous host plants (Kyndt et al., 2016).
Previously we showed an antagonistic interaction between the plant-pathogenic oomycete P. arrhenomanes and M. graminicola, where infection with the oomycete negatively affects the number of established nematodes and hampers nematode development in rice roots (Verbeek et al., 2016). We hypothesized that this antagonism could be related to changes in phytohormones and related induced defense responses in the rice roots. The goals of the current study were (i) to investigate how phytohormones SA, JA, AUX, and ABA are affected by single and double inoculation in rice roots, (ii) to investigate which phytohormones play a key role in this antagonistic interaction between both pathogens in rice roots, and (iii) to provide more insights into the transcriptional response of rice roots upon Pythium infection.
Materials and Methods
Rice Varieties
Rice (O. sativa) wild-type varieties used in this study include cultivar “Nipponbare” (provided by the U.S. Department of Agriculture; GSOR-100) and breeding line IR81413-BB-75-4 (provided by the Plant Breeding, Genetics and Biotechnology Division of International Rice Research Institute (IRRI). Transgenic and mutant lines include Tos17-insertion mutant Osjar1 [NC0364; http://tos.nias.affrc.go.jp/; (Miyao et al., 2003)] and transgenic OsCOI1-18 RNAi-line (Yang et al., 2012), which both have Nipponbare as background. Seeds were stored at 4°C. Seeds were dehusked, surface sterilized with 4% hypochlorite for 15 min, and subsequently washed three times with sterile water before germination.
Preparation of Pythium Inoculum
P. arrhenomanes (isolate PT60), isolated from an aerobic rice field in Tarlac, Philippines (Van Buyten et al., 2013), was maintained in water agar plugs submerged in sterile distilled water and kept at 15°C. Working cultures were revived on potato dextrose agar (PDA) and incubated at 28°C. Final inocula for the greenhouse experiment were prepared by inoculating one-fourth of a 3-day-old PDA plate into a glass jar containing 150 g sterile rice grain:rice hull (RG : RH, 1:3) substrate for 7 days.
Maintenance of M. graminicola
M. graminicola was originally isolated in the Philippines (Batangas) and was kindly provided by Dirk DeWaele (Catholic University, Leuven, Belgium). Nematode cultures were maintained in vivo on wild-type Nipponbare plants and grasses (Echinocloa crus-galli) as described by Nahar et al. (2011). Collection of nematodes was performed on three-months old plants with the Baermann funnel method described by Kyndt et al. (2017). Inoculation conditions are described below per experiment. The nematode infection level was assessed by counting the number of galls and nematodes after visualizing them by boiling whole roots for 3 min in 0.8% acetic acid and 0.013% acid fuchsin. Afterward, the roots were washed with running tap water and slowly destained in acidified glycerol. The developmental stage of each nematode was scored to assess the nematode development and egg masses were counted as an estimate of reproduction.
In Vitro P. Arrhenomanes Infection
Six sterilized rice seeds were placed 2 cm apart on square Petri dishes (120 × 120 mm) filled with 50 ml of Gamborg B5 basal medium with 1% plant agar. Plants were grown at 26°C under a 12-h/12-h light regime. For some experiments, Gamborg B5 basal medium was amended with 25 µM MeJA or 10 µM 5,8,11,14-eicosatetraynoic acid (ETYA). Three days post imbibition, when the primary roots were approximately 1 cm in length, the seeds were infected with P. arrhenomanes (PT60).Pythium plugs were pressed out with a cork screw (4#) from a PDA culture (henceforth named PaPDA). Plugs were placed between seedlings 1 and 2, seedlings 3 and 4, and seedlings 5 and 6. At 7 days post inoculation, the plants were measured and the plant health was assessed according to two scoring systems; (i) a shoot disease index (Van Buyten and Höfte, 2013): healthy green shoot (0); yellow spots (1); brown spots (2); dead (3), and (ii) a root disease index (De Vleesschauwer et al., 2012):
a. root length >60% of mock treatment, necrosis covering <20% of total root area,
b. root length >60% of mock treatment, necrosis covering >20% of total root area,
c. root length 20% to 60% of the respective mock treatment,
d. root length <20% of mock treatment, necrosis covering <75% of total root area,
e. root length <20% of mock treatment, necrosis covering >75% of total root area.
Disease index values were calculated according to the following formula:
Coinoculation Experiments
Greenhouse Experiment
Root samples of variety IR81413-BB-75-4 were collected during the greenhouse experiment performed at the IRRI in Los Baños, Philippines (Verbeek et al., 2016). Detailed experimental conditions were described in Verbeek et al. (2016). Sterilized seeds were germinated in tap water, which was refreshed daily for one week. Seven-day-old seedlings were transplanted in four equally spaced points per pot. One day prior to transplanting, 150 g of rice grain:rice hull (RG : RH) with P. arrhenomanes was incorporated in the soil (1:40 ratio) for the P. arrhenomanes inoculated pots. The same amount of sterile un-inoculated RG : RH was added to the other pots. Nematode inoculation was performed by adding 750 second-stage juveniles (J2) on each side of the seedlings at 1 day post transplanting (dpt), totaling 6,000 J2s per pot. The same inoculation procedures were followed for the P. arrhenomanes-M. graminicola combination treatments, using four different treatment groups with 18 plants: (i) un-inoculated control, (ii) P. arrhenomanes single inoculation + nematode mock inoculation, (iii) M. graminicola single inoculation + oomycete mock inoculation, and (iv) P. arrhenomanes + M. graminicola double inoculation. At dedicated time points, root samples were taken, flash frozen in liquid nitrogen and lyophilized for transport to Belgium for further analysis.
Plant Growth Room Experiments in SAP Substrate
Sterilized rice seeds were germinated for 3 days on wet filter paper at 30°C, and subsequently transferred to Sand and Absorbent Polymer (SAP) substrate placed in specially made polyvinyl-chloride (PVC) tubes (Reversat et al., 1999). Plants were grown at 26°C under a 12-h/12-h light regime. Five day old seedlings were inoculated with two PaPDA plugs by pressing the plugs in the SAP in close proximity of the root. Subsequently the SAP was drenched with 10 ml distilled water to cover the PaPDA plugs. Mock inoculated plants were treated with two empty PDA plugs. Four days later, 250 second-stage juveniles of M. graminicola were inoculated per plant (visualized in Figure S1). Two treatment groups, each consisting of eight individual plants, were used in these experiments: (i) M. graminicola single inoculation + oomycete mock inoculation, and (ii) P. arrhenomanes + M. graminicola double inoculation. At 12 days post nematode inoculation, nematode infection was evaluated by staining the root systems with acid fuchsin to visualize galls and nematodes.
For MeJA treatment, seedlings were sprayed 24 h prior to nematode inoculation with 100 µM MeJA (Sigma) or water. MeJA was first dissolved in 1 ml of methanol and subsequently diluted in 50 ml distilled water containing 0.02% (v/v) Tween 20. The solution was sprayed with a vaporizer on the shoots until run off. Distilled water containing 0.02% (v/v) Tween 20 was used as a control treatment. All experiments were independently repeated twice, with similar results, with in each case at least six individual plants per treatment.
SA, JA, IAA, and ABA Measurement
A cold extraction of 100 mg of homogenized rice material (root or shoot) was performed using the modified Bieleski solvent, followed by filtration and clean-up. Chromatographic separation of JA, SA, indole-3-acetic-acid (IAA), and ABA was achieved on an ultra-high performance liquid chromatography system coupled to a Q Exactive™ quadrupole Orbitrap mass spectrometer (Thermo Fisher Scientific). A Nucleodur C18 column (50 × 2 mm; 1.8 µm dp) was used, with a mobile phase gradient consisting of acidified methanol and water. Mass spectrometric analysis was carried out in both positive and negative electrospray ionization and in selected-ion monitoring mode, at a mass resolution of 70,000 full width at half maximum. For more details about this method, see Haeck et al. (2018).
Data Analysis
SPSS (version 23, IBM, USA) was used for all statistical analyses. The normality of the data was checked using the Kolmogorov-Smirnov test of composite normality (α = 0.05). None of the data were normally distributed; therefore, non-parametric Mann-Whitney U test (P ≤ 0.05) was performed. Specific details on the statistical analyses are given per experiment in the respective legends.
Transcriptome Analysis
Seeds were grown in Gamborg B5 medium as described above, using 0.6-cm PDA plugs of a 7-day-old culture of P. arrhenomanes (PT60). Samples from mock-inoculated and Pythium-infected seedlings were collected 2 days post inoculation (dpi). Three biological replicates were sampled, where each biological replicate represents a pool from at least 20 individual plants, giving a total of six RNA samples (two treatments × three biological replicates). Total RNA was extracted from frozen root tissue using the spectrum plant total RNA kit (Sigma-Aldrich) and subsequently Turbo DNase treated according to the provided protocol (Ambion). For transcriptome analysis, a two-dye method was used described by (Satoh et al., 2010), which will allow direct comparison between two samples and decreases technical bias. A total of 850 ng of RNA was synthesized to cyanine 3- or cyanine 5-labeled cDNA using a low-input RNA labeling kit (Agilent Technologies) and hybridized to custom-made 60-mer Agilent arrays according to the manufacturer’s protocols (Agilent Technologies). Slide image files were generated using a DNA microarray scanner (G2505B; Agilent Technologies) and signal intensities were extracted and normalized within each array using Feature Extraction version 9.5 (Agilent Technologies). Gene intensities of <100 in replicates were neglected, for these are not significant. Signal intensities among biological replicates per infection were normalized according to the quantile method for standardization (global scaling) using EXPANDER6. Significance analysis was performed using the LIMMA function in Multiple experiment Viewer. For each infection experiment the fold change (FC) of each gene was determined by dividing its expression level in the inoculated plants by its expression level in mock-inoculated plants and taking its Log base 2. Differentially expressed genes (DEGs) were defined as genes with a false discovery rate (FDR) of <0.05 and a cut-off value of Log2FC <−1.5 or Log2FC >1.5. Loci above the FDR threshold or below the Log2FC cut-off were considered as not differentially expressed. The data have been deposited in NCBI’s Gene Expression Omnibus and are accessible through GEO Series accession number GSE133268. Gene Ontology (GO) enrichment analysis was performed using the Parametric Analysis of Gene Set Enrichment (PAGE) of agriGO (Kim and Volsky, 2005; Du et al., 2010). MapMan (Thimm et al., 2004) was used to visualize the expression of biotic stress related genes and genes involved in hormonal pathways in rice.
RNA Extraction, cDNA Synthesis, and qRT-PCR
RNA extraction was performed with the RNeasy Plant Mini kit (Qiagen Hilden, Germany), followed by DNase treatment (DNaseI, Thermo Scientific) and cDNA synthesis using the Tetro cDNA Synthesis Kit (Bioline), as described by Kyndt et al. (2017). Two internal reference genes OsEXP and OsEXPnarsai were used to normalize the expression levels of rice genes (Nahar et al., 2012).
Results
Preliminary Hormone Measurements Show Minor JA Accumulation Upon P. Arrhenomanes Infection in Rice Roots
Previously, we have reported that prior infection by the oomycete P. arrhenomanes consistently reduces the penetration rate and development of the root-knot nematode M. graminicola in rice roots, even under different infection and plant growth conditions (Verbeek et al., 2016). To elucidate a potential mechanism behind this antagonism, we decided to investigate the levels of a set of plant hormones, namely JA, SA, IAA—an important AUX—and ABA, upon single and double inoculations, using root samples taken at two time points (11 and 13 days post germination, dpg) during the greenhouse experiment described in detail in Verbeek et al. (2016). Results, shown in Figure 1, revealed JA levels in the roots are significantly reduced at 3 days post single nematode inoculation (11 dpg) compared to the un-inoculated control (Figure 1A). Four days after single P. arrhenomanes inoculation, a minor JA increase was observed. However, in double inoculated roots, JA levels did not differ from the un-inoculated control. At 13 dpg, JA levels were significantly lower in all single or double inoculated plants compared to the control (Figure 1B). The level of SA was significantly higher at 11 dpg in single nematode and double inoculated plants, compared to control and single P. arrhenomanes inoculated roots. Although not statistically significant, this trend was still visible at 13 dpg. ABA levels were very low in the rice roots, and only upon single nematode inoculation a significantly increased ABA level was observed at 13 dpg, confirming observations of Kyndt et al. (2017). Although fairly constant levels of IAA were detected at 11 dpg, at 13 dpg the IAA level dropped significantly in single P. arrhenomanes inoculated as well as in double-inoculated plants (Figure 1B).
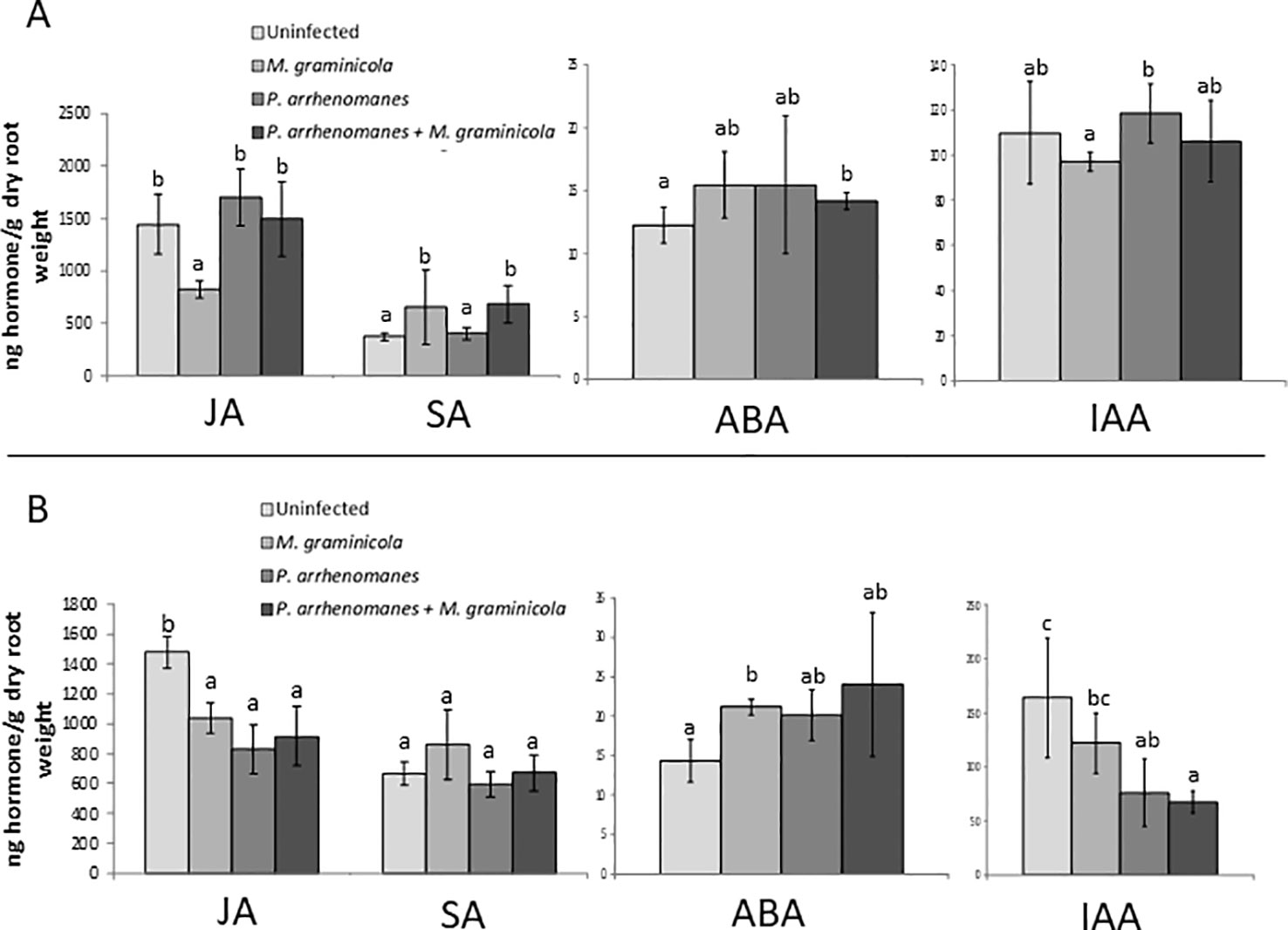
Figure 1 Hormone levels of jasmonic acid (JA), salicylic acid (SA), abscisic acid (ABA), and indole-3-acetic acid (IAA) in the roots of rice variety IR81413-BB-75-4 at 11 days (A) and 13 days (B) post germination (dpg). (A) corresponds with 4 days post oomycete inoculation (dpoi) and 3 days post nematode inoculation (dpni), (B) corresponds with 6 days post oomycete inoculation (dpoi) and 5 days post nematode inoculation (dpni). Treatments are: uninfected roots, single M. graminicola inoculation; single P. arrhenomanes inoculation; and P. arrhenomanes prior to M. graminicola inoculation. Bars represent mean ± standard deviation (SD) of three biological replicates, each consisting of a pool of six root systems. Different letters indicate statistical significant (α = 0.05) differences for that hormone at that time point.
Based on these data and the well-known importance of JA for basal defense against this nematode (Nahar et al., 2011), we hypothesized that an early JA accumulation—even earlier than the 4 days post oomycete inoculation time point analyzed in this greenhouse experiment—might be responsible for reduced nematode infection upon P. arrhenomanes infection in rice roots. This hypothesis was strengthened by the fact that the nematode is no longer able to suppress JA levels in double-inoculated plants (Figure 1A).
The JA Pathway Is Activated Early Upon P. Arrhenomanes Inoculation in Rice, as Part of a Plant Defense Response
Based on the above-described observations we hypothesized that JA might be accumulating very early upon P. arrhenomanes inoculation. To test this hypothesis, hormone measurements were done under controlled in vitro conditions [as described in De Vleesschauwer et al. (2012)], as these are more adequate for standardized infection pressure, at both 2 and 4 dpi.
Probably due to the young tissue, only SA and JA levels were above the detection limit in this experiment. The data confirmed a strong accumulation of JA at 2 dpi with P. arrhenomanes and a lower but still significant accumulation at 4 dpi, whereas SA levels were unaffected by P. arrhenomanes infection in rice roots (Figure 2), as also observed in the greenhouse experiment (Figure 1).
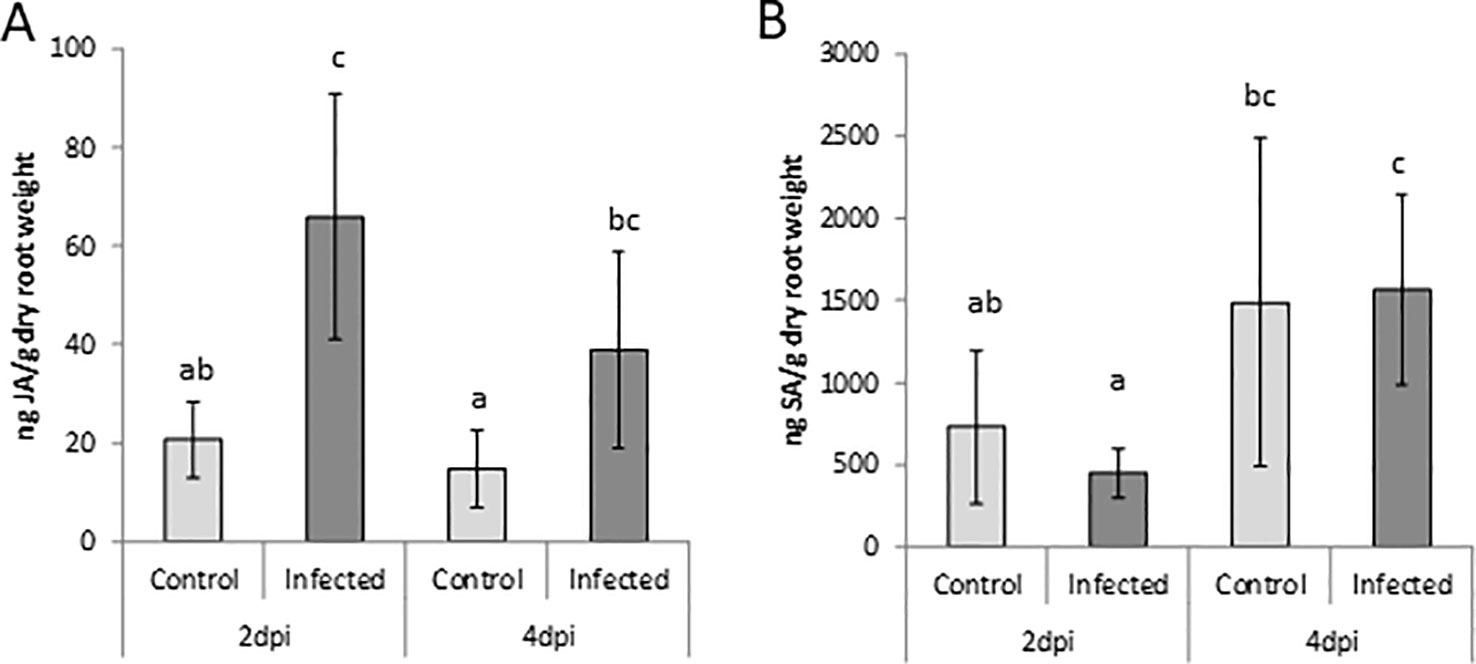
Figure 2 Jasmonic acid (JA; A) and salicylic acid (SA; B) levels in roots of Nipponbare plants grown on Gamborg B5 medium at 2 and 4 days post P. arrhenomanes inoculation compared to uninfected roots. Statistics were performed using the Mann-Whitney test (α = 0.05). Bars represent mean ± SD of five biological replicates, each consisting of a pool of 12 root systems. Different letters represent significant differences.
Next, we investigated the role of JA in basal plant defense against P. arrhenomanes by amending the growth medium with MeJA or ETYA (jasmonate biosynthesis inhibitor). In addition, the susceptibility of the JA-insensitive Osjar1 mutant line and the OsCOI1 RNAi-line was tested. Confirming the well-known inhibitory effect of JA on root growth, supply of MeJA significantly shortened rice root length with 47% (Figure 3A). Amending the plant growth medium with 25 µM MeJA significantly reduced the shoot and root disease index of the P. arrhenomanes inoculated plants. However, supply of ETYA did not significantly affect Pythium-induced disease symptoms in rice seedlings. The Osjar1-mutant, which contains lower levels of JA-Ile and is less sensitive to JA (Riemann et al., 2008; Fukumoto et al., 2013), was slightly more susceptible to P. arrhenomanes than wild-type plants (Figure 4). Similarly, the JA-insensitive OsCOI1 RNAi-line (Yang et al., 2012), was significantly more susceptible to P. arrhenomanes than the control line (Figure 4).
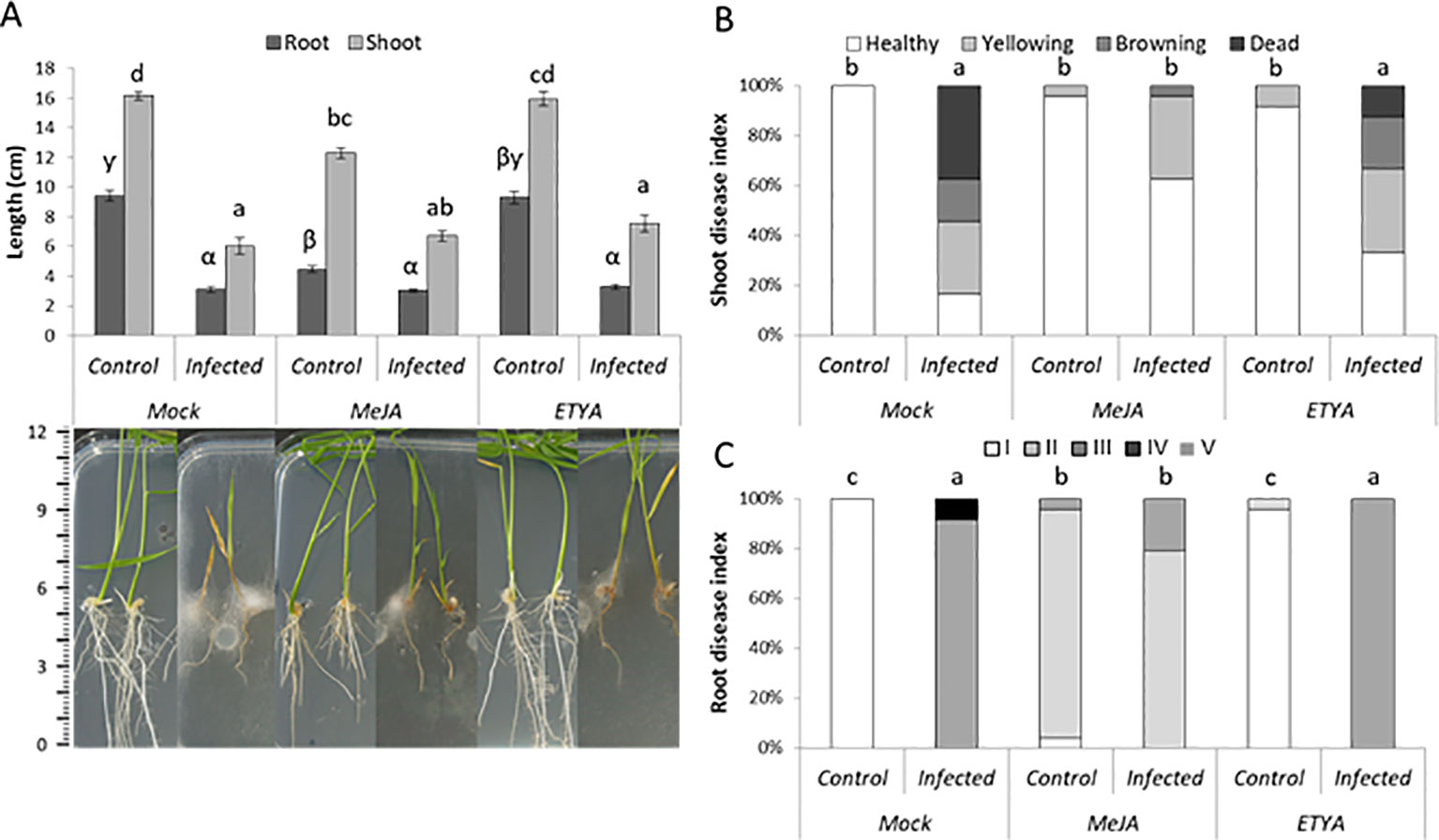
Figure 3 Effect of exogenous application of 10µM JA biosynthesis inhibitor ETYA and 25µM MeJA on P. arrhenomanesinduced disease symptoms at 10 dpi, compared to non-treated rice seedlings. (A) root and shoot length with representative pictures of plants, (B) shoot disease index, and (C) root disease index. Root disease index: (I) root length (RL) > 60% of the respective mock treatment, necrosis covering <20% of total root area; (II) RL >60%, necrosis >20%; (III) RL 20% to 60%; (IV) RL <20%, necrosis <75%, and (V) RL <20%, necrosis >75%. Error bars represent the standard error (SE). An indicative scale bar (in cm) is shown on the left. Different letters represent statistically significant differences among the treatments at α = 0.05, P ≤ α performed by Mann–Whitney non-parametric tests. In (A), statistical differences for shoot data are shown in Roman letters, while root differences are shown in Greek letters.
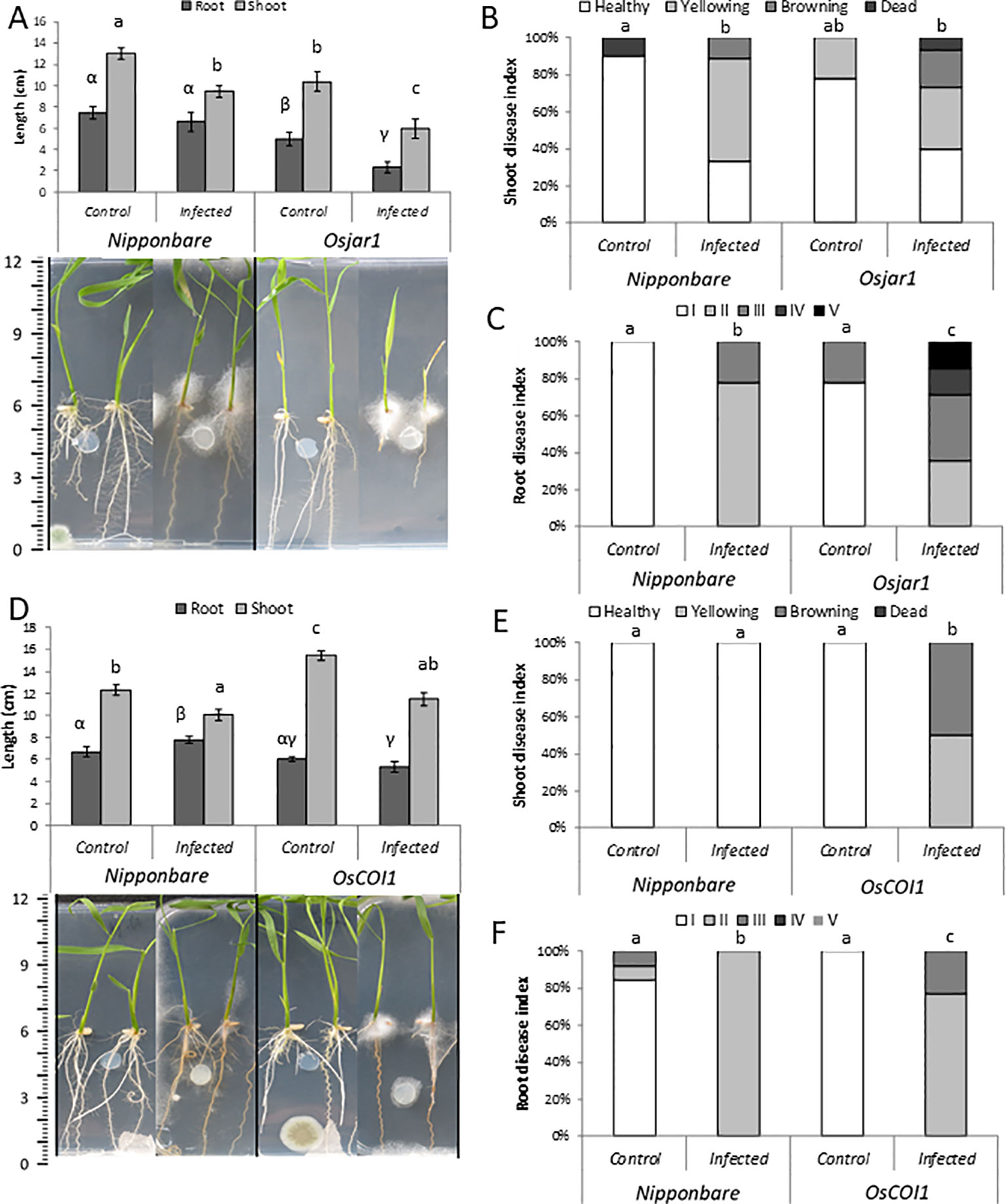
Figure 4 Infection of P. arrhenomanes on Nipponbare and Osjar1 mutant (A–C), and on the OsCOI1 RNAi-line (D–F) at 7 days post P. arrhenomanes inoculation (n = 18). (A, D) root and shoot length with representative pictures of plants; (B, E) shoot disease index; (C, F) root disease index. Root disease index: (I); root length (RL) > 60% of the respective mock treatment, necrosis covering <20% of total root area, (II); RL >60%, necrosis >20%, (III); RL 20% to 60%, (IV); RL <20%, necrosis <75%, and (V); RL <20%, necrosis >75%. Different letters represent statistically significant differences among the treatments at α = 0.05, P≤ α performed by Mann-Whitney non-parametric tests. Error bars represent the SE. An indicative scale bar (in cm) is shown on the left. In (A), statistical differences for shoot data are shown in Roman letters, while root differences are shown in Greek letters.
These data demonstrate that the JA pathway is induced upon P. arrhenomanes infection in rice roots as part of a plant defense response and lead to the hypothesis that JA induction would be the causal mechanism behind the antagonistic interaction with M. graminicola, reported in Verbeek et al. (2016).
The Importance of the JA Pathway in the Antagonism Between P. arrhenomanes and M. graminicola in Rice Roots
A set of double inoculation experiments was done with both P. arrhenomanes and M. graminicola. In these experiments, the role of JA in the antagonistic interaction between P. arrhenomanes and M. graminicola was evaluated by working with the previously described JA-mutants or exogenous supply of MeJA. An inoculation substrate optimized for M. graminicola infection, designed by Reversat et al. (1999), was used (see Methods and Timeline in Figure S1). Confirming the importance of JA in rice defense against this nematode (Nahar et al., 2011), the Osjar1 mutant was more susceptible to M. graminicola (Figures 5D–I), whereas foliar application with MeJA significantly reduced the level of M. graminicola infection. However, the OsCOI1 RNAi line was only marginally more susceptible to nematodes than wild-type plants.
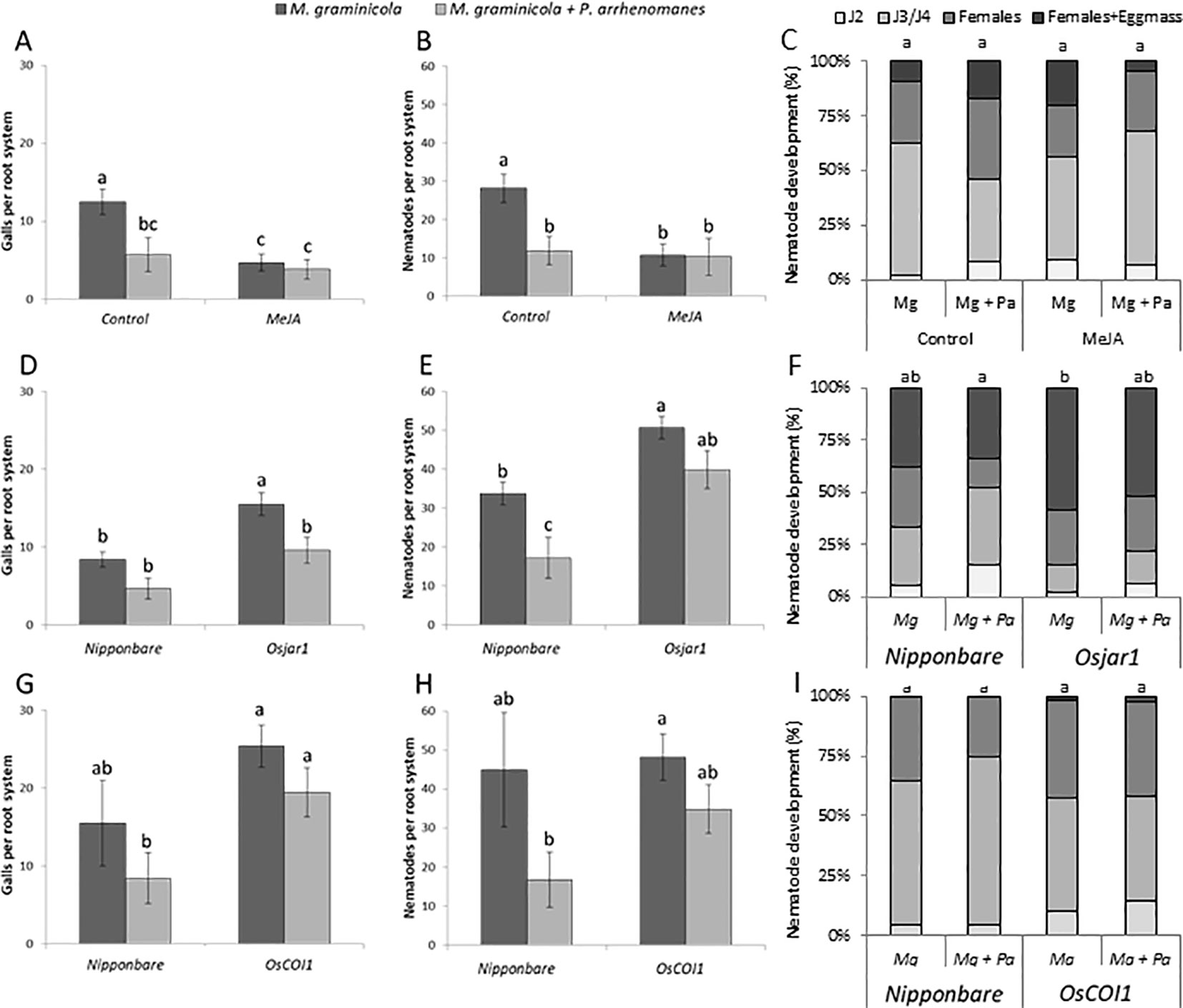
Figure 5 Gall formation (A,D,G), nematode establishment (B, E, H), and nematode development (C, F, I) in wild-type (Nipponbare); after treatment with MeJA (A–C; n = 8), in the JA biosynthesis mutant Osjar1 (NC2728; (D–F); n = 8), and in the JA signaling OsCOI1 RNAi-line (G–I; n = 8). Statistics were performed using the Mann-Whitney test (α = 0.05). Different letters represent significant differences. Error bars represent the SE. Statistics for (C, F, I) were performed by giving each group a total score; where the percentage of each stage has a value; juvenile stage 2 (J2) = 1; juvenile stages 3 and 4 (J3/J4) = 2; females = 3; and females with eggmass = 4.
Although not always statistically significant, a reduced number of galls and number of penetrating nematodes was seen in the double-inoculated plants in comparison with single-nematode inoculated plants. Contrary to our previous observations in greenhouse experiments (Verbeek et al., 2016), no significant inhibitory effect of Pythium infection on nematode development was observed in the here-used substrate (Figure 5).
The reduction in nematode penetration and gall formation, triggered by MeJA application, phenocopies the reduction caused by P. arrhenomanes infection (Figures 5A, B). Furthermore, P. arrhenomanes infection on MeJA treated plants does not further reduce gall formation by M. graminicola (Figures 5A, B). Noteworthy, MeJA treatment reduces root growth, but when M. graminicola infection was expressed as nematodes per cm root, the same trends were seen (Figure S2). When considering the number of galls, in the Osjar1 mutant and the OsCOI1 RNAi-line, the antagonism was less strong compared to wild-type plants (Figures 5D–I). Taken together, these data indicate that the antagonism between these two pathogens in rice roots is partially dependent on JA signaling.
To further explore which JA-dependent or JA-independent defense mechanisms could be responsible for the antagonism, micro-array analysis was used to investigate gene expression in rice roots at 2 days after P. arrhenomanes inoculation. The gene expression profile revealed that 5147 rice genes are DEGs in inoculated roots compared to the mock inoculated control (FDR <0.05). Genes belonging to GO-categories “signaling,” “response to stimulus,” and “catalytic activity” were most upregulated, while genes associated with “structural molecule activity,” “organelle,” “macromolecular complex,” and “cellular component organization” were most downregulated (Figure S3). Genes involved in AUX biosynthesis and signaling were generally downregulated upon Pythium infection in rice roots, a phenomenon which was already detected in the hormone measurements reported in Figure 1 (Table S1). Confirming our hypothesis of an early JA activation, transcription of JA biosynthesis and signaling genes—e.g. genes encoding JAZ—was strongly upregulated at 2 dpi with P. arrhenomanes (Table S2).
Among the strongest down-regulated “biotic stress-related” genes were many dirigent genes, two nucleotide-binding site leucine-rich repeat (LRR) disease resistance genes, and two LRR protein genes.
The strongest upregulated biotic stress genes all appear to be involved in the biosynthesis of diterpenoid phytoalexins (DP) such as phytocassanes, oryzalexins, and momilactones via the methylerythritol phosphate (MEP) pathway (Figure 6, Table 1). Table 2 lists TFs that were reported to be involved in the regulation of DP production. OsTGAP1 is a chitin-elicitor induced basic leucine zipper (bZIP) TF involved in the regulation of momilactone and phytocassane biosynthesis in rice cells (Okada et al., 2009). OsbZIP79 is a protein that interacts with OsTGAP1 and acts as a suppressor of chitin-elicitor inducible expression of DP (Miyamoto et al., 2014). These regulators do not seem to be involved in the Pythium-induced DP induction. It is clear that especially the DP factor (DPF) is strongly upregulated by P. arrhenomanes inoculation. Strikingly, almost all MEP and DP biosynthesis genes listed in Table 1 are co-expressed with DPF. Interestingly, also other genes co-expressed with DPF as reported in Yamamura et al. (2015) were upregulated by P. arrhenomanes inoculation (see DPF regulon in Table 2). DPF is known to specifically accumulate in roots and husks and is induced by MeJA. Recently it was shown by Fukushima et al. (2016) and Akagi et al. (2014) that the WRKY45-WRKY62 heterodimer acts as a strong activator of DP biosynthetic genes. The simultaneous introduction of WRKY45 and WRKY62 resulted in a strong upregulation of the DPF promoter. These TFs were previously described to be induced by benzathiadiazole, an analogue of SA (Fukushima et al., 2016). Our data reveals that both TFs are induced in similar levels by P. arrhenomanes infection. Also activation of the OsMKK4-OsMPK3/OsMPK6 cascade leads to DP accumulation (Kishi-Kaboshi et al., 2010). Expression of OsWRKY53, one of the components of the downstream signaling of this cascade (Chujo et al., 2014), was also induced by P. arrhenomanes infection (Table 2). Next to the DPF regulon, also the recently described TF OsERF83 is strongly upregulated by P. arrhenomanes infection. This TF is transiently induced by treating rice seedlings with SA or ethephon and more constantly triggered by MeJA (Tezuka et al., 2019). PR genes upregulated in rice lines overexpressing OsERF83 are also transcriptionally activated upon P. arrhenomanes infection (Table 2).
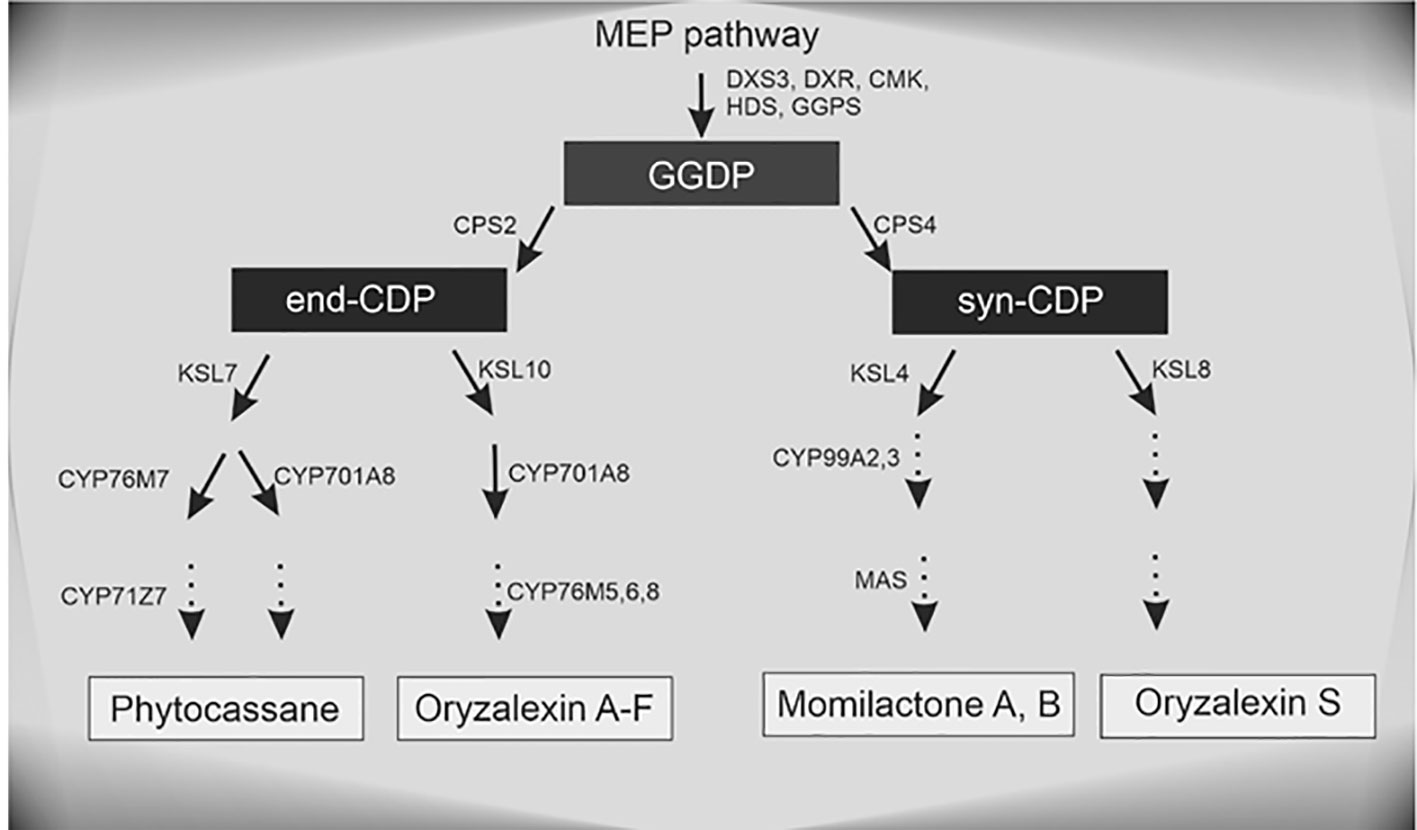
Figure 6 Methylerythritol 4-phosphate (MEP) pathway and subsequent diterpenoid phytoalexin biosynthesis in rice, based on Miyamoto et al. (2014).
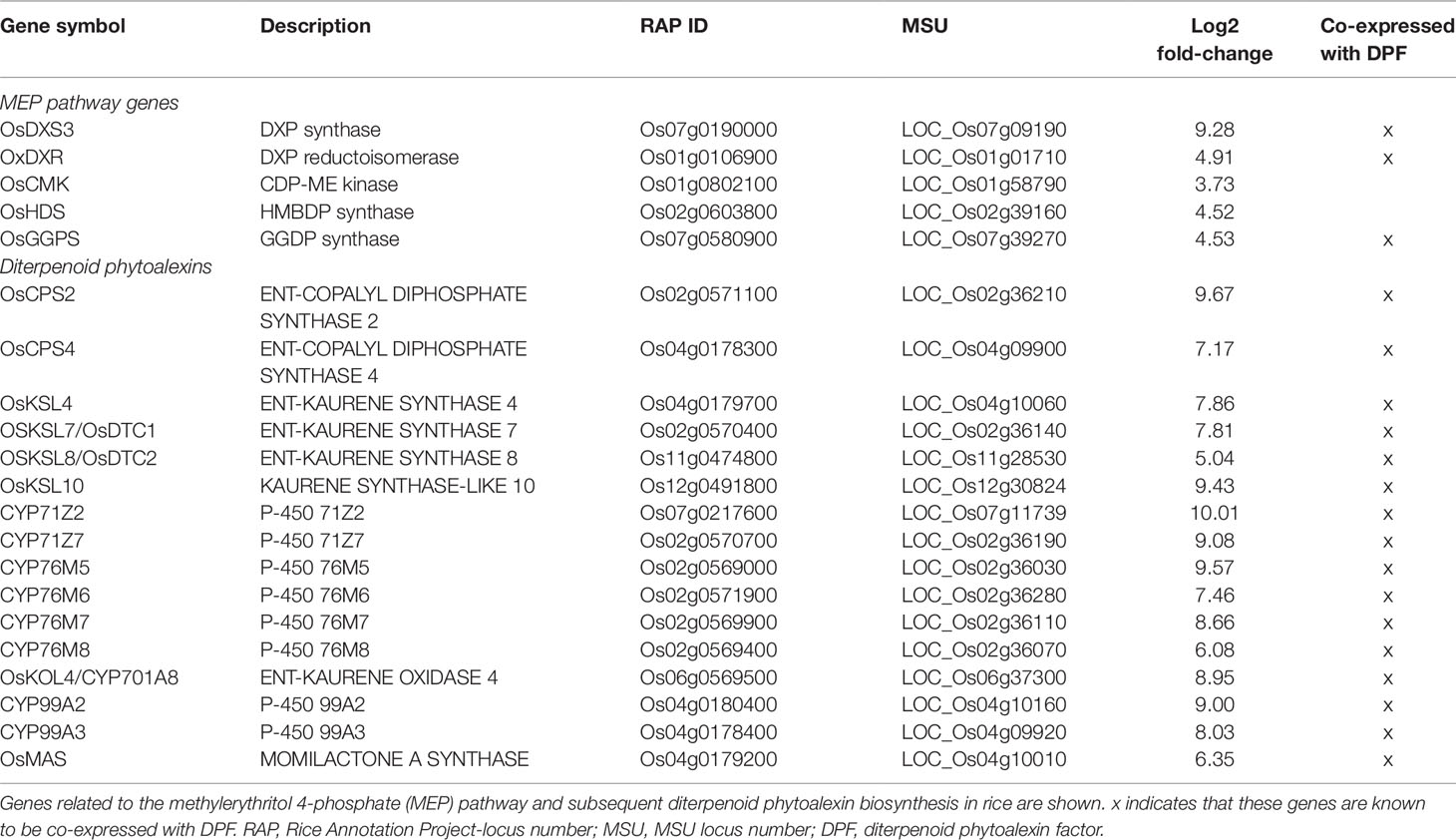
Table 1 Overview of transcriptional changes (log2 fold-change) as observed by micro-array analysis of Pythium arrhenomanes infected rice roots, at 2 days post inoculation in comparison with mock-inoculated roots.
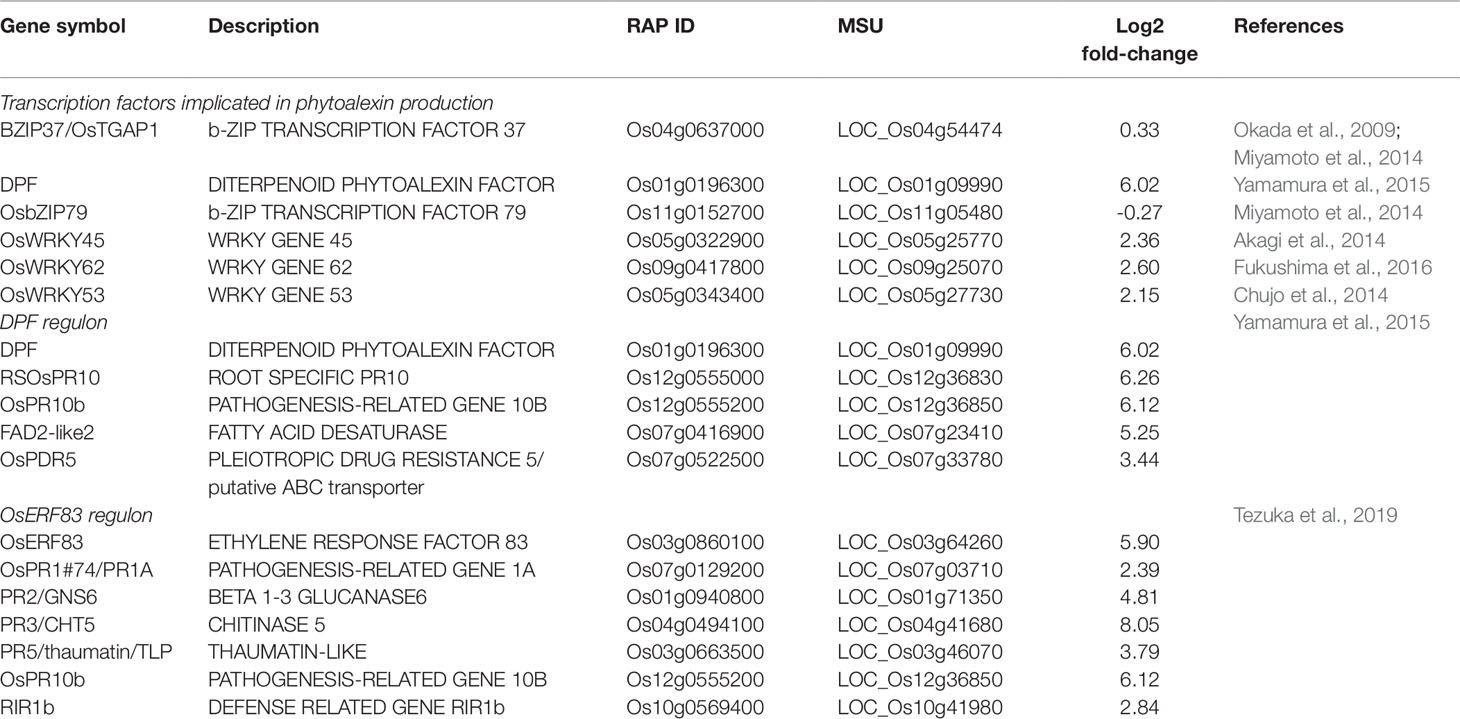
Table 2 Overview of transcriptional changes (log2 fold-change) in the expression of transcription factors involved in diterpenoid phytoalexin biosynthesis and genes known to be regulated by DPF and OsERF83, as observed by micro-array analysis of Pythium arrhenomanes infected rice roots at 2 days post inoculation, in comparison with mock-inoculated roots.
These data were validated and extended by qRT-PCR targeting jasmonate biosynthesis gene allene oxide synthase 2 (OsAOS2) and pathogenesis-related gene 10 (OsPR10), in single and double-inoculated rice plants. At this early time point after nematode inoculation (1 day), both genes were activated in the rice roots, probably corresponding to an early plant defense response triggered by nematode migration. Our data confirmed strongly induced expression of both genes at two days after P. arrhenomanes infection (Figure S4).
Discussion
In our previous work we have documented the antagonistic interaction between P. arrhenomanes and M. graminicola in rice grown under different experimental conditions (Verbeek et al., 2016). We observed that prior inoculation by P. arrhenomanes results in a reduction of the number of established nematodes, gall formation, and slower nematode development in rice roots. Samples taken during the previously described greenhouse experiment (Verbeek et al., 2016) were in this manuscript analyzed further by executing phytohormone measurements and gene expression analyses in rice roots.
Confirming the Dynamic Role of JA in the Warfare Between Rice and M. graminicola
Our data showed that JA levels are suppressed in rice roots upon M. graminicola infection. Ji et al. (2013) already reported that transcription of JA biosynthesis genes is locally suppressed in nematode-induced giant cells. Nahar et al. (2011) observed a similar phenomenon in very young gall tissue (1–2 dpi) and provided data showing that the JA pathway plays a pivotal role in rice defense against M. graminicola, information which was confirmed and extended here by the increased susceptibility of the JA-insensitive Osjar1 mutant (Figure 5). Nevertheless, at 3 dpi, JA has also been reported to be accumulating in gall tissue (Yimer et al., 2018). Transcriptome data of Kyndt et al. (2012) showed that while some JA related genes are suppressed, others are activated in 3 dpi galls. Our data show a similar view where OsAOS2 was also upregulated at 1 day after nematode infection (Figure S4). This activation is most probably due to minor root damage upon nematode penetration and gall expansion. The JA level in nematode-infected roots hence strongly varies depending on the tissue and time point that is being evaluated.
Pythium Infection Activates JA and Related Plant Defense Responses in Roots, Which Could Hamper Subsequent M. graminicola Infection
Our results showed a slight JA accumulation upon P. arrhenomanes infection at 4 days after inoculation. However, more importantly, JA suppression by M. graminicola infection was no longer observed when the roots were prior infected with P. arrhenomanes (Figure 1). Based on this observation, we hypothesized that JA might play a role in the antagonism, and a set of experiments was executed to confirm the induction of the JA pathway in host roots upon P. arrhenomanes infection. Two days after P. arrhenomanes inoculation on in vitro grown rice seedlings a clear induction of JA biosynthesis and JA signaling genes was detected using micro-array analysis, an observation which was correlated with increased JA levels in the roots (Figure 2). Increases in JA were previously described upon Pythium infection in moss (Oliver et al., 2009) and apple (Shin et al., 2014). The JA peak in rice roots appeared to be transient, as the boosted endogenous JA peak vanished at later time points (Figures 1 and 2). A similar transient peak was observed by Shin et al. (2014), who observed that transcription of an apple JA biosynthesis AOS gene peaked within 48 h after inoculation with P. ultimum and receded within 96 h. Interestingly, upon double inoculation of P. arrhenomanes and M. graminicola the JA levels in the roots can no longer be suppressed by the nematode (Figure 1).
The Role of JA in Plant Defense Against P. arrhenomanes
Confirming observations from the interaction between P. irregulare and Arabidopsis (Staswick et al., 1998), the JA pathway was found to negatively affect oomycete infection in rice roots. MeJA-amended rice seedlings were less susceptible to P. arrhenomanes, whereas increased susceptibility was observed in the Osjar1 transgenic line and the OsCOI1 RNAi-line (Figure 4). Noteworthy, application of a JA-biosynthesis inhibitor did not affect rice susceptibility to this oomycete, indicating that JA-signaling is more important for plant defense against this oomycete than actual JA levels. Interestingly, genes encoding proteins belonging to the JAZ family are strongly upregulated after P. arrhenomanes infection (Table S2). JAZ domains repress the transcription of JA responsive genes by binding to MYC2 domains. The SCFCOI1E3 ligase complex, which perceives JA-Ile presence, binds to the Jas domain of JAZ proteins and marks them for proteasomal degradation (Pauwels and Goossens, 2011). An effector protein of the ectomycorrhizal fungus Laccaria bicolor, MiSSP7, interacts with PtJAZ6 (Populus trichocarpa) in order to prevent JA-induced degradation of PtJAZ6 (Plett et al., 2014). P. arrhenomanes might possess similar effectors that can stabilize the JAZ proteins, as a mechanism to prevent JA-related defense gene expression in the host.
In double-inoculated plants amended with MeJA, nematode infection is suppressed and P. arrhenomanes is not able to further increase defense (Figure 5), indicating that the JA pathway plays a role in the interaction. However, it could be argued that exogenous application of MeJA has prevented P. arrhenomanes colonization and in this way indirectly precluded any antagonistic effect on the nematode. Nevertheless, in this double-inoculation experiment, P. arrhenomanes was inoculated 3 days prior to MeJA application, and Van Buyten and Höfte (2013) showed that P. arrhenomanes colonizes the inner root tissues 2 days upon inoculation, which renders this hypothesis questionable. It is therefore most likely that M. graminicola is affected by the P. arrhenomanes-induced JA-defense mechanisms, up to a level that cannot be suppressed efficiently by the nematode. However, the fact that the antagonism is reduced but still observable upon genetic or chemical inhibition of this pathway reveals that additional JA-independent mechanisms seem to be involved.
Transcriptional Changes Upon Pythium Infection in Rice: JA-Regulated Defense Responses, Involving DPF and ERF83
Interestingly, the micro-array analysis of P. arrhenomanes-infected root systems revealed a significant induction of genes involved in DP biosynthesis. Phytoalexins are known to be synthesized in infected plants in response to infection and can be secreted from root tissue, for example to act as allelochemicals (Toyomasu et al., 2008). In literature, a clear correlation between resistance against nematodes and levels of different phytoalexins has been reported in different host plants, e.g. resistant lima bean (Phaseolus lunatus) infected by Pratylenchus penetrans produces the phytoalexin coumestrol and banana cultivars resistant to Radopholous similis accumulate significant amounts of phenalenone-type phytoalexins, which are derived from the phenylpropanoid pathway (Hölscher et al., 2014). Similarly, production and exudation of phytoalexins by soybean root tissues infected with the cyst nematode Heterodera glycines are also restricted to resistant cultivars (Huang and Barker, 1991). Overexpression of the Arabidopsis phytoalexin-deficient 4 gene (AtPAD4) enhances resistance in soybean roots in response to the phytoparasitic nematode species Meloidogyne incognita (root-knot nematode) and H. glycines (Youssef et al., 2013). Upon overexpression of the rice momilactone A synthase gene in soybean, the H. glycines female index decreased to 41% of the control (Matthews et al., 2013). Nevertheless, except for the observation that systemic tissues of Hirschmaniella oryzae-infected plants show enhanced expression of a terpene synthase (LOC_Os12g30824; OsKSL10), necessary for the biosynthesis of ent-sandaracopimaradiene, a precursor of the DPs oryzalexin A–F (Kyndt et al., 2013), nothing more is known about the role of rice phytoalexins in the interaction with plant-parasitic nematodes. The phenolic sakuranetin possesses strong antimicrobial activity against the blast fungus (Kodama et al., 1992), while DP compounds from rice exhibit antibiotic activity against Rhizoctonia solani (Koga et al., 1997). However, we found no evidence of induction of flavonoid phytoalexins by P. arrhenomanes infection. NARINGENIN 7-O-METHYLTRANSFERASE (OsNOMT)(Os12g0240900), the key gene in sakuranetin biosynthesis, was not upregulated. Most rice phytoalexins are diterpenoid compounds, including momilactones, phytocassanes, and oryzalexins, and all of them have been found in extracts of, and exudates from, the roots of rice seedlings (Toyomasu et al., 2008). Data on potential nematicidal or nematode-repelling effects of these compounds have, however, not been reported in scientific literature and deserve to be further explored. The DP pathway is known to be induced by OsTGAP1, the OsMKK4-OsMPK3/MPK6 pathway probably via OsWRKY53 activation, by MeJA reviewed by Miyamoto et al. (2014) and by the BTH-inducible WRKY45-WRKY62 heterodimer (Fukushima et al., 2016). The most important regulator of the diterpenoid pathway is the DPF (Yamamura et al., 2015). This TF is highly upregulated by P. arrhenomanes infection and is inducible by MeJA. In addition, WRKY45-WRKY62 heterodimer-induced and OsMKK4-induced phytoalexin production appears to be mediated by DPF (Yamamura et al., 2015; Fukushima et al., 2016). This may explain why Osjar1-2 mutants inoculated with Magnaporthe oryzae could still produce DPs, showing that JA is not essential for DP biosynthesis (Shimizu et al., 2013). In this context it is interesting to notice that P. arrhenomanes could still reduce the numbers of nematode galls per root system in the Osjar1 mutant. Also other DPF-regulated genes are highly induced upon Pythium infection. Co1 or FAD2-like1, which is mainly expressed in roots, was also strongly induced by P. arrhenomanes. According to Yamamura et al. (2015) the protein encoded by FAD2-like1 is homologous to OsFAD2, a fatty acid desaturase that is a key enzyme for the conversion of oleic acid (18:1) into linoleic acid (18:2). The protein may be involved in pathogen-induced JA biosynthesis through production of the precursor, linoleic acid. OsPDR5 encodes a putative ABC transporter and may be involved in exporting antimicrobial metabolites, such as DPs (Yamamura et al., 2015). PR10b and RSOsPR10 are members of the PR10 family. RS0sPR10 is a root-specific protein that is specifically induced in roots in response to biotic and abiotic stress such as wounding (Bertini et al., 2019). Genes of several classes of PR proteins are also induced by OsERF83, a recently described TF with high similarity to the Arabidopsis TFs ERF15, ORA59, and ERF1 (Tezuka et al., 2019). OsERF83 is strongly expressed in roots and inducible by JA, SA, and ET. This TF and its regulon are upregulated by Pythium infection. A speculative model how DP biosynthestic genes and genes encoding PR proteins are upregulated upon P. arrhenomanes infection is presented in Figure 7.
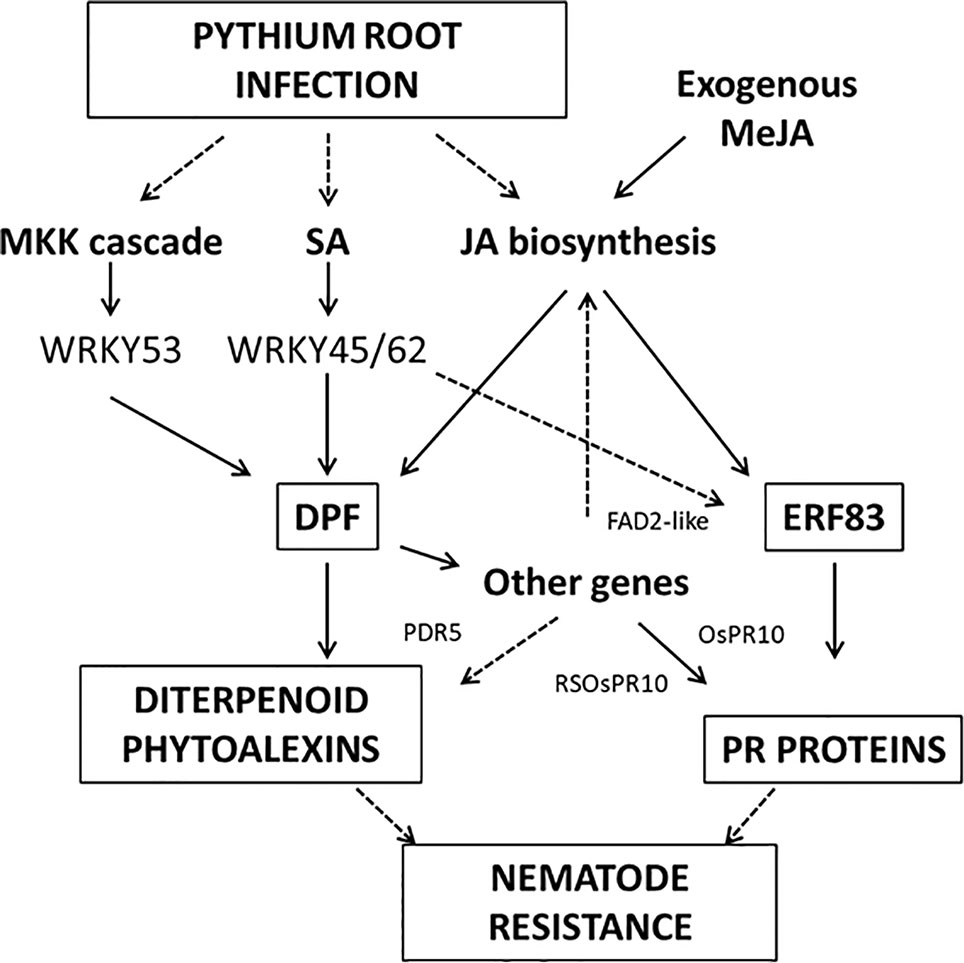
Figure 7 Speculative model showing how P. arrhenomanes infection can lead to the activation of genes involved in the biosynthesis of diterpenoid phytoalexins (DP) and PR proteins, resulting in induced nematode resistance.
A Potential Role for Auxin in the Antagonism Between P. arrhenomanes and M. graminicola in Rice?
Next to the induction of JA, hormone analyses also showed that the IAA level in the root is depleted in P. arrhenomanes infected roots at 6 days after inoculation (13 dpg, Figure 1). Auxin is important for gall formation by M. incognita in Arabidopsis, and AUX transporters re-distribute AUX from the rest of the root system toward the gall (Grunewald et al., 2009; Kyndt et al., 2016). Also in rice, blocking AUX transport significantly reduces the M. graminicola-induced gall formation and delays nematode development (Yimer et al., 2018). Although further confirmation is needed, a depleted level of IAA in the plant caused by P. arrhenomanes infection could be the reason for the hampered nematode feeding site formation and nematode development as previously seen in double-inoculated plants under high Pythium infection pressure (Verbeek et al., 2016). In this previous publication, the antagonism of P. arrhenomanes against M. graminicola has been observed in a variety of different experimental set-ups, and appeared to be positively correlated with P. arrhenomanes infection pressure (Verbeek et al., 2016). In the current manuscript we confirmed a similar antagonism under controlled laboratory conditions. However, while ideal for nematode inoculations, the SAP-substrate seemed less suited for P. arrhenomanes infection and therefore resulted in a weaker antagonism against M. graminicola, as compared to the previously published data. For example, under greenhouse conditions the antagonism was still observed when M. graminicola was inoculated six days after P. arrhenomanes inoculation (Verbeek et al., 2016), while in the SAP substrate the antagonism was only observable when using a maximum four-day interval between both inoculations (data not shown). Additionally, negative effects were only consistently observed on gall formation and nematode penetration, while nematode development was in some experiments unaffected under laboratory conditions (Figure 5). As observed before (Verbeek et al., 2016), the strength of the antagonism versus nematode infection seems strongly dependent on the infection pressure of P. arrhenomanes.
General Conclusion
Altogether, the here-reported data indicate that an early activation of the host jasmonate pathway upon P. arrhenomanes infection, correlated with the activation of ERF83 and DP genes, is at least partially responsible for a reduced number of nematodes infecting the root. Although further experimental confirmation is needed, an additional effect on nematode feeding site formation could potentially be related to AUX depletion upon P. arrhenomanes infection in the roots.
Data Availability Statement
The datasets generated for this study can be found in the GEO: number GSE133268.
Author Contributions
RV, GG, MH, and TK designed the study. EB and DV developed and performed the in vitro Pythium experiments. JB, DV, and SK performed the microarray study. AH and KD performed the hormone measurements. RV and MA performed the co-inoculation experiments. RV performed the statistical analyses and wrote the paper. GG, MH, and TK helped in revising the paper. All authors read and approved the final manuscript.
Funding
This research was supported by FWO-project G.0315.13 N and GOA-project G.03013.
Conflict of Interest
The authors declare that the research was conducted in the absence of any commercial or financial relationships that could be construed as a potential conflict of interest.
Acknowledgments
We would like to thank the International Rice Research Institute and more specially Casiana Vera Cruz and Dirk de Waele for the help with the initial greenhouse experiments. The authors also acknowledge the financial support (AUGE/11/016) from the Hercules Foundation of the Flemish Government for the UHPLC-Q-ExactiveTM mass spectrometry equipment used for hormone analysis.
Supplementary Material
The Supplementary Material for this article can be found online at: https://www.frontiersin.org/articles/10.3389/fpls.2019.01515/full#supplementary-material
References
Akagi, A., Fukushima, S., Okada, K., Jiang, C.-J., Yoshida, R., Nakayama, A., et al. (2014). WRKY45-dependent priming of diterpenoid phytoalexin biosynthesis in rice and the role of cytokinin in triggering the reaction. Plant Mol. Biol. 86,171–183. doi: 10.1007/s11103-014-0221-x
Bertini, L., Palazzi, L., Proietti, S., Pollastri, S., Arrigoni, G., Polverino de Laureto, P., et al. (2019). Proteomic analysis of meja-induced defense responses in rice against wounding. Int. J. Mol. Sci. 20, 2525.
Blake, S. N., Barry, K. M., Gill, W. M., Reid, J. B., Foo, E. (2015). The role of strigolactones and ethylene in disease caused by Pythium irregulare. Mol. Plant Pathol. 17, 680–690. doi: 10.1111/mpp.12320
Bond, J. P., McGawley, E. C., Hoy, J. W. (2004). Sugarcane growth as influenced by nematodes and Pythium arrhenomanes. Nematropica 34, 245–256.
Chujo, T., Miyamoto, K., Ogawa, S., Masuda, Y., Shimizu, T., Kishi-Kaboshi, M., et al. (2014). Overexpression of phosphomimic mutated OsWRKY53 leads to enhanced blast resistance in rice. PloS One 9, e98737. doi: 10.1371/journal.pone.0098737
De Vleesschauwer, D., van Buyten, E., Satoh, K., Balidion, J., Mauleon, R., Choi, I. R., et al. (2012). Brassinosteroids antagonize gibberellin- and salicylate-mediated root immunity in rice. Plant Physiol. 158, 1833–1846. doi: 10.1104/pp.112.193672
De Vleesschauwer, D., Gheysen, G., Höfte, M. (2013). Hormone defense networking in rice: tales from a different world. Trends In Plant Sci. 18, 555–565. doi: 10.1016/j.tplants.2013.07.002
Du, Z., Zhou, X., Ling, Y., Zhang, Z., Su, Z. (2010). agriGO: a GO analysis toolkit for the agricultural community. Nucleic Acids Res. Adv. Access 38, W64–W70. doi: 10.1093/nar/gkq310
Fukumoto, K., Alamgir, K. M., Yamashita, Y., Mori, I. C., Matsuura, H., Galis, I. (2013). Response of rice to insect elicitors and the role of OsJAR1 in wound and herbivory-induced JA-Ile accumulation. J. Integr. Plant Biol. 55, 775–784. doi: 10.1111/jipb.12057
Fukushima, S., Mori, M., Sugano, S., Takatsuji, H. (2016). Transcription factor WRKY62 plays a role in pathogen defense and hypoxia-responsive gene expression in rice. Plant Cell Physiol. 57, 2541–2551. doi: 10.1093/pcp/pcw185
Grunewald, W., Van Noorden, G., Van Lsterdael, G., Beeckman, T., Gheysen, G., Mathesius, U. (2009). Manipulation of auxin transport in plant roots during rhizobium symbiosis and nematode parasitism. Plant Cell 21,2553–2562. doi: 10.1105/tpc.109.069617
Hölscher, D., Dhakshinamoorthy, S., Alexandrov, T., Becker, M., Bretschneider, T., Buerkert, A., et al. (2014). Phenalenone-type phytoalexins mediate resistance of banana plants (Musa spp.) to the burrowing nematode Radopholus similis. Proc. Natl. Acad. Sci. 111, 105–110. doi: 10.1073/pnas.1314168110
Haeck, A., Van Langenhove, H., Harinck, L., Kyndt, T., Gheysen, G., Höfte, M. (2018). Trace analysis of multi-class phytohormones in Oryza sativa using different scan modes in high-resolution Orbitrap mass spectrometry: method validation, concentration levels, and screening in multiple accessions. Anal. Bioanal. Chem. 410, 4527–4539. doi: 10.1007/s00216-018-1112-9
Huang, J.-S., Barker, K. R.. (1991). Glyceollin I in soybean-cyst nematode interactions: spatial and temporal distribution in roots of resistant and susceptible soybeans. Plant Physiol. 96, 1302–1307. doi: 10.1104/pp.96.4.1302
Ji, H. L., Gheysen, G., Denil, S., Lindsey, K., Topping, J. F., Nahar, K., et al. (2013).Transcriptional analysis through RNA sequencing of giant cells induced by Meloidogyne graminicola in rice roots. J. Exp. Bot. 64, 3885–3898. doi: 10.1093/jxb/ert219
Kim, S.-Y., Volsky, D. J.(2005). PAGE: Parametric Analysis of Gene Set Enrichment. BMC Bioinf. 6, 144–144. doi: 10.1186/1471-2105-6-144
Kishi-Kaboshi, M., Okada, K., Kurimoto, L., Murakami, S., Umezawa, T., Shibuya, N., et al. (2010). A rice fungal MAMP-responsive MAPK cascade regulates metabolic flow to antimicrobial metabolite synthesis. Plant J. 63, 599–612. doi: 10.1111/j.1365-313X.2010.04264.x
Kodama, O., Miyakawa, J., Akatsuka, T., Kiyosawa, S. (1992). Sakuranetin, a flavanone phytoalexin from ultraviolet-irradiated rice leaves. Phytochemistry 31, 3807–3809. doi: 10.1016/S0031-9422(00)97532-0
Koga, J., Ogawa, N., Yamauchi, T., Kikuchi, M., Ogasawara, N., Shimura, M. (1997). Functional moiety for the antifungal activity of phytocassane E, a diterpene phytoalexin from rice. Phytochemistry 44, 249–253. doi: 10.1016/S0031-9422(96)00534-1
Kreye, C., Bouman, B. A. M., Reversat, G., Fernandez, L., Vera Cruz, C., Elazegui, F., et al. (2009a). Biotic and abiotic causes of yield failure in tropical aerobic rice.Field Crops Res. 112, 97–106. doi: 10.1016/j.fcr.2009.02.005
Kreye, C., Bouman, B. A. M., Castañeda, A. R., Lampayan, R. M., Faronilo, J. E., Lactaoen, A. T., et al. (2009b). Possible causes of yield failure in tropical aerobic rice. Field Crops Res. 111, 197–206. doi:10.1016/j.fcr.2008.12.007
Kyndt, T., Denil, S., Haegeman, A., Trooskens, G., Bauters, L., Van Criekinge, W., et al. (2012). Transcriptional reprogramming by root knot and migratory nematode infection in rice. New Phytol. 196, 887–900. doi: 10.1111/j.1469-8137.2012.04311.x
Kyndt, T., Vieira, P., Gheysen, G., de Almeida-Engler, J. (2013). Nematode feeding sites: unique organs in plant roots. Planta 238, 807–818. doi:10.1007/s00425-013-1923-z
Kyndt, T., Goverse, A., Haegeman, A., Warmerdam, S., Wanjau, C., Jahani, M., et al. (2016). Redirection of auxin flow in Arabidopsis thaliana roots after infection by root-knot nematodes. J. Exp. Bot. 67, 4559–4570. erw230. doi: 10.1093/jxb/erw230
Kyndt, T., Nahar, K., Haeck, A., Verbeek, R., Demeestere, K., Gheysen, G. (2017). Interplay between carotenoids, abscisic acid and jasmonate guides the compatible rice-Meloidogyne graminicola interaction. Front. In Plant Sci. 67 (8), 951. doi: 10.3389/fpls.2017.00951
Lyons, R., Manners, J. M., Kazan, K. (2013). Jasmonate biosynthesis and signaling in monocots: a comparative overview. Plant Cell Rep. 32, 815–827. doi: 10.1007/s00299-013-1400-y
Mantelin, S., Bellafiore, S., Kyndt, T. (2017). Meloidogyne graminicola: a major threat to rice agriculture. Mol. Plant Pathol. 18 (1), 3–15.doi: 10.1111/mpp.12394
Matthews, B. F., Beard, H., MacDonald, M. H., Kabir, S., Youssef, R. M., Hosseini, P., et al. (2013). Engineered resistance and hypersusceptibility through functional metabolic studies of 100 genes in soybean to its major pathogen, the soybean cyst nematode. Planta 237, 1337–1357. doi: 10.1007/s00425-013-1840-1
Miyamoto, K., Shimizu, T., Okada, K. (2014). Transcriptional regulation of the biosynthesis of phytoalexin: a lesson from specialized metabolites in rice. Plant Biotechnol. 31 (14), 377–388. doi: 10.5511/plantbiotechnology.14.0730a
Miyao, A., Tanaka, K., Murata, K., Sawaki, H., Takeda, S., Abe, K., et al. (2003).Target site specificity of the Tos17 retrotransposon shows a preference for insertion within genes and against insertion in retrotransposon-rich regions of the genome. Plant Cell 15, 1771–1780. doi: 10.1105/tpc.012559
Mojdehi, H., Singleton, L. L., Richardson, P. E. (1991). Histopathology of wheat seedling roots infected with Pythium arrhenomanes. J. Phytopathol. Z. 132, 75–83. doi: 10.1111/j.1439-0434.1991.tb00095.x
Nahar, K., Kyndt, T., de Vleesschauwer, D., Höfte, M., Gheysen, G. (2011). The jasmonate pathway is a key player in systemically induced defense against root-knot nematodes in rice. Plant Physiol. 157, 305–316. doi: 10.1104/pp.111.177576
Nahar, K., Kyndt, T., Nzogela, Y. B., Gheysen, G. (2012). Abscisic acid interacts antagonistically with classical defense pathways in rice-migratory nematode interaction. New Phytol. 196, 901–913. doi: 10.1111/j.1469-8137.2012.04310.x
Okada, A., Okada, K., Miyamoto, K., Koga, J., Shibuya, N., Nojiri, H., et al. (2009). Os, TGAP1, a bZIP transcription factor, coordinately regulates the inductive production of diterpenoid phytoalexins in rice. J. Biol. Chem. 284, 26510–26518. doi: 10.1074/jbc.M109.036871
Oliver, J. P., Castro, A., Gaggero, C., Cascón, T., Schmelz, E. A., Castresana, C., et al. (2009). Pythium infection activates conserved plant defense responses in mosses. Planta 230, 569–579. doi: 10.1007/s00425-009-0969-4
Pauwels, L., Goossens, A. (2011). The JAZ Proteins: a crucial interface in the Jasmonate signaling cascade. Plant Cell Online 23, 3089–3100. doi: 10.1105/tpc.111.089300
Pieterse, C. M., Van der Does, D., Zamioudis, C., Leon-Reyes, A., Van Wees, S. C. (2012). Hormonal modulation of plant immunity. Annu. Rev. Cell Dev. Biol. 28, 489–521. doi: 10.1146/annurev-cellbio-092910-154055
Plett, J. M., Daguerre, Y., Wittulsky, S., Vayssières, A., Deveau, A., Melton, S. J., et al. (2014). Effector MiSSP7 of the mutualistic fungus Laccaria bicolor stabilizes the Populus JAZ6 protein and represses jasmonic acid (JA) responsive genes.Proc. Natl. Acad. Sci. 111, 8299–8304. doi: 10.1073/pnas.1322671111
Reversat, G., Boyer, J., Sannier, C., Pando-Bahuon, A. (1999). Use of a mixture of sand and water-absorbent synthetic polymer as substrate for the xenic culturing of plant-parasitic nematodes in the laboratory. Nematology 1, 209–212. doi: 10.1163/156854199508027
Riemann, M., Riemann, M., Takano, M. (2008). Rice JASMONATE RESISTANT 1 is involved in phytochrome and jasmonate signalling. Plant Cell Environ. 31, 783–792. doi: 10.1111/j.1365-3040.2008.01790.x
Satoh, K., Kondoh, H., Sasaya, T., Shimizu, T., Choi, I. R., Omura, T., et al. (2010). Selective modification of rice (Oryza sativa) gene expression by rice stripe virus infection. J. Gen. Virol. 91, 294–305. doi: 10.1099/vir.0.015990-0
Shimizu, T., Miyamoto, K., Miyamoto, K., Minami, E., Nishizawa, Y., Iino, M., et al. (2013). OsJAR1 contributes mainly to biosynthesis of the stress-induced jasmonoyl-isoleucine involved in defense responses in rice. Biosci. Biotechnol. Biochem. 77, 1556–1564. doi: 10.1271/bbb.130272
Shin, S., Lv, J., Fazio, G., Mazzola, M., Zhu, Y. (2014). Transcriptional regulation of ethylene and jasmonate mediated defense response in apple (Malus domestica) root during Pythium ultimum infection. Hortic. Res. 1, 14053. doi: 10.1038/hortres.2014.53
Staswick, P. E., Yuen, G. Y., Lehman, C. C. (1998). Jasmonate signaling mutants of Arabidopsis ares usceptible to the soil fungus Pythium irregulare. Plant J. 15,747–754. doi: 10.1046/j.1365-313X.1998.00265.x
Tezuka, D., Kawamata, A., Kato, H., Saburi, W., Mori, H., Imai, R. (2019). The rice ethylene response factor OsERF83 positively regulates disease resistance to Magnaporthe oryzae. Plant Physiol. Biochem. 135, 263–271. doi: 10.1016/j.plaphy.2018.12.017
Thimm, O., Bläsing, O., Gibon, Y., Nagel, A., Meyer, S., Krüger, P., et al. (2004).Mapman: a user-driven tool to display genomics data sets onto diagrams of metabolic pathways and other biological processes. Plant J. 37, 914–939. doi: 10.1111/j.1365-313X.2004.02016.x
Toyomasu, T., Kagahara, T., Okada, K., Koga, J., Hasegawa, M., Mitsuhashi, W., et al. (2008). Diterpene phytoalexins are biosynthesized in and exuded from the roots of rice seedlings. Biosci. Biotechnol. Biochem. 72, 562–567. doi: 10.1271/bbb.70677
Van Buyten, E., Höfte, M. (2013). Pythium species from rice roots differ in virulence, host colonization and nutritional profile. BMC Plant Biol. 13, 203. doi: 10.1186/1471-2229-13-203
Van Buyten, E., Banaay, C. G. B., Vera Cruz, C., Höfte, M. (2013). Identity and variability of Pythium species associated with yield decline in aerobic rice cultivation in the Philippines. Plant Pathol. 62, 139–153. doi: 10.1111/j.1365-3059.2012.02607.x
Verbeek, R. E. M., Banaay, C. G. B., Sikder, M., De Waele, D., Vera Cruz, C. M., Gheysen, G., et al. (2016). Interactions between the oomycete Pythium arrhenomanes and the rice root-knot nematode Meloidogyne graminicolain aerobic Asian rice varieties. Rice 9, 1–12. doi: 10.1186/s12284-016-0108-3
Yamamura, C., Mizutani, E., Okada, K., Nakagawa, H., Fukushima, S., Tanaka, A., et al. (2015). Diterpenoid phytoalexin factor, a bHLH transcription factor, plays a central role in the biosynthesis of diterpenoid phytoalexins in rice. Plant J. 84, 1100–1113. doi: 10.1111/tpj.13065
Yang, D.-L., Yao, J., Mei, C.-S., Tong, X.-H., Zeng, L.-J., Li, Q., et al. (2012). Plant hormone jasmonate prioritizes defense over growth by interfering with gibberellin signaling cascade. Proc. Natl. Acad. Sci. 109 (19), E1192–E1200. doi: 10.1073/pnas.1201616109
Yimer, H. Z., Nahar, K., Kyndt, T., Haeck, A., Van Meulebroek, L., Vanhaecke, L., et al. (2018). Gibberellin antagonizes jasmonate-induced defense against Meloidogyne graminicola in rice. New Phytol. 218, 646–660. doi: 10.1111/nph.15046
Youssef, R. M., MacDonald, M. H., Brewer, E. P., Bauchan, G. R., Kim, K.-H., Matthews, B. F. (2013). Ectopic expression of AtPAD4 broadens resistance of soybean to soybean cyst and root-knot nematodes. BMC Plant Biol. 13, 67. doi: 10.1186/1471-2229-13-67
Keywords: rice, oomycete, root-knot nematode (Meloidogyne graminicola), jasmonate, auxin, antagonism
Citation: Verbeek REM, Van Buyten E, Alam MZ, De Vleesschauwer D, Van Bockhaven J, Asano T, Kikuchi S, Haeck A, Demeestere K, Gheysen G, Höfte M and Kyndt T (2019) Jasmonate-Induced Defense Mechanisms in the Belowground Antagonistic Interaction Between Pythium arrhenomanes and Meloidogyne graminicola in Rice. Front. Plant Sci. 10:1515. doi: 10.3389/fpls.2019.01515
Received: 08 July 2019; Accepted: 31 October 2019;
Published: 22 November 2019.
Edited by:
Víctor Flors, University of Jaume I, SpainReviewed by:
Silvia Proietti, Università degli Studi della Tuscia, ItalySanushka Naidoo, University of Pretoria, South Africa
Raquel Campos-Herrera, Institute of Vine and Wine Sciences (ICVV), Spain
Copyright © 2019 Verbeek, Van Buyten, Alam, De Vleesschauwer, Van Bockhaven, Asano, Kikuchi, Haeck, Demeestere, Gheysen, Höfte and Kyndt. This is an open-access article distributed under the terms of the Creative Commons Attribution License (CC BY). The use, distribution or reproduction in other forums is permitted, provided the original author(s) and the copyright owner(s) are credited and that the original publication in this journal is cited, in accordance with accepted academic practice. No use, distribution or reproduction is permitted which does not comply with these terms.
*Correspondence: Tina Kyndt, VGluYS5LeW5kdEBVR2VudC5iZQ==