- 1Department of Agricultural Sciences, University of Naples Federico II, Naples, Italy
- 2Department of Biology, University of Naples Federico II, Naples, Italy
- 3Department of Environmental, Biological and Pharmaceutical Sciences and Technologies, University of Campania “Luigi Vanvitelli”, Caserta, Italy
Callistemon citrinus and Viburnum lucidum are appreciated and widespread ornamental shrubs for their abundant flowering and/or brilliant foliage. The intrinsic tolerance to drought/salinity supports their use in urban areas and in xeriscaping. Despite adaptive responses of these ornamental species to sodium chloride (NaCl) have been extensively explored, little is known on the effects of other salt solution, yet iso-osmotic, on their growth, mineral composition and metabolism. The present research was aimed to assess responses at the biochemical, physiological and anatomical levels to iso-osmotic salt solutions of NaCl and CaCl2 to discriminate the effects of osmotic stress and ion toxicity. The two ornamental species developed different salt-tolerance mechanisms depending on the salinity sources. The growth parameters and biomass production decreased under salinization in both ornamental species, independently of the type of salt, with a detrimental effect of CaCl2 on C. citrinus. The adaptive mechanisms adopted by the two ornamental species to counteract the NaCl salinity were similar, and the decline in growth was mostly related to stomatal limitations of net CO2 assimilation rate, together with the reduction in leaf chlorophyll content (SPAD index). The stronger reduction of C. citrinus growth compared to V. lucidum, was due to an exacerbated reduction in net photosynthetic rate, driven by both stomatal and non stomatal limitations. In similar conditions, V. lucidum exhibited other additional adaptive response, such as modification in leaf functional anatomical traits, mostly related to the reduction in the stomata size allowing plants a better control of stomata opening than in C. citrinus. However, C. citrinus plants displayed an increased ability to retain higher Cl- levels in leaves than in roots under CaCl2 salinity compared to V. lucidum, thus, indicating a further attempt to counteract chloride toxicity through an increased vacuolar compartmentalization and to take advantages of them as chip osmotica.
Introduction
The safeguard of water resources is an important issue for all Mediterranean countries, where the balance between the water demand for irrigation of food crops and its real availability has reached a critical level due to prolonged periods of water shortage and increased frequency of drought events (Ferchichi et al., 2018). The drought, salinity, and pollution from leachate, that contaminates the limited water resources, can have synergistic effects, thus increasing the seriousness of the phenomenon. Nonetheless, in this area highly intensive field and greenhouse cultivation is carried on to meet food demand; while, at the same time, the incessant growth of population is inevitably increasing the problem of water shortage and water quality crises (Saggaï et al., 2017).
Excessive concentrations of sodium chloride (NaCl) can affect and damage plants depending on the sensitivity of the species and/or genotypes (Colla et al., 2012; Liu et al., 2017), the phenological stage (Woodrow et al., 2017), and eventually the crop management practices (Colla et al., 2010). When sensitive plants are exposed to salinity, they first experiment osmotic stress because the increased osmotic strength of the soil solution reduces water uptake, causing inhibition of cell division and enlargement, and limitations of stomatal conductance, transpiration and photosynthesis (Lucini et al., 2015, 2016; Rouphael et al., 2016, 2018). Afterward, during long term salinity, plants withstand ionic stress, responsible for nutritional imbalance, oxidative stress, and inhibition of protein synthesis and enzyme activity, ultimately reducing plant growth, development and survival (Munns and Tester, 2008; Borgognone et al., 2016; Fascella et al., 2017; Carillo, 2018).
The majority of studies carried out on salinity, alone or in combination with other stresses, have mostly used NaCl as principal salt source, and the stress symptoms have been related to Na+ toxicity (Annunziata et al., 2017; Rouphael et al., 2017a,b; Woodrow et al., 2017). Less studies have concerned chloride (Geilfus, 2018) and other elements specific toxicity and/or conflicting effects on plants (Colla et al., 2012, 2013; Ntatsi et al., 2017; Rabhi et al., 2018), despite the fact that a multitude of salts are responsible for salinity and some of them, like Na2SO4 and CaCl2, have a concentration even higher than that of NaCl in soil and in groundwater of many areas in the world (Nedjimi et al., 2006; Ezlit et al., 2010). Depending on the type and concentration of salts in the water or growing substrate, ions can exert a beneficial or toxic action, because of a competition effect among ions or predominance of specific ions (Scagel et al., 2017). In particular, Ca2+ is able to mitigate salt effects in plants treated with NaCl by improving Ca2+/Na+ selectivity, and maintaining the structural and functional integrity of the membranes, protecting them from the detrimental effects of Na+, as well as reducing the leakage of cytosolic K+ (Cramer et al., 1985; Grattan and Grieve, 1999; Renault and Affifi, 2009; Korkmaz et al., 2017). Under short term NaCl stress, cytosolic Ca2+ at micromolar concentrations has been proven to bind and open the Arabidopsis vacuolar Two Pore K+ channel 1 (TPK1) to release K+ and maintain favorable Na+/K+ ratios; while in prolonged stress, sub-micromolar concentration of Ca2+ are part of longer-term K+ homeostasis in adapted roots (Latz et al., 2013; Wilkins et al., 2016). Addition of only 2–5 mM Ca2+ can increase nitrogen, Ca2+ and K+ selectivity, improve Reactive Oxygen Species (ROS) detoxification and Relative Water Content (RWC), and decrease membrane damages in both shoots and roots of plants under 50–200 mM of NaCl (Parvin et al., 2019).
However, the effectiveness of these responses depends on the source of Ca2+ ions (CaCl2 or CaSO4), in addition to the plant species/genotype reactivity to it (Volkmar et al., 1998). Several studies demonstrated that mild to moderate concentrations of CaCl2 can cause osmotic and ion specific effects more phytotoxic than NaCl in different horticultural species (Borghesi et al., 2013; Colla et al., 2013; Borgognone et al., 2014). The reduction in growth and yield observed in plants treated with CaCl2 could be attributed to the toxic effects of Cl-, whose uptake and transport to leaves seem to be less controlled than that of Na+, thus negatively affecting plant metabolism and development (Colla et al., 2013 and references therein). It is well established that herbaceous perennial species can be more sensitive to Cl- than to Na+, with critical levels of Cl- varying between 4 and 7 mg g-1 (Ju et al., 2018). Chloride toxic effects concern chlorophyll degradation, decline in PSII quantum yield and photosynthetic electron transport rate (Tavakkoli et al., 2010, 2011).
Notwithstanding the sub-optimal characteristics of reclaimed water, in particular for the high CaCl2 content, the scarcity of good quality water forces its use as an alternative water supply especially for irrigation. To this aim, being characterized by high adaptability to salinity, ornamental shrub and bush species could provide an interesting model to study the effects of salty wastewater use in plants (Acosta-Motos et al., 2015). Ornamental shrubs, naturally present in coastal arid and semiarid areas, as well as in marginal soils, are, in fact, endowed with unique morpho-anatomical, physiological and biochemical traits which allow them to cope with salinity, while maintaining good growth rate and abundant flowering, and therefore preserving their ornamental value (Tattini et al., 2006; Carillo et al., 2019).
Callistemon citrinus (Myrtaceae), known as red bottlebrush, and Viburnum lucidum (Adoxaceae), known as arrow-wood, are of great interest because they are highly sought-after on European markets as ornamental potted shrubs for their abundant flowering with bright colors and peculiar shapes and/or brilliant foliage (Vernieri et al., 2006; Gori et al., 2008), in addition to their moderate to high tolerance to drought and salinity which makes them suitable for use in urban areas and xeriscaping (Lippi et al., 2003; Vernieri et al., 2006; Álvarez and Sánchez-Blanco, 2014; Cirillo et al., 2014, 2016, 2017). Notwithstanding several studies have been performed to assess the physiological responses of these ornamental species to NaCl (Lippi et al., 2003; Álvarez and Sánchez-Blanco, 2015; Cirillo et al., 2016), little is known on the effects of other types of salinity on their growth, mineral composition and metabolism. Indeed, it is difficult to discriminate among plant responses due to osmotic and ionic stresses. Since osmotic stress is always proportional to salt concentration, iso-osmotic solutions of different salt types may trigger the same plant growth and physiological responses due to osmotic unbalance, still causing ion-specific stress effects linked to salt type and genotype-specific sensitivity (Pagter et al., 2009). Thus, it is of great importance to elucidate the biochemical, physiological and anatomical mechanisms of adaptation of potted C. citrinus and V. lucidum to iso-osmotic salt solutions of NaCl and CaCl2 in order to discriminate the effects of osmotic stress and ion toxicity, but excluding the further problem of Ca2+ deficiency. The responses of the two species to the two chloride salts were analyzed in terms of growth parameters, leaf gas exchange, photosystem efficiency, water relations and leaf functional anatomical traits. To achieve a comprehensive and integrated interpretation of all data, we applied tools of multivariate statistics, whose usefulness has been recently proved in horticultural species, including ornamentals, in response to salinity concentration (Rouphael et al., 2018; Carillo et al., 2019). The knowledge gained from this study could be used as a meaningful approach to discriminate between osmotic and ionic stresses responses in plants under salt stress conditions, in addition to provide practical specialist skills for the alternative re-use of reclaimed water for xeriscaping of urban, industrial and marginal areas of Mediterranean countries.
Materials and Methods
Plant Material and Experimental Conditions
Rooted cuttings of 2-year-old Callistemon citrinus and Viburnum lucidum, purchased from a specialized nursery (Vivaio Torsanlorenzo, Ardea, Italy), were transplanted on March 12 2014 into 1.5 L plastic pots filled with peat moss. Plants were placed inside a zinc-coated steel greenhouse at the experimental station of the University of Naples Federico II, South Italy (43° 31′ N, 14° 58′ E; 60 m above sea level). The pots were placed on 1.8 m wide × 7 m-long troughs, at a plant density of 2.4 per square meter. Plants were cultivated under natural light conditions using a 50% black shading net and photosynthetically active radiation (PAR) averaged 550 μmol m-2 s-1. Inside the glasshouse, the daily air temperature varied between 17 and 34°C and the relative humidity (R.H.) was 58%/75% during day/night, respectively.
For both ornamental species, the experiment treatments consisted of three nutrient solutions, a basic nutrient solution (control non-salt treatment) and two saline nutrient solutions obtained by adding 80 mM NaCl or 53.3 mM CaCl2 to the control solution. The study was performed in terms of equimolar concentrations of the two different chloride salts, in order to assess the salt-specific influence. Treatments were arranged in a completely randomized blocks design (CRBD) with four replicates per treatment. Each experimental unit included six plants (n = 72 plants for each species). The first and last plant of each experimental unit were considered as guards.
Nutrient Solution Management, Sodium Chloride and Calcium Chloride Application
The quality of the irrigation water was typical of the area and characterized by a high bicarbonate concentration (5.5 mM HCO3), 0.5 mM Ca, 0.5 mM Mg, 0.1 mM K, 0.4 mM Na, and 0.3 mM Cl. Values of the pH and EC of the irrigation water were 7.0 and 0.4 dS m-1, respectively. The composition of the basic nutrient solution (non-salt control) used to replenish nutrients absorbed by plants was as follows: 13.6 mM N-NO3, 2.0 mM S,1.4 mM P, 6.0 mM K, 4.5 mM Ca, 2.0 mM Mg, 1 mM Na, 1 mM Cl, 20 μM Fe, 9 μM Mn, 1.5 μM Cu, 3 μM Zn, 20 μM B and 0.3 μM Mo (Carillo et al., 2019) with an electrical conductivity (EC) of 2.0 dS m-1. The irrigation water 0.5 mM Ca natural content, and the additional 4.5 mM Ca in the basic nutrient solution, allowed to have a basic nutrient media containing 5 mM Ca, to allow plants having an optimal Ca concentration (Parvin et al., 2019). The saline nutrient solutions had the same basic composition, plus an additional 80 mM NaCl or 53.33 mM CaCl2, resulting in an EC values of 11.1 and 11.6 dS m-1, respectively. The pH in the three nutrient solutions was 6.0 ± 0.3. Saline treatments started on March 25 (12 days after transplanting, 12 DAT).
The nutrient solutions in all treatments were pumped from independent tanks through a drip irrigation system, with two emitters per plant at a flow rate of 2 L h-1 each. Irrigation timing varied from 2 to 4 fertigations per day of 1–3 min. The watering was performed with a leaching fraction of 25% higher than the amount of water required to restore field capacity. Leaching fractions of 20-130% are needed to maintain the EC in the growing medium at recommended level (Carillo et al., 2019).
Biomass Production, Partitioning and Growth Analysis
For both C. citrinus and V. lucidum, ten plants were sampled to measure the fresh and dry weight of the plants at the beginning of the experiment. At the end of the trial (127 DAT), four plants per experimental unit were harvested and dissected into leaves, stems and roots. The total leaf area per plant was measured using an electronic area meter (Li-Cor3000, Li-Cor, Lincoln, NE, United States), and the number of leaves as well as the plant height were also recorded. Then, all plant organs were dried to constant weight in a forced-air oven at 80°C for 72 h to determine the dry biomass. Shoot dry weight was equal to the sum of the aerial vegetative parts (leaves + stems), and the root-to-shoot ratio was also calculated. Dried plant organs were then sampled for ion analyses. Lastly, the relative growth rate (g g-1 day-1) was calculated using the equation reported by De Groot et al. (2001): RGR = (lnW2-lnW1)/(t2-t1) where W1 and W2 are the fresh masses (g) of the above-ground plant part (shoot) at times t1 and t2, corresponding to the beginning (1 DAT) and to the end of the experiment (127 DAT), respectively.
Soil Plant Analysis Development Index, Leaf Gas Exchange and Chlorophyll a Fluorescence Emission
At 122 DAT, the Soil Plant Analysis Development (SPAD) index was measured on fully expanded leaves of C. citrinus and V. lucidum by means of a portable chlorophyll meter SPAD-502 (KonicaMinolta, Japan) of five representative plants per experimental unit. Measurements were made by avoiding major veins, leaflet margins and damaged areas. Twenty leaves were randomly measured and averaged to a single SPAD value per each replicate.
On the same date, measurements of leaf gas exchanges and chlorophyll a fluorescence emission were conducted within 2 h across solar noon (i.e., between 11:00 and 13:00 h) on the youngest fully expanded leaves, using six replicates per each treatment as described in Carillo et al. (2019). Briefly, the net CO2 assimilation rate (Pn), stomatal conductance (gs) and transpiration rate (E) were determined with a portable gas-exchange analyzer (LCA 4; ADC BioScientific Ltd., Hoddesdon, United Kingdom), equipped with a broad-leaf PLC (cuvette window area, 6.25 cm2). PAR, R.H., and carbon dioxide concentrations were set at ambient value and the flow rate of air was 400 ml s-1.
Fluorescence measurements were also performed on six replicates per each treatment. For the chlorophyll a fluorescence emission measurements, a portable FluorPen FP100max fluorometer, equipped with a light sensor (Photon System Instruments, Brno, Czechia) was used. The ground fluorescence signal, Fo, was induced on 30′ dark adapted leaves, by a blue LED internal light of about 1–2 μmol m-2 s-1. The maximal fluorescence level in the dark, Fm, was induced by a 1s saturating light pulse of 3000 μmol m-2 s-1. The maximum quantum efficiency of PSII photochemistry, Fv/Fm, was calculated as (Fm - Fo)/Fm, according to Kitajima and Butler (1975). For the fluorescence measurements in the light, the fluorometer FluorPen FP100max was equipped with an open leaf-clip suitable for measurements under ambient light. The quantum yield of PSII electron transport (QY) was determined according to Genty et al. (1989). The linear electron transport rate (ETR) was expressed following Krall and Edwards (1992), whereas the photochemical (qP) and non-photochemical quenching (NPQ) were calculated as described by Quick and Horton (1984) and Bilger and Björkman (1990), respectively.
Stem Water Potential
Stem water potential (Ψmds) was measured at the same date of the physiological and biochemical measurements (122 DAT) by selecting one well-lit leaf per plant on three plants per replication. Stem water potential was measured using the pressure chamber (3005-series portable plant water status console, Soil Moisture Equipment Corp., Santa Barbara, CA, United States) technique (Scholander et al., 1965), taking the precautions proposed by Turner and Long (1980). The stem water potential measurement was made at midday (i.e., 12:00 h) on leaves located close to the trunk, bagged for at least 1 h before measurement.
Leaf and Root Mineral Analysis
The leaf and root dry tissues were finely ground through a mill (IKA, MF10.1, Staufen, Germany) with 0.5 mm-sieve, then 1 g samples were used for macro-minerals, sodium and chloride analyses. Nitrogen (total N) concentration in leaf and root tissues was determined after mineralization with sulfuric acid in the presence of potassium sulfate and a low concentration of copper by the Kjeldahl method (Bremner, 1965).
For the anions (PO43-, SO42- and Cl-) and cations (K+, Ca2+, Mg2+ and Na+) leaf and root analysis, 250 mg of the C. citrinus and V. lucidum dried material were suspended in 50 ml of ultrapure water (Milli-Q, Merck Millipore, Darmstadt, Germany) and subjected to three freeze-thaw cycles in liquid nitrogen followed by 10 min shaking in a water bath (ShakeTemp SW22, Julabo, Seelbach, Germany) at 80°C. The mixture was centrifuged at 6000 rpm for 10 min (R-10 M, Remi Elektrotechnik Limited, India), then filtered through a 0.20 μm filter paper (Whatman International Ltd., Maidstone, United Kingdom), as described previously in Rouphael et al. (2017c). Anions and cations in both C. citrinus and V. lucidum organs were separated and quantified by ion chromatography (ICS-3000, Dionex, Sunnyvale, CA, United States) coupled to a conductivity detector. The conductivity detector with IonPac CG12A (4 × 250 mm, Dionex, Corporation) guard column and IonPac CS12A (4 × 250 mm, Dionex, Corporation) analytical column were used for the cations analysis, while for the anions determination, an IonPac AG11-HC guard (4 × 50 mm) column and IonPac AS11-HC analytical column (4 × 250 mm) were employed.
Analysis of Leaf Anatomical Traits
On the same date of the physiological and biochemical measurements (122 DAT), leaves of both C. citrinus and V. lucidum were sampled by cutting three fully expanded leaves from three plants per treatment, and immediately fixing them in FAA (5 ml 40% formaldehyde, 5 ml glacial acetic acid, 90 ml 50% ethanol). Each leaf was dissected to remove the apical and basal portions, and dividing the median region of the lamina into two sub-samples: one for stomata analysis, the other for mesophyll characterization through thin sectioning.
Stomata characterization was performed on both adaxial and abaxial lamina surfaces in C. citrinus, being its leaves bifacial and amphystomatic, while only on the abaxial surface in dorsiventral V. lucidum leaves. Regions of the leaf lamina (5 × 5 mm) were dissected and directly mounted on microscope slides with distilled water and kept pressed by sealing the cover slip with adhesive tape to maintain the lamina as flattened as possible. The slides were observed under an epi-fluorescence microscope (BX60, Olympus, Hamburg, Germany) equipped with a Mercury lamp, band-pass filter 330–385 nm, dichromatic mirror 400 nm and above, and barrier filter 420 nm and above. Such filters allow highlighting stomata among epidermal cells, owing to the different excitation and emission properties of various compounds that make visible the guard cells, especially the thickened inner cell wall at the stomata aperture level (Ruzin, 1999; De Micco et al., 2011). Images of the lamina surface, from three separate regions avoiding main veins, were collected by means of a digital camera (CAMEDIA C4040, Olympus), taking care to avoid veins.
The following parameters were measured through the software program AnalySIS 12.0 (Olympus, Germany): stomata frequency (SF, calculated by counting the number of stomata in a given region of the epidermis and expressed as the number of stomata per mm2), and guard cell length (GCL, quantified by measuring the length, pole to pole) of 15 cells.
From the second group of subsamples, 5 × 5 mm portions of the leaf lamina were dissected and dehydrated in an ethanol series (up to 95%), infiltrated and embedded in the JB4 acrylic resin (Polysciences, United States). Thin cross sections (5 μm thick) were cut by means of a rotary microtome, stained with 0.025% toluidine blue in 0.1 M citrate buffer at pH 4.0 (Reale et al., 2012), and permanently mounted with mineral oil for microscopy. Sections were analyzed under a transmitted light microscope (BX60, Olympus, Germany) and digital images were collected and analyzed, as reported above, to quantify some functional anatomical traits, including: thickness of leaf lamina (TLL), palisade and spongy parenchymas (TPP and TSP), measured in five positions of the leaf lamina avoiding veins; quantity of intercellular spaces in the spongy parenchyma (ISS - expressed as the percentage of tissue occupied by intercellular spaces over a given surface, in six positions of the leaf lamina; De Micco et al., 2011). ISS was not measured in C. citrinus due to the compactness of the mesophyll.
Statistical Analysis
All experimental data for both ornamental species were statistically analyzed by one-way analysis of variance (ANOVA) using the SPSS 13 software package1. To separate treatment means for each measured parameter, Duncan’s Multiple Range Test was performed at a significance level of p ≤ 0.05. Principal component analysis (PCA) was also conducted using Minitab 16.2.1 statistical software, aimed to extract trends when multiple qualitative variables were used by formulating new variables correlated to the original ones. The PCA outputs included treatment component scores as well as variable loading to each selected component (Ciarmiello et al., 2015; Rouphael et al., 2017c).
Results
Plant Growth and Morphology
The growth parameters (plant height and number of leaves per plant) and biomass production decreased under salinity independently of the type of salt in both ornamental species, with a particular significant detrimental effect of CaCl2 on C. Citrinus (Table 1). Specifically, in C. citrinus the percentage of plant height, shoot dry weight and number of leaves reduction in comparison to non-salinized control plants was 16.1, 16.6, and 23.2% with NaCl and 25.4, 33.2, and 60.7% with CaCl2 (Table 1). The lowest biomass production observed in C. citrinus plants with CaCl2 compared to NaCl treatment was mainly attributed to the reduction in both leaf number and total leaf area (Tables 1, 2). Moreover, in V. lucidum the addition of 80 mM NaCl or 53.33 mM CaCl2 in the nutrient solution reduced plant height, leaf number per plant, shoot dry biomass as well as the relative growth rate by 24.3, 33.8, 28.1, and 40.0%, respectively, compared to the control with no significant difference between the two salinity sources (Table 1). An opposite trend was observed for the root-to-shoot (R/S); the ratio increased (by 22.4%) from 0.49 to an average of 0.6 in response to nutrient solution salinity (Table 1).
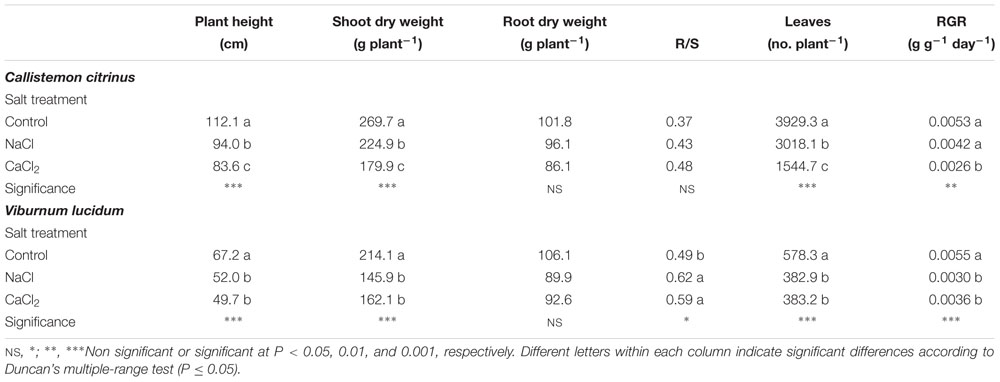
Table 1. Effects of salt treatment in the nutrient solution on plant height shoot and root dry weight, root-to-shoot (R/S) ratio, number of leaves and relative growth rate (RGR) of potted Callistemon citrinus and Viburnum lucidum plants.
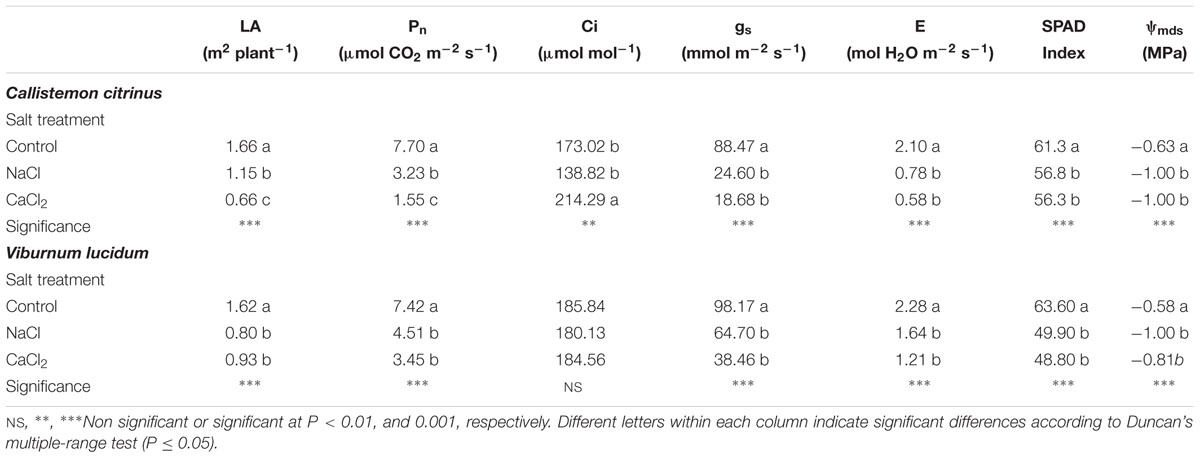
Table 2. Effects of salt treatment in the nutrient solution on total leaf area (LA), net photosynthetic rate (Pn), sub-stomatal CO2 concentration (Ci), stomatal conductance (gs), transpiration rate (E), Soil Plant Analysis Development (SPAD) index and midday stem water potential (ψmds) of potted Callistemon citrinus and Viburnum lucidum plants.
Physiological and Biochemical Parameters
Similarly to the effects on plant growth parameters, the total leaf area and net CO2 assimilation rate (Pn) in C. citrinus decreased in response to the increase in salinity concentration in the nutrient solution with detrimental effects more pronounced for CaCl2 treatment (Table 2). Furthermore, the addition of 80 mM NaCl or 53.33 mM CaCl2 in the nutrient solution reduced stomatal conductance (gs) and transpiration rate (E), greenness readings (i.e., SPAD index) and midday stem water potential (ψmds) by 75.5, 67.6, 7.7, and 37.0%, respectively, compared to the control with no significant difference between the two salinity sources (NaCl and CaCl2; Table 2). Moreover, it is worth noting that no significant difference among the physiological parameters was recorded in V. lucidum plants treated with NaCl or CaCl2 (Table 2). Specifically, under both saline conditions, the total leaf area, Pn, gs, E, SPAD index and ψmds averaged, respectively, 46.6, 46.4, 47.4, 37.7, 22.4, and 55.7% lower than those recorded in non-salinized control plants (Table 2).
The chlorophyll a fluorescence analysis evidenced significant differences in photochemistry among salt treatments between C. citrinus and V. lucidum. More specifically, for both species, the addition of NaCl in the nutrient solution did not determine changes in photochemical quenching (qP), quantum yield of PSII electron transport (QY), maximum quantum efficiency of PSII photochemistry (Fv/Fm) and linear electron transport rate (ETR) compared to control (Table 3). Conversely, for both species, the addition of CaCl2 in the nutrient solution elicited significant decrease in qP, QY, Fv/Fm and ETR compared to both non-saline and NaCl treatments, whereas an opposite trend was observed for NPQ (Table 3).
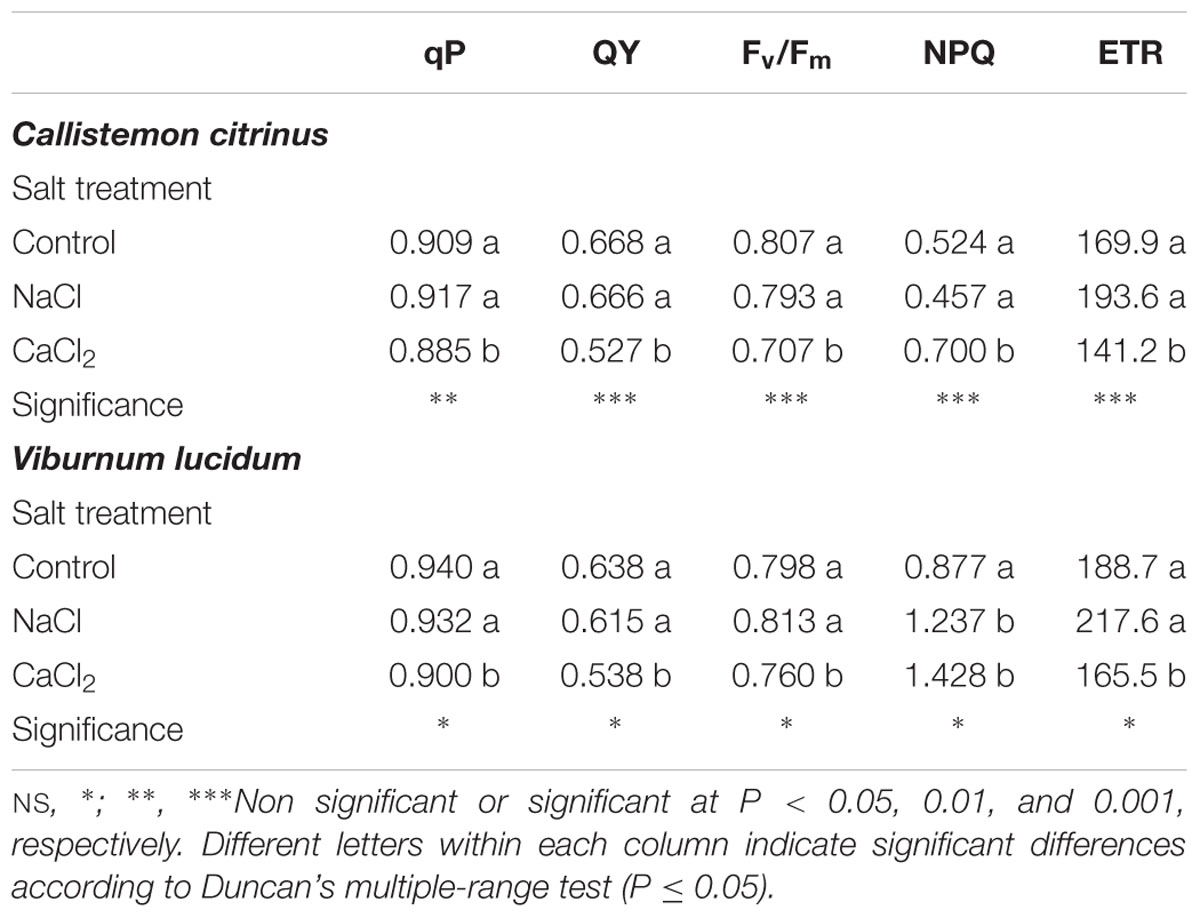
Table 3. Effects of salt treatment in the nutrient solution on photochemical quenching (qP), quantum yield of PSII electron transport (QY), maximum quantum efficiency of PSII photochemistry (Fv/Fm), non-photochemical quenching (NPQ) and linear electron transport rate (ETR) of potted Callistemon citrinus and Viburnum lucidum plants.
Ions Content and Partitioning
In C. citrinus, the total N and PO43- concentrations in leaves were negatively affected by salt stress treatment with more detrimental effects in presence of CaCl2 (Table 4). The application of CaCl2 in the nutrient solution significantly affected the K+ and Mg2+ concentrations in leaf tissue as well as Ca2+ concentration in both organs, which were higher than in both non-saline and NaCl treatments (Table 4). Sodium (Na+) concentration in leaves and roots increased under NaCl salinity, whereas chloride (Cl-) concentration was higher in both the saline treatments and occurred in increasing pattern with Cl- content in the external nutrient solution. The highest concentrations of Na+ and Cl- were found in leaves, and in particular in those from NaCl and CaCl2 treated plants, respectively (Table 4). Under NaCl treatment, Na+ concentration in C. citrinus leaves increased by 6.1-fold compared to non-salt control, whereas under NaCl and CaCl2 stress treatments, Cl- concentration in leaf tissue increased by 3.0 and 7.1-fold, respectively, compared to non-saline nutrient solution (Table 4). Moreover, under NaCl treatment, the decrease in K+/Na+ ratio in relation to the non-stressed control was higher in leaves than in roots, according to the higher levels of Na+ but not to the decrease in K+ concentration, which was not significant (Table 4).
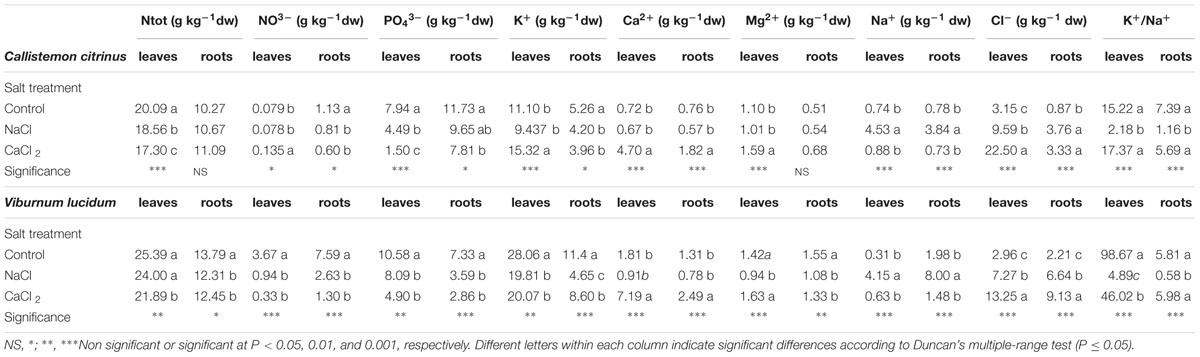
Table 4. Effects of salt treatment in the nutrient solution on macronutrients, sodium, chloride and potassium-to-sodium ratio of leaves and roots of potted Callistemon citrinus and Viburnum lucidum plants.
In V. lucidum, the N and PO43- concentrations in leaves were more negatively influenced by CaCl2 than by NaCl (Table 4). The application of 53.33 mM CaCl2 in the nutrient solution positively affected the Ca2+ concentration in both leaves and roots; and in particular, in leaves it was 3.97 and 7.9-fold higher than that of non-salinized and NaCl-treated plants, respectively (Table 4). Similarly, to C. citrinus, the toxic ion (Na+ and Cl-) concentrations in leaves and roots occurred in increasing pattern with increasing external NaCl and CaCl2 stress (Table 4). Under NaCl conditions, Na+ and Cl- concentrations in V. lucidum leaves and roots increased by 13.4/2.5-fold (for leaves) and 4.0/3.0-fold (for roots), respectively, compared to non-salt control (Table 4). Our results also showed that CaCl2 elicited a significant increase in Cl- concentration in both leaves and roots, 4.5 and 4.1-fold, respectively, compared to non-saline treatment (Table 4). Finally, the decrease in K+/Na+ ratio under NaCl conditions in relation to the non-stressed control was higher in leaves than in and roots (Table 4).
Leaf Functional Anatomical Traits
In C. citrinus leaves, the stomatal frequency (SF), guard cells length (GCL) and TLL were significantly affected by salinity sources (Table 5). More specifically, SF increased by 13% under NaCl, while GCL was decreased by NaCl treatment (by 12.3%) and increased by CaCl2 one (by 6%) (Table 5). TLL was decreased by both salinity treatments (Table 5). Moreover, no significant differences among treatments were observed for the incidence of both palisade and spongy parenchymas (TPP/TLL and TSP/TLL) over the total leaf lamina (Table 5).
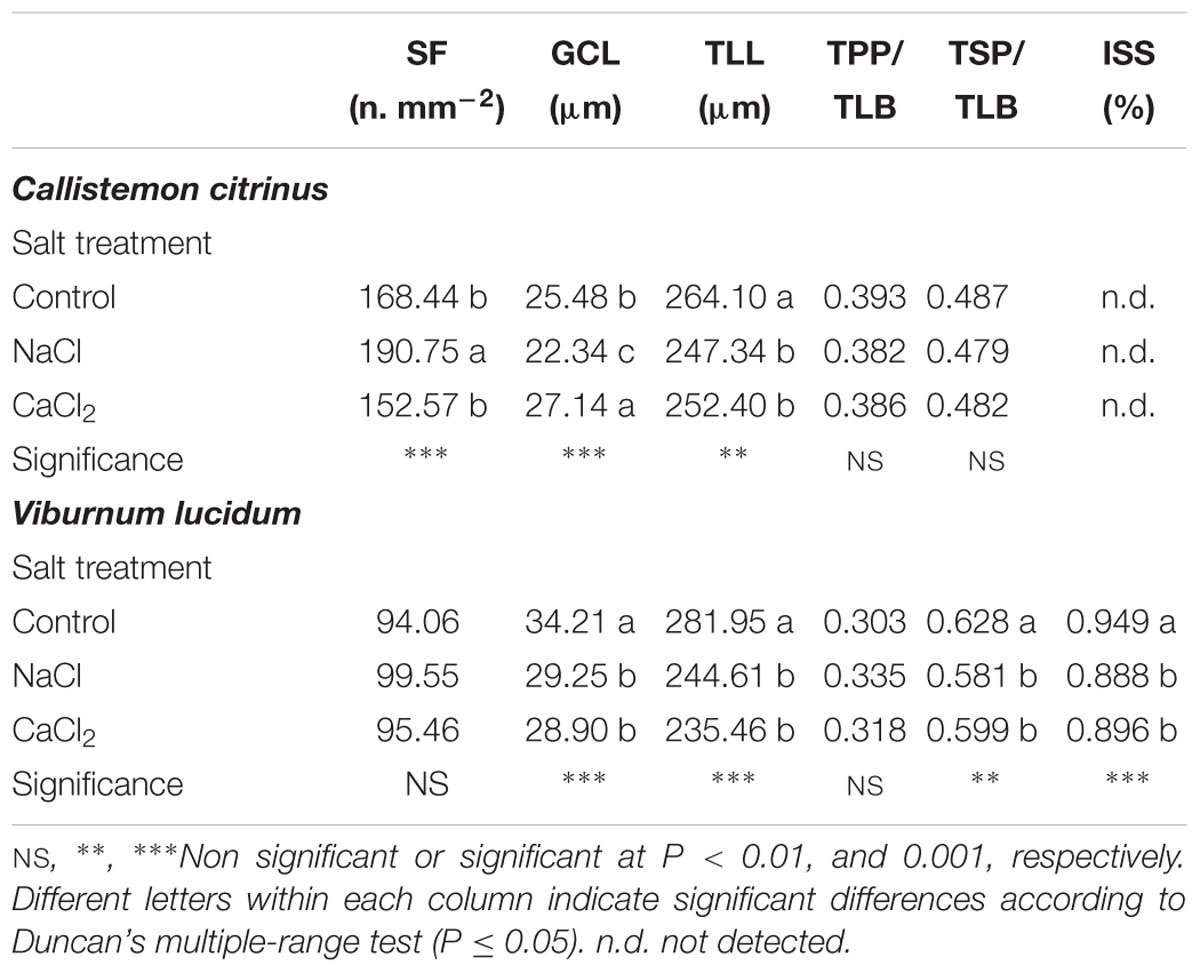
Table 5. Effects of salt treatment in the nutrient solution on stomatal frequency (SF), guard cell length (GCL), thickness of leaf lamina (TLL), thickness of palisade parenchyma (TPP)/thickness of leaf blade (TLB) ratio, thickness of spongy parenchyma (TSP)/thickness of leaf blade (TLB) ratio and quantity of intercellular spaces in the spongy parenchyma (ISS) of potted Callistemon citrinus and Viburnum lucidum plants.
In V. lucidum leaves, the GCL, TLL, incidence of spongy parenchyma over the total leaf lamina (TSP/TLL) as well as the quantity of intercellular spaces in the spongy parenchyma (ISS) were significantly affected by salinity sources (Table 5). The application of 80 mM NaCl or 53.33 mM CaCl2 in the nutrient solution determined a significant decrease in GCL, TLL, TSP/TLB and ISS (by 15.0, 14.9, 6.0, and 6.0%, respectively) with no significant difference between the two salinity sources (Table 5). Finally, no significant differences among salt treatments were observed for both SF (avg. 96.4 n. mm-2) and incidence of palisade parenchyma over the total leaf lamina TPP/TLL (avg. 0.319) (Table 5).
Heat Map Analysis
A heat map providing the morpho-anatomical, biochemical and physiological changes of potted C. citrinus and V. lucidum in response to salinity sources (non-saline, NaCl or CaCl2) was displayed in Figure 1. The heat-map identified two main clusters in both ornamental species, which, however, divided the analyzed samples differently (Figure 1). For instance, in C. citrinus CaCl2 was completely separated from control and NaCl treatments (Figure 1A), while in V. lucidum the non-saline control was clearly separated from the two salinity sources (Figure 1B). Our results indicated that while in C. citrinus Cl- and Ca2+ salinity in both leaves and roots were the main clustering factor, followed by Na+; in V. lucidum the negative effect of salinity, even depending on different ions, Na+ for NaCl salt stress and Cl- and Ca2+ for CaCl2, induced similar negative effects on plant morphological and physiological parameters compared to the non-saline treatment. In particular, CaCl2 treatment clustered separated from the other two treatments in C. citrinus because of its higher sub-stomatal CO2 concentration (Ci), NPQ, total N in the root, Cl- and K+ in leaves, the higher Ca2+ and Mg2+ concentrations in both dried tissues, as well as its lower QY, Fv/Fm, ETR, root dry weight, RGR and number of leaves compared to the other two treatments (Figure 1A). On the other hand, the two equimolar concentrations of NaCl and CaCl2 clustered separated from control treatment in V. lucidum mainly due to their lower K+ and PO43- concentrations in roots and leaves, as well as the lower photosynthetic performance (Pn), RGR, plant height, number of leaves, root and shoot dry weight (Figure 1B).
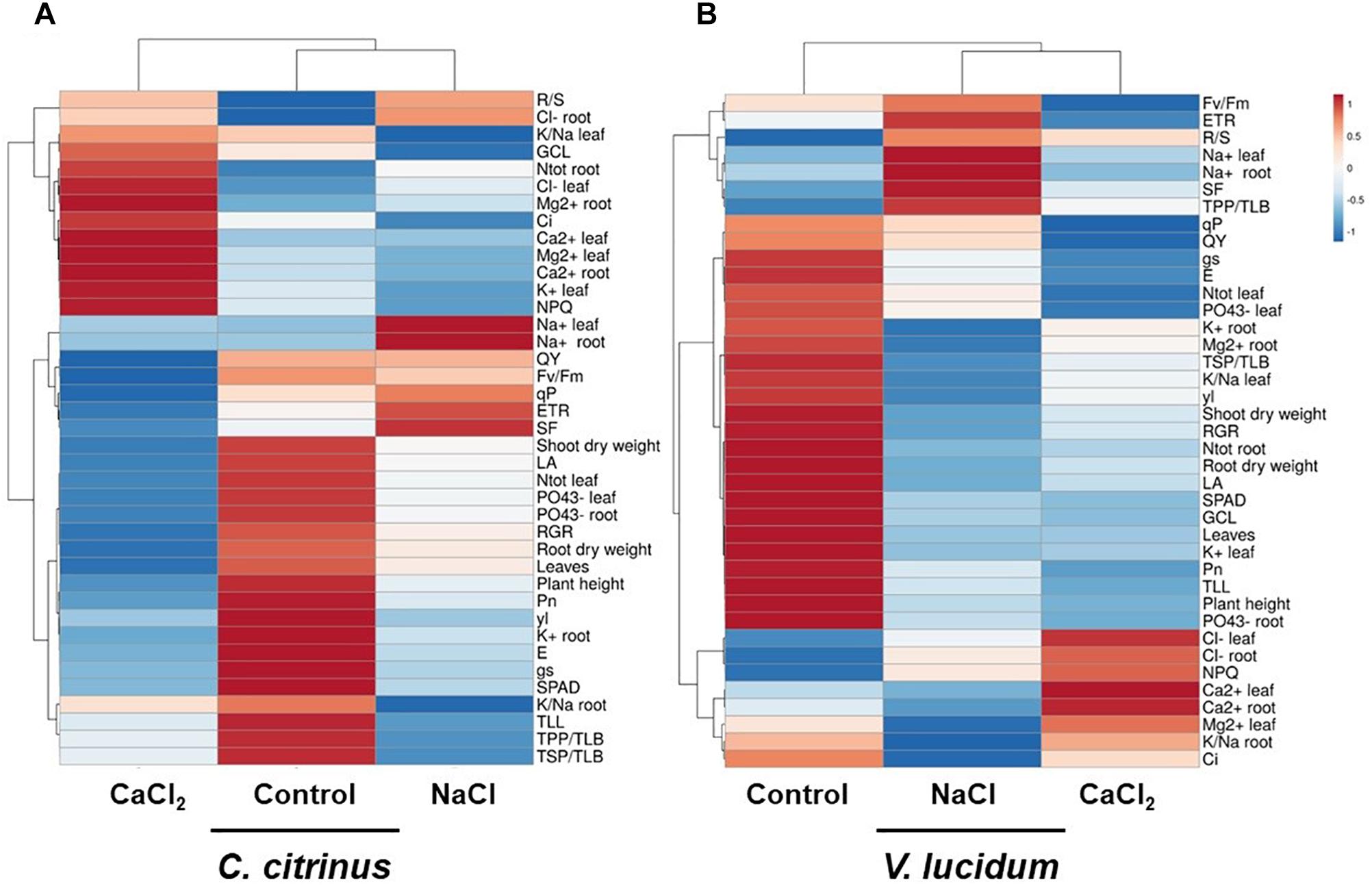
Figure 1. Cluster heat map analysis summarizing potted Callistemon citrinus (A) and Viburnum lucidum (B) responses to non-saline, NaCl and CaCl2 salinity treatments (performed in terms of equimolar concentrations). The heat map was generated using the https://biit.cs.ut.ee/clustvis/ online program package with Euclidean distance as the similarity measure and hierarchical clustering with complete linkage.
Principal Component Analysis
The principal component analysis (PCA) showed that for both species, the first two principal components (PCs) were associated with Eigen values higher than one and explained 100% of the cumulative variance, with PC1 and PC2 accounting for 65.5 and 34.5% for C. citrinus (Figure 2A) and 68.0 and 32.0% for V. lucidum (Figure 2B).
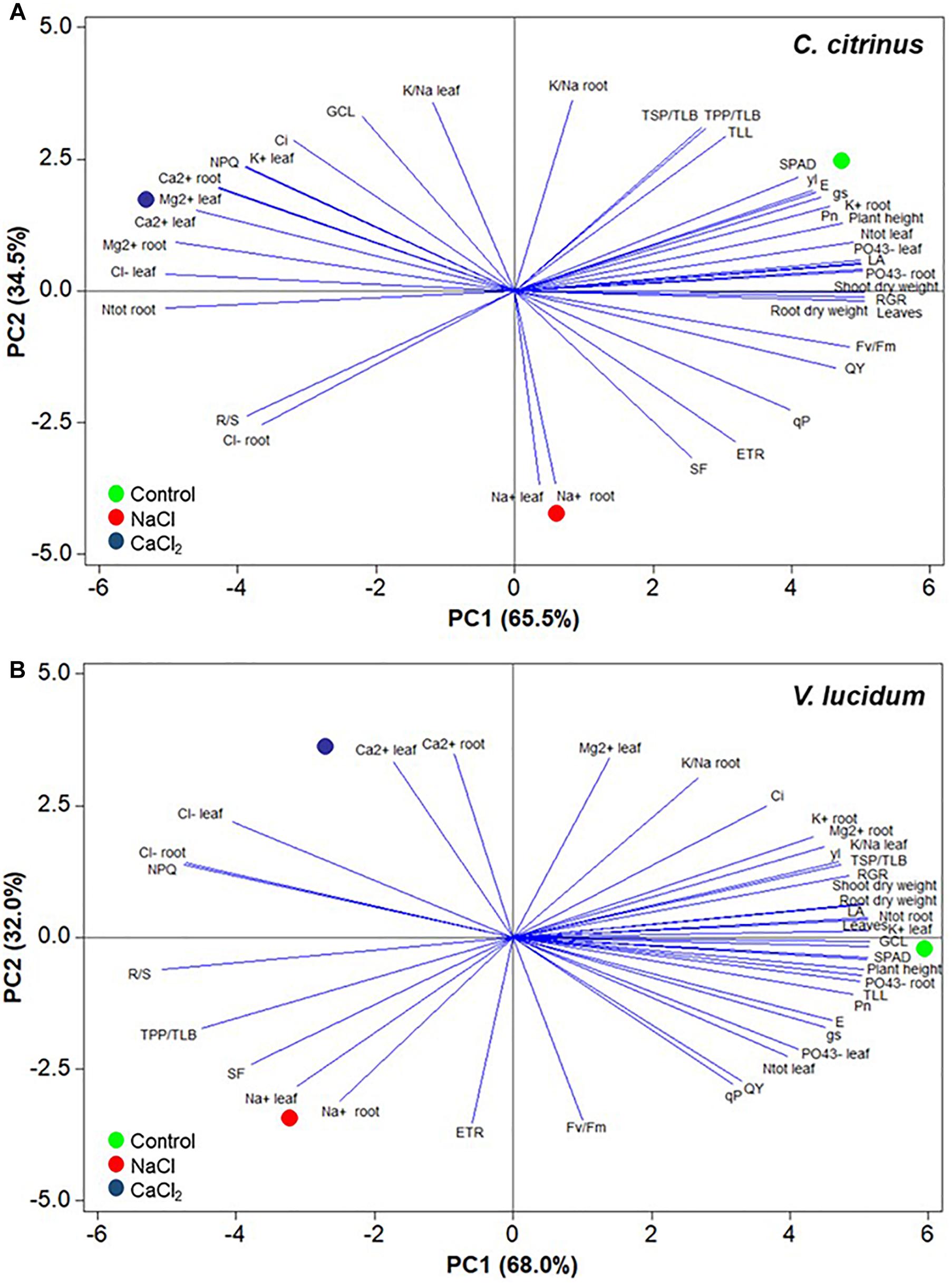
Figure 2. Principal component loading plot and scores of principal component analysis (PCA) of morpho-anatomical, biochemical, physiological parameters and ion contents and partitioning of potted Callistemon citrinus (A) and Viburnum lucidum (B) grown under non-saline, NaCl and CaCl2 salinity treatments.
In C. citrinus PC1 was positively correlated to RGR, number of leaves, root and shoot dry weight, leaf area, PO43- and N total concentrations in leaves, and also with PO43- and K+ in roots, QY, Pn, plant height, Fv/Fm and other photosynthetic parameters. PC1 was also negatively correlated to leaf Cl-, Ca2+ Mg2+ and K+ concentrations, as well as root N, Ca2+ and Mg2+ contents, NPQ and R/S ratio. Furthermore, PC2 was positively correlated to K+/Na+ in both plant tissues, GCL,TSP/TLB, TPP/TLB, TLL, and Ci, and negatively correlated to Na+ content in leaves and Na+ and Cl- content in roots, SF, ETR and R/S (Figure 2A). In V. lucidum PC1 was positively correlated to K+ in leaves, number of leaves, GCL, SPAD, N, and PO43- in roots, LA, root and shoot dry weight, plant height, TLL, RGR and Pn. PC1 was also negatively correlated to R/S ratio, NPQ, leaf and root TPP/TLB and SF. Finally, PC2 was positively correlated to root and leaf Ca2+, leaf Mg2+ content, K+/Na+ in roots and Ci, TSP/TLB, and negatively correlated to ETR, Fv/Fm, Na+ concentration in both organs, qP, QY and SF (Figure 2B).
In the current experiment, the score plot of the PCA superimposed on the above matrix of variables in both species revealed strong clustering of the three nutrient solutions, with C. citrinus treated with non-saline solution concentrating plant growth parameters, SPAD index, mineral status (N, P, K), photosynthesis activity, TPP/TLB, TSP/TLB, TLL, whereas the V. lucidum treated with non-salinized solution stands out for plant growth parameters, most mineral composition and physiological parameters. Particularly, the C. citrinus and V. lucidum under control treatment were positioned on the positive side of PC1 in the upper (for C. citrinus) and between the higher and lower right quadrants of the PCA score plot (for V. lucidum; Figure 2).
The lower right quadrant included C. citrinus treated with NaCl that delivered leaves and roots with high concentration of sodium, whereas in the upper left quadrant was positioned C. citrinus treated with CaCl2 characterized by high concentration of monovalent and bivalent cations and NPQ but also high chloride concentration (Figure 2). Finally, in V. lucidum the upper left quadrant depicted CaCl2 treatment characterized by high levels of calcium and chloride as well as high NPQ value, whereas the lower left quadrant (NaCl treatment) stands out for high leaf and root sodium concentrations and high values of R/S ratio, TPP/TLB, SF, and ETR (Figure 2).
Discussion
Even though many studies have been carried out on the effects of water salinity on growth performances and tolerance responses of ornamental shrubs (Álvarez and Sánchez-Blanco, 2014; Acosta-Motos et al., 2015; García-Caparrós and Lao, 2018 and therein literature), little information is available on their adaptive mechanisms to cope with different salt stress sources.
The two ornamental shrubs, C. citrinus and V. lucidum, showed different responses to salt treatments concerning growth, anatomical functional traits and photosynthesis. The reduction in plant height and shoot dry weight recorded in the analyzed species under 80 mM NaCl resulted slightly higher when compared to those reported by Álvarez and Sánchez-Blanco (2014) and Cassaniti et al. (2009) and lower compared to the results obtained when the two species were treated with 200 mM NaCl salinized water (Cirillo et al., 2016). In spite of the imposed iso-osmotic salinity of the compared treatments, the effect of 53.33 mM CaCl2 on the growth parameters was more marked in C. citrinus than in V. lucidum. To the best of our knowledge, there are no comparative studies on the responses of ornamental shrubs to iso-osmotic saline irrigation with NaCl and CaCl2, whereas only few reports on vegetable species are available on the CaCl2 salinity (Colla et al., 2012, 2013; Ntatsi et al., 2017; Rabhi et al., 2018). In particular, 40 mM CaCl2 significantly reduced the root, stem and leaf dry weight of saffron plants compared to control (Rabhi et al., 2018), while in sunflower plants, saline irrigation with NaCl or CaCl2 at EC of 5.0 dS m-1 induced a similar decline in flower size and biomass (de Sousa Júnior et al., 2017). The two ornamental species differently responded to the salinity treatments in the ratio between below- and above-ground biomass. Indeed, the increase in root to shoot ratio is a frequently detectable response to salt stress, more related to the osmotic effect than to a salt-specific effect (Hsiao and Xu, 2000). In V. lucidum the marked increase in root to shoot ratio, possibly favoring the retention of toxic Na+ ions at the root level, may be associated with lower salt tolerance (Dalton et al., 1997). In fact, V. lucidum, retained Na+ in roots at a higher extent than in the leaves; while C. citrinus had a higher ability to retain Na+ and Cl- levels in leaves than in roots under NaCl and CaCl2 salinity, respectively. This suggests the existence of an inclusion mechanism similar to that adopted by other Mediterranean ornamental plants under salinity (Navarro et al., 2008; Álvarez et al., 2012; Carillo et al., 2019), which is considered as a trait of salt tolerance that confers plants adaptive plasticity to osmotic and ionic stress (Läuchli and Epstein, 1990; Rodríguez et al., 2005; Woodrow et al., 2011). Indeed, the compartmentalization of toxic ions as cheap osmotica in the vacuole and the synthesis and accumulation of osmolytes in the cytosol are essential mechanisms of osmotic adjustment and oxidative stress protection in plant cells (Carillo, 2018; Ferchichi et al., 2018; Annunziata et al., 2019).
Acosta-Motos et al. (2017) reported that C. citrinus behaves as a typical Cl- accumulator, because it preferentially partitions Cl- into vacuoles. The ability of C. citrinus in compartmentalizing high concentration of Cl- in vacuoles allowed also these plants to avoid the competition of Cl- with NO3- for translocation within the plants by NO3- transporter proteins, leading to concentrations of NO3- in leaves which were unchanged or double compared to that of the non-saline control in NaCl and CaCl2 treatments, respectively, while, in V. lucidum leaves, the concentration of this ion underwent a strong decrease compared to the control. Notwithstanding the higher ability of C. citrinus to retain excess Cl- in the leaves, even if for the most part compartmentalized in the vacuole, it still caused in this plant the decrease in both PSII quantum yield and photosynthetic electron transport rate, usual symptoms of Cl- toxicity (Tavakkoli et al., 2010).
In V. lucidum the increase in uptake and transport of Na+ under NaCl salinity strongly interfered with K+ uptake in roots at the plasma membrane, even decreasing K+ transport to leaves (Gao et al., 2016), with a consequent strong reduction of K+ to Na+ ratio, a far more important parameter than the absolute amount of Na+ (Shabala and Munns, 2012). High Na+ concentrations are able to depolarize and damage the plasma membrane causing a restriction of K+ uptake and leaking; besides, Na+ can substitute K+ in key enzymatic reactions, further impairing cellular functions in cytosol and organelles (Almeida et al., 2017; Carillo et al., 2019). In addition, excess Ca2+, unlikely causing toxicity in itself, can restrain the uptake or availability of other nutrients such as K+ in V. lucidum, or PO43- in C. citrinus contributing to restrain RGR (White and Broadley, 2003).
Plants try to allocate Ca2+ and PO43- to different cell types in order to avoid the formation and precipitation of Ca3(PO4)2 crystals and render Ca2+ or PO43- unavailable for metabolism and growth (Conn and Gilliham, 2010; Ding et al., 2018). However, when plants are grown under CaCl2 salinity, Ca2+ is present at excessive concentrations in almost all root and leaf tissues, and therefore, interacting with PO43- or even oxalate, it is accumulated in crystals from which it will be released only when Ca2+ levels will decrease, in order to buffer its biologically active levels in plants (Nazrul Islam and Kawasaki, 2015). Thus, the concentrations of available PO43- tended to strongly decrease in C. citrinus leaves where the Ca2+ concentration showed the highest increase compared to the respective control.
Greenway and Munns (1980) reported that even if Na+ concentration in plant tissues increases under salinity, not necessarily the K+ to Na+ ratio in the cytoplasm is drastically reduced. C. citrinus has certainly the ability to retain cytosolic K+ concentration at a constant level or even increased by using the K+ stored in the vacuole compared to V. lucidum. The capability to satisfy plant metabolic demand for K+ under salinity by compartmentalizing Na+ in the vacuole and the majority of K+ in the cytosol could be another essential mechanism for C. citrinus salt tolerance (Wang et al., 2013). In fact, the K+ accumulated in the cytosol could also contribute to the osmotic balance of the toxic ions compartmentalized in the vacuole. In fact, even low K+ concentrations, if compartmentalized in the cytosol which usually occupies less than 10% of cell volume, can be sufficient to determine a significant osmotic pressure able to balance the vacuolar osmotic potential (Cuin et al., 2009).
The severe ion imbalance and physiological disorders related to salinity may also affect leaf gas exchange and plant growth (García-Caparrós and Lao, 2018). Based on our data, dark reactions of photosynthesis might be supposed more sensitive than light reactions to different salt treatments: gas exchanges evidenced a significant decline in net photosynthesis, stomatal conductance and transpiration for both species under NaCl as well as CaCl2 treatments compared to unstressed controls accompanied by the reduction of SPAD index and leaf area.
In several ornamental shrubs, NaCl salinity decreased net assimilation rate through stomatal and/or non stomatal limitations, such as the unbalancing of the electron transport chain and/or the impairment of the Calvin Cycle enzymes (Chaves et al., 2009; Álvarez et al., 2012; Álvarez and Sánchez-Blanco, 2014; Cirillo et al., 2016). Consistently with the data obtained for plant growth, in C. citrinus plants, NaCl lowered Pn rate less than CaCl2. The stronger decline of photosynthesis observed under CaCl2 treatment was not accompanied by an equally strong reduction in stomatal conductance. This latter, occurring at the same extent in both the saline treatments was not able to explain alone the dramatic reduction in the leaf photosynthetic rate.
The lower photosynthesis induced by salt treatments may be also a direct consequence of the salt-induced reduction of leaf lamina thickness: indeed, the area-based photosynthetic capacity is directly proportional to leaf thickness (Wyka et al., 2012). However, while C. citrinus plants exposed to NaCl salinity may partly compensate such a reduction in potential photosynthetic capacity thanks to the occurrence of more frequent but smaller stomata; the lack of such a structural adjustment in CaCl2-treated plants would support their more severe decline in photosynthesis. Indeed, the occurrence of more frequent but smaller stomata, exerting a better control of stomatal aperture, has been recorded also in other ornamental species subjected to salinity stress (Carillo et al., 2019), and is recognized as a structural adjustment to achieve a better control of gas exchanges (Raven, 2014).
On the contrary, in V. lucidum salt treated plants responded to both the salt sources with a similar reduction of stomatal conductance and Pn rate. Such common tendency of variation in V lucidum plants in response to the two salt types is maintained also in structural adjustments. V. lucidum NaCl- and CaCl2- treated plants behave as C. citrinum CaCl2-treated plants, developing thinner leaves with smaller, but not more frequent, stomata. The occurrence of thinner lamina under both salt treatments is not due to a reduction in the thickness of the palisade parenchyma, thus indicating the maintenance of the main tissue devoted to photosynthesis. However, the occurrence of less intercellular spaces at the spongy tissue level would also indicate the occurrence of changes in the mesophyll resistance (Sack and Frole, 2006). The increase in the amount of intercellular spaces has often been linked with the ability to cope with salinity by improving the CO2 diffusion in the mesophyll, thus compensating for salinity-induced increased stomatal limitations (Acosta-Motos et al., 2015; Rouphael et al., 2017c; Carillo, 2018). In V. lucidum, the decrease in intercellular spaces would indicate a different strategy pointing at increasing water use efficiency in terms of carbon fixed per water lost through transpiration (McAusland et al., 2016).
The slightest effects exerted by NaCl on the photochemistry of C. citrinus, in particular related to reduction in stomatal conductance or injuries to the photosynthetic apparatus, compared to CaCl2 were probably due to a direct effect of salt stress and were consistent with the lowest RGR (Rodríguez et al., 2005; Moradi and Ismail, 2007). In fact, in C. citrinus plants grown under CaCl2, conversely to plants treated with NaCl, the internal CO2 concentration was increased in sub-stomatal cavities (Ci of NaCl treated plants does not differ compared to control). This supported the hypothesis that, beside stomatal closure, the stronger photosynthetic decline associated with the addition of CaCl2 in the nutritional solution was also due to possible biochemical limitations, as indicated by the reduction in the electron transport rate. On the other hand, V. lucidum plants treated with both salts, exhibited a similar decline in stomatal conductance and transpiration but no changes in internal CO2 concentration. However, in both species, the efficiency of photosystem II in light harvesting and conversion declined under CaCl2 treatment as well as the electron transport rate, suggesting a simultaneous corruption of reductive power and proton gradient generation (for the synthesis of NADPH and ATP) in the light reactions of photosynthesis. This kind of damage might be due to the cytotoxic effect of the increased concentration of Cl- ions in the photosynthetic tissues (Tavakkoli et al., 2010, 2011). At the same time, in these plants, the excess of absorbed light not utilized in photochemistry was dissipated as heat as indicated by the rising non-photochemical quenching (NPQ) in response to the CaCl2 treatment. Under salt stress, as well as under unfavorable environmental conditions, the thermal dissipation within photosystems acts as a safe strategy for cutting down the surplus of light energy and minimizing ROS generation (Azzabi et al., 2012). Nevertheless, such a compensatory mechanism was not able to prevent photoinhibition, as suggested by the significant reduction of Fv/Fm in CaCl2 plants of both species compared to the respective control. A possible reason for the photochemical drop in CaCl2 plants may be related to the observed concurrent reduction of SPAD index and leaf area in these leaves, indicating, respectively, an impairment of the antenna system in light harvesting and/or a limitation in light absorption due to the reduced leaf lamina size, as previously mentioned. Besides, it cannot be excluded that salinity might have induced oxidative stress at the subcellular level, mainly in the chloroplasts, affecting the whole photosynthetic process.
Conclusion
The results of this study allowed discriminating the effects of specific osmotic stress and ion toxicity on Callistemon citrinus and Viburnum lucidum ornamental shrubs, assessing how they activated differential responses to a similar osmotic stress but induced by distinct salinity sources. Both the shrub species displayed multiple adaptive mechanisms to counteract harmful salinity effects, confirming their low sensitivity to a rather high threshold of salt concentration in the irrigation water imposed for a moderately long period. In particular, shoot dry weight, leaf number, total leaf area and net photosynthetic rate were similarly, restrained in both C. citrinus and V. lucidum under NaCl, whereas the two species exhibited a different response to CaCl2 salinity. C. citrinus plants exposed to NaCl salinity may partly compensate such a reduction in potential photosynthetic capacity thanks to the occurrence of more frequent but smaller stomata. On the contrary, V. lucidum responded to both the salt sources, as C. citrinum under CaCl2, developing thinner leaves with smaller, but not more frequent stomata. However, the strong drop in photosynthetic rate of C. citrinus induced by CaCl2, likely ascribed to both stomatal and non stomatal limitations, was more severe than that of the other plant and/or other treatment and determined a stronger growth reduction. In fact, in this species the reduction in photosynthesis occurred together with the decline in PSII photochemistry, and the increase in non-photochemical dissipation processes. However, these plants displayed an increased ability to retain higher Cl- levels in leaves than in roots under CaCl2 salinity compared to V. lucidum, thus, indicating a further attempt to counteract Cl- toxic effects through its increased vacuolar compartmentalization and use as cheap osmoticum. The results of this study may provide useful indications in the selection of shrubs suitable for the urban, industrial and marginal areas’ xeriscaping, when resorting to reclaimed water for plant irrigation may represent a compelling option to water scarcity.
Author Contributions
All authors listed have made a substantial, direct and intellectual contribution to the work, and approved it for publication.
Funding
This work was funded by the Italian Ministry of Education, University and Research Project No. PON01-01611, “Sustainability of Potted Plant Production in a Mediterranean Environment.”
Conflict of Interest Statement
The authors declare that the research was conducted in the absence of any commercial or financial relationships that could be construed as a potential conflict of interest.
The reviewer GF declared a past co-authorship with several of the authors CC, AP, SP, and YR to the handling Editor.
Footnotes
References
Acosta-Motos, J. R., Diaz-Vivancos, P., Álvarez, S., Fernández-García, N., Sánchez-Blanco, M. J., Hernández, J. A., et al. (2015). NaCl-induced physiological and biochemical adaptative mechanisms in the ornamental Myrtus communis L. plants. J. Plant Physiol. 183, 41–51. doi: 10.1016/j.jplph.2015.05.005
Acosta-Motos, J. R., Ortuño, M. F., Bernal-Vicente, A., Diaz-Vivancos, P., Sanchez-Blanco, M. J., and Hernandez, J. A. (2017). Plant responses to salt stress: adaptive mechanisms. Agronomy 7:18. doi: 10.3390/agronomy7010018
Almeida, D. M., Oliveira, M. M., and Saibo, N. J. M. (2017). Regulation of Na+ and K+ homeostasis in plants: towards improved salt stress tolerance in crop plants. Genet. Mol. Biol. 40(Suppl. 1), 326–345. doi: 10.1590/1678-4685-GMB-2016-2106
Álvarez, S., Gómez-Bellot, M. J., Castillo, M., Bañón, S., and Sánchez-Blanco, M. J. (2012). Osmotic and saline effect on growth, water relations, and ion uptake and translocation in Phlomis purpurea plants. Environ. Exp. Bot. 78, 138–145. doi: 10.1016/j.envexpbot.2011.12.035
Álvarez, S., and Sánchez-Blanco, M. J. (2014). Long-term effect of salinity on plant quality, water relations, photosynthetic parameters and ion distribution in Callistemon citrinus. Plant Biol. 16, 757–764. doi: 10.1111/plb.12106
Álvarez, S., and Sánchez-Blanco, M. J. (2015). Comparison of individual and combined effects of salinity and deficit irrigation on physiological, nutritional and ornamental aspects of tolerance in Callistemon laevis plants. J. Plant Physiol. 185, 65–74. doi: 10.1016/j.jplph.2015.07.009
Annunziata, M. G., Ciarmiello, L. F., Woodrow, P., Dell’Aversana, E., and Carillo, P. (2019). Spatial and temporal profile of glycine betaine accumulation in plants under abiotic stresses. Front. Plant Sci. 10:230. doi: 10.3389/fpls.2019.00230
Annunziata, M. G., Ciarmiello, L. F., Woodrow, P., Maximova, E., Fuggi, A., and Carillo, P. (2017). Durum wheat roots adapt to salinity remodeling the cellular content of nitrogen metabolites and sucrose. Front. Plant Sci. 7:2053. doi: 10.3389/fpls.2016.02035
Azzabi, G., Pinnola, A., Betterle, N., Roberto Bassi, R., and Alboresi, A. (2012). Enhancement of non-photochemical quenching in the bryophyte Physcomitrella patens during acclimation to salt and osmotic stress. Plant Cell Physiol. 53, 1815–1825. doi: 10.1093/pcp/pcs124
Bilger, W., and Björkman, O. (1990). Role of xanthophyll cycle and energy dissipation in differently oriented faces of light–induced absorbance changes, fluorescence and photosynthesis in Hedera canariensis. Photosynth. Res. 25, 173–185. doi: 10.1007/BF00033159
Borghesi, E., Carmassi, G., Uguccioni, M. C., Vernieri, P., and Malorgio, F. (2013). Effects of calcium and salinity stress on quality of lettuce in soilless culture. J. Plant Nutr. 36, 677–690. doi: 10.1080/01904167.2012.721909
Borgognone, D., Cardarelli, M., Lucini, L., and Colla, G. (2014). Does CaCl2 play a role in improving biomass yield and quality of cardoon grown in a floating system under saline conditions? HortScience 49, 1523–1528.
Borgognone, D., Rouphael, Y., Cardarelli, M., Lucini, L., and Colla, G. (2016). Changes in biomass, mineral composition, and quality of cardoon in response to NO3-:Cl- ratio and nitrate deprivation from the nutrient solution. Front. Plant Sci. 7:978. doi: 10.3389/fpls.2016.00978
Bremner, J. M. (1965). “Total nitrogen,” in Methods of Soil Analysis, Agronomy Monograph 9, eds C. A. Black, D. D. Evans, I. L. White, L. E. Ensminger, and F. E. Clark (Madison, WI: American Society of Agronomy), 1149–1178.
Carillo, P. (2018). GABA shunt in durum wheat. Front. Plant Sci. 9:100. doi: 10.3389/fpls.2018.00100
Carillo, P., Cirillo, C., De Micco, V., Arena, C., De Pascale, S., and Rouphael, Y. (2019). Morpho-anatomical, physiological and biochemical adaptive responses to saline water of Bougainvillea spectabilis Willd. trained to different canopy shapes. Agric. Water Manag. 212, 12–22. doi: 10.1016/j.agwat.2018.08.037
Cassaniti, C., Leonardi, C., and Flowers, T. J. (2009). The effects of sodium chloride on ornamental shrubs. Sci. Hort. 122, 586–593. doi: 10.1016/j.scienta.2009.06.032
Chaves, M. M., Flexas, J., and Pinheiro, C. (2009). Photosynthesis under drought and salt stress: regulation mechanisms from whole plant to cell. Ann. Bot. 103, 551–560. doi: 10.1093/aob/mcn125
Ciarmiello, L. F., Piccirillo, P., Carillo, P., De Luca, A., and Woodrow, P. (2015). Determination of the genetic relatedness of fig (Ficus carica L.) accessions using RAPD fingerprint and their agro-morphological characterization. S. Afr. J. Bot. 97, 40–47. doi: 10.1016/j.sajb.2014.11.012
Cirillo, C., De Micco, V., Rouphael, Y., Balzano, A., Caputo, R., and De Pascale, S. (2017). Morpho-anatomical and physiological traits of two Bougainvillea genotypes trained to two shapes under deficit irrigation. Trees-Struct. Funct. 31, 173–187. doi: 10.1007/s00468-016-1466-6
Cirillo, C., Rouphael, Y., Caputo, R., Raimondi, G., and De Pascale, S. (2014). The influence of deficit irrigation on growth, ornamental quality, and water use efficiency of three potted Bougainvillea genotypes grown in two shapes. HortScience 49, 1284–1291.
Cirillo, C., Rouphael, Y., Caputo, R., Raimondi, G., Sifola, M. I., and De Pascale, S. (2016). Effects of high salinity and the exogenous application of an osmolyte on growth, photosynthesis, and mineral composition in two ornamental shrubs. J. Hortic. Sci. Biotechnol. 91, 14–22. doi: 10.1080/14620316.2015.1110988
Colla, G., Rouphael, Y., Jawad, R., Kumar, P., Rea, E., and Cardarelli, M. (2013). The effectiveness of grafting to improve NaCl and CaCl2 tolerance in cucumber. Sci. Hortic. 164, 380–391. doi: 10.1016/j.scienta.2013.09.023
Colla, G., Rouphael, Y., Leonardi, C., and Bie, Z. (2010). Role of grafting in vegetable crops grown under saline conditions. Sci. Hortic. 127, 147–155. doi: 10.1016/j.scienta.2010.08.004
Colla, G., Rouphael, Y., Rea, E., and Cardarelli, M. (2012). Grafting cucumber plants enhance tolerance to sodium chloride and sulfate salinization. Sci. Hortic. 135, 177–185. doi: 10.1016/j.scienta.2011.11.023
Conn, S., and Gilliham, M. (2010). Comparative physiology of elemental distributions in plants. Ann. Bot. 105, 1081–1102. doi: 10.1093/aob/mcq027
Cramer, G. R., Läuchli, A., and Polito, V. S. (1985). Displacement of Ca2+ by Na+ from the plasmalemma of root cells: a primary response to salt stress? Plant Physiol. 79, 207–211. doi: 10.1104/pp.79.1.207
Cuin, T. A., Tian, Y., Betts, S. A., Chalmandrier, R., and Shabala, S. (2009). Ionic relations and osmotic adjustment in durum and bread wheat under saline conditions. Funct. Plant Biol. 36, 1110–1119. doi: 10.1071/fp09051
Dalton, F. N., Maggio, A., and Piccinni, G. (1997). Effect of root temperature on plant response functions for tomato: comparison of static and dynamic salinity stress indices. Plant Soil 192, 307–319. doi: 10.1023/A:1004263505595
De Groot, C. C., Marcelis, L. F. M., Van den Boogaard, R., and Lambers, H. (2001). “Regulation of growth by phosphorus supply in whole tomato plants,” in Plant Nutrition Developments in Plant and Soil Sciences, eds W. J. Horst, et al. (Dordrecht: Springer), 114–115. doi: 10.1007/0-306-47624-x_54
De Micco, V., Arena, C., Vitale, L., Aronne, G., and Virzo De Santo, A. (2011). Anatomy and photochemical behaviour of Mediterranean Cistus incanus winter leaves under natural outdoor and warmer indoor conditions. Botany 89, 677–688. doi: 10.1139/b11-059
de Sousa Júnior, J. R., Soares de Lima, G., Gheyi, H. R., Antunes de Lima, V. L., Batista dos Santos, J., Marques de Sousa, J. R., et al. (2017). Gas exchange and production of sunflower (Helianthus annuus L.) irrigated with water of different salinity, cationic nature and nitrogen doses. Aust. J. Crop Sci. 11, 300–307. doi: 10.21475/ajcs.17.11.03.pne441
Ding, W., Clode, P. L., Clements, J. C., and Lambers, H. (2018). Sensitivity of different Lupinus species to calcium under a low phosphorus supply. Plant Cell Environ. 41, 1512–1523. doi: 10.1111/pce.13179
Ezlit, Y. D., Smith, R. J., and Raine, R. S. (2010). A Review of Salinity and Sodicity in Irrigation, Irrigation Matters Series. Toowoomba, AUS: Cooperative Research Centre for Irrigation Futures.
Fascella, G., Mammano, M. M., Rouphael, Y., and Cirillo, C. (2017). Agronomical and physiological responses of containerized ornamentals to salinity induced by major nutrients. Acta Hort. 1170, 635–642. doi: 10.17660/ActaHortic.2017.1170.79
Ferchichi, S., Hessini, K., Dell’Aversana, E., D’Amelia, L., Woodrow, P., Ciarmiello, L. F., et al. (2018). Hordeum vulgare and Hordeum maritimum respond to extended salinity stress displaying different temporal accumulation pattern of metabolites. Funct. Plant Biol. 45, 1096–1109. doi: 10.1071/FP18046
Gao, Y., Lu, Y., Wu, M., Liang, E., Li, Y., Zhang, D., et al. (2016). Ability to remove Na+ and retain K+ correlates with salt tolerance in two maize inbred lines seedlings. Front. Plant Sci. 7:1716. doi: 10.3389/fpls.2016.01716
García-Caparrós, P., and Lao, M. T. (2018). The effects of salt stress on ornamental plants and integrative cultivation practices. Sci. Hortic. 240, 430–439. doi: 10.1016/j.scienta.2018.06.022
Geilfus, C. M. (2018). Chloride: from nutrient to toxicant. Plant Cell Physiol. 59, 877–886. doi: 10.1093/pcp/pcy071
Genty, B., Briantais, J. M., and Baker, N. R. (1989). The relationship between the quantum yield of photosynthetic electron transport and quenching of chlorophyll fluorescence. BBA-Gen Subjects 990, 87–92.
Gori, R., Lubello, C., Ferrini, F., Nicese, F. P., and Coppini, E. (2008). Reuse of industrial wastewater for the irrigation of ornamental plants. Water Sci. Technol. 57, 883–889. doi: 10.2166/wst.2008.185
Grattan, S. V., and Grieve, C. M. (1999). “Mineral nutrient acquisition and response by plants grown in saline environments,” in Handbook of Plant and Crop Stress, ed. M. Pessarakli (New York, USA: Marcel Dekker Inc.), 203–229. doi: 10.1201/9780824746728.ch9
Greenway, H., and Munns, R. (1980). Mechanisms of salt tolerance in nonhalophytes. Annu. Rev. Plant Physiol. 31, 149–190. doi: 10.1146/annurev.pp.31.060180.001053
Hsiao, T. C., and Xu, L. K. (2000). Sensitivity to growth of roots versus leaves to water stress: biophysical analysis and relation to water transport. J. Exp. Bot. 51, 1595–1616. doi: 10.1093/jexbot/51.350.1595
Ju, J. H., Yeum, K. J., Son, H. M., and Yoon, Y. H. (2018). Morphological and physiological responses of purple Chrysanthemum (Aster sphathulifolius) under long-term stress of calcium chloride as deicing salt. Appl. Ecol. Environ. Res. 16, 605–616. doi: 10.15666/aeer/1601_605616
Kitajima, M., and Butler, W. L. (1975). Excitation spectra for photosystem I and photosystem II in chloroplasts and the spectral characteristics of the distributions of quanta between the two photosystems. Biochim. Biophys. Acta 408, 297–305. doi: 10.1016/0005-2728(75)90131-0
Korkmaz, A., Karagöl, A., and Akınoğlu, G. (2017). The effects of CaCl2 on fruit yield, quality and nutrient contents of tomato under NaCl stress conditions. Eurasian J. Soil Sci. 6, 84–91. doi: 10.18393/ejss.284270
Krall, J. P., and Edwards, G. E. (1992). Relationship between photosystem II activity and CO2 fixation in leaves. Physiol. Plant. 86, 180–187. doi: 10.1111/j.1399-3054.1992.tb01328.x
Latz, A., Mehlmer, N., Zapf, S., Mueller, T. D., Wurzinger, B., Pfister, B., et al. (2013). Salt stress triggers phosphorylation of the Arabidopsis vacuolar K+ channel TPK1 by calcium-dependent protein kinases (CDPKs). Mol. Plant 6, 1274–1289. doi: 10.1093/mp/sss158
Läuchli, A., and Epstein, E. (1990). Plant responses to saline and sodic conditions. Agri. Salinity Assess. Manag. 71, 113–137.
Lippi, G., Serra, G., Vernieri, P., and Tognoni, F. (2003). Response of potted callistemon species to high salinity. Acta Hortic. 609, 247–250. doi: 10.17660/ActaHortic.2003.609.36
Liu, Q., Sun, Y., Niu, G., Altland, J., Chen, L., and Jiang, L. (2017). Morphological and physiological responses of ten ornamental taxa to saline water irrigation. HortScience 52, 1816–1822. doi: 10.21273/HORTSCI12463-17
Lucini, L., Borgognone, D., Rouphael, Y., Cardarelli, M., Bernardi, J., and Colla, G. (2016). Mild potassium chloride stress alters the mineral composition, hormone network, and phenolic profile in artichoke leaves. Front. Plant Sci. 7:948. doi: 10.3389/fpls.2016.00948
Lucini, L., Rouphael, Y., Cardarelli, M., Canaguier, R., Kumar, P., and Colla, G. (2015). The effect of a plant-derived biostimulant on metabolic profiling and crop performance of lettuce grown under saline conditions. Sci. Hortic. 182, 124–133. doi: 10.1016/j.scienta.2014.11.022
McAusland, L., Vialet-Chabrand, S., Davey, P., Baker, N. R., Brendel, O., and Lawson, T. (2016). Effects of kinetics of light-induced stomatal responses on photosynthesis and water-use efficiency. New Phytol. 211, 1209–1220. doi: 10.1111/nph.14000
Moradi, F., and Ismail, A. M. (2007). Responses of photosynthesis, chlorophyll fluorescence and ROS-scavenging systems to salt stress during seedling and reproductive stages in rice. Ann. Bot. 99, 1161–1179. doi: 10.1093/aob/mcm052
Munns, R., and Tester, M. (2008). Mechanisms of salinity tolerance. Annu. Rev. Plant Biol. 59, 651–681. doi: 10.1146/annurev.arplant.59.032607.092911
Navarro, A., Bañón, S., Conejero, W., and Sánchez-Blanco, M. J. (2008). Ornamental characters, ion accumulation and water status in Arbutus unedo seedlings irrigated with saline water and subsequent relief and transplanting. Environ. Exp. Bot. 62, 364–370. doi: 10.1016/j.envexpbot.2007.10.010
Nazrul Islam, M., and Kawasaki, M. (2015). Evaluation of calcium regulating roles of guttation and calcium oxalate crystals in leaf blades and petioles of hydroponically grown eddo. Plant Prod. Sci. 18, 11–21. doi: 10.1626/pps.18.11
Nedjimi, B., Daoud, J., and Touati, M. (2006). Growth, water relations, proline and ion content of in vitro cultured Atriplex halimus subsp. schweinfurthii as affected by CaCl2. CBCS 1, 79–89.
Ntatsi, G., Aliferis, K. A., Rouphael, Y., Napolitano, F., Makris, K., Kalala, G., et al. (2017). Salinity source alters mineral composition and metabolism of Cichorium spinosum. Environ. Exp. Bot. 141, 113–123. doi: 10.1016/j.envexpbot.2017.07.002
Pagter, M., Bragato, C., Malagoli, M., and Brix, H. (2009). Osmotic and ionic effects of NaCl and Na2SO4 salinity on Phragmites australis. Aqua. Bot. 90, 43–51. doi: 10.1016/j.aquabot.2008.05.005
Parvin, K., Nahar, K., Hasanuzzaman, M., Bhuyan, M. H. M., and Fujita, M. (2019). “Calcium-mediated growth regulation and abiotic stress tolerance in plants,” in Plant Abiotic Stress Tolerance, eds M. Hasanuzzaman, K. R. Hakeem, K. Nahar, and H. Alharby (Berlin: Springer International Publishing), 291–331.
Quick, W. P., and Horton, P. (1984). Studies on the induction of chlorophyll fluorescence in barley protoplasts. II. resolution of fluorescence quenching by redox state and the transthylakoid pH gradient. Proc. R. Soc. Lon. Series B Biol. Sci. 220, 371–382.
Rabhi, M., Farhat, N., Msilini, N., Rajhi, H., Smaoui, A., Abdelly, C., et al. (2018). Physiological responses of Carthamus tinctorius to CaCl2 salinity under Mg-sufficient and Mg-deficient conditions. Flora 246-247, 96–101. doi: 10.1016/j.flora.2018.07.008
Reale, L., Gigante, D., Landucci, F., Ferranti, F., and Venanzoni, R. (2012). Morphological and histo-anatomical traits reflect die-back in Phragmites australis (Cav.) Steud. Aquat. Bot. 103, 122–128. doi: 10.1016/j.aquabot.2012.07.005
Renault, S., and Affifi, M. (2009). Improving NaCl resistance of red-osier dogwood: role of CaCl2 and CaSO4. Plant Soil 315, 123–133. doi: 10.1007/s11104-008-9737-7
Rodríguez, P., Torrecillas, A., Morales, M. A., Ortuño, M. F., and Sánchez-Blanco, M. J. (2005). Effects of NaCl salinity and water stress on growth and leaf water relations of Asteriscus maritimus plants. Environ. Exp. Bot. 53, 113–123. doi: 10.1016/j.envexpbot.2004.03.005
Rouphael, Y., Cardarelli, M., Bonini, P., and Colla, G. (2017a). Synergistic action of a microbial-based biostimulant and a plant derived-protein hydrolysate enhances lettuce tolerance to alkalinity and salinity. Front. Plant Sci. 8:131. doi: 10.3389/fpls.2017.00131
Rouphael, Y., De Micco, V., Arena, C., Raimondi, G., Colla, G., and De Pascale, S. (2017b). Effect of Ecklonia maxima seaweed extract on yield, mineral composition, gas exchange, and leaf anatomy of zucchini squash grown under saline conditions. J. Appl. Phycol. 29, 459–470. doi: 10.1007/s10811-016-0937-x
Rouphael, Y., Colla, G., Giordano, M., El-Nakhel, C., Kyriacou, M. C., and De Pascale, S. (2017c). Foliar applications of a legume-derived protein hydrolysate elicit dose dependent increases of growth, leaf mineral composition, yield and fruit quality in two greenhouse tomato cultivars. Sci. Hortic. 226, 353–360. doi: 10.1016/j.scienta.2017.09.007
Rouphael, Y., Colla, G., Bernardo, L., Kane, D., Trevisan, M., and Lucini, L. (2016). Zinc excess triggered polyamines accumulation in lettuce root metabolome as compared to osmotic stress under high salinity. Front. Plant Sci. 7:842. doi: 10.3389/fpls.2016.00842
Rouphael, Y., Raimondi, G., Lucini, L., Carillo, P., Kyriacou, M. C., Colla, G., et al. (2018). Physiological and metabolic responses triggered by omeprazole improve tomato plant tolerance to NaCl stress. Front. Plant Sci. 9:249. doi: 10.3389/fpls.2018.00249
Ruzin, S. E. (1999). Plant Microtechnique and Microscopy. New York, NY: Oxford University Press, doi: 10.2307/1224595
Sack, L., and Frole, K. (2006). Leaf structural diversity is related to hydraulic capacity in tropical rain forest trees. Ecology 87, 483–491. doi: 10.1890/05-0710
Saggaï, M. M., Ainouche, A., Nelson, M., Cattin, F., and El Amrani, A. (2017). Long-term investigation of constructed wetland wastewater treatment and reuse: selection of adapted plant species for metaremediation. J. Environ. Manag. 201, 120–128. doi: 10.1016/j.jenvman.2017.06.040
Scagel, C. F., Bryla, D. R., and Lee, J. (2017). Salt exclusion and mycorrhizal symbiosis increase tolerance to NaCl and CaCl2 salinity in ‘Siam Queen’. Basil. HortScience 52, 278–287. doi: 10.21273/HORTSCI11256-16
Scholander, P. F., Hammel, H. T., Bradstreet, E. D., and Hemmingsen, E. A. (1965). Sap pressure in vascular plants. Science 148, 339–346. doi: 10.1126/science.148.3668.339
Shabala, S., and Munns, R. (2012). “Salinity stress: physiological constraints and adaptive mechanisms,” in Plant Stress Physiology, ed. S. Shabala (United Kingdom, UK: CABI), doi: 10.1079/9781845939953.0059
Tattini, M., Remorini, D., Pinelli, P., Agati, G., Saracini, E., Traversi, M. L., et al. (2006). Morpho-anatomical, physiological and biochemical adjustments in response to root zone salinity stress and high solar radiation in two Mediterranean evergreen shrubs, Myrtus communis and Pistacia lentiscus. New Phytol. 170, 779–794. doi: 10.1111/j.1469-8137.2006.01723.x
Tavakkoli, E., Fatehi, F., Coventry, S., Rengasamy, P., and McDonald, G. K. (2011). Additive effects of Na+ and Cl- ions on barley growth under salinity stress. J. Exp. Bot. 62, 2189–2203. doi: 10.1093/jxb/erq422
Tavakkoli, E., Rengasamy, P., and McDonald, G. K. (2010). High concentrations of Na+ and Cl- ions in soil solution have simultaneous detrimental effects on growth of faba bean under salinity stress. J. Exp. Bot. 61, 4449–4459. doi: 10.1093/jxb/erq251
Turner, N. C., and Long, M. J. (1980). Errors arising from rapid water loss in the measurement of leaf water potential by the pressure chamber technique. Func. Plant Biol. 7, 527–537. doi: 10.1071/PP9800527
Vernieri, P., Mugnai, S., Borghesi, E., Petrognani, L., and Serra, G. (2006). Non-chemical growth control of potted Callistemon laevis. Agric. Med. 160, 85–90.
Volkmar, K., Hu, Y., and Steppuhn, H. (1998). Physiological responses of plants to salinity: a review. Can. J. Plant Sci. 78, 19–27. doi: 10.4141/P97-020
Wang, M., Zheng, Q., Shen, Q., and Guo, S. (2013). The critical role of potassium in plant stress response. Int. J. Mol. Sci. 14, 7370–7390. doi: 10.3390/ijms14047370
White, P. J., and Broadley, M. R. (2003). Calcium in plants. Ann. Bot. 92, 487–511. doi: 10.1093/aob/mcg164
Wilkins, K. A., Matthus, E., Swarbreck, S. M., and Davies, J. M. (2016). Calcium-mediated abiotic stress signaling in roots. Fron. Plant Sci. 7:1296. doi: 10.3389/fpls.2016.01296
Woodrow, P., Ciarmiello, L. F., Annunziata, M. G., Pacifico, S., Iannuzzi, F., Mirto, A., et al. (2017). Durum wheat seedling responses to simultaneous high light and salinity involve a fine reconfiguration of amino acids and carbohydrate metabolism. Physiol. Plant. 159, 290–312. doi: 10.1111/ppl.12513
Woodrow, P., Pontecorvo, G., Ciarmiello, L., Fuggi, A., and Carillo, P. (2011). Ttd1a promoter is involved in DNA-protein binding by salt and light stresses. Mol. Biol. Rep. 38, 3787–3794. doi: 10.1007/s11033-010-0494-493
Wyka, T. P., Oleksyn, J., Zytkowiak, R., Karolewski, P., Jagodziński, A. M., and Reich, P. B. (2012). Responses of leaf structure and photosynthetic properties to intra-canopy light gradients: A common garden test with four broadleaf deciduous angiosperm and seven evergreen conifer tree species. Oecologia 170, 11–24. doi: 10.1007/s00442-012-2279-y
Keywords: arrow-wood, chlorophyll fluorescence, functional leaf anatomical traits, gas exchanges, osmoregulation, red bottlebrush, SPAD index, water relations
Citation: Cirillo C, De Micco V, Arena C, Carillo P, Pannico A, De Pascale S and Rouphael Y (2019) Biochemical, Physiological and Anatomical Mechanisms of Adaptation of Callistemon citrinus and Viburnum lucidum to NaCl and CaCl2 Salinization. Front. Plant Sci. 10:742. doi: 10.3389/fpls.2019.00742
Received: 07 January 2019; Accepted: 20 May 2019;
Published: 04 June 2019.
Edited by:
Susana M. P. Carvalho, Universidade do Porto, PortugalReviewed by:
Longxing Hu, Hunan Agricultural University, ChinaGiancarlo Fascella, Council for Agricultural and Economics Research, Italy
Copyright © 2019 Cirillo, De Micco, Arena, Carillo, Pannico, De Pascale and Rouphael. This is an open-access article distributed under the terms of the Creative Commons Attribution License (CC BY). The use, distribution or reproduction in other forums is permitted, provided the original author(s) and the copyright owner(s) are credited and that the original publication in this journal is cited, in accordance with accepted academic practice. No use, distribution or reproduction is permitted which does not comply with these terms.
*Correspondence: Chaira Cirillo Y2hhaXJhLmNpcmlsbG9AdW5pbmEuaXQ=; Youssef Rouphael, eW91c3NlZi5yb3VwaGFlbEB1bmluYS5pdA==