- 1Instituto de Biología Molecular y Celular de Plantas, Consejo Superior de Investigaciones Científicas (CSIC)—Universidad Politécnica de Valencia, Valencia, Spain
- 2Department of Biosciences, Durham University, Durham, United Kingdom
Efficient elimination of the editing machinery remains a challenge in plant biotechnology after genome editing to minimize the probability of off-target mutations, but it is also important to deliver end users with edited plants free of foreign DNA. Using the modular cloning system Golden Braid, we have included a fluorescence-dependent transgene monitoring module to the genome-editing tool box. We have tested this approach in Solanum lycopersicum, Oryza sativa, and Arabidopsis thaliana. We demonstrate that DsRED fluorescence visualization works efficiently in dry seeds as marker for the detection of the transgene in the three species allowing an efficient method for selecting transgene-free dry seeds. In the first generation of DsRED-free CRISPR/Cas9 null segregants, we detected gene editing of selected targets including homozygous mutants for the plant species tested. We demonstrate that this strategy allows rapid selection of transgene-free homozygous edited crop plants in a single generation after in vitro transformation.
Introduction
CRISPR/Cas technology, adapted from bacterial immune system (Bolotin et al., 2005; Mojica et al., 2005), for specific and precise modification of genomes (Jinek et al., 2012; Wiedenheft et al., 2012) has transformed molecular biology. The efficiency of CRISPR/Cas9 genome-editing methodology was quickly demonstrated in plants (Feng et al., 2013; Jiang et al., 2013). Arabidopsis thaliana can be easily transformed in planta (Bechtold and Pelletier, 1998), but this is actually an exception because in vitro transformation is the most common methodology to generate stable transformed plants, which is a labor-intensive procedure that requires appropriate infrastructures, and more importantly, the regeneration process is slow and, depending on the species, it can range from several months to a year (Busov et al., 2005). Many markers have been used for selecting transformed plants, but the most commonly used are genes that confer resistance to antibiotics or herbicides (Miki and Mchugh, 2004). Markers are key elements for in vitro selection of cells with transgene integration, the regeneration of plants, and selection in the next generations; however, the presence of resistance genes raises concerns about biosecurity, and several strategies have also been developed for their elimination while retaining the genes of interest, or for using marker-free transformation strategies (Darbani et al., 2007; Yau and Stewart, 2013), but these strategies are neither shorter nor simpler. Thus, quick elimination of the transgene remains a challenge in plant biotechnology after genome editing, especially for crops due to their long life cycle and multiploidy, not only to avoid transgene position effects and to minimize the probability of off-target mutation appearance but also to deliver end users with edited plants free of the recombinant gene-editing machinery. Counter selection based on resistance marker genes are inconvenient in the case of CRISPR/Cas applications because plants lacking the transgene cannot survive the selection, and thus two more generations must be screened to evaluate the presence of the transgene. In the case of some crops, generations can last between 4 and 6 months and a few years, and the workload may be a limiting factor because transgene detection by PCR requires germination of seeds, so selected plants must be grown until the next generation can be harvested. The expression of fluorescent proteins as selective markers has been successfully used in A. thaliana (Stuitje et al., 2003; Shimada et al., 2010) as a fast method for transgene presence detection prior to seed germination. Moreover, it has also been used in combination with CRISPR in Arabidopsis (Gao et al., 2016; Durr et al., 2018; Wu et al., 2018; Yu and Zhao, 2019). Despite its clear advantage, it has not been tested in species such as tomato or rice, because of the special requirements of in vitro transformation protocols. To overcome the abovementioned technical difficulty, we have adapted fluorescence-mediated monitoring of transgenes to genome-editing approaches in these species with the goal of obtaining transgene-free homozygous gene edited crop plants in two generations.
Materials and Methods
Plant Material and Growth Conditions
Seeds of A. thaliana (ecotype Col-0) was stratified in agarose 0.05% (in water) for 3 days at 4°C, sown on pots containing soil mix (1:1:1 perlite, vermiculite, and peat [DSM]) and grown in greenhouse growth chambers at 22°C under long day conditions (16 h of light and 8 h of darkness). Rice (Oryza sativa L. cv. Nipponbare) seeds were put on ½ MS basal salts with 1% sucrose left in dark at 28°C for 2 days and then transfer to Sanyo growth chamber with light on for 1 week. The seedlings were planted in pots (8 × 8 × 10 cm) containing 180-g water-soaked soil. Plants were grown in white fluorescent light (600 photons m−2 s−1, 14 h of light/10 h of dark) at 28°C/25°C) and 70% relative humidity. Tomato (Solanum lycopersicum cv. money maker) seeds were sown on pots containing soil mix [1:2 perlite and peat (OPM)] and grown in greenhouse chambers at 24°C under long day conditions (16 h of light and 8 h of darkness). Each new generation was obtained leaving each plant to be autopollinated.
Phylogenetic Analysis
BLAST against tomato (SGN) (Fernandez-Pozo et al., 2015) was performed using protein sequence of AtIAMT1 (At5g55250). The best four hits were used for alignment against IAMT1 protein sequences from A. thaliana, Brassica rapa, Medicago truncatula, and O. sativa using ClustalX2 (Larkin et al., 2007) with default parameters.
sgRNA Target Selection
ARES-GT software (https://github.com/eugomin/ARES-GT.git) was used for identification and selection of CRISPR targets. Parameters for identification of possible off-targets were: less than five mismatches (L0 = 4) or less than four mismatches (L1 = 3) if one mismatch is found in seed sequence. Targets with no expected off-targets and close to the start of the ORF were selected (Supplementary Table S1).
Plasmid Construction
All vectors used in this work have been designed using GoldenBraid system following the described assembly strategy (Sarrion-Perdigones et al., 2013; Sarrion-Perdigones et al., 2014; Vazquez-Vilar et al., 2015; Vazquez-Vilar et al., 2016). We generated vectors containing the hCas9 CDS (GB0575) under the control of optimal promoters for each species: AtUBQ10 (GB2478), ZmUBQ (EGM001), and CaMV 35S (GB0030) for Arabidopsis, rice, and tomato, respectively. Similarly, the sgRNA multiplexed transcriptional unit (TU) were placed under the control of the AtU6-26 promoter (GB1001) for Arabidopsis and tomato, and the OsU3 promoter (GB1184) for rice (Vazquez-Vilar et al., 2016). Specific resistance genes were also introduced into each vector for in vitro selection, as required by crop transformation protocols: hygromycin (GB0211) and kanamycin (GB0226) for rice and tomato, respectively. Finally, an additional TU for expression of the fluorescent protein DsRED under the control of the At2S3 promoter (Kroj et al., 2003; Ravi et al., 2014; Bernabé-Orts et al., 2019) (GB2482) for Arabidopsis or CaMV 35S promoter (GB0361) for rice, and tomato was added in each final vector (Figure 1B; Supplementary Figures 2 and 3; Supplementary Tables 2 and 3). In all cases, TU used for primary transformant selection was placed at the LB. In the case of rice and tomato, TU for expression of DsRED was placed in the RB. Sequences of GB-Parts are accessible at GB cloning website (https://gbcloning.upv.es/) using the GB database ID.
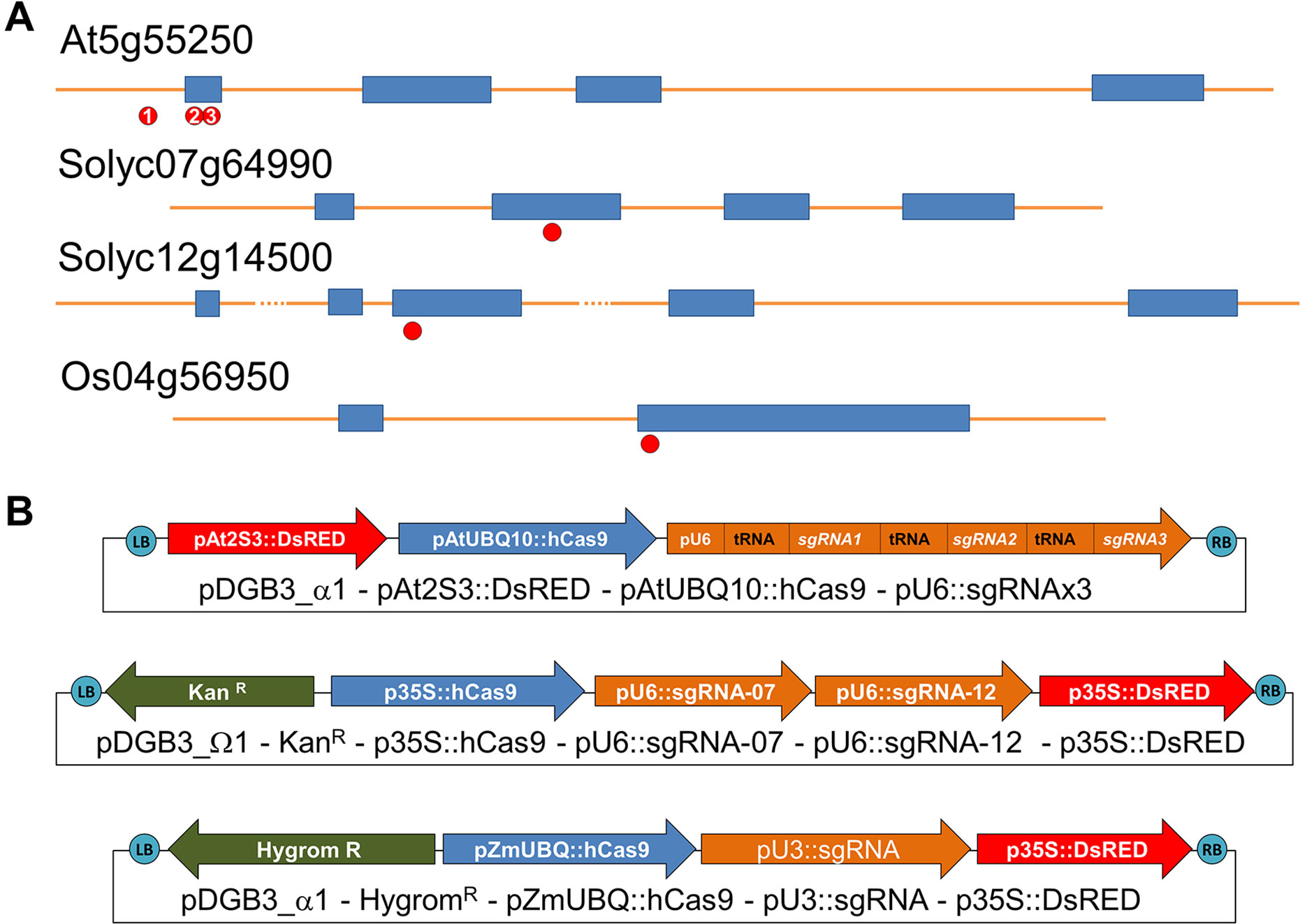
Figure 1 (A) Position of each CRISPR target in selected genes At5g55250 (Arabidopsis thaliana), Solyc07g64990 and Solyc12g14500 (Solanum lycopersicon), and Os04g56950 (Oryza sativa). (B) Description of transcriptional units (arrows) assembled in the vectors generated for plant transformation using the modular system GoldenBraid. Specific promoters were selected for expression of Cas9 protein in each species, pAtUBQ10 in Arabidopsis thaliana, p35S for Solanum Lycopersicum, and pZmUBQ for Oryza sativa. Adequate promoter for expression of sgRNA was selected: pAtU6-26 for dicotyledonous species (Solanum and Arabidopsis) and pOsU3 for monocotyledonous species (rice).
Plant Transformation
Arabidopsis was transformed by floral dip (Clough and Bent, 1998), with a minor modification: 1 min dipping into a solution (sucrose 5% + 0.2 ml Silwet-77/L) containing Agrobacterium tumefaciens. Protocol from Ellul et al. (2003) was followed for in vitro tomato transformation. All in vitro steps were carried out in a long-day growth chamber (16 h light/8 h dark, 24°C, 60–70% humidity, 250 μmol/m2/s). Protocol from Hiei and Komari (2008) till step 19 was used for in vitro transformation of rice; the regeneration (R-III) and rooting (HF) were done as described in Toki et al. (2006). Fluorescent seeds, containing the transgene, were identified in a Leica DMS1000 microscope with DsRED filter.
Plant Genotyping
Genomic DNA was obtained from young leaves in all cases. CTAB extraction protocol (Murray and Thompson, 1980) was used in the case of tomato and rice, with small modifications: 600 μl of CTAB fresh buffer (2% CTAB, 1.4 M NaCl, 20 mM EDTA, 100 mM Tris-HCl pH 8.0, 2% β-mercaptoethanol) is added to 100 mg of ground tissue powder prior 45-min incubation at 65°C; then, 600 μl of chloroform:isoamyl alcohol (24:1) is added, extract is emulsified by vortex, and water phase is recovered after 10 min of centrifugation (13,000 rpm); then, 1 volume of 2-propanol is added and mixed gently and, after 20 min at 80ºC, centrifuged for 10 min at 4°C (13,000 rpm); pellet is washed once with ethanol, and after 5-min centrifugation (13,000 rpm), supernatant is discarded; finally, dry pellet is resuspended with 100 μl of Milli-Q water. In the case of Arabidopsis, the protocol described in Edwards et al. (1991) was used. PCR was performed using the specific primers for each fragment (Supplementary Table S4) and the MyTaq Red DNA Polymerase (BIO21110 Bioline-Ecogen) following manufacturer specifications. Macherey-Nagel NucleoSpin® Gel and PCR Clean-Up Kit (ref. 740609.250) was used for PCR product purification, and sequences were determined by Sanger sequencing (GenoScreen services). Chromatograms of heterozygous and biallelics was analyzed by TIDE (Brinkman et al., 2014) and/or by visual inspection to determine the exact sequence of each allele.
Results and Discussion
As a proof of concept, we decided to use the gene encoding IAA methyl transferase (IAMT) as a gene-editing target in three plant model species (A. thaliana, O. sativa, and S. lycopersicum), given that loss of function results only in difficulty for hypocotyl reorientation after gravistimulation (Abbas et al., 2018b) and increased pollen tube growth rate (Abbas et al., 2018a), neither of which are traits that can bias our identification of mutations by direct observation unless specific tests are performed. In A. thaliana and O. sativa, only one gene per species has been identified that encodes an enzyme with IAMT activity (At5g55250 and Os04g56950, respectively) (Qin et al., 2005; Yang et al., 2008). However, in tomato, we have identified two orthologs of IAMT1 (Solyc07g64990 and Solyc12g14500) by phylogenetic analysis (Supplementary Figure 1). Therefore, we decided to test different editing strategies in each of the three selected species: targeting only one gene with one sgRNA (in rice), simultaneously targeting two genes with two sgRNAs (in tomato), and targeting different regions of a single gene (in Arabidopsis) to evaluate the efficiency of the vectors when looking for multiple mutations and larger deletions. Genomic DNA sequence for each selected gene in each species was analyzed with ARES-GT tool for the identification and selection of sgRNAs (Figure 1A; Supplementary Table 1).
Thanks to the modular design for construct generation through the GoldenBraid cloning system (Sarrion-Perdigones et al., 2013; Vazquez-Vilar et al., 2015; Vazquez-Vilar et al., 2016), we generated one vector for each plant species with all needed transcriptional units (Figure 1B; Supplementary Figures 2 and 3). Each final GoldenBraid construct was introduced into the corresponding plant species following published transformation protocols (see Materials and Methods) (Figure 2). Seeds from transformed Arabidopsis plants were harvested, and DsRED fluorescence was used to select 15 T1 seeds with high DsRED signal. In vitro selection of callus from tomato leaves and rice grains allowed the regeneration of eight kanamycin resistance transgenic tomato lines and 20 hygromycin resistance transgenic rice lines. Ideally, those primary transformant plants contain one copy of the transgene that will be segregated in the next generation independently of any CRISPR-induced mutations in germline; thus, we could use DsRED visualization as marker of transgene presence to select nonfluorescent seeds and then search for mutations. While all primary transformants of rice and Arabidopsis produced seeds, two of the tomato plants presented a dwarf phenotype and did not produce any fruit. Segregation analysis was done by visual observation of segregant dry seeds under a stereoscope equipped with DsRED filter. While signal in tomato presented a homogeneous pattern in the embryo in all lines, in rice, the intensity of DsRED signal in embryo and endosperm varies between lines (Supplementary Figure 4). First, we discarded two rice lines and two tomato lines in which no DsRED seeds were observed. Based on the expected 3:1 ratio of DsRED fluorescent vs. non-fluorescent seeds for one T-DNA insertion, 12 Arabidopsis, 4 tomato, and 14 rice lines were retained for further analysis (Table 1). It is worth noting that with DsRED-negative selection of segregants, lines with multiple insertions are not necessarily unwelcome. Those lines are usually discarded because stablishing stable transgenic lines need more work and very careful analysis of segregation of the different insertions. However, the use of DsRED counterselection facilitates the identification of non-transgenic seeds despite the very low frequency in which they may be present as a result of multiple insertions (for example, 1:15 in the case of two insertions). This is an advantage for species with low transformation rate or to maximize CRISPR efficiency. Of course, as in any Agrobacterium-mediated transformation method, incomplete transgene copies can be incorporated in some occasions thus absence of any truncated copy should be confirmed in selected mutated lines.
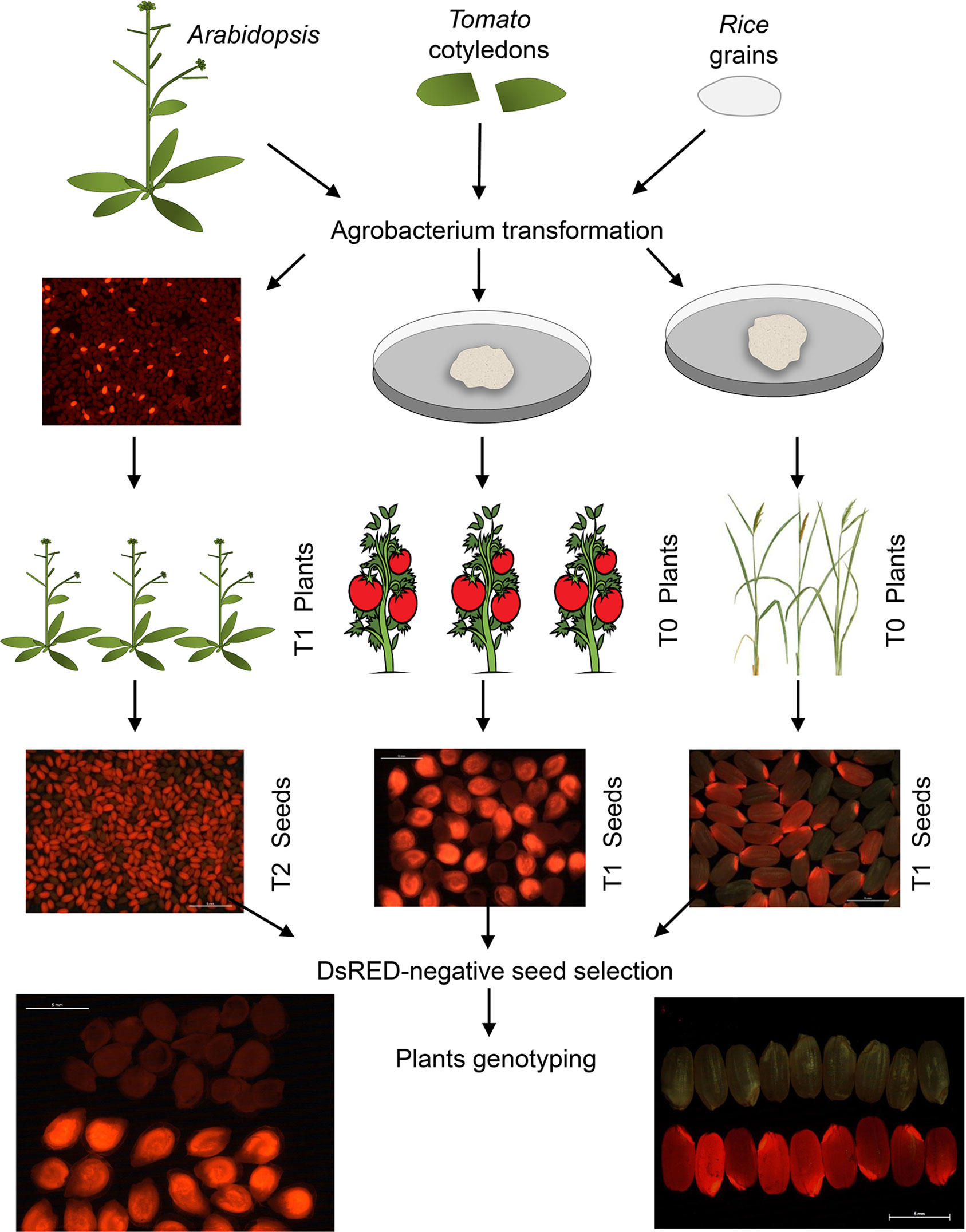
Figure 2 Diagram describing the steps for plant transformation and selection. Transformation of Arabidopsis thaliana was done by floral dip while in vitro transformation was used for Solanum lycopersicum and Oryza sativa. Selection of DsRED T1 seeds of Arabidopsis thaliana and DsRED-negative selection of segregant seeds from the three species was done by direct visualization under a stereoscope equipped with DsRED filter. Detection of fluorescence in seed is very clear, easy, and fast in all three species, and it allowed perfect separation of positive and negative fluorescent seeds.
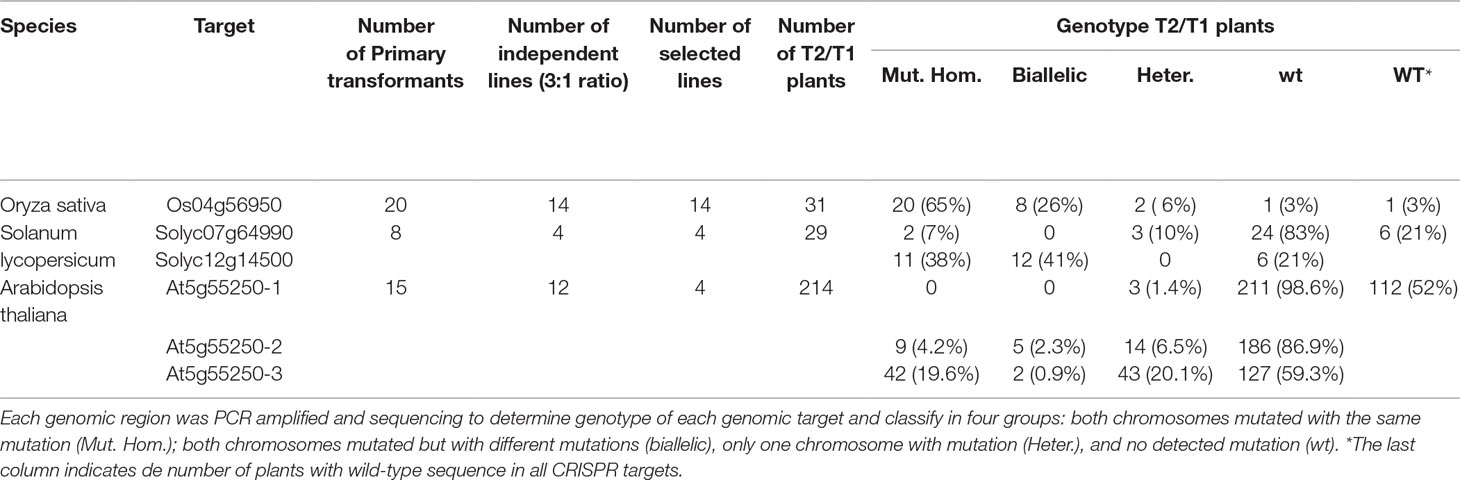
Table 1 Number of independent transgenic lines used for each species and genotyping of transgene-free T2 Plants.
First, we evaluated DsRED as effective transgene marker; thus, we selected seeds with and without DsRED signal from a few lines of each species to germinate and grow them under optimal greenhouse conditions until leaves were available for genomic DNA extraction. We confirmed that the Cas9-specific band was detected by PCR only in the plants from the DsRED-positive seeds (Figure 3A) in the three species. Next, we selected negative DsRED seeds because our main interest is the efficient identification of mutations in transgene-free plants; then, each CRISPR-target genomic region was PCR-amplified and sequenced only from individual plants originated from non-fluorescent seeds. We confirmed the presence of mutations in most of the transgene-free plants from all species (Table 1; Figure 3B; Supplementary Tables 5–10), indicating that stable mutations had been generated in the previous generation and inherited through the germline independently of transgene. Most of the plants presented mutations in heterozygosis, but more importantly, in all species, we did identify plants with mutations in homozygosis.
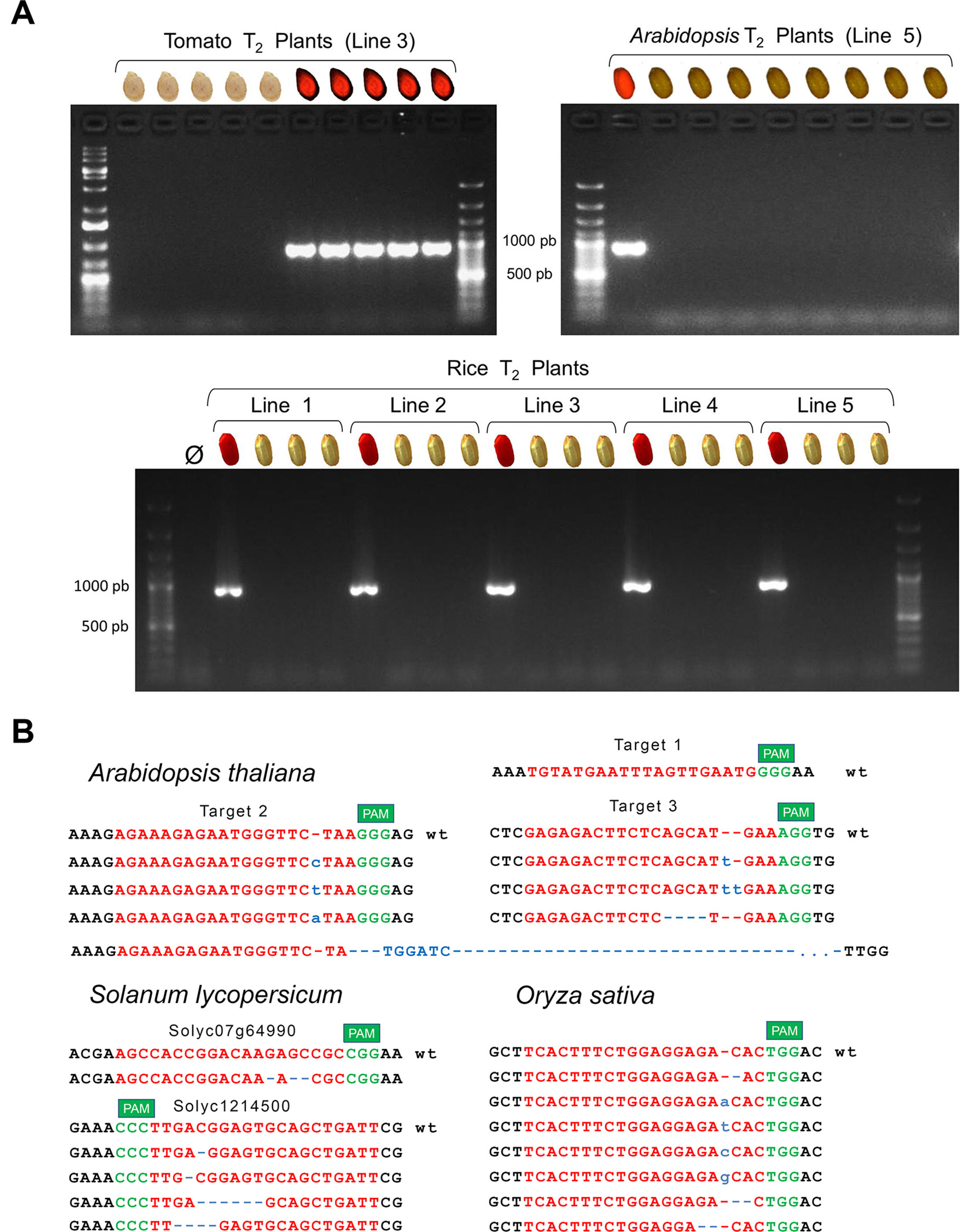
Figure 3 (A) Absence of transgene in plants from DsRED-negative selected seeds was confirmed by PCR using Cas9-specific primers. Examples are shown for tomato, Arabidopsis and rice, selecting different number of seeds and number of independent lines. In all cases, fluorescence and Cas9 PCR band did correlate perfectly (an image indicates the presence/absence of DsRED fluorescence in the original selected seed). (B) Sequence alignment of mutations detected in homozygosis in transgene-free T2 individual plants. CRISPR target sequence in red and PAM sequence in green. Nucleotide insertions are indicated in blue; deletions are also indicated with blue dashes. Deletion “del193” starts in target 2 and ends around 150 nts downstream target 3. It also includes an insertion of six nts that interestingly match with six nucleotides upstream target 2 (Supplementary Figure 5B).
In the case of tomato, where two different genes were targeted at the same time, eight DsRED-negative seeds were selected from each selected line, and the T1-germinated plants on soil were analyzed. We identified four different deletions in gene Solyc12g14500 in homozygosis in plants from three of the four lines; however, we only detected an atypical deletion of three nucleotides in homozygosis in Solyc07g64990 in one of the lines (Figure 3B; Supplementary Table 5). Variability in CRISPR-Cas9 efficiency has been widely described suggesting that accessibility of DNA or sequence composition of target can affect efficiency (Campenhout et al., 2019), but we can not discard a deleterious effect of a null mutant in that gene neither, albeit is not an objective in this work to elucidate it. Only 21% of all T1 plants did not present any mutation (actually, it corresponds to the six plants from line 6, in which no mutations were detected), while all the other T1 plants presented mutations in gene Solyc12g14500 either as biallelic or in homozygosis. Due to the low rate of mutations found in Solyc07g64990 target, most of the plants were wild type for Solyc07g64990 and mutated for Solyc12g14500. Actually, only two plants had both genes edited in homozygosis.
In rice, with 14 independent lines, 3 T1-negative DsRED seeds of each line were selected to be sown on soil. All germinated plants were analyzed, and mutations in target position were detected in plants from all lines (Supplementary Table 6). Only one T1 plant (from line 18) presented the wild-type allele in homozygosis, and we also found it in heterozygosis in two additional T1 plants from line 20. In line 4, the three T1 plants did present the same mutation in homozygosis (insertion of one “A”), suggesting that the corresponding T0 plant likely was already homozygous. We selected five new DsRED-negative seeds from that line, and we confirmed that all plants presented the same “A” insertion in homozygosis. The absence of wild-type allele was also observed in other lines, in which only mutated alleles were detected, both in heterozygosis (biallelic) and homozygosis, suggesting that both copies of the gene were mutated in the corresponding T0 plant. Taken into account all rice T1 plants, most of the plants had a mutation in homozygosis from which we identified three different deletion alleles and the four possible insertions of one nucleotide (Figure 3B; Supplementary Table 6).
In the case of Arabidopsis, we decided to select only four lines but a higher number of T2 plants to evaluate the presence of large deletions, spanning the whole region between two target sites. Between 50 and 80 DsRED-negative seeds were selected and sown on soil, and the germinated T2 plants were analyzed. Different individual mutations in homozygosis in two of the three targets were detected (Figure 3B; Supplementary Tables 7–10), while we only detect one plant with a small deletion (3 pb) in heterozygosis affecting target 1 (Supplementary Table 10). As the three targets are expressed in the same transcript using the strategy of multiplexing with tRNA autoprocessing (Xie et al., 2015), the result indicates a very low efficiency of RNA guide 1. Although the three targets matched the same genomic region, Arabidopsis presented the higher percentage of nonmutated T2 plants in comparison with rice and tomato. A deletion of 193pb affecting targets 2 and 3 was detected in homozygosis in three T2 plants from line 5, in addition to 2 heterozygote plants with the same deletion. A different big deletion (240 pb) was also detected in two plants of line 3 affecting targets 1 and 2, but only in heterozygotes (Supplementary Figure 5A and Supplementary Table 10). Visual inspection of chromatograms was allowed to determine the exact sequence of both deletions (Supplementary Figure 5B). Deletion del193 contains an insertion of six nucleotides that interestingly match six nucleotides upstream target 2. Insertions inside deletions have been previously described in genome editing (Bernabé-Orts et al., 2019), and it can be a result of DNA repairing mechanisms as MMEJ (Mcvey and Lee, 2008).
Taken together, these results are consistent with variable efficiency of sgRNAs reported in the literature, thus confirming that our vectors work as efficiently as other vector systems but with the advantage of using DsRED fluorescence as marker for transgene presence in dry seeds. It is noteworthy to mention that DsRED fluorescence has not decayed in seeds of the three species stored for almost 2 years. The identification of homozygous mutant plants has been successful in all cases despite the use of a low number of transformants. However, the same alleles are shared by all plants derived from the same individual primary transformant, thus exploring more independent lines is expected to be more effective in generating multiple alleles than increasing the number of selected individuals. From our data, the selection of only three seeds from each rice line instead of reducing the number of lines and selecting more T1 seeds did not reduce our efficiency in the number of different alleles found in homozygosis in comparison with tomato. Actually, the same mutations would be identified if only the first three plants of each tomato line are used compared with result from all tomato plants; only deletion of one “C” in homozygosis is missing though it would be detected in biallelic plants. Thus, this strategy could be advisable when the number of plants that can be growth is limited.
We have demonstrated that the use of DsRED fluorescence as a selectable marker of transgene in dry seeds is a robust method that facilitates the identification of transgene-free CRISPR-/Cas9-edited plants of rice and tomato in the second generation, minimizing the probability of off-target mutations. Seed DsRED fluorescence selection works in A. thaliana related species as Camelina sativa (Morineau et al., 2017) or Cardamine hirsuta (unpublished own data). Due to possible interference because of autofluorescence in plant tissues, different fluorescent protein must be evaluated to adapt vectors to different species with agronomical interest. We have shown that DsRED is a very good option for rice and tomato and probably for many species, as shown by a recent report with wheat (Okada et al., 2019). A limitation in many vector systems is the laborious task of adapting them to new species, changing promoters and transcriptional units. GoldenBraid cloning system ensures easy modification of vectors for other Cas proteins, like Cas12a/Cpf1 (Bernabé-Orts et al., 2019), other fluorescence proteins, and required resistance genes, meaning that generation of new vectors for in vitro transformation is not a limiting factor. This approach can easily be extended to additional crops and model plants, as long as optimal promoters are available.
Data Availability
All datasets for this study are included in the manuscript and the Supplementary Files.
Author Contributions
EM, MB and DA contributed conception and design of the study; NA-F and EM designed and generated the Golden Braid vectors and transformed Arabidopsis thaliana; SP and AG transformed tomato; CZ, AKS and AS transformed rice; EM and NA-F analysed T2 lines; EM and MB wrote the first draft of the manuscript; AS, AG, DA, CZ and NA-F contributed to manuscript revision. All authors read and approved the submitted version.
Funding
Grants AGL2014-57200-JIN (EGM), BFU2016-80621-P (MB), and BIO2016-78601-R (AG) from the current Spanish Ministry of Science, Innovation and Universities. Grants TRADITOM (634561), TomGEM (679796), Newcotiana (760331-2) and Pharma-Factory (SEP-210417525) from European Union H2020 program (AG). ERC grant “SUMOrice” and BBSRC grant “Flooding tolerance in rice” (AS).
Conflict of Interest Statement
The authors declare that the research was conducted in the absence of any commercial or financial relationships that could be construed as a potential conflict of interest.
Acknowledgments
We thank Diego Orzaez and Marta Vazquez for providing the material from the Golden Braid vector collection.
Supplementary Material
The Supplementary Material for this article can be found online at: https://www.frontiersin.org/articles/10.3389/fpls.2019.01150/full#supplementary-material
References
Abbas, M., Hernandez-Garcia, J., Blanco-Tourinan, N., Aliaga, N., Minguet, E. G., Alabadi, D., et al. (2018a). Reduction of indole-3-acetic acid methyltransferase activity compensates for high-temperature male sterility in Arabidopsis. Plant Biotechnol. J. 16, 272–279. doi: 10.1111/pbi.12768
Abbas, M., Hernandez-Garcia, J., Pollmann, S., Samodelov, S. L., Kolb, M., Friml, J., et al. (2018b). Auxin methylation is required for differential growth in Arabidopsis. Proc. Natl. Acad. Sci. U. S. A. 115, 6864–6869. doi: 10.1073/pnas.1806565115
Bechtold, N., Pelletier, G. (1998). In planta Agrobacterium-mediated transformation of adult Arabidopsis thaliana plants by vacuum infiltration. Methods Mol. Biol. 82, 259–266. doi: 10.1385/0-89603-391-0:259
Bernabé-Orts, J. M., Casas-Rodrigo, I., Minguet, E. G., Landolfi, V., Garcia-Carpintero, V., Gianoglio, S., et al. (2019). Assessment of Cas12a-mediated gene editing efficiency in plants. Plant Biotechnol. J. doi: 10.1111/pbi.13113
Bolotin, A., Quinquis, B., Sorokin, A., Ehrlich, S. D. (2005). Clustered regularly interspaced short palindrome repeats (CRISPRs) have spacers of extrachromosomal origin. Microbiology 151, 2551–2561. doi: 10.1099/mic.0.28048-0
Brinkman, E. K., Chen, T., Amendola, M., Van steensel, B. (2014). Easy quantitative assessment of genome editing by sequence trace decomposition. Nucleic Acids Res. 42, e168–e168. doi: 10.1093/nar/gku936
Busov, V. B., Brunner, A. M., Meilan, R., Filichkin, S., Ganio, L., Gandhi, S., et al. (2005). Genetic transformation: a powerful tool for dissection of adaptive traits in trees. New Phytologist. 167, 9–18. doi: 10.1111/j.1469-8137.2005.01412.x
Campenhout, C. V., Cabochette, P., Veillard, A.-C., Laczik, M., Zelisko-Schmidt, A., Sabatel, C., et al. (2019). Guidelines for optimized gene knockout using CRISPR/Cas9. BioTechniques 0, null. doi: 10.2144/btn-2018-0187
Clough, S. J., Bent, A. F. (1998). Floral dip: a simplified method for Agrobacterium-mediated transformation of Arabidopsis thaliana. Plant J. 16, 735–743. doi: 10.1046/j.1365-313x.1998.00343.x
Darbani, B., Eimanifar, A., Stewart, C. N., Jr., Camargo, W. N. (2007). Methods to produce marker-free transgenic plants. Biotechnol. J. 2, 83–90. doi: 10.1002/biot.200600182
Durr, J., Papareddy, R., Nakajima, K., Gutierrez-Marcos, J. (2018). Highly efficient heritable targeted deletions of gene clusters and non-coding regulatory regions in Arabidopsis using CRISPR/Cas9. Sci. Rep. 8, 4443. doi: 10.1038/s41598-018-22667-1
Edwards, K., Johnstone, C., Thompson, C. (1991). A simple and rapid method for the preparation of plant genomic DNA for PCR analysis. Nucleic Acids Res. 19, 1349. doi: 10.1093/nar/19.6.1349
Ellul, P., Garcia-Sogo, B., Pineda, B., Ríos, G., Roig, L., Moreno, V. (2003). The ploidy level of transgenic plants in Agrobacterium-mediated transformation of tomato cotyledons (Lycopersicon esculentum L.Mill.) is genotype and procedure dependent. Theor. Appl. Genet. 106, 231–238. doi: 10.1007/s00122-002-0928-y
Feng, Z., Zhang, B., Ding, W., Liu, X., Yang, D. L., Wei, P., et al. (2013). Efficient genome editing in plants using a CRISPR/Cas system. Cell Res. 23, 1229–1232. doi: 10.1038/cr.2013.114
Fernandez-Pozo, N., Menda, N., Edwards, J. D., Saha, S., Tecle, I. Y., Strickler, S. R., et al. (2015). The Sol Genomics Network (SGN)—from genotype to phenotype to breeding. Nucleic Acids Res. 43, D1036–D1041. doi: 10.1093/nar/gku1195
Gao, X., Chen, J., Dai, X., Zhang, D., Zhao, Y. (2016). An effective strategy for reliably isolating heritable and Cas9-free arabidopsis mutants generated by CRISPR/Cas9-mediated genome editing. Plant Physiol. 171, 1794–1800. doi: 10.1104/pp.16.00663
Hiei, Y., Komari, T. (2008). Agrobacterium-mediated transformation of rice using immature embryos or calli induced from mature seed. Nat. Protoc. 3, 824–834. doi: 10.1038/nprot.2008.46
Jiang, W., Zhou, H., Bi, H., Fromm, M., Yang, B., Weeks, D. P. (2013). Demonstration of CRISPR/Cas9/sgRNA-mediated targeted gene modification in Arabidopsis, tobacco, sorghum and rice. Nucleic Acids Res. 41, e188. doi: 10.1093/nar/gkt780
Jinek, M., Chylinski, K., Fonfara, I., Hauer, M., Doudna, J. A., Charpentier, E. (2012). A programmable dual-RNA–guided DNA endonuclease in adaptive bacterial immunity. Science 337, 816–821. doi: 10.1126/science.1225829
Kroj, T., Savino, G., Valon, C., Giraudat, J., Parcy, F. (2003). Regulation of storage protein gene expression in Arabidopsis. Development 130, 6065–6073. doi: 10.1242/dev.00814
Larkin, M. A., Blackshields, G., Brown, N. P., Chenna, R., Mcgettigan, P. A., Mcwilliam, H., et al. (2007). Clustal W and Clustal X version 2.0. Bioinformatics 23, 2947–2948. doi: 10.1093/bioinformatics/btm404
Mcvey, M., Lee, S. E. (2008). MMEJ repair of double-strand breaks (director’s cut): deleted sequences and alternative endings. Trends Genet. 24, 529–538. doi: 10.1016/j.tig.2008.08.007
Miki, B., Mchugh, S. (2004). Selectable marker genes in transgenic plants: applications, alternatives and biosafety. J. Biotechnol. 107, 193–232. doi: 10.1016/j.jbiotec.2003.10.011
Mojica, F. J. M., Díez-Villaseñor, C. S., García-Martínez, J., Soria, E. (2005). Intervening Sequences of regularly spaced prokaryotic repeats derive from foreign genetic elements. J. Mol. Evol. 60, 174–182. doi: 10.1007/s00239-004-0046-3
Morineau, C., Bellec, Y., Tellier, F., Gissot, L., Kelemen, Z., Nogué, F., et al. (2017). Selective gene dosage by CRISPR-Cas9 genome editing in hexaploid Camelina sativa. Plant Biotechnol. J. 15, 729–739. doi: 10.1111/pbi.12671
Murray, M. G., Thompson, W. F. (1980). Rapid isolation of high molecular weight plant DNA. Nucleic Acids Res. 8, 4321–4325. doi: 10.1093/nar/8.19.4321
Okada, A., Arndell, T., Borisjuk, N., Sharma, N., Watson-Haigh, N. S., Tucker, E. J., et al. (2019). CRISPR/Cas9-mediated knockout of Ms1 enables the rapid generation of male-sterile hexaploid wheat lines for use in hybrid seed production. Plant Biotechnol. J. doi: 10.1111/pbi.13106
Qin, G., Gu, H., Zhao, Y., Ma, Z., Shi, G., Yang, Y., et al. (2005). An indole-3-acetic acid carboxyl methyltransferase regulates Arabidopsis leaf development. Plant Cell 17, 2693–2704. doi: 10.1105/tpc.105.034959
Ravi, M., Marimuthu, M. P., Tan, E. H., Maheshwari, S., Henry, I. M., Marin-Rodriguez, B., et al. (2014). A haploid genetics toolbox for Arabidopsis thaliana. Nat. Commun. 5, 5334. doi: 10.1038/ncomms6334
Sarrion-Perdigones, A., Palaci, J., Granell, A., Orzaez, D. (2014). Design and construction of multigenic constructs for plant biotechnology using the GoldenBraid cloning strategy. Methods Mol. Biol. 1116, 133–151. doi: 10.1007/978-1-62703-764-8_10
Sarrion-Perdigones, A., Vazquez-Vilar, M., Palaci, J., Castelijns, B., Forment, J., Ziarsolo, P., et al. (2013). GoldenBraid 2.0: a comprehensive DNA assembly framework for plant synthetic biology. Plant Physiol. 162, 1618–1631. doi: 10.1104/pp.113.217661
Shimada, T. L., Shimada, T., Hara-Nishimura, I. (2010). A rapid and non-destructive screenable marker, FAST, for identifying transformed seeds of Arabidopsis thaliana. Plant J. 61, 519–528. doi: 10.1111/j.1365-313X.2009.04060.x
Stuitje, A. R., Verbree, E. C., Van Der Linden, K. H., Mietkiewska, E. M., Nap, J. P., Kneppers, T. J. (2003). Seed-expressed fluorescent proteins as versatile tools for easy (co)transformation and high-throughput functional genomics in Arabidopsis. Plant Biotechnol. J. 1, 301–309. doi: 10.1046/j.1467-7652.2003.00028.x
Toki, S., Hara, N., Ono, K., Onodera, H., Tagiri, A., Oka, S., et al. (2006). Early infection of scutellum tissue with Agrobacterium allows high-speed transformation of rice. Plant J. 47, 969–976. doi: 10.1111/j.1365-313X.2006.02836.x
Vazquez-Vilar, M., Bernabé-Orts, J. M., Fernandez-Del-Carmen, A., Ziarsolo, P., Blanca, J., Granell, A., et al. (2016). A modular toolbox for gRNA–Cas9 genome engineering in plants based on the GoldenBraid standard. Plant Methods 12, 10. doi: 10.1186/s13007-016-0101-2
Vazquez-Vilar, M., Sarrion-Perdigones, A., Ziarsolo, P., Blanca, J., Granell, A., Orzaez, D. (2015). Software-assisted stacking of gene modules using GoldenBraid 2.0 DNA-assembly framework. Methods Mol. Biol. 1284, 399–420. doi: 10.1007/978-1-4939-2444-8_20
Wiedenheft, B., Sternberg, S. H., Doudna, J. A. (2012). RNA-guided genetic silencing systems in bacteria and archaea. Nature 482, 331–338. doi: 10.1038/nature10886
Wu, R., Lucke, M., Jang, Y.-T., Zhu, W., Symeonidi, E., Wang, C., et al. (2018). An efficient CRISPR vector toolbox for engineering large deletions in Arabidopsis thaliana. Plant Methods 14, 65–65. doi: 10.1186/s13007-018-0330-7
Xie, K., Minkenberg, B., Yang, Y. (2015). Boosting CRISPR/Cas9 multiplex editing capability with the endogenous tRNA-processing system. Proc. Natl. Acad. Sci. 112, 3570–3575. doi: 10.1073/pnas.1420294112
Yang, Y., Xu, R., Ma, C. J., Vlot, A. C., Klessig, D. F., Pichersky, E. (2008). Inactive methyl indole-3-acetic acid ester can be hydrolyzed and activated by several esterases belonging to the AtMES esterase family of Arabidopsis. Plant Physiol. 147, 1034–1045. doi: 10.1104/pp.108.118224
Yau, Y.-Y., Stewart, C. N., Jr. (2013). Less is more: strategies to remove marker genes from transgenic plants. BMC Biotechnology 13, 36–36. doi: 10.1186/1472-6750-13-36
Keywords: CRISPR/Cas9, genome editing, DsRED, Solanum lycopersicum, Oryza sativa, Arabidopsis thaliana
Citation: Aliaga-Franco N, Zhang C, Presa S, Srivastava AK, Granell A, Alabadí D, Sadanandom A, Blázquez MA and Minguet EG (2019) Identification of Transgene-Free CRISPR-Edited Plants of Rice, Tomato, and Arabidopsis by Monitoring DsRED Fluorescence in Dry Seeds. Front. Plant Sci. 10:1150. doi: 10.3389/fpls.2019.01150
Received: 10 July 2019; Accepted: 23 August 2019;
Published: 18 September 2019.
Edited by:
Andrea Chini, Centro Nacional de Biotecnología (CNB), SpainReviewed by:
Jimmy R. Botella, University of Queensland, AustraliaLaurens Pauwels, Flanders Institute for Biotechnology, Belgium
Copyright © 2019 Aliaga-Franco, Zhang, Presa, Srivastava, Granell, Alabadí, Sadanandom, Blázquez and Minguet. This is an open-access article distributed under the terms of the Creative Commons Attribution License (CC BY). The use, distribution or reproduction in other forums is permitted, provided the original author(s) and the copyright owner(s) are credited and that the original publication in this journal is cited, in accordance with accepted academic practice. No use, distribution or reproduction is permitted which does not comply with these terms.
*Correspondence: Eugenio G. Minguet, ZWdvbWV6bUBpYm1jcC51cHYuZXM=