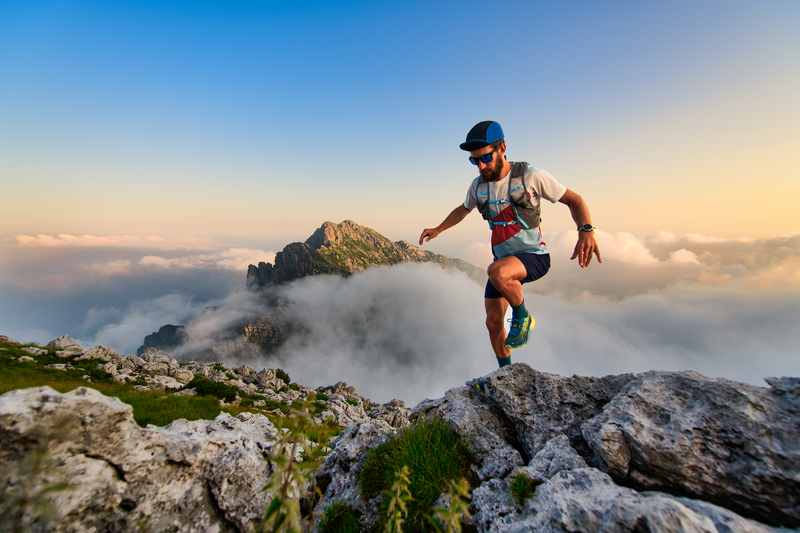
95% of researchers rate our articles as excellent or good
Learn more about the work of our research integrity team to safeguard the quality of each article we publish.
Find out more
ORIGINAL RESEARCH article
Front. Plant Sci. , 24 May 2019
Sec. Plant Physiology
Volume 10 - 2019 | https://doi.org/10.3389/fpls.2019.00597
This article is part of the Research Topic Modulation of Stomatal Response by Elevated CO2 in Plants under Drought and Heat Stress View all 6 articles
While nitrogen (N) derived from ammonium would be energetically less expensive than nitrate-derived N, the use of ammonium-based fertilizer is limited by the potential for toxicity symptoms. Nevertheless, previous studies have shown that exposure to elevated CO2 favors ammonium assimilation in plants. However, little is known about the impact of different forms of N fertilizer on stomatal opening and their consequent effects on CO2 and H2O diffusion in wheat plants exposed to ambient and elevated CO2. In this article, we have examined the response of the photosynthetic machinery of durum wheat (Triticum durum, var. Amilcar) grown with different types of N fertilizer (NO3−, NH4+, and NH4NO3) at 400 versus 700 ppm of CO2. Alongside gas exchange and photochemical parameters, the expression of genes involved in CO2 (PIP1.1 and PIP2.3) and H2O (TIP1) diffusion as well as key C and N primary metabolism enzymes and metabolites were studied. Our results show that at 400 ppm CO2, wheat plants fertilized with ammonium as the N source had stress symptoms and a strong reduction in stomatal conductance, which negatively affected photosynthetic rates. The higher levels of PIP1.1 and PIP2.3 expression in ammonium-fertilized plants at 400 ppm CO2 might reflect the need to overcome limitations to the CO2 supply to chloroplasts due to restrictions in stomatal conductance. This stomatal limitation might be associated with a strategy to reduce ammonium transport toward leaves. On the other hand, ammonium-fertilized plants at elevated CO2 did not show stress symptoms, and no differences were detected in stomatal opening or water use efficiency (WUE). Moreover, similar gene expression of the aquaporins TIP1, PIP1.1, and PIP2.3 in ammonium-fertilized plants grown at 700 ppm compared to nitrate and ammonium nitrate plants would suggest that an adjustment in CO2 and H2O diffusion is not required. Therefore, in the absence of a stress context triggered by elevated CO2, ammonium- and ammonium nitrate-fertilized plants were able to increase their photosynthetic rates, which were translated eventually into higher leaf protein content.
The increase in the world’s population predicted for the end of the twenty-first century1 will require that intensive agriculture employs large amounts of nitrogen (N) to increase maximum cereal yields.
Both nitrate and ammonium are the major N forms available in soils for plants (Andrews et al., 2013; Tegeder and Masclaux-Daubresse, 2017). In both natural and agricultural soils, microbial processes in soils favor a mixed nutrition with fluctuating contents of the respective sources depending on the environmental conditions of temperature and soil water content and the type of fertilizers applied, among other factors (Benckiser et al., 2015). In addition, when both inorganic-N sources are available in plants, ammonium is preferentially taken up and assimilated into organic molecules due to its more chemically reduced status than nitrate, thus saving metabolic energy during its assimilation (Andrews et al., 2013). Nevertheless, the application of ammonium as the sole N source generally elicits toxicity symptoms in many plant species (Britto and Kronzucker, 2002). In addition to the visual symptoms expressed as a global reduced plant growth or leaf chlorosis, excess ammonium causes an alteration in expression and activity of N-assimilating enzymes, disruptions in photosynthesis and hormonal homeostasis, deficiency in ion balance, or induction of higher photorespiration rates (Guo et al., 2007; Esteban et al., 2016). However, these effects are dependent on plant species, variety, or accession, as well as external ammonium availability (Britto and Kronzucker, 2002; Esteban et al., 2016). In addition, environmental factors such as light intensity (Ariz et al., 2011; Setién et al., 2013), pH conditions (Sarasketa et al., 2016), the external N concentration (Vega-Mas et al., 2015), and atmospheric CO2 concentration (Nimesha et al., 2017; Rubio-Asensio and Bloom, 2017; Vega-Mas et al., 2017) determine the threshold for ammonium toxicity. According to these studies, an adequate availability of photoassimilates is essential to maintain the internal ammonium homeostasis in the plant cell, thus avoiding toxic effects associated with ammonium nutrition (Vega-Mas et al., 2017).
Plants modulate stomatal opening in order to optimize the rate of CO2 diffusion and to minimize water loss, having a key role in photosynthetic machinery and water transport. The regulation of stomatal opening and closure has an important effect in limiting transpiration and therefore on water and nutrient transport through the plant. Increasing CO2 concentrations, exposure to heavy metals, and saline and soil water stress are environmental stimuli described as reducing the transpiration rate (Long et al., 2004; Bastías et al., 2010; Jauregui et al., 2015; Rucińska-Sobkowiak, 2016). Although exposure to elevated CO2 and toxic compounds such as surplus external ammonium induces stomatal closure (López-Millán et al., 2009; Aranjuelo et al., 2014), information on the involvement of ammonium in water transport within the plant is scarce. In addition to stomatal regulation, most water transport is mediated by aquaporins (AQPs) (Maurel et al., 1997; Henzler et al., 2004). Moreover, these water transport proteins have also been reported to have a role in responding to salinity, osmotic stress, or ammonia transport (Forrest and Bhave, 2007). More specifically, to maintain water balance and photosynthetic performance because AQPs can also mediate the flux of small non-charged molecules, in particular CO2, ammonium/ammonia, and urea (Ding et al., 2018; Pawłowicz and Masajada, 2019). Therefore, stomatal regulation together with the regulation of AQPs (abundance or activity) in plants may be essential to withstand future scenarios of elevated CO2 conditions and changing agricultural N management.
Plants are able to adapt their biochemical and physiological strategies to assimilate different N sources into organic compounds under changing CO2 atmospheres. The predicted rise in atmospheric CO2 would favor plant development under a nutrition based on ammonium fertilization because larger quantities of photoassimilates would be available for primary ammonium assimilation (Vega-Mas et al., 2015). Indeed, an elevated CO2 atmosphere promotes plant growth, which in turn overcomes the associated ammonium toxicity (Vega-Mas et al., 2015). Nevertheless, the photosynthetic response to elevated CO2 does not only depend on CO2 as a substrate, but also other physiological and environmental factors have effect on that response (Xu et al., 2016). Frequently, under elevated CO2 conditions, plants decrease stomatal conductance, which avoids water loss and increases water use efficiency (WUE). Under this scenario, the modulation of AQPs may be a determinant for absorption and assimilation of different N sources.
The present work has been conceived to evaluate the effect of elevated CO2 conditions on stomatal functioning and aquaporins involved in the uptake of water and nutrients in wheat plants. For this, durum wheat plants (Triticum durum Def. cv. Amilcar) were grown at two different concentrations of atmospheric CO2 (400 and 700 ppm) on different forms of N nutrition (NO3−, NH4+, and NH4NO3) for 2 weeks after a nitrate adaptation over 5 weeks. We observed that stomatal conductance is central to the responsiveness of ammonium-fertilized plants. Stomatal closure acts under ambient CO2 conditions to prevent massive ammonium accumulation in shoots, while at elevated CO2 conditions, the stomatal aperture ameliorates carbon and ammonium assimilation and minimizes symptoms of stress symptoms derived from ammonium nutrition.
Seeds of wheat plants (Triticum durum L. cv. Amilcar) were germinated on trays filled with perlite:vermiculite (1:1, v:v) and watered with deionized water. Seeds were maintained for 10 days in darkness and at 4°C to synchronize the germination. After this, seedlings were transferred to 5 L hydroponic pots in two independently controlled environmental chambers (Phytotron Service, SGIker, UPV/EHU), under 550 μmol m−2 s−1 light intensity, 25/17°C temperature, and 50/60% relative humidity during the 14/10 h of the day/night-photoperiod, respectively. Plants were grown under two different controlled atmospheres of 400 and 700 ppm CO2 levels. Hoagland solution (Arnon and Hoagland, 1940) was replaced three times per week. Wheat plants at Z39 (Zadoks scale) were grown for 5 weeks under nitric nutrition based on calcium nitrate. Afterward, for the following 2 weeks, the N source was modified with ammonium sulfate (NH4+) or ammonium nitrate (NH4NO3), keeping in parallel a set of control plants under nitrate nutrition (NO3−). The N source was supplied at a rate of 10 mM N. Gas exchange determinations were measured in flag leaves of wheat plants at Z51 (Zadoks scale) between 2 and 5 h after onset the photoperiod. After gas exchange parameter determination in flag leaves of four plants from each of the CO2 condition, shoot of wheat plants was harvested and dried in an oven at 80°C for 72 h for shoot biomass determination. For metabolic and enzymatic analysis, totally expanded flag leaves of four plants were harvested and stored at −80°C until further measurements.
Gas-exchange measurements were conducted in totally expanded flag leaves using a Li-COR 6400XP portable photosynthesis system (LI-COR Inc., Lincoln, NE, USA). The rate of CO2 assimilation (AN), stomatal conductance (gs), and intercellular CO2 (Ci) parameters was determined under light-saturated conditions with a photosynthetic photon flux density (PPFD) of 1,200 μmol m−2 s−1 at 25°C and with the reference CO2 concentration of the respective growth chamber. The instantaneous water use efficiency (WUEi) was determined dividing the rate of CO2 assimilation by the stomatal conductance (AN/gs). The thylakoid electron transport rate (ETR) and maximal quantum efficiency of PSII (Fv/Fm) were measured using a Leaf Chamber Fluorometer (LFC 6400–40; Li-COR) coupled to the Li-COR 6400XT portable photosynthesis system 45 min after the dark period started.
Starch was measured from 10 mg of lyophilized powdered samples of flag leaves by modified hydroalcoholic extraction as described by Fuertes-Mendizábal et al. (2010). The dry residue obtained in the hydroalcoholic extraction was resuspended, and starch was determined as glucose equivalents by using a test kit (Boehringer Mannheim, Germany) after α-amylase and amyloglucosidase digestion. Maltose was determined as described by Baslam et al. (2017). Briefly, 0.1 g of frozen plant powder was resuspended in 1 ml of 90% ethanol, incubated for 90 min at 70°C, and centrifuged at 13,000 g for 10 min. Maltose from supernatants was then determined by HPAEC-PAD on a DX-500 Dionex system by gradient separation with a CarboPac PA20 column according to the application method suggested by the supplier. Then, the extract was centrifuged at 13,000 g for 10 min. Inorganic forms of N as nitrate and ammonium were determined according to the methods described by Patton and Crouch (1977) and Cataldo et al. (1974), respectively.
The free amino acid profile was quantified at the Scientific and Technological Center of the University of Barcelona (CCiT UB). Amino acids were extracted from flag leaves homogenized with 1 M HCl (1:20, w:v). After 16 h of incubation at −20°C, the extracts were centrifuged at 10,000 g for 15 min and filtered. Norleucine was added as an internal standard to the five-time diluted amino acid extraction. Afterward, 20 μl of derivatized sample was injected for amino acid determination by HPLC using Waters Delta 600 chromatographic system with a column (Nova-Pak C18 4 μm, 3.9 × 150 mm) and an absorbance detector (Waters 2,487 Dual λ) coupled to an auto sampler (Waters 717plus) using the AccQTag pre-column derivatization method. The reaction of amino acids with 6-aminoquinolyl-N-hydroxysuccinimidyl carbamate yields derivatives that are detected at 254 nm, and their concentrations were calculated according to internal standards (Cohen and Michaud, 1993; Cohen and De Antonis, 1994).
Powdered frozen flag leaves were homogenized in an extraction buffer consisting of 100 mM HEPES pH 7.5, 2 mM EDTA, 2 mM dithiothreitol, 1 mM PMSF, 10 μl ml−1 protease inhibitor cocktail (Sigma P9599), and centrifuged at 14,000 g for 20 min. The supernatant was desalted by ultrafiltration on Vivaspin 500 centrifugal concentrator (Sartorius), and the protein extract thus obtained was assayed for enzymatic activities. Both α- and β-amylolytic activities were assayed as described by Sweetlove et al. (1996). Soluble starch synthase activity was measured in two steps: (1) incubation in a buffer reaction with 50 mM HEPES pH 7.5, 6 mM MgCl2, 3 mM dithiothreitol, 1 mM adenosine diphosphate glucose (ADPG), and 3% glycogen for 5 min at 37°C. After stopping the reaction by boiling for 2 min, (2) the ADP produced was measured by HPLC on a Waters Associates system fitted with a Partisil-10-SAX column. One unit (U) is defined as the amount of enzyme that catalyzes the production of 1 μmol of product per min.
Soluble protein was extracted from powdered frozen flag leaves homogenized with extraction buffer (1:20, w:v) based on Gibon et al. (2004). Soluble protein was measured according to Bradford (1976) from the supernatant recovered after centrifugation at 4,000 g for 30 min at 4°C. Nitrate reductase (NR) was determined as the maximum activity by incubating 50 μl of protein extract for 30 min at 30°C according to Baki et al. (2000). Glutamine synthetase (GS) and aminating-glutamate dehydrogenase activity (NADH-GDH) were determined as described by Sarasketa et al. (2016).
Total RNA was isolated from pulverized flag leaves using a Nucleospin RNA plant kit (Macherey-Nagel) according to the manufacturer’s recommendations. RNA integrity and purity were checked on a 1.5% (v:v) agarose gel, and 1 μg of RNA was retrotranscribed into cDNA using the PrimeScript™ RT reagent kit (Takara Bio Inc.). Gene expression was determined using a StepOne Plus Real-Time PCR System (Applied Biosystems) in a 15 μl reaction using the SYBR Premix ExTaq™ (Takara Bio Inc.), 200 nM of each gene-specific primer of the N-transporter (Vicente et al., 2015) and aquaporin (Jauregui et al., 2015) genes, and 2 μl of cDNA diluted 1:10. The PCR thermal profile was: 95°C for 10 min, 40 cycles (95°C for 15 s and 60°C for 1 min) and a final step to obtain the melting curve. Relative expression was calculated as the ∆Cp between each gene and the geometric average of the reference genes actin and RNase L inhibitor-like protein (Giménez et al., 2011).
Data sets were analyzed using IBM SPSS Statistics 22 software (Armonk, NY, USA). Normality of residuals and homogeneity of variance were analyzed with the Kolmogorov-Smirnov and Levene tests. The data that did not pass normality test were transformed. The effect size of each factor and their interactions were determined with the use of partial eta-squared, which describe a proportion of variability in a sample associated with an independent variable. Significant differences between N treatments and CO2 conditions were analyzed using multifactorial ANOVA followed by LSD test. All analyses were performed at a significance level, p < 0.05.
Growth of the durum wheat cv. Amilcar showed different behaviors depending on the N form supplied in the nutrient solution and the CO2 environmental conditions. Both nitrate and ammonium nitrate nutrition showed the highest biomass production, regardless the CO2 conditions. However, plants grown under exclusive ammonium nutrition showed a biomass 32 and 42% lower than nitrate-fertilized plants at ambient and elevated CO2 conditions, respectively (Table 2). In addition, plants fertilized with mixed nutrition of ammonium nitrate had increased shoot biomass compared to the respective plants at ambient CO2. However, no CO2-effect was observed for ammonium nutrition; thus, the biomass of plants grown under ammonium nutrition was reduced by about 40% with respect to the treatment fertilized with nitrate (Table 2).
Gas-exchange parameters measured in flag leaves of wheat plants at Z51 (Zadoks scale) were more affected by N source than by CO2 concentration (Table 1). In addition, the effect size of each factor was different (Table 1). Ammonium- and ammonium nitrate-fertilized plants grown under ambient CO2 conditions had a lower CO2 assimilation rate (AN), stomatal conductance (gs), and intercellular CO2 concentration (Ci) than nitrate-fertilized plants (Figures 1A–C). Moreover, the WUEi was higher in wheat plants grown at ambient CO2 conditions fertilized with ammonium or ammonium nitrate than under nitrate nutrition (Figure 1D). In addition, WUEi was higher in ammonium-fertilized plants than those fertilized with ammonium nitrate (Figure 1D). Under elevated CO2 conditions, only plants fertilized with nitrate showed lower stomatal conductance than their respective control plants grown at ambient CO2 (Figure 1B), but the photosynthetic rate was higher than the respective plants grown under ambient CO2 conditions (Figure 1A). By contrast, plants fertilized with ammonium nutrition exposed to elevated CO2 showed higher photosynthetic assimilation rates and stomatal conductance than the respective plants grown under ambient CO2 conditions (Figures 1A,B). Interestingly, ammonium nitrate-fertilized plants also showed an enhanced photosynthetic rate at elevated CO2 relative to ambient CO2 conditions, and in such a way that they showed values equal to the nitrate-fertilized plants (Figure 1A). The exposure to elevated CO2 increased WUEi in nitrate-fertilized plants but decreased WUEi in ammonium-fertilized plants as a consequence of the direct CO2 effect on stomatal conductance (Figure 1D).
Table 1. Significance and size effect of each factor (CO2 concentration and N source) and their interactions on the different variables measured.
Table 2. Shoot biomass (g DW plant−1) of wheat plants grown under different N source and ambient (400 ppm CO2) or elevated CO2 conditions (700 ppm CO2).
Figure 1. Net photosynthetic rate (A), stomatal conductance (B), intercellular CO2 mole fraction (C), instantaneous water use efficiency (D), maximal quantum efficiency of PSII (E), and thylakoid electron transport rate (F) of wheat plant grown under ambient (400 ppm CO2, grey bars) and elevated (700 ppm CO2, black bars) CO2 conditions. Significant differences (p < 0.05) between N treatments and CO2 conditions are indicated with different letters. Values represent mean ± SEM (n = 3).
Fv/Fm value of 0.83 was significantly lower under ammonium nutrition at ambient CO2 conditions than nitrate nutrition (Figure 1E). However, both ammonium and ammonium nitrate nutrition resulted in a reduced thylakoid electron transport rate (ETR) with respect to nitrate nutrition under ambient CO2 (Figure 1F). At the same time, and regardless of the nitrogen nutrition, elevated CO2 conditions stimulated the thylakoid ETR relative to plants grown at ambient CO2 (Figure 1F). Although differences in ETR among the N treatments were minimal for plants grown at elevated CO2, the highest values being observed in ammonium nitrate-fertilized plants (Figure 1F).
Aquaporins are protein channels involved in the flow of water, CO2, and even ammonia, and they can respond to environmental conditions. Aquaporin expressions were affected exclusively by the N source, but not by CO2 concentration (Table 1). Wheat plants grown under ammonium nutrition at ambient CO2 conditions presented a downregulation of tonoplast aquaporin TIP1 (Figure 2A) with respect to nitrate-fertilized plants but had stimulated expression of the PIP1.1 and PIP2.3 aquaporins in the plasma membrane (Figures 2B,C). These changes in aquaporin gene expression were not detected under elevated CO2. Plants exposed to elevated CO2 conditions showed higher expression of AMT2.1 (Figure 2D) than their respective controls at ambient CO2. Nitrate nutrition downregulated the expression of the NRT1.1 (Figure 2E) nitrate transporter under elevated CO2 conditions, and the expression of NRT1.7 (Figure 2F) showed a large stimulation in ammonium-fertilized plants at ambient CO2 conditions.
Figure 2. Aquaporins TIP1 (A), PIP1.1 (B), and PIP2.3 (C) and on nitrogen transporters AMT2.1 (D), NRT1.1 (E), and NRT1.7 (F) in leaves of wheat plants grown under ambient (400 ppm CO2, grey bars) and elevated (700 ppm CO2, black bars) CO2 conditions. Significant differences (p < 0.05) between N treatments and CO2 conditions are indicated with different letters. Values represent mean ± SEM (n = 4).
Photoassimilate levels were represented by the polysaccharide starch and soluble maltose, which are a product of starch degradation. Only total amylase and its product, maltose, were affected by CO2 concentration and N source, but not by its interaction (Table 1). Nitrate-fertilized plants exposed to elevated CO2 increased soluble starch synthase and reduced total amylase activity (Figures 3A,B), thus increasing the starch content relative to those grown at ambient CO2 (Figure 3C). Regarding maltose levels, plants fertilized with ammonium at ambient CO2 showed the highest contents of this sugar (Figure 3D). Furthermore, the maltose content was depleted in wheat plants, regardless the N source under elevated CO2 conditions.
Figure 3. Soluble starch synthase (A) and total amylase (B) activities and carbohydrate contents of starch (C) and maltose (D) in leaves of wheat plant grown under ambient (400 ppm CO2, grey bars) and elevated (700 ppm CO2, black bars) CO2 conditions. Significant differences (p < 0.05) between N treatments and CO2 conditions are indicated with different letters. Values represent mean ± SEM (n = 4).
While no significant interaction was detected between the main factors, the two factors assayed (namely CO2 concentration and N source) significantly affected (Table 1) ammonium content and its incorporation in amino acids and proteins, but interactions between these factors were not observed. As expected, ammonium nutrition caused an ammonium accumulation in leaves, regardless the atmospheric CO2 concentration (Figure 4A). Similarly, wheat plants grown under ammonium nutrition at ambient CO2 conditions had the highest values of total amino acids, while exposure to elevated CO2 conditions reduced the total amino acid content in these plants (Figure 4B). Moreover, exposure to elevated CO2 increased the total soluble protein content (Figure 4C). In accordance with the amino acid contents, wheat plants grown under ammonium and ammonium nitrate nutrition showed considerably increased proline content when they were grown at ambient CO2. However, under elevated CO2 conditions, proline levels dropped drastically (Figure 4D).
Figure 4. Ammonium (A), amino acids (B), soluble protein (C), and proline (D) contents in leaves of wheat plants grown under ambient (400 ppm CO2, grey bars) and elevated (700 ppm CO2, black bars) CO2 conditions. Significant differences (p < 0.05) between N treatments and CO2 conditions are indicated with different letters. Values represent mean ± SEM (n = 3–4).
The activity of N-metabolism enzymes changed depending on the CO2 levels and the type of N nutrition (Figure 5). Under ambient CO2 conditions, plants grown under ammonium nutrition showed lower NR and GS activities than nitrate nutrition (Figures 5A,B) and ammonium nitrate nutrition reduced the GS activity with respect to nitrate nutrition (Figure 5B). The exposure to elevated CO2 stimulated NR and GS activities in plants fertilized with nitrate, without changes in aminating-GDH activity (Figure 5). Meanwhile, ammonium-fertilized plants exposed to elevated CO2 stimulated both GS and aminating-GDH activities (Figures 5B,C). Exposure to elevated CO2 did not stimulate GS activity in plants fertilized with ammonium nitrate, and this activity was lower than observed in nitrate-fertilized plants (Figure 5B). Neither the NR nor the GDH activities indicated any CO2 effect under ammonium nitrate nutrition.
Figure 5. Nitrate reductase (A), glutamine synthetase (B) and aminating glutamate dehydrogenase (C) activities in leaves of wheat plants under ambient (400 ppm CO2, grey bars) and elevated (700 ppm CO2, black bars) CO2 conditions. Significant differences (p < 0.05) between N treatments and CO2 conditions are indicated with different letters. Values represent mean ± SEM (n = 4).
Ammonium fertilization often causes many toxicity symptoms in plants when it is supplied as the sole N source (Britto and Kronzucker, 2002). The overall reduction in growth of wheat shoots in the present work was clear evidence of the noxious effect of ammonium when it is employed as an exclusive N source, and the overcoming of this growth limitation under mixed nutrition with ammonium nitrate lend further support to this finding (Table 2). In agreement with other studies (Setién et al., 2013; Sarasketa et al., 2016; Piñero et al., 2018), our results show that wheat plants grown under exclusive ammonium nutrition at ambient CO2 conditions accumulate ammonium in leaf tissues (Figure 4), reflecting the imbalance between its uptake and its assimilation into organic molecules. Indeed, it is the disruption of cell ammonium homeostasis that leads eventually to poor shoot performance (Table 2). Ammonium-fertilized plants underwent drastic stomatal closure (depletion of gs) at ambient CO2 conditions, which might be a strategy to avoid further ammonium accumulation in leaves. However, this depletion of stomatal conductance caused an inhibition of photosynthetic activity, which has important consequences for plant productivity. Therefore, ammonium fertilization had an impact on photosynthetic assimilation by limiting the CO2 supply to Rubisco as a result of the depletion of stomatal conductance and subsequent depletion of the intercellular CO2 concentration (Ci). The regulation of stomata has been described as a process linked to transport and absorption of nutrients (Aranjuelo et al., 2014). This strategy has been reported under abiotic stresses such as salinity (Wang et al., 2017) or high temperature (Aranjuelo et al., 2006; Jauregui et al., 2015), among other factors.
Water and nutrient movements through the plant and, more specifically, into the cell require a large number of processes in addition to regulation of the stomata and transpiration. Among them, aquaporins are the major factors that regulate the flow of water through the cell. In plants, the main subfamilies of aquaporins are the plasma membrane intrinsic proteins (PIPs) and tonoplast intrinsic proteins (TIPs; Forrest and Bhave, 2007). Likewise, each subfamily is subdivided into groups with various isoforms, which may be present in specific locations or have specific functions. Therefore, although the main role attributed to aquaporins is water transport, the expression of these proteins has also been reported as regulating the transport of other molecules such as ammonia (Bertl and Kaldenhoff, 2007; Forrest and Bhave, 2007; Ding et al., 2016), CO2 (Flexas et al., 2006; Maurel et al., 2008), boron (Durbak et al., 2014), or silicon (Ma and Yamaji, 2006; Rios et al., 2017). According to Flexas et al. (2006), the higher levels of PIP1.1 and PIP2.3 expression detected in ammonium-treated plants at ambient CO2 (Figure 2) could be a mechanism to overcome the CO2-supply limitations that chloroplasts experience under stomatal and mesophyll resistance. Indeed, enhanced aquaporin expression has been considered as a plant strategy for facilitating CO2 diffusion to carboxylation sites in order to maintain the photosynthetic rate (Ding et al., 2016). Furthermore, the regulation of aquaporin genes might be involved in regulating the uptake of water, ammonia, and other molecules in order to redistribute them throughout the cells (Forrest and Bhave, 2007). Accordingly, the expression of the PIP1.1 and PIP2.3 genes (Figure 2) in wheat plants fertilized under ammonium nutrition would explain the efficient use of water by the plant (WUEi) in order to coordinate ammonium accumulation, CO2 assimilation, and H2O diffusion. However, the expression of the tonoplast aquaporin TIP1 was downregulated by ammonium under ambient CO2 conditions. AQPs genes are suggested to be involved in the responsiveness to osmotic stress, saline, and chilling stress (Forrest and Bhave, 2008). Therefore, the pattern of downregulation of TIP1 (Figure 2) observed in ammonium-fertilized plants resembled that of stomatal conductance (Figure 1) and its regulation could be involved in water loss from the vacuole and stomatal closure as a way to reduce the water loss from leaves and to maintain turgidity under stress conditions (Forrest and Bhave, 2007). The regulation of tonoplast AQPs can be considered as evidence of its potential role in the regulation of water or ammonia flow through the vacuole.
On the other hand, it should be also observed that the fact that the depletion in AN and gs values in ammonium-fertilized plants grown under ambient CO2 was more marked than the diminishment in Ci reveals that Rubisco carboxylation together with altered CO2 diffusion within the chloroplast would strongly condition photosynthetic performance. In addition, the lower Fv/Fm detected under ammonium nutrition indicated that ammonium-fertilized plants presented stress symptoms that might have affected Rubisco activity. On the other hand, both gas exchange and the AQPs gene expression analyses would also support the idea that CO2 diffusion from substomatal cavity toward chloroplast (referred to as mesophyll conductance; gm) would have been affected in plants fertilized with ammonium. Previous studies carried out in plants exposed to different environmental conditions, such as leaf CO2, temperature, and water stress remark how gm limits photosynthesis by the availability of CO2 within the chloroplast and how AQPs are involved in gm regulation (Bernacchi et al., 2002; Flexas et al., 2006, 2007; Galmés et al., 2007). Our study showed that ammonium exposure (provides as mixed or sole N source) constrained, in a first step stomatal conductance with the consequent CO2 diffusion limitation into the chloroplast, and in a second step net photosynthesis of plants grown at ambient CO2.
The depletion of the Fv/Fm index indicates that photo-inhibition occurred due to ammonium exposure (Figure 1). Indeed, stress symptoms caused by an intracellular redox imbalance within the mitochondrial electron transport chain have been described when plants are grown under ammonium nutrition (Jauregui et al., 2017; Liu and Von Wirén, 2017). Thus, our results might indicate that ammonium accumulation in leaves could have contributed to the appearance of reactive oxygen species (ROS), causing a depletion in the Fv/Fm. Moreover, our study supports the fact that the inhibition of a major electron sink (i.e., photosynthetic CO2 assimilation) would be involved in damage to PSII elicits an imbalance in the electron source to CO2 assimilation with the consequent photo-inhibition. In agreement with this finding, the enhanced proline content in ammonium-fed leaves (Figure 3) could have played a role in the response to the stress symptoms in the cell. Proline content is widely reported to increase under certain abiotic stresses, such as temperature or osmotic stress to prevent oxidative damage (Szabados and Savouré, 2009). Proline is a glutamate-derived amino acid; however, under certain stress conditions, maltose, which is a transitory product derived from starch degradation, can be connected with proline biosynthesis (Zanella et al., 2016). In this way, the high maltose content observed when wheat is fertilized with ammonium (Figure 3) could support the biosynthesis of proline (Zanella et al., 2016; Baslam et al., 2017) targeted toward neutralizing the stress symptoms elicited by ammonium accumulation.
Transpiration is a key process for the transport and movement of water and other compounds, either essential nutrients or xenobiotics, throughout the plant body. This process is greatly affected by ammonium nutrition in wheat plants. In parallel with other compounds that impair photosynthetic machinery via stomatal regulation (Aranjuelo et al., 2014) or to the “avoidance mechanism” under saline stress (Wang et al., 2017), ammonium-fertilized plants are able to regulate their stomatal function and thus prevent further ammonium accumulation in leaves via the transpiratory stream, which otherwise could be extremely harmful to cell functions. The counterbalance for the plant is that stomatal closure restricts the entrance of CO2, with negative consequences for photosynthesis and biomass productivity. Although ammonium nitrate also reduced stomatal conductance and ETR, the presence of half of the nitrogen in the form of nitrate might have reduced the ammonium toxicity under ambient CO2 conditions.
The enhancement of ammonium tolerance has been reported under a range of conditions such as high irradiance (Setién et al., 2013), high external pH in the growth medium (Sarasketa et al., 2016), increasing atmospheric CO2 (Rubio-Asensio et al., 2015; Vega-Mas et al., 2017), or fertilizing with a mixed ammonium nitrate nutrition (Zaghdoud et al., 2016). In our study, we have not observed any positive effect of elevated CO2 on biomass production under ammonium nutrition alone, unlike the observations under sole nitrate or mixed nutrition. Accordingly, the effect of elevated CO2 on the parameters related to photosynthetic performance and stomatal functioning was quite different, depending on the N source. Under elevated CO2, stomatal closure took place when nitrate was the N source, but contrary to this, the strong effect of ammonium on stomatal conductance observed under ambient conditions was offset during the exposure to an enriched CO2 atmosphere. Thus, wheat plants grown at elevated CO2, presented similar values of stomatal conductance and WUEi, regardless the N source (Figure 1).
The greater availability of carbon prevented photo-inhibition in the ammonium-stressed plants, which retained normal values of Fv/Fm (Figure 1). Therefore, the exposure to elevated CO2 lessened the stress observed in ammonium-fertilized plants, as indicated by the higher electron transport due to stimulation of CO2 assimilation. Further, these conditions meant that the proline content was lower (Figure 4). The compensatory CO2 effect on these physiological processes under ammonium nutrition matches the absence of significant differences in the expression of PIP1.1, PIP2.3, and N transporters (Figure 2). This indicates that in the absence of a metabolic stress context, ammonium-fertilized plants can maintain their stomatal conductance, and this removes the necessity to adjust cell physiology toward the diffusion of CO2 and H2O through the cell membranes.
The stimulation of net photosynthetic assimilation under elevated CO2 would allow more carbon skeletons to be diverted to the Krebs cycle (Setién et al., 2013) to maintain ammonium assimilation. The commonly described incorporation of ammonium into amino acids via GS and GOGAT activities, which are enhanced under elevated CO2, would improve glutamine and glutamate supplies, respectively. In addition, an alternative pathway proposed to reduce ammonium excess is GDH amination, which catalyzes the conversion of α-ketoglutarate into glutamate (Setién et al., 2013; Vega-Mas et al., 2015). The disappearance of stress observed at elevated CO2 conditions is concordant with a greater capacity of the plant to assimilate ammonium, improving its assimilation rates and leading to C and N being accumulated as proteins rather than carbohydrates or free amino acids (Figures 4, 5).
On the other hand, elevated CO2 favored behavior in ammonium nitrate-grown plants that resemble ammonium-fertilized plants in terms of stomatal physiology, without any changes in WUE or AQPs and N-transporter gene expression (Figures 1, 2). Indeed, the recovery of photosynthesis was even better than under the exclusive ammonium source, which contributed toward the biomass increasing under elevated CO2 conditions (Table 2). Our results are in line with many reports of maximal growth rates in plants being achieved with mixed nutrition (Piñero et al., 2018; Shang and Shen, 2018).
Under different types of abiotic stress or nutrient stress, the root system behaves not only as an organ that captures resources for the plant (Ye et al., 2018), but it also serves as a storage organ or barrier that protects the aerial parts from toxic elements (Setién et al., 2014; Rios et al., 2017; Vega-Mas et al., 2017). Preferential investment of resources and carbon allocation in the root system as the first organ to encounter ammonium nutrition could explain why wheat shoot physiology and metabolism recover partially from stress under high CO2 availability, but that ultimately, this better performance is not translated into shoot growth.
The present work underscores the impact of the N source and elevated CO2 on stomatal opening and plant physiology. Under ambient CO2 conditions, ammonium nutrition leads to toxic effects with stress symptoms and reductions in stomatal conductance and photosynthetic performance, with a consequent impact on plant biomass. Within this context, stomatal closure is interpreted as a strategy to diminish ammonium transport toward leaves due to a reduction in leaf transpiration (as also reflected in the lower expression of the TIP1 water transporter and the AMT2.1 ammonium transporter). Further, the gene expression analyses also highlighted that in ammonium fertilized plants, CO2 diffusion toward the chloroplasts might have been favored by overexpression of PIP1.1 gene in order to overcome the impact of Ci caused by a depletion of stomatal conductance. On the other hand, the study showed that under elevated CO2 conditions, in which photo-inhibition symptoms disappeared, physiological parameters such as stomatal conductance together with the expression of water, ammonium, and CO2 transporters (TIP1, AMT2.1 and PIP1.1, respectively) were maintained at control values. In addition, our study also revealed that in the context of rising CO2, ammonium-based fertilization might favor the photosynthetic performance toward greater redistribution of carbon assimilated via the Krebs cycle to maintain ammonium assimilation. Finally, our study also showed that the mixed ammonium nitrate nutrition could improve water use efficiency and plant productivity.
FT, IA, and CG-M designed the experiments and developed the structure. FT grew the plants, undertook the measurements, harvested the plants, and analyzed the data. EB-F analyzed the sugar content and starch synthesis and degradation activities. IA, MG-M, and CG-M supervised the experiments. FT, IA, and MG-M structured the paper, made critical revisions, and had primary responsibility for the final content. CG-M provided valuable discussion and finalized writing of the manuscript. All authors have read and approved the final manuscript.
FT held a PhD grant from the Ministry of Economy and Competitiveness of the Spanish Government. This work was funded by the Spanish Government MINECO/FEDER-UE (AGL2016-79868-R, AGL2015-64582-02-R, and AGL2013-41363-R) and by the Basque Government (IT-932-16).
The authors declare that the research was conducted in the absence of any commercial or financial relationships that could be construed as a potential conflict of interest.
Technical support provided by the Phytotron Service, SGIker (UPV/EHU), is gratefully acknowledged. We acknowledge support of the publication fee by the CSIC Open Access Publication Support Initiative through its Unit of Information Resources for Research (URICI).
Andrews, M., Raven, J. A., and Lea, P. J. (2013). Do plants need nitrate? The mechanisms by which nitrogen form affects plants. Ann. Appl. Biol. 163, 174–199. doi: 10.1111/aab.12045
Aranjuelo, I., Doustaly, F., Cela, J., Porcel, R., Müller, M., Aroca, R., et al. (2014). Glutathione and transpiration as key factors conditioning oxidative stress in Arabidopsis thaliana exposed to uranium. Planta 239, 817–830. doi: 10.1007/s00425-013-2014-x
Aranjuelo, I., Irigoyen, J. J., Perez, P., Martínez-Carrasco, R., and Sánchez-Díaz, M. (2006). Response of nodulated alfalfa to water supply, temperature and elevated CO2: productivity and water relations. Environ. Exp. Bot. 55, 130–141. doi: 10.1016/j.envexpbot.2004.10.007
Ariz, I., Artola, E., Asensio, A. C., Cruchaga, S., Aparicio-Tejo, P. M., and Moran, J. F. (2011). High irradiance increases NH4+ tolerance in Pisum sativum: higher carbon and energy availability improve ion balance but not N assimilation. J. Plant Physiol. 168, 1009–1015. doi: 10.1016/j.jplph.2010.11.022
Arnon, D. I., and Hoagland, D. R. (1940). A comparison of water culture and soil as media for crop production. Science 89, 512–514.
Baki, G. K. A., Siefritz, F., Man, H., Weiner, H., Kaldenhoff, R., and Kaiser, W. M. (2000). Nitrate reductase in Zea mays L. under salinity. Plant Cell Environ. 23, 515–521. doi: 10.1046/j.1365-3040.2000.00568.x
Baslam, M., Baroja-Fernández, E., Ricarte-Bermejo, A., Sánchez-López, A. M., Aranjuelo, I., Bahaji, A., et al. (2017). Genetic and isotope ratio mass spectrometric evidence for the occurrence of starch degradation and cycling in illuminated Arabidopsis leaves. PLoS One 12:e0171245. doi: 10.1371/journal.pone.0171245
Bastías, E., Alcaraz-López, C., Bonilla, I., Martínez-Ballesta, M. C., Bolaños, L., and Carvajal, M. (2010). Interactions between salinity and boron toxicity in tomato plants involve apoplastic calcium. J. Plant Physiol. 167, 54–60. doi: 10.1016/j.jplph.2009.07.014
Benckiser, G., Schartel, T., and Weiske, A. (2015). Control of NO3− and N2O emissions in agroecosystems: a review. Agron. Sustain. Dev. 35, 1059–1074. doi: 10.1007/s13593-015-0296-z
Bernacchi, C. J., Portis, A. R., Nakano, H., Von Caemmerer, S., and Long, S. P. (2002). Temperature response of mesophyll conductance. Plant Physiol. 130, 1992–1998. doi: 10.1104/pp.008250
Bertl, A., and Kaldenhoff, R. (2007). Function of a separate NH3-pore in Aquaporin TIP2;2 from wheat. FEBS Lett. 581, 5413–5417. doi: 10.1016/j.febslet.2007.10.034
Bradford, M. M. (1976). A rapid and sensitive method for the quantitation of microgram quantities of protein utilizing the principle of protein-dye binding. Anal. Chem. 72, 248–254.
Britto, D. T., and Kronzucker, H. J. (2002). NH4+ toxicity in higher plants: a critical review. J. Plant Physiol. 159, 567–584. doi: 10.1078/0176-1617-0774
Cataldo, D. A., Schrader, L. E., and Youngs, V. L. (1974). Analysis by digestion and colorimetric assay of total nitrogen in plant tissues high in nitrate. Crop Sci. 14, 854–856. doi: 10.2135/cropsci1974.0011183X001400060024x
Cohen, S. A., and De Antonis, K. M. (1994). Applications of amino acid derivatization with 6-aminoquinolyl-N-hydroxysuccinimidyl carbamate. Analysis of feed grains, intravenous solutions and glycoproteins. J. Chromatogr. A 661, 25–34. doi: 10.1016/0021-9673(93)E0821-B
Cohen, S. A., and Michaud, D. P. (1993). Synthesis of a fluorescent derivatizing reagent, 6-aminoquinolyl-N-hydroxysuccinimidyl carbamate, and its application for the analysis of hydrolysate amino acids via high-performance liquid chromatography. Anal. Biochem. 211, 279–287. doi: 10.1006/abio.1993.1270
Ding, L., Gao, L., Liu, W., Wang, M., Gu, M., Ren, B., et al. (2016). Aquaporin plays an important role in mediating chloroplastic CO2 concentration under high-N supply in rice (Oryza sativa) plants. Physiol. Plant. 156, 215–226. doi: 10.1111/ppl.12387
Ding, L., Lu, Z., Gao, L., Guo, S., and Shen, Q. (2018). Is nitrogen a key determinant of water transport and photosynthesis in higher plants upon drought stress? Front. Plant Sci. 9, 1–12. doi: 10.3389/fpls.2018.01143
Durbak, A. R., Phillips, K. A., Pike, S., O’Neill, M. A., Mares, J., Gallavotti, A., et al. (2014). Transport of boron by the tassel-less1 aquaporin is critical for vegetative and reproductive development in maize. Plant Cell 26, 2978–2995. doi: 10.1105/tpc.114.125898
Esteban, R., Ariz, I., Cruz, C., and Moran, J. F. (2016). Review: mechanisms of ammonium toxicity and the quest for tolerance. Plant Sci. 248, 92–101. doi: 10.1016/j.plantsci.2016.04.008
Flexas, J., Diaz-Espejo, A., Galmés, J., Kaldenhoff, R., Medrano, H., and Ribas-Carbo, M. (2007). Rapid variations of mesophyll conductance in response to changes in CO2 concentration around leaves. Plant Cell Environ. 30, 1284–1298. doi: 10.1111/j.1365-3040.2007.01700.x
Flexas, J., Ribas-Carbo, M., Hanson, D. T., Bota, J., Otto, B., Cifre, J., et al. (2006). Tobacco aquaporin NtAQP1 is involved in mesophyll conductance to CO2 in vivo. Plant J. 48, 427–439. doi: 10.1111/j.1365-313X.2006.02879.x
Forrest, K. L., and Bhave, M. (2007). Major intrinsic proteins (MIPs) in plants: a complex gene family with major impacts on plant phenotype. Funct. Integr. Genomics 7, 263–289. doi: 10.1007/s10142-007-0049-4
Forrest, K. L., and Bhave, M. (2008). The PIP and TIP aquaporins in wheat form a large and diverse family with unique gene structures and functionally important features. Funct. Integr. Genomics 8, 115–133. doi: 10.1007/s10142-007-0065-4
Fuertes-Mendizábal, T., Setién, I., Estavillo, J. M., and González-Moro, M. B. (2010). Late nitrogen fertilization affects carbohydrate mobilization in wheat. J. Plant Nutr. Soil Sci. 173, 907–919. doi: 10.1002/jpln.200900262
Galmés, J., Medrano, H., and Flexas, J. (2007). Photosynthetic limitations in response to water stress and recovery in Mediterranean plants with different growth forms. New Phytol. 175, 81–93. doi: 10.1111/j.1469-8137.2007.02087.x
Gibon, Y., Blaesing, O. E., Hannemann, J., Carillo, P., Höhne, M., Hendriks, J. H. M., et al. (2004). A robot-based platform to measure multiple enzyme activities in arabidopsis using a set of cycling assays: comparison of changes of enzyme activities and transcript levels during diurnal cycles and in prolonged darkness. Plant Cell 16, 3304–3325. doi: 10.1105/tpc.104.025973
Giménez, M. J., Pistón, F., and Atienza, S. G. (2011). Identification of suitable reference genes for normalization of qPCR data in comparative transcriptomics analyses in the Triticeae. Planta 233, 163–173. doi: 10.1007/s00425-010-1290-y
Guo, S., Zhou, Y., Shen, Q., and Zhang, F. (2007). Effect of ammonium and nitrate nutrition on some physiological processes in higher plants–growth, photosynthesis, photorespiration, and water relations. Plant Biol. 9, 21–29. doi: 10.1055/s-2006-924541
Henzler, T., Ye, Q., and Steudle, E. (2004). Oxidative gating of water channels (aquaporins) in Chara by hydroxyl radicals. Plant Cell Environ 27, 1184–1195. doi: 10.1111/j.1365-3040.2004.01226.x
Jauregui, I., Aparicio-Tejo, P. M., Baroja-Fernández, E., Avila, C., and Aranjuelo, I. (2017). Elevated CO2 improved the growth of a double nitrate reductase defective mutant of Arabidopsis thaliana: the importance of maintaining a high energy status. Environ. Exp. Bot. 140, 110–119. doi: 10.1016/j.envexpbot.2017.06.003
Jauregui, I., Aparicio-Tejo, P. M., Avila, C., and Rueda-lópez, M. (2015). Root and shoot performance of Arabidopsis thaliana exposed to elevated CO2: A physiologic, metabolic and transcriptomic response. J. Plant Physiol. 189, 65–76. doi: 10.1016/j.jplph.2015.09.012
Liu, Y., and Von Wirén, N. (2017). Ammonium as a signal for physiological and morphological responses in plants. J. Exp. Bot. 68, 2581–2592. doi: 10.1093/jxb/erx086
Long, S. P., Ainsworth, E. A., Rogers, A., and Ort, D. R. (2004). Rising atmospheric carbon dioxide: plants face the future. Annu. Rev. Plant Biol. 55, 591–628. doi: 10.1146/annurev.arplant.55.031903.141610
López-Millán, A. F., Sagardoy, R., Solanas, M., Abadía, A., and Abadía, J. (2009). Cadmium toxicity in tomato (Lycopersicon esculentum) plants grown in hydroponics. Environ. Exp. Bot. 65, 376–385. doi: 10.1016/j.envexpbot.2008.11.010
Ma, J. F., and Yamaji, N. (2006). Silicon uptake and accumulation in higher plants. Trends Plant Sci. 11, 392–397. doi: 10.1016/j.tplants.2006.06.007
Maurel, C. (1997). Aquaporins and water permeability of plant membranes. Annu. Rev. Plant Physiol. Plant Mol. Biol. 48, 399–429. doi: 10.1146/annurev.arplant.48.1.399
Maurel, C., Verdoucq, L., Luu, D., and Santoni, V. (2008). Plant Aquaporins: membrane channels with multiple integrated functions. Annu. Rev. Plant Biol. 59, 595–624. doi: 10.1146/annurev.arplant.59.032607.092734
Nimesha, F., Naoki, N., Panozzo, J., Tausz, M., Norton, R. M., and Seneweera, S. (2017). Lower grain nitrogen content of wheat at elevated CO2 can be improved through post-anthesis NH4+ supplement. J. Cereal Sci. 74, 79–85. doi: 10.1016/j.jcs.2017.01.009
Patton, C. J., and Crouch, S. R. (1977). Spectrophotometric and kinetics investigation of the Berthelot reaction for the determination of ammonia. Anal. Chem. 49, 464–469.
Pawłowicz, I., and Masajada, K. (2019). Aquaporins as a link between water relations and photosynthetic pathway in abiotic stress tolerance in plants. Gene 687, 166–172. doi: 10.1016/j.gene.2018.11.031
Piñero, M. C., Pérez-Jiménez, M., López-Marín, J., Varó, P., and Del Amor, F. M. (2018). Differential effect of the nitrogen form on the leaf gas exchange, amino acid composition, and antioxidant response of sweet pepper at elevated CO2. Plant Growth Regul. 86, 37–48. doi: 10.1007/s10725-018-0409-1
Rios, J. J., Martínez-Ballesta, M. C., Ruiz, J. M., Blasco, B., and Carvajal, M. (2017). Silicon-mediated improvement in plant salinity tolerance: the role of aquaporins. Front. Plant Sci. 8, 1–10. doi: 10.3389/fpls.2017.00948
Rubio-Asensio, J. S., and Bloom, A. J. (2017). Inorganic nitrogen form: a major player in wheat and Arabidopsis responses to elevated CO2. J. Exp. Bot. 68, 2611–2625. doi: 10.1093/jxb/erw465
Rubio-Asensio, J. S., Rachmilevitch, S., and Bloom, A. J. (2015). Responses of Arabidopsis and wheat to rising CO2 depend on nitrogen source and nighttime CO2 levels. Plant Physiol. 168, 156–163. doi: 10.1104/pp.15.00110
Rucińska-Sobkowiak, R. (2016). Water relations in plants subjected to heavy metal stresses. Acta Physiol. Plant. 38. doi: 10.1007/s11738-016-2277-5
Sarasketa, A., González-Moro, M. B., González-Murua, C., and Marino, D. (2016). Nitrogen source and external medium pH interaction differentially affects root and shoot metabolism in Arabidopsis. Front. Plant Sci. 7, 1–12. doi: 10.3389/fpls.2016.00029
Setién, I., Fuertes-Mendizábal, T., González, A., Aparicio-Tejo, P. M., González-Murua, C., González-Moro, M. B., et al. (2013). High irradiance improves ammonium tolerance in wheat plants by increasing N assimilation. J. Plant Physiol. 170, 758–771. doi: 10.1016/j.jplph.2012.12.015
Setién, I., Vega-Mas, I., Celestino, N., Calleja-Cervantes, M. E., González-Murua, C., Estavillo, J. M., et al. (2014). Root phosphoenolpyruvate carboxylase and NAD-malic enzymes activity increase the ammonium-assimilating capacity in tomato. J. Plant Physiol. 171, 49–63. doi: 10.1016/j.jplph.2013.10.021
Shang, H. Q., and Shen, G. M. (2018). Effect of ammonium/nitrate ratio on pak choi (Brassica chinensis L.) photosynthetic capacity and biomass accumulation under low light intensity and water deficit. Photosynthetica 56, 1039–1046. doi: 10.1007/s11099-018-0815-7
Sweetlove, L. J., Burrell, M. M., and ap Rees, T. (1996). Starch metabolism in tubers of transgenic potato (Solanum tuberosum) with increased ADPglucose pyrophosphorylase. Biochem. J. 320, 493–498. doi: 10.1042/bj3200493
Szabados, L., and Savouré, A. (2009). Proline: a multifunctional amino acid. Trends Plant Sci. 15, 89–97. doi: 10.1016/j.tplants.2009.11.009
Tegeder, M., and Masclaux-Daubresse, C. (2017). Source and sink mechanisms of nitrogen transport and use. New Phytol. 217, 35–53. doi: 10.1111/nph.14876
Vega-Mas, I., Marino, D., Sánchez-Zabala, J., González-Murua, C., Estavillo, J. M., and González-Moro, M. B. (2015). CO2 enrichment modulates ammonium nutrition in tomato adjusting carbon and nitrogen metabolism to stomatal conductance. Plant Sci. 241, 32–44. doi: 10.1016/j.plantsci.2015.09.021
Vega-Mas, I., Pérez-Delgado, C. M., Marino, D., Fuertes-Mendizábal, T., González-Murua, C., Márquez, A. J., et al. (2017). Elevated CO2 induces root defensive mechanisms in tomato plants when dealing with ammonium toxicity. Plant Cell Physiol. 58, 2112–2125. doi: 10.1093/pcp/pcx146
Vicente, R., Pérez, P., Martínez-Carrasco, R., Usadel, B., Kostadinova, S., and Morcuende, R. (2015). Quantitative RT-PCR platform to measure transcript levels of C and N metabolism-related genes in durum wheat: transcript profiles in elevated [CO2] and high temperature at different levels of N supply. Plant Cell Physiol. 56, 1556–1573. doi: 10.1093/pcp/pcv079
Wang, X., Wang, W., Huang, J., Peng, S., and Xiong, D. (2017). Diffusional conductance to CO2 is the key limitation to photosynthesis in salt-stressed leaves of rice (Oryza sativa). Physiol. Plant. 163, 45–58. doi: 10.1111/ppl.12653
Xu, Z., Jiang, Y., Jia, B., and Zhou, G. (2016). Elevated-CO2 response of stomata and its dependence on environmental factors. Front. Plant Sci. 7, 1–15. doi: 10.3389/fpls.2016.00657
Ye, H., Roorkiwal, M., Valliyodan, B., Zhou, L., Chen, P., Varshney, R., et al. (2018). Genetic diversity of root system architecture in response to drought stress in grain legumes Heng. J. Exp. Bot. 69, 3267–3277. doi: 10.1093/jxb/ery082
Zaghdoud, C., Carvajal, M., Ferchichi, A., and del Carmen Martínez-Ballesta, M. (2016). Water balance and N-metabolism in broccoli (Brassica oleracea L. var. Italica) plants depending on nitrogen source under salt stress and elevated CO2. Sci. Total Environ. 571, 763–771. doi: 10.1016/j.scitotenv.2016.07.048
Keywords: ammonium, nitrate, wheat, elevated CO2, N assimilation, stomata conductance, aquaporins
Citation: Torralbo F, González-Moro MB, Baroja-Fernández E, Aranjuelo I and González-Murua C (2019) Differential Regulation of Stomatal Conductance as a Strategy to Cope With Ammonium Fertilizer Under Ambient Versus Elevated CO2. Front. Plant Sci. 10:597. doi: 10.3389/fpls.2019.00597
Received: 25 January 2019; Accepted: 24 April 2019;
Published: 24 May 2019.
Edited by:
Xiangnan Li, Northeast Institute of Geography and Agroecology (CAS), ChinaReviewed by:
Charles L. Guy, University of Florida, United StatesCopyright © 2019 Torralbo, González-Moro, Baroja-Fernández, Aranjuelo and González-Murua. This is an open-access article distributed under the terms of the Creative Commons Attribution License (CC BY). The use, distribution or reproduction in other forums is permitted, provided the original author(s) and the copyright owner(s) are credited and that the original publication in this journal is cited, in accordance with accepted academic practice. No use, distribution or reproduction is permitted which does not comply with these terms.
*Correspondence: Fernando Torralbo, ZmVybmFuZG8udG9ycmFsYm9AZWh1LmV1cw==; Iker Aranjuelo, aWtlci5hcmFuanVlbG9AY3NpYy5lcw==
†Present address: Fernando Torralbo, Division of Plant Sciences, University of Missouri, Columbia, MO, United States
Disclaimer: All claims expressed in this article are solely those of the authors and do not necessarily represent those of their affiliated organizations, or those of the publisher, the editors and the reviewers. Any product that may be evaluated in this article or claim that may be made by its manufacturer is not guaranteed or endorsed by the publisher.
Research integrity at Frontiers
Learn more about the work of our research integrity team to safeguard the quality of each article we publish.