- 1Laboratory of Phytopathology, Department of Plants and Crops, Faculty of Bioscience Engineering, Ghent University, Ghent, Belgium
- 2Centre of Microbial and Plant Genetics, Faculty of Bioscience Engineering, KU Leuven, Heverlee, Belgium
Beneficial Pseudomonas spp. produce an array of antimicrobial secondary metabolites such as cyclic lipopeptides (CLPs). We investigated the capacity of CLP-producing Pseudomonas strains and their crude CLP extracts to control rice blast caused by Magnaporthe oryzae, both in a direct manner and via induced systemic resistance (ISR). In planta biocontrol assays showed that lokisin-, white line inducing principle (WLIP)-, entolysin- and N3-producing strains successfully induced resistance to M. oryzae VT5M1. Furthermore, crude extracts of lokisin, WLIP and entolysin gave similar ISR results when tested in planta. In contrast, a xantholysin-producing strain and crude extracts of N3, xantholysin and orfamide did not induce resistance against the rice blast disease. The role of WLIP in triggering ISR was further confirmed by using WLIP-deficient mutants. The severity of rice blast disease was significantly reduced when M. oryzae spores were pre-treated with crude extracts of N3, lokisin, WLIP, entolysin or orfamide prior to inoculation. In vitro microscopic assays further revealed the capacity of crude N3, lokisin, WLIP, entolysin, xantholysin and orfamide to significantly inhibit appressoria formation by M. oryzae. In addition, the lokisin and WLIP biosynthetic gene clusters in the producing strains are described. In short, our study demonstrates the biological activity of structurally diverse CLPs in the control of the rice blast disease caused by M. oryzae. Furthermore, we provide insight into the non-ribosomal peptide synthetase genes encoding the WLIP and lokisin biosynthetic machineries.
Introduction
Fluorescent Pseudomonas spp. belonging to the phylum Proteobacteria are prominent among rhizosphere and soil microbes (Philippot et al., 2013). The biocontrol capacities of rhizosphere-associated Pseudomonas species have been extensively studied (Haas and Défago, 2005; Weller, 2007; D’aes et al., 2010; Höfte and Altier, 2010; Olorunleke et al., 2015b; Stringlis et al., 2018). Pseudomonas species are metabolically versatile and produce an array of secondary metabolites including various antibiotics and cyclic lipopeptides (CLPs) (Gross and Loper, 2009). CLPs are amphiphilic molecules made up of a cyclic oligopeptide lactone ring linked to a fatty acid tail (Raaijmakers et al., 2006, 2010). CLPs are synthesized by non-ribosomal peptide synthetases (NRPSs) which encompass different modules containing condensation, adenylation and thiolation domains for co-linear synthesis of a specific (lipo)peptide and its release in linear or cyclic form by thioesterase activity (Finking and Marahiel, 2004; Strieker et al., 2010). Pseudomonas CLPs are currently classified into at least 14 different groups based on the length of the oligopeptide (Gross and Loper, 2009; Olorunleke et al., 2015b; Geudens and Martins, 2018). The lipid moiety in these groups is usually a hydroxydecanoic acid. Under in vitro and in vivo conditions, Pseudomonas CLPs have been shown to possess biocontrol potential against several plant pathogens including Pythium myriotylum (Oni et al., 2019a,b), Pythium aphanidermatum (Michelsen et al., 2015), Phytophthora infestans, Phytophthora capsici and Pythium ultimum (Van Der Voort et al., 2015), Rhizoctonia solani (D’aes et al., 2014; Michelsen et al., 2015; Olorunleke et al., 2015a), Cochliobolus miyabeanus, and Magnaporthe oryzae (Ma et al., 2017).
Biocontrol of plant pathogens using CLPs may involve direct antagonism and/or indirect activity via induced systemic resistance (ISR). ISR can occur in plants by the perception of elicitors secreted by microbes (or pathogens), similar to the microbe (or pathogen)-associated molecular patterns (MAMPs or PAMPs) (Newman et al., 2013). MAMPs or PAMPs are recognized by pattern recognition receptors associated with the cell membrane resulting in the elicitation of plant innate immunity (Boutrot and Zipfel, 2017). Diverse elicitors secreted by plant beneficial bacteria can trigger ISR (De Vleesschauwer and Höfte, 2009; Mariutto and Ongena, 2015), including some CLPs produced by Pseudomonas spp. (massetolide A, orfamide and sessilin) (Tran et al., 2007; Ma et al., 2016b, 2017), and by Bacillus spp. (surfactin, fengycin, and iturin) (Ongena et al., 2007; Jourdan et al., 2009; Henry et al., 2011). There are no indications, however, that plants perceive CLPs by specific receptors. Results with the Bacillus CLP surfactin indicate that perception relies on a lipid-driven process at the plasma membrane level (Henry et al., 2011).
Rice (Oryza sativa L.) is a major staple for more than 50% of the world population and is an essential food crop globally (Fageria and Baligar, 2003). However, this crop is affected by both abiotic and biotic stresses (Mittler, 2006; Pandey et al., 2015). Of the biotic stresses common to rice, the blast disease caused by the filamentous ascomycete, M. oryzae, has been ranked among one of the most important diseases of rice due to its widespread occurrence and destructiveness (Dean et al., 2005). This fungus is a hemibiotroph such that the infection process requires an initial biotrophic phase wherein the pathogen forms bulbous invasive hyphae within healthy plant cells (Koga, 1994). This is followed by a switch to necrotrophic growth, resulting in the death of plant cells. M. oryzae utilizes appressoria to penetrate the cuticle of rice by means of turgor pressure generation (Wilson and Talbot, 2009; Yan and Talbot, 2016).
Biological control of M. oryzae due to beneficial Pseudomonas species strains has been scantily reported (Spence et al., 2014; Hernández-Rodríguez et al., 2018; Wu et al., 2018). Orfamide produced by beneficial Pseudomonas species was reported to induce systemic resistance in rice against the brown spot fungus Cochliobolus miyabeanus but not to M. oryzae VT5M1 (Ma et al., 2017). More so, orfamide-producing strain Pseudomonas protegens CHA0 did not induce resistance to M. oryzae in rice (Spence et al., 2014). Another study showed the direct effect of orfamide in the inhibition of appressoria formation by M. oryzae and reduction of the severity of blast on rice (Ma et al., 2016a). Pseudomonas mosselii BS011 showed strong inhibitory activity against M. oryzae and a gene cluster that most likely mediates xantholysin production was required for this activity. In addition, a crude extract of P. mosselii BS011 inhibited the development of M. oryzae and impaired appressoria formation (Wu et al., 2018).
In a previous study, we characterized CLP-producing Pseudomonas strains associated with the cocoyam rhizosphere in Cameroon (Oni et al., 2019a). Representative CLP-producing strains effectively suppressed the cocoyam root rot disease caused by Pythium myriotylum. CLPs described in our previous study include entolysin, lokisin, putisolvin, xantholysin, WLIP, cocoyamide A and seven novel ones (Oni et al., 2019a). Furthermore, we showed that purified lokisin, entolysin, xantholysin, and WLIP could interact with the mycelium of P. myriotylum resulting in hyphal leakage and/or branching (Oni et al., 2019a). This rich CLP collection provided us with an opportunity to investigate the possible role of CLPs other than orfamide and xantholysin in the biological control of rice blast caused by M. oryzae via ISR or direct antagonism.
Materials and Methods
Strains, Media and Growth Conditions
Pseudomonas and M. oryzae strains used in this study are presented in Table 1. Pseudomonas wild type strains were cultured on King’s B (KB) (King et al., 1954) agar at 28°C for 48 h. WLIP-deficient mutants of P. putida RW10S2 were grown on KB agar supplemented with 50 μg/ml kanamycin at 28°C. Broth culture of Pseudomonas strains were obtained in KB broth on a rotary shaker at 150 revolutions per minute (rpm) and incubated at 28°C for about 24 h. M. oryzae isolate VT5M1 (Thuan et al., 2006) was grown on complete medium (CM) (Talbot et al., 1993), at 28°C for 5–8 days.
Extraction of Crude CLPs
Extraction of crude CLPs from Pseudomonas strains was carried out following an established protocol (Oni et al., 2019a). Specifically, Pseudomonas strains were grown on KB agar overnight after which seed cultures were obtained by growing bacterial cells in 5 ml KB broth in sterile glass tubes, at 150 rpm and 28°C for 24 h. The 24 h old culture was subsequently transferred into 2 L Erlenmeyer flasks containing 400 ml KB broth and incubated at 150 rpm and 28°C for 24 h. Cell-free supernatants were obtained by centrifugation of the culture at 10,000 g and 4°C for 10 min followed by acidification to pH 2 using 6 N hydrochloric acid with constant stirring. Acidified culture supernatant was kept overnight at 4°C in order to precipitate the CLPs. Precipitated CLPs were collected by centrifugation at 10,000 g for 10 min after which crude CLPs were extracted using 100% methanol. The solvent was evaporated at room temperature to obtain crude CLP samples. Crude orfamide A was extracted from Pseudomonas protegens CHA0 following a different published procedure (Ma et al., 2016a).
Plant Material and Growth Conditions
Rice (Oryza sativa L.) indica cv. CO-39 was used as plant material for all bioassays. Paddy rice seeds were dehusked and surface-sterilized with 2% (w/v) sodium hypochlorite solution by shaking on a rotary shaker for 10 min at 100 rpm, and room temperature. The seeds were rinsed several times with sterile distilled water, and air-dried. Air-dried seeds were germinated in the dark at 28°C on sterile filter paper in Petri dishes supplemented with 5 ml sterile distilled water. After 5 days, pre-germinated rice seeds were exposed to light at 30 ± 4°C for 2 days. Rice seedlings were transplanted into plastic trays (23 × 16 × 6 cm, 12 plants in each) using non-sterile potting soil (Structural; Snebbout, Kaprijke, Belgium) as substrate (De Vleesschauwer et al., 2006; Ma et al., 2016a, 2017). Rice plants were kept in a greenhouse (photoperiod with 12 h light at 30 ± 4°C). Watering was done every 3 days, and 200 ml of nutrient [FeSO4; 2 g/L and (NH4)2SO4; 1 g/L] was applied to each tray once a week.
ISR Potential of Pseudomonas spp. Against M. oryzae VT5M1
For ISR assays in rice, Pseudomonas wild type strains were grown on KB agar at 28°C for 48 h, while WLIP-mutants were grown on KB agar supplemented with 50 μg/ml of kanamycin. Bacteria were scraped from plates and suspended in 0.85% sodium chloride (w/v). Bacterial density was determined by measuring the optical density (OD) at 620 nm. The inoculum of bacteria was calculated based on 600 g of soil and adjusted to a final density of 1 × 107 CFU/g. Twenty five μg/ml of S-methyl 1,2,3-benzothiadiazole-7-carbothioate (BTH) (a kind gift from Syngenta Crop Protection, Brussels, Belgium) was used as the positive control. Bacterial suspensions and BTH were diluted with 250 ml of water and mixed with 600 g of potting soil for 5 min. Roots of rice seedlings obtained as mentioned earlier were inoculated with the standardized bacterial inoculum or BTH by soaking for 10 min before planting them in plastic trays containing potting soil mixed with the respective bacterial suspension or BTH. For each strain and the BTH control, three biological replicates of 12 plants were used. Bacteria and BTH were added as soil drench 3 days before inoculating 4 weeks-old rice plants with M. oryzae VT5M1. Spores of 5-days old M. oryzae VT5M1 were suspended in 0.5% (w/v) gelatin to final concentration 5 × 104 spores/ml. Rice plants were evenly sprayed with 1 ml of spore suspension using a compressor-powered airbrush gun (Badger Airbrush model 150TM). Inoculated rice plants were kept for 22 h in a dark chamber (relative humidity ≥ 90%; 25 ± 5°C), and further transferred to the greenhouse for disease development. Disease was scored 6 days after infection by counting the number of susceptible lesions (ellipsoid to round-shaped lesion with a gray center indicative of sporulation) shown by the second stage youngest leaves (De Vleesschauwer et al., 2006; Ma et al., 2016a). Pictures of typical disease symptoms were taken 7 days after infection. The bioassay was carried out twice.
Root Colonization Assay
Colonization of rice roots was evaluated as published previously (Ma et al., 2017; Oni et al., 2019a). Specifically, roots of five rice plants from each treatment were randomly chosen after disease evaluation, and rinsed carefully with water to remove soil. The roots were cut into small pieces and weighed. Bacterial suspension was obtained by crushing the roots using a mortar and pestle in 10 ml sterile saline (0.85% sodium chloride, w/v) and fine sand. Exactly 100 μl of the serially diluted suspension was plated on KB agar and incubated for 24 h at 28°C. Fluorescent Pseudomonas bacterial colonies were counted after 24 h and the data were log10 transformed prior to statistical analysis.
Induction of Systemic Resistance to Rice Blast by Crude Pseudomonas CLPs
Crude CLPs extracts were dissolved in 250 ml of distilled water to obtain a final concentration of 25 μg/ml. Twenty five μg/ml of BTH was used as positive control. Crude CLPs and BTH were separately mixed with potting soil for 5 min. Rice seedlings obtained as earlier described, were soaked in 25 μg/ml of each CLP/BTH for 10 min and planted in plastic trays (containing 600 g of soil mixed with crude CLPs, three biological replicates of 12 plants per tray). A second application of 25 μg/ml of each crude CLP or BTH was conducted using 250 ml of tap water as soil drench 3 days before inoculation of the rice plants with M. oryzae VT5M1. Preparation of spore suspension, inoculation of the rice plants, and disease evaluation were done as described above. The bioassay was conducted twice.
Co-application of CLP-M. oryzae Spore Mixtures on Rice Leaves
Pre-germinated rice seedlings were grown in plastic trays containing 600 g of non-sterile potting soil as mentioned above, and maintained under greenhouse condition for 4 weeks. Spore suspensions of M. oryzae VT5M1 were prepared using the modified method described previously (Thuan et al., 2006). Specifically, cultures of M. oryzae VT5M1 were grown for 8 days at 28°C. Spores were collected and suspended in 0.5% (w/v) gelatin, mixed with crude CLPs to final concentration of 5 × 104 spores/ml, and 25 μg/ml of each CLP. The mixture was evenly sprayed on rice plants using a compressor-powered airbrush gun, while the trays were frequently rotated during this procedure. Each plant received 1 ml of the mixture. Healthy control plants received only 0.5% gelatin suspension, positive control plants were sprayed with a mixture of 0.5% gelatin, 5 × 104 spores/ml and 25 μg/ml of BTH, while diseased control plants received 1 ml 5 × 104 spores/ml and 0.5% gelatin mixture. Preparation of spore suspension, inoculation of the rice plants, and disease evaluation were done as described above. The bioassay was conducted twice.
In vitro Bioassay With M. oryzae Isolate VT5M1 and Crude CLPs
Different crude CLPs used in this study were dissolved in 100% dimethylsulfoxide (DMSO) as stocks. Further dilutions were made in 0.1% DMSO to get the desired concentrations for bioassays. Five-day old M. oryzae isolate VT5M1 spores (5 × 104 spores/ml) were collected from CM agar plates and mixed with different crude CLP extracts and 0.1% DMSO was used as a control. In this experiment, 100 μl of different concentrations: 1, 5, 10, and 25 μg/ml of each crude CLP was mixed with 100 μl of M. oryzae while the negative control received 100 μl of 0.1% DMSO. Fifty μl of the mixture was transferred onto a plastic slide (Fisher Scientific, Belgium) and incubated at 28°C. Spore germination was evaluated by counting the number of germination tubes after a 4 h incubation period. Appressoria formation was assessed by randomly counting at least 50 spores after 8 h incubation. Microscopic observations were carried out using an Olympus BX51 Microscope. The experiment was carried out twice.
In vitro Antagonism of Xantholysin-Producing Strains Against M. oryzae
A Petri dish assay was used to investigate the in vitro antagonism of the xantholysin-producing strains COR51 and BW11M1 against M. oryzae VT5M1 and M. oryzae Guy11, as described by Desai et al. (2002) and Wu et al. (2018). Specifically, 3 μl of overnight Luria Bertani (LB) broth culture of the test bacteria were spotted on either side of CM plates spanning 2 cm from the center. The plates were incubated for 24 h at 25°C, after which a mycelium plug of VT5M1 or Guy11 (5 mm in diameter) was placed at the center of the plate. Plates were re-incubated at 25°C for 6–10 days.
Draft Genome Sequencing of CLP-Producing Pseudomonas Strains
Genomic DNA was obtained from 24 h old culture of the lokisin-producing Pseudomonas strains COR10 and the WLIP-producing strain NSE1 grown in LB broth using the Wizard Genomic DNA Purification Kit from Promega (Promega Corporation, 2800 Woods Hollow Road Madison, WI, United States). Genomes were sequenced using the Illumina Hiseq 2500 or Miseq system platform at BaseClear (BaseClear B.V., Leiden, Netherlands). Paired-end sequence reads of the genomic DNA was generated using the Illumina HiSeq2500 system. FASTQ sequence files were generated using the Illumina Casava pipeline version 1.8.3. Initial and second quality assessments was based on data passing the Illumina Chastity filtering and using FASTQC quality control tool version 0.10.0 respectively. De novo assembly was performed using the CLC Genomics Workbench version 8.5.1. Mis-assemblies and nucleotide disagreement between the Illumina data and the contig sequences was corrected with Pilon version 1.11 (Walker et al., 2014). The scaffolding analysis was done using the SSPACE Premium scaffolder version 2.3 (Boetzer et al., 2011). Automated gap closure analysis was done using GapFiller version 1.10 (Boetzer and Pirovano, 2012).
Genome Mining and Bioinformatics Analyses
For automated genome annotation and mining, genomic sequences of the Pseudomonas sp. COR10 and NSE1, together with the genomes of Pseudomonas strains listed in Supplementary Table S1 and extracted from the Genbank database, were submitted to RAST Server (Aziz et al., 2008) and antiSMASH 4.0 (Blin et al., 2017). Genome mining was conducted on the annotated genomes, and comparison of NRPS proteins with other protein sequences in GenBank database was done by BLAST search1. The adenylation (A) and condensation (C) domains sequences of the non-ribosomal peptide synthase (NRPS) genes were extracted. Sequence alignment was carried out using MUSCLE (Edgar, 2004) in the software package Geneious Prime, 2019, and the cladograms were inferred by Neighbor Joining2. The amino acids (AA) sequence generated by the biosynthetic gene clusters were predicted by submitting the A domains to NRPSpredictor2 database (Röttig et al., 2011).
Phylogenetic Analyses of Pseudomonas spp.
The rpoB and rpoD sequences of the lokisin-, WLIP- and xantholysin-producing Pseudomonas strains used in this study were extracted from the draft genome sequences of the strains. Sequences of selected Pseudomonas type strains were retrieved from the GenBank (Supplementary Table S1). The sequences were aligned using MUSCLE (Edgar, 2004) in MEGA6 (Tamura et al., 2013). A phylogeny tree was constructed by Maximum likelihood with 1000 bootstrap replication, and P. aeruginosa was used as outgroup. rpoB and rpoD trees were generated separately after which a concatenated tree was obtained by combining the aligned sequences of the two genes.
Statistical Data Analysis
SPSS 25 statistical software was employed for data analysis. To compare across treatments, univariate ANOVA followed by Tukey’s post hoc tests were used and results were considered to be statistically different when p < 0.05.
Results
Potential of CLP-Producing Pseudomonas Strains to Induce Systemic Resistance Against M. oryzae on Rice
The capacity of CLP-producing Pseudomonas strains to induce resistance against M. oryzae in rice was investigated by soil inoculation. Results showed that N3-, WLIP-, lokisin- and entolysin-producing strains, COW3, COW10, COR10 and COR5, respectively, successfully induced resistance to M. oryzae in rice. These CLPs-producing strains gave an intermediate level of protection of 52, 54, 58, and 48% for COW3, COW10, COR10 and COR5, respectively, in comparison to 86% reduction in disease severity for the positive control (BTH). However, the xantholysin-producing isolate, COR51 only reduced disease severity with 10%, and could not significantly protect the rice plants against M. oryzae (Figures 1A,B). Furthermore, rice roots were successfully colonized by Pseudomonas isolates tested in the range of 108 to 109 CFU/g fresh roots (Table 2).
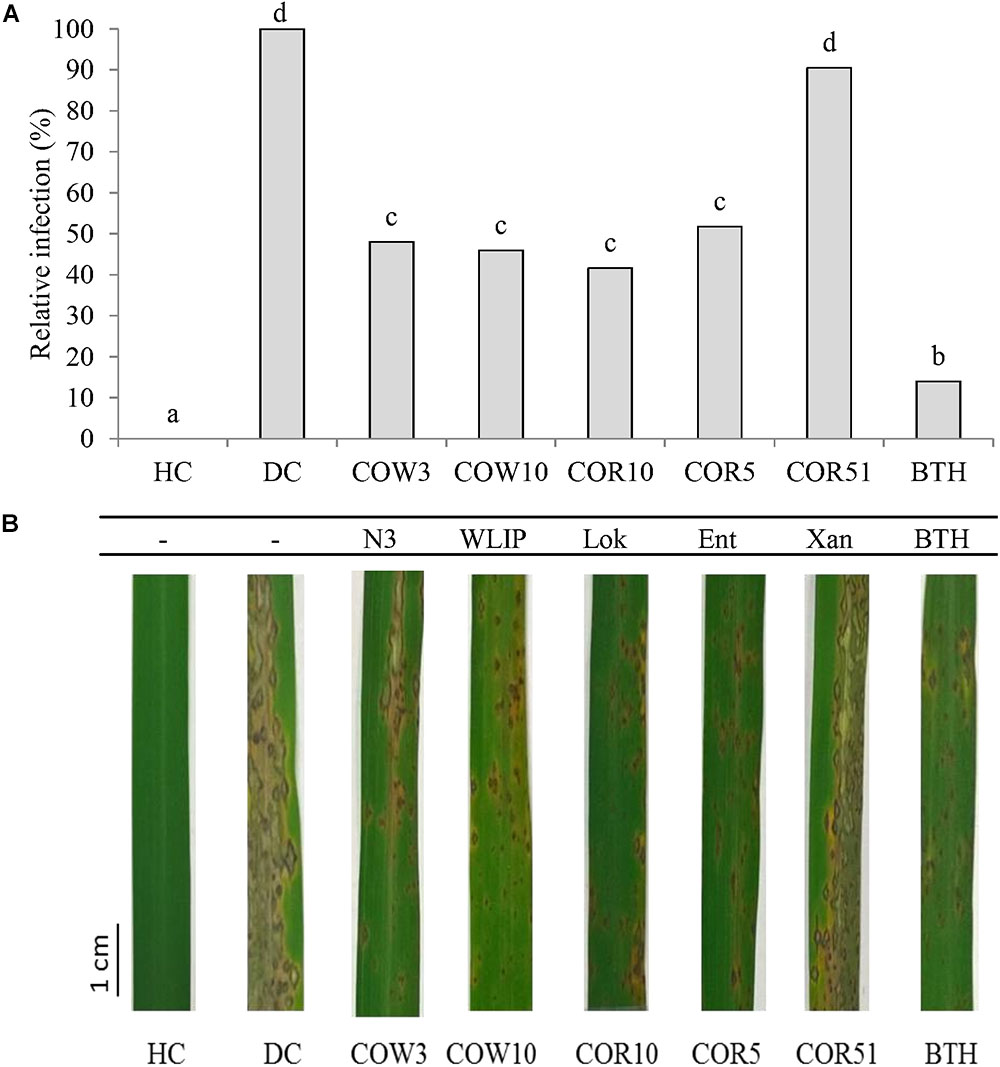
Figure 1. Induced systemic resistance (ISR) capacity of CLP-producing Pseudomonas spp. to M. oryzae in rice. (A) The potential of root application with Pseudomonas sp. strains COW3 (N3), COW10 (WLIP), COR10 (lokisin), COR5 (entolysin) and COR51 (xantholysin) to induce systemic resistance (ISR) against M. oryzae isolate VT5M1 on rice. HC: healthy control and DC: disease control plants were treated with water. Data of one experiment made up of three biological replicates, each consisting of twelve (12) plants are shown. The experiment was repeated with similar results. Different letters indicated statistically significant differences among different treatments (ANOVA followed by a Tukey’s tests: n = 36; α = 0.05), Lok, lokisin; Ent, entolysin; Xan, xantholysin; BTH, S-methyl 1,2,3-benzothiadiazole-7-carbothioate. (B) Representative pictures of disease symptoms observed on the second youngest leaf after 6 days post-inoculation with M. oryzae. Scale bar represents 1 cm.
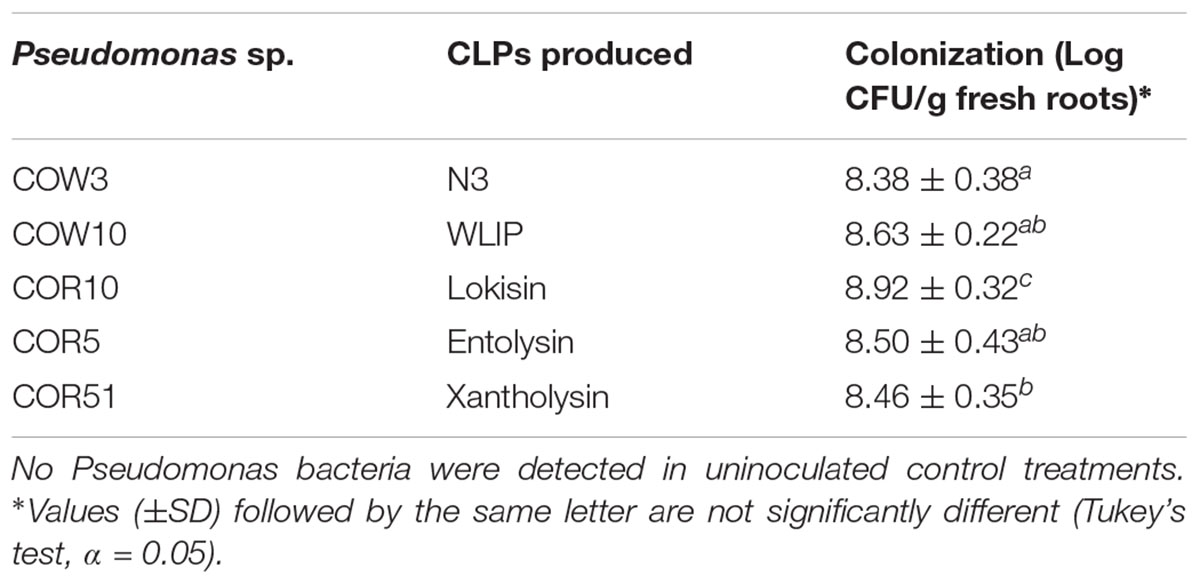
Table 2. Population of CLP-producing Pseudomonas strains used in induced resistance assays on rice roots.
Potential of Crude CLP Extracts to Induce Systemic Resistance to M. oryzae in Rice
The capacity of 25 μg/ml of crude extracts of N3, WLIP, lokisin, entolysin and xantholysin to induce resistance to M. oryzae VT5M1 on rice plants was tested in soil drench bioassays. Soil drenched with 25 μg/ml BTH was used as a positive control. Orfamide was used as a negative control since a previous study showed that this CLP is unable to induce resistance to rice blast (Ma et al., 2017). Lokisin, WLIP, and entolysin exhibited ISR against M. oryzae in rice plants. Lokisin gave a level of protection of 78%, followed by WLIP (61%), and entolysin (50%). In contrast, both xantholysin and orfamide reduced disease severity with 14%, while N3 reduced disease severity with 17%. These values were not significantly different from the diseased control (Figures 2A,B).
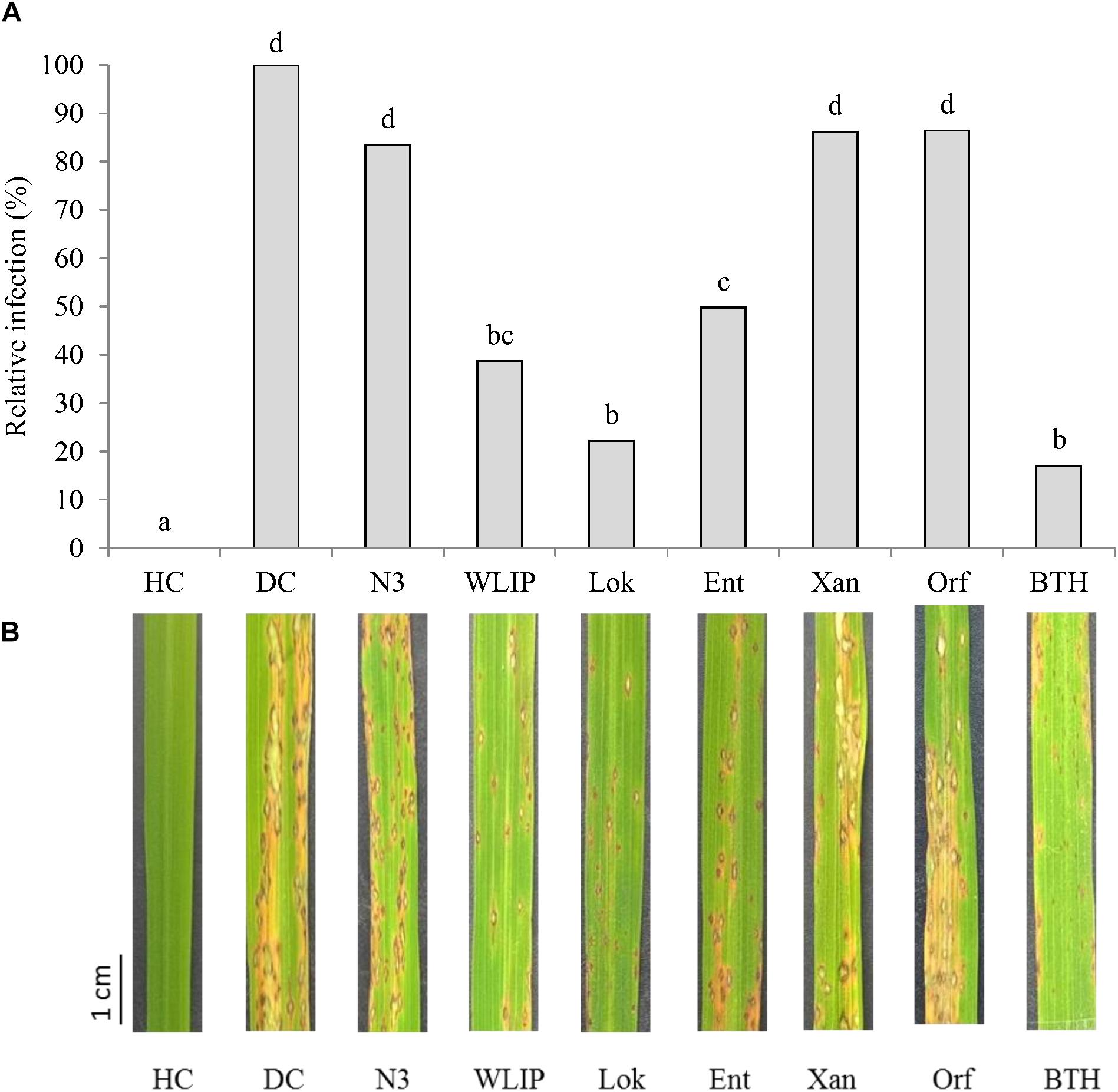
Figure 2. Induce systemic resistance capacity of crude CLP extracts against rice blast caused by M. oryzae. (A) ISR potential of crude WLIP, lokisin, entolysin, orfamide, and xantholysin to M. oryzae on rice. For each treatment, potting soil was mixed with 25 μg/ml of each CLP prior to experimental set-up. Subsequently, roots of rice seedlings were dipped in 25 μg/ml solution of each CLP for 10 min before planting. Data of one experiment made up of three biological replicates, consisting of 12 plants each are shown. The experiment was repeated with comparable results. Different letters indicate statistically significant differences among treatments (ANOVA followed by a Tukey’s test: n = 36; α = 0.05). (B) Representative disease symptoms on second youngest leaf at 6 days post-inoculation with M. oryzae. Scale bar represents 1 cm. DC, disease control; HC, healthy control; Lok, lokisin; Ent, entolysin; Xan, xantholysin; Orf, orfamide; BTH, S-methyl 1,2,3-benzothiadiazole-7-carbothioate.
Role of WLIP in Induced Systemic Resistance Against M. oryzae in Rice
To further decipher the role of WLIP in ISR, we used the WLIP-producing Pseudomonas strains COW10, NSE1 and RW10S2 and previously generated WLIP-deficient mutants (Pseudomonas putida CMPG2120, CMPG2169, and CMPG2170) in the RW10S2 background (see Table 1) in a rice-M. oryzae bioassay. Results showed that WLIP-producing RW10S2 induces resistance to M. oryzae in a manner similar to other WLIP producers (COW10 and NSE1), but portrays an intermediate level of protection in comparison to the BTH control. However, WLIP-deficient mutants CMPG2120, CMPG2169, and CMPG2170 lost the capacity to induce resistance against M. oryzae (Figures 3A,B). The root colonizing capacity of all strains used attained 108 CFU/g fresh roots (Table 3).
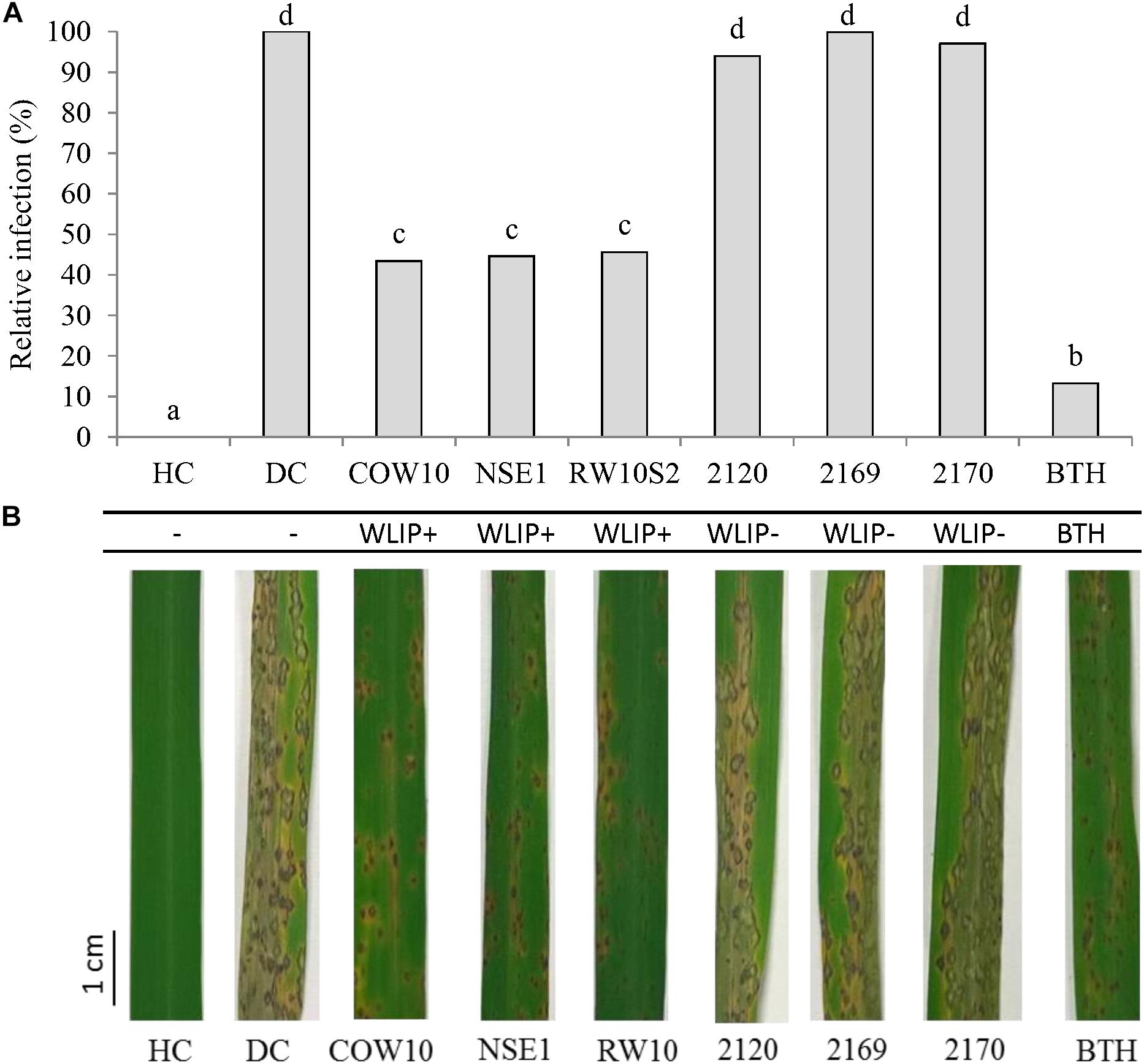
Figure 3. Role of WLIP in ISR against M. oryzae in rice. (A) Potential of WLIP-producing strain P. putida RW10S2, and biosynthetic mutants (CMPG2120, CMPG2169, and CMPG2170) in ISR against M. oryzae VT5M1 on rice. WLIP producers (COW10 and NSE1) and S-methyl 1,2,3-benzothiadiazole-7-carbothioate (BTH)-treated plants were used as positive controls. Data of one experiment made up of three biological replicates, consisting of 12 plants each are shown. The experiment was repeated with comparable results. Different letters indicate statistically significant differences among treatments (ANOVA followed by a Tukey’s test: n = 36; α = 0.05). (B) Representative disease symptoms on second youngest leaf at 6 days post-inoculation with M. oryzae. Scale bar represents 1 cm.
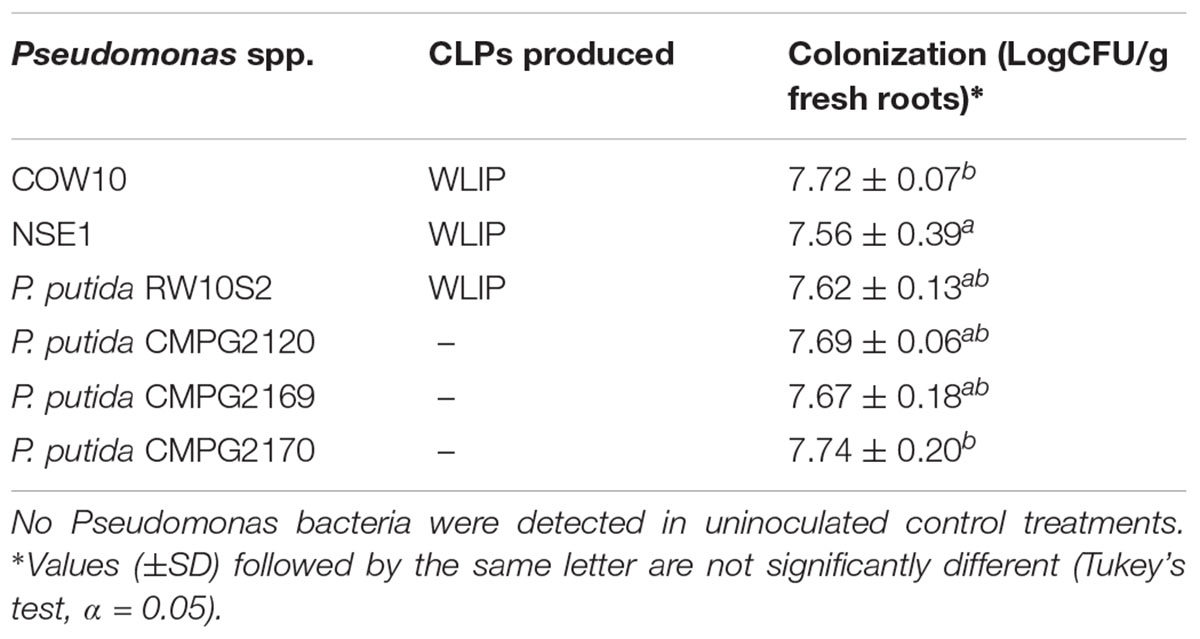
Table 3. Population of WLIP-producing Pseudomonas strains and mutants used in induced resistance assays on rice roots.
Potential of Crude CLPs to Reduce Rice Blast Severity When Co-applied With M. oryzae Spores on Rice Leaves
Crude CLP extracts were mixed with M. oryzae spores prior to fungal inoculation on rice leaves. N3, WLIP, lokisin, entolysin and orfamide extracts led to the reduction of blast disease severity on rice (Figures 4A,B). Relative reduction in blast severity ranged from 29% by entolysin and orfamide to 69% by lokisin, the latter providing protection comparable to BTH (79% reduction). Treatments with crude extracts of N3 (44%) and WLIP (47%) resulted in intermediate reduction in blast severity. However, the xantholysin extract reduced blast disease severity by only 15%, not significantly different from the diseased control.
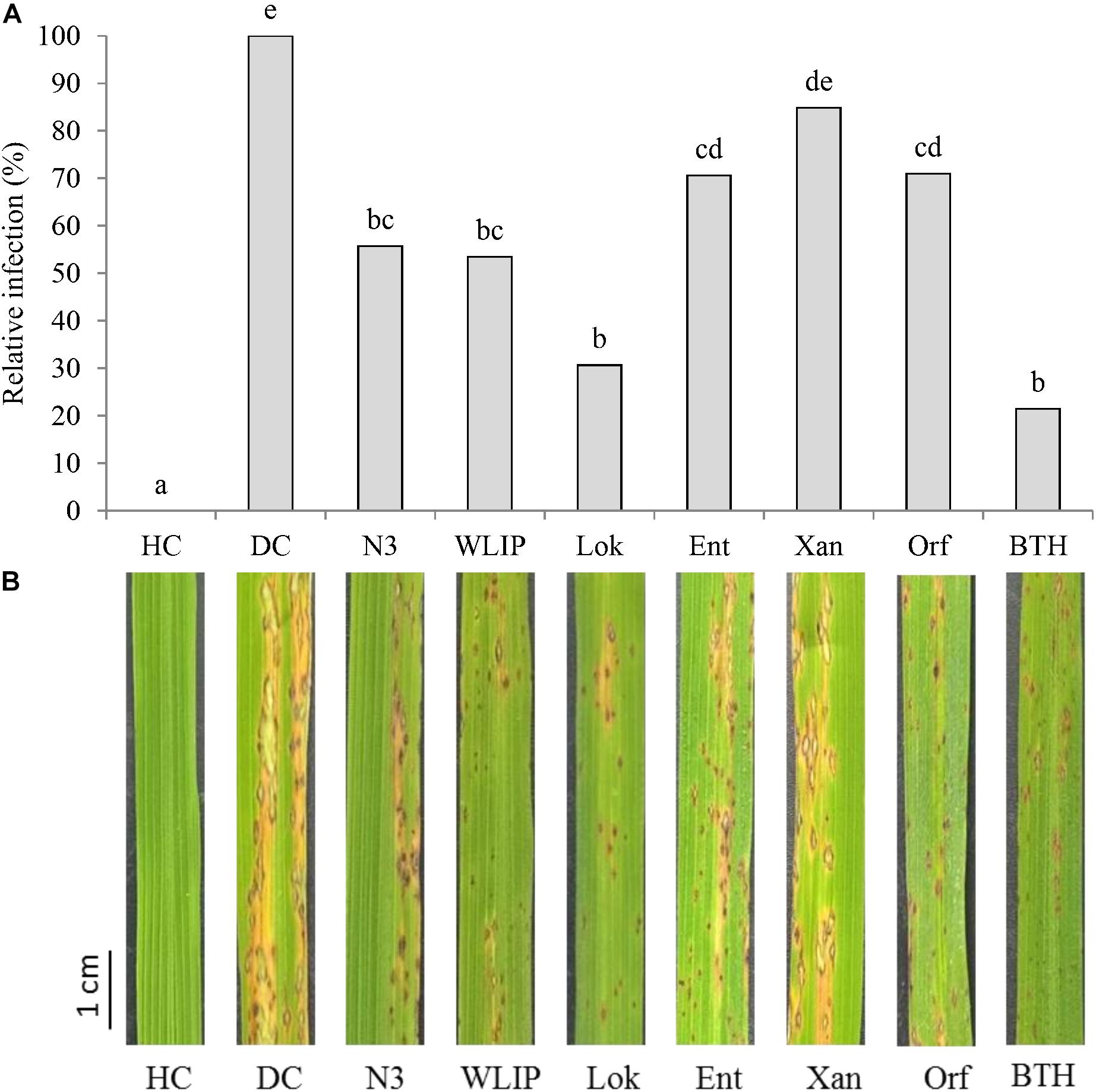
Figure 4. Effects of crude CLP extracts co-applied with M. oryzae spores on rice leaves on rice blast disease. (A) Effects of co-application of N3, WLIP, lokisin, entolysin, xantholysin, and orfamide extracts with fungal spores on rice blast caused by M. oryzae. Disease assessment was done at 6 days post-inoculation by counting the number of sporulating susceptible-type lesions occurring on the second youngest leaf of the rice plants. Data of one experiment made up of three biological replicates, consisting of 12 plants each are shown. The experiment was repeated with comparable results. Different letters indicate statistically significant differences among treatments (ANOVA followed by a Tukey’s tests: n = 36; α = 0.05). (B) Representative disease symptoms on second youngest leaf after 6 days post-inoculation with M. oryzae. Scale bar represents 1 cm. DC, disease control; HC, healthy control; Lok, lokisin; Ent, entolysin; Xan, xantholysin; Orf, orfamide; BTH, S-methyl 1,2,3-benzothiadiazole-7-carbothioate.
Effect of Crude CLP Extracts on M. oryzae Spore Germination and Appressoria Formation
Under in vitro conditions, the direct effect of N3, WLIP, lokisin, entolysin, xantholysin, and orfamide extracts on M. oryzae VT5M1 spore germination and appressoria formation was investigated. Since orfamide was effective against the same pathogen during a previous study (Ma et al., 2016a), we included it in our study as a positive control. Orfamide and xantholysin extracts had no effect on the spore germination capacity of M. oryzae when compared to the DMSO control. Significant differences in spore germination relative to the DMSO control were observed for N3 (82%) and WLIP (70%) at 25 μg/ml, for lokisin at 5 μg/ml (80%) and for entolysin at 1 (80%) and 10 μg/ml (82%) (Supplementary Figure S1). However, all CLPs tested could inhibit appressoria formation at 10 and 25 μg/ml (Figure 5A). Relative appressoria formation is in the following order, WLIP (24%) > N3/orfamide (36%) > lokisin (48%) > xantholysin (55%) > entolysin (59%). Representative pictures showing the effect of crude lokisin on appressoria formation are shown in Figure 5B.
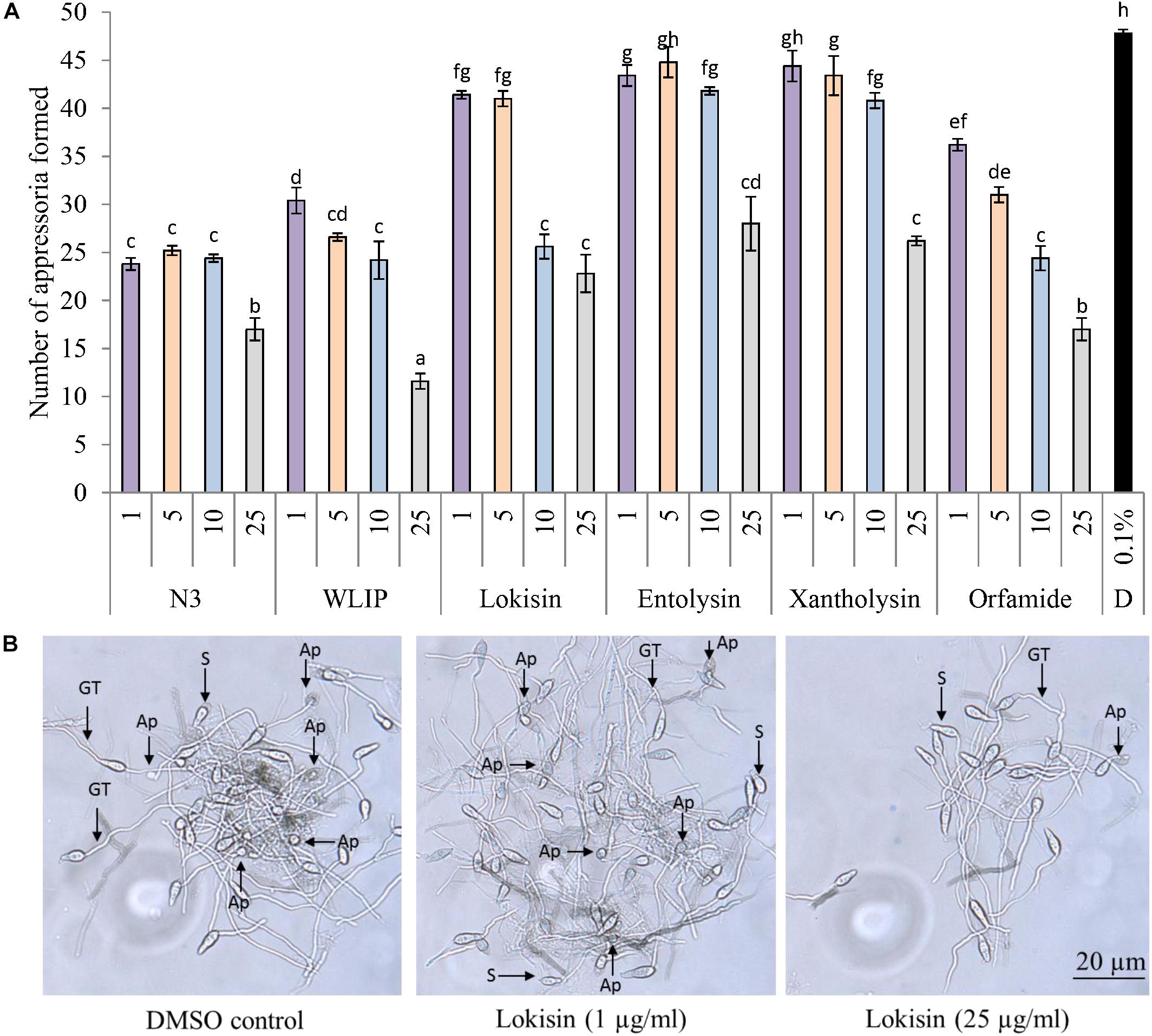
Figure 5. Direct effects of crude CLP extracts on appressoria formation in M. oryzae. (A) Direct effect of structurally diverse CLPs (1 – 25 μg/ml) on the formation of appressoria by M. oryzae VT5M1. Data shown are representative of two independent experiments. Different letters indicate statistically significant differences among the different treatments (ANOVA followed by a Tukey’s tests; α = 0.05), D: dimethyl sulfoxide (DMSO). (B) Representative pictures showing appressoria formation by M. oryzae VT5M1 in 0.1% DMSO and lokisin after 8 h of incubation. Ap, appressoria; GT, germ tube; S, spore. Scale bar represents 20 μm.
In vitro Antagonism of Xantholysin-Producing Strains
In a previous study, crude xantholysin extract from P. mosselii BS011 significantly reduced blast severity when mixed with M. oryzae Guy11 and sprayed on rice plants (Wu et al., 2018). However, crude xantholysin from COR51 could not protect rice plants against M. oryzae following treatment with its spores-crude CLPs mixture. To further investigate the discrepancy with our results related to xantholysin and the report by Wu et al. (2018), we co-cultured M. oryzae VT5M1 and M. oryzae Guy11 with xantholysin-producing strains COR51 and BW11M1. P. mosselii BW11M1 is closely related to P. mosselii BS011 strain used by Wu et al. (2018), and the NRPS clusters encoding xantholysin of both strains are highly similar. M. oryzae Guy11 was more sensitive to COR51 than VT5M1, but the growth of both fungi was not completely inhibited. In contrast, BW11M1 strongly inhibited the growth of both fungi (Supplementary Figure S2). These observations warrant further investigation, but indicate that the strong antagonism caused by BW11M1 is probably not only due to xantholysin.
Genome Mining for NRPS Genes Encoding Lokisin
Genome mining carried out on the draft genome of the lokisin-producing Pseudomonas sp. strain COR10 showed the presence of three NRPS genes (Figure 6A). Integration of results obtained by submitting the NRPS gene cluster to the NRPSpredictor2, AntiSMASH 4.0, BLAST search and A domain analyses revealed the capacity to synthesize a CLP consisting of 11 AAs, with a peptide sequence identical to that of lokisin, produced by Pseudomonas sp. strain DSS41 (Sørensen et al., 2002) and anikasin produced by P. fluorescens HKI0770 (Götze et al., 2017) (Supplementary Table S2) and in agreement with the chemical structure elucidation reported for COR10 by Oni et al. (2019a). As the AA stereochemistry is not fully resolved for DSS41 lokisin (Sørensen et al., 2002) and not yet determined for COR10 lokisin, it can at present not be assessed unequivocally whether lokisin and anikasin (same lipopeptide sequence, absolute configuration determined; Götze et al., 2017) are different CLPs. The three NRPS genes of Pseudomonas sp. COR10 designated as lokA, lokB, and lokC, encode two, four, and five AAs respectively (Figure 6A). The two terminal thioesterase (TE) domains release the peptides from the NRPSs with concomitant cyclization. The NRPS genes are flanked by a nodT-like outer membrane lipoprotein gene, which we termed lokT and a luxR-type transcriptional regulator gene, which we termed lokR, upstream, and two transporter genes which are predicted to encode macrolide efflux proteins MacA and MacB, downstream. There is no luxR-type transcriptional regulator gene in the downstream region of the lokisin NRPS cluster (Figure 6A).
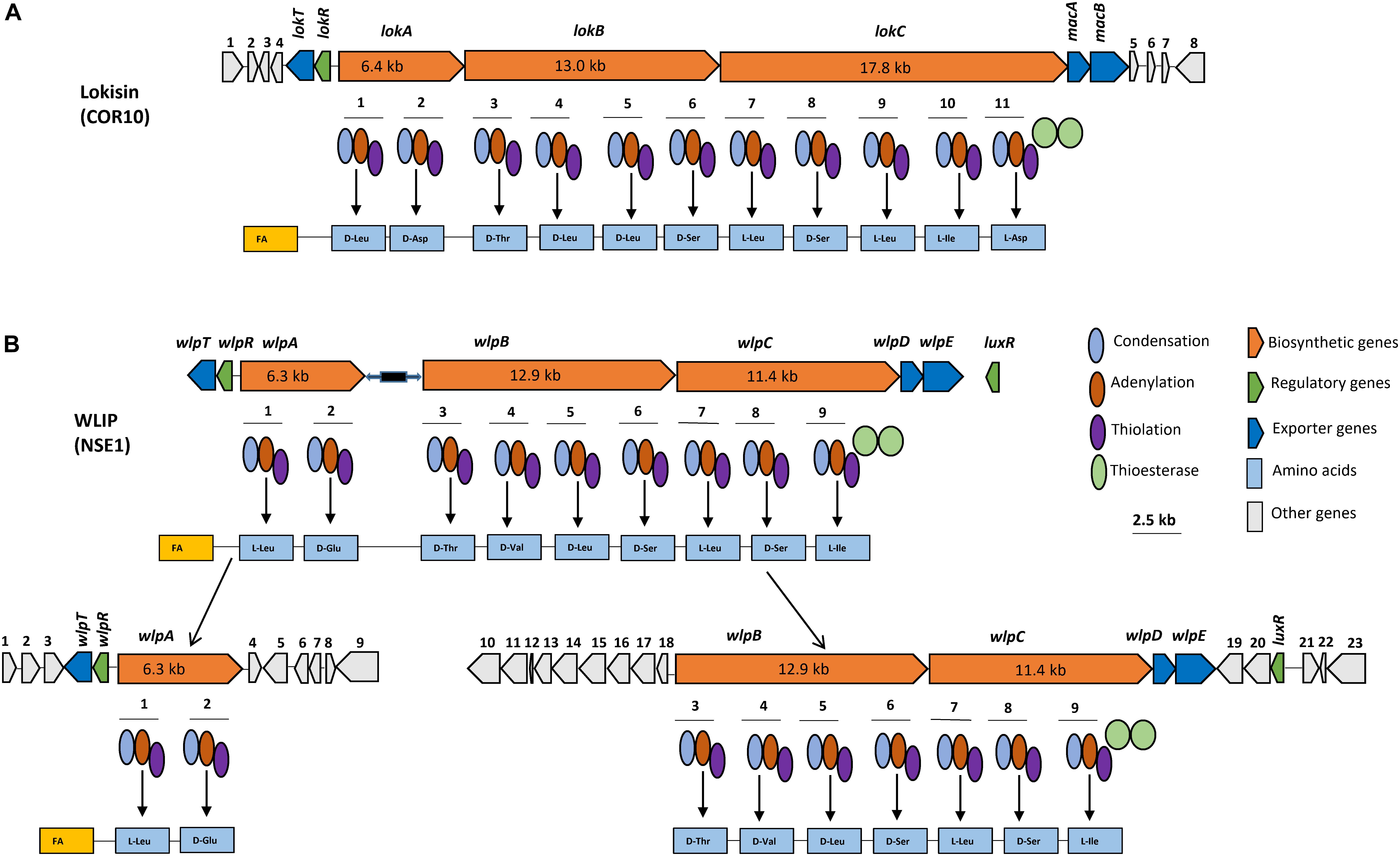
Figure 6. In silico analyses of NRPS gene clusters encoding WLIP and lokisin. (A) Gene clusters comprising three structural NRPS genes, designated lokA, lokB, and lokC responsible for lokisin biosynthesis in COR10. Detailed comparison of lokisin-synthetases and proteins encoded by the flanking regions are shown in Supplementary Table S3. (B) Gene cluster comprising three structural genes, designated wlpA, wlpB, and wlpC responsible for WLIP biosynthesis in NSE1. Detailed comparison of WLIP-synthetases and proteins encoded by the flanking regions are shown in Supplementary Table S4. Each module contains an adenylation (A), condensation (C) and thiolation (T) domains, and two TE domains for peptide release and cyclization. Scale bar represents 2.5 kb.
The genomic sequence of lokisin-producing strain Pseudomonas sp. strain DSS41 is not available in the GenBank database for comparative in silico analysis. However, BLASTp comparison showed a high identity of COR10 synthetases with arthrofactin synthetase of Pseudomonas sp. MIS38 (90–97%) and putative NRPS proteins of Pseudomonas sp. URIL14HWK12:I6 (97%), P. koreensis P19E3 (94–96%), P. koreensis BS3658 (86–93%), P. koreensis CRS05-R5 (86–92%), Pseudomonas sp. GM30 (90–96%), and Pseudomonas sp. Irchel s3a10 (90–97%). A somewhat lower identity was found with the anikasin synthetases of P. fluorescens HKI0770 (76–88%) and NRPS proteins in P. chlororaphis EA105 (77–83%), but the efflux and regulatory proteins in these last two strains showed a high identity with LokR (90%), MacA and Mac B (91%) of COR10 (Supplementary Table S3). NRPS A domains retrieved from these Pseudomonas strains were used to construct a phylogenetic tree, showing that strains URIL14HWK12:I6 and P19E3 are likely to produce lokisin. On the other hand, for strains GM30, BS3658, CRS05-R5 and Irchel s3a10 an isoleucine is predicted at the ninth position, similarly to the arthrofactin-producer MIS38, and they are probably also arthrofactin producers. The NRPS of EA105 would also recruit an isoleucine at position 9, but is predicted to incorporate a glutamine at the eighth position instead of the serine which is present in lokisin and arthrofactin, indicating that this strain may produce a novel member within the amphisin family (Supplementary Figure S3 and Supplementary Table S2).
The predicted D- or L- configuration of the AA residues of lokisin and other putative amphisin group CLPs based on C-domain phylogeny is shown (Supplementary Table S2 and Supplementary Figure S4). Although chemical validation is required to confirm the predicted stereochemistry, it can serve as a reliable clue to elucidate the configuration chemically. The lokisin biosynthetic gene cluster and flanking regions of Pseudomonas sp. COR10 have been deposited in Genbank with accession number MK534107.
Genome Mining for NRPS Genes Encoding WLIP
Genome mining carried out on the draft genome of Pseudomonas sp. NSE1 showed three NRPS genes. Combined analyses by NRPSpredictor2, AntiSMASH 4.0, BLAST search and A domain analyses revealed synthetic capacity for a CLP comprising nine AAs, apparently identical to WLIP produced by Pseudomonas putida RW10S2 (Supplementary Table S5). WLIP synthetase of Pseudomonas sp. NSE1 is made up of three NRPS genes designated as wlpA, wlpB, and wlpC, which incorporate two, four, and three AAs respectively (Figure 6B). The biosynthetic gene cluster is flanked by a nodT-like outer membrane lipoprotein gene (wlpT) and a luxR-type transcriptional regulator gene (wlpR) upstream of wlpA, and two transporter genes (wlpD and wlpE) which encode putative macrolide efflux protein MacA and MacB downstream of wlpC (Figure 6B). Furthermore, BLASTp searches of the NRPS cluster of Pseudomonas sp. NSE1 revealed as high identity with the corresponding proteins in Pseudomonas sp. RW10S2 (94–96%), and an even higher identity (99%) with Pseudomonas putida NX-1 (Xu et al., 2018) and Pseudomonas putida PC2 (Song et al., 2017) (Supplementary Table S4). In line with this, A-domain phylogenetic analysis (Supplementary Figure S3) suggests that Pseudomonas spp. NSE1, NX-1 and PC2 are WLIP producers. The result showed the same AAs as the previously reported WLIP producer, RW10S2, were recruited by our strain, NSE1, together with NX-1 and PC2 strains (Supplementary Table S5 and Supplementary Figure S5). The predicted isomery (D and L configurations) of the AAs sequences of WLIP based on C-domain phylogeny are shown (Supplementary Table S5 and Supplementary Figure S6). The predicted chemical isomery could offer a clue to elucidating the chemical configuration for validation purpose. The WLIP biosynthetic gene cluster and flanking regions of Pseudomonas sp. NSE1 have been deposited in GenBank with accession numbers MK534106 and MK650230.
Phylogenetic Analysis of Pseudomonas spp. Based on rpoD and rpoB Gene Sequences
Results from the phylogenetic analyses using rpoD and rpoB partial sequences of CLP-producing Pseudomonas strains show that lokisin-producing strains and arthrofactin-producing strains clustered with putative lokisin and putative arthrofactin producers, respectively. These strains all belong to the P. koreensis group and cluster with the type strain P. koreensis LMG21318T. Our results also suggest that P. chlororaphis EA105 and P. fluorescens HKI0770 are closely related to the P. koreensis group. It is clear that P. chlororaphis EA105 does not cluster with the type strain P. chlororaphis LMG 1245T. Our result also show that the WLIP-producers Pseudomonas sp. NSE1 and COW10 and potential WLIP-producing strains NX-1 and PC2 are closely related and also form a cluster with the WLIP-producer RW10S2. The xantholysin producing strain COR51 clustered separately from two other xantholysin producers, BW11M1 and BS011 (Figure 7). The phylogenetic analysis of the housekeeping gene (rpoD and rpoB) is in agreement with the BLASTp comparison and phylogenetic analysis of the A domains from the CLPs biosynthetic genes.
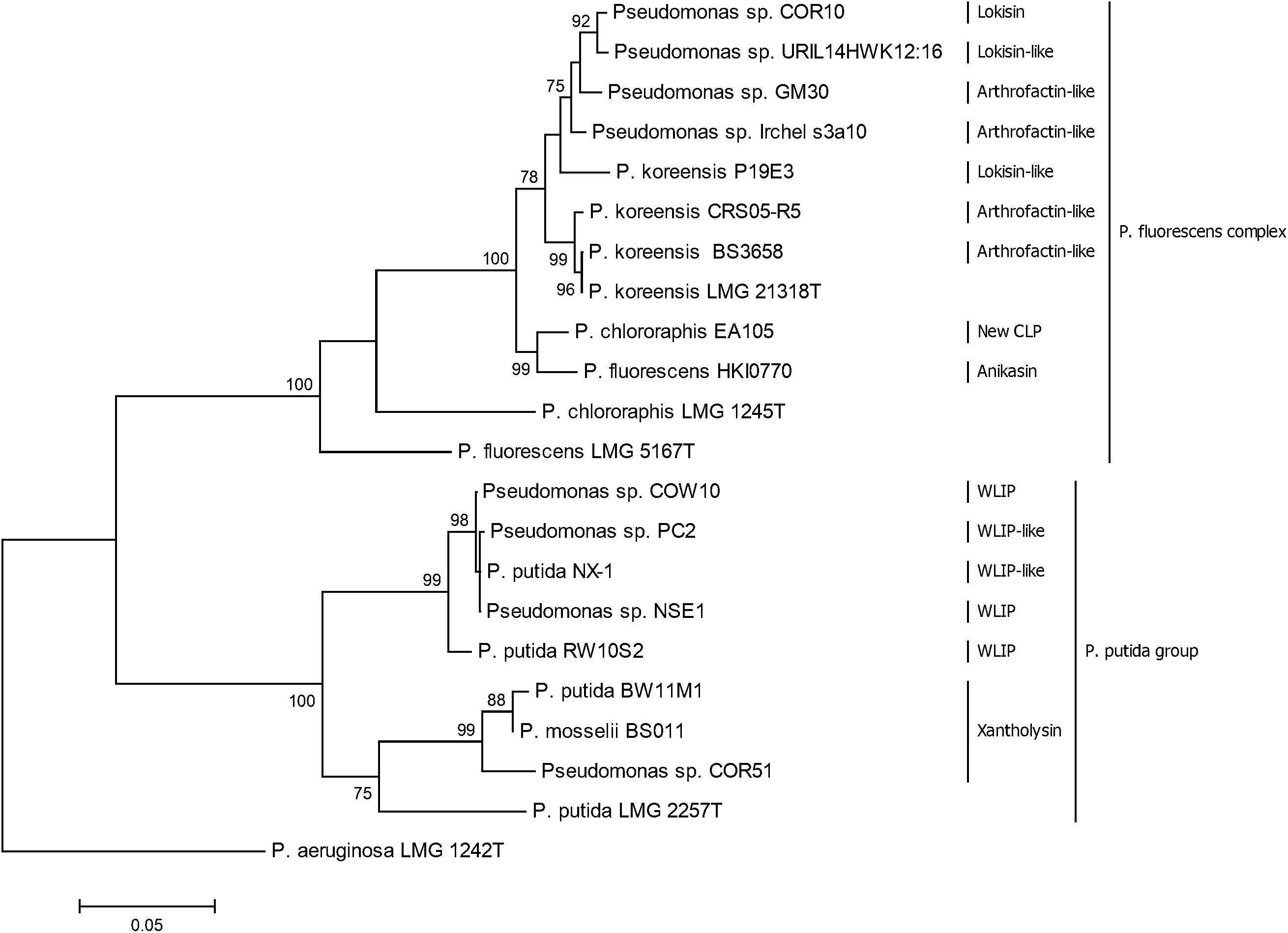
Figure 7. Phylogenetic analysis of rpoD and rpoB partial sequences of Pseudomonas strains used in this study. The tree was constructed with MEGA6 using the Maximum Likelihood Method with 1000 bootstrap replicates. Only bootstrap values above 70% are indicated.
Discussion
In this study, we investigated the potential of previously characterized Pseudomonas strains and their CLPs (Oni et al., 2019a; Geudens, 2017) to control M. oryzae on rice by ISR and direct contact. A summary of the results is given in Table 4. Upon root inoculation Pseudomonas strains producing the CLPs N3, lokisin, WLIP and entolysin successfully induced resistance to rice blast, while the xantholysin producer (COR51) did not. In addition, crude CLP extracts obtained from these strains were applied to the rice plant by a soil drench. Lokisin-, WLIP- and entolysin-drenched rice plants successfully induced resistance to the blast disease. However, treatments involving crude extracts of N3 and xantholysin were not effective. These data suggest that the ISR-inducing capacity of Pseudomonas strains COR10, COW10 and COR5 is caused by their respective CLPs lokisin, WLIP and entolysin. The CLP xantholysin is not able to trigger ISR against rice blast, while the CLP N3 does not seem to be responsible for the observed ISR in strain COW3. In the case of WLIP, we could further validate the role of this CLP in induced resistance by using the WLIP-producing strain P. putida RW10S2 and its WLIP-deficient mutants that were generated previously (Rokni-Zadeh et al., 2012). The wild type strain induced resistance to rice blast to a similar extent as WLIP-producing strains COW10 and NSE1. In contrast, plants treated with all three WLIP mutant strains, were as susceptible to rice blast as the disease control plants.
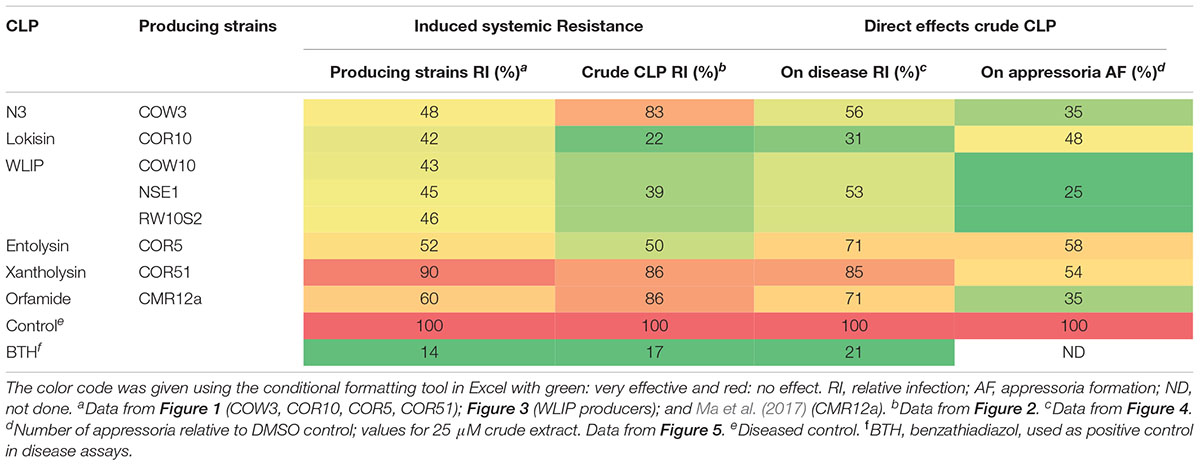
Table 4. Summarizing table showing the potential of Pseudomonas strains and their CLPs to control M. oryzae on rice by induced systemic resistance and direct effects.
Our study presents the first report of the capacity of CLP-producing Pseudomonas strains to elicit ISR against rice blast. Pseudomonas CLPs have been shown before to be bacterial determinants of ISR. Massetolide, a CLP related to WLIP, was active in tomato against Phytophthora infestans (Tran et al., 2007), while orfamide triggered ISR in bean against web blight caused by Rhizoctonia solani (Ma et al., 2016b) and in rice against the brown spot pathogen Cochliobolus miyabeanus (Ma et al., 2017). The orfamide-producing P. protegens strain CHA0, however, was unable to trigger ISR against rice blast (Spence et al., 2014). In addition, we showed that purified orfamide does not elicit ISR against rice blast which confirms results obtained in earlier studies (Ma et al., 2017). The orfamide-producing Pseudomonas sp. strain CMR12a however, is able to induce resistance to rice blast, but mutant analysis has revealed that phenazines rather than orfamide are responsible for the observed ISR (Ma et al., 2017). It has been shown that P. chlororaphis EA105, an isolate obtained from rice roots in California, can induce systemic resistance against rice blast and triggers jasmonate- and ethylene-dependent defense responses (Spence et al., 2014), but the bacterial determinants involved in ISR were not elucidated. Interestingly, our bioinformatics analysis has revealed that strain EA105 is likely to produce a novel CLP of the amphisin group that is related to lokisin (Supplementary Table S2 and Supplementary Figure S3). It is tempting to speculate that this CLP could play a role in the ISR observed by Spence et al. (2014). In this context it should be noted that Pseudomonas sp. GM30, an isolate obtained from Populus deltoides roots in the US, is able to trigger ISR against P. syringae in Arabidopsis thaliana and induces the jasmonate marker PDF1.2 (Weston et al., 2012). We showed that this strain is a putative arthrofactin-producer (Supplementary Table S2 and Supplementary Figure S3). P. koreensis CRS05-R5, another putative arthrofactin producer (Supplementary Table S2 and Supplementary Figure S3), was isolated from rice roots in China (Lin et al., 2016). These results suggest that amphisin group CLPs are produced by Pseudomonas isolates associated with the rice rhizosphere and it is worth investigating whether not only lokisin, but also other amphisin group CLPs are ISR elicitors. However, the capacity of CLPs to induce systemic resistance is structure, plant and pathogen dependent. It remains to be investigated whether xantholysin or N3 can act as a resistance inducer in other plant–pathogen combinations.
In addition to ISR, we tested the crude CLP extracts for their capacity to control M. oryzae when co-applied with fungal spores on rice leaves. We observed a significant reduction in the number of susceptible blast lesions following treatment of M. oryzae VT5M1 spores with 25 μg/ml of each CLP prior to pathogen application on rice leaves, with the exception of xantholysin. Similar observations were observed in a recent study in which up to 50 μM of orfamides could directly reduce rice blast severity after pre-treatment of M. oryzae spores prior to foliar inoculation (Ma et al., 2016a). In contrast, 25 μg/ml of crude xantholysin did not protect rice against blast disease. Our findings contradict a previous study which recorded a significant reduction of rice blast severity after spraying rice leaves with 10 and 25 μg/ml of a crude xantholysin extract (obtained from P. mosselii BS011) mixed with M. oryzae Guy11 spores (Wu et al., 2018). This may be explained by differences in sensitivity toward xantholysin between M. oryzae isolates or differences in the bacterial strains used. Wu et al. (2018) observed a strong antagonism between P. mosselii BS011 and M. oryzae Guy11 in plate assays. We did some preliminary antagonistic tests with M. oryzae VT5M1 and M. oryzae Guy11 using COR51 and P. putida BW11M1, a strain very similar to BS011. Wu et al. (2018) have reported that the xantholysin cluster in BS011 is nearly identical to the cluster in BW11M1 and Figure 7 shows that both strains are also taxonomically closely related. Guy11 is more strongly repressed by COR51 than VT5M1. Moreover, BW11M1 can completely inhibit the growth of both fungi, while this is not the case for COR51 (Supplementary Figure S2). These observations clearly warrant further investigation. In a comparative analysis of the genomes of field isolates of M. oryzae it was shown that the field isolates have hundreds of isolate-specific genes, some of which affect virulence (Xue et al., 2012). Thus, possible genetic and physiological differences between M. oryzae VT5M1 and M. oryzae Guy11 may further account for the different observations in the bioactivity of xantholysin. Besides, it is plausible that different Pseudomonas strains produce different amounts of CLPs and in different proportions of congeners. Since the crude CLP extracts used in these studies were not directly quantified, these two studies are not exactly comparable. Future studies using purified CLPs will shed more light on the differences in level of bioactivity among structurally different or similar CLPs.
By means of appressoria, M. oryzae penetrates the rice cuticles through turgor pressure generation (Wilson and Talbot, 2009). The direct effect of crude CLPs against spore germination and appressoria formation in M. oryzae were tested under in vitro conditions. Microscopic examination revealed that there was no difference in spore germination between CLP treatments and the DMSO control. This result is consistent with earlier reports about the effect of orfamide (Ma et al., 2016a) and xantholysin (Wu et al., 2018) on spore germination in M. oryzae. In our study, all CLPs tested significantly inhibited appressoria formation at concentrations ranging from 10 to 25 μg/ml. Previous studies showed that purified orfamide A, produced by P. protegens CHA0, inhibited appressoria formation at 10 and 50 μM (Ma et al., 2016a). A similar effect on appressoria formation was reported for crude xantholysin produced by P. mosselii BS011 when tested against M. oryzae at 25 μg/ml (Wu et al., 2018). Another study showed the direct inhibition of appressoria formation in M. oryzae by Pseudomonas sp. EA105 and P. protegens CHA0 (Spence et al., 2014). However, the role of CLPs in the inhibition of appressoria by these bacteria was not investigated. As discussed above EA105 likely produces an amphisin-type CLP (this work) while CHA0 is an orfamide producer (Ma et al., 2017). The obstruction of appressoria formation in M. oryzae can efficiently lower blast severity on rice plants (Liu et al., 2011; Ma et al., 2016a). Additionally, previous studies showed the inhibition of appressoria formation by orfamides on rice leaves (Ma et al., 2016a) and by mannosylerythritol lipids and Tween 20 on a hydrophobic surface (Yoshida et al., 2015). Yoshida et al. (2015) hypothesized that the inhibition of appressoria formation by M. oryzae may be facilitated by changes in surface hydrophobicity due to prior application of surfactants. In our study, we found that M. oryzae VT5M1 produces fewer appressoria on microscopic glass slides in comparison with microscopic plastic slides (data not shown). Similar studies showed the capacity of M. grisea Guy11 to form appressoria on plastic cover slips but not on glass slides (Skamnioti and Gurr, 2007).
Lokisin was first isolated from Pseudomonas strain DSS41 and is a member of the amphisin class of CLPs (Nielsen et al., 2002; Sørensen et al., 2002). In previous studies on the biological activity of CLP-producing strains, whole cells of lokisin-producing Pseudomonas sp. DSS41 strain showed antagonistic activity against Pythium ultimum and Rhizoctonia solani in in vitro tests (Nielsen et al., 2002). Also lokisin obtained from P. koreensis 2.74 showed biocontrol against P. ultimum in a hydroponic tomato cultivation system (Hultberg et al., 2010), while our lokisin-producing COR10 isolate is antagonistic against P. myriotylum on cocoyam (Oni et al., 2019a). Till date, the biosynthetic gene cluster of lokisin is yet to be described. Mining of the draft genome of Pseudomonas sp. COR10 revealed the presence of three clustered NRPS genes, lokA, lokB and lokC, which are responsible for lokisin biosynthesis in Pseudomonas sp. COR10, conforming with the “colinearity rule.” Results of our in silico analyses are in congruence with the chemical analysis of lokisin earlier published for Pseudomonas sp. COR10 (Oni et al., 2019a). Similar to most CLP gene clusters, the NRPS genes of lokisin are flanked upstream by nodT-like and luxR-type genes designated lokT and lokR, respectively. More so, genes encoding the macrolide export protein (MacA) and the ABC transporter (MacB) are located downstream of the lokABC genes. In contrast to most CLPs, there is no luxR transcriptional regulator downstream of the lokisin NRPS cluster (Figure 6A). This is a rare occurrence in CLP-producing Pseudomonas strains. For single CLP-producing strains, the absence of a luxR transcriptional regulator downstream of the NRPS cluster is noticeable in the entolysin biosynthetic cluster (Vallet-Gely et al., 2010), in the xantholysin biosynthetic clusters of strain BW11M1 (Li et al., 2013) and the closely related strain BS011 (Wu et al., 2018). In the dual CLP producer Pseudomonas sp. CMR12a, a luxR regulator was found to be lacking in the downstream position of one of the two CLP gene clusters present (D’aes et al., 2014). Anikasin produced by P. fluorescens HKI0770, an isolate obtained from forest soil in Germany (Götze et al., 2017) has the same AAs sequence as lokisin. The predicted D/L-configuration of AAs in COR10 lokisin (to be chemically validated), does also not deviate from the absolute configuration of anikasin. Hence, it cannot be excluded that strains HKI0770 and COR10 produce the same CLP. Both strains appear to be taxonomically related, since P. fluorescens HKI0770 also clusters with the P. koreensis group (Figure 7). However, significant divergence of the respective NRPS sequences is evident and the genomic context of the biosynthetic gene clusters differs between these strains (Supplementary Table S3 and Supplementary Figure S3).
Unfortunately, we were not able to study the entolysin biosynthetic gene cluster of Pseudomonas sp. COR5 and the xantholysin cluster in Pseudomonas sp. COR51 in detail because the available genome sequences were very fragmented. We previously showed that Pseudomonas sp. COR5 has strong biocontrol activity against P. myriotylum on cocoyam (Oni et al., 2019a), while pure entolysin caused hyphal leakage of P. myriotylum at concentrations of 25 and 50 μM (Oni et al., 2019a). The NRPS cluster of entolysin has been described for P. entomophila L48 (Vallet-Gely et al., 2010). This strain was lethal for insects when ingested at high dose and further showed biocontrol ability against Pythium ultimum. However, mutant analysis revealed that its virulence against insects and biocontrol activity were not linked to entolysin production. We found various entolysin producers in the rhizosphere of cocoyam in Cameroon, and these strains, including COR5 belong to the P. putida group and are taxonomically related to P. entomophila L48. To our knowledge, so far no other entolysin-producing bacteria have been described.
In this study, the role of WLIP in induced resistance to M. oryzae was established using the rice rhizosphere isolate, RW10S2, and its WLIP mutants. The anti-Xanthomonas activity of rice-rhizospheric Pseudomonas putida RW10S2 has been attributed to WLIP production (Rokni-Zadeh et al., 2012). In addition, other WLIP-producing strains were tested including the cocoyam rhizosphere isolate, NSE1. Pseudomonas sp. NSE1 showed similar ISR results as RW10S2. Considering the fact that the WLIP biosynthetic machinery of the RW10S2 has been fully elucidated (Rokni-Zadeh et al., 2012), it was interesting to investigate if NSE1 possesses a similar WLIP system as that of RW10S2. We identified the NRPS genes, wlpA, wlpB and wlpC which are responsible for WLIP biosynthesis by Pseudomonas sp. NSE1. Similar to RW10S2 (Rokni-Zadeh et al., 2013), wlpA and wlpB-wlpC are located in distant NRPS clusters (Figure 6B). A similar split organization is found for entolysin (Vallet-Gely et al., 2010), xantholysin (Li et al., 2013), viscosin (De Bruijn and Raaijmakers, 2009), and poaeamide (Zachow et al., 2015). This is contrary to most CLPs which have three biosynthetic genes in the same NRPS cluster, including lokisin (this study), anikasin (Götze et al., 2017), bananamide (Nguyen et al., 2016), arthrofactin (Roongsawang et al., 2003), putisolvin (Dubern et al., 2008), orfamide (Ma et al., 2016a), and gacamide (Jahanshah et al., 2019) among others. WLIP-producing Pseudomonas species involved in this study were isolated from diverse ecologies in various countries; rhizosphere of crops (cocoyam and rice) in tropical rain forests of Cameroon (COW10), Nigeria (NSE1), and Sri Lanka (RW10S2). Potential WLIP-producing strains, PC2 and NX-1, isolated from subtropical monsoon climates in China, were obtained from Pistacia chinensis and leaf mold, respectively (Supplementary Table S1). This suggests that WLIP is likely found in various ecologies and can probably be named as a core CLP which plays specific roles within the producing strains in their associated ecologies.
The CLPs that we used in our study are structurally diverse and show variation in length of fatty acid tail, number of AAs in the entire oligopeptide length and the size of the macrocyclic lactone ring. At this moment it is not clear which parts of the CLP molecule determine biological activity. All the CLPs tested in this study have a similar 3-hydroxy C10 fatty acid tail except for orfamide A which has a 3-OH C14. However, xantholysin and orfamide, two CLPs that do not elicit ISR against rice blast, differ from the bioactive CLPs (lokisin, WLIP and entolysin) by the presence of eight AA in the peptide ring (Table 5). This relationship is worth exploring in future studies. We recently elucidated the structure of N3 and revealed that it is a novel member of the bananamide group with eight AAs, 6 of which are present in the peptide ring. The full structural elucidation and genome organization of N3 will be published elsewhere.
Correct taxonomic assignment of CLP-producing Pseudomonas species bacteria has been highlighted as a useful clue to identify known and novel CLPs (Oni et al., 2019a). In this study, the taxonomic affiliation of the WLIP-, lokisin-, and xantholysin-producing strains was further established by phylogenetic analysis using partial sequences of rpoD and rpoB genes. This taxonomic clustering based on housekeeping genes is in congruence with clustering of corresponding A domains and protein sequences for the WLIP and lokisin gene clusters. Our results confirm that of Oni et al. (2019a) and show that a clear taxonomic designation of a CLP-producing Pseudomonas strain could serve as a clue for CLP prediction.
Conclusion
Our study demonstrates the capacity of WLIP-, lokisin-, and entolysin-producing Pseudomonas strains to control the rice blast disease caused by M. oryzae by ISR and direct effects. The CLPs orfamide and N3 are unable to mount ISR against rice blast but can control the pathogen when co-inoculated together on rice leaves. Additionally, we described the WLIP biosynthetic gene cluster for Pseudomonas sp. NSE1 and the lokisin biosynthetic gene cluster for Pseudomonas sp. COR10. Our results highlight the biological activity potential of structurally diverse CLPs. Future research is aimed at unraveling the mechanisms by which CLPs trigger defense responses and further studying structure/function relationships in Pseudomonas CLPs.
Data Availability
The datasets generated for this study are available in Genbank, MK534107, MK534106, and MK650230.
Author Contributions
MH, FO, and OO conceived and designed the research. RD supplied RW10S2 and BW11M1, and their corresponding mutants. OO, HB, and HY conducted the plant experiments. FO isolated Pseudomonas sp. NSE1 and prepared DNA materials for genome sequencing. OO performed the rest of the experiments, in silico analyses, and analyzed the data. OO and FO wrote the manuscript. MH and RD revised the manuscript and helped in structuring the work. All authors revised the manuscript and approved the final version for submission.
Funding
OO acknowledges funding from the INTERREG France-Wallonie-Vlaanderen Program SmartBiocontrol, and the Tertiary Education Trust Fund (TETFUND) by the Federal Government of Nigeria. FO was funded by the Schlumberger Faculty for the Future Ph.D. Fellowship and the EOS grant 30650620. CLP research in the laboratory of MH was supported by the INTERREG SMARTBIOCONTROL Program. CLP research in the labs of RD and MH was also supported by the EOS grant 30650620.
Conflict of Interest Statement
The authors declare that the research was conducted in the absence of any commercial or financial relationships that could be construed as a potential conflict of interest.
Supplementary Material
The Supplementary Material for this article can be found online at: https://www.frontiersin.org/articles/10.3389/fpls.2019.00901/full#supplementary-material
Footnotes
References
Aziz, R. K., Bartels, D., Best, A., DeJongh, M., Disz, T., Edwards, R. A., et al. (2008). The RAST server: rapid annotations using subsystems technology. BMC Genomics 9:75. doi: 10.1186/1471-2164-9-75
Blin, K., Wolf, T., Chevrette, M. G., Lu, X., Schwalen, C. J., Kautsar, S. A., et al. (2017). AntiSMASH 4.0-improvements in chemistry prediction and gene cluster boundary identification. Nucleic Acids Res. 45, W36–W41. doi: 10.1093/nar/gkx319
Boetzer, M., Henkel, C. V., Jansen, H. J., Butler, D., and Pirovano, W. (2011). Scaffolding pre-assembled contigs using SSPACE. Bioinformatics 27, 578–579. doi: 10.1093/bioinformatics/btq683
Boetzer, M., and Pirovano, W. (2012). Toward almost closed genomes with GapFiller. Genome Biol. 13:R56. doi: 10.1186/gb-2012-13-6-r56
Boutrot, F., and Zipfel, C. (2017). Function, discovery, and exploitation of plant pattern recognition receptors for broad-spectrum disease resistance. Annu. Rev. Phytopathol. 55, 257–286. doi: 10.1146/annurev-phyto-080614-120106
D’aes, J., De Maeyer, K., Pauwelyn, E., and Höfte, M. (2010). Biosurfactants in plant-Pseudomonas interactions and their importance to biocontrol. Environ. Microbiol. Rep. 2, 359–372. doi: 10.1111/j.1758-2229.2009.00104.x
D’aes, J., Kieu, N. P., Léclère, V., Tokarski, C., Olorunleke, F. E., De Maeyer, K., et al. (2014). To settle or to move? The interplay between two classes of cyclic lipopeptides in the biocontrol strain Pseudomonas CMR12a. Environ. Microbiol. 16, 2282–2300. doi: 10.1111/1462-2920.12462
De Bruijn, I., and Raaijmakers, J. M. (2009). Diversity and functional analysis of LuxR-type transcriptional regulators of cyclic lipopeptide biosynthesis in Pseudomonas fluorescens. Appl. Environ. Microbiol. 75, 4753–4761. doi: 10.1128/AEM.00575-09
De Vleesschauwer, D., Cornelis, P., and Höfte, M. (2006). Redox-active pyocyanin secreted by Pseudomonas aeruginosa 7NSK2 triggers systemic resistance to Magnaporthe grisea but enhances Rhizoctonia solani susceptibility in rice. Mol. Plant Microbe Interact. 19, 1406–1419. doi: 10.1094/MPMI-19-1406
De Vleesschauwer, D., and Höfte, M. (2009). Rhizobacteria-induced systemic resistance. Adv. Bot. Res. 51, 223–281. doi: 10.1016/s0065-2296(09)51006-3
Dean, R. A., Talbot, N. J., Ebbole, D. J., Farman, M. L., Mitchell, T. K., Orbach, M. J., et al. (2005). The genome sequence of the rice blast fungus Magnaporthe grisea. Nature 434, 980–986. doi: 10.1038/nature03449
Desai, S., Reddy, M., and Kloepper, J. (2002). “Chapter 17: comprehensive testing of biocontrol agents,” in Biological Control of Crop Diseases, ed. S. S. Gnanamanickam (New York, NY: Marcel Dekker), 387–420.
Dubern, J. F., Coppoolse, E. R., Stiekema, W. J., and Bloemberg, G. V. (2008). Genetics and functional characterization of the gene cluster directing the biosynthesis of putisolvin I and II in Pseudomonas putida strain PCL1445. Microbiology 154, 2070–2083. doi: 10.1099/mic.0.2008/016444-0
Edgar, R. C. (2004). MUSCLE: multiple sequence alignment with high accuracy and high throughput. Nucleic Acids Res. 32, 1792–1797. doi: 10.1093/nar/gkh340
Fageria, N. K., and Baligar, V. C. (2003). Methodology for evaluation of lowland rice genotypes for nitrogen use efficiency. J. Plant Nutr. 26, 1315–1333. doi: 10.1081/PLN-120020373
Finking, R., and Marahiel, M. A. (2004). Biosynthesis of non-ribosomal peptides. Ann. Rev. Microbiol. 58, 453–488.
Geudens, N. (2017). Insights in the Structure-Function and Membrane Interactions of Natural Cyclic Lipodepsipeptides. Ph.D. Thesis, Faculty of Science, Ghent University, Belgium.
Geudens, N., and Martins, J. C. (2018). Cyclic lipodepsipeptides from Pseudomonas spp. - biological swiss-army knives. Front. Microbiol. 9:1867. doi: 10.3389/fmicb.2018.01867
Götze, S., Herbst-Irmer, R., Klapper, M., Gorls, H., Schneider, K. R. A., Barnett, R., et al. (2017). Structure, biosynthesis, and biological activity of the cyclic lipopeptide anikasin. ACS Chem. Biol. 12, 2498–2502. doi: 10.1021/acschembio.7b00589
Gross, H., and Loper, J. E. (2009). Genomics of secondary metabolite production by Pseudomonas spp. Nat. Prod. Rep. 26, 1408–1446. doi: 10.1039/b817075b
Haas, D., and Défago, G. (2005). Biological control of soilborne pathogens by fluorescent pseudomonads. Nat. Rev. Microbiol. 3, 307–319. doi: 10.1038/nrmicro1129
Henry, G., Deleu, M., Jourdan, E., Thonart, P., and Ongena, M. (2011). The bacterial lipopeptide surfactin targets the lipid fraction of the plant plasma membrane to trigger immune-related defence responses. Cell. Microbiol. 13, 1824–1837. doi: 10.1111/j.1462-5822.2011.01664.x
Hernández-Rodríguez, A., Acebo-Guerrero, Y., Fernández, G. P., de la Osa, A. D., and Restrepo-Franco, G. M. (2018). Characterization of fluorescent Pseudomonas from Oryza sativa L. rhizosphere with antagonistic activity against Pyricularia oryzae (SACC.). Afr. J. Biotechnol. 17, 1196–1206. doi: 10.5897/ajb2018.16478
Höfte, M., and Altier, N. (2010). Fluorescent pseudomonads as biocontrol agents for sustainable agricultural systems. Res. Microbiol. 161, 464–471. doi: 10.1016/j.resmic.2010.04.007
Hultberg, M., Alsberg, T., Khalil, S., and Alsanius, B. (2010). Suppression of disease in tomato infected by Pythium ultimum with a biosurfactant produced by Pseudomonas koreensis. BioControl 55, 435–444. doi: 10.1007/s10526-009-9261-6
Jahanshah, G., Yan, Q., Gerhardt, H., Pataj, Z., Lämmerhofer, M., Pianet, I., et al. (2019). Discovery of the cyclic lipopeptide gacamide a by genome mining and repair of the defective gacA regulator in Pseudomonas fluorescens Pf0-1. J. Nat. Prod 82, 301–308. doi: 10.1021/acs.jnatprod.8b00747
Jourdan, E., Henry, G., Duby, F., Dommes, J., Barthélemy, J. P., Thonart, P., et al. (2009). Insights into the defense-related events occurring in plant cells following perception of surfactin-type lipopeptide from Bacillus subtilis. Mol. Plant Microbe Interact. 22, 456–468. doi: 10.1094/mpmi-22-4-0456
King, E. O., Ward, M. K., and Raney, D. E. (1954). Two simple media for the demostration of pyocyanin and fluorescin. J. Lab. Clin. Med. 44, 301–307.
Koga, H. (1994). Hypersensitivity death, autofluorescence, and ultrastructural-changes in cells of leaf sheaths of susceptible and resistant near-isogenic lines of rice [PI-Z(T)] in relation to penetration and growth of Pyricularia oryzae. Can. J. Bot. 72, 1463–1477. doi: 10.1139/b94-180
Leung, H., Borromeo, E. S., Bernardo, M. A., and Notteghem, J. L. (1988). Genetic analysis of virulence in the rice blast fungus Magnaporthe grisea. Phytopathology 78, 1227–1233.
Li, W., Rokni-Zadeh, H., De Vleeschouwer, M., Ghequire, M. G. K., Sinnaeve, D., Xie, G. L., et al. (2013). The antimicrobial compound xantholysin defines a new group of Pseudomonas cyclic lipopeptides. PLoS One 8:e62946. doi: 10.1371/journal.pone.0062946
Lin, H., Hu, S., Liu, R., Chen, P., Ge, C., Zhu, B., et al. (2016). Genome sequence of Pseudomonas koreensis CRS05-R5, an antagonistic bacterium isolated from rice paddy field. Front. Microbiol. 7:1756. doi: 10.3389/fmicb.2016.01756
Liu, W., Zhou, X., Li, G., Li, L., Kong, L., Wang, C., et al. (2011). Multiple plant surface signals are sensed by different mechanisms in the rice blast fungus for appressorium formation. PLoS Pathog. 7:e1001261. doi: 10.1371/journal.ppat.1001261
Ma, Z., Geudens, N., Kieu, N. P., Sinnaeve, D., Ongena, M., Martins, J. C., et al. (2016a). Biosynthesis, chemical structure, and structure-activity relationship of orfamide lipopeptides produced by Pseudomonas protegens and related species. Front. Microbiol. 7:382. doi: 10.3389/fmicb.2016.00382
Ma, Z., Hoang Hua, G. K. H., Ongena, M., and Höfte, M. (2016b). Role of phenazines and cyclic lipopeptides produced by pseudomonas sp. CMR12a in induced systemic resistance on rice and bean. Environ. Microbiol. Rep. 8, 896–904. doi: 10.1111/1758-2229.12454
Ma, Z., Ongena, M., and Höfte, M. (2017). The cyclic lipopeptide orfamide induces systemic resistance in rice to Cochliobolus miyabeanus but not to Magnaporthe oryzae. Plant Cell Rep. 36, 1731–1746. doi: 10.1007/s00299-017-2187-z
Mariutto, M., and Ongena, M. (2015). Molecular patterns of rhizobacteria involved in plant immunity elicitation. Adv. Bot. Res. 75, 21–56. doi: 10.1016/bs.abr.2015.07.002
Michelsen, C. F., Watrous, J., Glaring, M. A., Kersten, R., Koyama, N., and Dorrestein, P. C. (2015). Nonribosomal peptides, key biocontrol components for Pseudomonas fluorescens In5, isolated from a Greenlandic suppressive soil. mBio 6:e00079. doi: 10.1128/mBio.00079-15.Editor
Mittler, R. (2006). Abiotic stress, the field environment and stress combination. Trends Plant Sci. 11, 15–19. doi: 10.1016/j.tplants.2005.11.002
Newman, M.-A., Sundelin, T., Nielsen, J. T., and Erbs, G. (2013). MAMP (microbe-associated molecular pattern) triggered immunity in plants. Front. Plant Sci. 4:139. doi: 10.3389/fpls.2013.00139
Nguyen, D. D., Melnik, A. V., Koyama, N., Lu, X., Schorn, M., Fang, J., et al. (2016). Indexing the pseudomonas specialized metabolome enabled the discovery of poaeamide b and the bananamides. Nat. Microbiol. 2:16197. doi: 10.1038/nmicrobiol.2016.197
Nielsen, T. H., Sorensen, D., Tobiasen, C., Andersen, J. B., Christophersen, C., Givskov, M., et al. (2002). Antibiotic and biosurfactant properties of cyclic lipopeptides produced by fluorescent Pseudomonas spp. from the sugar beet rhizosphere. Appl. Environ. Microbiol. 68, 3416–3423. doi: 10.1128/aem.68.7.3416-3423.2002
Olorunleke, F. E., Kieu, N. P., and Hofte, M. (2015b). “”Recent advances in Pseudomonas biocontrol,” in Bacterial-Plant Interactions: Advance Research and Future Trends, eds J. Murillo, B. A. Vinatzer, R. W. Jackson, and D. L. Arnold (Norfolk: Caister Academic Press), 167–198. doi: 10.21775/9781908230584.07
Olorunleke, F. E., Hua, G. K. H., Kieu, N. P., Ma, Z., and Höfte, M. (2015a). Interplay between orfamides, sessilins and phenazines in the control of Rhizoctonia diseases by Pseudomonas sp. CMR12a. Environ. Microbiol. Rep. 7, 774–781. doi: 10.1111/1758-2229.12310
Ongena, M., Jourdan, E., Adam, A., Paquot, M., Brans, A., Joris, B., et al. (2007). Surfactin and fengycin lipopeptides of Bacillus subtilis as elicitors of induced systemic resistance in plants. Environ. Microbiol. 9, 1084–1090. doi: 10.1111/j.1462-2920.2006.01202.x
Oni, F. E., Olorunleke, O. F., and Höfte, M. (2019b). Phenazines and cyclic lipopeptides produced by Pseudomonas sp. CMR12a are involved in the biological control of Pythium myriotylum on cocoyam (Xanthosoma sagittifolium). Biol. Control 129, 109–114. doi: 10.1016/j.biocontrol.2018.10.005
Oni, F. E., Geudens, N., Omoboye, O. O., Bertier, L., Hua, H. G. K., Adiobo, A., et al. (2019a). Fluorescent Pseudomonas and cyclic lipopeptide diversity in the rhizosphere of cocoyam (Xanthosoma sagittifolium). Environ. Microbiol. 21, 1019–1034. doi: 10.1111/1462-2920.14520
Pandey, P., Ramegowda, V., and Senthil-Kumar, M. (2015). Shared and unique responses of plants to multiple individual stresses and stress combinations: physiological and molecular mechanisms. Front. Plant Sci. 6:723. doi: 10.3389/fpls.2015.00723
Philippot, L., Raaijmakers, J. M., Lemanceau, P., and van der Putten, W. H. (2013). Going back to the roots: the microbial ecology of the rhizosphere. Nat. Rev. Microbiol. 11, 789–799. doi: 10.1038/nrmicro3109
Raaijmakers, J. M., de Bruijn, I., and de Kock, M. J. (2006). Cyclic lipopeptide production by plant-associated Pseudomonas spp. diversity, activity, biosynthesis, and regulation. Mol. Plant Microbe Interact. 19, 699–710. doi: 10.1094/mpmi-19-0699
Raaijmakers, J. M., de Bruijn, I., Nybroe, O., and Ongena, M. (2010). Natural functions of lipopeptides from Bacillus and Pseudomonas: more than surfactants and antibiotics. FEMS Microbiol. Rev. 34, 1037–1062. doi: 10.1111/j.1574-6976.2010.00221.x
Rokni-Zadeh, H., Li, W., Sanchez-Rodriguez, A., Sinnaeve, D., Rozenski, J., Martins, J. C., et al. (2012). Genetic and functional characterization of cyclic lipopeptide white-line-inducing principle (WLIP) production by rice rhizosphere isolate Pseudomonas putida RW10S2. Appl. Environ. Microbiol. 78, 4826–4834. doi: 10.1128/AEM.00335-12
Rokni-Zadeh, H., Li, W., Yilma, E., Sanchez-Rodriguez, A., and De Mot, R. (2013). Distinct lipopeptide production systems for WLIP (white line-inducing principle) in Pseudomonas fluorescens and Pseudomonas putida. Environ. Microbiol. Rep. 5, 160–169. doi: 10.1111/1758-2229.12015
Roongsawang, N., Hese, K. I., Haruki, M., Imanaka, T., Morikawa, M., and Kanaya, S. (2003). Cloning and characterization of the gene cluster encoding arthrofactin synthetase from Pseudomonas sp. MIS38. Cell Chem. Biol. 10, 869–880. doi: 10.1016/j.chembiol.2003.09.004
Röttig, M., Medema, M. H., Blin, K., Weber, T., Rausch, C., and Kohlbacher, O. (2011). NRPSpredictor2 - A web server for predicting NRPS adenylation domain specificity. Nucleic Acids Res. 39, 362–367. doi: 10.1093/nar/gkr323
Skamnioti, P., and Gurr, S. J. (2007). Magnaporthe grisea cutinase2 mediates appressorium differentiation and host penetration and is required for full virulence. Plant Cell 19, 2674–2689. doi: 10.1105/tpc.107.051219
Song, C., Liu, Z., Xie, Q., Wang, H., Huang, Y., Ruan, Y., et al. (2017). Characterization of a novel thermo-stable lipase from endophyte Pseudomonas putida in Pistacia chinensis Bunge. Appl. Biochem. Microbiol. 53, 524–532. doi: 10.1134/S0003683817050143
Sørensen, D., Nielsen, T. H., Sørensen, J., and Christophersen, C. (2002). Cyclic lipoundecapeptide lokisin from Pseudomonas sp. strain DSS41. Tetrahedron Lett. 43, 4421–4423. doi: 10.1016/S0040-4039(02)00856-0
Spence, C., Alff, E., Johnson, C., Ramos, C., Donofrio, N., Sundaresan, V., et al. (2014). Natural rice rhizospheric microbes suppress rice blast infections. BMC Plant Biol. 14:130. doi: 10.1186/1471-2229-14-130
Strieker, M., Tanoviæ, A., and Marahiel, M. A. (2010). Nonribosomal peptide synthetases: structures and dynamics. Curr. Opin. Struct. Biol. 20, 234–240. doi: 10.1016/j.sbi.2010.01.009
Stringlis, I. A., Zhang, H., Pieterse, C. M. J., Bolton, M. D., and De Jonge, R. (2018). Microbial small molecules-weapons of plant subversion. Nat. Prod. Rep. 35, 410–433. doi: 10.1039/c7np00062f
Talbot, N. J., Ebbole, D. J., and Hamer, J. E. (1993). Identification and characterization of MPG1, a gene involved in pathogenicity from the rice blast fungus Magnaporthe grisea. Plant Cell 5, 1575–1590. doi: 10.1105/tpc.5.11.1575
Tamura, K., Stecher, G., Peterson, D., Filipski, A., and Kumar, S. (2013). MEGA6: molecular evolutionary genetics analysis version 6.0. Mol. Biol. Evol. 30, 2725–2729. doi: 10.1093/molbev/mst197
Thuan, N. T. N., Bigirimana, J., Roumen, E., Van Der Straeten, D., and Höfte, M. (2006). Molecular and pathotype analysis of the rice blast fungus in North Vietnam. Eur. J. Plant Pathol. 114, 381–396. doi: 10.1007/s10658-006-0002-8
Tran, H., Ficke, A., Asiimwe, T., Höfte, M., and Raaijmakers, J. M. (2007). Role of the cyclic lipopeptide massetolide a in biological control of Phytophthora infestans and in colonization of tomato plants by Pseudomonas fluorescens. New Phytol. 175, 731–742. doi: 10.1111/j.1469-8137.2007.02138.x
Vallet-Gely, I., Novikov, A., Augusto, L., Liehl, P., Bolbach, G., Péchy-Tarr, M., et al. (2010). Association of hemolytic activity of Pseudomonas entomophila, a versatile soil bacterium, with cyclic lipopeptide production. Appl. Environ. Microbiol. 76, 910–921. doi: 10.1128/AEM.02112-09
Van Der Voort, M., Meijer, H. J. G., Schmidt, Y., Watrous, J., Dekkers, E., Mendes, R., et al. (2015). Genome mining and metabolic profiling of the rhizosphere bacterium Pseudomonas sp. SH-C52 for antimicrobial compounds. Front. Microbiol. 6:693. doi: 10.3389/fmicb.2015.00693
Walker, B. J., Abeel, T., Shea, T., Priest, M., and Abouelliel, A. (2014). Pilon: an integrated tool for comprehensive microbial variant detection and genome assembly improvement. PLoS One 9:112963. doi: 10.1371/journal.pone.0112963
Weller, D. M. (2007). Pseudomonas biocontrol agents of soilborne pathogens: looking back over 30 years. Phytopathology 97, 250–256. doi: 10.1094/phyto-97-2-0250
Weston, D. J., Pelletier, D. A., Morrell-Falvey, J. L., Tschaplinski, T. J., Jawdy, S. S., Lu, T.-Y., et al. (2012). Pseudomonas fluorescens induces strain-dependent and strain-independent host plant responses in defense networks, primary metabolism, photosynthesis, and fitness. Mol. Plant Microbe Interact. 25, 765–778. doi: 10.1094/MPMI-09-11-0253
Wilson, R. A., and Talbot, N. J. (2009). Under pressure: investigating the biology of plant infection by Magnaporthe oryzae. Nat. Rev. Microbiol. 7, 185–195. doi: 10.1038/nrmicro2032
Wu, L., Xiao, W., Chen, G., Song, D., Khaskheli, M. A., Li, P., et al. (2018). Identification of Pseudomonas mosselii BS011 gene clusters required for suppression of rice blast fungus Magnaporthe oryzae. J. Biotechnol. 282, 1–9. doi: 10.1016/j.jbiotec.2018.04.016
Xu, Z., Qin, L., Cai, M., Hua, W., and Jin, M. (2018). Biodegradation of kraft lignin by newly isolated Klebsiella pneumoniae, Pseudomonas putida, and Ochrobactrum tritici strains. Environ. Sci. Pollut. Res. 25, 14171–14181. doi: 10.1007/s11356-018-1633-y
Xue, M., Yang, J., Li, Z., Hu, S., Yao, N., Dean, R. A., et al. (2012). Comparative analysis of the genomes of two field isolates of the rice blast fungus Magnaporthe oryzae. PLoS Genet. 8:e1002869. doi: 10.1371/journal.pgen.1002869
Yan, X., and Talbot, N. J. (2016). Investigating the cell biology of plant infection by the rice blast fungus Magnaporthe oryzae. Curr. Opin. Microbiol. 34, 147–153. doi: 10.1016/j.mib.2016.10.001
Yoshida, S., Koitabashi, M., Nakamura, J., Fukuoka, T., Sakai, H., Abe, M., et al. (2015). Effects of biosurfactants, mannosylerythritol lipids, on the hydrophobicity of solid surfaces and infection behaviours of plant pathogenic fungi. J. Appl. Microbiol. 119, 215–224. doi: 10.1111/jam.12832
Keywords: lokisin, anikasin, WLIP, entolysin, xantholysin, CLP N3, non-ribosomal peptide synthetase, ISR
Citation: Omoboye OO, Oni FE, Batool H, Yimer HZ, De Mot R and Höfte M (2019) Pseudomonas Cyclic Lipopeptides Suppress the Rice Blast Fungus Magnaporthe oryzae by Induced Resistance and Direct Antagonism. Front. Plant Sci. 10:901. doi: 10.3389/fpls.2019.00901
Received: 27 April 2019; Accepted: 26 June 2019;
Published: 10 July 2019.
Edited by:
Choong-Min Ryu, Korea Research Institute of Bioscience & Biotechnology (KRIBB), South KoreaReviewed by:
Linda Thomashow, Agricultural Research Service (USDA), United StatesYoun-Sig Kwak, Gyeongsang National University, South Korea
Copyright © 2019 Omoboye, Oni, Batool, Yimer, De Mot and Höfte. This is an open-access article distributed under the terms of the Creative Commons Attribution License (CC BY). The use, distribution or reproduction in other forums is permitted, provided the original author(s) and the copyright owner(s) are credited and that the original publication in this journal is cited, in accordance with accepted academic practice. No use, distribution or reproduction is permitted which does not comply with these terms.
*Correspondence: Monica Höfte, monica.hofte@ugent.be