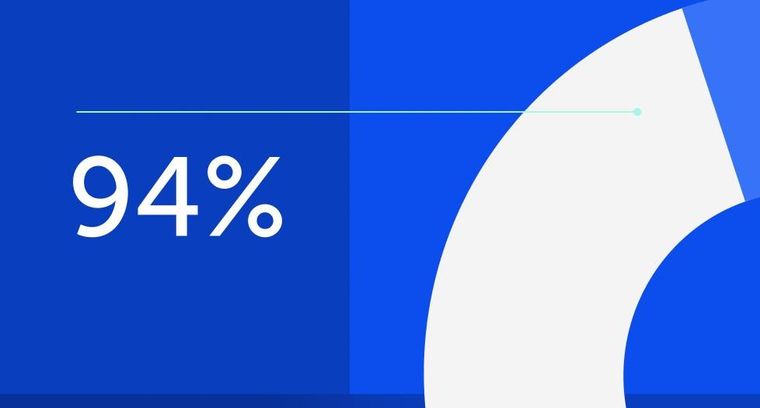
94% of researchers rate our articles as excellent or good
Learn more about the work of our research integrity team to safeguard the quality of each article we publish.
Find out more
MINI REVIEW article
Front. Plant Sci., 26 July 2019
Sec. Plant Physiology
Volume 10 - 2019 | https://doi.org/10.3389/fpls.2019.00960
This article is part of the Research TopicNAD Metabolism and Signaling in PlantsView all 7 articles
Upon illumination, photosystem I in chloroplasts catalyzes light-driven electron transport from plastocyanin to ferredoxin, followed by the reduction of NADP+ to NADPH by ferredoxin:NADP+ reductase for CO2 fixation. At the beginning of photosynthesis, NADP+ supply control is dominated by de novo NADP+ synthesis rather than being recycled from the Calvin cycle. Importantly, ferredoxin distributes electrons to NADP+ as well as to thioredoxins for light-dependent regulatory mechanisms, to cyclic electron flow for more adenosine triphosphate (ATP) production, and to several metabolites for reductive reactions. We previously demonstrated that the NADP+ synthesis activity and the amount of the NADP pool size, namely the sum of NADP+ and NADPH, varies depending on the light conditions and the ferredoxin-thioredoxin system. In addition, the regulatory mechanism of cytoplasmic NAD+ supply is also involved in the chloroplastic NADP+ supply control because NAD+ is an essential precursor for NADP+ synthesis. In this mini-review, we summarize the most recent advances on our understanding of the regulatory mechanisms of NADP+ production, focusing on the interactions, crosstalk, and co-regulation between chloroplasts and the cytoplasm at the level of NAD+ metabolism and molecular transport.
Nicotinamide adenine dinucleotide (NAD) and its phosphorylated form (NADP) are essential electron acceptors/donors in a broad range of cellular redox processes (Pollak et al., 2007). Interestingly, these two cofactors have rather distinct biological roles. NAD is mainly used in catabolic processes to produce cellular energy as an oxidant (Geigenberger, 2003), whereas NADP is often involved in anabolic processes to produce photosynthates, fatty acids, and carbon skeletons to support plant growth as a reductant (Kramer et al., 2004; Noctor et al., 2006). The major NADPH-generating source in darkness is the oxidative pentose phosphate pathway (OPPP) coupled to central carbon metabolism in chloroplasts (Kruger and von Schaewen, 2003). Redox regulation of OPPP enzymes in chloroplasts relies on thioredoxin (Trx) and NADPH-dependent Trx reductase C (NTRC) (Perez-Ruiz et al., 2017), thereby balancing the redox status for protection against oxidative damage (Perez-Ruiz et al., 2006).
Under sunlight, photosynthetic electron transfer chains (PETC) are the primary source of NADPH. Plants use sunlight as a primary energy source for photosynthesis in chloroplasts (Ort and Yocum, 1996). During this process, light drives electron transfer reactions by which protons are transferred from the stroma into the thylakoid lumen generating proton motive force (pmf) that is used for ATP synthesis (Kanazawa et al., 2017). Most pmf appears to be generated though linear electron flow (LEF) in which electrons released from water in photosystem II (PSII) are eventually transferred to NADP+ through photosystem I (PSI; Figure 1, red line; Joliot and Joliot, 2005). Thus, photosynthesis provides NADPH as reducing power for the Calvin-Benson cycle (CBC) to assimilate carbon dioxide. After use of the reducing power in CBC, NADP+ is again recycled back as an electron acceptor in PSI. However, under stress conditions that weaken CBC enzymatic activity, a declining rate of NADPH usage and NADP recycling occurs and LEF can be overloaded resulting in generation of reactive oxygen species from the photosystems (Hajiboland, 2014; Foyer, 2018). Hence, a number of studies have elucidated the protective mechanisms, including antioxidant production, antenna size regulation, and alternative electron flow, as regulatory mechanisms of photosynthesis under natural environments (Demmig-Adams and Adams, 1992; Takahashi and Badger, 2011; Pinnola and Bassi, 2018). For example, the “malate valve” is a representative redox shuttle system of the balancing redox state in chloroplasts and exclusively exports the excess reducing power of NADPH in chloroplasts to NAD+ in cytosol (Selinski and Scheibe, 2019), by which NADP+ could be replenished to PSI instead of CBC. Thus, NADP+ re-supply is crucial for the redox balancing system in chloroplasts. Here, a simple question arises: why is NADP+ additionally provided for LEF via de novo NADP+ synthesis in chloroplasts when or before it starves? To answer this, we need to understand the uncharacterized regulatory mechanisms of NADP+ synthesis in chloroplasts.
Figure 1. Regulation of NADP pool size in photosynthesis. Schematic representation of the relationship between NADP pool size regulation and photosynthetic electron flow was shown. Red arrow indicates linear electron flow (LEF), and blue arrow indicates cyclic electron flow. Black arrow indicates molecular conversion. PSI, photosystem I; PSII, photosystem II; cytb6f, cytochrome b6f complex; PQH2, plastoquinol; PC, plastocyanin; PGR5, proton gradient regulation 5; PGRL1, PGR5-like 1; NDH, chloroplast NAD(P)H dehydrogenase-like complex.
NAD+ is the only promising candidate to be the precursor for NADP+ synthesis and is converted to NADP+ by ATP-dependent NAD kinase (NADK) (McGuinness and Butler, 1985). Although NAD is exclusively produced in the cytosol (Hashida et al., 2009), NADP production is executed at on demand sites by various isoforms of NADK. For example in Arabidopsis, NADK1 is located in the cytosol, whereas NADK2 and NADK3 are targeted to chloroplasts and peroxisomes, respectively (Waller et al., 2009). Moreover, NADK1 and NADK2 use NAD+ as the preferred substrate, whereas NADK3 demonstrates a strong preference for NADH (Berrin et al., 2005; Chai et al., 2005, 2006). For decades, the Ca2+/Calmodulin (CaM)-dependence of NADK activity has been recognized (Muto and Miyachi, 1977; Anderson et al., 1980; Karita et al., 2004) and NADK2 was reported capable of binding to CaM in vitro (Turner et al., 2004). However, no candidate CaM and no response to Ca2+ in NADK2 activity have been reported elsewhere (Dell’Aglio et al., 2016). Instead of NADK2, it was recently reported that Arabidopsis P-loop ATPase has CaM-dependent NADK activity (Dell’Aglio et al., 2019). Rather than Ca2+, the light signal appears to control chloroplastic NADK2 activity and NADP+ production according to the current knowledge of NADP response to light conditions (Thormahlen et al., 2017). The goal of this mini-review is to highlight the importance of regulating NAD+ supply for chloroplastic NADP+ synthesis that is specific to photosynthetic organisms.
According to the rationale of photosynthesis, light drives NADP+ reduction, meaning NADPH generation. Most typical illustrations concerning photosynthesis demonstrate the qualitative interpretation of the photochemical process but do not give any quantitative interpretations about NADP (Figure 1). Under steady-state exposure conditions, the balance between the generation and utilization of reducing power is equilibrated by complicated interactions between LEF, CBC, redox shuttle, and cyclic electron flow (CEF), which exclusively generate pmf contributing to ATP production (Munekage et al., 2004; Alric and Johnson, 2017), such that the NADPH/NADP+ ratio can remain stable. In other words, generated NADPH via PETC is immediately consumed by CBC and/or redox shuttle and recycled back to the photosystem as NADP+. However, photosynthesis does not occur in darkness in nature, and constant fluctuation of light intensity occurs during the day because of changes in radiation angles, cloud cover, and shade by leaf overlap in natural environments. Here, we speculate that the redox state of the NADP pool might vary in response to fluctuating light conditions and that the NADPH/NADP+ ratio might be at its peak because of massive NADPH generation at very high-light intensities. Similarly, we might misbelieve that NADP+ exclusively accumulates in the chloroplasts at night because there is no light that drives PETC to generate NADPH. However, in addition to NADPH, NADP+ also displays basal levels after dark acclimation for at least 30–60 min (Thormahlen et al., 2017; Hashida et al., 2018). In darkness, the redox status of NADP would be balanced by OPPP enzyme activity mediated via NTRC-dependent redox regulation (Michalska et al., 2009). At the timing of re-exposure to light, NADP+ increases before NADPH because NADP+ is indispensable as an electron acceptor for LEF execution. As a result, the sum of NADP+ and NADPH, here designated as the NADP pool, is dynamically adjusted to fluctuating light conditions under natural environments. These dynamics shed light on the importance of chloroplastic NADP homeostasis, including NADP pool size regulation, as well as redox regulation, in the research field of photosynthesis. Undoubtedly, the regulatory mechanism of chloroplastic NADP pool size depends on the balance between decreased and increased flow, rather than the redox interconversion between NADP+ and NADPH (Figure 2).
Figure 2. Inter-organelle nicotinamide adenine dinucleotide (NAD) metabolism associated with chloroplastic NADP dynamics. Schematic representation of NADP+ biosynthesis and NAD+ biosynthesis and part of their metabolism and inter-organelle transport. Solid arrow indicates the identified molecular conversion, and dashed arrow indicates unidentified molecular conversion. Green line highlights the de novo pathway, and blue line highlights the salvage pathway of NAD+ biosynthesis. Red line highlights the light activation pathway. Abbreviations are as shown in the text except for Nam, MeNa, and NMN indicating nicotinamide, methyl nicotinate, and nicotinamide mononucleotide, respectively.
NADP metabolism, but not redox interconversion, contributes to a decrease in the NADP pool size. For example, NADP+ dephosphorylation results in NAD+ generation that can be supplied for the re-synthesis of NADP+ and is possibly a convenient strategy for the temporal sequestration of electron acceptors from photochemistry. Some research groups have reported NADP+ dephosphorylation activity in plants (Gallais et al., 2000; Hunt and Gray, 2009), but no NADP+ dephosphorylation activity has been shown at present in isolated chloroplasts. Deamidation converts NADP to deamide-NADP, or the so-called nicotinate adenine dinucleotide phosphate (NaADP) (Chini and Dousa, 1995). At the final step of NAD synthesis, nicotinate adenine dinucleotide (NaAD) serves in the deamidation reaction by ATP-dependent NAD synthetase (Hashida et al., 2009, 2016) so that NaADP might serve as an alternative precursor for NADP synthesis. However, NaADP molecules have never been reported in chloroplasts, and neither has NADP deamidation activity nor NaADP amidation activity been reported in chloroplasts or total leaf extracts. Nonetheless, these temporal NADP decreasing flows are attractive because they enable the re-supply of NADP for photochemistry in 1-step enzymatic reactions. On the contrary, it is known that nucleotide diphosphate linked to X hydrolase (NUDX) plays a significant role in NADP decrease because it cleaves the pyrophosphate bridge in the NAD skeleton (Yoshimura and Shigeoka, 2014). In Arabidopsis, AtNUDX19 aggressively participates in regulating the NADPH level as an alternative to dissipating excess reducing equivalents under intense light conditions (Maruta et al., 2016). Unlike dephosphorylation and deamidation of NADP, NUDX definitely consumes the NADP molecule, meaning that the NAD+ supply is indispensable for NADP+ re-synthesis as a photosynthetic electron acceptor in chloroplasts. Therefore, under high-light conditions, NADP+ has to be constitutively provided to complement and maintain the NADP pool size. The fate of NADPH consumed by NUDX under high-light conditions and decreased NADP+ under dark conditions has not been fully determined (Figure 2, dashed lines).
Based on enzymatic property, NADP+ synthesis requires the substrates NAD+ and ATP besides NADK activity (McGuinness and Butler, 1985). If one of them is lacking, NADP+ supply is arrested and quiescent NADP+ synthesis spontaneously decreases the pool size when the NADP pool size is determined under dynamic equilibrium. Hence, the NADP increasing flow is strictly controlled by at least three factors in chloroplasts: (1) regulation of NADK activity, (2) ATP supply, and (3) NAD+ supply.
Notably, light-excited electrons were mainly distributed from ferredoxin (Fd) to downstream electron acceptors in two aspects, as reducing power in metabolic reactions and as redox regulator in signal transductions. Besides the reduction of NADP+ by Fd-dependent NADP+ reductase in LEF (Figure 1, red lines), electrons are used as reducing power in the nitrate assimilation pathway and various metabolic reactions by Fd-dependent enzymes (Hanke and Mulo, 2013). In the redox signaling network, electrons could be handed over from Fd and NADPH to thioredoxin (Trx) as reducing equivalents for the downstream signaling cascade by Fd/Trx reductase and NTRC (Nikkanen and Rintamaki, 2019). Otherwise, electrons are transferred back to the plastoquinone pool by CEF (Figure 1, blue lines; Alric and Johnson, 2017). Thus, starvation of NADP+ as an electron acceptor results in the induction of redox regulatory flow or cyclic flow. It has recently been reported that light exposure is required for the activation of NADK2 in chloroplasts and that Fd/Trx redox signaling is directly or indirectly involved in the regulation of NADK2 activity (Hashida et al., 2018), though the regulatory mechanisms remain to be elucidated. Unlike NADK1 in cytosol and NADK3 in the peroxisome, NADK2 in chloroplasts has a long N-terminal extension, which is conserved throughout green plants (Viridiplantae, excluding glaucophytes and rhodophytes) (Li et al., 2014), but the extended amino acid sequence is originally unnecessary for enzymatic activity. However, it is still unknown whether the extension is involved in regulating NADK2 activity.
CEF operates at the maximum after dark acclimation, owing to the partial inactivation of CBC (Joliot and Johnson, 2011). As NADP+ shortage caused by lack of NADK2 activation results in accumulation of reduced Fd, it contributes to CEF efficiency (Breyton et al., 2006; Okegawa et al., 2008). Then, the CEF efficiency decreases shortly after light exposure (Joliot and Joliot, 2005), implying that ATP synthesis could be provided by CEF at the beginning of light perception for NADP+ synthesis. Therefore, CEF operation seems to be important to NADP+ supply and the onset of LEF, leading to light acclimation. In fact, impairment of the CEF pathway reduced the photosynthetic rate under fluctuating light conditions, causing a decrease in plant biomass (Yamori et al., 2016), possibly owing to the delay in light acclimation, namely a delay in LEF establishment. In brief, the photochemical electron transfer network is profoundly associated with NADP+ synthesis in chloroplasts.
NADP+ biosynthesis in chloroplasts is activated in the presence of sufficient ATP and NAD+ under light conditions. Where does NAD+ come from? In response to light, the chloroplastic NADP pool size doubles within 1 h by consuming an equivalent amount of NAD+ in chloroplasts (Hashida et al., 2018). Currently, the NAD pool size (sum of NAD+ and NADH) in chloroplasts is still under estimation but the sum of cellular NAD and NADP pool could increase in response to light. According to previous reports, promotion of chloroplastic NADP synthesis by NADK2 overexpression did not significantly decrease the cellular NAD pool and in contrast, enhancement of NAD biosynthesis increased the cellular NADP pool (Takahashi et al., 2009; Hashida et al., 2010). Thus, the increase in NADP is accompanied by an increase in the total NAD and NADP pool sizes. The total pool increase indicates activation of NAD+ biosynthesis. This activation could be stimulated by chloroplastic NADP+ synthesis accompanied by NAD+ uptake into chloroplasts (Figure 2). As NAD biosynthesis exclusively occurs outside of chloroplasts (Hashida et al., 2010; Di Martino and Pallotta, 2011), the cytoplasmic NAD pool could decrease by the uptake. Therefore, the mechanism of NAD+ supply underpins the light responsive NADP increase for LEF in photosynthesis, and chloroplastic NAD+ transportation is a crucial pathway for light adaptation in terrestrial plants (Hashida et al., 2010, 2016; Vialet-Chabrand et al., 2017). However, chloroplasts have been less investigated with respect to NAD+ transport compared to mitochondria and peroxisomes (Palmieri et al., 2008; Agrimi et al., 2012). In Arabidopsis, NDT1 encodes a carrier protein that is capable of transporting NAD+ and targeting it to the inner membrane of chloroplasts (Todisco et al., 2006; Palmieri et al., 2009). At present, the physiological functions in chloroplastic NAD+ transportation are largely unknown. Surprisingly, a mutant lacking chloroplastic NADK activity, nadk2, can survive under light and conditionally grow to a size comparable to wild type, albeit having a pale green leaf (Chai et al., 2005; Takahashi et al., 2009), implying a possible complement of NADP+ to chloroplasts from cytosol in the nadk2 mutant. However, neither NDT1 nor NDT2 transports NADP+ or NADPH according to biochemical experiments (Palmieri et al., 2009). Otherwise, NAD+ in chloroplasts may act as an inefficient but valuable alternative for NADP+ in photosynthetic electron transport. Because, nadk2 showed a larger NAD pool size than the wild type (Takahashi et al., 2006), light-driven chloroplastic NAD+ transport could be promoted and the chloroplastic NAD pool might cover the increase.
NAD+ also has a significant function in photosynthesis through regulation of various CBC enzymes; for example, via the assembly of the protein complex glyceraldehyde-3-phosphate dehydrogenase and phosphoribulokinase mediated by CP12 protein (Wedel et al., 1997). Therefore, chloroplasts contain considerable amounts of NAD+ and chloroplastic NAD+ transportation is not just for NADP+ synthesis. Nevertheless, the basal level of NAD+ cannot be accounted for by the increased NADP+ level in response to light, suggesting that NAD+ biosynthesis is also required for light acclimation (Figure 2, orange lines and captions). In the final two steps of NAD+ biosynthesis, nicotinate mononucleotide (NaMN) is transformed via adenylation to nicotinate adenine dinucleotide (NaAD), followed by amidation of NaAD to NAD+. However, enhancement of enzymatic activity in these steps does not particularly contribute to the NAD pool size (Hashida et al., 2010, 2016). Here, NaMN is of particular importance as an intermediate, which is a product of two independent pathways, the de novo and the salvage pathway. In heterologous systems, quinolinate (Qa) application with E. coli nadC gene encoding Qa phosphoribosyl transferase (QPT) successively increases the NAD pool size (Petriacq et al., 2012). Therefore, the supply of NaMN is a key regulator of cellular NAD pool size. In brief, the light responsive NADP increase in chloroplasts will provoke NaMN supply for NAD+ production. According to current knowledge, de novo synthesis of NaMN is executed in chloroplasts, and the generated NaMN is exported to cytosol and salvage synthesis of NaMN from nicotinate is conducted in the cytosol (Katoh et al., 2006). Therefore, the de novo pathway rather than the salvage pathway has better linkage to chloroplastic events (Figure 2, green and red lines).
According to a recent report, L-Asp oxidase (LASPO), which is the first enzyme of de novo NAD+ biosynthesis and converts L-Asp to imino-Asp, has intriguing biochemical properties associated with the light/dark condition (Hao et al., 2018). LASPO activity may be inhibited at night and stimulated by light exposure, as well as NADK2. Moreover, NADP+ can be a competitive inhibitor for LASPO, suggesting that an early step of the de novo NAD+ biosynthesis is orchestrated by chloroplastic NADP+ status. As the next step of de novo NAD+ biosynthesis, Qa synthase (QS) produces Qa from imino-Asp. Interestingly, QS protein harbors the cys desulfurase domain that stimulates reconstitution of the oxygen-sensitive Fe-S cluster and QS as part of the cys desulfurase complex (Murthy et al., 2007; Schippers et al., 2008). Hence, QS may monitor chloroplastic redox status in a Cys desulfurase complex and a reductive environment could stimulate QS activity. In chloroplasts, a Fe-S cluster and assembly are involved in the redox regulatory network downstream of Fd in PSI photochemistry (Van Hoewyk et al., 2007). Because the Fd/thioredoxin-m redox regulatory pathway negatively regulates NADP+ synthesis (Hashida et al., 2018), these opposite regulations may coordinate the precursor supply for de novo NAD+ biosynthesis that is required for NADP+ synthesis to save excess energy (ATP) consumption. Further, the above-mentioned heterologous system uses cytoplasmic ATP for NaMN synthesis. Therefore, as is the case of NADK in chloroplasts, ATP supply could be a rate-limiting factor for QPT activity. Although NaMN needs to be exported to the cytoplasm from chloroplasts for the synthesis of NAD+, the transport mechanism is unknown. Here, NDT1 could be a candidate NaMN transporter in addition to the ability of NAD+ transport because NDT1 protein can mediate NaMN as well (Palmieri et al., 2009). In brief, the light responsive NADP increase could be underpinned by the export of NaMN from chloroplasts to cytosol and the import of NAD+ from the cytosol to chloroplasts.
Although genes associated with NAD+ and NADP+ biosynthesis and metabolism have been continuously characterized and identified for decades, their regulators and transport mechanisms remain largely unknown. Because NADP+ biosynthesis is mainly supported by the amount of available NAD+, NAD+ supply is the key regulator of NADP+ synthesis. Importantly, NADP+ synthesis proceeds on demand even though NAD+ synthesis is exclusively carried out in the cytosol. Therefore, inter-organelle signaling is rather important to communicate NAD+ demand for each location where NADP+ synthesis occurs. Recent studies have revealed the existence of a dynamic response of the chloroplastic NADP pool to light, underpinning NAD and NADP metabolism including degradation, metabolism, and biosynthesis. Moreover, the early steps of de novo NAD+ synthesis in chloroplasts appear to be controlled by redox regulation. Future dissection of inter-organelle NAD and NADP metabolism is thus essential for understanding the fate of chloroplastic NADP under various light conditions.
All authors listed have made a substantial, direct and intellectual contribution to the work, and approved it for publication.
This work was supported by the JSPS KAKENHI Grant Number 17H05714 and 19H04715 to MK-Y.
The authors declare that the research was conducted in the absence of any commercial or financial relationships that could be construed as a potential conflict of interest.
We would like to thank Editage (www.editage.com) for English language editing.
Agrimi, G., Russo, A., Pierri, C. L., and Palmieri, F. (2012). The peroxisomal NAD+ carrier of Arabidopsis thaliana transports coenzyme A and its derivatives. J. Bioenerg. Biomembr. 44, 333–340. doi: 10.1007/s10863-012-9445-0
Alric, J., and Johnson, X. (2017). Alternative electron transport pathways in photosynthesis: a confluence of regulation. Curr. Opin. Plant Biol. 37, 78–86. doi: 10.1016/j.pbi.2017.03.014
Anderson, J. M., Charbonneau, H., Jones, H. P., McCann, R. O., and Cormier, M. J. (1980). Characterization of the plant nicotinamide adenine dinucleotide kinase activator protein and its identification as calmodulin. Biochemistry 19, 3113–3120.
Berrin, J. G., Pierrugues, O., Brutesco, C., Alonso, B., Montillet, J. L., Roby, D., et al. (2005). Stress induces the expression of AtNADK-1, a gene encoding a NAD(H) kinase in Arabidopsis thaliana. Mol. Gen. Genomics. 273, 10–19. doi: 10.1007/s00438-005-1113-1
Breyton, C., Nandha, B., Johnson, G. N., Joliot, P., and Finazzi, G. (2006). Redox modulation of cyclic electron flow around photosystem I in C3 plants. Biochemistry 45, 13465–13475. doi: 10.1021/bi061439s
Chai, M. F., Chen, Q. J., An, R., Chen, Y. M., Chen, J., and Wang, X. C. (2005). NADK2, an Arabidopsis chloroplastic NAD kinase, plays a vital role in both chlorophyll synthesis and chloroplast protection. Plant Mol. Biol. 59, 553–564. doi: 10.1007/s11103-005-6802-y
Chai, M. F., Wei, P. C., Chen, Q. J., An, R., Chen, J., Yang, S., et al. (2006). NADK3, a novel cytoplasmic source of NADPH, is required under conditions of oxidative stress and modulates abscisic acid responses in Arabidopsis. Plant J. 47, 665–674. doi: 10.1111/j.1365-313X.2006.02816.x
Chini, E. N., and Dousa, T. P. (1995). Enzymatic synthesis and degradation of nicotinate adenine dinucleotide phosphate (NAADP), a Ca(2+)-releasing agonist, in rat tissues. Biochem. Biophys. Res. Commun. 209, 167–174.
Dell’Aglio, E., Giustini, C., Kraut, A., Couté, Y., Mazars, C., Matringe, M., et al. (2019). Identification of the calmodulin-dependent NAD+ kinase sustaining the elicitor-induced oxidative burst in plants. bioRxiv [Preprint]. doi: 10.1101/521658
Dell’Aglio, E., Salvi, D., Kraut, A., Baudet, M., Macherel, D., Neveu, M., et al. (2016). No plastidial calmodulin-like proteins detected by two targeted mass-spectrometry approaches and GFP fusion proteins. New Negat. Plant Sci. 3–4, 19–26. doi: 10.1016/j.neps.2016.08.001
Demmig-Adams, B., and Adams, W. W. I. (1992). Photoprotection and other responses of plants to high light stress. Annu. Rev. Plant Physiol. Plant Mol. Biol. 43, 599–626. doi: 10.1146/annurev.pp.43.060192.003123
Di Martino, C., and Pallotta, M. L. (2011). Mitochondria-localized NAD biosynthesis by nicotinamide mononucleotide adenylyltransferase in Jerusalem artichoke (Helianthus tuberosus L.) heterotrophic tissues. Planta 234, 657–670. doi: 10.1007/s00425-011-1428-6
Foyer, C. H. (2018). Reactive oxygen species, oxidative signaling and the regulation of photosynthesis. Environ. Exp. Bot. 154, 134–142. doi: 10.1016/j.envexpbot.2018.05.003
Gallais, S., de Crescenzo, M. A., and Laval-Martin, D. L. (2000). Evidence of active NADP(+) phosphatase in dormant seeds of Avena sativa L. J. Exp. Bot. 51, 1389–1394. doi: 10.1093/jxb/51.349.1389
Geigenberger, P. (2003). Response of plant metabolism to too little oxygen. Curr. Opin. Plant Biol. 6, 247–256. doi: 10.1016/S1369-5266(03)00038-4
Hajiboland, R. (2014). “Chapter 1 – reactive oxygen species and photosynthesis” in Oxidative damage to plants. ed. P. Ahmad (San Diego: Academic Press), 1–63.
Hanke, G., and Mulo, P. (2013). Plant type ferredoxins and ferredoxin-dependent metabolism. Plant Cell Environ. 36, 1071–1084. doi: 10.1111/pce.12046
Hao, J., Petriacq, P., de Bont, L., Hodges, M., and Gakiere, B. (2018). Characterization of l-aspartate oxidase from Arabidopsis thaliana. Plant Sci. 271, 133–142. doi: 10.1016/j.plantsci.2018.03.016
Hashida, S. N., Itami, T., Takahara, K., Hirabayashi, T., Uchimiya, H., and Kawai-Yamada, M. (2016). Increased rate of NAD metabolism shortens plant longevity by accelerating developmental senescence in Arabidopsis. Plant Cell Physiol. 57, 2427–2439. doi: 10.1093/pcp/pcw155
Hashida, S. N., Itami, T., Takahashi, H., Takahara, K., Nagano, M., Kawai-Yamada, M., et al. (2010). Nicotinate/nicotinamide mononucleotide adenyltransferase-mediated regulation of NAD biosynthesis protects guard cells from reactive oxygen species in ABA-mediated stomatal movement in Arabidopsis. J. Exp. Bot. 61, 3813–3825. doi: 10.1093/jxb/erq190
Hashida, S. N., Miyagi, A., Nishiyama, M., Yoshida, K., Hisabori, T., and Kawai-Yamada, M. (2018). Ferredoxin/thioredoxin system plays an important role in the chloroplastic NADP status of Arabidopsis. Plant J. 95, 947–960. doi: 10.1111/tpj.14000
Hashida, S. N., Takahashi, H., and Uchimiya, H. (2009). The role of NAD biosynthesis in plant development and stress response. Ann. Bot. 103, 819–824. doi: 10.1093/aob/mcp019
Hunt, L., and Gray, J. E. (2009). The relationship between pyridine nucleotides and seed dormancy. New Phytol. 181, 62–70. doi: 10.1111/j.1469-8137.2008.02641.x
Joliot, P., and Johnson, G. N. (2011). Regulation of cyclic and linear electron flow in higher plants. Proc. Natl. Acad. Sci. USA 108, 13317–13322. doi: 10.1073/pnas.1110189108
Joliot, P., and Joliot, A. (2005). Quantification of cyclic and linear flows in plants. Proc. Natl. Acad. Sci. USA 102, 4913–4918. doi: 10.1073/pnas.0501268102
Kanazawa, A., Ostendorf, E., Kohzuma, K., Hoh, D., Strand, D. D., Sato-Cruz, M., et al. (2017). Chloroplast ATP synthase modulation of the thylakoid proton motive force: implications for photosystem I and photosystem II photoprotection. Front. Plant Sci. 8:719. doi: 10.3389/fpls.2017.00719
Karita, E., Yamakawa, H., Mitsuhara, I., Kuchitsu, K., and Ohashi, Y. (2004). Three types of tobacco calmodulins characteristically activate plant NAD kinase at different Ca2+ concentrations and pHs. Plant Cell Physiol. 45, 1371–1379. doi: 10.1093/pcp/pch158
Katoh, A., Uenohara, K., Akita, M., and Hashimoto, T. (2006). Early steps in the biosynthesis of NAD in Arabidopsis start with aspartate and occur in the plastid. Plant Physiol. 141, 851–857. doi: 10.1104/pp.106.081091
Kramer, D. M., Avenson, T. J., and Edwards, G. E. (2004). Dynamic flexibility in the light reactions of photosynthesis governed by both electron and proton transfer reactions. Trends Plant Sci. 9, 349–357. doi: 10.1016/j.tplants.2004.05.001
Kruger, N. J., and von Schaewen, A. (2003). The oxidative pentose phosphate pathway: structure and organisation. Curr. Opin. Plant Biol. 6, 236–246. doi: 10.1016/S1369-5266(03)00039-6
Li, W. Y., Wang, X., Li, R., Li, W. Q., and Chen, K. M. (2014). Genome-wide analysis of the NADK gene family in plants. PLoS One 9:e101051. doi: 10.1371/journal.pone.0116565
Maruta, T., Ogawa, T., Tsujimura, M., Ikemoto, K., Yoshida, T., Takahashi, H., et al. (2016). Loss-of-function of an Arabidopsis NADPH pyrophosphohydrolase, AtNUDX19, impacts on the pyridine nucleotides status and confers photooxidative stress tolerance. Sci. Rep. 6:37432. doi: 10.1038/srep37432
McGuinness, E. T., and Butler, J. R. (1985). NAD+ kinase--a review. Int. J. Biochem. 17, 1–11. doi: 10.1016/0020-711X(85)90079-5
Michalska, J., Zauber, H., Buchanan, B. B., Cejudo, F. J., and Geigenberger, P. (2009). NTRC links built-in thioredoxin to light and sucrose in regulating starch synthesis in chloroplasts and amyloplasts. Proc. Natl. Acad. Sci. USA 106, 9908–9913. doi: 10.1073/pnas.0903559106
Munekage, Y., Hashimoto, M., Miyake, C., Tomizawa, K., Endo, T., Tasaka, M., et al. (2004). Cyclic electron flow around photosystem I is essential for photosynthesis. Nature 429, 579–582. doi: 10.1038/nature02598
Murthy, N. M., Ollagnier-de-Choudens, S., Sanakis, Y., Abdel-Ghany, S. E., Rousset, C., Ye, H., et al. (2007). Characterization of Arabidopsis thaliana SufE2 and SufE3: functions in chloroplast iron-sulfur cluster assembly and Nad synthesis. J. Biol. Chem. 282, 18254–18264. doi: 10.1074/jbc.M701428200
Muto, S., and Miyachi, S. (1977). Properties of a protein activator of NAD kinase from plants. Plant Physiol. 59, 55–60. doi: 10.1104/pp.59.1.55
Nikkanen, L., and Rintamaki, E. (2019). Chloroplast thioredoxin systems dynamically regulate photosynthesis in plants. Biochem. J. 476, 1159–1172. doi: 10.1042/BCJ20180707
Noctor, G., Queval, G., and Gakiere, B. (2006). NAD(P) synthesis and pyridine nucleotide cycling in plants and their potential importance in stress conditions. J. Exp. Bot. 57, 1603–1620. doi: 10.1093/jxb/erj202
Okegawa, Y., Kagawa, Y., Kobayashi, Y., and Shikanai, T. (2008). Characterization of factors affecting the activity of photosystem I cyclic electron transport in chloroplasts. Plant Cell Physiol. 49, 825–834. doi: 10.1093/pcp/pcn055
Ort, D. R., and Yocum, C. F. (1996). Oxygenic photosynthesis: The light reactions. Netherlands: Springer.
Palmieri, F., Rieder, B., Ventrella, A., Blanco, E., Do, P. T., Nunes-Nesi, A., et al. (2009). Molecular identification and functional characterization of Arabidopsis thaliana mitochondrial and chloroplastic NAD+ carrier proteins. J. Biol. Chem. 284, 31249–31259. doi: 10.1074/jbc.M109.041830
Palmieri, L., Santoro, A., Carrari, F., Blanco, E., Nunes-Nesi, A., Arrigoni, R., et al. (2008). Identification and characterization of ADNT1, a novel mitochondrial adenine nucleotide transporter from Arabidopsis. Plant Physiol. 148, 1797–1808. doi: 10.1104/pp.108.130310
Perez-Ruiz, J. M., Naranjo, B., Ojeda, V., Guinea, M., and Cejudo, F. J. (2017). NTRC-dependent redox balance of 2-Cys peroxiredoxins is needed for optimal function of the photosynthetic apparatus. Proc. Natl. Acad. Sci. USA 114, 12069–12074. doi: 10.1073/pnas.1706003114
Perez-Ruiz, J. M., Spinola, M. C., Kirchsteiger, K., Moreno, J., Sahrawy, M., and Cejudo, F. J. (2006). Rice NTRC is a high-efficiency redox system for chloroplast protection against oxidative damage. Plant Cell 18, 2356–2368. doi: 10.1105/tpc.106.041541
Petriacq, P., de Bont, L., Hager, J., Didierlaurent, L., Mauve, C., Guerard, F., et al. (2012). Inducible NAD overproduction in Arabidopsis alters metabolic pools and gene expression correlated with increased salicylate content and resistance to Pst-AvrRpm1. Plant J. 70, 650–665. doi: 10.1111/j.1365-313X.2012.04920.x
Pinnola, A., and Bassi, R. (2018). Molecular mechanisms involved in plant photoprotection. Biochem. Soc. Trans. 46, 467–482. doi: 10.1042/BST20170307
Pollak, N., Dolle, C., and Ziegler, M. (2007). The power to reduce: pyridine nucleotides – small molecules with a multitude of functions. Biochem. J. 402, 205–218. doi: 10.1042/BJ20061638
Schippers, J. H., Nunes-Nesi, A., Apetrei, R., Hille, J., Fernie, A. R., and Dijkwel, P. P. (2008). The Arabidopsis onset of leaf death5 mutation of quinolinate synthase affects nicotinamide adenine dinucleotide biosynthesis and causes early ageing. Plant Cell 20, 2909–2925. doi: 10.1105/tpc.107.056341
Selinski, J., and Scheibe, R. (2019). Malate valves: old shuttles with new perspectives. Plant Biol. 21(Suppl. 1), 21–30. doi: 10.1111/plb.12869
Takahashi, S., and Badger, M. R. (2011). Photoprotection in plants: a new light on photosystem II damage. Trends Plant Sci. 16, 53–60. doi: 10.1016/j.tplants.2010.10.001
Takahashi, H., Takahara, K., Hashida, S. N., Hirabayashi, T., Fujimori, T., Kawai-Yamada, M., et al. (2009). Pleiotropic modulation of carbon and nitrogen metabolism in Arabidopsis plants overexpressing the NAD kinase2 gene. Plant Physiol. 151, 100–113. doi: 10.1104/pp.109.140665
Takahashi, H., Watanabe, A., Tanaka, A., Hashida, S. N., Kawai-Yamada, M., Sonoike, K., et al. (2006). Chloroplast NAD kinase is essential for energy transduction through the xanthophyll cycle in photosynthesis. Plant Cell Physiol. 47, 1678–1682. doi: 10.1093/pcp/pcl029
Thormahlen, I., Zupok, A., Rescher, J., Leger, J., Weissenberger, S., Groysman, J., et al. (2017). Thioredoxins play a crucial role in dynamic acclimation of photosynthesis in fluctuating light. Mol. Plant 10, 168–182. doi: 10.1016/j.molp.2016.11.012
Todisco, S., Agrimi, G., Castegna, A., and Palmieri, F. (2006). Identification of the mitochondrial NAD+ transporter in Saccharomyces cerevisiae. J. Biol. Chem. 281, 1524–1531. doi: 10.1074/jbc.M510425200
Turner, W. L., Waller, J. C., Vanderbeld, B., and Snedden, W. A. (2004). Cloning and characterization of two NAD kinases from Arabidopsis. Identification of a calmodulin binding isoform. Plant Physiol. 135, 1243–1255. doi: 10.1104/pp.104.040428
Van Hoewyk, D., Abdel-Ghany, S. E., Cohu, C. M., Herbert, S. K., Kugrens, P., Pilon, M., et al. (2007). Chloroplast iron-sulfur cluster protein maturation requires the essential cysteine desulfurase CpNifS. Proc. Natl. Acad. Sci. 104, 5686–5691. doi: 10.1073/pnas.0700774104
Vialet-Chabrand, S., Matthews, J. S., Simkin, A. J., Raines, C. A., and Lawson, T. (2017). Importance of fluctuations in light on plant photosynthetic acclimation. Plant Physiol. 173, 2163–2179. doi: 10.1104/pp.16.01767
Waller, J. C., Dhanoa, P. K., Schumann, U., Mullen, R. T., and Snedden, W. A. (2009). Subcellular and tissue localization of NAD kinases from Arabidopsis: compartmentalization of de novo NADP biosynthesis. Planta. 231, 305–317. doi: 10.1007/s00425-009-1047-7
Wedel, N., Soll, J., and Paap, B. K. (1997). CP12 provides a new mode of light regulation of Calvin cycle activity in higher plants. Proc. Natl. Acad. Sci. USA 94, 10479–10484.
Yamori, W., Makino, A., and Shikanai, T. (2016). A physiological role of cyclic electron transport around photosystem I in sustaining photosynthesis under fluctuating light in rice. Sci. Rep. 6:20147. doi: 10.1038/srep20147
Keywords: NAD+ supply, NAD+ synthesis, NADP+ synthesis, light response, linear electron transfer, photosynthesis, chloroplasts
Citation: Hashida S and Kawai-Yamada M (2019) Inter-Organelle NAD Metabolism Underpinning Light Responsive NADP Dynamics in Plants. Front. Plant Sci. 10:960. doi: 10.3389/fpls.2019.00960
Received: 17 May 2019; Accepted: 09 July 2019;
Published: 26 July 2019.
Edited by:
Paula Mulo, University of Turku, FinlandReviewed by:
Lauri Emil Nikkanen, University of Turku, FinlandCopyright © 2019 Hashida and Kawai-Yamada. This is an open-access article distributed under the terms of the Creative Commons Attribution License (CC BY). The use, distribution or reproduction in other forums is permitted, provided the original author(s) and the copyright owner(s) are credited and that the original publication in this journal is cited, in accordance with accepted academic practice. No use, distribution or reproduction is permitted which does not comply with these terms.
*Correspondence: Shin-nosuke Hashida, c2hhc2hpZGFAY3JpZXBpLmRlbmtlbi5vci5qcA==
Disclaimer: All claims expressed in this article are solely those of the authors and do not necessarily represent those of their affiliated organizations, or those of the publisher, the editors and the reviewers. Any product that may be evaluated in this article or claim that may be made by its manufacturer is not guaranteed or endorsed by the publisher.
Research integrity at Frontiers
Learn more about the work of our research integrity team to safeguard the quality of each article we publish.